- 1Department of Biology, University of Maryland, College Park, MD, United States
- 2Department of Animal and Avian Sciences, University of Maryland, College Park, MD, United States
- 3School of Biological Sciences, University of Oklahoma, Norman, OK, United States
- 4Department of Biology, University of Southern Denmark, Odense, Denmark
Location of sound sources is a fundamental task of the auditory system. Recent studies have shown that land vertebrates employ an array of sound localization strategies. We have therefore compared auditory brainstem circuits by measuring cell numbers in the cochlear nuclei in relation to brain weight among different groups of reptiles to determine if these behavioral differences are reflected in the organization of the brainstem. In extant archosaurs, the birds and crocodilians, the two ears are weakly connected pressure receivers, and sound direction is computed by binaural interactions in brain involving parallel processing of interaural time and level differences. The first-order cochlear nuclei are nucleus magnocellularis (NM) and nucleus angularis (NA). NM projects bilaterally to the nucleus laminaris (NL), where interaural time differences are computed in archosaurs. Relative to brain size, NA, NM and NL cell counts of the American alligator (Alligator mississippiensis) are similar to those of birds. Testudines (turtles and tortoises), sister group to archosaurs, are also assumed to compute sound location from binaural interactions in the brain due to weakly connected middle ears. Compared to archosaurs, NA, NM and NL of the red-eared slider (Trachemys scripta), common snapping turtle (Chelydra serpentina) and Hermann’s tortoise (Testudo hermanni) are all proportionally small. In lizards, due to the strong internal coupling of the middle ears, the cochlear nerve responses are directional, and interaural time and level differences are co-dependent and frequency dependent, suggesting that the neural processing of sound direction may be different from archosaurs. Compared to archosaurs, NM and NL of the tokay gecko (Gekko gecko) and green iguana (Iguana iguana) are proportionally small, but NA is well-developed, suggesting a greater importance of the NA pathway for the processing of the high-frequency directional information generated by the coupled ears. Snakes originated from lizard ancestors, but have secondarily lost their eardrums, and their sound localization strategies are unknown. NA and NM of the western ratsnake (Pantherophis obsoletus) are proportionally smaller than those of the lizards.
Introduction
When the terrestrial vertebrates (tetrapods) emerged in the Devonian, the adaptation of their auditory systems for air-borne hearing led to many changes, and eventually to the emergence of an evolutionary novelty, the tympanic middle ear, in the Triassic (Clack, 1997; Christensen-Dalsgaard and Carr, 2008). Prior to its appearance, early tetrapods had a bulky stapes that is hypothesized to have served as a structural support in the skull, and their ability to hear airborne sound was likely poor (Bolt and Lombard, 1985; Robinson et al., 2005; Sigurdsen, 2008; Sigurdsen and Bolt, 2010; Clack et al., 2017).
Tympanic ears appeared independently in all major tetrapod groups (amphibians, lepidosaurs, chelonians, archosaurs and mammals), and many aspects of the tympanic structures show similarities due to convergent or parallel evolution. One evident advantage of animals with tympanic ears compared to their non-tympanic ancestors is that sensitivity to sound is increased, particularly at higher frequencies. The tympanic ear also changed the directional hearing of animals, particularly in the lizards and archosaurs, and enabled different sound localization strategies. Directional hearing in all animals is based on processing of the physical cues associated with the propagating sound wave, and especially important in vertebrates are the binaural neural comparisons of the inputs from the two ears, chiefly the level of neural activity and the timing of neural activity in the two auditory nerves. The magnitude of these cues depends on the physical characteristics of the animal, most fundamentally its size. If the animal is small compared to the wavelength of sound it will not create a strong sound shadow, and therefore the interaural level difference (ILD) will be small. Similarly, the time difference between the sound wave arriving at the two ears (ITD) depends on size. However, if sound can pass from one eardrum to the other (called acoustical coupling) this interaction produces a directional response of the eardrums, and thus a much larger difference in neural activity in the two auditory nerves even in animals that are small compared to the wavelength of sound (Christensen-Dalsgaard, 2011).
Here, we review the brainstem cochlear nuclei of lizards, snakes, testudines, and archosaurs, with a focus on directional cues provided by the new tympanic ears. The tympanic ears of lizards are acoustically coupled across the pharynx, and thus highly directional (Christensen-Dalsgaard and Manley, 2005, 2008). Thus, already a simple binaural comparison in the CNS produces a lateralized response (Christensen-Dalsgaard et al., 2021). Snakes, which originated from lizard ancestors, have secondarily lost their eardrums (Rieppel, 1988). In archosaurs, by contrast, the two middle ears are joined by sinuses, creating weakly connected pressure receivers. In this group, both acoustic coupling and additional binaural interactions in the brain provide cues for computation of sound source location, mostly based on ITDs, since interaural level differences ILDs are small in small animals. In turtles, which are a sister group to archosaurs, the middle ear cavities are connected to the pharynx by relatively narrow Eustachian tubes, reducing the acoustic coupling of the eardrums. Consequently, the turtle and tortoise eardrum exhibit less directionality than lizards and archosaurs (Courte-Pinault and Christensen-Dalsgaard, 2024), and turtles may need additional computation of sound location based on binaural comparisons (Carr and Christensen-Dalsgaard, 2016; Willis and Carr, 2017).
Since sensory systems allow organisms to perceive environmental stimuli, behavioral acuity can be associated with the enlargement of the relevant brain regions (Catania and Kaas, 1995; Kubke et al., 2004). Thus, the relative size of sensory regions in the brain can reveal a species’ reliance on a particular sensory modality (Iwaniuk and Wylie, 2020). In birds, this approach has been used extensively to better understand the evolution of differences in sensory acuity and sensitivity across species (Iwaniuk et al., 2006; Wylie et al., 2015). We have compared brainstem circuits for processing cochlear input among different groups of reptiles to determine if differences among tympanic ears are reflected in the organization of the brainstem cochlear nuclei, and in sound localization strategies mentioned above. We have focused on the first order recipients of the cochlear nerve, with cell counts of the nucleus angularis (NA) and the nucleus magnocellularis (NM), and the binaural nucleus laminaris (NL) in two species of archosaurs (the barn owl and the American alligator), three species of turtles (the red-ear slider, the common snapping turtle and Hermann’s tortoise), and three species of lepidosaurs (the tokay gecko, the green iguana and the western ratsnake).
Brain size in vertebrates varies principally with body size, and relative brain size has been used to predict sensory capacity (Corfield et al., 2016; Jerison, 1973; Kubke et al., 2004; but also see Olkowicz et al., 2016). Increases in relative brain size would be expected to be costly because of the energetic cost of maintaining a larger brain mass (Laughlin et al., 1998), and the relative cost may be larger in ectothermic animals with their generally lower metabolism. There have been few studies of brain size in reptiles, apart from birds (Font et al., 2019; Kverková et al., 2022; Herculano-Houzel, 2023; Song et al., 2023). We have therefore included relative brain and brainstem sizes from our sample, in addition to our measures of neuron number in brainstem cochlear nuclei. The results show that NM was largest in the archosaurs, which compute sound source location in the brain, and smallest in lepidosaurs, which receive directional information from their coupled ears (Christensen-Dalsgaard et al., 2011). NA, NM, and NL were small in testudines compared to archosaurs, which may reflect turtles’ limited and low-frequency hearing range (Christensen-Dalsgaard et al., 2012; Willis and Carr, 2017). In lizards, NA was comparable in size to NA in birds, and larger than in turtles, while NM and NL were small relative to birds, which suggests that NA pathways may mediate processing of the high-frequency directional information generated by the coupled ear. Both NA, and in particular NM, were reduced in the western ratsnake (Han and Carr, 2023).
Materials and methods
We obtained the brain weights and the cell numbers of NM, NA and NL in two species of archosaurs [the barn owl (Tyto alba) and the American alligator (Alligator mississippiensis)], three species of turtles [the red-ear slider (Trachemys scripta), common snapping turtle (Chelydra serpentina) and Hermann’s tortoise (Testudo hermanni)], and three species of lepidosaurs [the tokay gecko (Gekko gecko), the green iguana (Iguana iguana) and the western ratsnake (Pantherophis obsoletus)]. Animals were obtained commercially and used in previous studies (Christensen-Dalsgaard et al., 2011; Carr et al., 2015; Willis and Carr, 2017; Kettler and Carr, 2019; Han and Carr, 2023). All procedures and protocols were approved by the University of Maryland Institutional Animal Care and Use Committees and complied with the National Institutes of Health Guide for the use and care of laboratory animals.
Brain and body weights
Brain and body weights for our material (Table 1) were quantified by direct weighing or obtained from literature. The weights of adult specimens were used for data analysis. We also measured the brain and body weights of 25 juvenile alligators and 45 juvenile western ratsnakes, which were not included in the data analysis. Additionally, we directly measured the weights of the forebrain and brainstem in four adult barn owls, four juvenile alligators, one Hermann’s tortoise and one tokay gecko.
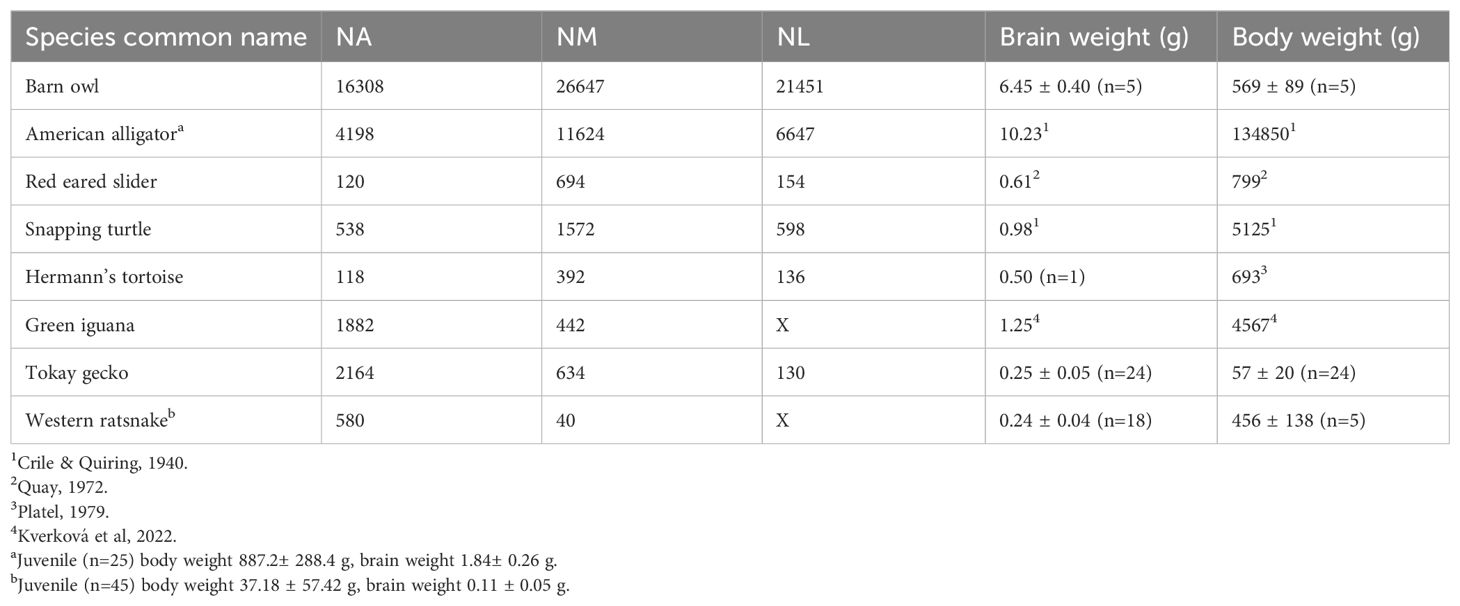
Table 1. List of reptile species surveyed, number of neurons in cochlear nuclei, and adult brain weight (g) and body weight (g).
