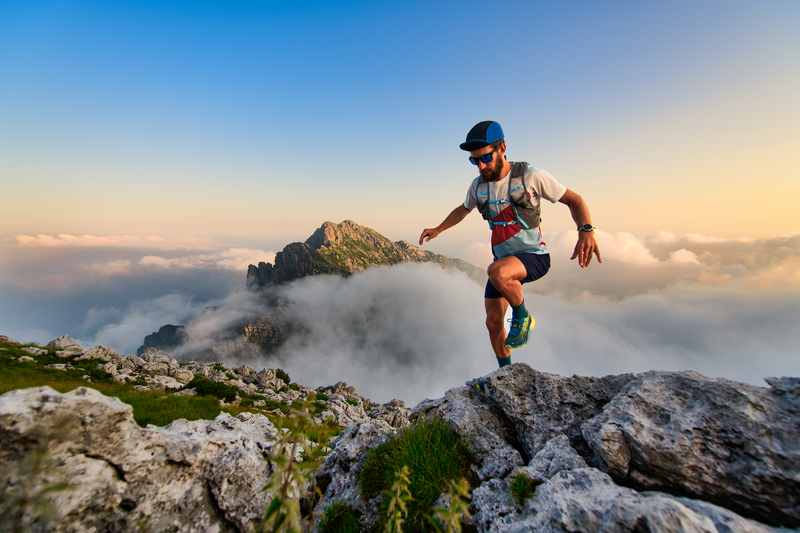
95% of researchers rate our articles as excellent or good
Learn more about the work of our research integrity team to safeguard the quality of each article we publish.
Find out more
REVIEW article
Front. Anal. Sci. , 07 September 2022
Sec. Pharmaceutical analysis
Volume 2 - 2022 | https://doi.org/10.3389/frans.2022.1004113
This article is part of the Research Topic The Protagonism of Bioanalytical Methods in High-Throughput Drug Discovery View all 5 articles
Enzymes are targets commonly explored in screening assays aiming to discover new leads in the drug development process. Among the diverse assay models to identify new enzymatic inhibitors, on-flow assays based on liquid chromatography (LC) can be highlighted. In these approaches, the ligand-enzyme interaction can be examined by monitoring the catalytic activity or the affinity/retention. Most applications use the biological target immobilized in solid supports resulting in the acquisition of an immobilized enzymatic reactor (IMER). Coupling IMERs to LC or mass spectrometry (MS) systems allows monitoring enzyme activity online and studying binding events between target and ligands. On-flow screening assays present many advantages for the hit-to-lead process, such as the possibility of system automation, reusability, and high stability. This review covers articles from the last decade that combine the use of varied immobilization methods on different solid supports and several equipment setups in on-flow systems, emphasizing the performance and capacity of recognizing and identifying biologically active compounds in various matrices.
Several analytical approaches have been applied for the screening of potential enzyme inhibitors, such as colorimetric (Rennó et al., 2012) and fluorescent (Liu et al., 2018a) assays. However, these methods present some advantages. Colorimetric and fluorescent assays are only suitable for monitoring reactions in which the substrate and products have significant difference in their spectrophotometric properties. Additionally, fluorescence signal could be also interfered by background absorption and auto-fluorescence in biological samples (Cheng and Chen, 2018).
More recently, new reliable methodologies have been developed to improve the understanding of enzyme-inhibitor interaction and allow the high-throughput screening of large libraries. Within this context, methodologies that involve solid-supported enzymes have emerged as promising due to cost-saving, reusability, increased stability, and operational optimization.
Immobilized Enzyme Reactors (IMERs, occasionally named immobilized capillary enzyme reactors, ICERs) can be defined as devices that contain the target enzyme, physically confined or localized, with retention of its catalytic activity. At this condition, the immobilized enzyme can be used repeatedly and continuously, and the catalytic reaction can occur under on-flow or static conditions (with the paused flow) (Wouters et al., 2021). Different solid support can be used to create IMERs, such as silica particles (Yuan et al., 2020), magnetic particles (Ximenes et al., 2022), monolithic supports (De Moraes et al., 2014; Wang et al., 2018), and silica fused capillaries (Vanzolini et al., 2013; Seidl et al., 2019).
IMERs can be essential parts of the analytical workflow for screening assays, and can be employed online or offline to an analytical technique, such as liquid chromatography (LC). However, offline approaches generally are time-consuming and involve more sample manipulation in multi-step workflows. On the other hand, IMERs can be prepared by immobilizing the target enzyme in solid supports suitable to LC systems, exhibiting rapid mass transfer capacity, low backpressure, and adequate efficiency, besides being mechanically and chemically stable (Temporini et al., 2016). Furthermore, the solid support should be able to retain the target enzyme even in the on-flow conditions. In this case, on-flow approaches, in which the binding event occurs within the liquid chromatographic system for instance, can be developed. Examples of solid supports suitable for LC systems include open tubular (de Castro et al., 2022) and packed fused silica capillaries (De Moraes et al., 2014), monolithic supports (Kubota et al., 2017), and immobilized artificial membranes (IAM) (Russo et al., 2018). On-flow screening assays based on liquid chromatographic methods furnish high-throughput, automation, and mimic the biochemical system more accurately, given that in vivo ligand-enzyme interactions occur in an on-flow system.
Concerning the on-flow liquid chromatography-based screening assays, the terms online and inline are used for methods that do not require manual transference of the analytes (Minnich et al., 2016; Calleri et al., 2021). Online analytical setup refers to a platform configuration where the aliquots periodically collected in a first dimension are automatically analyzed in a second dimension (de Moraes et al., 2013), whereas inline set-up involves the in-series connection of the IMER and the analytical instrument (Ximenes et al., 2022), with the continuous and total transfer of the analytes.
Herein, we presented a review focused on on-flow enzymatic inhibitor screening assays based on liquid chromatographic methods from 2012 to 2022. The aim of this contribution is to help the pharmaceutical research community to select the most promising approach for the preparation of the IMER with the biomolecule of interest, and to evaluate the ideal instrumental setup for the assay to be developed, considering the objective of the screening assays and the available equipment. Initially, the importance of enzymes as biological targets in the drug development and discovery process is highlighted, followed by a summary of the most common immobilization methods to produce IMERs compatible with LC systems. Finally, an overview of the affinity- and activity-based on-flow screening assays will be covered. Outside the scope of this review are capillary-electrophoresis methods and screening assays involving biomolecules other than enzymes.
Enzymes are involved in several biochemical processes and therefore are essential for living beings. These classes of proteins are extraordinary biocatalysts and can bind to specific substrates and promote several reactions with a high rate of conversion.
Efficient therapies can be achieved by using enzymes as drugs or in diagnostics (Farhadi et al., 2018; Rufer, 2021). On the other hand, the lack, overexpression, or malfunction of a particular type of enzyme can be associated with many diseases making these macromolecules a hallmark group for drug development and target (Robertson, 2007).
Several diseases require the use of enzyme inhibitors, indeed, a substantial part of the drugs applied for clinical purposes are enzyme inhibitors (Drews, 2000; Hopkins and Groom, 2002; Robertson, 2007). The clinical treatment of many diseases such as cancer (Dhokne et al., 2021), neurological disorders (Saxena and Dubey, 2019), infections (Zhang et al., 2021), viruses (Hľasová et al., 2021; Kharkwal et al., 2021), and others, usually involves enzymes as the drug target. In fact, in the last few years (2015–2020), FDA has approved several drugs that act as enzyme inhibitors, those classes include kinase inhibitors for cancer therapy, CY3PA4 inhibitors for neurological, metabolic disorders, and infections, and others (Bhutani et al., 2021a).
Enzymatic inhibition aims to cause any change in the protein conformation, usually by blocking its active site and limiting enzymatic activity. The main challenges in this process are designing molecules with high specificity and being able to act under physiologic conditions (Bhutani et al., 2021b). In this case, a large group of compounds is routinely submitted to screening assays as potential enzymatic inhibitors (Davies et al., 2021).
Understanding the mechanisms involved in enzyme-inhibitor interaction is central to the design of new compounds as potent enzymatic inhibitors. In a biochemical reaction, when the concentration of the enzyme is kept constant and the substrate concentration is increased, the Michaelis-Menten equation (Eq. 1) provides parameters that can be expressed as a function of the substrate concentration and offer information about the kinetics of enzyme-substrate interaction at specific experimental conditions. The parameters V, Vmax, S, and Km are respectively defined as the reaction rate, maximum reaction rate, substrate concentration, and Michaelis-Menten constant. The last one, Km, is also defined as the substrate concentration at half of the maximum velocity and is an intrinsic parameter for each enzyme-substrate system. In general, the enzymatic reaction follows the same behavior: the first-order reaction when the reaction rate is proportional to substrate concentration, and it is a zero-order reaction when the enzyme is saturated by substrate (Sirajuddin and Saqib Ali, 2013; Cornish-bowden, 2015).
Michaelis-Menten equation.
When an inhibitor is added to an enzymatic reaction, new parameters should be considered, such as inhibitor concentration and inhibition constant (Ki). The value of half maximal inhibitory concentration (IC50) provides the inhibitor concentration required to decrease at half the rate of the enzyme activity, while Ki is the dissociation constant describing the binding affinity between the enzyme and the inhibitor. The deductions of IC50 and Ki and their thermodynamic considerations are already properly described in the literature and are out of the scope of this review (Copeland, 2000; Burlingham and Widlanski, 2003; Buker et al., 2019; Georgakis et al., 2020).
The process of inhibition depends on the molecular dynamics between the inhibitor (I), the enzyme (E), and the substrate (S), and the types of inhibition can be fundamentally classified into reversible and irreversible.
