- Stress Physiology Laboratory, Department of Animal Science, University of Nebraska-Lincoln, Lincoln, NE, United States
Maternofetal stress induces fetal programming that restricts skeletal muscle growth capacity and metabolic function, resulting in intrauterine growth restriction (IUGR) of the fetus. This thrifty phenotype aids fetal survival but also yields reduced muscle mass and metabolic dysfunction after birth. Consequently, IUGR-born individuals are at greater lifelong risk for metabolic disorders that reduce quality of life. In livestock, IUGR-born animals exhibit poor growth efficiency and body composition, making these animals more costly and less valuable. Specifically, IUGR-associated programming causes a greater propensity for fat deposition and a reduced capacity for muscle accretion. This, combined with metabolic inefficiency, means that these animals produce less lean meat from greater feed input, require more time on feed to reach market weight, and produce carcasses that are of less quality. Despite the health and economic implications of IUGR pathologies in humans and food animals, knowledge regarding their specific underlying mechanisms is lacking. However, recent data indicate that adaptive programing of adrenergic sensitivity in multiple tissues is a contributing factor in a number of IUGR pathologies including reduced muscle mass, peripheral insulin resistance, and impaired glucose metabolism. This review highlights the findings that support the role for adrenergic programming and how it relates to the lifelong consequences of IUGR, as well as how dysfunctional adrenergic signaling pathways might be effective targets for improving outcomes in IUGR-born offspring.
Introduction
Intrauterine growth restriction (IUGR) is the result of fetal developmental programming aimed at increasing the chances of surviving poor intrauterine conditions by promoting thrifty growth and metabolism. Unfortunately, these same developmental changes also diminish metabolic health and quality of life after birth (Hales and Barker, 2001; Wells, 2011). The link between stress-induced IUGR and post-natal metabolic disorders was first described by Barker and Hales, whose epidemiological studies correlated low birthweight with adulthood obesity, type II diabetes, insulin resistance, and hypertension (Hales et al., 1991; Hales and Barker, 1992; Ariouat and Barker, 1993; Barker et al., 1993). Their dogma-establishing discovery, which they termed the Thrifty Phenotype Hypothesis, has been substantiated by thorough subsequent research that collectively estimates IUGR-born individuals to be at 18-fold greater risk for developing clinical metabolic disease by adulthood (reviewed in detail by Crispi et al., 2017; Yates et al., 2018). IUGR affects up to 25% of pregnancies worldwide and is the 2nd leading cause of perinatal mortality and morbidity (Wu et al., 2006; Hoyert and Gregory, 2020; Tesfa et al., 2020). Moreover, the impact of IUGR on the health of surviving offspring can manifest as early as 3 years of age (Iniguez et al., 2006; Milovanovic et al., 2014). Advances in pre-natal and neonatal care for IUGR infants have markedly improved survival rates in IUGR infants, which makes identifying targetable programming mechanisms for improving long-term metabolic health in these individuals an emerging priority (Hoyert and Gregory, 2020).
Low birthweight in livestock due to IUGR is a barrier to the economic sustainability of meat production, as these animals exhibit greater early-life mortality and lifelong performance deficits (Yates et al., 2018). Severe maternofetal stress is linked to high rates of conceptus loss, which not only reduces the number of offspring but also diminishes the dam's productivity index (Doney et al., 1976; Hansen, 2014). This may result in pre-mature culling of otherwise desirable females, leading to the loss of high-quality genetics and increasing costs associated with replacement females. In addition to pre-natal demise, IUGR often results in offspring that are born live but weak and lack the vigor needed to stand and nurse appropriately, which frequently leads to secondary starvation, hypothermia, and injury (Wu et al., 2006; Reynolds and Caton, 2011; Flinn et al., 2020). The failure of low birthweight newborns to thrive increases total pre-weaning death losses by up to 15% across livestock species (Wu et al., 2006; Flinn et al., 2020). Although perinatal death loss is a concerning animal welfare issue that warrants improvement, the most common and economically relevant outcomes for low birthweight livestock are poor growth performance and metabolic inefficiency. In response to nutrient restriction (typically associated with placental insufficiency), the fetus develops an asymmetrical growth pattern by preferentially supporting vital tissue growth at the expense of peripheral tissues, primarily skeletal muscle (Bell and Greenwood, 2016; Gibbs et al., 2020). In fact, muscle mass in the IUGR fetus may be restricted by over 50% near term due to thrifty programming (Beede et al., 2019). These deficits persist after birth, as IUGR-born offspring continue to exhibit reduced muscle growth capacity, metabolic dysfunction, and poor body composition (i.e., greater fat-to-lean mass ratios) (Cadaret et al., 2019c; Gibbs et al., 2019, 2020; Yates et al., 2019). Consequently, low birthweight animals require more time on feed to reach market weight and produce lighter, less meritorious carcasses with smaller retail cuts and increased fat trim (Robinson et al., 2013; Bell and Greenwood, 2016; Greenwood and Bell, 2019).
The establishment and characterization of several animal models for IUGR provides the opportunity to study the fetal programming mechanisms underlying IUGR pathologies in livestock and humans (Reynolds et al., 2010; Beede et al., 2019). Currently, these mechanisms are not comprehensively understood, but recent findings implicate developmental changes in adrenergic regulation of muscle and other tissues that are relevant to nutrient utilization, metabolic homeostasis, and peripheral tissue growth (Yates et al., 2011, 2019; Cadaret et al., 2019c; Gibbs et al., 2020). In this review, we highlight the evidence for adrenergic adaptations in IUGR tissues and their roles in impaired post-natal muscle growth and metabolic dysfunction.
The Consequences of IUGR
Etiology of IUGR in Livestock and Humans
There is a broad range of causes for IUGR in livestock and humans that result in varying degrees of fetal growth restriction, which have been reviewed in greater detail elsewhere (Greenwood and Cafe, 2007; Beede et al., 2019). In livestock, IUGR is most associated with environmental conditions that produce sustained maternal stress responses or that limit nutrient availability. Such conditions commonly include heat stress events, prolonged cold exposure, elevation-associated hypoxia, drought, overgrazing, inadequate nutrient supplementation, and feed or forage toxicity (Wu et al., 2006; Reynolds et al., 2010; Robinson et al., 2013). IUGR can also result from multifetal pregnancies, especially in species for which multiple births are uncommon (Yates et al., 2018; Flinn et al., 2020). IUGR cases in humans can result from environmental factors but are more frequently related to diet and lifestyle factors that lead to maternal nutrient imbalance, which can impact the fetal nutrient supply as well as the maternofetal endocrine milieu (Barker et al., 1993). Additional causes in humans include substance abuse, chronic or acute illness, and the use of assisted reproductive technologies (Barker, 1990; Woo et al., 2017). When such conditions are present during the critical window for placental development, placental stunting ensues (Cheema et al., 2006; Blasio et al., 2007; Carr et al., 2012; Brown et al., 2016; Zhang et al., 2016; Burton and Jauniaux, 2017). The result is a permanent state of placental insufficiency, whereby the stunted placenta is unable to meet the nutritional requirements of the fetus during its exponential growth phase late in gestation (Limesand et al., 2018; Yates et al., 2018). As described in more detail below, IUGR is in essence the tertiary result of fetal programming responses to this secondary insult of placental insufficiency more so than to the primary maternal stressor. Consequently, fetal outcomes are rather consistent despite the broad range of maternal causes.
Placental Insufficiency, Poor Intrauterine Conditions, and the Inevitability of IUGR
IUGR is necessitated by the increase in nutrient requirements to support normal fetal growth and the inability of the compromised placenta to meet them. Peak placental development occurs from the mid-1st trimester to the late 2nd trimester, which is from approximately day 30 to 100 of gestation in sheep and day 50 to 90 in humans (Burton and Jauniaux, 2017; Flinn et al., 2020). Most maternal stressors that occur during this critical window shift blood flow (and thus nutrient delivery) away from the gravid uterus (Lang et al., 2000; Limesand et al., 2018). For example, heat stress that increases maternal body temperature by as little as 0.7°C redirects maternal blood flow to the skin and nasal mucosa in order to dissipate heat, resulting in concomitant decreases in blood flow through caruncles and cotyledons (Alexander et al., 1987). When sustained, reduced uterine blood flow results in a smaller placenta with diminished vascular density, increased vascular resistance, and reduced expression of nutrient transporters (Regnault et al., 2002; Wallace et al., 2003; Cheema et al., 2006; Brown et al., 2011; Burton and Jauniaux, 2017; Limesand et al., 2018). Collectively, these and other microanatomical changes create a robust impairment in placental transport capacity.