Cell counts
Neurons were quantified by manual counting under 400x magnification or by stereological counting under 1000x magnification in cresyl-violet stained sections. The total unilateral population of neurons were counted manually for the red-ear slider, Hermann’s tortoise, tokay gecko, and green iguana following procedures described in Han and Carr (2023). In brief, we first labelled the contour of each cochlear nucleus using Neurolucida (MBF Bioscience, Williston, VT, USA) in conjunction with a light microscope (Olympus BX60), and then placed a marker in every neuron with a clear nucleolus within the nuclear boundaries to count total cell numbers. As a result of the thickness of our sections (>50 µm), overestimation of total cell number from double counting split nucleoli is negligible (Abercrombie, 1946). Neuron counts of the American alligator, barn owl and snapping turtle cochlear nuclei were obtained using the optical fractionator methods (Gundersen et al., 1999) implemented in Stereo Investigator (MBF Bioscience) in conjunction with a light microscope (Olympus BX60). Neurons were counted unilaterally for NM, NA, and NL in every second section. Neurons were counted if they contained a distinct nucleolus and intact cellular membrane. We measured the thickness of sections at each counting site as the distance between the first and last particle coming in and out of focus (West et al., 1991). For neuron counts of the barn owl and American alligator, we implemented a guard zone of 5 µm with 20 µm dissector height, 137 x 187 µm grid size and a counting frame of 80 x 80 µm. For neuron counts of the common snapping turtle, we implemented a guard zone of 5 µm with 15 µm dissector height, 60x60 µm grid size and a counting frame of 85 x 85 µm.
Data analyses
To visualize the allometric relationship between brain and body weight, we obtained values for brain weight and body weight data of lepidosaurs, turtles and archosaurs from Kverková et al. (2022), with additional species supplemented by dataset in Font et al. (2019). Brain weight and body weight were log10 transformed and plotted. Species surveyed in this study are highlighted in green and red. To visualize how neuron counts scale with brain weight in non-avian reptiles compared to birds, we obtained the cell counts of NA, NM and NL of 30 avian species with available brain weights from Winter (1963) and Winter and Schwartzkopff (1961). To demonstrate the scaling relationship between the brain weight and number of neurons in avian species, we log10 transformed brain weight and neuron numbers then performed least squares linear regression. We then calculated 95% confidence intervals for the regression lines. In this study, we used data for barn owls measured in our laboratory, instead of that of Winter and Schwartzkopff (1961). The dataset from Winter and Schwartzkopff (1961) and Winter (1963) is subject to “errors caused by the difficulty of delimiting a given nuclear area from surrounding nervous tissue (Winter, 1963)”, which has been resolved over the years. As such, we have updated the neuron count data for the barn owl. For the other avian species, Winter and Schwartzkopff (1961) and Winter (1963) remain the most complete dataset and was used for data analysis. We added lepidosaur, alligator and turtle data to the plot for comparison. Species surveyed in this study are highlighted in color.
Results
We provide a general description of the middle ear and cochlear nuclei for the animals in this study, followed by neuron counts for the brainstem cochlear nuclei.
Overview of middle ear and cochlear nuclei
Crocodilia
Pneumatized tympanic sinuses among the archosaurs couple the middle ears and create a substrate for directional sensitivity (Figure 1A) (Carr et al., 2016; Larsen et al., 2016). These sinuses have been previously described (Witmer and Ridgely, 2009; Dufeau, 2011; Bierman et al., 2014; Larsen et al., 2016) and are only briefly reviewed here in the context of their contribution to detection of sound sources. In extant archosaurs, the birds and crocodilians, the effect of coupling by interaural sinuses is greatest at low frequencies, generating both larger ILDs and effectively increasing the range of ITDs for coupled ears than would be predicted from the head size alone (Calford and Piddington, 1988; Carr et al., 2009; Bierman et al., 2014; Kettler et al., 2016; Larsen et al., 2016; Kettler and Carr, 2019). Thus, animals with large heads necessarily have a wider range of ITDs than those with small heads, and animals with coupled ears again have a wider range of ITDs than animals without coupled ears.
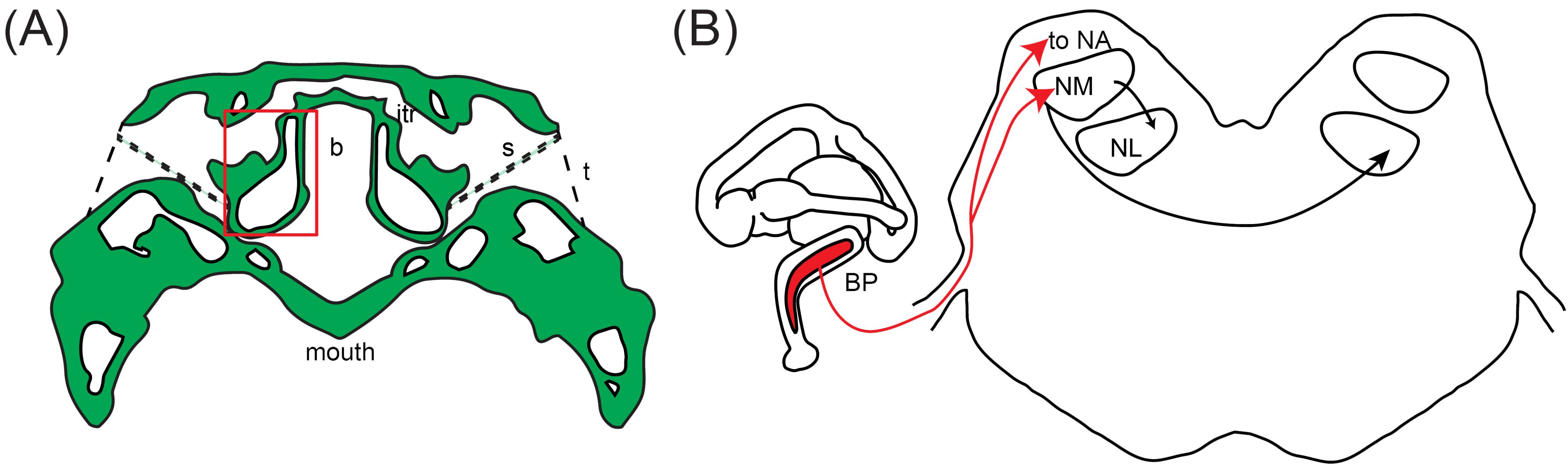
Figure 1. Schematic of the auditory structures of reptiles. (A) The middle ear, depicted in the American alligator. The middle ears of other species are listed as panel (A) in Figures 3–5. Tracing of transverse CT scan shows the connected middle ear cavities via sinuses dorsal and ventral to the brain case. from Bierman and Carr (2015). The red box indicates the otic capsule. CT scan acquired by the University of Texas High-Resolution X-ray CT Facility, courtesy of Jessie Maisano, Alan Resetar, and DigiMorph.org. itr, intertympanic recess; s, stapes; b, brain case; t, tympanum. (B) The basilar papilla (BP) and its projections to the brainstem cochlear nuclei. Schematic of the inner ear, depicted in the crocodile, is modified from Maddin and Anderson (2012). The length of the basilar papilla varies depending on the species. Schematic of a transverse section showing the cochlear nuclei of reptiles (NA, nucleus angularis; NM, nucleus magnocellularis; NL, nucleus laminaris) is modified from Walton et al., 2017.
The first order recipients of cochlear nerve projections in all diapsids, including crocodilians, are the nucleus angularis (NA) and the nucleus magnocellularis (NM; Figure 1B) (Leake, 1974). NA occupies the rostrolateral part of the acoustic tubercle (Figure 2A), with a prominent periventricular tail-like extension that extends caudally to lie over NM and NL (Figures 2B, C, arrows). This extension is both similar in location and more developed than that described in chick quail chimeras by Marín and Puelles (1995). NM is located caudal to NA (Figure 2B), and projects bilaterally to the binaural nucleus laminaris (NL; Ramón y Cajal, 1908). Among archosaurs, i.e. in alligator, emu and chicken, NL typically forms a compact layer of bitufted neurons (Figure 2C; Kubke and Carr, 2006; MacLeod et al., 2006). In owls and some songbirds, NL increases in size and loses its laminar organization (Kubke and Carr, 2006).