Reversible inhibition should lead to the regeneration of the enzyme activity in the media and can be classified into four categories: competitive when the I binds directly to the E; non-competitive, when I binds equally to the free enzyme E and the ES complex; uncompetitive which means that the inhibitor binds exclusively to the ES complex; and mixed inhibition when I binds to both E and ES, but not equivalently as in non-competitive type (Buker et al., 2019).
An example of a competitive inhibitor is Statin which, according to the FDA, is classified as a group of marketed drugs used for cholesterol being effective to reduce the rates of myocardial infarction and mortality (Dimmitt et al., 2018). Among Statin group, the active compounds include Simvastatin (IC50 11 nmol/L), Fluvastatin (IC50 28 nmol/L), Atorvastatin (IC50 8 nmol/L), and Rosuvastatin (IC50 5 nmol/L). Those compounds are responsible for inhibiting the enzyme 3-hydroxy-3-methylglutaryl-coenzyme A reductase (HMG-CoA) and consequently reducing the levels of mevalonate, a metabolite of cholesterol synthesis (Corsini et al., 1999; Istvan, 2002; Rosendo et al., 2007; Dimmitt et al., 2018).
Donepezil is a drug clinically used for Alzheimer’s disease that acts as an acetylcholinesterase (AChE) non-competitive and mixed competitive inhibitor. The multistable modes of interaction with the enzyme allow that donepezil blocks the active site of the enzyme as well binds to the enzyme-substrate complex with similar stability (Silva et al., 2020).
Unlike reversible inhibitors, irreversible inhibitors covalently bind to the enzyme and obstruct its regeneration into free form. This phenomenon can be seen through the low values of the dissociation constant (in the range of nmol/L) (Palmer and Bonner, 2007). There are several examples of irreversible covalent inhibitors in clinical use today, such as omeprazole (Johnson et al., 2010; De Cesco et al., 2017). Omeprazole inhibits the activity of the H+/K+ ATPase through the disulfide bonds formed with cysteine residues, as a consequence of the linkage between the omeprazole and the enzyme, the levels of gastric acid are reduced in the stomach (Cartee and Wang, 2020; Gehringer, 2020).
On one hand, enzymes combine some ideal features, like selectivity and high activity combined with mild condition processes, such as aqueous medium and atmospheric pressure. On the other hand, these biomolecules are susceptible to denaturation due to temperature and pH value variations, the presence of organic solvents, etc. The enzyme in its free form is highly soluble in the reaction medium, which is excellent for the catalytic activity, however, it results in considerable instability and prevents its reuse. Enzyme immobilization on solid supports promotes stabilization and easy recovery of these biomolecules, converting the initial homogenous catalytic system into a heterogeneous one, facilitating the isolation step and obtaining considerably high catalytic activity.
Some studies have shown that enzyme immobilization could provide an extension of the optimal pH and temperature ranges of the enzyme activity compared to the free enzyme (Liu et al., 2018b, 2018a). Also, the wide range of materials used as solid support for enzyme immobilization provides cost reduction and better operational stability, besides avoiding time-laborious steps (Hanefeld et al., 2009; Franssen et al., 2013; Liu et al., 2017). For instance, screening assays of lipase inhibitors demonstrated that the immobilized enzyme on Fe3O4@TiO2/CRL magnetic nanoparticles presented better activity in all evaluated conditions such as temperature, time, stability, and reusability (Liu et al., 2020).
An enzyme can be attached to a solid support through different methodologies, including physical and chemical approaches, as illustrated in Figure 1.
Physical methods, considered one of the easiest and cheapest methodologies, encompass weak interactions such as electrostatic, hydrophobic, entrapment, and biospecific adsorption. The main advantages of the physical methods arise from the simplicity of the immobilization procedure, demanding few equipment and reagents, and resulting in few changes in the enzyme conformation (Rodriguez et al., 2020). Physical immobilization is a reversible method, i.e., the enzyme is attached by weak interaction forces that can be ruptured releasing the biomolecule in solution. Depending on the purpose of immobilization this can be helpful because it is possible to retrieve the support. The reversible condition of this attachment represents a major drawback in on-flow systems, and it narrows the applicability of physical immobilization.
Hou et al (Hou et al., 2020) created a new stationary phase containing α-glucosidase immobilized in porous silica gel with liposome vesicles to screen bioactive compounds from herbal extracts. Based on the hydrophilic behavior of the biological target, it was encapsulated in the aqueous phase of the lipid bilayer. A control stationary phase was prepared in the same conditions but without the α-glucosidase enzyme. Differences in retention times of known α-glucosidase inhibitors in both stationary phases were used to validate the methodology before performing screening assays on Schisandra chinensis. In this case, this methodology explores the interactions between ligand and biological target and the ligand capacity to cross the double-layer membrane of liposomes, proving insight into the performance in studies involving cells and animals.
The biospecific adsorption immobilization procedure involves affinity binding. Usually considered as a subclass of physical immobilization, affinity immobilization is ruled by the bio-specific interactions between a tag (or entity) and a target (like an enzyme). The biomolecule attachment to the support surface is performed by using a second biological structure: at one end this structure attaches to the solid support surface, and at the other one, it binds to the target. In this methodology, the selective interactions between the tag and the target are explored, and the immobilization occurs in a nearly ordered orientation on the support surface. Therefore, that immobilization protocol can avoid random attachment and furnish minimal conformational changes. The main goal of affinity binding immobilization is to maximize the availability of the biological target active site (Mohamad et al., 2015; Reis et al., 2019; Zhang et al., 2019a; Rodriguez et al., 2020).
A classic example of this guided immobilization is the use of biotin, which can strongly bind to streptavidin/neutravidin/avidin, so the biotin pair intermediates the link between the solid support surface and target. In the proposal of a new miniaturized system, André et al. (André and Guillaume, 2021) performed the immobilization of arginase using this approach. Separately, neutravidin was covalently bonded on the support surface and the target was biotinylated. When in contact the pair biotin/neutravidin promoted a fast and strong binding between the solid support and the enzyme. The arginase capillary column produced showed to be stable, it retained 97% of activity after 3 months.
The second type of immobilization procedure is the chemical method, in which the enzyme is attached to the support surface by strong chemical bonds, more specifically, covalent bonds. Chemical immobilization methods avoid enzyme desorption, frequently observed in physical methods, mainly in on-flow assays. The main advantage of chemical immobilization lies in the fact that the target will be anchored in the solid support surface through specific functional groups (such as aldehyde, carboxylic acid, thiol, amine, epoxy, and maleimide) by the formation of a covalent bond (Mohamad et al., 2015; Bilal and Iqbal, 2019; Bilal et al., 2019), enhancing its stability. Usually, in this configuration, the biomolecule is less susceptible to temperature and pH changes, and more stable in the presence of organic solvents. The chemical immobilization methods involve cross-linking and covalent bonds.
Due to their advantages, chemical methods represent the main procedure for enzyme immobilization in the development of on-flow inhibitor screening assays. Frequently, chemical immobilization methods require the use of a bifunctional reagent, namely a linker or cross-linker agent, which should react with the support surface by one end, leaving the other one free to react with functional groups present in the enzyme surface. Consequently, the immobilized enzyme exhibits more mobility favoring its conformational structure. The robustness brought by chemical immobilization comes with a price, to provide stability, the target must be irreversibly attached to the support, which means that there is no recovery of the enzyme (Meryam Sardar, 2015; Wahab et al., 2020).
One of the most used protocols for chemical immobilization involves the formation of Schiff bases using glutaraldehyde as a linking agent. This reaction can be performed under aqueous media and mild conditions which is very attractive for biochemical purposes (Jia and Li, 2015). Evaluating a new selective substrate for Beta-secretase1 (BACE1), De Simone et al (De Simone et al., 2014) used an amino monolithic disk to immobilize the biological target. The support surface was activated with glutaraldehyde, a molecule with an aldehyde in both ends, to then immobilize the BACE1. The BACE1 disk was coupled to the LC system. A known and a new inhibitor of this enzyme were used to validate the selective substrate. The on-flow assay presented a good response for screening potential inhibitors without having long incubation ties and aggregation. The application of this selective substrate avoided the interference of inhibitors with a strong fluorescent response. Not only was developed a fast, high throughput screening (HTS) assay but also a more selective one.
In an innovative strategy, Ximenes et al. (Ximenes et al., 2022) assemble an inline system using magnetic particles as support to immobilize human purine nucleoside phosphorylase (HsPNP). Glutaraldehyde was used as a spacer and cross-linking agent for the covalent immobilization of the target enzyme onto the magnetic particles (MPs) surface. The HsPNP-coated MPs were trapped inside a PEEK tube using a series of magnets and inserted into the on-flow system. The assay was successfully applied in the study of the fourth-generation Immucillin derivative (DI4G), a known inhibitor, determining IC50 and Ki values. The enzyme immobilization on magnetic particles proved to be a versatile approach in which offline and inline modes could be applied using the same target-support system. Other biomolecules were immobilized by covalent bond through the formation of Schiff bases, like as trypsin (Liu et al., 2019), human Thymidylate Kinase (hTMPK) and the human Nucleoside Diphosphate Kinase (hNDPK) (Ferey et al., 2019), cathepsin D (Cornelio et al., 2018), acetylcholinesterase (AChE) (Vandeput et al., 2015; Seidl et al., 2019), butyrylcholinesterase (BChE) (Seidl et al., 2019), Phospholipase A2 (PLA2) (Wei et al., 2021), and others.
