- Alltech European Bioscience Centre, Sarney, Dunboyne, Co. Meath, Ireland
Unsustainable antimicrobial use in industrial agriculture has contributed to the rise in antimicrobial resistance and there is an urgent need to find alternative and more sustainable strategies to traditional antimicrobials. Prebiotics, such as mannan-rich fraction (MRF), a cell wall product from Saccharomyces cerevisiae, have demonstrated an ability to alter the growth of antibiotic susceptible and resistant Escherichia coli and improve the efficacy of antibiotics through modulation of cellular activity. In this study the impact of mannan based prebiotics on growth and respiration of E. coli was assessed by observing microbial growth, oxygen consumption rate and extracellular acidification rate in the presence and absence of tetracycline. The findings further demonstrate the capabilities of MRF with respect to improving microbial antibiotic sensitivity, particularly in resistant strains. This potentially enables a more efficient control of resistant pathogens with food safety implications and promotion of more sustainable use of antibiotics in animal production systems.
Introduction
Since their discovery over 60 years ago, antibiotics have transformed healthcare, however our reliance on antibiotics has become unsustainable (Merrett et al., 2016), and their overuse has led to the development of resistance in many once easily treated bacterial strains. Antibiotic resistance has become one of the most prevalent health concerns of our time.
While human misuse and over-prescription has been a factor in the rise of resistance, agricultural practises have long been reported to contribute greatly to this rise in AMR (Dewey et al., 1996). The European Centre for Disease Control (ECDC) and European Food Safety Authority (EFSA) joint 2018/2019 EU summary report on antimicrobial resistance in zoonotic and indicator bacteria from humans, animals and food indicated that of the Salmonella spp. recovered from broiler carcasses, resistance to fluroquinolone antimicrobial agents was extremely high, with reported levels of 51.4% and 48.8% resistance to ciprofloxacin and nalidixic acid respectively. Most alarmingly, high levels of resistance to tetracycline in Salmonella spp. were reported in isolates taken from humans (European Food Safety Authority and European Centre for Disease Prevention and Control, 2021). More recently, a Canadian broiler study (Romero-Barrios et al., 2020) reported that 82.8% of E. coli isolates and 58.8% of Salmonella isolates tested demonstrated resistance to at least one antimicrobial. Of these isolates tested, 46.1% of E. coli and 42.9% Salmonella were resistant to tetracycline.
The problem also extends to the pig production industry. A study to examine antibiotic resistance in Danish pigs found 73.3% of E. coli isolates to be resistant to tetracycline (Holmer et al., 2019). In China, an investigation of swine farms found 46.3% of E. coli isolates tested were found to harbour the oqxA gene responsible for plasmid-mediated quinolone resistance. In addition, the study reported that 30.3% of human commensal E. coli isolates examined were positive for the resistance gene, despite the individuals never having been treated with antibiotics previously, a worrying indication of resistance transmission from pig to human (Yang et al., 2019). Of the antimicrobials to which resistance has been found in the agricultural industry, many are included on the World Health Organisation (WHO) list of critically important antimicrobials (CIA) for human medicine, with quinolones and tetracyclines considered highest priority and highly important respectively (WHO, 2019). As evidence of microbial resistance transmission between animals and humans grows, a reduction and more sustainable model of antimicrobial use is a priority.
Such is the extent of the problem of unsustainable use of antimicrobials in agriculture and the evident AMR development that it has prompted policy makers around the globe to take action. Antibiotic use for growth promotion has been banned in the EU since 2006, and new stringent regulations have come into force in 2022, such as the requirement for veterinary diagnosis before prescription and earmarking several key antibiotics as human-use only (European Union, 2018). Under these new regulations, meat and food imports destined for human consumption in the EU must also comply, with the aim of reducing antibiotic use in agriculture outside of the scope of EU legislation. The United States (US) has also introduced new rules regarding antibiotic use, implementing their own ban on antibiotics as growth promotors in 2016 (Armbruster and Roberts, 2018). These regulations have meant that the development of more sustainable animal production methods and integration of alternative feed supplements in animal diets have become huge areas of interest.
One such area of interest is in the use of prebiotics in production animal diets. Prebiotics are defined as “a selectively fermented ingredient that results in specific changes in the composition and/or activity of the gastrointestinal microbiota, thus conferring benefit(s) upon host health” (Gibson et al., 2010). The majority of prebiotics are a subset of carbohydrate groups and are mostly oligosaccharide carbohydrates including fructo-oligosaccharides (FOS) (Ricke, 2015), galacto-oligosaccharides (GOS) (Davani-Davari et al., 2019), and mannan oligosaccharides (MOS) (Spring et al., 2015). MOS, with its ability to bind and limit colonisation of gut pathogens, has been applied in antibiotic-free diets, providing support for immunity and digestion (Spring et al., 2015). In the presence of MOS products, type 1 fimbriated pathogens attach to the supplemental dietary mannan compounds instead of the epithelia, thereby reducing their colonisation capability and providing the host with a natural defence against some pathogenic bacteria, of which many are resistant to antimicrobials.
More recently, mannan-rich fraction (MRF), a MOS derived product has been shown to result in improved weight gain, enhanced feed conversion, decreased mortality rates and reduced pathogen loading when added to the diets of piglets (Spring et al., 2015). Similar success has been noted in poultry, with MRF reducing intestinal colonisation by pathogenic bacteria such as Escherichia coli and Salmonella spp. (Biggs et al., 2007), in addition to Campylobacter (Corrigan et al., 2017). As two industries with a high reliance on antimicrobial use, these findings are highly important when attempting to further reduce antibiotic use in pig and poultry production. Additionally, MRF has been found to modulate bacterial cellular respiration, potentially impacting antibiotic efficacy and bacterial metabolism (Smith et al., 2020).
This study aimed to explore the properties of several yeast product preparations (YPP), including MRF, as more sustainable prebiotic alternatives to traditional antibiotic use by examining their impact on bacterial growth and metabolism. Growth curve and subsequent growth rate analysis were examined and interpreted alongside corresponding energy maps generated by the Seahorse XFe96 Analyser. These results were used to determine the metabolic effects of YPP on resistant bacteria and if YPP addition can improve the efficacy of the agriculturally relevant bacteriostatic antibiotic tetracycline when used in a combination treatment regime.
Methods
Strains, media and growth conditions
One Shot® TOP10 chemically competent E. coli (Invitrogen™) was used as the host strain for transformation. Recombinant E. coli was transformed using pBR322 (Invitrogen™) to produce the resistant E. coli, and this was used as the resistant strain throughout. Frozen stocks of these bacteria were prepared and stored at − 70°C in 70% glycerol and LB medium. Both strains of E. coli were prepared by culturing overnight in Luria Broth (LB) (Sigma) at 37°C in a rotating shaker at 180rpm, with media for the resistant strain being supplemented with 100 μg mL-1 of ampicillin and 30 μg mL-1 tetracycline (TET).