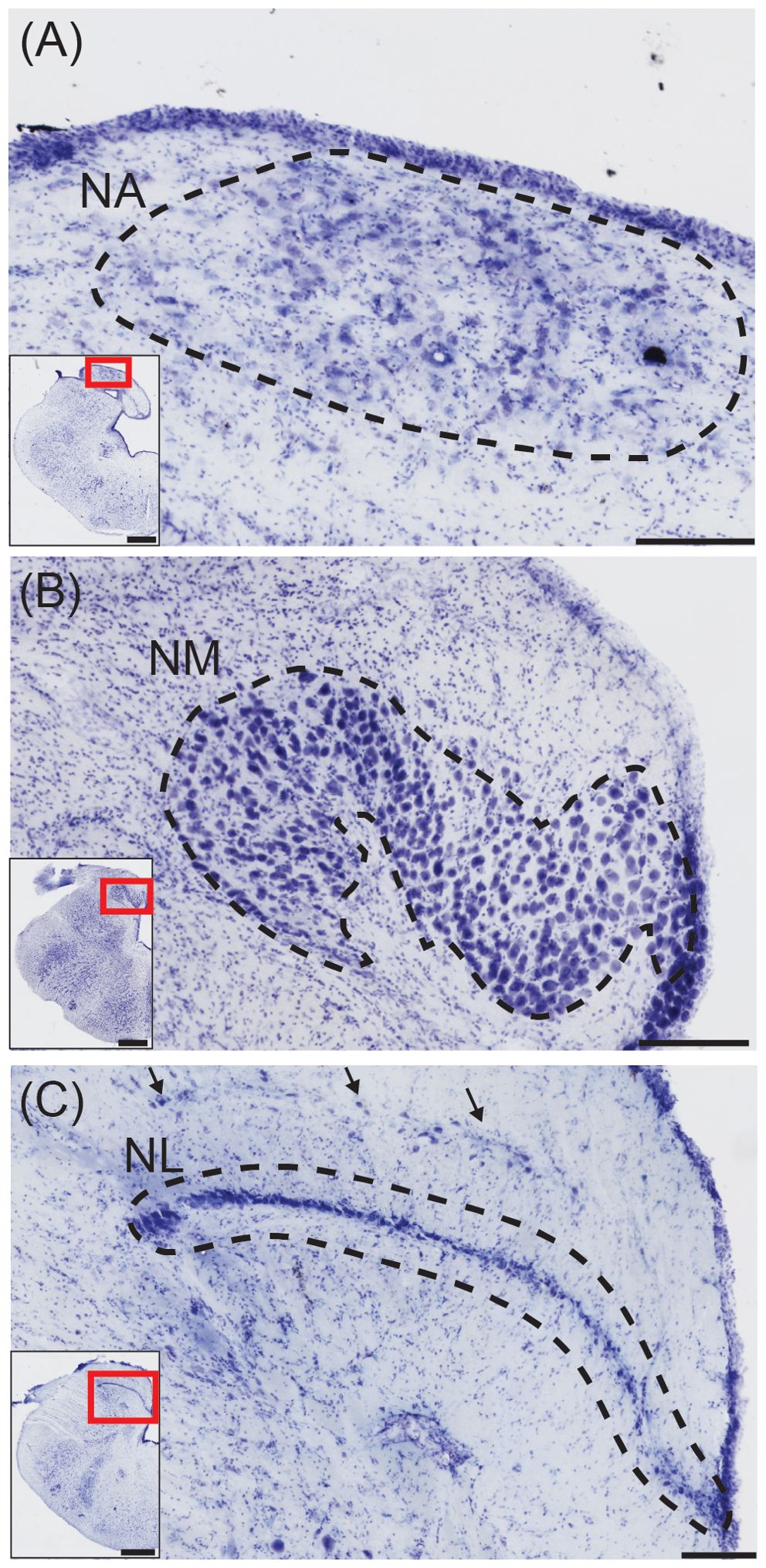
Figure 2. Structures for sound localization in the American alligator. (A) Transverse view of nucleus angularis (NA; dashed line), stained with cresyl violet. Inset: Location of NA. Scale bar = 200 μm for (A–C); scale bar = 1 mm for (A–C) insets. (B) Transverse view of nucleus magnocellularis (NM; dashed line). Inset: Location of NM. (C) Transverse view of nucleus laminaris (NM; dashed line). Inset: Location of NL.
Testudines
As stated above, the directional responses of the turtle middle ear are less than in lizards, probably demanding more binaural processing in the CNS. Given the low-frequency sensitivity of turtles (200-1000 Hz) and the strong phase locking in auditory nerve fibers at these low frequencies (Crawford and Fettiplace, 1980), it seems likely that binaural ITD comparison would be useful (Willis and Carr, 2017).
In the turtle species sampled, NA consists of sparsely distributed neurons located superficially in the acoustic tubercle caudal to the cerebellar peduncle, and rostral to NM (Figure 3B). Both NM and NL are caudal to NA, and their borders are more distinct than that of NA. In transverse sections, NM forms a crescent shape composed of large round neurons adjacent to the ventricle (Figure 3C), while NL forms a compact layer of bitufted neurons ventral to rostral NM (Figure 3D; Willis and Carr, 2017). We found little variation in the cytoarchitecture of cochlear nuclei among the turtle and tortoise species sampled, consistent with Miller and Kasahara (1979).
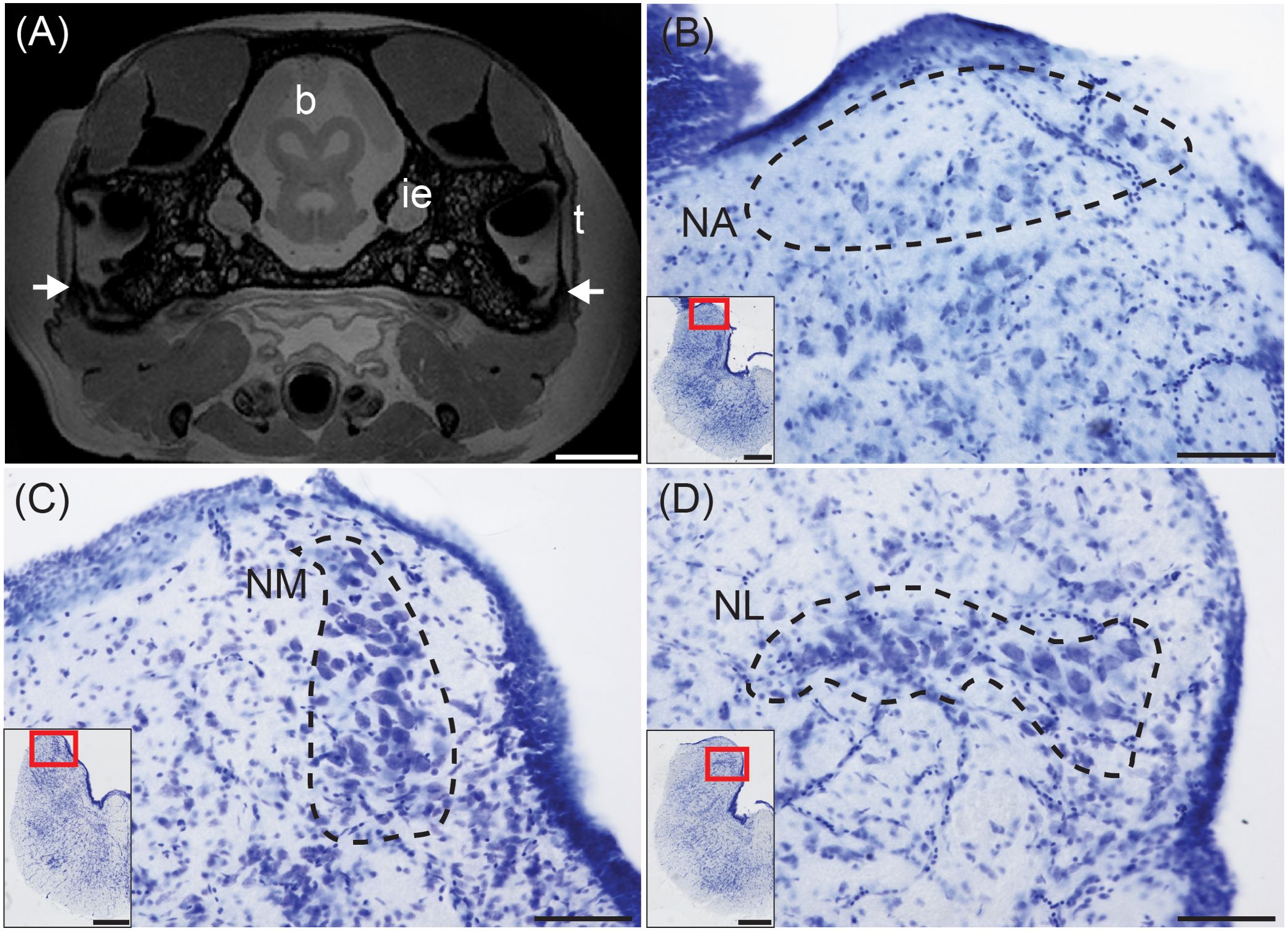
Figure 3. Structures for sound localization in the red-eared slider. (A) Transverse MRI of a red-eared slide head at the level of the rostral midbrain. Arrows indicated narrow but detectable Eustacian tubes. Scale bar = 500 mm. MR images acquired at the Armed Forces Institute of Pathology (Rockville, MD).b, brain; ie, inner ear; t, tympanum. (B) Transverse view of nucleus angularis (NA; dashed line), stained with cresyl violet. Inset: Location of NA. Scale bar = 100 μm for (B–D); scale bar = 500 μm for (B–D) insets. (C) Transverse view of nucleus magnocellularis (NM; dashed line). Inset: Location of NM. (D) Transverse view of nucleus laminaris (NM; dashed line). Inset: Location of NL.
Lepidosauria (lizards)
In extant lizards the eardrums are strongly coupled and interact acoustically to produce a strongly directional response from the tympanic membranes (Figure 4A; Christensen-Dalsgaard and Manley, 2005, 2008). The strongest directionality is produced at frequencies above 1 kHz. Recordings from cochlear nerve fibers in the tokay gecko (Gekko gecko) show that all responses are directional, and that in principle, comparison of cochlear nerve activity in the left and right cochlear nerve should give a robust indication of the animal’s direction to the sound source (Christensen-Dalsgaard et al., 2011, 2021; Christensen-Dalsgaard and Carr, 2018).