In the realm of chemical immobilization techniques, Qiu and collaborators (Qiu et al., 2020) immobilized α-glucosidase using a Metal-Organic Framework (MOF), ZIF-90. This porous material can involve the enzyme in its structure by forming bonds between aldehyde groups from the organic part of ZIF-90 and amino groups from the enzyme, forming a “cluster” around the target. The microreactor containing the ZIF-90@α-glucosidase was coupled to an LC system, resulting in an automated screening system that was applied to screen selective inhibitors from Dioscorea opposite Thunb peel and other Chinese herbs extract (Qiu et al., 2020).
As illustrated in Table 1, the use of chemical immobilization through the formation of covalent bonds represents the main choice to attach the biological target to the solid support surface in the development of on-flow screening assays. The main reason lies in the stability furnished by this type of immobilization procedure, which is highly recommended for on-flow systems.
TABLE 1. Different system setups, solid support, immobilization methods, and target analytes used in the development of LC-based on-flow screening assays.
Solid supports employed for enzyme immobilization aiming for the development of on-flow assays should attend the maximum as possible of the following desired attributes: 1) susceptible to specific chemical reactions for surface functionalization to achieve the desired enzyme immobilization; 2) appropriate superficial area dimension, enabling the enzyme immobilization avoiding overloading that could affect enzyme-ligand interactions; 3) compatible with high-pressure conditions in HPLC systems; 4) support green chemistry purposes to achieve environment-friendly experimental conditions; 6) good to the excellent cost-benefit relationship (Liu and Dong, 2020; Rodriguez et al., 2020; Wahab et al., 2020).
Different solid supports can be employed for enzyme immobilization in the development of LC-based on-flow screening assays. Silica-based supports (de Moraes et al., 2012, 2013; Vanzolini et al., 2013; da Silva et al., 2013; Hu et al., 2015; Silva et al., 2015; Vilela et al., 2015, 2018; Calil et al., 2016; Magalhães et al., 2016; Sarria et al., 2016; Ferreira Lopes Vilela and Cardoso, 2017; Cornelio et al., 2018; Lima et al., 2019; Medina et al., 2019; Qian et al., 2019; Seidl et al., 2019, 2022; Ferey et al., 2019; Chapla et al., 2020; Hou et al., 2020; de Castro et al., 2022) present a versatile and largely explored motif due presence of many hydroxyl groups on its surface. For example, those groups are readily reactive with 3-aminopropyltriethoxysilane (APTES), furnishing an amine-rich surface that can further react with glutaraldehyde (GA), yielding a terminal reactive aldehyde surface susceptible reactions with nucleophilic groups present on the enzyme surface (Figure 2B). Seidl et al. (2022) successfully explored this approach by immobilizing AChE in a fused silica capillary for inhibitors screening in bulbs of Hippeastrum calyptratum. Moreover, the readily available fused silica capillary might justify the majority of the works (>80%) employing silica-based supports for on-flow assays (Table 1).
FIGURE 2. Examples of immobilization approach in the production of IMERs for on-flow screening assays: (A) Epoxy-based monolith; (B) Silica surface and an example of immobilization procedure using GA to form a Schiff base; and (C) Magnetic particles coated with the target enzyme are trapped inside a peek tube.
Monolith-based supports (GMA-co-EDMA, GMA-co-GlyMA, and Acrylamine) (De Moraes et al., 2014; Wang et al., 2018; Zhang et al., 2019b) are described as a synthetically versatile motif (especially when comprising epoxy groups), compatible with a wide flow rate range and detaining a highly porous structure that leads to better interactions between the stationary phase and mobile phase (Figure 2A) (Faria et al., 2006; Groarke and Brabazon, 2016). The use of this material for enzyme immobilization in the development of on-flow assays was found in 10% of the works considered in this review. Based on the aforementioned, pepsin was covalently immobilized on a polymer monolith using glutaraldehyde as a linker by Zhang et al. (Zhang et al., 2019b) to conduct screening assays in nine different natural products.
Lastly, magnetic particles (Figure 2C) have emerged as a promising alternative for enzyme immobilization in screening assays (Trindade Ximenes et al., 2021; Ximenes et al., 2022), representing 7% of the supports used for enzyme immobilization in the development of on-flow screening assays. Magnetic particles (MPs) coated with the target enzyme can be promptly recovered from the reaction medium, resulting in a simple process of enzyme reuse. Wu et al. (Wu et al., 2020) described the covalent immobilization of α-glucosidase onto the aminated MPs surface which was furtherly trapped inside a peek tube by an external magnetic field. Inhibitors from natural sources could be screened from complex natural matrices using the developed approach.
It should be mentioned that offline assays, which can employ different analytical techniques to monitor the protein-ligand interaction, are compatible with particularly interesting supports, such as nanotubes (Wang et al., 2015), zeolite (Tao et al., 2016), and hollow fibers (Zhao et al., 2021). In this review, supports compatible with LC on-flow assays are discussed.
On-flow assays encompass analyses involving analyte separation, purification, identification, and quantification in a single, continuous, and automatized process (Calleri et al., 2021). Based on that, two possible on-flow approaches are applicable for screening assays and will be discussed in this review. The former employs activity-based methods, where the screening process occurs through enzyme activity monitoring. The second one employs affinity-based methods, where the screening process occurs through ligand-enzyme interactions.
The choice of approach to be explored in the development of an on-flow screening method essentially depends on the characteristics of the biological target and the assay purposes. When the biological target has a well-established catalytic function, activity-based assays provide a direct response to the interaction between the potential ligands and the catalytic site. In other cases, a broader approach, such as affinity-based assays, provides quick evidence of the interaction between the whole structure of the biological target and the potential ligands. Therefore, the desired modulating effect should be furtherly verified. Furthermore, when the interest of the screening assay is to isolate a ligand from a complex mixture, affinity-based approaches are essential.
The simplest analytical setup for on-flow screening assays employing HPLC encompasses the direct coupling of an IMER to a selective detector (Figure 3A). Mass spectrometers, for example, can distinguish enzymatic product, substrate, and inhibitor, even if they coelute, without further purification or separation steps, once the IMER does not possess any chromatographic resolution. The main advantage of this approach is fast analysis. However, in screening assays, the evaluated compound (potential inhibitor) coelutes to the quantified enzymatic product, which can result in signal suppression at the ionization source, making necessary to conduct routine control experiments.
FIGURE 3. Simplified LC systems representations for on-flow screening assays with selective detector and absence of a separation step. (A) LC system with the IMER directly coupled to a selective detector. (B) LC system with two IMERs in parallel coupled to a selective detector. (C) LC system with the two different enzymes IMER directly coupled to a selective detector.
Butyrylcholinesterase (BChE) was covalently immobilized into silica-fused capillaries by Vilela et al. (2018). The IMER obtained was coupled to a mass spectrometer and the enzyme activity was evaluated by monitoring the choline precursor ion in an analysis of only 3 min. IC50 and Ki of two known inhibitors (tacrine and galanthamine) were determined to validate the assay in ligands recognition.
Posteriorly, Seidl et al. (2019) described a simultaneous on-flow dual parallel enzyme assay based on two different IMERs containing AChE and BChE enzymes (Figure 3B). Both IMERs were inserted in parallel and placed between an LC system and a mass spectrometer. The resulting system allowed monitoring of the activity of both enzymes with only one single injection and in less than 6 min. Inhibition studies were conducted using galanthamine as a known inhibitor, through IC50 and Ki determination.
More recently, Vilela et al. (Ferreira et al., 2021) described the co-immobilization of beta-secretase1 (BACE1) and AChE in the same silica-fused capillary (Figure 3C), yielding a dual enzymatic assay by LC-MS. Galanthamine (AChE inhibitor) and β-secretase inhibitor (BACE1 inhibitor) were used to validated the analytical platform for screening of ligands. Each target enzyme exhibited selectivity and specificity for its substrate and inhibitor, and the co-immobilization did not affect the affinity of AChE and BACE1 for its ligands.
Other models of LC-based on-flow screening assays allow the use of more affordable detectors such as UV. This approach must include a second dimension in the equipment setup, which provides analytical separation of the residual substrate and the formed product. Despite requiring more sophisticated instruments, these assays can be considered more reliable for screening purposes because the detection of the product, and its subsequent quantification, is less susceptible to interferences than the previously mentioned methods.
Within this context, Magalhães et al. (2016) described the covalent immobilization of Nucleoside triphosphate diphosphohydrolase (NTPDase) into silica-fused capillaries. Activity assays were conducted using a multidimensional chromatographic method with a C8 column in the second dimension to provide the analytical separation of the substrates and products (Figure 4A). The described method was successfully employed in the evaluation and characterization of suramin as NTPDase inhibitor.
FIGURE 4. Simplified LC system representations for on-flow screening assays with emphasis on the dimension dispositions. (A) LC system containing an IMER in the first dimension coupled to an analytical column. (B) LC system with two parallel IMERs (a control and an AChE-containing IMER) in the first dimension and a separation column in the second one. (C) LC system setup to perform online microfractionation before inhibition assay.
An online ligand fishing assay for screening AChE inhibitors was reported by Wang et al. (2018). AChE was immobilized on methacrylate-based monolithic capillaries and inserted in an LC-MS system for ligand fishing and identification from mixtures. To distinguish true ligands from false positives, a negative control-IMER was inserted in parallel to the IMER in the system (Figure 4B). Eight compounds were identified as AChE ligands in extracts of Corydalis yanhusuo, and their AChE inhibitory activities were further determined using an in vitro enzymatic assay.
