- 1School of Chemical Engineering and Translational Nanobioscience Research Center, Sungkyunkwan University, Suwon, South Korea
- 2Natural Biologics Inc., Newfield, NY, United States
- 3Department of Animal Science, Cornell University, Ithaca, NY, United States
The development of natural, broadly acting antimicrobial solutions to combat viral and bacterial pathogens is a high priority for the livestock industry. Herein, we cover the latest progress in utilizing lipid-based monoglycerides as feed additives to address some of the biggest challenges in animal agriculture. The current industry needs for effective antimicrobial strategies are introduced before discussing why medium-chain monoglycerides are a promising solution due to attractive molecular features and biological functions. We then critically analyze recent application examples in which case monoglycerides demonstrated superior activity to prevent feed transmission of viruses in swine and to mitigate bacterial infections in poultry along with gut microbiome modulation capabilities. Future innovation strategies are also suggested to expand the range of application possibilities and to enable new monoglyceride delivery options.
Introduction
Infectious diseases caused by viral and bacterial pathogens are a major challenge in the livestock industry, contributing to significant productivity and economic losses (Swayne, 2013; Vanderwaal and Deen, 2018; Shurson et al., 2022). Hence, there is widespread interest in developing strategies to prevent and treat pathogenic infections related to various commercially significant viruses and bacteria.
One widely used industry approach is the prophylactic use of antimicrobial compounds, often delivered in the form of feed or water supplements, to inhibit pathogens (Page and Gautier, 2012; Dittoe et al., 2018; Stewart et al., 2020; Silveira et al., 2021). Historically, the most widely used type of antimicrobial compounds has been antibiotics, which can specifically inhibit bacteria and can also enhance animal growth performance in some cases (Dibner and Richards, 2005). However, growing attention to the rise of antibiotic-resistant bacteria (Nhung et al., 2017; Haulisah et al., 2021) has led to stricter regulations such as the Veterinary Feed Directive issued by the US Food and Drug Administration (FDA) and resulted in more judicious antibiotic use (Dillon and Jackson-Smith, 2021). Likewise, antiviral compounds such as the anti-influenza drug amantadine have been used as prophylactics to prevent viral outbreaks from spreading in livestock populations, but such widespread usage has been suggested to cause a rise in drug-resistant viral strains (Yuan et al., 2022). Additionally, more broadly acting, disinfecting compounds such as formaldehyde are used to inhibit both bacteria and viruses (Bleichert et al., 2014), but health concerns related to carcinogenicity (Swenberg et al., 2013; Andersen et al., 2019) have led to its ban for use in feedstuffs in certain jurisdictions (e.g., in the European Union, Japan, and Korea) and reduced use in general (Śmiechowska et al., 2021).
As such, there is extensive interest in exploring the use of naturally occurring antimicrobial compounds that can work against a wide range of bacteria and viruses, are safe to use, and do not elicit pathogen resistance. Considering these points, one ideal pathogen target is the lipid membrane that surrounds bacterial cells and most viruses that pose a threat to the livestock industry (Yoon et al., 2020a). Recent findings from the human antiviral medicine field demonstrate the performance merits of targeting pathogen membranes in physiological environments (Jackman et al., 2018; Jackman, 2022) and thus support the potential for translating such strategies to animal agriculture, especially if economical antimicrobial compounds with acceptable regulatory profiles can be utilized.
Towards this goal, natural antimicrobial lipids such as medium-chain monoglycerides (MCMG) have emerged as a promising option to tackle the virus and bacteria challenges facing the swine and poultry industries and are covered herein. Particular focus is placed on critically analyzing how MCMG are being utilized to support agricultural biosecurity and animal health in the swine and poultry production sectors. Notably, MCMG have had wide use and established regulatory acceptance (e.g., as a food substance, they are determined to be Generally Recognized as Safe by the US FDA, the European Commission, and the Food Chemicals Codex) as antimicrobial preservatives, lubricants, stabilizers, and emulsifiers in other aspects of food production (Luo et al., 2022), which reinforces their application potential in the present context. Ongoing research has explored how MCMG can be utilized for specific application needs such as virus mitigation in feed and supporting animal health during bacterial infection. Recent attention to the molecular-level properties of MCMG is also helping to determine structure-function relationships and to rationalize why certain MCMG are more potent than other ones.
Monoglyceride structure and functions
MCMG are derivatives of medium-chain fatty acids (MCFA), which are classified as saturated fatty acids with 6- to 12-carbon long, aliphatic chains. Structurally, MCMG are esterified adducts of an MCFA and a glycerol molecule and have nonionic headgroups, which enable pH-stable behavior that supports robust performance in aqueous environments (Figure 1A). By contrast, MCFA have a carboxylic acid headgroup that is typically negatively charged above pH 5 and its ionization state is sensitive to the pH environment (Valle-Gonzaílez et al., 2018). Owing to their distinct melting points, C6 and C8 monoglycerides are typically found in liquid form while C10 and C12 monoglycerides are typically in powder form. Of note, MCMG mixtures are often supplied in liquid form due to MCMG miscibility.