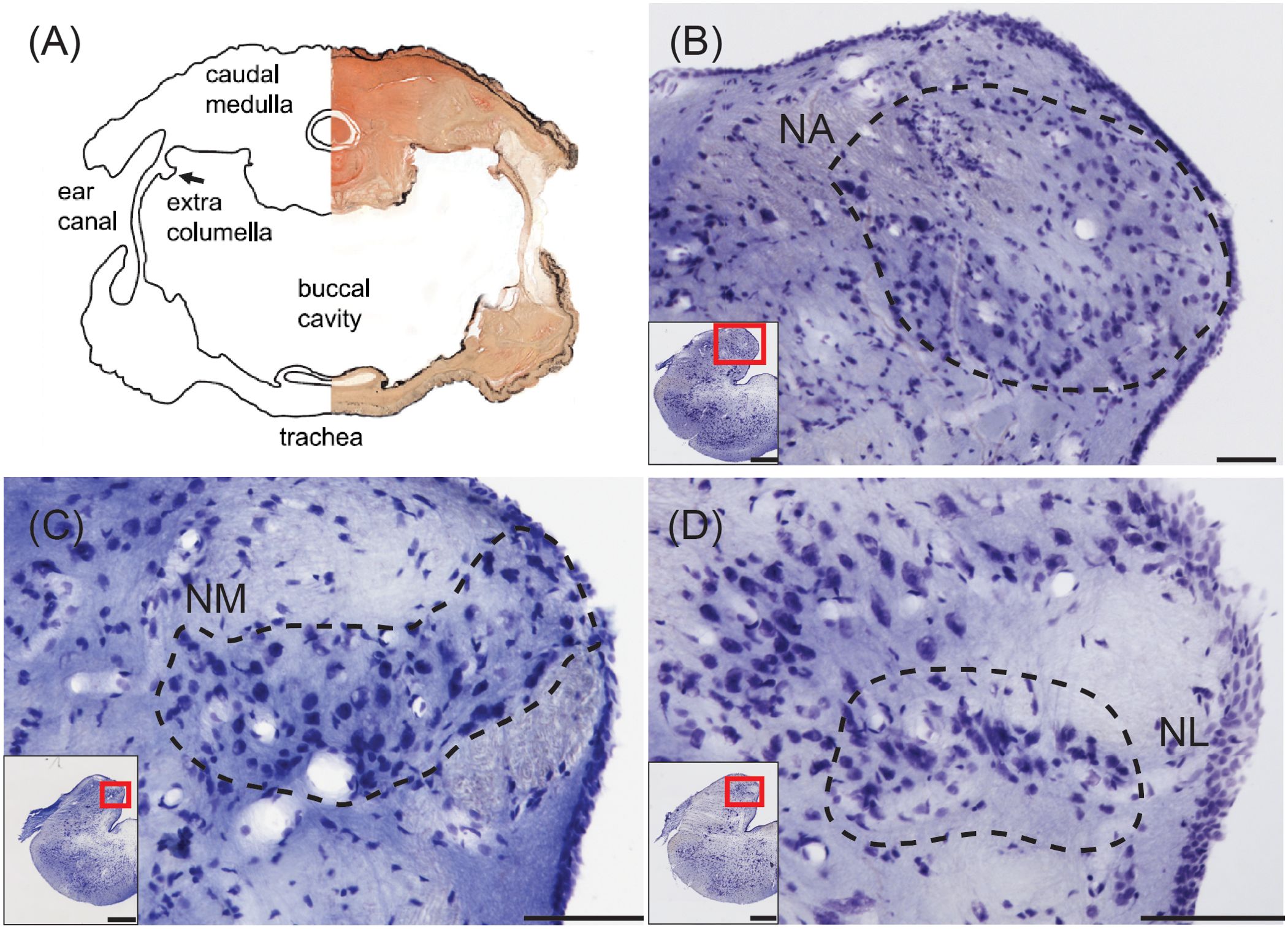
Figure 4. Structures for sound localization in the tokay gecko. (A) Combined photomicrograph and drawing of a transverse section at the level of the caudal medulla showing connected middle ears. From Christensen-Dalsgaard et al. (2011). (B) Transverse view of nucleus angularis (NA; dashed line), stained with cresyl violet. Inset: Location of NA. Scale bar = 100 μm for (B–D); scale bar = 500 μm for (B–D) insets. (C) Transverse view of nucleus magnocellularis (NM; dashed line). Inset: Location of NM. (D) Transverse view of nucleus laminaris (NM; dashed line). Inset: Location of NL.
In both the tokay gecko and the green iguana, NA is located in the rostral part of the acoustic tubercle, spanning across the mediolateral axis in transverse sections (Figure 4B). The caudally located NM forms a medial cluster or band in transverse sections (Figure 4C), and NL is either a small nucleus situated beneath rostral NM in the tokay gecko (Figure 4D), or indistinguishable in the green iguana. Miller (1975) was “never able to define an unequivocal NL in lizards” and noted that small fusiform cells ventral to NM can only be “occasionally” observed. In the tokay gecko, NL neurons were distinguished by immunoreactivity against calretinin (Yan et al., 2010), by their bitufted morphology, and by ascending connections to the torus (Yan et al., 2010; Tang et al., 2012).
Lepidosauria (snakes)
Snakes originated from lizard ancestors (Rieppel, 1988), but have secondarily lost their eardrums. Snakes lack a Eustachian tube, and the stapes/columella passes through skeletal muscle and vasculature (Figure 5A). The proximal end of the stapes expands to a footplate resting in the oval window, as in other lizards, but the distal end of the stapes is connected to the quadrate and coupled to lower jaw elements. Snakes are sensitive to low-frequency substrate vibration but can only detect airborne sound via sound-induced head vibration, i.e. bone conduction (Hartline and Campbell, 1969; Hartline, 1971; Christensen et al., 2012).
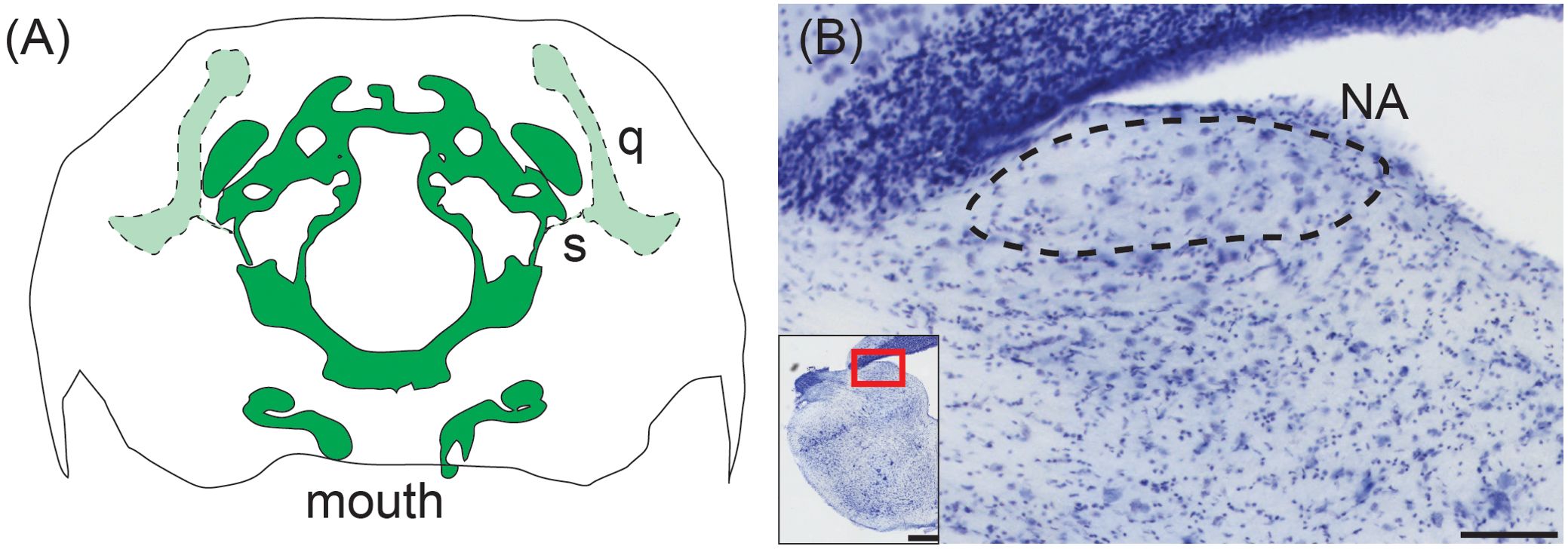
Figure 5. Middle ear and cochlear nucleus of the western ratsnake. (A) Tracing of transverse CT scan showing connection of the stapes (s) and its connection to the quadrate (q). There is no visible middle ear cavity. The stapedial footplate is in the same plane as the brain case (dark green), while the stapes and quadrate are located in more caudal sections (light green). Illustration based on CT data acquired by the University of Texas High-Resolution X-ray CT Facility for the black racer (Coluber constrictor), a close relative of the western ratsnake (Pyron et al., 2013). Data made available courtesy of Jessie Maisano, Alan Resetar, and DigiMorph.org. (B) Transverse view of nucleus angularis (NA; dashed line), stained with cresyl violet. Scale bar = 100 μm. Inset: Location of NA. Scale bar = 500 μm.
The organization of the western ratsnake (Pantherophis obsoletus) brainstem cochlear nuclei reflects this diminished sensitivity to airborne sound. The ratsnake has a distinct NA located superficially in the rostral acoustic tubercle (Figure 5B). NM, however, is indistinguishable from surrounding neuropil and can only be discerned via calbindin immunohistochemistry as a cluster of approximately 40 neurons (Han and Carr, 2023). We cannot find NL in the western ratsnake.
Differences in cell numbers among different species
The cell counts of cochlear nuclei, as well as adult brain weight and body weight of surveyed species, are listed in Table 1. N=1 for all cell counts, except for the western ratsnake where N=3. We acknowledge the potential variability caused by the small sample size. The cell counts for the barn owl obtained from stereological counts exceeded that of earlier studies (Winter and Schwartzkopff, 1961). The current barn owl counts in NM and NA are supported by counts of cross sections through the cochlear nerve, which yielded mean counts of 31,142 axons (Köppl, 1997; Köppl et al., 2000). The brain and body weight relationship of surveyed species, as well as other members in their respective clade, are illustrated in Figure 6.