Inoculum preparation and storage
Frozen stocks of bacteria [5% (v/v)] in 70% glycerol were stored at − 70°C in LB medium. These were thawed and growth in LB overnight before use. Working plates were prepared by transferring 100µl of diluted overnight glycerol stock inoculum, using spread plate technique, to fresh agar and incubating overnight at 37°C. For the recombinant strain, AMP and TET were added to a final concentration of 100 μg mL−1 and 30 μg mL−1, to both LB and agar. Spread plates were stored at 4°C for up to six months and warmed to 37°C before use. Fresh inoculum was prepared by isolating a single colony from working plates into approximately 25ml of LB broth and grown overnight at 37°C. Optical density (OD) at λ595nm was adjusted accordingly by spectrophotometer (Shimadzu UV-1601PC).
Yeast product preparations
YPPA/Product A, a yeast mannan-rich fraction (MRF) from the cell wall of S. cerevisiae, was provided by Alltech Biotechnology (Alltech Biotechnology, Nicholasville, KY). YPPB/Product B and YPPC/Product C were sourced from independent distributors. Mannose (YYPD/Product D) and Inulin (YPPE/Product E) were sourced from Sigma. For determination of bacterial kinetic growth and respiratory analysis, treatments of mannose, inulin, YPPA, B and C (0.01% w/v and 0.1% w/v) were added to either LB or M9 minimal media (supplemented with 0.2% casamino acids and 10mM glucose). Samples were then sonicated using an HTU Soni 130 ultrasonic processor at a power setting of 130 Watts on ice for 1 min per 500ml. Aliquots were stored at 4°C prior to use or at − 70°C for long term storage. All antibiotics were obtained from Sigma.
Kinetic microplate growth analysis
In this study both susceptible and resistant E. coli were examined to determine if YPP had an impact on growth rate. Growth curves of both susceptible and resistant E. coli were produced by growing the bacteria in media supplemented with 0.01% w/v of the relevant YPP, Mannose, Inulin or glucose control treated in combination with and without increasing concentrations of tetracycline ranging from 0mg/ml to 1mg/ml, with optical density (OD) recorded hourly using a microplate reader. YPP were prepared in LB broth and aliquots of 200μL per well and analysed in triplicate at OD λ595nm in a sterile 96 well flat-bottom microtiter plate. Tetracycline was prepared separately and added per well (10µL). To this, 20µL aliquots of the test organism [adjusted to 0.015 OD at λ595nm (approx. 1 × 10–8 CFU mL−1)] was added to each well. The plate was then incubated under appropriate growth conditions; in this case 37°C. The OD was read every hour following a medium shake for a total of 18 hours. Optical density measurements following 18 hours incubation were taken and compared to a reference control. Replicate growth curve analysis was conducted using a Biotek Synergy HT microplate reader (n=3). Growth curve values were then used to produced growth rate values (n=3).
Statistical analysis
Statistical evaluation of growth curve data was assessed using GrowthRate (GR) software, downloadable from https://sourceforge.net/projects/growthrates/24. GrowthRate reports correlation coefficient, R, of the best fit line to the natural log of the optical density (ln (OD)) over time for each culture. For each set of replicate cultures, GR reports the mean growth rate, the mean R, max OD and lag time. DesignExpert Software (Version 13, Stat-Ease Inc., Minneapolis, MN, USA) was then used to perform one-way analysis of variance (ANOVA) of data. Model variables included product concentration (% w/v), tetracycline (mg/ml) and product type (YPPA, YPPB, YPPC, mannose, inulin). Post-hoc analysis of growth rates was also carried out using Dunnett and Paired t-test (Minitab, LLC, 2021. Minitab, Available at: https://www.minitab.com).
Bacterial respiration
The main parameters required to measure change in cellular energetics are oxygen consumption rate (OCR) and extracellular acidification rate (ECAR). OCR provides information on bacterial oxygen use, while ECAR is a measure of glycolytic flux. Examining the ratio of OCR to ECAR provides an indication of the bioenergetic phenotype of bacteria under the different test conditions of the experiment; different YPP types, different concentrations of tetracycline, susceptible and resistant strains etc. Each combination of factors has a unique basal metabolic activity rate, with subsequent readings aiming to demonstrate the change in metabolic rate over time, resulting in an overview of the bioenergetic profile of the test bacterial strain in response to different treatments. A low ECAR result and low OCR result are representative of a less energetic bacteria, increasing the ECAR while OCR remains low is indicative of bacteria moving to a more glycolytic state. Bacteria producing low ECAR results and high OCR results are considered to be in an aerobic energy state. High ECAR and high OCR readings on an energy map are representative of bacteria in a highly energetic state.
To determine bacterial respiration, overnight cultures of both E. coli strains were diluted to an OD λ595nm of 0.1 in fresh LB, supplemented with antibiotics for the resistant strain, and grown to an OD λ595nm of ~0.3 to reach exponential phase. Cells were then centrifuged at 4000rpm for 5 minutes and washed using fresh M9-minimal medium (M9). This was repeated twice. The pellet was resuspended in fresh M9 and the suspension diluted to 0.015 OD using either M9 or fresh M9 supplemented with 0.01% w/v or 0.1%w/v of either YPPA, YPPB, YPPC, YPPD or YPPE. For OCR and ECAR measurements, 90µl of this bacterial cell with/without treatment suspension was added to the XFe96 Cell Culture Microplates, precoated with 15µl poly-d-lysine (Sigma). These plates were then centrifuged at 1400 rpm for 10 minutes before an additional 90µl of fresh M9 was added to each well. The Seahorse XFe96 Sensor Cartridge, which had been hydrated overnight using Seahorse XF Calibrant solution as per manufactures instructions (Agilent), was prepared. To this, 20µl of antibiotic was added to the relevant port before the plate was added to the Seahorse XFe96 analyser for the equilibration and calibration steps. Once complete, the cell culture plate containing the E. coli cell solutions was also added to the analyser and OCR and ECAR were measured at approximately 6-minute intervals for 3.5 hours. These measurements were used to form energy map diagrams of pmol/min vs mph/min to examine cellular energy state.
Results
Yeast product preparations modulate growth rate of susceptible and resistant E. coli
In this study, tetracycline susceptible and resistant E. coli strains were examined to determine if YPP had an impact on growth rate in the presence and absence of antibiotic. Mannose and inulin were included as monomer and prebiotic controls and all products were examined using Dunnett analysis. Of the five products shown in Table 1, three YPP’s, mannose and inulin, 0.01% YPPA produced significantly different growth rates to the mannose control in resistant E. coli strains when used in combination with 0mg/ml, 0.001mg/ml and 0.1mg/ml tetracycline (Table 1, S5). YPPB was also found to be significantly different to the mannose control, but only in one of the tetracycline combinations tested. Post-hoc analysis using a paired t-test showed that 0.01% YPPA was the only product to demonstrate a significant difference in growth rate when compared to 0% product, with 4 of the 5 antibiotic combinations showing this difference (Table S4). Inulin treated susceptible E. coli were found to produce significantly higher growth rates than that of the mannose control when used in combination with 0.001mg/ml and 0.01mg/ml tetracycline. 0.1mg/ml tetracycline and 0.01% w/v inulin demonstrated a significantly lower mean growth rate to that of the control.