Placental stunting has little to no effect on the fetus during early and mid-gestation, when its relatively small size creates only modest nutrient demands. However, discrepancies between fetal nutrient requirements and placental capacity begin to appear early in the 3rd trimester and progressively worsen toward term due to rapid fetal growth in late gestation (Yates et al., 2018; Posont and Yates, 2019), as illustrated in Figure 1. The inadequate supply of glucose and O2 by the placenta creates chronic fetal hypoxemia and hypoglycemia (Limesand et al., 2007; Macko et al., 2013). The 30–50% reductions in blood O2 and glucose initiate fetal stress responses marked by pronounced increases in circulating concentrations of the catecholamines, epinephrine and norepinephrine (Leos et al., 2010; Rozance et al., 2018), in addition to inflammatory cytokines and other endocrine factors (Jones et al., 2018; Cadaret et al., 2019b). Increased adrenergic activity alters nutrient utilization, blood flow, and insulin secretion so as to preferentially redirect glucose and O2 that is available to the most essential tissues (Morrison, 2008; Camacho et al., 2017; Rozance et al., 2018; Yates et al., 2019; Davis et al., 2020). Like other nutrients, placental transport of amino acids is also reduced in IUGR pregnancies (Brown et al., 2011). In response, the IUGR fetus slows protein accretion in peripheral tissues substantially, which helps to maintain blood concentrations for most amino acids and provides an additional energy substrate for essential tissues (Rozance et al., 2018). Less is understood about fatty acid fluxes in the IUGR fetus. Recent findings in IUGR baboon fetuses indicate that circulating free fatty acid concentrations near term appear to be normal (Chassen et al., 2020). However, adrenergic activation under non-pathological conditions is known to increase fatty acid mobilization via lipolysis (Beard et al., 2018). Similar effects in the IUGR fetus may ultimately diminish fat stores, which would help to explain the well-documented disruption in perinatal thermoregulation (Flinn et al., 2020). It is important to note that developmental responses of the IUGR fetus to intrauterine stress increase its chances for survival and only become problematic after birth, when the absence of chronic stress creates a mismatch with the IUGR-born offspring's metabolic programming (Boehmer et al., 2017; Limesand and Rozance, 2017; Yates et al., 2019).
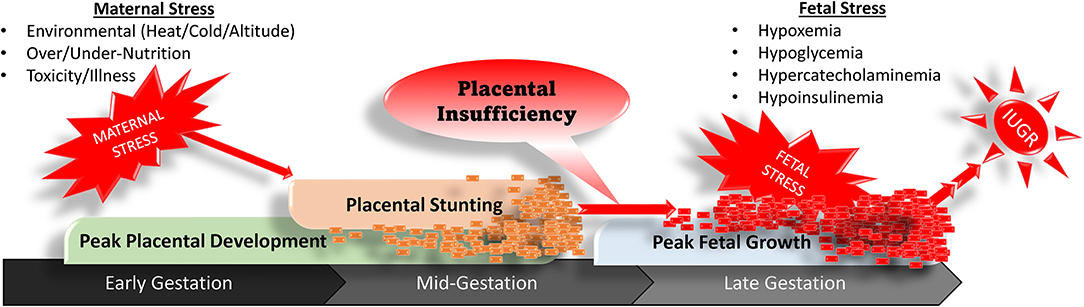
Figure 1. Progression of stress-induced placental stunting in early to mid-gestation that results in placental insufficiency, fetal stress, and intrauterine growth restriction in late gestation.
The Tissue-Specific Impacts of IUGR
In response to chronic stress conditions, IUGR fetuses undergo a number of programming adjustments that disproportionally suppress growth and metabolism of peripheral tissue relative to that of bone, neural, and hepatic tissues (Yates et al., 2011, 2019; Macko et al., 2013, 2016; Posont et al., 2018; Cadaret et al., 2019c). The sparing of certain tissues at the expense of others creates the asymmetric growth patterns that are hallmark to IUGR fetuses and offspring. In IUGR fetal, neonatal, and juvenile lambs this has been shown to manifest morphometrically as greater body length-to-bodyweight, brain-to-bodyweight, and liver-to-bodyweight ratios (Cadaret et al., 2019c; Yates et al., 2019; Gibbs et al., 2020). Skeletal muscle is perhaps the most profoundly targeted peripheral tissue due it its high rates of glucose and O2 consumption and its sensitivity to adrenergic regulation. In the uncompromised fetus, skeletal muscle accounts for ~65% of total glucose consumption and 85% of insulin-stimulated glucose utilization, and these processes can be interrupted by increased catecholamine concentrations (Brown, 2014). Consequently, adaptive programming restricts skeletal muscle growth capacity and metabolic function (Yates et al., 2012b, 2014; Chang et al., 2019), as summarized in Figure 2. Histological assessments in fetal sheep show that IUGR skeletal muscle fibers are smaller in diameter and contain fewer myonuclei, which limits the capacity for protein synthesis and accretion (Yates et al., 2014, 2016). Reductions in the myonuclear accumulation essential for hypertrophic muscle fiber growth are the result of intrinsic functional impairments in myogenic stem cells known as myoblasts. These cells comprehensively exhibit diminished proliferation capacity (Yates et al., 2014; Soto et al., 2017; Posont et al., 2018) and may also exhibit reduced differentiation (Posont et al., 2021), both of which are rate-limiting steps in the facilitation of muscle fiber growth through fusion-mediated nuclei donation. Impaired myonuclear accumulation and reduced protein synthesis relative to protein degradation lead to reductions in muscle mass (Brown et al., 2011; Yates et al., 2014; Rozance et al., 2018; Chang et al., 2019).
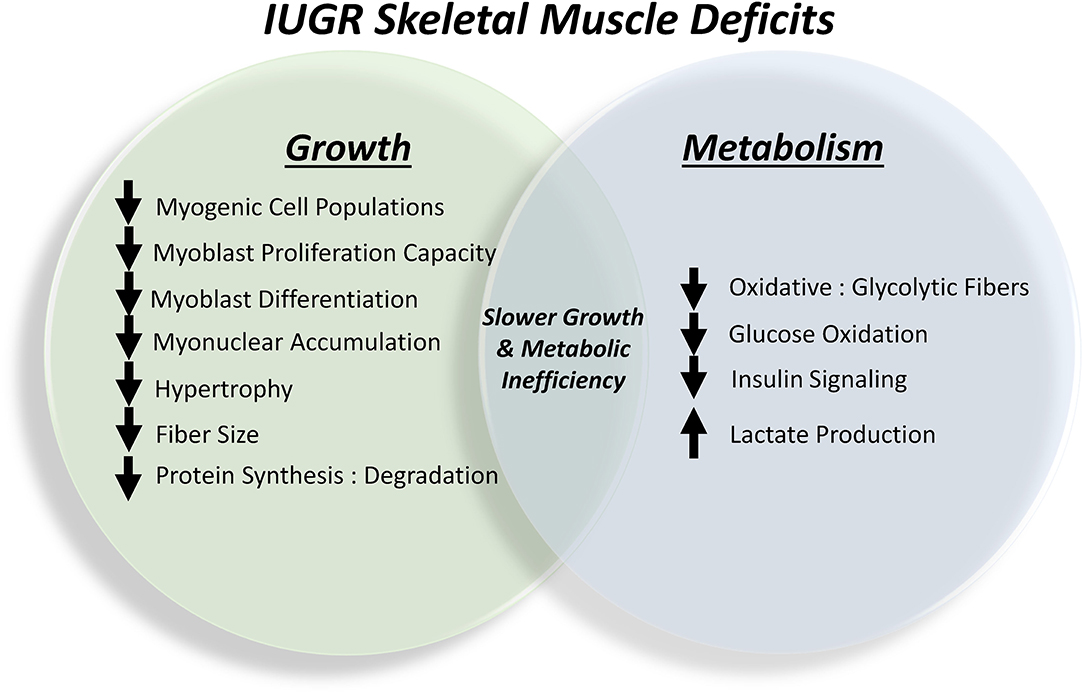
Figure 2. Summary of the outcomes of skeletal muscle programming in the IUGR fetus that contribute to lifelong impairments in growth capacity and metabolic homeostasis.
In addition to limiting muscle mass, IUGR fetal adaptations also spare nutrients and O2 by directly altering metabolic processes in skeletal muscle (Posont and Yates, 2019; Pendleton et al., 2021). Specifically, less glucose is oxidized by muscle in favor of greater glycolytic lactate production, which provides a substrate source for hepatic gluconeogenesis via the Cori cycle. In fetal, neonatal, and juvenile lambs, this manifested in reductions of up to 50% in hindlimb-specific glucose oxidation rates, which were concomitant with increased blood lactate concentrations (Cadaret et al., 2019a,c; Yates et al., 2019; Gibbs et al., 2021; Posont et al., 2021). Similar impairment of glucose oxidation was observed in ex vivo assessments of primary skeletal muscle isolated from these animals. Because the majority of glucose is metabolized by skeletal muscle, reduced whole-body glucose oxidation rates were also observed in IUGR fetal sheep (Limesand et al., 2007; Brown et al., 2015). Interestingly, these reductions in muscle glucose oxidation were independent of muscle glucose utilization and insulin sensitivity in most cases. The shift in skeletal muscle metabolism coincided with changes in fiber type proportions, as semitendinosus and biceps femoris (i.e., upper hindlimb) muscles from near-term IUGR fetal sheep exhibited 20–50% reductions in the proportion of oxidative fibers (i.e., Types I and IIa) relative to glycolytic fibers (i.e., Type IIx) (Yates et al., 2016). Changes in metabolic processes are accompanied by disruption of insulin signaling pathways, as demonstrated by reduced phosphorylation of the insulin signaling hub Akt in primary flexor digitorum superficialis muscle from IUGR fetal and neonatal sheep when incubated with low or high insulin concentrations (Cadaret et al., 2019a,c; Yates et al., 2019; Posont et al., 2021). This impairment is almost certainly a contributing factor in the greater frequency of insulin resistance in IUGR-born offspring (Dulloo et al., 2006; Lorenzo et al., 2008; Macko et al., 2013, 2016; Camacho et al., 2017).