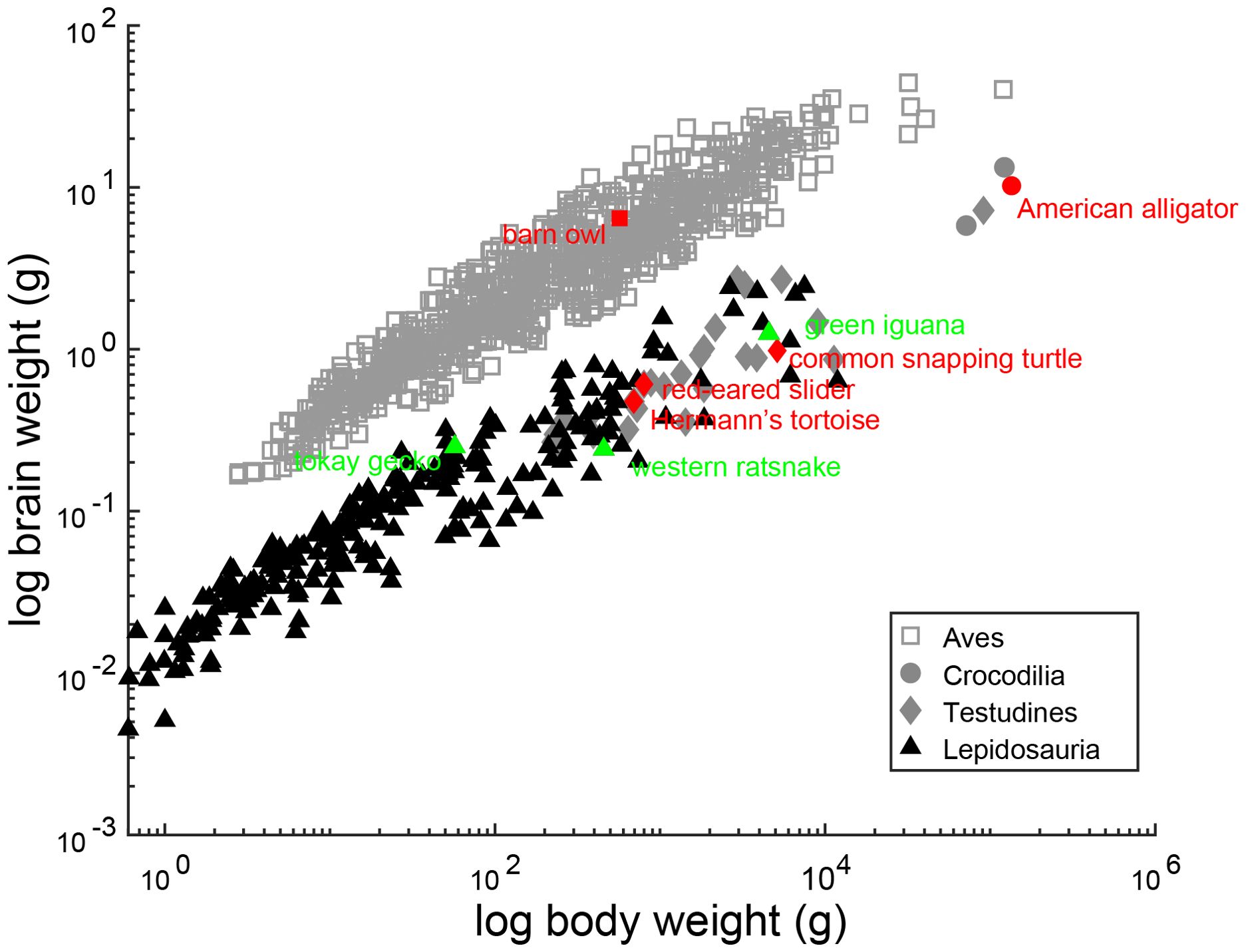
Figure 6. Allometric relationship between brain weight and body weight in reptiles. Scaling of brain weight with body weight. Scatter plots show the logarithms for brain weight as a function of the logarithms of body weight. Each data point represents an individual species. The species surveyed in this study are colored red and green and annotated, and sources for brain and body weight are listed in Table 1. Other reptile species in their respective clades are colored grey and black, and dataset for brain and body weight is from Kverková et al. (2022), supplemented by dataset compiled in Font et al. (2019).
In birds, the number of neurons in NA, NM, and NL increases with brain size (Figure 7). Neuron counts from the alligator cochlear nuclei reveal comparable numbers of neurons in NA, NM and NL to that predicted from avian data (Figure 7). Alligator NM in particular contains a large number of neurons. Neuron counts from the cochlear nuclei of the snapping turtle, red eared slider and Hermann’s tortoise reveal proportionally fewer neurons in NA, NM and NL than would be predicted from their brain weight (Figure 7). Neuron counts from both the tokay gecko and the green iguana cochlear nuclei reveal a well-developed NA comparable in size to archosaur NA (Figure 7A), and proportionally fewer neurons in NM and NL (Figures 7B, C). Neuron counts from the western ratsnake cochlear nuclei reveal a smaller NA than in comparable lizards (Figure 7A), and far fewer neurons in NM than predicted from the avian data (Figures 7B, C).
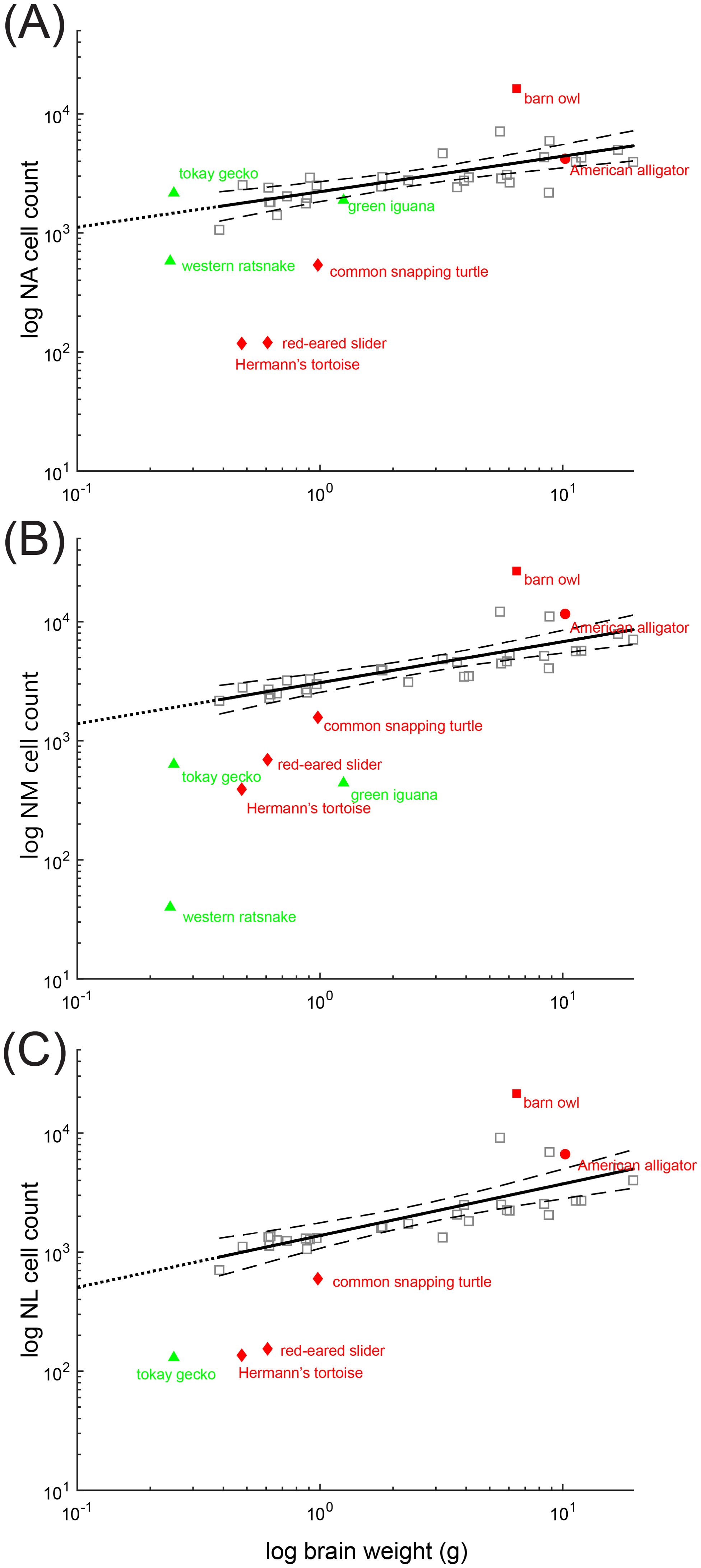
Figure 7. Scaling of neuron number in non-avian reptiles compared to birds. Scaling of neuron number with body weight. Scatter plots of the logarithm of neuron number of (A) nucleus angularis (NA), (B) nucleus magnocellularis (NM) and (C) nucleus laminaris (NL) plotted as a function of log brain weight. Each data point represents an individual species. The species surveyed in this study are colored red and green and annotated. Bird data (except the barn owl) is colored grey. Dataset for brain weight is from Kverková et al. (2022), supplemented by dataset compiled in Font et al. (2019). Neuron counts are from Winter (1963) and Winter and Schwartzkopff (1961). Solid lines indicate least squares linear regression line from bird data (including the barn owl), extended to intersect with x=101(dotted line). Dashed line indicates 95% confidence intervals.
We noted that NA cell numbers, plotted with respect to whole brain weight, were comparable among the lizards, the ratsnake, and the two archosaurs, the alligator and barn owl. This was surprising because barn owls (and other owls) have hypertrophied cochlear nuclei (Kubke et al., 2004). Since the trend might be attributed to the effects of whole brain weight, we measured both forebrain and brainstem weight of barn owls, American alligators, a Hermann’s tortoise and a tokay gecko. For adult barn owls (n=4), forebrain weight = 4.28 ± 0.78 g, brainstem weight = 1.92 ± 0.50 g. For juvenile American alligators (n=4), forebrain weight = 0.85 ± 0.13 g, brainstem weight = 0.73 ± 0.08 g. For an adult Hermann’s tortoise (n =1), forebrain weight = 0.25 g, brainstem weight = 0.25 g. For an adult tokay gecko (n=1), forebrain weight = 0.13 g, brainstem weight = 0.13 g. Even with reference to brainstem weight, as opposed to whole brain weight, the size of NA in the tokay gecko remained large, comparable to that of the avian data in Figure 7A.
Characteristics of the basilar papilla, middle ear and cochlear nuclei of different reptile groups are summarized in Table 2.
Discussion
We have compared brainstem circuits for processing cochlear input among reptiles with different sound localization strategies to determine if these differences are reflected in the first order nuclei. Overall, NM is largest in the archosaurs and turtles, which compute interaural time differences in the brain, and smallest in snakes and lepidosaurs, which receive directional information from their coupled ears. In lepidosaurs, NA is proportionally large, which suggests a greater prominence of the NA pathway for processing of the high-frequency directional information generated by their coupled ears. Both NA and NM are proportionally small in turtles.