More recently, an innovative platform for ligand screening in natural products that do not require pre-treatment steps of the sample was described by Seidl et al. (2022). An analytical column inserted in the first dimension (Figure 4C) provided the online microfractionation of the extract components, while the AChE-IMER in the second dimension yielded the inhibition profile of each chromatogram zone. As proof of concept, three known AChE inhibitors (tacrine, galanthamine, and donepezil) and an ethanolic extract obtained from the dry bulbs of Hippeatrum calyptratum were investigated. Specific regions in the chromatogram of the crude extract exhibited AChE inhibitory activity, demonstrating the method’s capability to eliminate previous fractionation steps.
Activity-based on-flow assays are fundamental for the kinetic characterization and stability studies of the immobilized enzyme. When applied to ligand screening assays, they provide direct data regarding the modulation of the catalytic activity of the target enzyme by a ligand and, in a second moment, can furnish the inhibition mechanism and constants (Ki) involved in the enzymatic process. Moreover, the zonal elution mode allows the use of minimal amounts of samples per injection.
For screening purposes, after the initial characterization of the immobilized enzyme, known inhibitors are frequently evaluated as proof of concept. Rodrigues et al. (Rodrigues et al., 2020) developed a screening assay for the identification of xanthine oxidase (XO) inhibitors using an IMER-MS/MS platform. Kaempferol, a competitive XO inhibitor, was used as a known inhibitor as proof of concept and exhibited an IC50 of 4.50 μmol/L. After that, the new screening assay was applied in the evaluation of a library containing thirty natural compounds as potential inhibitors. Each chromatographic process took approximately 5 min. Dihydroartemisinin and artesunic acid were found to be strong XO inhibitors with IC50 of 1.90 μmol/L and 1.77 μmol/L, respectively. Pfaffic acid was identified as a weak inhibitor (IC50 = 57.90 μmol/L).
Recently, after gathering information about the MtPNP-IMER stability, de Castro et al. (de Castro et al., 2022) conducted kinetic studies to access the KM value and understand the binding parameters of the substrate regarding the immobilized enzyme. In brief, low KM values stand for strong interactions between enzyme-substrate. The obtained values for free enzyme were 58.5 μmol/L and 40 μmol/L for inorganic phosphate and inosine substrates, respectively. While 748.6 μmol/L and 59.19 μmol/L were obtained for the IMER regarding the same substrates, respectively. That data pointed significant influence of the immobilization procedure and on-flow conditions over the enzymatic behavior against inorganic phosphate. Moreover, the authors also characterized the inhibition pattern of an Imucillin derivative (DI4G) as a competitive inhibitor. Lastly, the inhibition constant (Ki) of 34.8 nmol/L extracted from the plot, pointed out the selectivity of DI4G towards MtPNP compared to HsPNP, once Ki (HsPNP) = 64.3 nmol/L.
Affinity-based assays evaluate the binding events between the target biomolecule and a ligand. In a general form, the interactions can occur in any region of the enzyme, in an active site or not (not specific binding). Affinity-based assays can furnish the isolation of bioactive compounds from complex mixtures, the ligand ranking according to the strength of the interaction ligand-enzyme, and the determination of affinity constants. On-flow affinity-based assays encompass three different approaches: frontal affinity chromatography (FAC); zonal affinity chromatography (ZAC); and ligand fishing, as illustrated in Figure 5.
Frontal affinity chromatography (FAC) is an affinity chromatography technique capable to quantify the weak interactions between a ligand and a target through breakthrough curves, allowing determination of the dissociation constants (KD), ranking ligands in a mixture, determination of the number of available binding sites, and investigation of binding sites.
This technique is based on the dynamic equilibrium state between the immobilized biological target and the ligand. In this assay, the mobile phase contains the ligand at a specific concentration, A0. Since there is a continuous infusion of ligand into the affinity column, the chromatographic response is equivalent to a front followed by a plateau. In other words, at the beginning of the analysis, the eluting ligand interacts with the target promoting a delay. After that, a saturation of the active sites for binding is reached and from this point on there is no alteration in the ligand concentration eluting from the affinity column. A problematic point of this technique involves a large amount of sample required for the ligand’s continuous infusion into the system (Kasai et al., 1986; Calleri et al., 2011; Kasai, 2021).
FAC assay provides valuable information regarding the bioaffinity column and the binding event, such as the dissociation constant (KD), the number of active sites (n) present in the IMER, and the number of ligands interacting with the target (nL). Exploring this approach, angiogenesis inhibitor Kringle 5 (Bian et al., 2015), TEM-1 beta-lactamase (Chen et al., 2017), trypsin (Qian et al., 2019), thrombin (Yang et al., 2017) and many other proteins were successfully studied. These parameters give information that allows ranking the ligands regarding their binding affinity to the biomolecule proving to be a useful tool to better understand binding events. Moraes et al. (De Moraes et al., 2014) proposed an assay using a mixture of inhibitors with different IC50 for human purine nucleoside phosphorylase inhibition to rank and point out ligands in a mixture. The developed system besides providing insight into interactions could also be used as a screening methodology for human purine nucleoside phosphorylase inhibitors.
Zonal affinity chromatography (ZAC) requires the injection of small quantities of the ligand. The introduction of potential ligands into the bioaffinity column allows binding events to take place, resulting in the increased retention time of substances that can interact and bind to the immobilized target. Likewise, the compounds that have no affinity for the target flow through the column and exhibited shortened retention times. In this assay, it is possible to obtain data regarding the affinity between ligand and biomolecule and the influence of external factors (such as temperature and mobile phase composition) in the ligand-target interaction (Chaiken, 1986, 1987). The extension and strength of interactions between the ligand and the biomolecule will affect the ligand’s retention time. These alterations in experimental data can be translated to the retention factor (k), which can be described as a function of retention time and void time. Based on these relationships it is possible to obtain qualitative and quantitative information in a ZAC experiment. Different equations can be applied to determine binding extension, binding strength, characterization of binding sites, determination of the type of interaction, and other possibilities (Hage, 2017; Tao et al., 2018; Lecas et al., 2021).
Shi et al. (2020) optimize the thrombin immobilization into amino-functionalized silica gel to apply this affinity stationary phase in the screening of ligands by ZAC. After validating the methodology with a selective known inhibitor, the system was used to recognize active components present in the Radix Salviae Miltiorrhiae extract with potential anticoagulant activity. Three compounds (Cryptotanshinone, dihydrotanshinone I, and tanshinone IIA) were identified as thrombin inhibitors. In order to verify the biological activity, these three potential binders were isolated and tested by an anticoagulant in vitro experiment to confirm their response as thrombin inhibitors.
Ligand fishing assay is based on the affinity selection of a ligand from a complex sample by an immobilized biological target. This screening assay comprises 3 different steps: 1) Incubation—a bioactive separation, in which the biological target is incubated with the library containing the potential ligands, allowing the selective formation of ligand-target complexes; 2) Wash—once “fished out” the ligands, the ligand-target complex is extensively washed, so it possible to elute any ligand that weakly interacts with the biomolecule; 3) Elution—after the separation of the ligand-target complex from the unbound compounds via dialysis, magnetic extraction, ultrafiltration, etc.; the ligands are eluted and characterized by different analytical techniques.
Ligand fishing assays can be performed in offline and online mode when coupled to liquid chromatographic systems. It is possible to find studies that perform the ligand fishing assay but do not use this term to define the methodology. Sometimes, terminologies such as magnetic/affinity solid phase extraction and magnetic microbead affinity selection screening (MagMASS) are used to describe screening experiments that are based on the same principle of ligand fishing.
In 2007, Moaddel and collaborators (Moaddel et al., 2007) intended to use a new support—magnetic beads—for the immobilization of a model target, Human Serum Albumin (HSA) to perform an offline ligand fishing assay. Briefly, the immobilized target was incubated with a solution containing ligands and non-ligands. After magnetic separation, the supernatant was collected, and the HSA-coated magnetic beads were washed twice with a buffer solution. Then the supernatant was removed, and a mixture of buffer and the organic solvent was used to elute the retained compounds (with affinity for the HSA). The article describes a simple and effective assay to assess the capacity of HSA to differentiate known ligands from non-ligands in a mixture, and, specially, to demonstrate the potential of immobilizing the target onto the magnetic support. The application of magnetic beads as support for ligand fishing assay became an eminent choice of support (Trindade Ximenes et al., 2021). One reason for that relies on its execution, the target can be easily recovered by an external magnetic field.
However, the transposition of these principles to an on-flow system requires a more complex apparatus, which unfortunately represents more costs. The use of switch valves and loops allows the implementation of affinity assays in one dimension and chromatographic separation in another. Since the incubation occurs in an independent dimension, the unbound components present in the sample can be directed to waste, as illustrated in Figure 6. In Position A (Figure 6), the sample is inserted into the affinity column to allow the interaction between ligands and target, while in the second dimension the analytical column is being conditioned using pump B. Switching valves to Position B (Figure 6), the second configuration, the retained ligands are eluted and transferred to the analytical column for separation and identification. The change in valve position allows that first and second dimensions operate at the same time on different mobile phase compositions and flow rates. In a general mode, these are the steps of this affinity-based assay, however, valves and loops can differ from each experiment. Therefore, exists a diverse number of system setups to perform this assay.
The on-flow method proposed by Peng et al. (2016) employed four-port and six-port valves to screen and identify xanthine oxidase inhibitors from L. macranthoides extract. In the optimization process of the ligand fishing assay, it was established that methanol was the adequate solvent to elute the retained compounds. Even though it was used as an organic solvent for the elution of the retained ligands, the xanthine oxidase enzyme remained with high activity (96%) after ten consecutive cycles. The immobilization promoted better chemical stability to the target allowing its reuse.