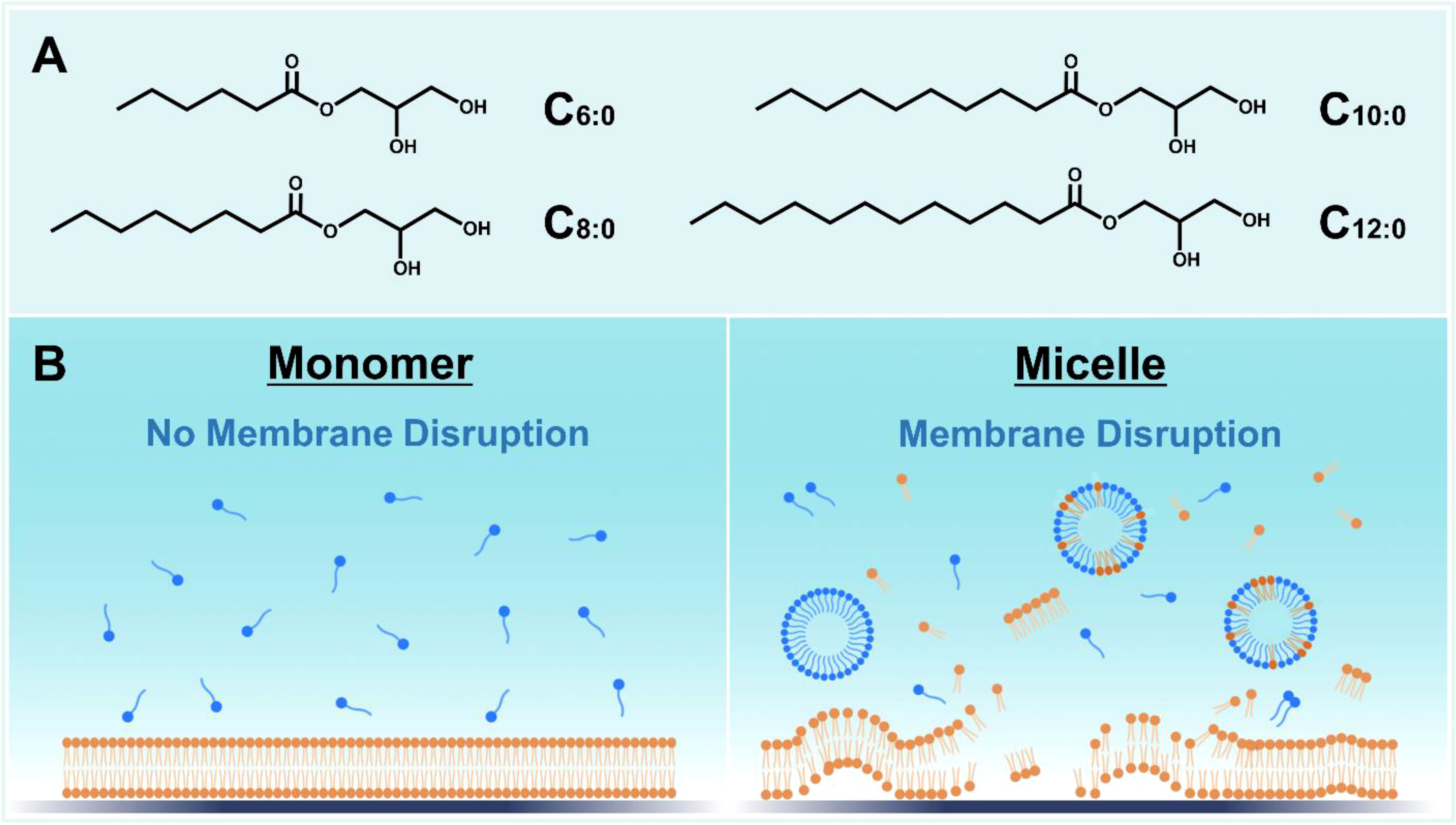
Figure 1 Overview of medium-chain monoglyceride (MCMG) structure and function. (A) Chemical structures of MCMG with saturated chains. (B) Schematic illustration depicting how MCMG in micellar form can disrupt phospholipid membranes such as those of bacteria and enveloped viruses while MCMG in monomeric form are largely inactive.
The main biologically active constructs of MCMG are micelles, which are self-assembled structures of individual molecules that form above a critical micelle concentration (CMC) and the CMC value varies depending on the particular compound or mixture thereof (Yoon et al., 2020b). In general, MCMG with longer hydrocarbon chains have lower CMC values than MCMG with shorter chains due to stronger hydrophobic interactions between chains that promote self-assembly (Yoon et al., 2017). The nonionic character of MCMG enables micelle formation at lower compound concentrations than MCFA, which have anionic character and hence intermolecular electrostatic repulsion between the headgroups that can hinder micelle formation at low concentrations. This biophysical feature helps to explain why MCMG are typically more potent than MCFA since MCMG form micelles at lower concentrations.
Mechanistically, MCMG can inhibit a wide range of membrane-enveloped viruses and bacteria by displaying membrane-disruptive properties, which often occur at and above the CMC (Figure 1B). Fatty acids and monoglycerides mainly exhibit membrane-disruptive properties in their micellar form above their corresponding CMC values (Yoon et al., 2015), which is therefore an important determinant of potency, while they are appreciably less active or inactive in monomeric form. In the case of bacteria, MCMG treatment can induce bacterial cell membrane disruption, which leads to abrogating cell growth or viability depending on the specific conditions (Bergsson et al., 1998; Bergsson et al., 2001). The mechanisms involved in this antibacterial activity can include increased membrane permeability and/or lysis and concomitant effects on membrane-related cellular functions such as hindered electron transport chains, oxidative phosphorylation processes, and enzyme activities (Yoon et al., 2018; Casillas-Vargas et al., 2021). Most of the latter effects are consequences of increased bacterial cell membrane permeability, i.e., membrane leakage that disrupts ion and chemical gradients between the extracellular and intracellular spaces. MCMG can also inhibit membrane-enveloped viruses by damaging virus particles, which hinders infectivity and it is understood that membrane lysis is the main contributing factor to antiviral activity (Thormar et al., 1987; Thormar et al., 1994).
MCMG have been reported to inhibit a wide range of viral and bacterial pathogens that are important to swine and poultry production (Jackman et al., 2020a; Chen et al., 2021). Susceptible bacterial pathogens include Escherichia coli, Streptococcus suis, Salmonella poona, Listeria monocytogenes, and Clostridium perfringens (Wang and Johnson, 1992; Skřivanová et al., 2006; Wang et al., 2018), and susceptible viral pathogens include membrane-enveloped viruses such as avian influenza virus (AIV), infectious bronchitis virus, Newcastle disease virus, African swine fever virus (ASFV), porcine reproductive respiratory syndrome virus (PRRSV), and porcine epidemic diarrhea virus (PEDV) (Nur Ika, 2011; Jackman et al., 2020b; Lerner et al., 2020; Dee et al., 2021; Nefedova et al., 2021; Saleh et al., 2021). A common theme of these pathogens is that they are all coated with a lipid membrane envelope, which is the primary target for pathogen inhibition. Mechanistic studies have revealed that C10 and C12 monoglycerides have particularly high antimicrobial potency due to low CMC values, which allow them to be in the active micellar form at lower concentrations than shorter-chain monoglycerides (Kabara et al., 1972). Nevertheless, different bacteria and viruses have distinct lipid compositions and membrane properties so empirical testing is warranted to identify the best-performing MCMG or combination thereof for the target pathogen(s).
Compared to antibiotics, there are also two main advantages of MCMG for dealing with infectious diseases in livestock production: (1) MCMG exhibit broad-spectrum antimicrobial activity to inhibit viruses and bacteria, whereas antibiotics only work against bacteria (Churchward et al., 2018); and (2) there is anticipated to be a high barrier for pathogens to develop resistance to MCMG (Schlievert and Peterson, 2012), which supports the feasibility of using them across various applications such as mitigation rather than only in more specific therapeutic contexts. As described above, MCMG are also more potent than MCFA and have a more acceptable safety profile than other options like formaldehyde.