Glucose homeostasis in the IUGR fetus and offspring is further impeded by reductions in pancreatic islet mass and function (Boehmer et al., 2017; Camacho et al., 2017; Chen et al., 2017). β cells located within pancreatic islets produce and secrete insulin in response to elevated blood glucose, which in turn stimulates muscle and other insulin-sensitive tissues to clear glucose from circulation for metabolism or storage. However, this function is diminished by IUGR pathologies that reduce islet size, β cell proliferation rates, and development of islet microvasculature (Lee and Hennighausen, 2005; Limesand et al., 2005; Kostromina et al., 2013). These disruptions in development impair islet functionality, leading to reduced insulin content and a poor capacity for glucose-stimulated insulin secretion (Limesand et al., 2006, 2007). As with skeletal muscle, functional deficits in pancreatic islets persist in offspring. Indeed, IUGR-born lambs continued to exhibit reduced islet insulin content and impaired glucose-stimulated insulin secretion as neonates (Cadaret et al., 2019c; Yates et al., 2019).
Restricted nutrient availability results in a substantial reduction in fat deposition by the IUGR fetus. By the mid-3rd trimester, IUGR fetal sheep exhibited reduced mass of abdominal, pericardial, and hindlimb adipose deposits, which resulted in less whole-body lipid content (Alexander, 1978). Bioelectrical impedance estimates in IUGR-born lambs indicated that fat mass continued to be reduced in the neonatal stage (Gibbs et al., 2019). The lack of fat in IUGR newborns coupled with a 2.5-fold reduction in expression of uncoupling protein 1 (UCP1) helps to explain their reduced capacity for thermoregulation (Flinn et al., 2020). Unlike deficits in skeletal muscle mass, the discrepancies in fat mass begin to wane beyond the neonatal stage, as illustrated in Figure 3. In fact, IUGR-born lambs and children begin to exhibit greater-than-normal fat deposition as juveniles (Dulloo et al., 2006; Zinkhan et al., 2018; Gibbs et al., 2020). This process, known as catch-up growth, coincides with enhanced expression of adipogenic promoters including PPARγ, fatty acid synthase, and acetyl-CoA carboxylase α (Desai and Ross, 2011; Zinkhan et al., 2018). The excessive storage of nutrients as fat is a reflection of the mismatch created by thrifty metabolic programming but adequate nutrient availability after birth (Desai and Ross, 2011). Moreover, the greater propensity for fat deposition combined with restricted muscle growth capacity leads to less desirable body composition and carcass merit that is observed in IUGR-born livestock at harvest (Robinson et al., 2013; Bell and Greenwood, 2016; Greenwood and Bell, 2019).
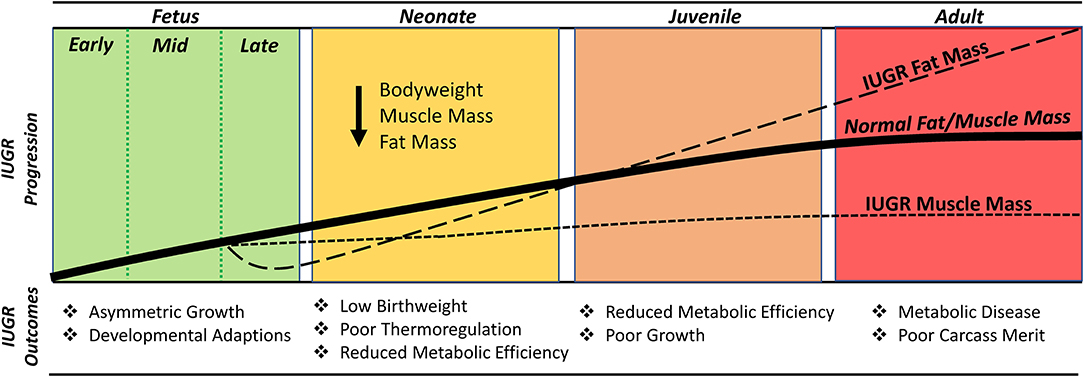
Figure 3. Timeline illustrating the changes in muscle growth capacity and fat deposition in the IUGR fetus/offspring relative to a normal (i.e., uncompromised) fetus/offspring.
Adrenergic Programming Is a Mechanistic Driver of IUGR Outcomes
Adrenergic Regulation of Growth and Metabolism
The adrenergic system is an intricate and robust regulatory system that utilizes multiple signaling pathways to elicit changes associated with stress responses, growth, and metabolism across many tissue types. Epinephrine and norepinephrine are catecholamines that serve as the primary ligands for the adrenergic system (Diego et al., 2008). Although norepinephrine is an important neurotransmitter, almost all catecholamines released into circulation during stress originate from the chromaffin cells of the adrenal medulla (Yates et al., 2012b). Catecholamines act by binding G protein-coupled adrenoceptors on cellular surfaces, which in turn activate 2nd messenger pathways. Adrenoceptors (Adr) exist in nine subtypes among two major classes (α1A, α1B, α1D, α2A, α2B, α2C, β1, β2, and β3), which are expressed in tissue-specific combinations throughout the body (Ciccarelli et al., 2017). As summarized in Figure 4, the tissue specificity of Adr profiles allow intricate regulation of physiological processes associated with growth and development, blood flow, metabolism, and other cellular functions in skeletal muscle, adipose tissue, and pancreatic islets, among other tissue types (Beermann, 2002; Anthony and Henry, 2015; Chen et al., 2017; Beard et al., 2018).
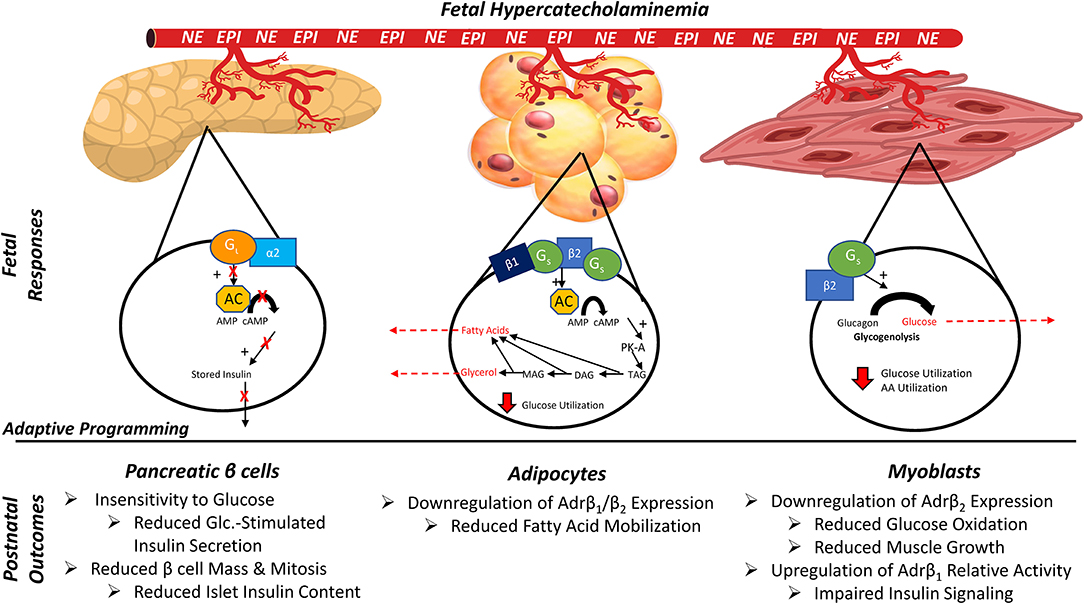
Figure 4. Tissue-specific outcomes of adrenergic programming in the IUGR fetus/offspring due to chronic exposure to elevated circulating catecholamine concentrations in utero. EPI, Epinephrine; NE, norepinephrine; Gi, inhibitory G-protein α subunit; Gs, stimulatory G-protein α subunit; α2, β1, β2, adrenergic receptors; AC, adenylyl cyclase; cAMP, cyclic AMP; PKA, protein kinase A; TAG/DAG/MAG, tri/di/monoacylglycerol; AA, amino acids.