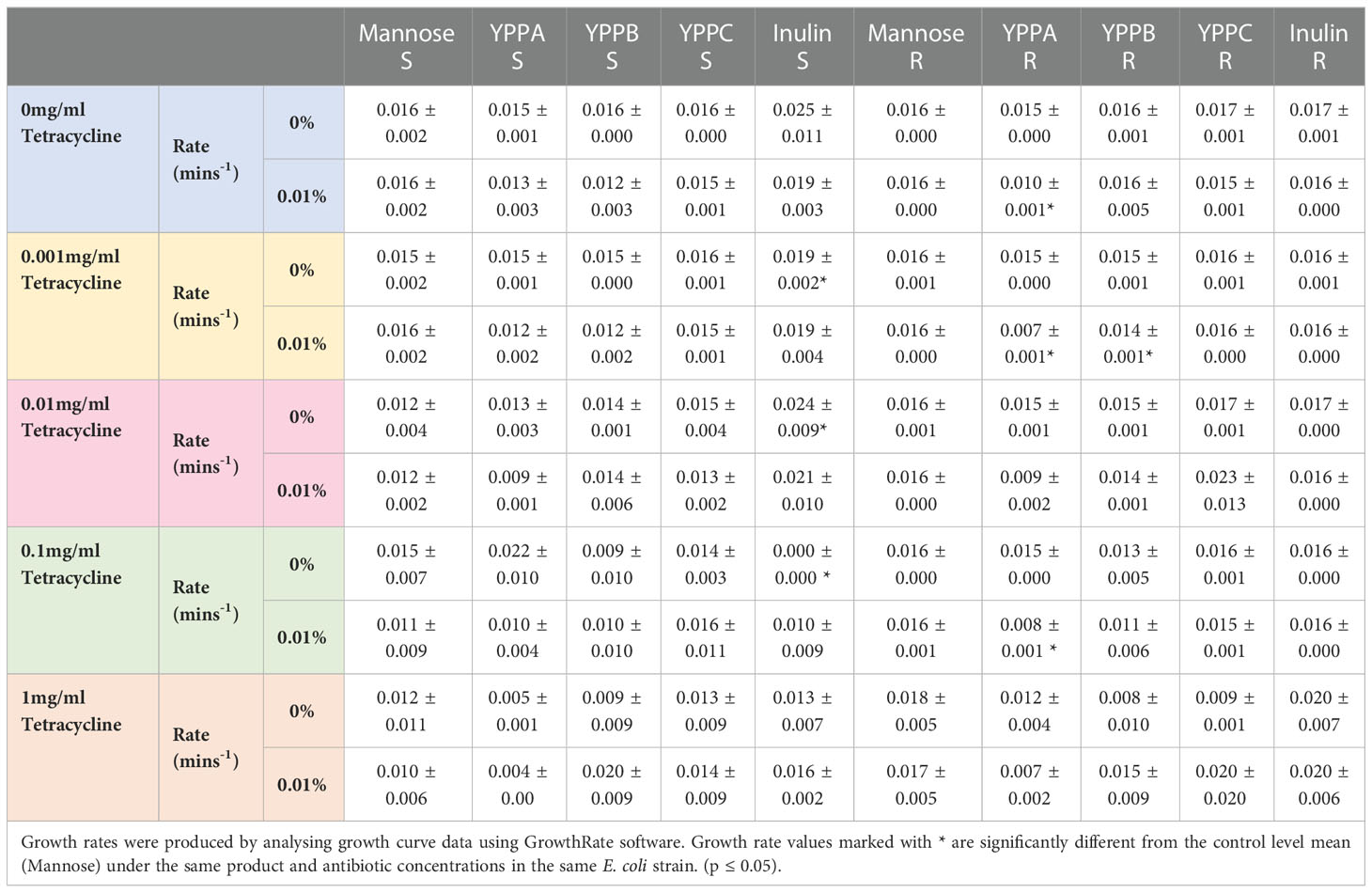
Table 1 Data represents the average growth rate (n=3) for susceptible (S) and resistant (R) E. coli treated with 0mg/ml-1mg/ml tetracycline in combination with either 0% w/v or 0.01% w/v yeast product preparation.
Design Expert analysis of susceptible E. coli indicated that tetracycline and product type were significant model terms (p=0.0005 and p<0.0001 respectively). Analysis of the resistant bacteria indicated that product type (p<0.0001) was the significant factor that impacted growth rate (Tables S3A and S3B). DOE analysis (Tables S1 and S2) of the growth rate data allowed for the development of 3D models (Figure 1) indicating the impact of different combinations of 0.01% w/v YPP and increasing tetracycline concentration on growth rate. Coloured 3D bars represent predicted values for each YPP and control tested. YPPA (red bars) presented the greatest reduction in predicted growth rate for both susceptible and resistant E. coli when compared with the 4 other products tested. YPPA produced the lowest predicted growth rates when used in combination with tetracycline in resistant E. coli (Figure 1). For both susceptible and resistant strains, the observed and predicted growth rate values correlated well.
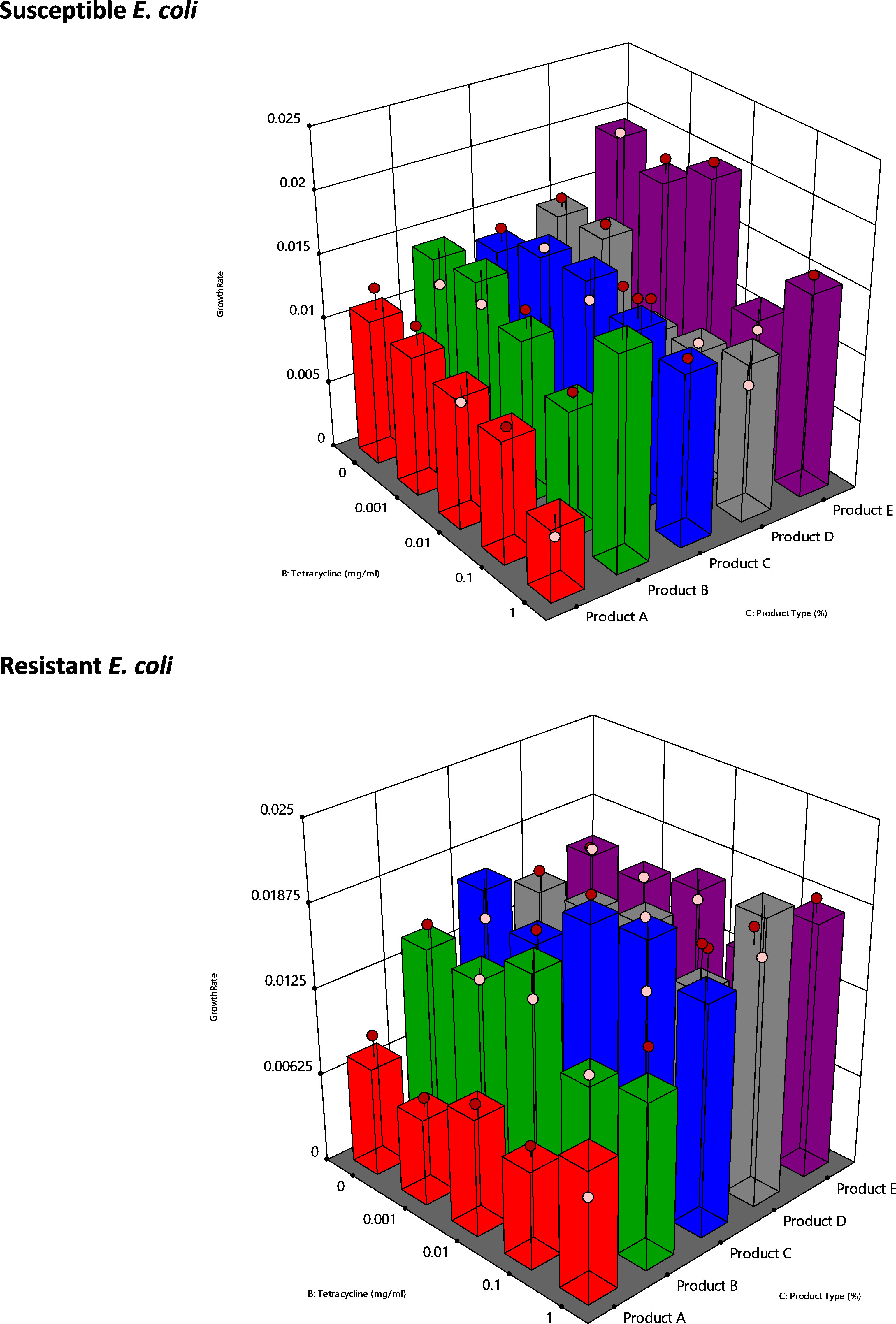
Figure 1 3D Surface graphs of growth rate, product type and antibiotic concentration generated by analysing bacterial growth rate data using DOE assessment. Susceptible (top) and resistant (bottom) E. coli were grown in the presence of 0.01% w/v of product. Tetracycline concentrations tested ranged from 0mg/ml to 1mg/ml. Height of