Bioavailability considerations
In terms of supply options, MCMG are widely found in various natural sources such as coconut oil and insect-derived oils (Dayrit, 2015; Borrelli et al., 2021; Dabbou et al., 2021). However, they are mainly present in triglyceride form, which typically do not exhibit antimicrobial activity and require enzymatic breakdown to yield the active MCMG form (Zentek et al., 2011). In addition to enzymatic breakdown of triglycerides, MCMG themselves can be broken down into free fatty acids and glycerol products by lipolytic enzymes. For example, certain bacteria such as Staphylococcus aureus can produce enzymes to hydrolyze MCMG (i.e., glycerol monolaurate) and the resulting fatty acids can also exhibit similar biological activities by themselves (Ruzin and Novick, 2000) and in combination with MCMG (i.e., in mixed micelles).
The gastrointestinal tract also contains lipolytic enzymes that can degrade MCMG, while it has also been discussed how MCMG can be absorbed in intact form and the hydrolytic propensity of MCMG can also vary depending on the specific stereochemistry of its molecular structure (Kabara, 2005). Indeed, MCMG are also found intact in complex biological matrices such as the milk of some mammals (Schlievert et al., 2019), and can alternatively be provided in highly purified form. The latter option is useful to incorporate a defined amount of MCMG into an aqueous or feed matrix, especially when antimicrobial activity is desired in the matrix itself. At present, feed delivery is the most widely used method for MCMG and we cover application examples of particular importance to dealing with microbial pathogens in swine and poultry production.
Application studies
Prevention of virus transmission in swine feed
Feed has been identified as a vector for pathogen transmission in swine production and there is ongoing exploration of chemical mitigants to stop feed transmission of viruses and bacteria (Niederwerder, 2021). One longstanding option has been formaldehyde, which can induce protein cross-linking to inhibit pathogens (Wilton et al., 2014; Dee et al., 2015). However, its use is being reduced or stopped in certain parts of the world due to recently enacted regulations as described above (Gosling et al., 2021). As such, there has been ongoing exploration of new classes of regulatory acceptable mitigants and one of the most promising options is membrane-disrupting MCFA (Baltić et al., 2017). In light of the typically greater biological potency of MCMG compared to MCFA and the demonstrated ability of MCFA to mitigate pathogens in feed, there have been several recent investigations exploring how MCMG might be useful to inhibit swine-related pathogens (Table 1).
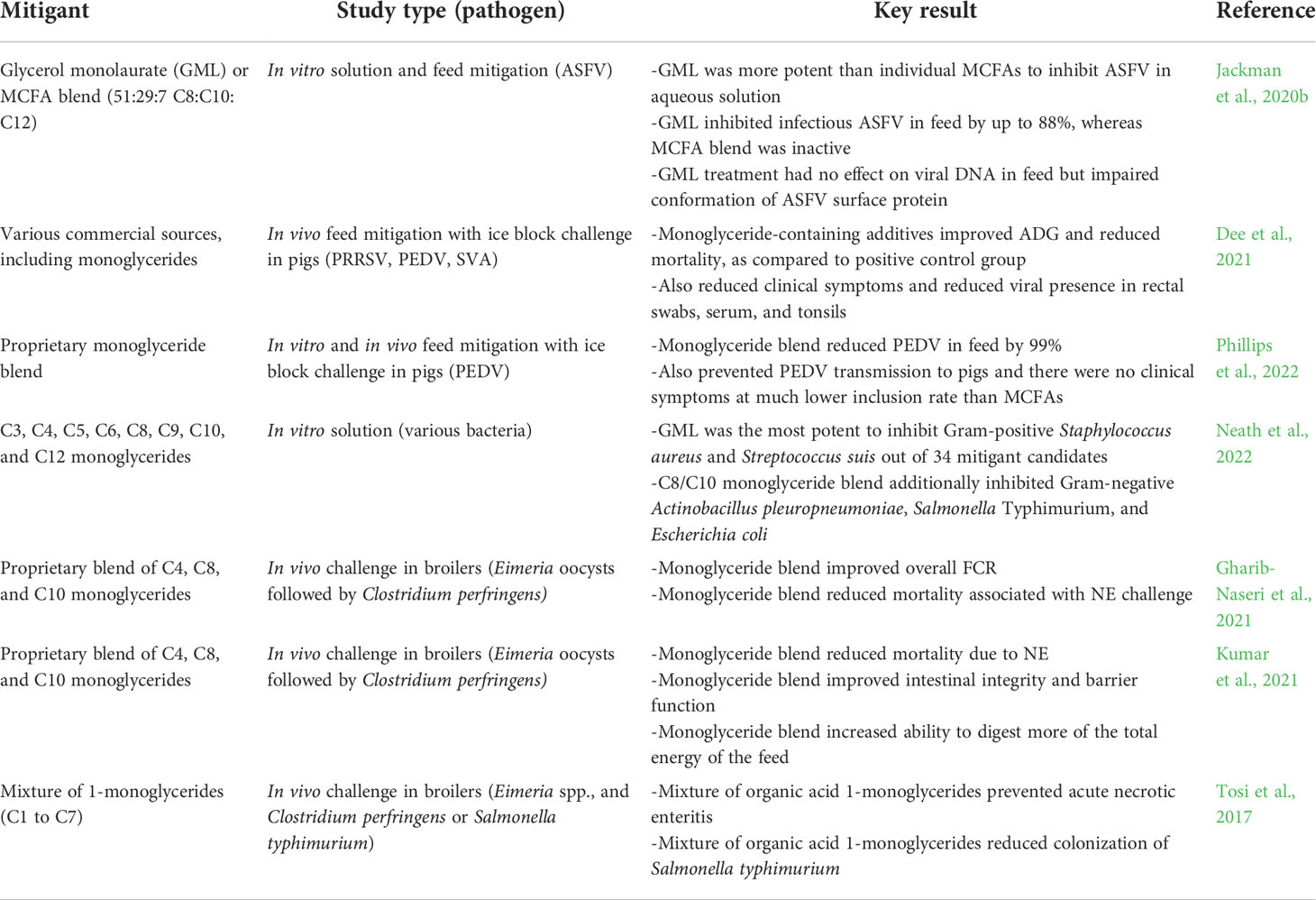
Table 1 Recent examples of monoglycerides demonstrating in vitro and in vivo efficacy to inhibit swine- and poultry-related viral and bacterial pathogens.