Some clues to the differences between archosaurs and lepidosaurs might be found in their evolutionary and life history. Both the fossil record and recent developmental studies support the parallel and independent development of the tympanum in lepidosaurs and archosaurs (Sobral et al., 2016b; Clack et al., 2017; Lessner and Stocker, 2017; Müller et al., 2018). The invention of the tympanum would have led to more sensitive, higher frequency hearing in both groups, and potentially to the appearance of distinct localization strategies among these animals, because there are differences among tympanic ears. Archosaur middle ears are coupled by sinuses (Witmer and Ridgely, 2009; Bierman et al., 2014; Sobral et al., 2016b). The coupling provides some directionality at low frequencies (Moiseff and Konishi, 1981; Kettler et al., 2016; Larsen et al., 2016), and also selective pressure to enhance the central computation of sound source location through binaural comparisons. Lepidosaur middle ears are widely open to the mouth cavity, and thus highly directional (Christensen-Dalsgaard and Manley, 2005, 2008). Turtle ears are adapted to aquatic life, with narrow Eustachian tubes, and weak coupling (Courte-Pinault and Christensen-Dalsgaard, 2024).
We will discuss and review the evolution of directional hearing, the reorganization of the auditory system after formation of the tympanum, and sound localization strategies in lepidosaurs and archosaurs.
Evolution of directional hearing and the origins of NA and NM
The tetrapods and possibly their sarcopterygian ancestors are characterized by a new hearing organ, the basilar papilla or cochlea. It is not clear whether it emerged once or multiple time among the tetrapods (Lombard and Bolt, 1979; Fritzsch, 1987). Regardless of whether the new papilla occurred prior to or after the emergence of land vertebrates, its formation would have exerted a reorganizing effect on its central auditory targets including NA and NM (Wilczynski, 1984; Fritzsch, 1992, 1999). We have hypothesized that an Osteichthyan-like circuit could have been transformed to one like that found in diapsids (Walton et al., 2017). In this scenario, afferents from the new papilla might have first overlapped with the saccular input to the “auditory” portion of the descending octaval nucleus, since, in fish, auditory afferents from the saccule enter the medulla and terminate in the anterior octaval nucleus and the dorsal portions of descending octaval nucleus (Edds-Walton, 1998; Kozloski and Crawford, 1998; McCormick, 1999; Bass et al., 2001). These first order auditory nuclei may correspond to the diapsid NA and NM (Lipovsek and Wingate, 2018; Walton et al., 2017).
The early tetrapods may have retained some directional sensitivity after their transition from water to land, in the form of ‘bone conduction’, i.e. sound-induced motion of the skull. Support for this hypothesis comes from recent work on atympanate salamanders which exhibit some directional sensitivity in air (Capshaw et al., 2021, 2022). The further development of tetrapod auditory systems may reflect the increased sensitivity and frequency range conveyed by the acquisition of tympana among different lineages. The ancestral atympanate diapsids may have had a short, low-frequency sensitive papilla (Manley, 2023) much as is the case in extant atympanate amphibians, tuatara and snakes (Gans and Wever, 1976; Christensen et al., 2012; Womack et al., 2017; Capshaw et al., 2020; Han and Carr, 2023). Bone conduction might have conferred some directional sensitivity upon these low frequency auditory responses. It is unclear what form binaural comparisons might have taken in these early tetrapods. It is clear, however, because of the strong phase-locking to low-frequency stimuli, that interaural time differences would have been well represented in the auditory input to the binaural neurons. The configuration of early tetrapod second order nuclei may have begun with binaural comparisons between such low frequency inputs, either excitatory (EE) or excitatory/inhibitory (EI). In early archosaurs, the NM-NL configuration may have evolved from low-frequency comparisons to computation of ITD.
Central computation of sound source direction in lepidosaurs
In lepidosaurs, the emergence of tympanic hearing appears to have been marked by a period of “experimentation” in the organization of the lizard papilla (Köppl and Manley, 1992; Miller, 1992; Manley, 2002). Both lepidosaurs and archosaurs evolved longer, differentiated basilar papillae. Manley has proposed that lepidosaurs retained the ancestral low frequency (below 1 kHz) sensitive papilla and added new areas with responses to frequencies above 1 kHz (Manley, 2023). Typical high-frequency hearing limits of lepidosaurs do not exceed 7 kHz, although high frequency hearing (up to 18 kHz) has evolved in the pygopod geckos (Manley and Kraus, 2010). These new high best frequency regions of the papilla may have exerted a reorganizing effect on the first order nuclei, with unique cochlear nerve projections to a high best frequency region of NA (Szpir et al., 1990; Tang et al., 2012). Lower best frequency regions project to both NA and NM, in the canonical vertebrate pattern discussed above (Ryugo and Parks, 2003). Lepidosaur first-order cochlear nuclei show an increase in the size of NA in the tokay gecko and green iguana examined here, consistent with a role for NA in processing high best frequency sound.
The increased size of the brainstem cochlear nuclei in lepidosaurs with respect to, for example, turtles, may reflect the increased importance of sound and growth of the papilla (Manley, 2023). The increase in size of NA may have been driven by an increased number of high best frequency hair cells in the papilla. Lizard ears are coupled across the mouth cavity and therefore cochlear nerve responses are inherently directional, obviating the need for central computation of sound source location (Christensen-Dalsgaard et al., 2021). Furthermore, the typical archosaur circuit (see below) would not work. The archosaur circuit has excitatory delay line axons from NM that synapse upon binaural coincidence detectors in NL. The coincidence detection model supposes equivalently strong ipsi- and contralateral NM inputs converge, while the strongly lateralized neural input in the lizards should only produce weak coincidence at the frontal angles (Christensen-Dalsgaard and Carr, 2018). By comparison, binaural comparisons that use EI processing with inhibition from one side and excitation from the other, should yield directional information. This logic supports the likelihood of an NA (E) – Superior Olive (I) pathway for the binaural processing of directional information generated by the coupled ears.
While western ratsnakes lack the coupled middle ear and specialized high-frequency hair cells of lizards, their NA is relatively well-developed, which may reflect symplesiomorphy with their lizard relatives (Rieppel, 1988; Gauthier et al., 2012; Pyron et al., 2013). It is unclear whether snakes have directional sensitivity to airborne sound mediated by bone conduction, however it has been hypothesized that the snakes can localize the source of substrate vibration by computing time differences from differential vibration of the two sides of the lower jaw (Friedel et al., 2008). Behavioral evidence in the horned viper (Cerastes cerastes) supports this hypothesis (Young and Morain, 2002). While Young and Morain (2002) concluded that the horned viper uses vibrational cues to locate prey, they did not investigate which sensory systems were involved. Considering that there is poor anatomical support for ITD computation - both NM and NL are small in the western ratsnake - somatosensory pathways may provide a plausible route for vibration detection (Hartline, 1971). We note that there is also considerable variation within snake species regarding the size of acoustic tubercle, more so than other reptile clades (personal observations; Miller, 1980), and cochlear nuclei are often poorly differentiated. A survey of different snakes using neuronal tract tracing combined with behavioral and physiological testing would help to evaluate a role for directional responses to vibrational stimuli.
Central computation of sound source direction in archosaurs
In archosaurs, sensory hair cells are tonotopically organized along the basilar papilla. Crocodilians have a 5 mm-long papilla, and a relatively low upper frequency limit around 4 kHz (Klinke and Pause, 1980; Higgs et al., 2002). Avian papillae are 3 to 5 mm in length, being shorter in small species; owl papillae exceptionally reach 12 mm with an upper limit above 10 kHz (Gleich and Manley, 2000). The cochlear nerve forms a tonotopic projection from the sensory hair cells to both NA and NM (Carr and Boudreau, 1991; Köppl, 2001), and we assume that the large size of the first and second order nuclei in archosaurs is related to the increased frequency range in this group.
Archosaur middle ears are acoustically coupled through cranial cavities (Witmer, 1990; Witmer and Ridgely, 2009; Bierman et al., 2014; Kettler et al., 2016; Larsen et al., 2016) and are thus able to function in part as pressure difference receivers (van Hemmen et al., 2016). Depending on the degree of coupling, interaural sound transmission may increase the physiological ITD range by a factor of 3, as shown experimentally in birds (Moiseff and Konishi, 1981; Calford and Piddington, 1988; Hyson et al., 1994; Larsen et al., 2016) and alligators (Carr et al., 2009; Bierman et al., 2014; Bierman and Carr, 2015; Kettler and Carr, 2019). Extant archosaurs, birds and crocodilians use ITDs for sound source localization in azimuth (Bala et al., 2003; Papet et al., 2020). In those birds (barn owl, chicken, and emu) examined, and in alligators, best ITDs of ITD-sensitive neurons in NL vary systematically (MacLeod et al., 2006; Köppl and Carr, 2008; Carr et al., 2009, 2015; Kettler and Carr, 2019). The similarities between birds and alligators suggest that an ordered representation of ITD might also have been found in their common archosaur ancestor. Additionally, the size of the brainstem cochlear nuclei may reflect the requirements for computation of ITDs in archosaurs. Overall, NM and NL were largest in the archosaurs, and among birds, largest in auditory specialists like the barn owl and songbirds (Kubke et al., 2004).
Turtles are a sister group to archosaurs (Wang et al., 2013; Field et al., 2014), and do not have coupled middle ears. Instead, their middle ears are connected to the buccal cavity by thin eustachian tubes (Willis et al., 2013; Foth et al., 2019). Laser vibrometry studies further show very little directionality to the turtle and tortoise eardrum (Courte-Pinault and Christensen-Dalsgaard, 2024). The hearing range is small, from about 50 to 700 Hz (Wever and Vernon, 1956; Manley, 1970; Christensen-Dalsgaard et al., 2012; Willis and Carr, 2017) in all turtles and tortoises studied so far, including pleurodirans (Courte-Pinault and Christensen-Dalsgaard, 2024) that have high-frequency vocalizations. The low frequency hearing range can constrain sound localization, since many turtles have small heads in addition to their weakly coupled middle ears and therefore weak ILD cues. Turtles are, however, sensitive to ITDs. Physiological recordings from both NM and NL reveal ITD sensitivity, responses from the NL are binaural, and within their range of physiological ITDs (Willis and Carr, 2017). We note, however, that the cochlear nuclei of red-eared slider turtle and the Hermann’s tortoise are proportionally smaller than those of the snapping turtle, and smaller than alligators. A review of the fossil record suggests that the modern turtle ear evolved during the Early to Middle Jurassic in stem turtles broadly adapted to freshwater and terrestrial settings (Sobral et al., 2016a). Previous studies suggest that tympanic hearing in turtles evolved as a compromise between land and underwater hearing (Foth et al., 2019). It is also likely that the major modifications of the skull of turtles have constrained the evolution of their auditory apparatus.
