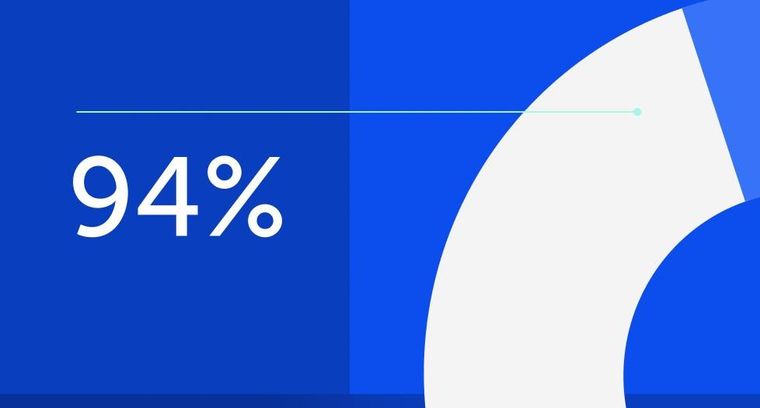
94% of researchers rate our articles as excellent or good
Learn more about the work of our research integrity team to safeguard the quality of each article we publish.
Find out more
ORIGINAL RESEARCH article
Front. Anim. Sci., 24 November 2022
Sec. Animal Nutrition
Volume 3 - 2022 | https://doi.org/10.3389/fanim.2022.1062324
This study collates compositional analysis of seaweeds data with information generated from in vitro gas production assays in the presence and absence of seaweeds. The aim was to assess and rank 27 native northern European seaweeds as potential feed ingredients for use to reduce methane emissions from ruminants. It provides information for use in future in vivo dietary trials concerning feed manipulation strategies to reduce CH4 emissions efficiently from domestic ruminants based on dietary seaweed supplementation. The seaweeds H. siliquosa and A. nodosum belonging to phylum Phaeophyta displayed the highest concentration of phlorotannins and antioxidant activity among the macroalgae giving anti-methanogenic effect in vitro, while this explanation was not valid for the observed reduction in methane when supplementing with C. filum and L. digitata in this study. D. carnosa and C. tenuicorne belonging to phylum Rhodophyta had the highest protein content among the macroalgae that reduced methane production in vitro. There were no obvious explanation from the compositional analysis conducted in this study to the reduced methane production in vitro when supplementing with U. lactuca belonging to phylum Chlorophyta. The strongest and most complete methane inhibition in vitro was observed when supplementing with Asparagopsis taxiformis that was used as a positive control in this study.
There are environmental goals setting out a vision of climate neutrality by 2050 in the EU. These goals are in line with the global climate commitment regulated in the Paris agreement of limiting global warming optimal to 1.5°C of the pre-industrial baseline (European Commission, 2022). Emissions of methane (CH4) from ruminant livestock (enteric fermentation and anaerobic manure storage) comprise 33% of the total anthropogenic sources and represent the second greatest anthropogenic CH4 source after fossil fuels (Jackson et al., 2020). Concentrations of CH4 in the atmosphere have been rising over the past decade and since it, heats 28 times more on a 100-year time scale than the corresponding carbon dioxide (CO2) reduced concentrations would give an immediate effect on the global temperature scale. Recent data based on radioactive carbon concentration in CH4 indicated that anthropogenic emissions last decade have been higher than previously estimated (Petrenko et al., 2017). Further, satellite data have suggested that the increased global emissions of CH4 were mostly due to increased extraction of shale gas, and that the natural fossil fuel industry contributes twice the amount of CH4 emissions as animal agriculture (Howarth, 2019). Despite this, the global cattle population is predicted to increase because of future greater demand for sustainable animal food products. To date, two thirds of the world’s agricultural land is composed of pastures and permanent meadows and is used for livestock (FAO, 2019).
There are few feed manipulation strategies for mitigating enteric CH4 production from ruminants that have been identified, as efficient and applicable alternatives in real farming situation are required. The inhibitor 3-nitroxypropanol has successfully demonstrated CH4 reducing effects supplemented at low doses to dairy cows (Hristov et al., 2015). The tropical macroalgae Asparagopsis taxiformis has recently shown the greatest CH4 reducing effect of all dietary inhibitors both in vitro and in vivo, and is also regarded a natural supplement (Machado et al., 2016; Chagas et al., 2019; Stefenoni et al., 2021). The reduction of CH4 has been attributed to inhibition of ruminal archaea by the volatile compound bromoform, which is found in red seaweeds like Asparagopsis sp. However, other compounds including peptides, carbohydrates, lipids, and phlorotannins in green and brown macroalgae also show promise to inhibit methanogens and reduce CH4 emissions. Additionally, the benefits of brown and green seaweeds for animal health are recognized as additional positive attributes for their use as feed additives for ruminants (Abbott et al., 2020).
This study aimed to characterize a number of seaweeds native to Northern Europe and use generated data in combination with in vitro gas production assays in the presence and absence of the same seaweeds to rank the potential of seaweeds for use as CH4 mitigating feed additives. This work also provides background information for future in vivo evaluations of feed manipulation strategies for efficiently mitigating enteric CH4 production from domestic ruminants based on dietary seaweed supplementation.
The handling of animals in this experiment was approved by the Swedish Ethics Committee on Animal Research (Dnr A 32-16), represented by the Court of Appeal for Northern Norrland in Umeå, and the experiment was carried out in accordance with laws and regulations governing experiments performed with live animals in Sweden.