In skeletal muscle, acute stimulation of Adrβ2 (the most abundant isoform expressed by muscle tissues) modestly reduces glucose uptake but increases glycogen breakdown, glucose oxidation, and protein synthesis (Claeys et al., 1989; Anthony and Henry, 2015; Cadaret et al., 2017; Kelly et al., 2018). Adrβ2 pathways also work additively with insulin to activate Akt via phosphorylation and to increase insulin-stimulated glucose oxidation (Bodine et al., 2001; Rommel et al., 2001; Cadaret et al., 2017; Camacho et al., 2017). Sustained stimulation of Adrβ2 increases muscle mass and leanness by enhancing myoblast proliferation and myonuclear accumulation in muscle fibers, which in turn increases their capacity for protein synthesis and accretion (Beermann, 2002; Cadaret et al., 2017). Findings in adult muscle indicate that activation of Adrβ1 under normal conditions can impede insulin-stimulated Akt phosphorylation, but low expression of this isoform by muscle creates only modest implications for myoblast function, protein synthesis, and metabolism (Yates et al., 2012a; Cadaret et al., 2017). In contrast, adipose tissue contains substantial amounts of Adrβ1 and Adrβ3 that, when activated, reduce glucose uptake and increase lipolysis and fatty acid mobilization in concert with Adrβ2 and hormone sensitive lipase (Lafontan and Langin, 2009). Mobilized free fatty acids can then be utilized for β oxidation by muscle, liver, and other tissues (Anthony and Henry, 2015; Beard et al., 2018). Adrenergic regulation of pancreatic islets, which has been reviewed in great detail elsewhere (Boehmer et al., 2017; Limesand and Rozance, 2017), is somewhat paradoxical. Elevated circulating catecholamine concentrations profoundly inhibit glucose-stimulated insulin secretion, primarily through the activation of Adrα2C and other α isoforms in β cells (Leos et al., 2010; Andrews et al., 2015). However, basal adrenergic activity appears to be essential for proper islet development in utero, as adrenal demedullation in otherwise uncompromised fetal sheep diminished insulin secretion (Yates et al., 2012b; Macko et al., 2016).
Chronic Catecholamine Exposure Alters Adrenergic Programming in IUGR Tissues
In response to progressive hypoxemia and hypoglycemia during late gestation, the IUGR fetus sustains increases of up to 7-fold in circulating catecholamines, which are only alleviated by birth (Leos et al., 2010; Macko et al., 2013, 2016). Brief spikes in fetal catecholamines occur periodically even in uncompromised pregnancies. However, when hypercatecholaminemia is sustained for days or even weeks (as it is in the IUGR fetus), fetal programming responses are induced that alter adrenergic responsiveness in catecholamine-sensitive tissues. Protein and gene expression analyses in skeletal muscle and myoblasts from IUGR fetal and neonatal sheep indicate substantial reductions in Adrβ2 but normal expression of Adrβ1 (Yates et al., 2018, 2019). This appears to shift the relative adrenergic tone by reducing the effects of stimulatory Adrβ2 pathways relative to those of inhibitory Adrβ1 pathways. The nature of this change in adrenergic tone is consistent with impaired muscle insulin signaling, myoblast proliferation and differentiation, and insulin-stimulated glucose oxidation (in the absence of impaired glucose uptake) described in earlier sections. Additional work is warranted to more thoroughly characterize the observed adrenergic programming, but existing evidence indicates that it almost certainly contributes to the persistent deficits in muscle mass and glucose oxidation exhibited by IUGR-born offspring (Cadaret et al., 2019c; Gibbs et al., 2019, 2020, 2021; Posont et al., 2021).
Chronic exposure to elevated catecholamines also decreased Adrβ2 and increased Adrβ3 in IUGR fetal and neonatal adipose tissue, with no apparent effect on Adrβ1 expression (Myers et al., 2008; Chen et al., 2010). As in skeletal muscle, the programmed desensitization of Adrβ2 expression and function in adipose tissue serves as a compensatory mechanism to partially offset chronic adrenergic stimulation. This adaptation benefits the developing fetus but has metabolic ramifications following birth, when circulating catecholamine concentrations return to normal. Specifically, IUGR neonatal lambs exhibited a 55% reduction in fatty acid mobilization when infused with epinephrine (Chen et al., 2010). Impaired fat mobilization together with the increased propensity for fat storage helps to explain adipose driven catch-up growth typically observed in IUGR-born offspring as they approach the juvenile stage (Dulloo et al., 2006; Lafontan and Langin, 2009; Desai and Ross, 2011; Gibbs et al., 2020).
Adrenergic programming in IUGR pancreatic islets has been comprehensively characterized by the work of SW Limesand, PJ Rozance, and others (Boehmer et al., 2017; Limesand and Rozance, 2017). Their studies have utilized fetal adrenal demedullation, pharmaceutical adrenergic blockade, and direct norepinephrine infusions to demonstrate that elevated circulating catecholamines are the primary inhibitors of insulin secretion in IUGR fetal sheep (Leos et al., 2010; Yates et al., 2012b; Macko et al., 2013, 2016; Chen et al., 2014, 2017). Chronic exposure of fetal islets to catecholamines during late gestation also reduced gene expression for Adrβ1, Adrα1D, Adrα2A and Adrα2C, indicating robust adrenergic insensitivity (Chen et al., 2014, 2017). Because Adrα2 pathways inhibit glucose stimulus-secretion coupling in islet β cells (Sperling et al., 1980; Jackson et al., 1993, 2000), fetal glucose-stimulated insulin secretion was actually enhanced (i.e., greater than in uncompromised fetuses) when elevated adrenergic activity was removed or blocked (Leos et al., 2010; Chen et al., 2014, 2017). Moreover, enhanced glucose-stimulated insulin secretion persisted in IUGR newborn lambs (Camacho et al., 2017), which may contribute to the dangerous perinatal hypoglycemia that occurs in low birthweight babies. Nevertheless, enhancement of β cell stimulus-secretion coupling is transient, and glucose-stimulated insulin secretion begins to falter in the late neonatal stage and into adulthood (Thorn et al., 2011; Cadaret et al., 2019c; Yates et al., 2019; Gibbs et al., 2021). This may be due to the intrinsic reductions in Adrβ expression, as β adrenergic activity is necessary for proper islet development (Borden et al., 2013).
Targeting Adrenergic Adaptations Improves IUGR Outcomes
The identification and characterization of adrenergic programming mechanisms in IUGR tissues provides a target for potential treatment and intervention strategies. Indeed, animal studies have begun to provide the fundamental basis for adrenergic manipulation as a strategy to improve growth and metabolic outcomes in IUGR fetuses and offspring. In IUGR fetal sheep, pharmaceutical blockade of elevated adrenergic activity via direct fetal infusion of Adrβ/α antagonists yielded immediate recovery of glucose-stimulated insulin secretion (Leos et al., 2010; Macko et al., 2013). Although fetal infusions are perhaps not a realistic option for livestock or even humans in most cases, these studies provide the basis for strategies that target fetal hypercatecholaminemia in more practical ways. For example, a follow-up study found that inducing normoxia in IUGR fetal sheep via maternal O2 supplementation also improved glucose-stimulated insulin secretion (Macko et al., 2016). Moreover, intermittent daily maternal hyperoxygenation of ewes carrying IUGR fetuses for the final 2 weeks of gestation improved fetal O2 status, which in turn improved their birthweight, post-natal growth and body composition, neonatal insulin secretion, and skeletal muscle glucose oxidation (Cadaret et al., 2019c). Although hypercatecholaminemia is not the only outcome of chronic fetal hypoxemia, it is reasonable to assume that part of the benefit observed with O2 supplementation was due to moderation of heightened adrenergic activity. In addition to pre-natal interventions, adrenergic programming may be an effective target for post-natal treatment strategies as well. In IUGR-born neonatal sheep, daily oral administration of the Adrβ2 agonist clenbuterol together with the Adrβ1 antagonist atenolol and the Adrβ3 antagonist SR59230A from birth to 30 days of age improved peripheral tissue insulin sensitivity and enhanced glucose utilization rates (Yates et al., 2019). However, this approach failed to recover deficits in skeletal muscle growth and glucose oxidation or in glucose-stimulated insulin secretion, perhaps indicating that oral administration was ineffective. In a subsequent study, IUGR-born lambs were administered the Adrβ2 agonist clenbuterol via daily intramuscular injection (rather than by oral bolus). By 60 days of age, these lambs exhibited substantial improvements in growth, muscle mass, and body symmetry (Gibbs et al., 2020). Greater fat deposition was observed in IUGR lambs at 60 days of age that was not observed at 30 days of age, but this too was improved by daily clenbuterol injections. In vivo and ex vivo metabolic studies showed that daily clenbuterol injections at least partially recovered glucose-stimulated insulin secretion and skeletal muscle glucose oxidation, which was reflected by improvements in early-life whole-body O2 consumption rates (Gibbs et al., 2020, 2021).
The IUGR Phenotype Does Not Result From Adrenergic Programming Alone
The complexity of IUGR programming means that targeting adrenergic dysfunction alone is unlikely to fully recover growth and metabolic deficits in their entirety. For example, adrenal demedullation of IUGR fetal sheep did not improve deficits in pancreatic islet development and only partially corrected insulin secretion (Davis et al., 2015; Macko et al., 2016). Moreover, infusion-induced fetal hypercatecholaminemia in the absence of hypoxia, hypoglycemia, hypoinsulinemia, and other IUGR conditions resulted in less profound impairment of growth and metabolic function (Bassett and Hanson, 1998, 2000; Chen et al., 2014, 2017; Davis et al., 2020, 2021). When maternofetal O2 supplementation was used to improve fetal oxemic status in sheep, metabolic improvements exceeded the impact on apparent adrenergic tone. Specifically, acute fetal normoxia improved insulin secretion in the IUGR fetus prior to reductions in circulating norepinephrine (Macko et al., 2016), and daily maternofetal oxygenation of IUGR pregnancies late in gestation improved post-natal skeletal muscle growth and metabolism without recovering Adrβ2 content (Cadaret et al., 2019c). Recent studies in sheep and other animal models for IUGR have indicated major roles for inflammatory programming (Cadaret et al., 2019a,b; Beer et al., 2021; Lacey et al., 2021; Posont et al., 2021), glucocorticoid exposure (Miller et al., 2012; Morrison et al., 2012), and poor amino acid balance (Wai et al., 2018; Stremming et al., 2020) in the development of IUGR pathologies. Although manipulation of adrenergic activity was effective in improving some key IUGR pathologies, it is clear that a more comprehensive understanding of the independent and interacting mechanisms that contribute to the IUGR phenotype is necessary to fully recover metabolic health in IUGR-born offspring.