Data availability statement
The raw data supporting the conclusions of this article will be made available by the authors, without undue reservation.
Ethics statement
The animal study was approved by University of Maryland College Park Animal Care and Use Committee. The study was conducted in accordance with the local legislation and institutional requirements.
Author contributions
DH: Conceptualization, Data curation, Formal analysis, Investigation, Methodology, Project administration, Software, Supervision, Validation, Visualization, Writing – original draft, Writing – review & editing. RF: Data curation, Formal analysis, Investigation, Methodology, Validation, Writing – review & editing. KW: Conceptualization, Data curation, Writing – review & editing. JC-D: Conceptualization, Data curation, Formal analysis, Funding acquisition, Investigation, Methodology, Project administration, Resources, Software, Supervision, Validation, Visualization, Writing – original draft, Writing – review & editing. CC: Conceptualization, Data curation, Formal analysis, Funding acquisition, Investigation, Methodology, Project administration, Resources, Software, Supervision, Validation, Visualization, Writing – original draft, Writing – review & editing.
Funding
The author(s) declare financial support was received for the research, authorship, and/or publication of this article. This research is supported by NIH grant R01DC019341 (CC) and CEBH T32DC000046E (KW) and the Grass Foundation.
Acknowledgments
We gratefully acknowledge Waisudin Kamal and Nada Abouelseoud for their assistance with cell counting and Grace Capshaw for her input on turtle ear morphology.
Conflict of interest
The authors declare that the research was conducted in the absence of any commercial or financial relationships that could be construed as a potential conflict of interest.
Publisher’s note
All claims expressed in this article are solely those of the authors and do not necessarily represent those of their affiliated organizations, or those of the publisher, the editors and the reviewers. Any product that may be evaluated in this article, or claim that may be made by its manufacturer, is not guaranteed or endorsed by the publisher.
References
Abercrombie M. (1946). Estimation of nuclear population from microtome sections. Anat Rec 94, 239–247. doi: 10.1002/ar.1090940210
Bala A. D. S., Spitzer M. W., Takahashi T. T. (2003). Prediction of auditory spatial acuity from neural images on the owl’s auditory space map. Nature 424, 771–774. doi: 10.1038/nature01835
Bass A. H., Bodnar D. A., Marchaterre M. A. (2001). Acoustic nuclei in the medulla and midbrain of the vocalizing Gulf toadfish (Opsanus beta). Brain Behav. Evol. 57, 63–79. doi: 10.1159/000047226
Bierman H. S., Carr C. E. (2015). Sound localization in the alligator. Hear Res. 329, 11–20. doi: 10.1016/j.heares.2015.05.009
Bierman H. S., Thornton J. L., Jones H. G., Koka K., Young B. A., Brandt C., et al. (2014). Biophysics of directional hearing in the American alligator (Alligator mississippiensis). J. Exp. Biol. 217, 1094–1107. doi: 10.1242/jeb.092866
Bolt J. R., Lombard R. E. (1985). Evolution of the amphibian tympanic ear and the origin of frogs. Biol. J. Linn. Soc. 24, 83–99. doi: 10.1111/j.1095-8312.1985.tb00162.x
Calford M. B., Piddington R. W. (1988). Avian interaural canal enhances interaural delay. J. Comp. Physiol. A 162, 503–510. doi: 10.1007/BF00612515
Capshaw G., Christensen-Dalsgaard J., Carr C. E. (2022). Hearing without a tympanic ear. J. Exp. Biol. 225. doi: 10.1242/jeb.244130
Capshaw G., Christensen-Dalsgaard J., Soares D., Carr C. E. (2021). Bone conduction pathways confer directional cues to salamanders. J. Exp. Biol. 224. doi: 10.1242/jeb.243325
Capshaw G., Soares D., Christensen-Dalsgaard J., Carr C. E. (2020). Seismic sensitivity and bone conduction mechanisms enable extratympanic hearing in salamanders. J. Exp. Biol. 223. doi: 10.1242/jeb.236489
Carr C. E., Boudreau R. E. (1991). Central projections of auditory nerve fibers in the barn owl. J. Comp. Neurol. 314, 306–318. doi: 10.1002/cne.903140208
Carr C. E., Christensen-Dalsgaard J. (2016). Evolutionary trends in directional hearing. Curr. Opin. Neurobiol. 40, 111–117. doi: 10.1016/j.conb.2016.07.001
Carr C. E., Christensen-Dalsgaard J., Bierman H. (2016). Coupled ears in lizards and crocodilians. Biol. Cybern 110, 291–302. doi: 10.1007/s00422-016-0698-2
Carr C. E., Shah S., McColgan T., Ashida G., Kuokkanen P. T., Brill S., et al. (2015). Maps of interaural delay in the owl’s nucleus laminaris. J. Neurophysiol. 114, 1862–1873. doi: 10.1152/jn.00644.2015
Carr C. E., Soares D., Smolders J., Simon J. Z. (2009). Detection of interaural time differences in the alligator. J. Neurosci. 29, 7978–7982. doi: 10.1523/JNEUROSCI.6154-08.2009
Catania K. C., Kaas J. H. (1995). Organization of the somatosensory cortex of the star-nosed mole. J. Comp. Neurol. 351, 549–567. doi: 10.1002/cne.903510406
Christensen C. B., Christensen-Dalsgaard J., Brandt C., Madsen P. T. (2012). Hearing with an atympanic ear: Good vibration and poor sound-pressure detection in the royal python, Python regius. J. Exp. Biol. 215, 331–342. doi: 10.1242/jeb.062539
Christensen-Dalsgaard J. (2011). Vertebrate pressure-gradient receivers. Hear Res. 273, 37–45. doi: 10.1016/j.heares.2010.08.007
Christensen-Dalsgaard J., Brandt C., Willis K. L., Christensen C. B., Ketten D., Edds-Walton P., et al. (2012). Specialization for underwater hearing by the tympanic middle ear of the turtle, Trachemys scripta elegans. Proc. R. Soc. B: Biol. Sci. 279, 2816–2824. doi: 10.1098/rspb.2012.0290
Christensen-Dalsgaard J., Carr C. E. (2008). Evolution of a sensory novelty: Tympanic ears and the associated neural processing. Brain Res. Bull. 75, 365–370. doi: 10.1016/j.brainresbull.2007.10.044
Christensen-Dalsgaard J., Carr C. (2018). Processing of directional information in the gecko auditory nerve. Acta Acustica united Acustica 104, 848–851. doi: 10.3813/AAA.919242
Christensen-Dalsgaard J., Kuokkanen P., Matthews J. E., Carr C. E. (2021). Strongly directional responses to tones and conspecific calls in the auditory nerve of the Tokay gecko, Gekko gecko. J. Neurophysiol. 125, 887–902. doi: 10.1152/jn.00576.2020
Christensen-Dalsgaard J., Manley G. A. (2005). Directionality of the lizard ear. J. Exp. Biol. 208, 1209–1217. doi: 10.1242/jeb.01511
Christensen-Dalsgaard J., Manley G. A. (2008). Acoustical coupling of lizard eardrums. JARO - J. Assoc. Res. Otolaryngol. 9, 407–416. doi: 10.1007/s10162-008-0130-2
Christensen-Dalsgaard J., Tang Y., Carr C. E. (2011). Binaural processing by the gecko auditory periphery. J. Neurophysiol. 105, 1992–2004. doi: 10.1152/jn.00004.2011
Clack J. A. (1997). The evolution of tetrapod ears and the fossil record. Brain Behav. Evol. 50, 198–212. doi: 10.1159/000113334
Clack J. A., Fay R. R., Popper A. N. (2017). “Evolution of the vertebrate ear: Evidence from the fossil record,” in Springer Handbook of Auditory Research, vol. 59. (Springer, Cham).
Corfield J. R., Long B., Krilow J. M., Wylie D. R., Iwaniuk A. N. (2016). A unique cellular scaling rule in the avian auditory system. Brain Struct. Funct. 221, 2675–2693. doi: 10.1007/s00429-015-1064-1
Courte-Pinault A., Christensen-Dalsgaard J. (2024). “In-air and underwater hearing of turtles and tortoises from four families,” in International Congress of Neuroethology Abstract, vol. 15. (Berlin).
Crawford A. C., Fettiplace R. (1980). The frequency selectivity of auditory nerve fibres and hair cells in the cochlea of the turtle. J. Physiol. 306, 79–125. doi: 10.1113/jphysiol.1980.sp013387
Dufeau D. L. (2011). The evolution of cranial pneumaticity in Archosauria: Patterns of paratympanic sinus development [Doctoral dissertation, Ohio University]. OhioLINK Electronic Theses and Dissertations Center. Available at: http://rave.ohiolink.edu/etdc/view?acc_num=ohiou1313622931.