The macroalgal samples represented the three main phyla: Chlorophyta (green), Phaeophyta (brown) and Rhodophyta (red). Most of the macroalgae were harvested from Skagerrak at Tjärnö (58°52′N 11°9′E) on the West coast of Sweden between August and September 2021. A few macroalgae were also harvested from the Baltic Sea in Karlshamn (56°10′N 14°51′E), Kalmar (56°39′N 16°21′E), Norra Möckleby (56°38′N 16°40′E) and Sölvesborg (56°1′N 14°34′E). The red seaweed Asparagopsis taxiformis (AT) was harvested from the Atlantic Ocean at the Azores (38.6°N, 28°W), Portugal, in October 2018. All macroalgae used in the in vitro experiment are presented in Table 1. Seaweeds were harvested from the shore in accordance with the Nagoya protocol guidelines (https://www.cbd.int/abs/doc/protocol/nagoya-protocol-en.pdf), packed in cool boxes, and transported via overnight courier to Teagasc Food Research Centre, Ashtown, Dublin 15, Ireland on dry ice. Samples were washed to remove sand and epiphytes and stored at -18°C. All samples were frozen and freeze-dried using an industrial-scale FD 80 model freeze-drier (Cuddon Engineering, Marlborough, New Zealand) as previously described (Fitzgerald et al., 2014). A freeze-dried reference sample for each species was stored at Teagasc Ashtown and a fraction of each freeze-dried seaweed sent by courier on dry ice to the Swedish University of Agricultural Sciences for in vitro assessment of CH4 mitigating capacity. Further samples were sent to the Atlantic Technical University (ATU, formerly Sligo IT, Ireland) to quantify the halocarbon and bromoform content of the samples. Samples for analysis were ground into a powder using a WaringÒ blender and stored in vacuum-packed bags at - 80°C until further use.
Table 1 Swedish seaweeds and Asparagopsis taxiformis (positive control) screened in vitro for CH4 mitigating effect.
All seaweeds were supplemented independently at 5 and 10% on an organic matter (OM) basis to an experimental diet that consisted solely of a timothy (Phleum pratense) dominated grass silage. Bonnemaisonia hamifera was an exception, and was dosed at two lower supplementation levels of 1 and 2% on an OM basis due to limitations in amount of pure biomass available at the time of incubation. The supplementation was made so that 1.00 g of OM was incubated across all samples. The grass silage used was originally harvested from Röbäcksdalens research farm in Umeå (63°45´N, 20°17´E), Sweden.
Two lactating Swedish Red cows, fed ad libitum on a diet of 600 g/kg grass silage and 400 g/kg concentrate on a dry matter (DM) basis, were used as donor animals of rumen inoculum for all incubations. The rumen fluid from each cow was filtered separately using a double layer of cheesecloth into Thermos flasks that were pre-warmed and flushed with CO2 prior to collection. Rumen fluid was directly transported to the laboratory after collection (within 15 min). Equal amounts from each cow were blended, strained through four layers of cheesecloth, and added to buffered mineral solution (Menke and Steingass, 1988) including Peptone™ (pancreatic digested casein; Merck, Darmstadt, Germany) at 39°C under constant mixing and CO2 flushing, to give a buffered rumen fluid solution with a rumen fluid:buffer ratio of 1:4 (v:v). Prior to the in vitro incubations, the grass silage sample was dried at 60°C for 48 h and milled in a Retsch SM 2000 cutting mill (Retsch GmbH, Haan, Germany) to pass through a 1-mm screen. The substrates were weighed into serum bottles flushed with CO2 prior to start of incubation, and 60 mL of the previously prepared buffered rumen fluid was added. All bottles were placed in a water-bath and continuously agitated at 39°C during an incubation period of 48 h. The samples were in total distributed over ten consecutive in vitro runs to get the same number of valid replications of each sample, with three replicates of each sample. All runs included duplicate bottles with blanks (i.e. bottles with 60 mL of buffered rumen fluid with no sample or treatment in), control sample either consisting solely of grass silage or grass silage added AT. The AT was added only in 0.5% on OM basis so that no replacement of the grass silage was made. All samples were randomly distributed between the in vitro bottles in each run, and never incubated in the same bottle between the runs.
Gas production was measured using a fully automated system (Gas Production Recorder, GPR-2, Version 1.0 2015, Wageningen UR). The system make automated readings every 12 min and all readings are corrected to the normal air pressure (101.3 kPa) (Cone et al., 1996). Measurement of CH4 in vitro was performed by withdrawing gas samples (0.2 mL) at 2, 4, 8, 24, 32, and 48 h during the incubation from all bottles. The concentration of CH4 was determined by immediately after collection injecting the gas sample in a Trace 1300 gas chromatograph (Thermo Fisher Scientific, Waltham, MA, USA). Sample gas production was corrected for mean blank gas production within each run. The CH4 concentration (mL/g sample) of all samples were calculated from total headspace volume (265 mL), and the ratio of CH4 emissions in the outflow gas from the in vitro system (0.55). A ruminal retention time of 50 h, divided in 20 h in the first compartment and 30 h in the second compartment, corresponding to the maintenance level of feed intake, was used in model simulations to achieve in vivo predicted CH4 according to Ramin and Huhtanen (2012).
Only seaweeds that showed a CH4 mitigating effect compared to pure grass silage incubated in vitro were further analyzed in terms of composition, antioxidant activity and content of bioactive substances polyphenols, phlorotannins, iodine and bromoform. All chemicals used in the analytical work were of reagent-grade and all solvents used were of high performance liquid chromatography-grade.