Implications
Stress-induced fetal programming mechanisms that create the thrifty IUGR phenotype and impair post-natal growth capacity and metabolic function have not been comprehensively characterized, despite the impact of IUGR in humans and livestock. However, recent studies in sheep and other animal models have implicated developmental changes in adrenergic sensitivity of several tissues as a contributing factor for IUGR pathologies. Although additional underlying mechanisms almost certainly exist, the ability of adrenergic programming to be manipulated with well-characterized pharmaceuticals make it an appealing potential target for intervention and treatment strategies. Indeed, the broad availability of isoform-specific adrenoceptor agonists and antagonists could provide a variety of options for therapeutic strategies to improve outcomes in IUGR-born offspring. Low birthweight due to IUGR remains a global health issue and barrier to sustainable meat animal production that will only worsen with the emergence of climate change. Realistic options for improving lifelong metabolic health in IUGR-born individuals and for recovering growth performance and efficiency in livestock are needed, and growing evidence indicates that strategies built around the correction of adrenergic tissue regulation may be an effective approach.
Author Contributions
RG and DY contributed to the preparation and editing of this review manuscript. All authors contributed to the article and approved the submitted version.
Funding
This work was supported in part by the USDA National Institute of Food and Agriculture Foundational Grants 2019-67015-29448 and 2020-67015-30825, the National Institute of General Medical Sciences Grant 1P20GM104320 (J. Zempleni, Director), the Nebraska Agricultural Experiment Station with funding from the Hatch Act (Accession Number 1009410), and Hatch Multistate Research capacity funding program (Accession Numbers 1011055 and 1009410) through the USDA National Institute of Food and Agriculture. The Biomedical and Obesity Research Core (BORC) in the Nebraska Center for Prevention of Obesity Diseases (NPOD) receives partial support from NIH (NIGMS) COBRE IDeA award NIH 1P20GM104320.
Author Disclaimer
The contents of this publication are the sole responsibility of the authors and do not necessarily represent the official views of the NIH or NIGMS.
Conflict of Interest
The authors declare that the research was conducted in the absence of any commercial or financial relationships that could be construed as a potential conflict of interest.
Publisher's Note
All claims expressed in this article are solely those of the authors and do not necessarily represent those of their affiliated organizations, or those of the publisher, the editors and the reviewers. Any product that may be evaluated in this article, or claim that may be made by its manufacturer, is not guaranteed or endorsed by the publisher.
References
Alexander, G. (1978). Quantitative development of adipose tissue in foetal sheep. Aust. J. Biol. Sci. 31, 489–503. doi: 10.1071/BI9780489
Alexander, G., Hales, J., Stevens, D., and Donnelly, J. (1987). Effects of acute and prolonged exposure to heat on regional blood flows in pregnant sheep. J. Dev. Physiol. 9, 1–15.
Andrews, S. E., Brown, L. D., Thorn, S. R., Limesand, S. W., Davis, M., Hay, W. W. Jr., et al. (2015). Increased adrenergic signaling is responsible for decreased glucose-stimulated insulin secretion in the chronically hyperinsulinemic ovine fetus. Endocrinology 156, 367–376. doi: 10.1210/en.2014-1393
Ariouat, J. F., and Barker, D. J. (1993). The diet of girls and young women at the beginning of the century. Nutr. Health 9, 15–23. doi: 10.1177/026010609300900102
Barker, D. J. (1990). The fetal and infant origins of adult disease. BMJ 301:1111. doi: 10.1136/bmj.301.6761.1111
Barker, D. J., Godfrey, K. M., Gluckman, P. D., Harding, J. E., Owens, J. A., and Robinson, J. S. (1993). Fetal nutrition and cardiovascular disease in adult life. Lancet 341, 938–941. doi: 10.1016/0140-6736(93)91224-A
Bassett, J. M., and Hanson, C. (1998). Catecholamines inhibit growth in fetal sheep in the absence of hypoxemia. Am. J. Physiol. 274, R1536–R45. doi: 10.1152/ajpregu.1998.274.6.R1536
Bassett, J. M., and Hanson, C. (2000). Prevention of hypoinsulinemia modifies catecholamine effects in fetal sheep. Am. J. Physiol. Regul. Integr. Comp. Physiol. (2000) 278, R1171–R81. doi: 10.1152/ajpregu.2000.278.5.R1171
Beard, J. K., Mulliniks, J. T., and Yates, D. T. Function dysfunction of fatty acid mobilization: a review. Diabesity (2018) 5:53. doi: 10.15562/diabesity.2019.53
Beede, K. A., Limesand, S. W., Petersen, J. L., and Yates, D. T. (2019). Real supermodels wear wool: summarizing the impact of the pregnant sheep as an animal model for adaptive fetal programming. Animal Front. 9, 34–43. doi: 10.1093/af/vfz018
Beer, H. N., Lacey, T. A., Gibbs, R. L., Most, M. S., Hicks, Z. H., Grijalva, P. C., et al. (2021). Placental insufficiency improves in intrauterine growth-restricted fetal sheep receiving daily ω-3 fatty acid infusions. Transl. Anim. Sci. 5(Suppl. 1). doi: 10.1093/tas/txab166
Beermann, D. (2002). Beta-adrenergic receptor agonist modulation of skeletal muscle growth. J. Anim. Sci. 80(E-Suppl. 1):E18–E23. doi: 10.2527/animalsci2002.0021881200800ES10004x
Bell, A. W., and Greenwood, P. L. (2016). Prenatal origins of postnatal variation in growth, development and productivity of ruminants. Anim. Prod. Sci. 56, 1217–1232. doi: 10.1071/AN15408
Blasio, D. E., Gatford, M. J., Robinson, K. L., Owens, J. S., and Placental, J. A. (2007). restriction of fetal growth reduces size at birth and alters postnatal growth, feeding activity, and adiposity in the young lamb. Am. J. Physiol. Regul. Integr. Comp. Physiol. 292, R875–R86. doi: 10.1152/ajpregu.00430.2006
Bodine, S. C., Stitt, T. N., Gonzalez, M., Kline, W. O., Stover, G. L., and Bauerlein, R. (2001). Akt/mTOR pathway is a crucial regulator of skeletal muscle hypertrophy and can prevent muscle atrophy in vivo. Nat. Cell Biol. 3, 1014–1019. doi: 10.1038/ncb1101-1014
Boehmer, B. H., Limesand, S. W., and Rozance, P. J. (2017). The impact of IUGR on pancreatic islet development and beta-cell function. J. Endocrinol. 235, R63–R76. doi: 10.1530/JOE-17-0076
Borden, P., Houtz, J., Leach, S. D., and Kuruvilla, R. (2013). Sympathetic innervation during development is necessary for pancreatic islet architecture and functional maturation. Cell Rep. (2013) 4, 287–301. doi: 10.1016/j.celrep.2013.06.019
Brown, L. D. (2014). Endocrine regulation of fetal skeletal muscle growth: impact on future metabolic health. J. Endocrinol. 221, R13–29. doi: 10.1530/JOE-13-0567
Brown, L. D., Green, A. S., Limesand, S. W., and Rozance, P. J. (2011). Maternal amino acid supplementation for intrauterine growth restriction. Front. Biosci. (Scholar Ed.) 3:428. doi: 10.2741/s162
Brown, L. D., and Hay, W. W. Jr. (2016). Impact of placental insufficiency on fetal skeletal muscle growth. Mol. Cell. Endocrinol. (2016) 435, 69–77. doi: 10.1016/j.mce.2016.03.017
Brown, L. D., Rozance, P. J., Bruce, J. L., Friedman, J. E., and Hay, W. W. Jr. (2015). Wesolowski SR. Limited capacity for glucose oxidation in fetal sheep with intrauterine growth restriction. Am. J. Physiol. Regul. Integr. Comp. Physiol. 309, R920–R928. doi: 10.1152/ajpregu.00197.2015
Burton, G. J., and Jauniaux, E. (2017). Pathophysiology of placental-derived fetal growth restriction. Am. J. Obstet. Gynecol. (2018) 218, S745–s61. doi: 10.1016/j.ajog.2017.11.577
Cadaret, C. N., Beede, K. A., Riley, H. E., and Yates, D. T. (2017). Acute exposure of primary rat soleus muscle to zilpaterol HCl (β2 adrenergic agonist), TNFα, or IL-6 in culture increases glucose oxidation rates independent of the impact on insulin signaling or glucose uptake. Cytokine (2017) 96, 107–113. doi: 10.1016/j.cyto.2017.03.014
Cadaret, C. N., Merrick, E. M., Barnes, T. L., Beede, K. A., Posont, R. J., and Petersen, J. L. (2019a). Sustained maternal inflammation during the early third-trimester yields intrauterine growth restriction, impaired skeletal muscle glucose metabolism, and diminished beta-cell function in fetal sheep. J. Anim. Sci. 97, 4822–4833. doi: 10.1093/jas/skz321