In one study, the in vitro antiviral activity of GML monoglyceride against ASFV was compared to that of several individual MCFA in aqueous solution (Jackman et al., 2020b). It was observed that GML had the greatest antiviral potency and exhibited virucidal activity along with additional antiviral mechanisms. Practically, it was further demonstrated that GML could inhibit ASFV in a feed matrix, as indicated by a dose-dependent drop in viral infectivity, and the membrane-disrupting mechanism also caused changes in the conformational properties of membrane-associated viral surface proteins. Specifically, the conformational stability of viral surface proteins depends on membrane integrity and membrane disruption therefore causes the loss of native protein conformation, impairing structure and potentially function as well (Salimi et al., 2020). Of note, the antiviral activity of GML did not cause a drop in viral nucleic acids found in the feed (i.e., located in the core of ASFV particles), highlighting that GML can impair viral infectivity even when viral nucleic acids are still present. Together, these findings support that GML impaired viral infectivity by disrupting the lipid membrane envelope surrounding ASFV particles within the feed matrix, indicating that this MCMG can directly inhibit enveloped virus particles.
There has also been interest in developing swine models to evaluate the potential of MCMG-containing mitigant products to inhibit pathogen transmission. One popular model is based on an ‘ice-block’ challenge, whereby feed is inoculated with a frozen ice sample containing PRRSV, Senecavirus A (SVA), and PEDV that melts and disperses to contaminate the feed (Dee et al., 2021). Pigs are then fed the contaminated feed and biomarkers related to growth performance, clinical status, and virological infection signs are tracked over subsequent days as pigs respond to the pathogen exposure. The feed can be premixed with a mitigant candidate so that the effects of a mitigant on preventing disease transmission are evaluated, i.e., it is envisioned that the mitigant inactivates the virus present within the feed matrix. Using this approach, several MCMG-containing mitigant products were shown to prevent disease transmission, as indicated by preventing infection-related mortality and reducing clinical symptoms. Sufficiently high inclusion rates of the MCMG-containing products in the feed also reduced viral presence in rectal swabs, serum, and tonsils, and also improved average daily gain (ADG).
Additional efforts have focused on understanding the range of effective inclusion rates of MCMG-containing products compared to that of formaldehyde-containing products, which is around 3.25 kg per ton in the latter case (Phillips et al., 2022). In vitro studies showed that 1.5 to 3.5 kg per ton inclusion rates of an MCMG-containing product inhibited PEDV infectivity in feed while further testing in the ice-block challenge model verified that these inclusion rates also effectively prevented disease transmission to pigs. On the other hand, an MCFA-containing product tested in parallel was only effective at an inclusion rate of 10 kg per ton. The higher effectiveness of the MCMG-containing product was rationalized by taking into account how MCMG is typically more potent than MCFA at a molecular level, and the data reinforced that MCMG is a potentially useful mitigant option to replace formaldehyde in light of similarly low effective inclusion rates.
While most recent MCMG mitigant studies have focused on preventing viral disease transmission in pigs, there is also potential to explore whether such mitigants can prevent bacterial disease transmission as well. For example, GML has been reported to most potently inhibit Gram-positive bacteria such as Staphylococcus aureus and Streptococcus suis out of over 30 mitigant candidates while a blend of 8- and 10-carbon long, saturated monoglycerides additionally inhibited Gram-negative bacteria such as Actinobacillus pleuropneumoniae, Salmonella typhimurium, and Escherichia coli (Neath et al., 2022). This knowledge can promote the development of improved MCMG-containing mitigant products that might be tailored for broad-spectrum antimicrobial activity or for more specific mitigation needs depending on the application context.
Mitigation of necrotic enteritis infection in poultry
Necrotic enteritis (NE) is a severe poultry disease that is caused by Clostridium perfringens, which is a Gram-positive bacteria (Timbermont et al., 2011). Necrotic enteritis is characterized by gross lesions in the jejunum and ileum of the small intestine as well as a sudden increase in mortality in two- to five-week-old broilers. Traditionally, NE was controlled by the use of antibiotics in feed. However, today, the decrease in use of antibiotics along with the increased use of coccidiosis (a predisposing factor to the disease) vaccines, has resulted in an increased incidence of NE (Adhikari et al., 2020). Thus, to combat the disease, the poultry industry is evaluating and utilizing non-antibiotic alternatives such as MCMG and related antimicrobial lipids that can inhibit the bacteria and curb the disease (Gomez-Osorio et al., 2021), as summarized in Table 1.
To evaluate the effectiveness of a short- and medium-chain monoglyceride blend to control NE, Gharib-Naseri et al. orally challenged broilers with Eimeria oocysts at nine days of age followed by inoculation with Clostridium perfringens at around 14-15 days of age (Gharib-Naseri et al., 2021). Broilers fed the monoglyceride blend had a numerically lower mortality rate than broilers that were not fed the blend. Also, from 0 to 35 days of age, challenged broilers fed the monoglyceride blend had a better feed conversion ratio (FCR). Therefore, the monoglyceride blend was able to alleviate some of the negative effects of NE. Additionally, in the trial, the monoglyceride blend was fed to broilers at two different inclusion rates (0.3% in starter and 0.2% in grower and finisher phases, or 0.3% in starter, 0.15% in grower, and 0.075% in finisher phases). Feed conversion was similar for challenged broilers fed either inclusion rate of the monoglyceride blend. However, the mortality rate tended to be lower for challenged broilers fed the higher level of the monoglyceride blend in the grower and finisher phases. Since all of the mortality occurred within the first four days following the challenge at 14 days of age, it is possible that the lower inclusion rate was not adequate. Thus, there is interest in determining the optimal rate of inclusion and ensuring a sufficiently high level to control NE. Further analysis indicated that the monoglyceride blend improved cecal microbiome diversity, eliciting positive effects on healthy bacteria such as Bacillus species that are associated with growth performance and improved feed efficiency (Gharib-Naseri et al., 2021). These findings support that the monoglyceride blend may have enhanced immune function through microbiome modulation while interestingly the cecal level of C. perfringens was not affected.