The total protein content of supplied Swedish seaweed samples was determined with a LECO FP628 Protein analyzer (LECO Corp., St. Joseph, MI, United States) applying the Dumas method and according to the Association of Official Analytical Chemists (AOAC) method 955.04 (AOAC, 2000). A nitrogen to protein conversion factor specific to the seaweed type was used to calculate protein concentration in the samples as described previously by Angell et al. (2016). The concentration of ash in the samples was determined after subsequent drying at 550°C for 4 h according to previously published methods (Verspreet et al., 2021). Quantification of the lipid content of seaweed samples was achieved using the AOAC Method 2008.06 with an Oracle rapid NMR fat analyser. Samples were prepared according to standard procedures used for these analyses as described previously (AOAC, 1998).
The total antioxidant capacity assay kit based on 2, 2’-azino-bis (3-ethylbenzothiazoline-6-sulphonic acid) (ABTS) inhibition was supplied by BioVision Inc. (Milpitas, CA, USA). The antioxidant activity of seaweed extracts was determined using this assay and performed as described previously by Dave and colleagues (Dave et al., 2016). A standard curve was made using Trolox (Merck Life Sciences, Arklow, Co. Wicklow, Ireland) with blank assessment from the assay buffer. Resveratrol (Merck Chemical, Cork, Ireland) was used as a positive control. Antioxidant activity was assessed as mM Trolox equivalents (TE). The total phenolic content (TPC) of supplied seaweeds was determined using a method described by Singleton et al. (1999). Briefly, Phloroglucinol (Merck, Dublin, Ireland) was used to formulate a standard curve and quantify the phenol content of samples. The stock solution of phloroglucinol (120 mg/l in ddH2O) was diluted with water (100, 80, 60, 40, 20 mg ml/l). A 100 µl of seaweed sample, diluted to 0.75 mg ml-1, or standard solution, 100 µl of methanol, 100 µl of Folin–Ciocalteu reagent and 700 µl of 20% sodium carbonate were added to micro-centrifuge tubes. All samples were immediately vortexed and incubated at room temperature in the dark for 20 min. Samples were subsequently centrifuged at 13,000 rpm for 3 min and the absorbance of the supernatant was measured at 735 nm using a Shimadzu PharmaSpec UV-1700 spectrophotometer (Shimadzu Scientific Instruments, MD, USA). All measurements were carried out in triplicate (n=3). The calibration curve was used to obtain the TPC values of the seaweed samples expressed as micrograms of phloroglucinol equivalents (PE) per milligram of sample (µg PE mg-1).
Phlorotannins were extracted from the brown seaweeds using the previously published method of Koivikko and co-workers (Koivikko et al., 2005). Lipids were removed from aliquots of 0.5 g of seaweed samples dissolved in n-hexane (n=3). 10 ml of aqueous acetone (7:3 acetone: water v; v) was then applied to de-fatted material, four times, for 1 hour at 400 rpm. Samples were then centrifuged for 5 minutes at 4000 rpm and evaporated to dryness under reduced pressure, at 30°C. The method of Fairhead and colleagues (Fairhead et al., 2005) was used to extract phlorotannins from the brown seaweed polyphenol extracts. Briefly, the dried acetone: water extract was re-suspended in 30 mL of methanol, adsorbed into cellulose (approximately 2× the residue weight) and then dried under reduced pressure, at 30°C. Further, subsequently washed with toluene to remove pigments and phlorotannins eluted from the cellulose using acetone: water (70:30 v: v). Phlorotannin content was determined using phloroglucinol external standard in a linear calibration curve (y = 0.0254x; presenting an r2 value of 0.9998) ranging between 0.98 and 62.50 µg phloroglucinol/mL.
An Iodine colorimetric assay kit (Catalog #K2037-100) containing Iodine standard, Iodine substrate, treatment reagent, sample diluent and precipitation solution was purchased from BioVision Incorporated (Milpitas Blvd., Milpitas, CA, USA) and used to determine the iodine content of supplied Swedish seaweeds in accordance with the manufacturer’s recommendations. The iodine content of Swedish seaweeds was determined using the Iodide Colorimetric Assay Kit (BioVision, Milpitas, California, USA). Briefly, 100 mg of seaweed was weighed and transferred to an Eppendorf tube (n=3). 0.5 ml of sample diluent was added to the tube and homogenized for 10 min using a dounce tissue homogenizer (BioVision, Milpitas, CA, USA). Subsequently samples were incubated at room temperature for 10 min. Thereafter, centrifuged at 12,000 g for 15 min and the supernatant collected. 10 µl of this clear supernatant was used in the assay, which was performed in accordance with the manufacturer’s instructions.