each bar is a predicted value based on the conditions of the experiment. Pink and Red dots represent observed data. Bar colour is representative of product type. For both susceptible and resistant E. coli experiments Product Type was a significant factor, with p-values of p<0.0001 and p<0.0001 respectively. Product A = YPPA, Product B = YPPB, Product C = YPPC. Product D = Mannose, Product E = Inulin.
Yeast product preparations modulate the metabolic state of bacteria
By examining the ratio of OCR to ECAR ratio, an indication of the bioenergetic phenotype of bacteria under the different test conditions of the experiment can be assessed. Test conditions included different YPP types, different concentrations of tetracycline, susceptible and resistant strains. The data collected in this experiment are presented in Figures 2–4, S1, S2 with each figure presenting the results of a different YPP and controls respectively. Table 2A provides numeric values for the previously mentioned Figures.
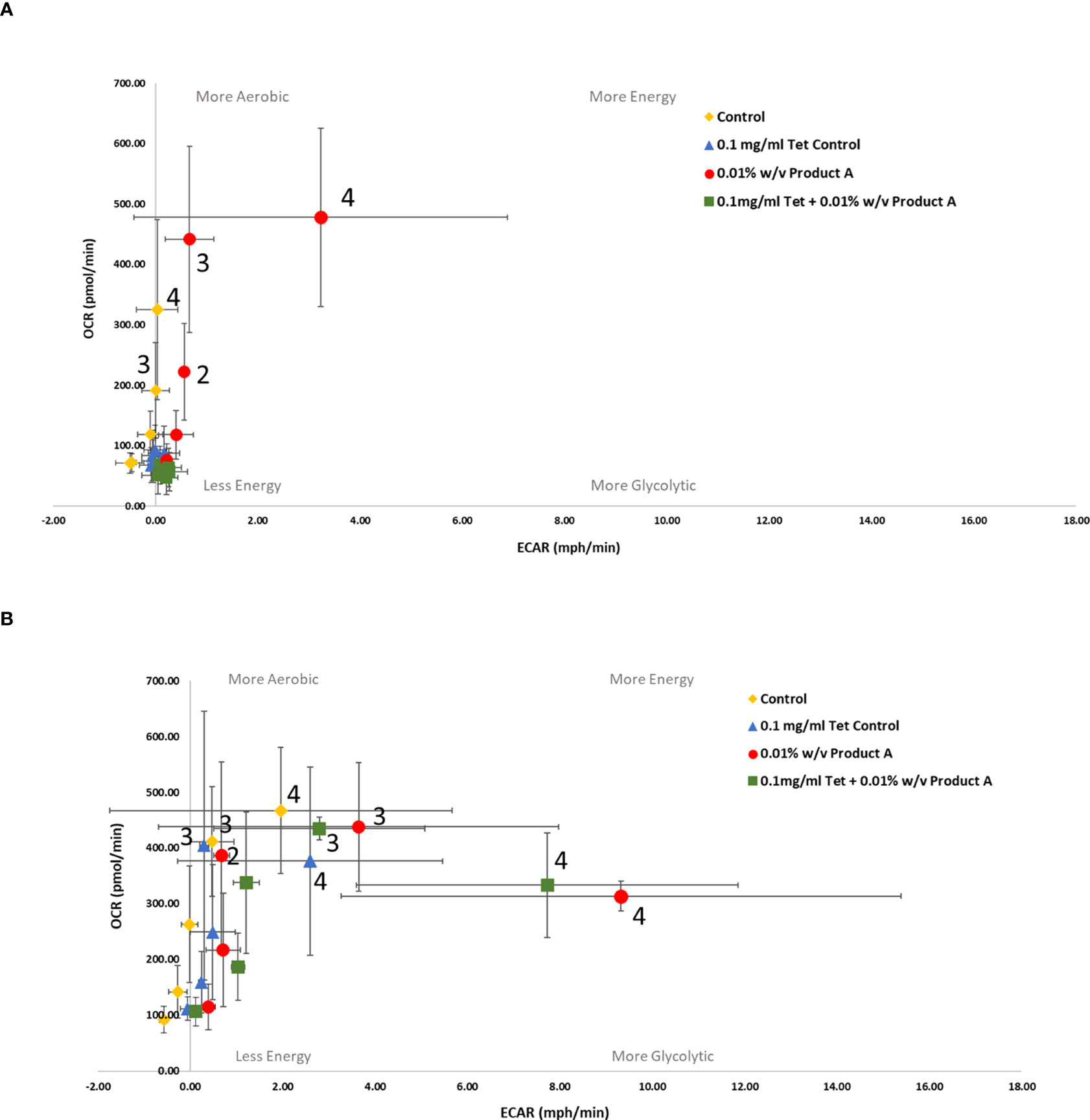
Figure 2 Bioenergetic real time changes in OCR/ECAR ratio of (A) antibiotic susceptible E. coli and (B) antibiotic resistant E. coli when treated with YPPA. Numbered data points represent the following time-points (0) 14 mins (1) 52 mins (2) 98 mins (3) 149 mins (4) 201 mins. Several data points have been labelled with their representative time-points for ease of results visibility. Each value was expressed using mean of triplicates for each biological replicate (n=3), error bars are representative of standard deviation. Control refers to no product/tetracycline supplementation. Product A = YPPA.