In another study with a similar Eimeria spp. and C. perfringens challenge model, feeding broilers the monoglyceride blend only in the starter phase (0 to 10 days of age), at a rate of 0.5% of the diet, significantly reduced NE-related mortality compared to broilers that were not fed the monoglyceride blend (Kumar et al., 2021). In addition, feeding the monoglyceride blend to broilers in the starter phase increased the messenger RNA (mRNA) levels of jejunal genes related to tight junction protein (TJP1) and immunoglobulin G (IgG), which are important proteins that support gut integrity and immune health, respectively. During NE, the intestinal epithelium is damaged, resulting in a reduced immune response and reduced rate of nutrient absorption across the intestinal wall. Since challenged broilers fed the monoglyceride blend had upregulated TJP1 and IgG levels, this indicates that intestinal integrity and intestinal barrier function of broilers with NE disease can be improved by including the monoglyceride blend in the feed. Furthermore, this improved intestinal health resulted in increased rates of digestion and nutrient absorption. The latter was evidenced by the reported increase in digestion of the total energy in the diet of challenged broilers that were fed the monoglyceride blend. As described in the previous example, the cecal levels of C. perfringens in the treated group were not reduced compared to the control group, suggesting that the monoglyceride blend mainly exerted positive health effects by supporting immune functions, such as increased expression of jejunal tight junction and immunoglobulin genes associated with gut barrier functions, that may have counteracted the initial Eimeria spp. challenge prior to C. perfringens challenge (Kumar et al., 2021).
While alleviating the negative effects of NE is very important, means of preventing NE are also necessary for the poultry industry. Towards this goal, Tosi et al. reported that a specific mixture of short- and medium-chain monoglycerides, provided in feed at a rate of 0.5% from day 1 to 10 and at a rate of 0.025% from day 11 to 21, prevented NE (Tosi et al., 2017). Also, the specific mixture provided in the feed at a rate of 0.3% from day 1 to 34 reduced Salmonella colonization in broilers. Collectively, these data support the efficacy of MCMG within complex formulations to inhibit NE-related bacterial infections in poultry. Thus far, the mixtures used have been dry formulations and it would be desirable to further develop and test MCMG mixtures that can be supplied in liquid form.
Microbiome modulation
Another emerging application area for MCMG is microbiome modulation, which can support animal health and help to overcome viral and bacterial infections. Due to antimicrobial functions, MCMG have been shown to modulate the gut microbiome of swine and poultry by promoting the growth of healthy bacteria and helping to decrease the relative amounts of pathogenic bacteria. In the swine context, the addition of 1000 mg/kg GML to the daily diet of weaned piglets reduced the rate of diarrhea and led to marked alterations in gut microbiota (Li et al., 2022). Microbiome analysis of cecal contents indicated that GML promoted increased levels of healthy bacteria such as Firmicutes, Lactobacillus, and Blautia species while reducing the proportion of Bacteroidota and Campilobacterota species that are associated with various medical disorders.
There have also been numerous recent studies demonstrating that MCMG can modulate the gut microbiome in poultry as well. For example, a mixture of GML and C10 monoglyceride was added to the diet of laying hens at a dose of 300 mg/kg and caused a marked decrease in the cecal prevalence of the phylum Proteobacteria, which is associated with poor gut health, and increased levels of various healthy bacteria (Liu et al., 2020). Different doses (300-600 mg/kg) of the same MCMG mixture were incorporated into the diet of broiler chicks and increased the cecal prevalence of bacteria such as Bifidobacteriaceae and Bacteroides, the latter of which play important roles in gut metabolism and helps to protect against pathogenic microbes (Liu et al., 2022). Similarly, various doses (300-1200 mg/kg) of GML alone have also been added to the diets of broiler chicks and led to improved diversity of the cecal microbiome as well as increased levels of Bacteroides (Kong et al., 2021). GML supplementation at a dose of 1200 mg/kg has also been shown to protect against immune stress and intestinal injury in liposaccharide-challenged broilers (Kong et al., 2022). Notably, these positive health effects were correlated with increased abundance of healthy gut bacteria involved in anti-inflammatory and antioxidant processes, and indicate that MCMG supplementation can modulate the gut microbiome to support animal health in addition to directly inhibiting pathogenic viruses and bacteria.
Future opportunities
The documented use of MCMG in recent research studies has demonstrated that they are effective in reducing viral infectivity in swine feed and in controlling bacterial-related infections in poultry. These application successes have been enabled by understanding how MCMG operate at a molecular level and rationalizing why they are typically more potent than MCFA, which has translated into lower molar concentrations and inclusion rates. Moving forward, one of the areas of greatest opportunity lies in expanding the scope of MCMG-related studies to develop optimized mixtures in terms of not only antimicrobial efficacy but also in terms of controlling formulation properties, i.e., powder or liquid supply, stability, and solubility. Current application uses have focused on incorporating MCMG into feed and developing water-miscible MCMG formulations for drinking water applications would also be advantageous, especially to rapidly respond to potential disease outbreaks. Such approaches might take advantage of recent innovations in lipid nanostructures and could also pave the way to developing aerosol formulations.
In terms of application scope, most studies have focused on preventing and treating viral infections while a renewed focus on mitigating disease transmission in livestock populations would be advantageous, especially to address some of the most pressing industry challenges. Since MCMG are broad-spectrum antimicrobial agents that target the lipid membrane surrounding bacteria and enveloped viruses, they also stand excellent potential to be readily deployed against evolving pathogen threats in the future and hence should be mainstays of the livestock industry for years to come. These findings also support that MCMG are active in vivo—an area that needs further exploration in the context of disease challenges in order to further understand how and where MCMG function. In the context of pathogen feed mitigation, it is also important to determine the extent to which MCMG inactivate pathogens in the feed matrix itself vs. in saliva upon ingestion. Altogether, there is excellent potential to continue exploring the use of MCMG to stop pathogenic viruses and bacteria while also building a more collective picture of how they modulate microbiome populations and of the interplay between these different functionalities to optimize practical utilization of MCMG in animal agriculture.
Author contributions
JJ and CE conceived and designed the study. JJ, TL, and CE performed the literature review. JJ, TL, and CE analyzed the data and wrote the manuscript. All authors contributed to the article and approved the submitted version.
Funding
This work was partially supported by the SKKU Global Research Platform Research Fund, Sungkyunkwan University, 2022.
Acknowledgments
Schematic illustrations were created with BioRender.com under an academic lab subscription.
Conflict of interest
TL and CE are employed by and JJ serves as a board member to the company Natural Biologics Inc.
Publisher’s note
All claims expressed in this article are solely those of the authors and do not necessarily represent those of their affiliated organizations, or those of the publisher, the editors and the reviewers. Any product that may be evaluated in this article, or claim that may be made by its manufacturer, is not guaranteed or endorsed by the publisher.