The analytical grade solvents hexane and dichloromethane (DCM) were purchased from Lennox (Dublin, Ireland). Bromoform analytical standard and MS grade hexane were both purchased from Merck Life Science Ltd. (Wicklow, Ireland). Bromoform analysis of each seaweed was carried using a modified version of the method previously described by Machado et al. (2016). Freeze dried seaweed samples were defatted, using hexane, followed by exhaustive solid-liquid extraction using DCM. Extracts of each seaweed were then dried using a rotary evaporator (Buchi Rotavapor R-3000, Switzerland). Prior to analysis, DCM extracts were reconstituted (1 mg/ml) using MS grade hexane and centrifuged at 13,000 rpm for 2 min. Analysis of each sample extract was carried out using an Agilent 7890A Gas Chromatography System coupled to an Agilent 5975C triple axis detector (TAD) mass spectrometer (Agilent Technologies Ltd., Cork, Ireland), managed by Agilent ChemStation software. Separation was achieved using an Agilent DB Wax fused silica column (30 m, 0.25 mm ID and 0.25 µm film thickness) with 1µl injections in splitless mode. For each run, the oven temperature was held at 40°C for 1 min, before ramping to 230°C over 16 min, where it was then held for a further 2 min. The flow rate of the Helium (He) carrier gas was 1.2 ml/min. The mass spectrometer was operated using electron ionization (EI) at 70 eV, in the scan range m/z 60 – 300. The ion source was set at 230°C. Detection of bromoform was conducted by retention time (7.39 min) and mass spectrum comparison to a reference bromoform standard, as well as comparison to NIST17 Mass Spectral Library (v2.0). Quantification of bromoform was achieved using a calibration curve of a reference bromoform standard and expressed as micrograms per gram of dry weight seaweed sample (µg/g DWS).
Data of total gas in vitro and in vivo predicted CH4 production were analysed using the GLM procedure in SAS (SAS Institute Inc., Cary, NC, version 9.4), by a model correcting for effect of run and treatment:
where Yij is dependent variable ij, µ is overall mean, Ti is treatment i, Rj is run j, and eij ~ N(0, ) is the random residual error. Contrasts were used for evaluation of control diet vs. treatment and of linear responses to level of treatment.
The control sample of grass silage produced 200 mL/kg OM of total gas in vitro and in vivo predicted CH4 was 30.4 mL/kg OM. The positive control of grass silage sample added Asparagopsis taxiformis produced 187 mL/kg OM of total gas in vitro and in vivo predicted CH4 was 0 mL/kg OM, which was significantly different from all other treatments (P< 0.01). There were no linear effects detected between the levels of any of the seaweed supplementation (P > 0.05). Further, there were no differences between any of the controls and the seaweed supplementations in terms of total gas (P > 0.05). The only seaweed in the phylum Chlorophyta that displayed a lower (P< 0.05) production of CH4 compared to the control grass silage sample, which was Ulva lactuca of 24.3 mL/kg OM (Figure 1A). In the phylum Phaeophyceae four different seaweed displayed a lower (P< 0.05) production of CH4 compared to the control grass silage sample, which were Chorda filum, Laminara digitata, Halidrys siliquasa and Ascophyllum nodosum (Figure 1B). Dilsea carnosa and Ceramium tenuicorne belonging to Rhodophyta produced less CH4 of 25.2 and 22.8 mL/kg OM when added to the grass silage compared to the control without any seaweed added (Figure 1C).
Figure 1 (A–C): Total gas and in vivo predicted CH4 production (mL/kg organic matter (OM)) for the three main phyla Chlorophyta (green; a), Phaeophyceae (brown; b) and Rhodophyta (red; c) of experimental seaweeds added to grass silage. The standard errors of the least squares means were 14.1 for total gas and 1.76 mL/kg of OM for CH4. Asterisks indicate least significant difference of seaweed treatment compared to control sample of grass silage (CH4): **P< 0.01 and *P< 0.05. There were no differences between control samples and treatments in terms of total gas (P > 0.05) and no CH4 was produced with Asparagopsis taxiformis, which was significantly different from all other treatments (P< 0.01) (results not indicated with asterisks).
Compositional analysis in terms of the protein content of the seaweeds examined in this study and identified as having potential to reduce CH4 in vitro ranged from 15.5% of DM for the red seaweed Dilsea carnosa to 6.12% of DM for Halidrys siliquosa (lowest protein content observed). The highest lipid level was observed in the brown macroalga Ascophyllum nodosum (5.78% of DM) and the lowest lipid content found in the green alga Ulva lactuca (0.45% of DM). The ash content of the seaweeds examined was between 12.2% of DM for D. carnosa and 38.9% of DM for Ulva lactuca,
The polyphenol content of Swedish seaweeds determined using the Folin-Ciocalteu method is reported in Table 2. Variations were observed in terms of the phenolic content between the different seaweeds with the brown seaweeds H. siliquosa found to contain 226.15-µg Phloroglucinol equivalents (PGE)/mg sample and A. nodosum containing 212.6-µg PGE/mg sample showing significantly higher phenolic content than all other red and green seaweeds analyzed. The iodine levels determined for seaweeds assessed in this study ranged from 1.14 µg/100 mg sample for L. digitata to 3.4 µg/100 mg sample for C. filum.
There were no bromoform detected in any of the seaweeds examined in this study and identified as having potential to reduce CH4 in vitro. Total antioxidant capacity by 2, 2′-azino-bis (3-ethylbenzothiazoline-6-sulphonic acid) (ABTS) inhibition by seaweed polyphenol extracts of CH4 mitigating efficient seaweeds is given in Figure 2.
Figure 2 Total antioxidant capacity by 2, 2′-azino-bis (3-ethylbenzothiazoline-6-sulphonic acid) (ABTS) inhibition by seaweed polyphenol extracts. All of the samples and the positive control resveratrol were tested at a concentration of 1 mg/mL and the results are expressed in mM Trolox equivalents.