Cadaret, C. N., Posont, R. J., Beede, K. A., Riley, H. E., Loy, J. D., and Yates, D. T. (2019b). Maternal inflammation at midgestation impairs subsequent fetal myoblast function and skeletal muscle growth in rats, resulting in intrauterine growth restriction at term. Transl. Anim. Sci. 3, 867–876. doi: 10.1093/tas/txz037
Cadaret, C. N., Posont, R. J., Swanson, R. M., Beard, J. K., Barnes, T. L., and Beede, K. A. (2019c). Intermittent maternofetal O2 supplementation during late gestation rescues placental insufficiency-induced intrauterine growth restriction and metabolic pathologies in the neonatal lamb. Transl. Anim. Sci. 3(Suppl. 1), 1696–700. doi: 10.1093/tas/txz060
Camacho, L. E., Chen, X., Hay, W. W. Jr, and Limesand, S. W. (2017). Enhanced insulin secretion and insulin sensitivity in young lambs with placental insufficiency-induced intrauterine growth restriction. Am. J. Physiol. Regul. Integr. Comp. Physiol. 313, R101–R9. doi: 10.1152/ajpregu.00068.2017
Carr, D. J., Aitken, R. P., Milne, J. S., David, A. L., and Wallace, J. M. (2012). Fetoplacental biometry and umbilical artery Doppler velocimetry in the overnourished adolescent model of fetal growth restriction. Am. J. Obstetr. Gynecol. (2012) 207, 141. e6–e15. doi: 10.1016/j.ajog.2012.05.008
Chang, E. I., Rozance, P. J., Wesolowski, S. R., Nguyen, L. M., Shaw, S. C., and Sclafani, R. A. (2019). Rates of myogenesis and myofiber numbers are reduced in late gestation IUGR fetal sheep. J. Endocrinol. 244, 339–352. doi: 10.1530/JOE-19-0273
Chassen, S. S., Ferchaud-Roucher, V., Palmer, C., Li, C., Jansson, T., Nathanielsz, P. W., et al. (2020). Placental fatty acid transport across late gestation in a baboon model of intrauterine growth restriction. J. Physiol. 598, 2469–2489. doi: 10.1113/JP279398
Cheema, R., Dubiel, M., and Gudmundsson, S. (2006). Fetal brain sparing is strongly related to the degree of increased placental vascular impedance. J. Perinat. Med. 34, 318–322. doi: 10.1515/JPM.2006.061
Chen, X., Fahy, A. L., Green, A. S., Anderson, M. J., Rhoads, R. P., and Limesand, S. W. (2010). β2-Adrenergic receptor desensitization in perirenal adipose tissue in fetuses and lambs with placental insufficiency-induced intrauterine growth restriction. J. Physiol. 588, 3539–3549. doi: 10.1113/jphysiol.2010.192310
Chen, X., Green, A. S., Macko, A. R., Yates, D. T., Kelly, A. C., and Limesand, S. W. (2014). Enhanced insulin responsiveness and islet adrenergic desensitization after discontinuing chronic norepinephrine suppression in fetal sheep. Am. J. Physiol. Endocrinol. Metab. 306, E58–64. doi: 10.1152/ajpendo.00517.2013
Chen, X., Kelly, A. C., Yates, D. T., Macko, A. R., Lynch, R. M., and Limesand, S. W. (2017). Islet adaptations in fetal sheep persist following chronic exposure to high norepinephrine. J. Endocrinol. 232:285. doi: 10.1530/JOE-16-0445
Ciccarelli, M., Sorriento, D., Coscioni, E., Iaccarino, G., and Santulli, G. (2017). “Adrenergic receptors,” in Endocrinology of the Heart in Health and Disease, eds Schisler, J., Lang, C., and Willis, M., (London: Academic Press), 285–315. doi: 10.1016/B978-0-12-803111-7.00011-7
Claeys, M., Mulvaney, D., McCarthy, F, Gore, M, Marple, D, and Sartin, J. (1989). Skeletal muscle protein synthesis and growth hormone secretion in young lambs treated with clenbuterol. J. Anim. Sci. 67, 2245–2254. doi: 10.2527/jas1989.6792245x
Crispi, F., Miranda, J., and Gratacós, E. (2017). Long-term cardiovascular consequences of fetal growth restriction: biology, clinical implications, and opportunities for prevention of adult disease. Am. J. Obstet. Gynecol. 218, S869–s79. doi: 10.1016/j.ajog.2017.12.012
Davis, M. A., Camacho, L. E., Anderson, M. J., Steffens, N. R., Pendleton, A. L., and Kelly, A. C. (2020). Chronically elevated norepinephrine concentrations lower glucose uptake in fetal sheep. Am. J. Physiol. Regul. Integr. Comp. Physiol. 319, R255–r63. doi: 10.1152/ajpregu.00365.2019
Davis, M. A., Camacho, L. E., Pendleton, A. L., Antolic, A. T., Luna-Ramirez, R. I., and Kelly, A. C. (2021). Augmented glucose production is not contingent on high catecholamines in fetal sheep with IUGR. J. Endocrinol. 249, 195–207. doi: 10.1530/JOE-21-0071
Davis, M. A., Macko, A. R., Steyn, L. V., Anderson, M. J., and Limesand, S. W. (2015). Fetal adrenal demedullation lowers circulating norepinephrine and attenuates growth restriction but not reduction of endocrine cell mass in an ovine model of intrauterine growth restriction. Nutrients 7, 500–516. doi: 10.3390/nu7010500
Desai, M., and Ross, M. G., (eds.). (2011). Fetal programming of adipose tissue: effects of intrauterine growth restriction and maternal obesity/high-fat diet. Seminars in reproductive medicine: © Thieme Medical Publishers. doi: 10.1055/s-0031-1275517
Diego, D. E., Gandia, A., and Garcia, L. (2008). A physiological view of the central and peripheral mechanisms that regulate the release of catecholamines at the adrenal medulla. Acta Physiol. 192, 287–301. doi: 10.1111/j.1748-1716.2007.01807.x
Doney, J., Smith, W., and Gunn, R. (1976). Effects of post-mating environmental stress or administration of ACTH on early embryonic loss in sheep. J Agric. Sci. 87, 133–136. doi: 10.1017/S002185960002668X
Dulloo, A. G., Jacquet, J., Seydoux, J., and Montani, J. P. (2006). The thrifty 'catch-up fat' phenotype: its impact on insulin sensitivity during growth trajectories to obesity and metabolic syndrome. Int. J. Obes (Lond) 30(Suppl. 4), S23–35. doi: 10.1038/sj.ijo.0803516
Flinn, T., Kleemann, D. O., Swinbourne, A. M., Kelly, J. M., Weaver, A. C., and Walker, S. K. (2020). Neonatal lamb mortality: major risk factors and the potential ameliorative role of melatonin. J. Anim. Sci. Biotechnol. 11:107. doi: 10.1186/s40104-020-00510-w
Gibbs, R. L., Cadaret, C. N., Swanson, R. M., Beede, K. A., Posont, R. J., and Schmidt, T. B. (2019). Body composition estimated by bioelectrical impedance analyses is diminished by prenatal stress in neonatal lambs and by heat stress in feedlot wethers. Transl. Anim. Sci. 3(Suppl. 1), 1691–5. doi: 10.1093/tas/txz059
Gibbs, R. L., Swanson, R. M., Beard, J. K., Schmidt, T. B., Petersen, J. L., and Yates, D. (2020). Deficits in growth, muscle mass, and body composition following placental insufficiency-induced intrauterine growth restriction persisted in lambs at 60 d of age but were improved by daily clenbuterol supplementation. Transl. Anim. Sci. 4(Suppl. 1), S53–S57. doi: 10.1093/tas/txaa097
Gibbs, R. L., Swanson, R. M., Beard, J. K., Schmidt, T. B., Petersen, J. L., and Yates, D. T. (2021). Deficits in skeletal muscle glucose metabolism and whole-body oxidative metabolism in the IUGR juvenile lamb are improved by daily treatment with clenbuterol. Transl. Anim. Sci. 5(Suppl. 1), S53–S57. doi: 10.1093/tas/txab187
Greenwood, P. L., and Bell, A. W. (2019). Developmental programming and growth of livestock tissues for meat production. Vet. Clin. North Am. Food Anim. Pract. 35, 303–319. doi: 10.1016/j.cvfa.2019.02.008
Greenwood, P. L., and Cafe, L. M. (2007). Prenatal and pre-weaning growth and nutrition of cattle: long-term consequences for beef production. Animal 1, 1283–1296. doi: 10.1017/S175173110700050X
Hales, C. N., and Barker, D. J. (1992). Type 2 (non-insulin-dependent) diabetes mellitus: the thrifty phenotype hypothesis. Diabetologia 35, 595–601. doi: 10.1007/BF00400248
Hales, C. N., and Barker, D. J. (2001). The thrifty phenotype hypothesis. BrMedBull 60, 5–20. doi: 10.1093/bmb/60.1.5
Hales, C. N., Barker, D. J., Clark, P. M., Cox, L. J., Fall, C., and Osmond, C. (1991). Fetal and infant growth and impaired glucose tolerance at age 64. BMJ 303, 1019–1022. doi: 10.1136/bmj.303.6809.1019
Hansen, P. J. (2014). “Early embryonic loss due to heat stress,” in Bovine Reproduction Inc (Ames, IA: John Wiley & Sons Inc), 580–588. doi: 10.1002/9781118833971.ch64
Hoyert, D. L., and Gregory, E. C. W. (2020). Cause-of-death data from the fetal death file, 2015-2017. Natl. Vital. Stat. Rep. 69, 1–20.