References
Adhikari P., Kiess A., Adhikari R., Jha R. (2020). An approach to alternative strategies to control avian coccidiosis and necrotic enteritis. J. Appl. Poult. Res. 29, 515–534. doi: 10.1016/j.japr.2019.11.005
Andersen M. E., Gentry P. R., Swenberg J. A., Mundt K. A., White K. W., Thompson C., et al. (2019). Considerations for refining the risk assessment process for formaldehyde: results from an interdisciplinary workshop. Regul. Toxicol. Pharmacol. 106, 210–223. doi: 10.1016/j.yrtph.2019.04.015
Baltić B., Starčević M., Đorđević J., Mrdović B., Marković R. (2017). “Importance of medium chain fatty acids in animal nutrition,” in IOP conf. ser.: Earth environ. Sci (Zlatibor, Serbia: IOP Publishing). doi: 10.1088/1755-1315/85/1/012048
Bergsson G., Arnfinnsson J., Karlsson S. M., Steingrímsson Ó., Thormar H. (1998). In vitro inactivation of chlamydia trachomatis by fatty acids and monoglycerides. Antimicrob. Agents Chemother. 42, 2290–2294. doi: 10.1128/AAC.42.9.2290
Bergsson G., Arnfinnsson J., Steingrímsson Ó., Thormar H. (2001). Killing of gram-positive cocci by fatty acids and monoglycerides. APMIS 109, 670–678. doi: 10.1034/j.1600-0463.2001.d01-131.x
Bleichert P., Espírito Santo C., Hanczaruk M., Meyer H., Grass G. (2014). Inactivation of bacterial and viral biothreat agents on metallic copper surfaces. Biometals 27, 1179–1189. doi: 10.1007/s10534-014-9781-0
Borrelli L., Varriale L., Dipineto L., Pace A., Menna L. F., Fioretti A. (2021). Insect derived lauric acid as promising alternative strategy to antibiotics in the antimicrobial resistance scenario. Front. Microbiol. 12. doi: 10.3389/fmicb.2021.620798
Casillas-Vargas G., Ocasio-Malavé C., Medina S., Morales-Guzmán C., Del Valle R. G., Carballeira N. M., et al. (2021). Antibacterial fatty acids: An update of possible mechanisms of action and implications in the development of the next-generation of antibacterial agents. Prog. Lipid Res. 82, 101093. doi: 10.1016/j.plipres.2021.101093
Chen S., Zhang L., Wang L., Ouyang H., Ren L. (2021). Viruses from poultry and livestock pose continuous threats to human beings. Proc. Natl. Acad. Sci. U. S. A. 118, e2022344118. doi: 10.1073/pnas.2022344118
Churchward C. P., Alany R. G., Snyder L. A. (2018). Alternative antimicrobials: The properties of fatty acids and monoglycerides. Crit. Rev. Microbiol. 44, 561–570. doi: 10.1080/1040841X.2018.1467875
Dabbou S., Lauwaerts A., Ferrocino I., Biasato I., Sirri F., Zampiga M., et al. (2021). Modified black soldier fly larva fat in broiler diet: Effects on performance, carcass traits, blood parameters, histomorphological features and gut microbiota. Animals 11, 1837. doi: 10.3390/ani11061837
Dayrit F. M. (2015). The properties of lauric acid and their significance in coconut oil. J. Am. Oil Chem. Soc 92, 1–15. doi: 10.1007/s11746-014-2562-7
Dee S., Neill C., Clement T., Singrey A., Christopher-Hennings J., Nelson E. (2015). An evaluation of porcine epidemic diarrhea virus survival in individual feed ingredients in the presence or absence of a liquid antimicrobial. Porc. Health Manage. 1, 1–10. doi: 10.1186/s40813-015-0003-0
Dee S. A., Niederwerder M. C., Edler R., Hanson D., Singrey A., Cochrane R., et al. (2021). An evaluation of additives for mitigating the risk of virus-contaminated feed using an ice-block challenge model. Transboundary. Emerging. Dis. 68, 833–845. doi: 10.1111/tbed.13749
Dibner J., Richards J. (2005). Antibiotic growth promoters in agriculture: history and mode of action. Poult. Sci. 84, 634–643. doi: 10.1093/ps/84.4.634
Dillon M. E., Jackson-Smith D. (2021). Impact of the veterinary feed directive on Ohio cattle operations. PloS One 16, e0255911. doi: 10.1371/journal.pone.0255911
Dittoe D. K., Ricke S. C., Kiess A. S. (2018). Organic acids and potential for modifying the avian gastrointestinal tract and reducing pathogens and disease. Front. Vet. Sci. 5. doi: 10.3389/fvets.2018.00216
Gharib-Naseri K., Kheravii S. K., Li L., Wu S.-B. (2021). Buffered formic acid and a monoglyceride blend coordinately alleviate subclinical necrotic enteritis impact in broiler chickens. Poult. Sci. 100, 101214. doi: 10.1016/j.psj.2021.101214
Gomez-Osorio L.-M., Yepes-Medina V., Ballou A., Parini M., Angel R. (2021). Short and medium chain fatty acids and their derivatives as a natural strategy in the control of necrotic enteritis and microbial homeostasis in broiler chickens. Front. Vet. Sci. 8. doi: 10.3389/fvets.2021.773372
Gosling R. J., Mawhinney I., Richardson K., Wales A., Davies R. (2021). Control of salmonella and pathogenic e. coli contamination of animal feed using alternatives to formaldehyde-based treatments. Microorganisms 9, 263. doi: 10.3390/microorganisms9020263
Haulisah N. A., Hassan L., Bejo S. K., Jajere S. M., Ahmad N. I. (2021). High levels of antibiotic resistance in isolates from diseased livestock. Front. Vet. Sci. 8. doi: 10.3389/fvets.2021.652351
Jackman J. A. (2022). Antiviral peptide engineering for targeting membrane-enveloped viruses: Recent progress and future directions. Biochim. Biophys. Acta. Biomembr. 1864, 183821. doi: 10.1016/j.bbamem.2021.183821
Jackman J. A., Boyd R. D., Elrod C. C. (2020a). Medium-chain fatty acids and monoglycerides as feed additives for pig production: towards gut health improvement and feed pathogen mitigation. J. Anim. Sci. Biotechnol. 11, 1–15. doi: 10.1186/s40104-020-00446-1
Jackman J. A., Hakobyan A., Zakaryan H., Elrod C. C. (2020b). Inhibition of African swine fever virus in liquid and feed by medium-chain fatty acids and glycerol monolaurate. J. Anim. Sci. Biotechnol. 11, 1–10. doi: 10.1186/s40104-020-00517-3
Jackman J. A., Shi P.-Y., Cho N.-J. (2018). Targeting the Achilles heel of mosquito-borne viruses for antiviral therapy. ACS Infect. Dis. 5, 4–8. doi: 10.1021/acsinfecdis.8b00286
Kabara J. J., Swieczkowski D. M., Conley A. J., Truant J. P. (1972). Fatty acids and derivatives as antimicrobial agents. Antimicrob. Agents Chemother. 2, 23–28.