Fu et al. (2014) developed an online ligand fishing assay using the free target, i.e., xanthine oxidase was not immobilized on a solid support. This approach was made possible by the ability of turbulent flow chromatography to retain small molecules and allow the easy elution of big structures, such as biomolecules. In this multidimensional setup was necessary the usage of three columns: in the first dimension, the first turbulent flow column separated the unbounded components of the S. miltiorrhiza from the ligand-target complex. The complex flowed to a loop to elute the binders and the eluent was inserted into the second turbulent flow column, where the small molecules (ligands) were retained, and the target easily eluted the column. After separation, the retained ligands were introduced in the third column to be chromatographically separated and identified. This 2D-affinity chromatography was able to recognize three potential inhibitors for the xanthine oxidase, in which two of which confirmed their inhibitory activity.
A different strategy for the ligand fishing assay was proposed by Tao et al. (2013). Three different targets (maltase, invertase, and lipase) were immobilized onto different magnetic particles. After that, permanent magnets were used in chambers containing the MPs coated with each target and connected in series. The developed multi-target affinity selection assay did not use valves; the samples were inserted by a peristaltic pump in a continuous flow. This simpler approach was able to identify seven ligands from a Traditional Chinese Medicine (TCM) plant extract for those three targets. Inhibition studies demonstrated that five out of seven ligands act as enzyme inhibitors.
The improvement of screening assays not only aims to provide reliable data but also to effectively contribute to shortening the time spent in the discovery of new bioactive compounds. The advances in technology demand investments and these online methodologies are no different, usually, they involve relatively expensive accessories and instruments which can be a major drawback to the usage of these techniques.
Regardless of the sample matrices, on-flow systems have been applied to screen potential inhibitors from a variety of targets. Most of the assays used solid-supported enzymes, and, therefore, this review presented the most relevant characteristics of the immobilization process, mentioning the advantages and drawbacks of each methodology always highlighting the most recurrent solid supports and immobilization types.
The versatility of on-flow setups was continually discussed through the many examples presented. Some recent studies enable to conduct automated selectivity studies concurrently with the inhibition assays, or even use two enzymes as biological targets concomitantly. Other setups encompass online microfractionation of complex matrices with subsequent evaluation of the inhibitory profile of each microfraction. These advances, as well as other examples discussed in this review, are examples of the emerging success of liquid-chromatography on-flow assays to screen and identify new inhibitors. The coupling of the separation capacity of the liquid chromatography technique with the high selectivity of ligand-target interaction furnishes a high throughput and reliable screening assay.
All bioassays described have proven to be powerful tools in the discovery of new inhibitors from natural or synthetic sources. The on-flow approach still has a lot to explore, new targets, and configurations. This shows that apart from the benefits there is still a lot of potential in studying this field.
PD designed the review, wrote the section about affinity-based assays and revised the whole manuscript. RL wrote the section about solid supports, on-flow screening assays, and activity-based assays. MC wrote about the methods for enzyme immobilization. CW wrote the introduction and the enzymes as special targets section. MD discussed the structure of the manuscript and contributed to writing the whole manuscript.
We would like to thank the National Council for Scientific and Technological Development (CNPq), the Carlos Chagas Filho Foundation for Research Support in the State of Rio de Janeiro (FAPERJ, Grants E-26/202.909/2019, E-26/010.002128/2019, E-26/010.000978/2019, SEI-260003/015693/2021, and SEI-260003/001167/2020), and the Coordenação de Aperfeiçoamento de Pessoal de Nível Superior—Brazil (CAPES)—Financial Code 001, including the Capes-PrInt Program, project number: 88887.310269/2018–00.
The authors declare that the research was conducted in the absence of any commercial or financial relationships that could be construed as a potential conflict of interest.
All claims expressed in this article are solely those of the authors and do not necessarily represent those of their affiliated organizations, or those of the publisher, the editors and the reviewers. Any product that may be evaluated in this article, or claim that may be made by its manufacturer, is not guaranteed or endorsed by the publisher.
André, C., and Guillaume, Y. C. (2021). Development of a new nano arginase HPLC capillary column for the fast screening of arginase inhibitors and evaluation of their binding affinity. J. Chromatogr. B Anal. Technol. Biomed. Life Sci. 1175, 122751. doi:10.1016/j.jchromb.2021.122751
Bhutani, P., Joshi, G., Raja, N., Bachhav, N., Rajanna, P. K., Bhutani, H., et al. (2021a). U.S. FDA approved drugs from 2015-june 2020: A perspective. J. Med. Chem. 64, 2339–2381. doi:10.1021/acs.jmedchem.0c01786
Bhutani, U., Basu, T., and Majumdar, S. (2021b). Oral drug delivery: Conventional to long acting new-age designs. Eur. J. Pharm. Biopharm. 162, 23–42. doi:10.1016/j.ejpb.2021.02.008
Bian, L., Li, Q., and Ji, X. (2015). Binding of angiogenesis inhibitor kringle 5 to its specific ligands by frontal affinity chromatography. J. Chromatogr. A 1401, 42–51. doi:10.1016/j.chroma.2015.04.058
Bilal, M., Asgher, M., Cheng, H., Yan, Y., and Iqbal, H. M. N. (2019). Multi-point enzyme immobilization, surface chemistry, and novel platforms: A paradigm shift in biocatalyst design. Crit. Rev. Biotechnol. 39, 202–219. doi:10.1080/07388551.2018.1531822
Bilal, M., and Iqbal, H. M. N. (2019). Chemical, physical, and biological coordination: An interplay between materials and enzymes as potential platforms for immobilization. Coord. Chem. Rev. 388, 1–23. doi:10.1016/j.ccr.2019.02.024
Buker, S. M., Boriack-Sjodin, P. A., and Copeland, R. A. (2019). Enzyme–inhibitor interactions and a simple, rapid method for determining inhibition modality. SLAS Discov. 24, 515–522. doi:10.1177/2472555219829898
Burlingham, B. T., and Widlanski, T. S. (2003). An intuitive look at the relationship of Ki and IC50: A more general use for the dixon plot J. Chem Educ., 80 (2), 214. doi:10.1021/ed080p214
Calil, F. A., Lima, J. M., De Oliveira, A. H. C., Mariotini-Moura, C., Fietto, J. L. R., and Cardoso, C. L. (2016). Immobilization of NTPDase-1 from trypanosoma cruzi and development of an online label-free assay. J. Anal. Methods Chem. 2016, 1–9. doi:10.1155/2016/9846731
Calleri, E., Temporini, C., Colombo, R., Tengattini, S., Rinaldi, F., Brusotti, G., et al. (2021). Analytical settings for in-flow biocatalytic reaction monitoring. TrAC Trends Anal. Chem. 143, 116348. doi:10.1016/j.trac.2021.116348
Calleri, E., Temporini, C., and Massolini, G. (2011). Frontal affinity chromatography in characterizing immobilized receptors. J. Pharm. Biomed. Anal. 54, 911–925. doi:10.1016/j.jpba.2010.11.040
Cartee, N. M. P., and Wang, M. M. (2020). Binding of omeprazole to protein targets identified by monoclonal antibodies. PLoS One 15, e0239464. doi:10.1371/journal.pone.0239464
Chaiken, I. M. (1986). Analytical affinity chromatography in studies of molecular recognition in biology: A review. J. Chromatogr. 376, 11–32. doi:10.1016/S0378-4347(00)80821-X
Chapla, V. M., Honório, A. E., Gubiani, J. R., Vilela, A. F. L., Young, M. C. M., Cardoso, C. L., et al. (2020). Acetylcholinesterase inhibition and antifungal activity of cyclohexanoids from the endophytic fungus Saccharicola sp. Phytochem. Lett. 39, 116–123. doi:10.1016/j.phytol.2020.07.016
Chen, X., Li, Y., Zhang, Y., Yang, J., and Bian, L. (2017). Binding of TEM-1 beta-lactamase to beta-lactam antibiotics by frontal affinity chromatography. J. Chromatogr. B Anal. Technol. Biomed. Life Sci. 1051, 75–83. doi:10.1016/j.jchromb.2017.03.013