This in vitro study evaluated the dietary CH4 mitigating potential of a larger number of seaweeds by predicting in vivo related CH4 production by cattle. This type of larger screenings are not feasible in vivo. The in vitro gas production system generates a large number of registrations of total gas and CH4 produced, but has some limitations since it is a batch culture approach with no ruminal passage and digestion over time. The in vitro method used in this study comprised a modeling approach originally developed with CH4 data generated from the automated gas in vitro system (Ramin and Huhtanen, 2012) to correct for this. Ramin and Huhtanen (2012) assumed a concentration of gross energy of 18.5 MJ/kg DM and the predicted proportion of CH4 energy for a 1000 mg sample was calculated to be 0.061. This proportion of CH4 energy was similar to in vivo values observed in dairy cows at production levels of intake (Yan et al., 2000). The in vivo CH4 prediction by Ramin and Huhtanen (2012) was evaluated by Danielsson et al. (2017) using data from in vivo studies with CH4 emissions measured in respiration chambers. The results showed a high correlation (R2 = 0.96) between chamber data and predicted in vivo CH4 values, confirming that the in vitro system is a useful tool for experimental screening.
In the present study, in vivo predicted CH4 production was inhibited completely in the positive control consisting of grass silage with Asaparagopsis taxiformis at an inclusion of 0.5% on an OM basis. This is agreement with an earlier study in our laboratory by Chagas et al. (2019) that found almost complete inhibition of CH4 production, and with neglectable impact on ruminal fermentation at the same inclusion level. Results in the literature has varied somewhat, but generally the natural anti-methanogenic red seaweed Asparagopsis taxiformis has shown a high CH4 inhibitory effect in vitro of 84.7 and 99% at inclusion levels of 1 and 2% on OM basis, respectively (Machado et al., 2016). Additionally, Stefenoni et al. (2021) showed in a short-term in vivo experiment that inclusion of AT at 0.5% of DM intake decreased total daily CH4 emission in lactating dairy cows by 35%.
Methanogenesis is the main mechanism to remove H2 from the rumen, to maintain effective fermentation. Red seaweeds contain over 1500 secondary metabolites and can be rich in the halogenated aliphatic organic compounds, e.g. chlorobromomethane and bromoform. Species of the Bonnemaisoniaceae family (Rhodophyta) have been characterized with high and broad spectrum of antimicrobial activity due to their high concentration and diverseness of volatile halogenated compounds (Abbott et al., 2020). The major halogenated natural anti-methanogenic substance in Asparagopsis taxiformis is bromoform (Machado et al., 2016). The anti-methanogenic mechanism by bromoform is an inhibition of the cobamide dependent methyl transfer required in the synthesis of coenzyme-M involved in the last step of methanogenesis (Machado et al., 2016). There were no bromoform detected in any of the macroalgae that showed a CH4 inhibitory effect in vitro in this study.
Other seaweed bioactives can also have an impact on methanogens. Compounds including peptides, carbohydrates, lipids, phlorotannins, saponins, sulfonated glycans and bacteriocins have also been associated with inhibitory effects on methanogens and decreased CH4 emissions (Abbott et al., 2020). Some of these compounds have also been found in macroalgae. The brown seaweed H. siliquosa and A. nodosum showed anti-methanogenic activity and contained the highest concentration of phlorotannins among all seaweeds in this study. Phlorotannins display antimicrobial activity and have shown to act on the rumen cellulolytic bacterium Fibrobacter succinogenes. Visser et al. (2017) concluded that phlorotannins from L. digitata decreased degradation of protein and inhibited methanogenesis in vitro. In this study L. digitata showed the lowest concentration of phlorotannins among the brown seaweed displaying anti-methanogenic activity in vitro. Concentrations of phlorotannins in brown seaweeds have been shown to be highly variable and likely this is due to variations in biotic and abiotic environmental conditions (Jormalainen et al., 2003). Their concentration varies between species, and has been reported to range from 0.5 to 30% dry weight of the alga. Brown seaweeds from locations at higher latitudes contain more phlorotannins perhaps due to the need of seaweeds to modulate chemical defense production in response to stimuli at these latitudes compared to seaweeds from tropical regions where the environmental conditions are more constant (Ank et al., 2019). Several seaweeds are rich in complex polysaccharides, amongst green seaweed of the genus Ulva are known to have a high carbohydrate content (Holdt and Kraan, 2011). Additionally, Chlorophyta are reported to be rich in marinen saponins (Feroz, 2018). Neither specific complex carbohydrates nor saponins were analyzed in this study, but are likely anti-methanogenic substances in U. lactuca. Peptides and bacteriocins from red seaweeds could also inhibit the production of CH4 by acting on archaea and protozoa (Abbott et al., 2020). This would be in line with the higher protein content of D. carnosa and C. tenuicorne.
Several factors like species, season of harvest, growth habitat, and environmental conditions impact and create variation in the chemical composition of seaweeds. Indeed, growth rate and chemical composition are influenced by sunlight, salinity, sea depth and water currents (Øverland et al., 2019). Protein values reported are low compared to terrestrial, vegetable plant protein sources such as soybean that can contain ~ 40% protein on a DW basis (Karr-Lilienthal et al., 2006). Protein content values of up to 47% of the DW of seaweeds were reported previously for some species such as Porphyra sp. Despite the low protein content observed here, seaweeds could still be considered as a source of protein in animal production since the content of total essential amino acid of seaweed derived proteins is high (Fleurence, 2004), but do not likely impact on seaweed CH4 mitigation effect. Supplementary protein feeding can decrease CH4 emissions per unit of product in dairy cows due to ruminal fermentation that produce less CH4 from protein compared with carbohydrates, and due to increased milk yield (Gidlund et al., 2015). However, overall potential to mitigate CH4 and especially total greenhouse gas emissions by increased protein supplementation from seaweed supplementation must be regarded small. The ash content of seaweeds in this study compares favorably with values identified previously for Swedish seaweeds (Olsson et al., 2020). A factor contributing to ash content could be salt present in seawater associated with the biomass, despite the fact that seaweeds were washed with tap water prior to freeze-drying. Toth and colleagues previously found that high nitrate levels decreased the total ash content of the seaweeds, specifically U. fenestrata (Toth et al., 2020). The lipid values observed are broadly in line with lipid values reported previously for seaweed species, but according to findings from the literature relative to seaweed lipid content and lipid content rarely exceeds 4% of total biomass of seaweeds (Øverland et al., 2019). However, the lipid content of A. nodosum corresponds well with values observed previously and fall within the range of 2-7% of DM as reported by Kumari et al. (2010). The dry matter (%DM) content of all analyzed seaweeds (Table 3) compares favorably with reports concerning the same in the literature and ranged from 5.69% for L. digitata to 13.96% for D. carnosa.