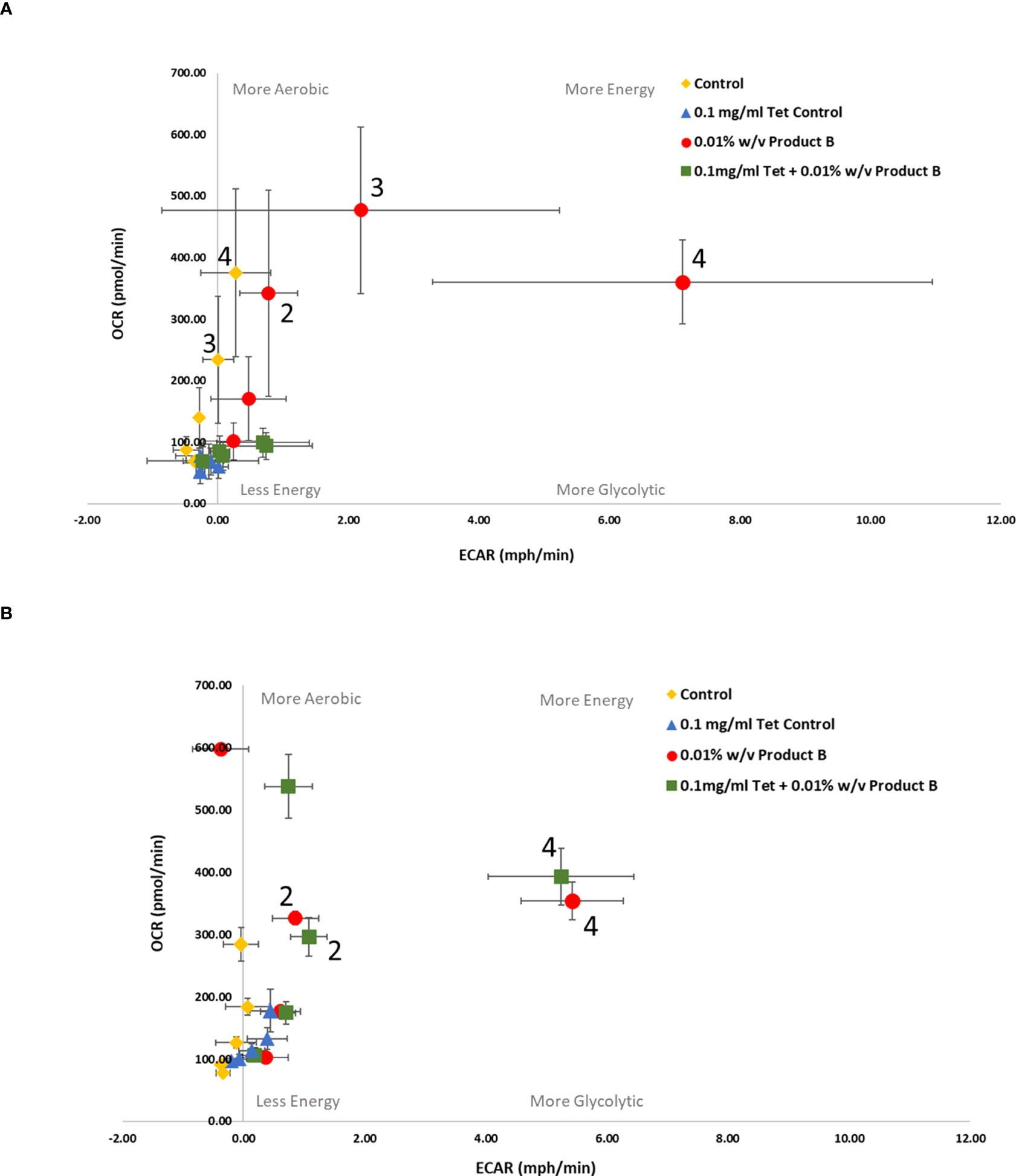
Figure 3 Bioenergetic real time changes in OCR/ECAR ratio of (A) antibiotic susceptible E. coli and (B) antibiotic resistant E. coli when treated with YPPB. Numbered data points represent the following time-points (0) 14 mins (1) 52 mins (2) 98 mins (3) 149 mins (4) 201 mins. Several data points have been labelled with their representative time-points for ease of results visibility. Each value was expressed using mean of triplicates for each biological replicate (n=3), error bars are representative of standard deviation. Control refers to no product/tetracycline supplementation. Product B = YPPB.
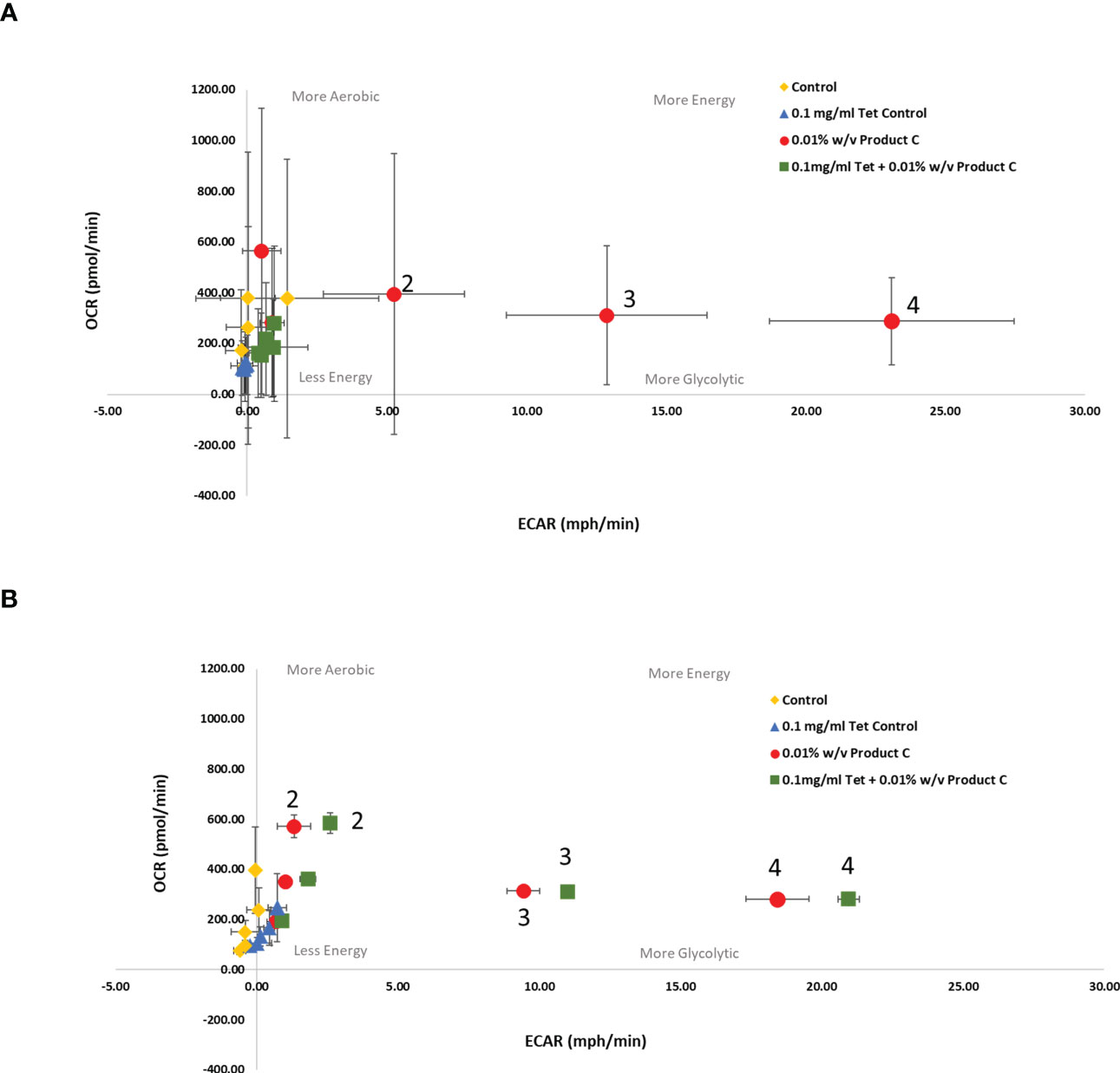
Figure 4 Bioenergetic real time changes in OCR/ECAR ratio of (A) antibiotic susceptible E. coli and (B) antibiotic resistant E. coli when treated with YPPC. Numbered data points represent the following time-points (0) 14 mins (1) 52 mins (2) 98 mins (3) 149 mins (4) 201 mins. Several data points have been labelled with their representative time-points for ease of results visibility. Each value was expressed using mean of triplicates for each biological replicate (n=3), error bars are representative of standard deviation. Control refers to no product/tetracycline supplementation. Product C = YPPC.