Cheng, M., and Chen, Z. (2018). Recent advances in screening of enzymes inhibitors based on capillary electrophoresis. J. Pharm. Anal. 8, 226–233. doi:10.1016/j.jpha.2018.05.002
Cornelio, V. E., de Moraes, M. C., Domingues, V. de C., Fernandes, J. B., da Silva, M. F., das, G. F., et al. (2018). Cathepsin D immobilized capillary reactors for on-flow screening assays. J. Pharm. Biomed. Anal. 151, 252–259. doi:10.1016/j.jpba.2018.01.001
Cornish-bowden, A. (2015). One hundred years of michaelis – menten. Perspect. Sci. 4, 3–9. doi:10.1016/j.pisc.2014.12.002
Corsini, A., Bellosta, S., Baetta, R., Fumagalli, R., Paoletti, R., and Bernini, F. (1999). New insights into the pharmacodynamic and pharmacokinetic properties of statins. Pharmacol. Ther. 84, 413–428. doi:10.1016/S0163-7258(99)00045-5
da Silva, J. I., de Moraes, M. C., Vieira, L. C. C., Corrêa, A. G., Cass, Q. B., and Cardoso, C. L. (2013). Acetylcholinesterase capillary enzyme reactor for screening and characterization of selective inhibitors. J. Pharm. Biomed. Anal. 73, 44–52. doi:10.1016/j.jpba.2012.01.026
Davies, G., Semple, H., McCandless, M., Cairns, J., and Holdgate, G. A. (2021). High-Throughput mechanism of inhibition. SLAS Discov. 26, 248–256. doi:10.1177/2472555220983809
de Castro, A. C., Lessa, R. C. S., Wegermann, C. A., and de Moraes, M. C. (2022). A direct OnFlow assay to monitor the activity of purine nucleoside phosphorylase from Mycobacterium tuberculosis. Chromatographia 85, 519–528. doi:10.1007/s10337-022-04158-8
De Cesco, S., Kurian, J., Dufresne, C., Mittermaier, A. K., and Moitessier, N. (2017). Covalent inhibitors design and discovery. Eur. J. Med. Chem. 138, 96–114. doi:10.1016/j.ejmech.2017.06.019
de Moraes, M. C., Cardoso, C. L., and Cass, Q. B. (2013). Immobilized purine nucleoside phosphorylase from Schistosoma mansoni for specific inhibition studies. Anal. Bioanal. Chem. 405, 4871–4878. doi:10.1007/s00216-013-6872-7
de Moraes, M. C., Ducati, R. G., Donato, A. J., Basso, L. A., Santos, D. S., Cardoso, C. L., et al. (2012). Capillary bioreactors based on human purine nucleoside phosphorylase: A new approach for ligands identification and characterization. J. Chromatogr. A 1232, 110–115. doi:10.1016/j.chroma.2011.10.056
De Moraes, M. C., Temporini, C., Calleri, E., Bruni, G., Ducati, R. G., Santos, D. S., et al. (2014). Evaluation of capillary chromatographic supports for immobilized human purine nucleoside phosphorylase in frontal affinity chromatography studies. J. Chromatogr. A 1338, 77–84. doi:10.1016/j.chroma.2014.02.057
De Simone, A., Seidl, C., Santos, C. A. M., and Andrisano, V. (2014). Liquid chromatographic enzymatic studies with on-line Beta-secretase immobilized enzyme reactor and 4-(4-dimethylaminophenylazo) benzoic acid/5-[(2-aminoethyl) amino] naphthalene-1-sulfonic acid peptide as fluorogenic substrate. J. Chromatogr. B Anal. Technol. Biomed. Life Sci. 954, 108–114. doi:10.1016/j.jchromb.2014.01.056
Dhokne, P., Sakla, A. P., and Shankaraiah, N. (2021). Structural insights of oxindole based kinase inhibitors as anticancer agents: Recent advances. Eur. J. Med. Chem. 216, 113334. doi:10.1016/j.ejmech.2021.113334
Dimmitt, S. B., Stampfer, H. G., and Warren, J. B. (2018). The pharmacodynamic and clinical trial evidence for statin dose. Br. J. Clin. Pharmacol. 84, 1128–1135. doi:10.1111/bcp.13539
Drews, J. J. (2000). Drug discovery: A historical perspective. Science 287, 1960–1964. doi:10.1126/science.287.5460.1960
Farhadi, S. A., Bracho-Sanchez, E., Freeman, S. L., Keselowsky, B. G., and Hudalla, G. A. (2018). Enzymes as immunotherapeutics. Bioconjug. Chem. 29, 649–656. doi:10.1021/acs.bioconjchem.7b00719
Faria, A. M., Bottoli, C. B. G., Jardim, I. C. S. F., and Collins, C. H. (2006). Fases estacionárias monolíticas para separações cromatográficas. Quim. Nova 29, 300–309. doi:10.1590/s0100-40422006000200022
Ferey, J., Da Silva, D., Colas, C., Lafite, P., Topalis, D., Roy, V., et al. (2019). Monitoring of phosphorylation using immobilized kinases by on-line enzyme bioreactors hyphenated with High-Resolution Mass Spectrometry. Talanta 205, 120120. doi:10.1016/j.talanta.2019.120120
Ferreira, A., Vilela, L., Eduardo, V., and Cardoso, C. L. (2021). Co-immobilized capillary enzyme reactor based on beta-secretase1 and acetylcholinesterase : A model for dual-ligand screening. Front. Chem. 9, 708374–708410. doi:10.3389/fchem.2021.708374
Ferreira Lopes Vilela, A., and Cardoso, C. L. (2017). An on-flow assay for screening of β-secretase ligands by immobilised capillary reactor-mass spectrometry. Anal. Methods 9, 2189–2196. doi:10.1039/c7ay00284j
Franssen, M. C. R., Steunenberg, P., Scott, E. L., Zuilhof, H., and Sanders, J. P. M. (2013). Immobilised enzymes in biorenewables production. Chem. Soc. Rev. 42, 6491–6533. doi:10.1039/c3cs00004d
Fu, Y., Mo, H. Y., Gao, W., Hong, J. Y., Lu, J., Li, P., et al. (2014). Affinity selection-based two-dimensional chromatography coupled with high-performance liquid chromatography-mass spectrometry for discovering xanthine oxidase inhibitors from Radix Salviae Miltiorrhizae. Anal. Bioanal. Chem. 406, 4987–4995. doi:10.1007/s00216-014-7902-9
Gehringer, M. (2020). Covalent inhibitors: Back on track? Fut. Med. Chem. 12 (15), 1363–1368. doi:10.4155/fmc-2020-0118
Georgakis, N., Ioannou, E., Varotsou, C., Premetis, G., Chronopoulou, E. G., and Labrou, N. E. (2020). Determination of half-maximal inhibitory concentration of an enzyme inhibitor. Methods Mol. Biol., 41–46. doi:10.1007/978-1-0716-0163-1_3
Groarke, R., and Brabazon, D. (2016). Methacrylate polymer monoliths for separation applications. Mater. (Basel) 9, 446. doi:10.3390/ma9060446
Hage, D. S. (2017). Analysis of biological interactions by affinity chromatography: Clinical and pharmaceutical applications. Clin. Chem. 63, 1083–1093. doi:10.1373/clinchem.2016.262253
Hanefeld, U., Gardossi, L., and Magner, E. (2009). Understanding enzyme immobilisation. Chem. Soc. Rev. 38, 453–468. doi:10.1039/B711564B
Hľasová, Z., Pažitná, L., Ondrejovič, M., and Katrlík, J. (2021). Lectin-based assay for the determination of the inhibition activity of small molecule inhibitors of neuraminidases. J. Biotechnol. 325, 65–72. doi:10.1016/j.jbiotec.2020.11.016
Hopkins, A. L., and Groom, C. R. (2002). The druggable genome. Nat. Rev. Drug Discov. 1, 727–730. doi:10.1038/nrd892
Hou, X., Lou, X., Guo, Q., Tang, L., and Shan, W. (2020). Development of an immobilized liposome chromatography method for screening and characterizing α-glucosidase-binding compounds. J. Chromatogr. B Anal. Technol. Biomed. Life Sci. 1148, 122097. doi:10.1016/j.jchromb.2020.122097
Hu, Y., Qian, J., Guo, H., Jiang, S., and Zhang, Z. (2015). Application of frontal affinity chromatography to study the biomolecular interactions with trypsin. J. Chromatogr. Sci. 53, 898–902. doi:10.1093/chromsci/bmu141
Istvan, E. S. (2002). Structural mechanism for statin inhibition of 3-hydroxy-3-methylglutaryl coenzyme A reductase. Am. Heart J. 144, S27–S32. doi:10.1067/mhj.2002.130300
Jia, Y., and Li, J. (2015). Molecular assembly of Schiff base interactions: Construction and application. Chem. Rev. 115, 1597–1621. doi:10.1021/cr400559g
Johnson, D. S., Weerapana, E., and Cravatt, B. F. (2010). Strategies for discovering and derisking covalent, irreversible enzyme inhibitors. Future Med. Chem. 2, 949–964. doi:10.4155/fmc.10.21
Kasai, K. (2021). Frontal affinity chromatography: An excellent method of analyzing weak biomolecular interactions based on a unique principle. Biochim. Biophys. Acta. Gen. Subj. 1865, 129761. doi:10.1016/j.bbagen.2020.129761
Kasai, K. I., Oda, Y., Nishikata, M., and Ishii, S. I. (1986). Frontal affinity chromatography: Theory for its application to studies on specific interactions of biomolecules. J. Chromatogr. 376, 33–47. doi:10.1016/S0378-4347(00)80822-1
Kharkwal, H., Kumar, B. K., Murugesan, S., Singhvi, G., Avasthi, P., Goyal, A., et al. (2021). Search for new therapeutics against HIV-1 via dual inhibition of RNase H and integrase: Current status and future challenges. Future Med. Chem. 13, 269–286. doi:10.4155/fmc-2020-0257
Kubota, K., Kubo, T., Tanigawa, T., Naito, T., and Otsuka, K. (2017). New platform for simple and rapid protein-based affinity reactions. Sci. Rep. 7, 178. doi:10.1038/s41598-017-00264-y
Lecas, L., Dugas, V., and Demesmay, C. (2021). Affinity chromatography: A powerful tool in drug discovery for investigating ligand/membrane protein interactions. Sep. Purif. Rev. 50, 315–332. doi:10.1080/15422119.2020.1749852