Table 3 Polyphenol and iodine content of Swedish seaweeds expressed in terms of phloroglucinol equivalents (µg PGE/mg sample) and µg iodine/100 mg of seaweed. Average and standard deviation (±) values shown (n = 3). N/D = Not detected.
Jiménez-Escrig et al. (2001) previously reported similar findings concerning the phenolic content of brown seaweeds compared to red seaweeds. In addition, and in agreement with previous studies by Nagai and Yukimoto (2003) and Duan et al. (2006), there was a significant correlation observed in this work between antioxidant activity determined using the ABTS assay method and phenolic content of the CH4 reducing seaweeds analyzed. C. tenuicorne, H. siliquosa and A. nodosum demonstrated 1.83 mM Trolox equivalent, (TE/g sample) 1.38 mM TE/g sample and 0.45 mM TE/g sample, respectively compared to the control Resveratrol (5.2 mM TE/g sample). Electron transfer in anaerobic systems such as the rumen is thought to competitively suppress the reduction of other terminal electron acceptors including carbon dioxide under conditions of methanogenesis. The donor/acceptor capacities of the seaweeds selected was assessed in this work. Seaweeds contain phenolics, specifically phlorotannins in brown seaweeds and the electron donor activity (antioxidant activity) is reflected using the ABTS antioxidant assay. There are several studies regarding the use of flavonoid polyphenols as methane mitigating compounds and some reports of positive reduction of methane using antioxidants both in vitro and in vivo to date. For example, Lee et al. (2020) demonstrated the CH4 emissions reduction effects of antioxidants from Pinus densiflora and Mentha Canadensis in rumen fermentation.
The iodine content of selected seaweeds was determined due to limitations concerning the quantity of iodine allowed in animal feeds and additives. Seaweeds, in particular brown seaweeds, can bio-accumulate iodine especially species belonging to the Kelp family Nitschke and Stengel (2015). Kelps can accumulate up to 2000 mg/kg fresh weight, iodine by absorbing it from seawater. The European maximum levels for iodine in animal feed are outlined in Regulation (EU) 2015/861 and for ruminants for milk production; the limit is 5 mg iodine/kg in complete feed per day (12% moisture), which imply that a dairy cow consuming 20 kg of DM daily will tolerate only 114 mg iodine daily.
The raw data supporting the conclusions of this article will be made available by the authors, without undue reservation.
The handling of animals in this experiment was approved by the Swedish Ethics Committee on Animal Research (Dnr A 32-16), represented by the Court of Appeal for Northern Norrland in Umeå, and the experiment was carried out in accordance with laws and regulations governing experiments performed with live animals in Sweden.
SK, MH, and MR: writing manuscript, conducting in vitro, and compositional analysis; FG: writing manuscript and providing macroalgae; PO’H and OK: conducting compositional analysis and revising manuscript. All authors contributed to the article and approved the submitted version.
The project received funding from the European Union’s Horizon2020 Research & Innovation Programme under grant agreement No 696356. FACCE ERA-GAS is the ERA-NET Cofund for monitoring & mitigation of Greenhouse gases from agri- and silvi-culture. The aim of ERA-GAS is to strengthen the transnational coordination of research programs and provide added value to research and innovation on greenhouse gas (GHG) mitigation in the European Research Area. ERA-GAS is initiated by the Joint Programming Initiative on Agriculture, Food Security and Climate Change (FACCE-JPI).
The authors declare that the research was conducted in the absence of any commercial or financial relationships that could be construed as a potential conflict of interest.
All claims expressed in this article are solely those of the authors and do not necessarily represent those of their affiliated organizations, or those of the publisher, the editors and the reviewers. Any product that may be evaluated in this article, or claim that may be made by its manufacturer, is not guaranteed or endorsed by the publisher.
Øverland M., Mydland L. T., Skrede A. (2019). Marine macroalgae as sources of protein and bioactive compounds in feed for monogastric animals. J. Sci. Food Agric. 99.1, 13–24. doi: 10.1002/jsfa.9143
Abbott D. W., Aasen I. M., Beauchemin K. A., Gröndahl F., Gruninger R., Hayes M., et al. (2020). Seaweed and seaweed bioactives for mitigation of enteric methane: Challenges and opportunities. Animals 10, 2432. doi: 10.3390/ani10122432
Angell A. R., Leonardo M., de Nys R., Paul N. A. (2016). The protein content of seaweeds: a universal nitrogen to protein conversion factor of five. J. Appl. Phycol. 28, 511–514. doi: 10.1007/s10811-015-0650-1
Ank G., da Gama B. A. P., Pereira R. C. (2019). Latitudinal variation in phlorotannin contents from southwestern Atlantic brown seaweeds. Peer J. Life Environ. 7, 7379–7398. doi: 10.7717/peerj.7379
Association of Official Analytical (AOAC) (1998). Official methods of analysis of AOAC international (Maryland: AOAC International).