Kabara J. (2005). Pharmacological effects of coconut oil vs. monoglycerides. INFORM-Internat. News Fats. Oils. Related. Mater. 16, 386–387.
Kong L., Wang Z., Xiao C., Zhu Q., Song Z. (2021). Glycerol monolaurate ameliorated intestinal barrier and immunity in broilers by regulating intestinal inflammation, antioxidant balance, and intestinal microbiota. Front. Immunol. 12. doi: 10.3389/fimmu.2021.713485
Kong L., Wang Z., Xiao C., Zhu Q., Song Z. (2022). Glycerol monolaurate attenuated immunological stress and intestinal mucosal injury by regulating the gut microbiota and activating AMPK/Nrf2 signaling pathway in lipopolysaccharide-challenged broilers. Anim. Nutr. 10, 347–359. doi: 10.1016/j.aninu.2022.06.005
Kumar A., Kheravii S. K., Li L., Wu S.-B. (2021). Monoglyceride blend reduces mortality, improves nutrient digestibility, and intestinal health in broilers subjected to clinical necrotic enteritis challenge. Animals 11, 1432. doi: 10.3390/ani11051432
Lerner A. B., Cochrane R. A., Gebhardt J. T., Dritz S. S., Jones C. K., Derouchey J. M., et al. (2020). Effects of medium chain fatty acids as a mitigation or prevention strategy against porcine epidemic diarrhea virus in swine feed. J. Anim. Sci. 98, skaa159. doi: 10.1093/jas/skaa159
Liu T., Guo L., Zhangying Y., Ruan S., Liu W., Zhang X., et al. (2022). Dietary medium-chain 1-monoglycerides modulates the community and function of cecal microbiota of broilers. J. Sci. Food Agric. 102, 2242–2252. doi: 10.1002/jsfa.11562
Liu T., Tang J., Feng F. (2020). Medium-chain α-monoglycerides improves productive performance and egg quality in aged hens associated with gut microbiota modulation. Poult. Sci. 99, 7122–7132. doi: 10.1016/j.psj.2020.07.049
Li L., Wang H., Zhang N., Zhang T., Ma Y. (2022). Effects of α-glycerol monolaurate on intestinal morphology, nutrient digestibility, serum profiles, and gut microbiota in weaned piglets. J. Anim. Sci. 100, skac046. doi: 10.1093/jas/skac046
Luo X., Liu W., Zhao M., Huang Y., Feng F. (2022). Glycerol monolaurate beyond an emulsifier: synthesis, in vivo fate, food quality benefits and health efficacies. Trends Food Sci. Technol 127, 291–302. doi: 10.1016/j.tifs.2022.05.017
Neath C., Portocarero N., Jones C. (2022). In vitro susceptibility of swine pathogens to feed additives and active ingredients with potential as antibiotic replacements. J. Appl. Microbiol. 132, 1713–1723. doi: 10.1111/jam.15318
Nefedova E., Koptev V., Bobikova A. S., Cherepushkina V., Mironova T., Afonyushkin V., et al. (2021). The infectious bronchitis coronavirus pneumonia model presenting a novel insight for the SARS-CoV-2 dissemination route. Vet. Sci. 8, 239. doi: 10.3390/vetsci8100239
Nhung N. T., Chansiripornchai N., Carrique-Mas J. J. (2017). Antimicrobial resistance in bacterial poultry pathogens: A review. Front. Vet. Sci. 4. doi: 10.3389/fvets.2017.00126
Niederwerder M. C. (2021). Risk and mitigation of African swine fever virus in feed. Animals 11, 792. doi: 10.3390/ani11030792
Nur Ika H. (2011). Avian influenza virus inactivation by caprylic acid, sodium caprylate, and monocaprylin. Health Sci. J. Indones. 2, 41–45. doi: 10.22435/hsji.v2i1Apr.62.41-45
Page S., Gautier P. (2012). Use of antimicrobial agents in livestock. Rev. Sci. Tech. 31, 145. doi: 10.20506/rst.31.1.2106
Phillips F. C., Rubach J. K., Poss M. J., Anam S., Goyal S. M., Dee S. A. (2022). Monoglyceride reduces viability of porcine epidemic diarrhoea virus in feed and prevents disease transmission to post-weaned piglets. Transboundary. Emerging. Dis. 69, 121–127. doi: 10.1111/tbed.14353
Ruzin A., Novick R. P. (2000). Equivalence of lauric acid and glycerol monolaurate as inhibitors of signal transduction in Staphylococcus aureus. J. Bacteriol. 182, 2668–2671. doi: 10.1128/jb.182.9.2668-2671.2000
Saleh A. A., El-Gharabawy B., Hassan A., Badawi N., Eid Y., Selim S., et al. (2021). Effect of dietary inclusion of alpha-monolaurin on the growth performance, lipid peroxidation, and immunity response in broilers. Sustainability 13, 5231. doi: 10.3390/su13095231
Salimi H., Jackson J., Flores M. G., Zhang M. S., O’Malley Y., Houtman J. C., et al. (2020). The lipid membrane of HIV-1 stabilizes the viral envelope glycoproteins and modulates their sensitivity to antibody neutralization. J. Biol. Chem. 295, 348–362. doi: 10.1074/jbc.RA119.009481
Schlievert P. M., Kilgore S. H., Seo K. S., Leung D. Y. (2019). Glycerol monolaurate contributes to the antimicrobial and anti-inflammatory activity of human milk. Sci. Rep. 9, 1–9. doi: 10.1038/s41598-019-51130-y
Schlievert P. M., Peterson M. L. (2012). Glycerol monolaurate antibacterial activity in broth and biofilm cultures. PloS One 7, e40350. doi: 10.1371/journal.pone.0040350
Shurson G. C., Urriola P. E., Van De Ligt J. L. (2022). Can we effectively manage parasites, prions, and pathogens in the global feed industry to achieve one health? Transboundary. Emerging. Dis. 69, 4–30. doi: 10.1111/tbed.14205
Silveira R. F., Roque-Borda C. A., Vicente E. F. (2021). Antimicrobial peptides as a feed additive alternative to animal production, food safety and public health implications: an overview. Anim. Nutr. 7, 896–904. doi: 10.1016/j.aninu.2021.01.004