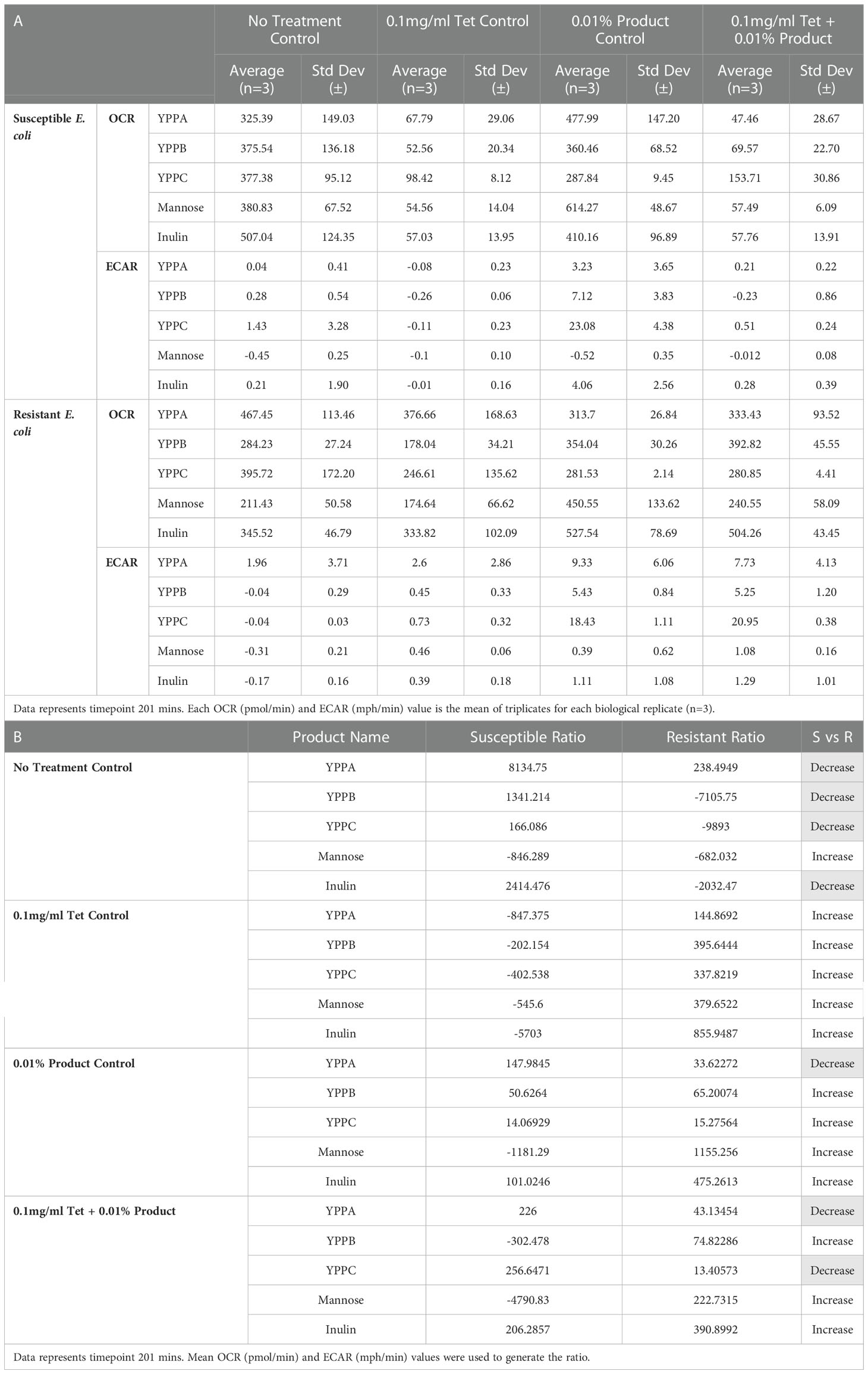
Table 2 (A) OCR and ECAR results for susceptible(S) and resistant (R) E. coli when treated with 0.01% (w/v) YPPA, YPPB, YPPC, mannose or inulin with and without tetracycline (0.1mg/ml).
(B) Ratio of OCR to ECAR for susceptible E. coli (S) versus resistant E. coli (R)
Results showed that susceptible and resistant E. coli untreated with either antibiotic or YPP (control) progress to a more aerobic energy state over time (indicated by yellow markers). There was no change in cellular energy recorded when susceptible cells were treated with 0.1mg/ml tetracycline, which is representative of the relationship between cellular metabolic state and antibiotic susceptibility (Figures 2A, 3A, 4A, S1A, S2A). Resistant cells behave in a similar way whether treated with or without antibiotic, demonstrating how their resistance results in respiration and metabolism being mostly unaltered by the presence of an antibiotic (Figures 2B, 3B, 4B, S1B, S2B).
Supplementation of media with YPP and/or tetracycline produced varying metabolic changes which were preparation specific. YPPA was the only product to produce a lower OCR : ECAR ratio (Table 2B, susceptible ratio = 147.9845, resistant ratio = 33.62272) when comparing the bioenergetic phenotype of susceptible versus resistant E. coli treated with YPP only (Figure 2). There were no treatment differences noted in the OCR : ECAR ratio when both susceptible and resistant strains were treated with 0.1mg/ml tetracycline only.