Iniguez, G., Ong, K., Bazaes, R., Avila, A., Salazar, T., and Dunger, D. (2006). Longitudinal changes in insulin-like growth factor-I, insulin sensitivity, and secretion from birth to age three years in small-for-gestational-age children. J. Clin. Endocrinol. Metab. 91, 4645–4649. doi: 10.1210/jc.2006-0844
Jackson, B. T., Cohn, H. E., Morrison, S. H., Baker, R. M., and Piasecki, G. J. (1993). Hypoxia-induced sympathetic inhibition of the fetal plasma insulin response to hyperglycemia. Diabetes 42, 1621–1625. doi: 10.2337/diab.42.11.1621
Jackson, B. T., Piasecki, G. J., Cohn, H. E., and Cohen, W. R. (2000). Control of fetal insulin secretion. Am. J. Physiol. Regul. Integr. Comp. Physiol. 279, R2179–R88. doi: 10.1152/ajpregu.2000.279.6.R2179
Jones, A. K., Hoffman, M. L., Pillai, S. M., (2018). McFadden, KK, Govoni, KE, Zinn, SA, et al. Gestational restricted- and over-feeding promote maternal and offspring inflammatory responses that are distinct and dependent on diet in sheep. Biol. Reprod. 98, 184–196. doi: 10.1093/biolre/iox174
Kelly, A. C., Bidwell, C. A., Chen, X., Macko, A. R., Anderson, M. J., and Limesand, S. W. (2018). Chronic adrenergic signaling causes abnormal RNA expression of proliferative genes in fetal sheep islets. Endocrinology 159, 3565–3578. doi: 10.1210/en.2018-00540
Kostromina, E., Wang, X., and Han, W. (2013). Altered islet morphology but normal islet secretory function in vitro in a mouse model with microvascular alterations in the pancreas. PLoS ONE. 8:e0071277. doi: 10.1371/journal.pone.0071277
Lacey, T. A., Gibbs, R. L., Most, M. S., Beer, H. N., Hicks, Z. H., and Grijalva, P. C. (2021). Decreased fetal biometrics and impaired β cell function in IUGR fetal sheep are improved by daily ω-3 PUFA infusion. Transl. Anim. Sci. 5(Suppl. 1). doi: 10.1093/tas/txab168
Lafontan, M., and Langin, D. (2009). Lipolysis and lipid mobilization in human adipose tissue. Prog. Lipid Res. 48, 275–297. doi: 10.1016/j.plipres.2009.05.001
Lang, U., Baker, R. S., Khoury, J., and Clark, K. E. (2000). Effects of chronic reduction in uterine blood flow on fetal and placental growth in the sheep. Am. J. Physiol. Regul. Integr. Comp. Physiol. 279, R53–R9. doi: 10.1152/ajpregu.2000.279.1.R53
Lee, J.-Y., and Hennighausen, L. (2005). The transcription factor Stat3 is dispensable for pancreatic β-cell development and function. Biochem. Biophys. Res. Commun. 334, 764–768. doi: 10.1016/j.bbrc.2005.06.162
Leos, R. A., Anderson, M. J., Chen, X., Pugmire, J., Anderson, K. A., and Limesand, S. W. (2010). Chronic exposure to elevated norepinephrine suppresses insulin secretion in fetal sheep with placental insufficiency and intrauterine growth restriction. Am. J. Physiol. Endocrinol. Metabol. 298, E770–E8. doi: 10.1152/ajpendo.00494.2009
Limesand, S. W., Camacho, L. E., Kelly, A. C., and Antolic, A. T. (2018). Impact of thermal stress on placental function and fetal physiology. Anim. Reprod. 15, 886–898. doi: 10.21451/1984-3143-AR2018-0056
Limesand, S. W., Jensen, J., Hutton, J. C., and Hay Jr, W. W. (2005). Diminished β-cell replication contributes to reduced β-cell mass in fetal sheep with intrauterine growth restriction. Am. J. Physiol. Regul. Integr. Comp. Physiol. 288, R1297–R305. doi: 10.1152/ajpregu.00494.2004
Limesand, S. W., and Rozance, P. J. (2017). Fetal adaptations in insulin secretion result from high catecholamines during placental insufficiency. J. Physiol. 595, 5103–5113. doi: 10.1113/JP273324
Limesand, S. W., Rozance, P. J., Smith, D., and Hay, W. W. Jr. (2007). Increased insulin sensitivity and maintenance of glucose utilization rates in fetal sheep with placental insufficiency and intrauterine growth restriction. Am. J. Physiol. Endocrinol. Metab. 293, E1716–E25. doi: 10.1152/ajpendo.00459.2007
Limesand, S. W., Rozance, P. J., Zerbe, G. O., Hutton, J. C., and Hay, W. W. (2006). Attenuated insulin release and storage in fetal sheep pancreatic islets with intrauterine growth restriction. Endocrinology 147, 1488–1497. doi: 10.1210/en.2005-0900
Lorenzo, M., Fernández-Veledo, S., Vila-Bedmar, R., Garcia-Guerra, L., Alvaro, D. E., and Nieto-Vazquez, C. Resistance induced by tumor necrosis factor-α in myocytes brown adipocytes. J. Anim. Sci. (2008) 86(Suppl. 14):E94–E104. doi: 10.2527/jas.2007-0462.
Macko, A. R., Yates, D. T., Chen, X., Green, A. S., Kelly, A. C., and Brown, L. D. (2013). Elevated plasma norepinephrine inhibits insulin secretion, but adrenergic blockade reveals enhanced β-cell responsiveness in an ovine model of placental insufficiency at 0.7 of gestation. J. Dev. Orig. Health Dis. 4, 402–410. doi: 10.1017/S2040174413000093
Macko, A. R., Yates, D. T., Chen, X., Shelton, L. A., Kelly, A. C., and Davis, M. A. (2016). Adrenal demedullation and oxygen supplementation independently increase glucose-stimulated insulin concentrations in fetal sheep with intrauterine growth restriction. Endocrinology 157, 2104–2115. doi: 10.1210/en.2015-1850
Miller, S. L., Sutherland, A. E., Supramaniam, V. G., Walker, D. W., Jenkin, G., and Wallace, E. M. (2012). Antenatal glucocorticoids reduce growth in appropriately grown and growth-restricted ovine fetuses in a sex-specific manner. Reprod. Fertil. Dev. 24, 753–758. doi: 10.1071/RD11143
Milovanovic, I., Njuieyon, F., Deghmoun, S., Chevenne, D., Levy-Marchal, C., and Beltrand, J. (2014). SGA children with moderate catch-up growth are showing the impaired insulin secretion at the age of 4. PLoS ONE 9:e100337. doi: 10.1371/journal.pone.0100337
Morrison, J. L. (2008). Sheep models of intrauterine growth restriction: fetal adaptations and consequences. Clin. Exp. Pharmacol. Physiol. 35, 730–743. doi: 10.1111/j.1440-1681.2008.04975.x
Morrison, J. L., Botting, K. J., Soo, P. S., McGillick, E. V., Hiscock, J., Zhang, S., et al. (2012). Antenatal steroids and the IUGR fetus: are exposure and physiological effects on the lung and cardiovascular system the same as in normally grown fetuses? J. Pregnancy 2012:839656. doi: 10.1155/2012/839656
Myers, D. A., Hanson, K., Mlynarczyk, M., Kaushal, K. M., and Ducsay, C. A. (2008). Long-term hypoxia modulates expression of key genes regulating adipose function in the late-gestation ovine fetus. Am. J. Physiol. Regul. Integr. Comp. Physiol. 294, R1312–R1318. doi: 10.1152/ajpregu.00004.2008
Pendleton, A. L., Wesolowski, S. R., Regnault, T. R. H., Lynch, R. M., and Limesand, S. W. Dimming the powerhouse: mitochondrial dysfunction in the liver skeletal muscle of intrauterine growth restricted fetuses. Front. Endocrinol. (2021) 12:888. doi: 10.3389/fendo.2021.612888
Posont, R. J., Beede, K. A., Limesand, S. W., and Yates, D. T. (2018). Changes in myoblast responsiveness to TNFα, and, IL-,6 contribute to decreased skeletal muscle mass in intrauterine growth restricted fetal sheep. Transl. Anim. Sci. 2(Suppl. 1):S44–S7. doi: 10.1093/tas/txy038
Posont, R. J., Cadaret, C. N., Beard, J. K., Swanson, R. M., Gibbs, R. L., and Marks-Nelson, E. S. (2021). Maternofetal inflammation induced for 2 wk in late gestation reduced birth weight and impaired neonatal growth and skeletal muscle glucose metabolism in lambs. J. Anim. Sci. 99:skab102. doi: 10.1093/jas/skab102
Posont, R. J., and Yates, D. T. (2019). Postnatal Nutrient Repartitioning due to Adaptive Developmental Programming. Vet. Clin. North. Am. Food Anim. Pract. 35, 277–288. doi: 10.1016/j.cvfa.2019.02.001
Regnault, T. R., Orbus, R. J., de Vrijer, B., Davidsen, M. L., Galan, H. L., Wilkening, R. B., et al. (2002). Placental expression of VEGF, PlGF and their receptors in a model of placental insufficiency-intrauterine growth restriction (PI-IUGR). Placenta 23, 132–144. doi: 10.1053/plac.2001.0757