Lima, J. M., Salmazo Vieira, P., Cavalcante de Oliveira, A. H., and Cardoso, C. L. (2016). Label-free offline versus online activity methods for nucleoside diphosphate kinase b using high performance liquid chromatography. Analyst 141, 4733–4741. doi:10.1039/C6AN00655H
Lima, J., Seidl, C., Cunha, E., Oliveira, A., and Cardoso, C. (2019). On-flow ligand screening assay based on immobilized nucleoside diphosphate kinase b from Homo sapiens. J. Braz. Chem. Soc. doi:10.21577/0103-5053.20190133
Liu, D.-M., Chen, J., and Shi, Y.-P. (2018a). Tyrosinase immobilization on aminated magnetic nanoparticles by physical adsorption combined with covalent crosslinking with improved catalytic activity, reusability and storage stability. Anal. Chim. Acta 1006, 90–98. doi:10.1016/j.aca.2017.12.022
Liu, D.-M., and Dong, C. (2020). Recent advances in nano-carrier immobilized enzymes and their applications. Process Biochem. 92, 464–475. doi:10.1016/j.procbio.2020.02.005
Liu, D.-M. M., Chen, J., and Shi, Y.-P. P. (2017). Screening of enzyme inhibitors from traditional Chinese medicine by magnetic immobilized α-glucosidase coupled with capillary electrophoresis. Talanta 164, 548–555. doi:10.1016/j.talanta.2016.12.028
Liu, D. M., Chen, J., and Shi, Y. P. (2018b). Advances on methods and easy separated support materials for enzymes immobilization. TrAC Trends Anal. Chem. 102, 332–342. doi:10.1016/j.trac.2018.03.011
Liu, J., Ma, R.-T. T., and Shi, Y.-P. P. (2020). An immobilization enzyme for screening lipase inhibitors from Tibetan medicines. J. Chromatogr. A 1615, 460711. doi:10.1016/j.chroma.2019.460711
Liu, X., Zhu, X., Camara, M. A., Qu, Q., Shan, Y., and Yang, L. (2019). Surface modification with highly-homogeneous porous silica layer for enzyme immobilization in capillary enzyme microreactors. Talanta 197, 539–547. doi:10.1016/j.talanta.2019.01.080
Magalhães, L., de Oliveira, A. H. C., de Souza Vasconcellos, R., Mariotini-Moura, C., de Cássia Firmino, R., Fietto, J. L. R., et al. (2016). Label-free assay based on immobilized capillary enzyme reactor of Leishmania infantum nucleoside triphosphate diphosphohydrolase (LicNTPDase-2-ICER-LC/UV). J. Chromatogr. B Anal. Technol. Biomed. Life Sci. 1008, 98–107. doi:10.1016/j.jchromb.2015.11.028
Medina, R. P., Araujo, A. R., Batista, J. M., Cardoso, C. L., Seidl, C., Vilela, A. F. L., et al. (2019). Botryane terpenoids produced by Nemania bipapillata, an endophytic fungus isolated from red alga Asparagopsis taxiformis - falkenbergia stage. Sci. Rep. 9, 12318. doi:10.1038/s41598-019-48655-7
Meryam Sardar, R. A. (2015). Enzyme immobilization: An overview on nanoparticles as immobilization matrix. Biochem. Anal. Biochem. 04. doi:10.4172/2161-1009.1000178
Minnich, C., Hardy, S., and Krämer, S. (2016). Stopping the babylonian confusion: An updated nomenclature for process analyzers in PAT applications. Chem. Ing. Tech. 88, 694–697. doi:10.1002/cite.201500188
Moaddel, R., Marszałł, M. P., Bighi, F., Yang, Q., Duan, X., and Wainer, I. W. (2007). Automated ligand fishing using human serum albumin-coated magnetic beads. Anal. Chem. 79, 5414–5417. doi:10.1021/ac070268+
Mohamad, N. R., Marzuki, N. H. C., Buang, N. A., Huyop, F., and Wahab, R. A. (2015). An overview of technologies for immobilization of enzymes and surface analysis techniques for immobilized enzymes. Biotechnol. Biotechnol. Equip. 29, 205–220. doi:10.1080/13102818.2015.1008192
Palmer, T., and Bonner, P. L. (2007). Enzymes: Biochemistry, biotechnology and clinical chemistry. second. Woodhead Publishing Limited.
Peng, M. J., Shi, S. Y., Chen, L., Zhang, S. H., Cai, P., and Chen, X. Q. (2016). Online coupling solid-phase ligand-fishing with high-performance liquid chromatography–diode array detector–tandem mass spectrometry for rapid screening and identification of xanthine oxidase inhibitors in natural products. Anal. Bioanal. Chem. 408, 6693–6701. doi:10.1007/s00216-016-9784-5
Qian, J., Zhao, C., Tong, J., Jiang, S., Zhang, Z., Lu, S., et al. (2019). Study the effect of trypsin enzyme activity on the screening of applying frontal affinity chromatography. Int. J. Biol. Macromol. 139, 740–751. doi:10.1016/j.ijbiomac.2019.07.218
Qiu, B., Shi, Y., Yan, L., Wu, X., Zhu, J., Zhao, D., et al. (2020). Development of an on-line immobilized α-glucosidase microreactor coupled to liquid chromatography for screening of α-glucosidase inhibitors. J. Pharm. Biomed. Anal. 180, 113047. doi:10.1016/j.jpba.2019.113047
Reis, C. L. B., de Sousa, E. Y. A., de França Serpa, J., Oliveira, R. C., and Dos Santos, J. C. S. (2019). Design of immobilized enzyme biocatalysts: Drawbacks and opportunities. Quim. Nova 42, 768–783. doi:10.21577/0100-4042.20170381
Rennó, M. N., França, T. C. C., Nico, D., Palatnik-de-Sousa, C. B., Tinoco, L. W., and Figueroa-Villar, J. D. (2012). Kinetics and docking studies of two potential new inhibitors of the nucleoside hydrolase from Leishmania donovani. Eur. J. Med. Chem. 56, 301–307. doi:10.1016/j.ejmech.2012.07.052
Robertson, J. G. (2007). Enzymes as a special class of therapeutic target: Clinical drugs and modes of action. Curr. Opin. Struct. Biol. 17, 674–679. doi:10.1016/j.sbi.2007.08.008
Rodrigues, M. V. N., Barbosa, A. F., da Silva, J. F., dos Santos, D. A., Vanzolini, K. L., de Moraes, M. C., et al. (2016). 9-Benzoyl 9-deazaguanines as potent xanthine oxidase inhibitors. Bioorg. Med. Chem. 24, 226–231. doi:10.1016/j.bmc.2015.12.006
Rodrigues, M. V. N., Corrêa, R. S., Vanzolini, K. L., Santos, D. S., Batista, A. A., and Cass, Q. B. (2015). Characterization and screening of tight binding inhibitors of xanthine oxidase: An on-flow assay. RSC Adv. 5, 37533–37538. doi:10.1039/c5ra01741f
Rodrigues, M. V. N., Rodrigues-Silva, C., Boaventura, S., Oliveira, A. S. S., Rath, S., and Cass, Q. B. (2020). On-Flow LC-MS/MS method for screening of xanthine oxidase inhibitors. J. Pharm. Biomed. Anal. 181, 113097. doi:10.1016/j.jpba.2020.113097
Rodriguez, E. L., Poddar, S., Iftekhar, S., Suh, K., Woolfork, A. G., Ovbude, S., et al. (2020). Affinity chromatography: A review of trends and developments over the past 50 years. J. Chromatogr. B Anal. Technol. Biomed. Life Sci. 1157, 122332. doi:10.1016/j.jchromb.2020.122332
Rosendo, A. B., Dal-Pizzol, F., Fiegenbaum, M., and Almeida, S. de (2007). [Pharmacogenetics and anti-inflammatory effect of HMG-CoA reductase inhibitors]. Arq. Bras. Endocrinol. Metabol. 51, 520–525. doi:10.1590/S0004-27302007000400004
Rufer, A. C. (2021). Drug discovery for enzymes. Drug Discov. Today 26, 875–886. doi:10.1016/j.drudis.2021.01.006
Russo, G., Grumetto, L., Szucs, R., Barbato, F., and Lynen, F. (2018). Screening therapeutics according to their uptake across the blood-brain barrier: A high throughput method based on immobilized artificial membrane liquid chromatography-diode-array-detection coupled to electrospray-time-of-flight mass spectrometry. Eur. J. Pharm. Biopharm. 127, 72–84. doi:10.1016/j.ejpb.2018.02.004
Sarria, A. L. F., Vilela, A. F. L., Frugeri, B. M., Fernandes, J. B., Carlos, R. M., da Silva, M. F., et al. (2016). Copper (II) and zinc (II) complexes with flavanone derivatives: Identification of potential cholinesterase inhibitors by on-flow assays. J. Inorg. Biochem. 164, 141–149. doi:10.1016/j.jinorgbio.2016.09.010
Saxena, M., and Dubey, R. (2019). Target enzyme in alzheimer’s disease: Acetylcholinesterase inhibitors. Curr. Top. Med. Chem. 19, 264–275. doi:10.2174/1568026619666190128125912
Seidl, C., Lima, J. M. de, Leme, G. M., Pires, A. F., Stoll, D. R., and Cardoso, C. L. (2022). A comprehensive 2D-LC/MS online platform for screening of acetylcholinesterase inhibitors. Front. Mol. Biosci. 9, 868597. doi:10.3389/fmolb.2022.868597
Seidl, C., Vilela, A. F. L., Lima, J. M., Leme, G. M., and Cardoso, C. L. (2019). A novel on-flow mass spectrometry-based dual enzyme assay. Anal. Chim. Acta 1072, 81–86. doi:10.1016/j.aca.2019.04.057
Shi, Y., Sun, W., Pan, X., Hou, X., Wang, S., and Zhang, J. (2020). Establishment of thrombin affinity column (TAC)-HPLC-MS/MS method for screening direct thrombin inhibitors from Radix Salviae Miltiorrhiae. J. Chromatogr. B Anal. Technol. Biomed. Life Sci. 1139, 121894. doi:10.1016/j.jchromb.2019.121894