Control cells untreated with YPPA, YPPB, YPPC and inulin or antibiotic produced a decrease in susceptible versus resistant OCR : ECAR ratio (Table 2B). YPP treatment in combination with 0.1mg/ml tetracycline resulted in susceptible cells remaining in a low energy state across all products tested. Resistant cells treated with the same combination of YPPA and antibiotic became more energised, as did cells treated with YPPA alone, indicative of its metabolic modulating abilities (Figure 2B, 201 mins: approx. 330pmol/min and 8mph/min) when used in combination with the bacteriostatic antibiotic. YPPB (Figure 3B, 201 mins: approx. 393pmol/min and 6mph/min) and YPPC (Figure 4B, 201 mins: approx. 280pmol/min, 20mph/min) supplementation in combination with 0.1mg/ml tetracycline also produced a change in metabolic state from less energetic to more energetic. 0.01% w/v YPPA and YPPC treatment in combination with 0.1mg/ml produced a decreased ratio of OCR : ECAR from susceptible to resistant E. coli (Table 2B, 135% and 180% decrease respectively) In contrast, the mannose (Figure S1B, 201 mins: approx. 240pmol.min, 1mph/min) and inulin (Figure S2B, 201 mins: approx. 504pmol.min, 1mph/min) controls evoked a metabolic state change from less energetic to more aerobic, producing little difference in terms of ECAR results across the different time points.
Discussion
An early study found that bacteria have a constant level of nutrient requirements to maintain growth (Pirt, 1965), and as growth rate slows, a greater proportion of consumed nutrients are required for maintenance, resulting in the observed yield of bacteria decreasing. Other studies have demonstrated the impact of metabolic state on antibiotic efficacy (Smith et al., 2020) and how this efficacy can be linked to bacterial cell respiration. These studies highlight the importance of nutrient impact, such as that of prebiotics, on metabolic state, and in turn on how nutrients can be used to improve antibiotic efficacy by modulating cell respiration.
Antibiotics, such as tetracycline, enact their bacteriostatic abilities by impacting the energy consumption processes of bacteria. This ability to perturb the respiration of cells is an important consequence of antibiotic-cell interactions within the cell (Lobritz et al., 2015). Extracellular addition of metabolites has been found to alter metabolism, potentiating their antibiotic activity against bacteria (Stokes et al., 2019). In addition, this metabolic modulating ability has also been shown to impart the capacity to improve antibiotic efficacy in resistant bacterial cells (Stokes et al., 2019).
In this study, yeast product preparations were shown to impact cell growth and metabolic state, the latter measured by examining the resultant changes in cellular respiration. YPP and antibiotic supplementation demonstrated the greatest capacity for respiration modulation, with 0.01% w/v YPPA supplementation resulting in resistant cells displaying a glycolytic phenotype and having significantly lower growth rates in resistant E. coli when compared to the other YPP tested (Table 1, S5). This ability to alter oxygen consumption from the basal level is indicative of a capacity to change the phenotypic bacteriostat susceptibility by altering cellular respiration (Dwyer et al., 2014). The lower growth rates noted with YPP treatment represents a potential strategy to enhance antibiotic efficacy against resistant E. coli. Of note are the differential results obtained with differing YPP, specifically YPPA which as previously explained effected the lowest growth rate relative to the other YPP treatments, in addition to exerting the ability to lower the OCR : ECAR ratio from susceptible to resistant bacteria both in the presence and absence of tetracycline (Table 2, Figures 2A, 2B). This combined with the ability of such preparations to agglutinate potentially resistant/pathogenic bacteria (Edwards et al., 2014), demonstrates their potential as modulators of bacterial growth and respiration. Results of the 3D surface graphs (Figure 1), and growth rate analysis (Table 1) and energy map analysis (Tables 2A and 2B) suggest that YPPA does not invoke a negative impact on cell viability, but instead lowers growth capacity by altering the respiration abilities of the cell, even without the presence of an antibiotic. Smith et al (2020) hypothesised that glycolytic intermediates which directly impact glycolysis and the pentose-phosphate pathway, may direct carbon metabolism to other pathways in an attempt to restore metabolite balance in stressed cells. This metabolic depletion can appear in stressed E. coli cells as growth inhibition (Richards et al., 2013), correlating to the lower growth rate observed in this study.
Current heavy reliance on antibiotics is unsustainable. High levels of consumption in the agriculture industry has created the perfect breeding ground for the development of resistance, and is quickly becoming an issue of growing magnitude across interlinked species via the food chain and water contamination (Berendonk et al., 2015). Consequently, the demand for alternative strategies to reduce antibiotic dependence is at an all-time high. Prebiotic YPP offer a natural alternative strategy, potentially allowing for antibiotic use reduction, while raising the efficacy of any antibiotic used as a necessity. Further research to fully understand the epidemiology of resistant bacterial strains and metabolic modulation by YPP may offer the opportunity to generate species-specific treatment strategies for enteric antibiotic resistant bacteria using prebiotics. Increasing our knowledge of prebiotic and antibiotic interactions offers the potential to naturally reduce antibiotic use. Antibiotics must be treated as a non-renewable source going forward (Lasserre and Smulders, 2013), and replacements and alternatives used where possible. A cross system approach is needed to change the way in which antibiotics are viewed and used (Lhermie et al., 2019) if we are to make significant progress in achieving sustainable use of antibiotics. The findings of this study highlight the metabolic signatures of bacteria and how they can be altered by prebiotics. This could be crucial in developing a targeted approach to antibiotic resistance and reduction of antibiotic use. By using knowledge of how an antibiotic and prebiotic combination treatment impacts bacterial respiration, the best course of treatment that minimises antibiotic use can be applied. It is hoped that this study warrants further exploration of prebiotics as a more sustainable strategy to combat antibiotic resistance in agriculture.
Data availability statement
The raw data supporting the conclusions of this article will be made available by the authors, without undue reservation.
Author contributions
SG wrote the main manuscript text and was involved with experiments, data analysis and interpretation. RM and HS are conceptually responsible for the research project. SG was responsible for preparation of all figures and tables. All authors contributed to the article and approved the submitted version.
Conflict of interest
Authors are employed by Alltech Biotechnology.
The authors declare that this study received funding from Alltech Biotechnology, which produce mannan prebiotics from S. cerevisiae. The funder was involved in the study design, collection, analysis, interpretation of data, the writing of this article and the decision to submit it for publication.
Publisher’s note
All claims expressed in this article are solely those of the authors and do not necessarily represent those of their affiliated organizations, or those of the publisher, the editors and the reviewers. Any product that may be evaluated in this article, or claim that may be made by its manufacturer, is not guaranteed or endorsed by the publisher.