Association of Official Analytical (AOAC) (2000). Official methods of analysis. the association of official analytical chemists. 17th Edn (Gaithersburg, MD: AOAC).
Chagas J. C., Ramin M., Krizsan S. J. (2019). In vitro evaluation of different dietary methane mitigation strategies. Animals 9, 1120. doi: 10.3390/ani9121120
Cone J. W., Van Gelder A. H., Visscher G. J. W., Oudshoorn L. (1969). Influence of rumen fluid and substrate concentration on fermentation kinetics measured with a fully automated time related gas production apparatus. Anim. Feed Sci. Technol. 61, 113–128.
Danielsson R., Ramin M., Bertilsson J., Lund P., Huhtanen P. (2017). Evaluation of an in vitro system for predicting methane production in vivo. J. Dairy Sci. 100, 8881–8894. doi: 10.3168/jds.2017-12675
Dave L. A., Hayes M., Mora L., Montoya C. A., Moughan P. J., Rutherfurd S. M. (2016). Gastrointestinal endogenous protein derived bioactive peptides: An in vitro study of their gut modulatory potential. Int. J. Mol. Sci. 17, 482. doi: 10.3390/ijms17040482
Duan X.-J., Zhang X.-M., Li X.-M., Wang B.-G. (2006). Evaluation of antioxidant property of extract and fractions obtained from a red alga, Polysiphonia urceolata. Food Chem. 95, 37–43. doi: 10.1016/j.foodchem.2004.12.015
European Commission. (2019). Available at: https://ec.europa.eu/clima/eu-action/climate-strategies-targets/2050-long-term-strategy_sv (Accessed 2022 09 12).
FAO. (2019). Available at: https://www.fao.org/statistics/en/ (Accessed 2022 10 05).
Fairhead V. A., Amsler C. D., McClintock J. B., Baker B. J. (2005). Variation in phlorotannin content with two species of brown macroalgae (Desmarestia anceps and d. menziesii) from theWestern antartic peninsula. Polar Biol. 28, 680–699. doi: 10.1007/s00300-005-0735-4
Feroz B. (2018). Saponins from marine macroalgae: A review. J. Mar. Sci. Res. Dev. 8, 4. doi: 10.4172/2155-9910.1000255
Fitzgerald C., Aluko R., Hossain M., Rai D., Hayes M. (2014). The potential of a renin inhibitory peptide from the red seaweed Palmaria palmata as a functional food ingredient following confirmation and characterisation of a hypotensive effect in spontaneously hypertensive rats (SHRs). J. Agric. Food Chem. 62, 8352–8356. doi: 10.1021/jf500983n
Fleurence J. (2004). “Seaweed proteins,” in Proteins in food processing. Ed. Yada R. Y. (Cambridge: Woodhead Publishing Limited), 197–213.
Gidlund H., Hetta M., Krizsan S. J., Lemosquet S., Huhtanen P. (2015). Effects of soybean meal or canola meal on milk production and methane emissions in lactating dairy cows fed grass silage-based diets. J. Dairy Sci. 98, 8093–8106. doi: 10.3168/jds.2015-9757
Holdt S. L., Kraan S. (2011). Bioactive compounds in seaweed: Functional food applications and legislation. J. Appl. Phycol. 23, 543–597. doi: 10.1007/s10811-010-9632-5
Howarth R. W. (2019). Ideas and perspectives: is shale gas a major driver of recent increase in global atmospheric methane? Biogeosciences 16, 3033–3046. doi: 10.5194/bg-16-3033-2019
Hristov A. N., Oh J., Giallongo F., Frederick T. W., Harper M. T., Weeks H. L., et al. (2015). An inhibitor persistently decreased enteric methane emission from dairy cows with no negative effect on milk production. Proc. Natl. Acad. Sci. 112, 10663–10668. doi: 10.1073/pnas.1504124112
Jackson R. B., Saunois M., Bousquet P., Canadell J. G., Poulter B., Stavert A. R., et al. (2020). Increasing anthropogenic methane emissions arise equally from agricultural and fossil fuel sources. Environ. Res. Lett. 15, 071002. doi: 10.1088/1748-9326/ab9ed2
Jiménez-Escrig A., Jiménez-Jiménez I., Pulido R., Saura-Calixto F. (2001). Antioxidant activity of fresh and processed edible seaweeds. J. Sci. Food Agricult. 81(5), 530–534. doi: 10.1002/jsfa.842
Jormalainen V., Honkanen T., Koivikko R., Eranen J. (2003). Induction of phlorotannin production in a brown alga: Defense or resource dynamics? OIKOS 103, 140–150. doi: 10.1034/j.1600-0706.2003.12635.x
Karr-Lilienthal L. K., Bauer L. L., Utterback P. L., Zinn K. E., Frazier R. L., Parsons C. M., et al. (2006). Chemical composition and nutritional quality of soybean meal prepared by extruder/expeller processing for use in poultry diets. J. Agric. Food Chem. 54(21), 8108–8114. doi: 10.1021/jf061425t
Koivikko R., Loponen J., Honkanen T., Jormalainen V. (2005). Contents of soluble, cell-wall-bound and exuded phlorotannins in the brown alga Fucus vesiculosus, with implications on their ecological functions. J. Chem. Ecol. 31, 195–212. doi: 10.1007/s10886-005-0984-2
Kumari P., Kumar M., Gupta V., Reddy C. R. K., Jha B. (2010). Tropical marine macroalgae as potential sources of nutritionally important PUFAs food chem. Food Chemistry 120, 740–757. doi: 10.1016/j.foodchem.2009.11.006
Lee S. J., Lee Y. J., Eom J. S., Kim H. S., Choi Y. Y., Jo S. U., et al. (2020). Effects of the appropriate addition of antioxidants from pinus densiflora and mentha canadensis extracts on methane emission and rumen fermentation. Animals 10, 1888. doi: 10.3390/ani10101888
Machado L., Magnusson M., Paul N. A., Kinley R., Nys R., Tomkins N. (2016). Identification of bioactives from the red seaweed asparagopsis taxiformis that promote antimethanogenic activity in vitro. J. Appl. Phycol. 28, 3117–3126. doi: 10.1007/s10811-016-0830-7
Menke K. H., Steingass H. (1998). Estimation of the energetic feed value obtained from chemical analysis and in vitro gas production using rumen fluid. Anim. Res. Dev. 28, 7–25.