Silva, J. I. da, Nicastro, P. C., Oliveira, P. C. M. de, Fossaluzza, P. C., Morais, É. P., Dias, K. S. T., et al. (2015). A comparative evaluation of acetylcholinesterase inhibition by AChE-ICER and in vitro ellman’s modified method of simplified analogs 3-O-acetyl-N-Benzyl-Piperidine of donepezil. Rev. Virtual Quím. 7, 2334–2346. doi:10.5935/1984-6835.20150139
Silva, M. A., Kiametis, A. S., and Treptow, W. (2020). Donepezil inhibits acetylcholinesterase via multiple binding modes at room temperature. J. Chem. Inf. Model. 60, 3463–3471. doi:10.1021/acs.jcim.9b01073
Sirajuddin, Muhammad, and Saqib Ali, A. B. (2013). “Drug–DNA interactions and their study by UV–Visible, fluorescence spectroscopies and cyclic voltametry,” in Introduction to biological and small molecule drug research and development (Elsevier), 1.doi:10.1016/B978-0-12-397176-0.00001-7
Tao, P., Poddar, S., Sun, Z., Hage, D. S., and Chen, J. (2018). Analysis of solute-protein interactions and solute-solute competition by zonal elution affinity chromatography. Methods 146, 3–11. doi:10.1016/j.ymeth.2018.01.020
Tao, Y., Chen, Z., Zhang, Y., Wang, Y., and Cheng, Y. (2013). Immobilized magnetic beads based multi-target affinity selection coupled with high performance liquid chromatography-mass spectrometry for screening anti-diabetic compounds from a Chinese medicine “Tang-Zhi-Qing”. J. Pharm. Biomed. Anal. 7879, 190–201. doi:10.1016/j.jpba.2013.02.024
Tao, Y., Jiang, Y., Li, W., and Cai, B. (2016). Zeolite based solid-phase extraction coupled with UPLC-Q-TOF-MS for rapid analysis of acetylcholinesterase binders from crude extract of Corydalis yanhusuo. RSC Adv. 6, 98476–98486. doi:10.1039/C6RA24585D
Temporini, C., Calleri, E., Brusotti, G., and Massolini, G. (2016). Protein-labs on separative analytical scale in medicinal chemistry: From the proof of concept to applications. Curr. Org. Chem. 20, 1169–1185. doi:10.2174/1385272819666150810220825
Trindade Ximenes, I. A., de Oliveira, P. C. O., Wegermann, C. A., and de Moraes, M. C. (2021). Magnetic particles for enzyme immobilization: A versatile support for ligand screening. J. Pharm. Biomed. Anal. 204, 114286. doi:10.1016/j.jpba.2021.114286
Vandeput, M., Parsajoo, C., Vanheuverzwijn, J., Patris, S., Yardim, Y., le Jeune, A., et al. (2015). Flow-through enzyme immobilized amperometric detector for the rapid screening of acetylcholinesterase inhibitors by flow injection analysis. J. Pharm. Biomed. Anal. 102, 267–275. doi:10.1016/j.jpba.2014.09.012
Vanzolini, K. L., Vieira, L. C. C., Corrêa, A. G., Cardoso, C. L., and Cass, Q. B. (2013). Acetylcholinesterase immobilized capillary reactors–tandem mass spectrometry: An on-flow tool for ligand screening. J. Med. Chem. 56, 2038–2044. doi:10.1021/jm301732a
Vilela, A. F. L., da Silva, J. I., Vieira, L. C. C., Bernasconi, G. C. R., Corrêa, A. G., Cass, Q. B., et al. (2014). Immobilized cholinesterases capillary reactors on-flow screening of selective inhibitors. J. Chromatogr. B Anal. Technol. Biomed. Life Sci. 968, 87–93. doi:10.1016/j.jchromb.2013.11.037
Vilela, A. F. L., Frugeri, B. M., Sarria, A. L. F., Kitamura, R. O. S., Fernandes, J. B., Silva, M. F. G. F., et al. (2015). Aminonaphthoquinone mannich bases derived from lawsone and their copper(II) complex derivatives: Synthesis and potential cholinesterase inhibitors as identified by on-flow assay. J. Braz. Chem. Soc. doi:10.5935/0103-5053.20150281
Vilela, A. F. L., Seidl, C., Lima, J. M., and Cardoso, C. L. (2018). An improved immobilized enzyme reactor-mass spectrometry-based label free assay for butyrylcholinesterase ligand screening. Anal. Biochem. 549, 53–57. doi:10.1016/j.ab.2018.03.012
Wahab, R. A., Elias, N., Abdullah, F., and Ghoshal, S. K. (2020). On the taught new tricks of enzymes immobilization: An all-inclusive overview. React. Funct. Polym. 152, 104613. doi:10.1016/j.reactfunctpolym.2020.104613
Wang, H., Zhao, X., Wang, S., Tao, S., Ai, N., and Wang, Y. (2015). Fabrication of enzyme-immobilized halloysite nanotubes for affinity enrichment of lipase inhibitors from complex mixtures. J. Chromatogr. A 1392, 20–27. doi:10.1016/j.chroma.2015.03.002
Wang, L., Zhao, Y., Zhang, Y., Zhang, T., Kool, J., Somsen, G. W., et al. (2018). Online screening of acetylcholinesterase inhibitors in natural products using monolith-based immobilized capillary enzyme reactors combined with liquid chromatography-mass spectrometry. J. Chromatogr. A 1563, 135–143. doi:10.1016/j.chroma.2018.05.069
Wei, N., Zhao, J., Wu, G., Cao, W., Luo, P., Zhang, Z., et al. (2021). Rapid screening and identification of antitumor ingredients from the mangrove endophytic fungus using an enzyme-immobilized magnetic nanoparticulate system. Molecules 26, 2255. doi:10.3390/molecules26082255
Wouters, B., Currivan, S. A., Abdulhussain, N., Hankemeier, T., and Schoenmakers, P. J. (2021). Immobilized-enzyme reactors integrated into analytical platforms: Recent advances and challenges. TrAC Trends Anal. Chem. 144, 116419. doi:10.1016/j.trac.2021.116419
Wu, X., Qiu, B., Chen, Y., Shi, Y., Zhu, J., Liu, X., et al. (2020). Online coupling Fe3O4@ZIF-67@α-glucosidase biomicroreactor with high performance liquid chromatography for rapid screening of α-glucosidase inhibitors in tea and their inhibitory activity research. J. Chromatogr. B Anal. Technol. Biomed. Life Sci. 1159, 122398. doi:10.1016/j.jchromb.2020.122398
Ximenes, I. A. T., Albino, M., Sangregorio, C., Cass, Q. B., and de Moraes, M. C. (2022). On-flow magnetic particle activity assay for the screening of human purine nucleoside phosphorylase inhibitors. J. Chromatogr. A 1663, 462740. doi:10.1016/j.chroma.2021.462740
Yang, Y.-X., Li, S.-Y., Zhang, Q., Chen, H., Xia, Z.-N., and Yang, F.-Q. (2017). Characterization of phenolic acids binding to thrombin using frontal affinity chromatography and molecular docking. Anal. Methods 9, 5174–5180. doi:10.1039/C7AY01433C
Yuan, Y., Zhao, M., Riffault-Valois, L., Ennahar, S., Bergaentzlé, M., and Marchioni, E. (2020). Online acetylcholinesterase inhibition evaluation by high-performance liquid chromatography–mass spectrometry hyphenated with an immobilized enzyme reactor. J. Chromatogr. A 1609, 460506. doi:10.1016/j.chroma.2019.460506
Zhang, H., Wu, Z. Y., Yang, Y. Y., Yang, F. Q., and Li, S. P. (2019a). Recent applications of immobilized biomaterials in herbal analysis. J. Chromatogr. A 1603, 216–230. doi:10.1016/j.chroma.2019.06.059
Zhang, M., Yu, W., Zhou, S., Zhang, B., Lo, E. C. M., Xu, X., et al. (2021). In vitro antibacterial activity of an FDA-approved H+-ATPase inhibitor, bedaquiline, against Streptococcus mutans in acidic milieus. Front. Microbiol. 12, 647611. doi:10.3389/fmicb.2021.647611
Zhang, Y., Wang, Y., Tang, Y., Li, R., and Ji, Y. (2019b). An online immobilized pepsin microreactor based on polymer monoliths for screening inhibitors from natural products. Anal. Methods 11, 2465–2472. doi:10.1039/C9AY00343F
Keywords: screening, activity-based assay, affinity-based assay, on-flow, enzyme reactors
Citation: De Oliveira PCO, Lessa RC, Ceroullo MS, Wegermann CA and De Moraes MC (2022) On-flow enzymatic inhibitor screening: The emerging success of liquid chromatography-based assays. Front. Anal. Sci. 2:1004113. doi: 10.3389/frans.2022.1004113
Received: 26 July 2022; Accepted: 17 August 2022;
Published: 07 September 2022.
Edited by:
Anderson R. Moraes De Oliveira, University of São Paulo, BrazilReviewed by:
Gabriella Massolini, University of Pavia, ItalyCopyright © 2022 De Oliveira, Lessa, Ceroullo, Wegermann and De Moraes. This is an open-access article distributed under the terms of the Creative Commons Attribution License (CC BY). The use, distribution or reproduction in other forums is permitted, provided the original author(s) and the copyright owner(s) are credited and that the original publication in this journal is cited, in accordance with accepted academic practice. No use, distribution or reproduction is permitted which does not comply with these terms.
*Correspondence: Marcela Cristina De Moraes, bWNtb3JhZXNAaWQudWZmLmJy
Disclaimer: All claims expressed in this article are solely those of the authors and do not necessarily represent those of their affiliated organizations, or those of the publisher, the editors and the reviewers. Any product that may be evaluated in this article or claim that may be made by its manufacturer is not guaranteed or endorsed by the publisher.
Research integrity at Frontiers
Learn more about the work of our research integrity team to safeguard the quality of each article we publish.