Supplementary material
The Supplementary Material for this article can be found online at: https://www.frontiersin.org/articles/10.3389/fanim.2022.1069280/full#supplementary-material
References
Armbruster W. J., Roberts T. (2018). The political economy of US antibiotic use in animal feed. Food Saf. Economics. 30, 293–322. doi: 10.1007/978-3-319-92138-9_15
Berendonk T. U., Manaia C. M., Merlin C., Fatta-Kassinos D., Cytryn E., Walsh F., et al. (2015). Tackling antibiotic resistance: The environmental framework. Nat. Rev. Microbiol. 13, 310–317. doi: 10.1038/nrmicro3439
Biggs P., Parsons C. M., Fahey G. C. (2007). The effects of several oligosaccharides on growth performance, nutrient digestibilities, and cecal microbial populations in young chicks. Poultry Sci. 86, 2327–2336. doi: 10.3382/ps.2007-00427
Corrigan A., Fay B. J., Corcionivoschi N., Murphy R. A. (2017). Effect of yeast mannan-rich fractions on reducing campylobacter colonization in broiler chickens. J. Appl. Poultry Res. 26, 350–357. doi: 10.3382/japr/pfx002
Davani-Davari D., Negahdaripour M., Karimzadeh I., Seifan M., Mohkam M., Masoumi S. J., et al. (2019). Prebiotics: Definition, types, sources, mechanisms, and clinical applications. Foods 8(3), 92. doi: 10.3390/foods8030092
Dewey C. E., Cox B. C., Straw B. E., Bush E. J., Hurd H. S. (1996). Associations between off-label feed additives and farm size, veterinary consultant use, and animal age. Preventative Veterinary Medicine Elsevier 31, 133–146. doi: 10.1016/s0167-5877(96)01077-x
Dwyer D. J., Belenky P. A., Yang J. H., Macdonald I. C., Martell J. D., Takahashi N., et al. (2014). Antibiotics induce redox-related physiological alterations as part of their lethality. Proc. Natl. Acad. Sci. 111, E2100–E2109. doi: 10.1073/pnas.1401876111
Edwards M. V., Edwards A. C., Millard P., Kocher A. (2014). Mannose rich fraction of saccharomyces cerevisiae promotes growth and enhances carcass yield in commercially housed grower–finisher pigs. Anim. Feed Sci. Technol. 197, 227–232. doi: 10.1016/j.anifeedsci.2014.08.004
European Food Safety Authority and European Centre for Disease Prevention and Control (2021). The European union summary report on antimicrobial resistance in zoonotic and indicator bacteria from humans, animals and food in 2018/2019. EFSA J. 19, e06490.
European Union (2018). Council directive 2019/5/EC on the community procedures for the authorisation and supervision of medicinal products for human and veterinary use and establishing a European Medicines Agency. (2019) Official Journal L4/24
Gibson G. R., Scott K. P., Rastall R. A., Tuohy K. M., Hotchkiss A., Dubert-Ferrandon A., et al. (2010). Dietary prebiotics: current status and new definition. Food Sci. Technol. Bulletin: Funct. Foods 7, 1–19. doi: 10.1616/1476-2137.15880
Holmer I., Salomonsen C. M., Jorsal S. E., Astrup L. B., Jensen V. F., Høg B. B., et al. (2019). Antibiotic resistance in porcine pathogenic bacteria and relation to antibiotic usage. BMC Veterinary Res. 15, 449. doi: 10.1186/s12917-019-2162-8
Lasserre P., Smulders S. (2013). An ABC of nonrenewable–renewable resource interactions: Antibiotics, biofuels, carbon decay, expropriation, forestry. Resource Energy Economics 35, 558–571. doi: 10.1016/j.reseneeco.2013.09.003
Lhermie G., Wernli D., Jørgensen P. S., Kenkel D., Tauer L. W., Gröhn Y. T. (2019). Global resistance to antimicrobials and their sustainable use in agriculture. Lancet Planetary Health 3, e109–e110. doi: 10.1016/S2542-5196(18)30251-1
Lobritz M. A., Belenky P., Porter C. B., Gutierrez A., Yang J. H., Schwarz E. G., et al. (2015). Antibiotic efficacy is linked to bacterial cellular respiration. Proc. Natl. Acad. Sci. U.S.A. 112, 8173–8180. doi: 10.1073/pnas.1509743112
Merrett G. L., Bloom G., Wilkinson A., Macgregor H. (2016). Towards the just and sustainable use of antibiotics. J. Pharm. Policy Pract. 9, 31. doi: 10.1186/s40545-016-0083-5
Pirt S. J. (1965). The maintenance energy of bacteria in growing cultures. R. Soc. Publishing 163, 224–221. doi: 10.1098/rspb.1965.0069
Richards G. R., Patel M. V., Lloyd C. R., Vanderpool C. K. (2013). Depletion of glycolytic intermediates plays a key role in glucose-phosphate stress in escherichia coli. J. Bacteriol 195, 4816–4825. doi: 10.1128/JB.00705-13
Ricke S. C. (2015). Potential of fructooligosaccharide prebiotics in alternative and nonconventional poultry production systems. Poultry Sci. 94, 1411–1418. doi: 10.3382/ps/pev049
Romero-Barrios P., Deckert A., Parmley E. J., Leclair D. (2020). Antimicrobial resistance profiles of escherichia coli and salmonella isolates in Canadian broiler chickens and their products. Foodborne Pathog. Dis. 17, 672–678. doi: 10.1089/fpd.2019.2776
Smith H., Grant S., Parker J., Murphy R. (2020). Yeast cell wall mannan rich fraction modulates bacterial cellular respiration potentiating antibiotic efficacy. Sci. Rep. 10, 21880. doi: 10.1038/s41598-020-78855-5
Spring P., Wenk C., Connolly A., Kiers A. (2015). A review of 733 published trials on Bio-mos®, a mannan oligosaccharide, and Actigen®, a second generation mannose rich fraction, on farm and companion animals. J. Appl. Anim. Nutr. 3, E8. doi: 10.1017/jan.2015.6
Stokes J. M., Lopatkin A. J., Lobritz M. A., Collins J. J. (2019). Bacterial metabolism and antibiotic efficacy. Cell Metab. 30, 251–259. doi: 10.1016/j.cmet.2019.06.009
WHO (2019). WHO list of critically important antimicrobials for human medicine 6th revision (World Health Organisation (WHO).
Keywords: antimicrobial resistance (AMR), prebioitcs, antibiotic, tetracycline, cellular respiration, sustainable agriculture
Citation: Grant S, Smith H and Murphy R (2023) Mannan based prebiotics modulate growth rate and energy phenotype of tetracycline resistant E. coli. Front. Anim. Sci. 3:1069280. doi: 10.3389/fanim.2022.1069280
Received: 13 October 2022; Accepted: 15 December 2022;
Published: 13 January 2023.
Edited by:
Todd Riley Callaway, University of Georgia, United StatesReviewed by:
Uttam Kumar Jana, Saha Institute of Nuclear Physics (SINP), IndiaMohammad Munir, Universiti Malaysia Sarawak, Malaysia
Copyright © 2023 Grant, Smith and Murphy. This is an open-access article distributed under the terms of the Creative Commons Attribution License (CC BY). The use, distribution or reproduction in other forums is permitted, provided the original author(s) and the copyright owner(s) are credited and that the original publication in this journal is cited, in accordance with accepted academic practice. No use, distribution or reproduction is permitted which does not comply with these terms.
*Correspondence: Sharon Grant, c2dyYW50QGFsbHRlY2guY29t