Skřivanová E., Marounek M., Benda V., Březina P. (2006). Susceptibility of Escherichia coli, Salmonella sp. and Clostridium perfringens to organic acids and monolaurin. Vet. Med. 51, 81–88. doi: 10.17221/5524-VETMED
Śmiechowska M., Newerli-Guz J., Skotnicka M. (2021). Spices and seasoning mixes in European union–innovations and ensuring safety. Foods 10, 2289. doi: 10.3390/foods10102289
Stewart S. C., Dritz S. S., Woodworth J. C., Paulk C., Jones C. K. (2020). A review of strategies to impact swine feed biosecurity. Anim. Health Res. Rev. 21, 61–68. doi: 10.1017/S146625231900015X
Swenberg J. A., Moeller B. C., Lu K., Rager J. E., Fry R. C., Starr T. B. (2013). Formaldehyde carcinogenicity research: 30 years and counting for mode of action, epidemiology, and cancer risk assessment. Toxicol. Pathol. 41, 181–189. doi: 10.1177/0192623312466459
Thormar H., Isaacs C. E., Brown H. R., Barshatzky M. R., Pessolano T. (1987). Inactivation of enveloped viruses and killing of cells by fatty acids and monoglycerides. Antimicrob. Agents Chemother. 31, 27–31. doi: 10.1128/AAC.31.1.27
Thormar H., Isaacs C. E., Kim K., Brown H. R. (1994). Inactivation of visna virus and other enveloped viruses by free fatty acids and monoglycerides. Ann. N. Y. Acad. Sci. 724, 465–471. doi: 10.1111/j.1749-6632.1994.tb38948.x
Timbermont L., Haesebrouck F., Ducatelle R., Van Immerseel F. (2011). Necrotic enteritis in broilers: an updated review on the pathogenesis. Avian Pathol. 40, 341–347. doi: 10.1080/03079457.2011.590967
Tosi G., Fiorentini L., Massi P., Paoli A., Parini M. (2017). “Effect of 1-monoglycerides of organic acid in controlling clostridium perfringens and salmonella typhimurium in experimentally infected broiler chickens,” in 4th international poultry meat congress, 26-30 April 2017 (Antalya, Turkey: Beyaz et sanayicileri ve damızlıkçıları birligi dernegi (BESD-BIR)) 117–123.
Valle-Gonzaílez E. R., Jackman J. A., Yoon B. K., Park S., Sut T. N., Cho N.-J. (2018). Characterizing how acidic pH conditions affect the membrane-disruptive activities of lauric acid and glycerol monolaurate. Langmuir 34, 13745–13753. doi: 10.1021/acs.langmuir.8b02536
Vanderwaal K., Deen J. (2018). Global trends in infectious diseases of swine. Proc. Natl. Acad. Sci. U.S.A. 115, 11495–11500. doi: 10.1073/pnas.1806068115
Wang L. L., Johnson E. A. (1992). Inhibition of Listeria monocytogenes by fatty acids and monoglycerides. Appl. Environ. Microbiol. 58, 624–629. doi: 10.1128/aem.58.2.624-629.1992
Wang J., Ma M., Yang J., Chen L., Yu P., Wang J., et al. (2018). In vitro antibacterial activity and mechanism of monocaprylin against Escherichia coli and Staphylococcus aureus. J. Food Prot. 81, 1988–1996. doi: 10.4315/0362-028X.JFP-18-248
Wilton T., Dunn G., Eastwood D., Minor P. D., Martin J. (2014). Effect of formaldehyde inactivation on poliovirus. J. Virol. 88, 11955–11964. doi: 10.1128/JVI.01809-14
Yoon B. K., Jackman J. A., Kim M. C., Cho N.-J. (2015). Spectrum of membrane morphological responses to antibacterial fatty acids and related surfactants. Langmuir 31, 10223–10232. doi: 10.1021/acs.langmuir.5b02088
Yoon B. K., Jackman J. A., Kim M. C., Sut T. N., Cho N.-J. (2017). Correlating membrane morphological responses with micellar aggregation behavior of capric acid and monocaprin. Langmuir 33, 2750–2759. doi: 10.1021/acs.langmuir.6b03944
Yoon B. K., Jackman J. A., Valle-González E. R., Cho N.-J. (2018). Antibacterial free fatty acids and monoglycerides: Biological activities, experimental testing, and therapeutic applications. Int. J. Mol. Sci. 19, 1114. doi: 10.3390/ijms19041114
Yoon B. K., Jeon W.-Y., Sut T. N., Cho N.-J., Jackman J. A. (2020a). Stopping membrane-enveloped viruses with nanotechnology strategies: Toward antiviral drug development and pandemic preparedness. ACS Nano. 15, 125–148. doi: 10.1021/acsnano.0c07489
Yoon B. K., Park S., Ma G. J., Kolahdouzan K., Zhdanov V. P., Jackman J. A., et al. (2020b). Competing interactions of fatty acids and monoglycerides trigger synergistic phospholipid membrane remodeling. J. Phys. Chem. Lett. 11, 4951–4957. doi: 10.1021/acs.jpclett.0c01138
Yuan S., Jiang S. C., Zhang Z. W., Fu Y. F., Zhu F., Li Z. L., et al. (2022). Abuse of amantadine in poultry may be associated with higher fatality rate of H5N1 infections in humans. J. Med. Virol. 94, 2588–2597. doi: 10.1002/jmv.27664
Keywords: monoglycerides, antimicrobial, antiviral, swine, poultry, pathogen, virus, bacteria
Citation: Jackman JA, Lavergne TA and Elrod CC (2022) Antimicrobial monoglycerides for swine and poultry applications. Front. Anim. Sci. 3:1019320. doi: 10.3389/fanim.2022.1019320
Received: 15 August 2022; Accepted: 13 October 2022;
Published: 28 October 2022.
Edited by:
Johan Osorio, Virginia Tech, United StatesCopyright © 2022 Jackman, Lavergne and Elrod. This is an open-access article distributed under the terms of the Creative Commons Attribution License (CC BY). The use, distribution or reproduction in other forums is permitted, provided the original author(s) and the copyright owner(s) are credited and that the original publication in this journal is cited, in accordance with accepted academic practice. No use, distribution or reproduction is permitted which does not comply with these terms.
*Correspondence: Joshua A. Jackman, amphY2ttYW5Ac2trdS5lZHU=; Charles C. Elrod, Y2NlMUBjb3JuZWxsLmVkdQ==