Nagai T., Yukimoto T. (2003). Preparation and functional properties of beverages from sea algae. Food Chem. 81, 327–332. doi: 10.1016/S0308-8146(02)00426-0
Nitschke U., Stengel D. B. (2015). A new HPLC method for the detection of iodine applied to natural samples of edible seaweeds and commercial seaweed food products. Food Chem. 172, 326–334. doi: 10.1016/j.foodchem.2014.09.030
Olsson J., Toth G. B., Albers E. (2020). Biochemical composition of red, green and brown seaweeds on the Swedish west coast. J. Appl. Phycol 32, 3305–3317. doi: 10.1007/s10811-020-02145-w
Petrenko V. V., Smith A. M., Schaefer H., Riedel K., Brook E., Baggenstos D., et al. (2017). Minimal geological methane emissions during the younger dryas–preboreal abrupt warming event. Nature 548, 443–446. doi: 10.1038/nature23316
Ramin M., Huhtanen P. (2012). Development of an in vitro method for determination of methane production kinetics using a fully automated in vitro gas system – a modeling approach. Anim. Feed Sci. Technol. 174, 190–200. doi: 10.1016/j.anifeedsci.2012.03.008
Singleton V. L., Orthofer R., Lamuela-Raventos R. M. (1999). Analysis of total phenols and other oxidation substrates and antioxidants by means of folin-ciocalteu reagent. Methods Enzymol 299, 152–178. doi: 10.1016/S0076-6879(99)99017-1
Stefenoni H. A., Räisänen S. E., Cueva S. F., Wasson D. E., Lage C. F. A., Melgar A., et al. (2021). Effects of the macroalga asparagopsis taxiformis and oregano leaves on methane emission, rumen fermentation, and lactational performance of dairy cows. J. Dairy Sci. 104, 4157–4173. doi: 10.3168/jds.2020-19686
Toth G. B., Harrysson H., Wahlström N. (2020). Effects of irradiance, temperature, nutrients, and pCO2 on the growth and biochemical composition of cultivated Ulva fenestrata. J. Appl. Phycol 32, 3243–3254. doi: 10.1007/s10811-020-02155-8
Verspreet J., Soetemans L., Gargan C., Hayes M., Bastiaens L. (2021). Nutritional profiling and preliminary bioactivity screening of five micro-algae strains cultivated in Northwest Europe. Foods 10, 1516. doi: 10.3390/foods10071516
Visser A. M., Brok A. E., Westphal A. H., Hendriks W. H., Gruppen H., Vincken J.-P. (2017). Resolubilisation of protein from water-insoluble phlorotannin-protein complexes upon classification. J. Agric. Food Chem. 65, 9595–9602. doi: 10.1021/acs.jafc.7b03779
Keywords: phlorotannins, methane, macroalgae, ruminants, greenhouse gas
Citation: Krizsan SJ, Hayes M, Gröndahl F, Ramin M, O’Hara P and Kenny O (2022) Characterization and in vitro assessment of seaweed bioactives with potential to reduce methane production. Front. Anim. Sci. 3:1062324. doi: 10.3389/fanim.2022.1062324
Received: 05 October 2022; Accepted: 09 November 2022;
Published: 24 November 2022.
Edited by:
Luciano Pinotti, University of Milan, ItalyReviewed by:
Obert Chenjerayi Chikwanha, Stellenbosch University, South AfricaCopyright © 2022 Krizsan, Hayes, Gröndahl, Ramin, O’Hara and Kenny. This is an open-access article distributed under the terms of the Creative Commons Attribution License (CC BY). The use, distribution or reproduction in other forums is permitted, provided the original author(s) and the copyright owner(s) are credited and that the original publication in this journal is cited, in accordance with accepted academic practice. No use, distribution or reproduction is permitted which does not comply with these terms.
*Correspondence: Sophie J. Krizsan, c29waGllLmtyaXpzYW5Ac2x1LnNl
Disclaimer: All claims expressed in this article are solely those of the authors and do not necessarily represent those of their affiliated organizations, or those of the publisher, the editors and the reviewers. Any product that may be evaluated in this article or claim that may be made by its manufacturer is not guaranteed or endorsed by the publisher.
Research integrity at Frontiers
Learn more about the work of our research integrity team to safeguard the quality of each article we publish.