Reynolds, L. P., Borowicz, P. P., Caton, J. S., Vonnahme, K. A., Luther, J. S., and Hammer, C. J. (2010). Developmental programming: the concept, large animal models, and the key role of uteroplacental vascular development 1, 2. J. Ani. Sci. 88(Suppl. 13):E61–E72. doi: 10.2527/jas.2009-2359
Reynolds, L. P., and Caton, J. S. (2011). Role of the pre-and post-natal environment in developmental programming of health and productivity. Mol. Cell. Endocrinol. 354, 54–59. doi: 10.1016/j.mce.2011.11.013
Robinson, D. L., Cafe, L. M., and Greenwood, P. L. (2013). Meat science and muscle biology symposium: developmental programming in cattle: consequences for growth, efficiency, carcass, muscle, and beef quality characteristics 1,2. J. Anim. Sci. 91, 1428–1442. doi: 10.2527/jas.2012-5799
Rommel, C., Bodine, S. C., Clarke, B. A., Rossman, R., Nunez, L., and Stitt, T. N. (2001). Mediation of IGF-1-induced skeletal myotube hypertrophy by PI (3) K/Akt/mTOR and PI (3) K/Akt/GSK3 pathways. Nat. Cell Biol. 3, 1009–1013. doi: 10.1038/ncb1101-1009
Rozance, P. J., Zastoupil, L., Wesolowski, S. R., Goldstrohm, D. A., Strahan, B., Cree-Green, M., et al. (2018). Skeletal muscle protein accretion rates and hindlimb growth are reduced in late gestation intrauterine growth-restricted fetal sheep. J. Physiol. 596, 67–82. doi: 10.1113/JP275230
Soto, S. M., Blake, A. C., Wesolowski, S. R., Rozance, P. J., Barthel, K. B., and Gao, B. (2017). Myoblast replication is reduced in the IUGR fetus despite maintained proliferative capacity in vitro. J. Endocrinol. 232, 475–491. doi: 10.1530/JOE-16-0123
Sperling, M. A., Christensen, R. A., Ganguli, S., and Anand, R. (1980). Adrenergic modulation of pancreatic hormone secretion in utero: studies in fetal sheep. Pediatr. Res. 14, 203–208. doi: 10.1203/00006450-198003000-00005
Stremming, J., Jansson, T., Powell, T. L., Rozance, P. J., and Brown, L. D. (2020). Reduced Na(+) K(+) -ATPase activity may reduce amino acid uptake in intrauterine growth restricted fetal sheep muscle despite unchanged ex vivo amino acid transporter activity. J. Physiol. 598, 1625–1639. doi: 10.1113/JP278933
Tesfa, D., Tadege, M., Digssie, A., and Abebaw, S. (2020). Intrauterine growth restriction and its associated factors in South Gondar zone hospitals, Northwest Ethiopia, 2019. Arch. Public Health. 78:89. doi: 10.1186/s13690-020-00475-2
Thorn, S. R., Rozance, P. J., Brown, L. D., and Hay, W. W. (2011). The intrauterine growth restriction phenotype: fetal adaptations and potential implications for later life insulin resistance and diabetes. Semin. Reprod. Med. 29, 225–236. doi: 10.1055/s-0031-1275516
Wai, S. G., Rozance, P. J., Wesolowski, S. R., Hay, W. W. Jr, and Brown, LD. (2018). Prolonged amino acid infusion into intrauterine growth restricted fetal sheep increases leucine oxidation rates. Am. J. Physiol. Endocrinol. Metab. 315, E1143–E1153. doi: 10.1152/ajpendo.00128.2018
Wallace, J. M., Bourke, D. A., Aitken, R. P., Milne, J. S., and Hay, W. W. Jr. (2003). Placental glucose transport in growth-restricted pregnancies induced by overnourishing adolescent sheep. J. Physiol. 547, 85–94. doi: 10.1113/jphysiol.2002.023333
Wells, J. C. (2011). The thrifty phenotype: an adaptation in growth or metabolism? Am. J. Hum. Biol. 23, 65–75. doi: 10.1002/ajhb.21100
Woo, I., Hindoyan, R., Landay, M., Ho, J., Ingles, S. A., McGinnis, LK, et al. (2017). Perinatal outcomes after natural conception versus in vitro fertilization (IVF) in gestational surrogates: a model to evaluate IVF treatment versus maternal effects. Fertil. Steril. 108, 993–998. doi: 10.1016/j.fertnstert.2017.09.014
Wu, G., Bazer, F., Wallace, J., and Spencer, T. (2006). Board-invited review: intrauterine growth retardation: implications for the animal sciences. J. Anim. Sci. 84, 2316–2337. doi: 10.2527/jas.2006-156
Yates, D., Green, A., and Limesand, S. W. (2011). Catecholamines mediate multiple fetal adaptations during placental insufficiency that contribute to intrauterine growth restriction: lessons from hyperthermic sheep. J. Preg. 2011:740408. doi: 10.1155/2011/740408
Yates, D., Macko, A., Nearing, M., Chen, X., Rhoads, R., and Limesand, S. W. (2012a). Developmental programming in response to intrauterine growth restriction impairs myoblast function and skeletal muscle metabolism. J. Preg. 2012:631038 doi: 10.1155/2012/631038
Yates, D. T., Cadaret, C. N., Beede, K. A., Riley, H. E., Macko, A. R., and Anderson, M. J. (2016). Intrauterine growth-restricted sheep fetuses exhibit smaller hindlimb muscle fibers and lower proportions of insulin-sensitive Type I fibers near term. Am. J. Physiol. Regul. Integr. Comp. Physiol. 310, R1020–R9. doi: 10.1152/ajpregu.00528.2015
Yates, D. T., Camacho, L. E., Kelly, A. C., Steyn, L. V., Davis, M. A., and Antolic, A. T. (2019). Postnatal β2 adrenergic treatment improves insulin sensitivity in lambs with IUGR but not persistent defects in pancreatic islets or skeletal muscle. J. Physiol. 597, 5835–5858. doi: 10.1113/JP278726
Yates, D. T., Clarke, D. S., Macko, A. R., Anderson, M. J., Shelton, L. A., and Nearing, M. (2014). Myoblasts from intrauterine growth-restricted sheep fetuses exhibit intrinsic deficiencies in proliferation that contribute to smaller semitendinosus myofibres. J. Physiol. 592, 3113–3125. doi: 10.1113/jphysiol.2014.272591
Yates, D. T., Macko, A. R., Chen, X., Green, A. S., Kelly, A. C., and Anderson, M. J. (2012b). Hypoxaemia-induced catecholamine secretion from adrenal chromaffin cells inhibits glucose-stimulated hyperinsulinaemia in fetal sheep. J. Physiol. 590, 5439–5447. doi: 10.1113/jphysiol.2012.237347
Yates, D. T., Petersen, J. L., Schmidt, T. B., Cadaret, C. N., Barnes, T. L., and Posont, R. J. (2018). ASAS-SSR triennnial reproduction symposium: looking back and moving forward—how reproductive physiology has evolved: fetal origins of impaired muscle growth and metabolic dysfunction: lessons from the heat-stressed pregnant ewe. J. Anim. Sci. 96, 2987–3002. doi: 10.1093/jas/sky164
Zhang, S., Barker, P., Botting, K. J., Roberts, C. T., McMillan, CM, McMillen, IC, et al. (2016). Early restriction of placental growth results in placental structural and gene expression changes in late gestation independent of fetal hypoxemia. Physiol. Rep. 4(23). doi: 10.14814/phy2.13049
Keywords: adaptive fetal programming, developmental origins of health and disease (DOHAD), fetal growth restriction, intrauterine growth restriction (IUGR), low birthweight, metabolic programming
Citation: Gibbs RL and Yates DT (2021) The Price of Surviving on Adrenaline: Developmental Programming Responses to Chronic Fetal Hypercatecholaminemia Contribute to Poor Muscle Growth Capacity and Metabolic Dysfunction in IUGR-Born Offspring. Front. Anim. Sci. 2:769334. doi: 10.3389/fanim.2021.769334
Received: 01 September 2021; Accepted: 19 November 2021;
Published: 09 December 2021.
Edited by:
Caleb Lemley, Mississippi State University, United StatesReviewed by:
Sha Tao, University of Georgia, United StatesLaura Brown, University of Colorado, United States
Copyright © 2021 Gibbs and Yates. This is an open-access article distributed under the terms of the Creative Commons Attribution License (CC BY). The use, distribution or reproduction in other forums is permitted, provided the original author(s) and the copyright owner(s) are credited and that the original publication in this journal is cited, in accordance with accepted academic practice. No use, distribution or reproduction is permitted which does not comply with these terms.
*Correspondence: Dustin T. Yates, ZHVzdGluLnlhdGVzQHVubC5lZHU=