Edds-Walton P. L. (1998). Projections of primary afferents from regions of the saccule in toadfish (Opsanus tau). Hear Res. 115, 45–60. doi: 10.1016/S0378-5955(97)00179-2
Field D. J., Gauthier J. A., King B. L., Pisani D., Lyson T. R., Peterson K. J. (2014). Toward consilience in reptile phylogeny: MiRNAs support an archosaur, not lepidosaur, affinity for turtles. Evol. Dev. 16, 189–196. doi: 10.1111/ede.12081
Font E., García-Roa R., Pincheira-Donoso D., Carazo P. (2019). Rethinking the effects of body size on the study of brain size svolution. Brain Behav. Evol. 93, 182–195. doi: 10.1159/000501161
Foth C., Evers S. W., Joyce W. G., Volpato V. S., Benson R. B. J. (2019). Comparative analysis of the shape and size of the middle ear cavity of turtles reveals no correlation with habitat ecology. J. Anat 235, 1078–1097. doi: 10.1111/joa.13071
Friedel P., Young B. A., Van Hemmen J. L. (2008). Auditory localization of ground-borne vibrations in snakes. Phys. Rev. Lett. 100, 48701. doi: 10.1103/PhysRevLett.100.048701
Fritzsch B. (1987). Inner ear of the coelacanth fish Latimeria has tetrapod affinities. Nature 327, 153–154. doi: 10.1038/327153a0
Fritzsch B. (1992). “The water-to-land transition: evolution of the tetrapod basilar papilla, middle ear, and auditory nuclei,” in The Evolutionary Biology of Hearing. Eds. Webster D. B., Popper A. N., Fay R. R. (Springer, New York), 351–375. doi: 10.1007/978-1-4612-2784-7_22
Fritzsch B. (1999). “Hearing in two worlds: Theoretical and actual adaptive changes of the aquatic and terrestrial ear for sound reception,” in Comparative hearing: Fish and amphibians. Springer handbook of auditory research, vol. 11 . Eds. Fay R. R., Popper A. N. (Springer New York, New York), 15–42. doi: 10.1007/978-1-4612-0533-3_2
Gans C., Wever E. G. (1976). Ear and hearing in Sphenodon punctatus. Proc. Natl. Acad. Sci. U.S.A. 73, 4244–4246. doi: 10.1073/pnas.73.11.4244
Gauthier J. A., Kearney M., Maisano J. A., Rieppel O., Behlke A. D. B. (2012). Assembling the squamate tree of life: Perspectives from the phenotype and the fossil record. Bull. Peabody Museum Natural History 53, 3–308. doi: 10.3374/014.053.0101
Gleich O., Manley G. A. (2000). “The hearing organ of birds and crocodilia,” in Comparative hearing: birds and reptiles. Springer handbook of auditory research, vol. 13 . Eds. Dooling R. J., Fay R. R., Popper A. N. (Springer, New York), 70–138. doi: 10.1007/978-1-4612-1182-2_3
Gundersen H. J. G., Jensen E. B. V., Kiêu K., Nielsen J. (1999). The efficiency of systematic sampling in stereology - Reconsidered. J. Microsc 193, 199–211. doi: 10.1046/j.1365-2818.1999.00457.x
Han D., Carr C. E. (2023). Central projections of auditory nerve fibers in the western rat snake (Pantherophis obsoletus). J. Comp. Neurol. 531, 1261–1273. doi: 10.1002/cne.25495
Hartline P. H. (1971). Physiological basis for detection of sound and vibration in snakes. J. Exp. Biol. 54, 349–371. doi: 10.1242/jeb.54.2.349
Hartline P. H., Campbell H. W. (1969). Auditory and vibratory responses in the midbrains of snakes. Science 163, 1221–1223. doi: 10.1126/science.163.3872.1221
Herculano-Houzel S. (2023). Theropod dinosaurs had primate-like numbers of telencephalic neurons. J. Comp. Neurol. 531, 962–974. doi: 10.1002/cne.25453
Higgs D., Brittan-Powell E., Soares D., Souza M., Carr C. E., Dooling R. J., et al. (2002). Amphibious auditory responses of the American alligator (Alligator mississipiensis). J. Comp. Physiol. A 188, 501–502. doi: 10.1007/s00359-002-0324-8
Hyson R. L., Overholt E. M., Lippe W. R. (1994). Cochlear microphonic measurements of interaural time differences in the chick. Hear Res. 81, 109–118. doi: 10.1016/0378-5955(94)90158-9
Iwaniuk A. N., Clayton D. H., Wylie D. R. W. (2006). Echolocation, vocal learning, auditory localization and the relative size of the avian auditory midbrain nucleus (MLd). Behav. Brain Res. 167, 305–317. doi: 10.1016/j.bbr.2005.09.015
Iwaniuk A. N., Wylie D. R. (2020). Sensory systems in birds: What we have learned from studying sensory specialists. J. Comp. Neurol. 528, 2902–2918. doi: 10.1002/cne.24896
Jerison H. J. (1973). Evolution of the brain and intelligence (New York: Academic Press). doi: 10.2307/4512058
Kettler L., Carr C. E. (2019). Neural maps of interaural time difference in the American alligator: A stable feature in modern Archosaurs. J. Neurosci. 39, 3882–3896. doi: 10.1523/JNEUROSCI.2989-18.2019
Kettler L., Christensen-Dalsgaard J., Larsen O. N., Wagner H. (2016). Low frequency eardrum directionality in the barn owl induced by sound transmission through the interaural canal. Biol. Cybern 110, 333–343. doi: 10.1007/s00422-016-0689-3
Klinke R., Pause M. (1980). Discharge properties of primary auditory fibres in caiman crocodilus: Comparisons and contrasts to the mammalian auditory nerve. Exp. Brain Res. 38, 137–150. doi: 10.1007/BF00236735
Köppl C. (1997). Number and axon calibres of cochlear afferents in the barn owl. Audit Neurosci. 3, 313–334.
Köppl C. (2001). Tonotopic projections of the auditory nerve to the cochlear nucleus angularis in the barn owl. J. Assoc. Res. Otolaryngol. 2, 41–53. doi: 10.1007/s101620010027
Köppl C., Carr C. E. (2008). Maps of interaural time difference in the chicken’s brainstem nucleus laminaris. Biol. Cybern 98, 541–559. doi: 10.1007/s00422-008-0220-6
Köppl C., Manley G. A. (1992). “Functional consequences of morphological trends in the evolution of lizard hearing organs,” in The evolutionary biology of hearing. Eds. Webster D. B., Popper A. N., Fay R. R. (Springer New York, New York), 489–509. doi: 10.1007/978-1-4612-2784-7_31
Köppl C., Wegscheider A., Gleich O., Manley G. A. (2000). A quantitative study of cochlear afferent axons in birds. Hear Res. 139, 123–143. doi: 10.1016/S0378-5955(99)00178-1
Kozloski J., Crawford J. D. (1998). Functional neuroanatomy of auditory pathways in the sound-producing fish Pollimyrus. J. Comp. Neurol. 401, 227–252. doi: 10.1002/(SICI)1096-9861(19981116)401:2<227::AID-CNE6>3.0.CO;2-R
Kubke M. F., Carr C. E. (2006). Morphological variation in the nucleus laminaris of birds. Int. J. Comp. Psychol. 19, 83–97. doi: 10.46867/ijcp.2006.19.01.03
Kubke M. F., Massoglia D. P., Carr C. E. (2004). Bigger brains or bigger nuclei? Regulating the size of auditory structures in birds. Brain Behav. Evol. 63, 169–180. doi: 10.1159/000076242
Kverková K., Marhounová L., Polonyiová A., Kocourek M., Zhang Y., Olkowicz S., et al. (2022). The evolution of brain neuron numbers in amniotes. Proc. Natl. Acad. Sci. U.S.A. 119. doi: 10.1073/pnas.2121624119
Larsen O. N., Christensen-Dalsgaard J., Jensen K. K. (2016). Role of intracranial cavities in avian directional hearing. Biol. Cybern 110, 319–331. doi: 10.1007/s00422-016-0688-4
Laughlin S. B., De Ruyter Van Steveninck R. R., Anderson J. C. (1998). The metabolic cost of neural information. Nat. Neurosci. 1, 36–41. doi: 10.1038/236
Leake P. A. (1974). Central projections of the statoacoustic nerve in Caiman crocodilus. Brain Behav. Evol. 10, 170–196. doi: 10.1159/000124311
Lessner E. J., Stocker M. R. (2017). Archosauriform endocranial morphology and osteological evidence for semiaquatic sensory adaptations in phytosaurs. J. Anat 231, 655–664. doi: 10.1111/joa.12668
Lipovsek M., Wingate R. J. T. (2018). Conserved and divergent development of brainstem vestibular and auditory nuclei. Elife 7, 1–25. doi: 10.7554/eLife.40232
Lombard R. E., Bolt J. R. (1979). Evolution of the tetrapod ear: an analysis and reinterpretation. Biol. J. Linn. Soc. 11, 19–76. doi: 10.1111/j.1095-8312.1979.tb00027.x
MacLeod K. M., Soares D., Carr C. E. (2006). Interaural timing difference circuits in the auditory brainstem of the Emu (Dromaius novaehollamdiae). J. Comp. Neurol. 495, 185–201. doi: 10.1002/cne.20862
Maddin H. C., Anderson J. S. (2012). Evolution of the amphibian ear with implications for Lissamphibian phylogeny: insight gained from the caecilian inner ear. Fieldiana Life Earth Sci. 5, 59–76. doi: 10.3158/2158-5520-5.1.59
Manley G. A. (1970). Comparative studies of auditory physiology in reptiles. Z Vgl Physiol. 67, 363–381. doi: 10.1007/BF00297906
Manley G. A. (2002). Evolution of structure and function of the hearing organ of lizards. J. Neurobiol. 53, 202–211. doi: 10.1002/neu.10115
Manley G. A. (2023). Evolution of diversity in the auditory papillae of reptiles. Diversity (Basel) 15. doi: 10.3390/d15060730
Manley G. A., Kraus J. E. M. (2010). Exceptional high-frequency hearing and matched vocalizations in Australian pygopod geckos. J. Exp. Biol. 213, 1876–1885. doi: 10.1242/jeb.040196
Marín F., Puelles L. (1995). Morphological fate of rhombomeres in quail/chick chimeras: A segmental analysis of hindbrain nuclei. Eur. J. Neurosci. 7, 1714–1738. doi: 10.1111/j.1460-9568.1995.tb00693.x
McCormick C. A. (1999). “Anatomy of the central auditory pathways of fish and amphibians,” in Comparative hearing: Fish and amphibians. Springer handbook of auditory research, vol. 11 . Eds. Fay R. R., Popper A. N. (Springer, New York), 155–217. doi: 10.1007/978-1-4612-0533-3_5
Miller M. R. (1975). The cochlear nuclei of lizards. J. Comp. Neurol. 159, 375–406. doi: 10.1002/cne.901590306
Miller M. R. (1980). The cochlear nuclei of snakes. J. Comp. Neurol. 192, 717–736. doi: 10.1002/cne.901920407
Miller M. R. (1992). “The evolutionary implications of the structural variations in the auditory papilla of lizards,” in The evolutionary biology of hearing. Eds. Webb J. F., Popper A. N., Fay R. R. (Springer, New york), 463–487. doi: 10.1007/978-1-4612-2784-7_30
Miller M. R., Kasahara M. (1979). The cochlear nuclei of some turtles. J. Comp. Neurol. 185, 221–235. doi: 10.1002/cne.901850202
Moiseff A., Konishi M. (1981). The owl’s interaural pathway is not involved in sound localization. J. Comp. Physiol. 144, 299–304. doi: 10.1007/BF00612561
Müller J., Bickelmann C., Sobral G. (2018). The evolution and fossil history of sensory perception in amniote vertebrates. Annu. Rev. Earth Planet Sci. 46, 495–519. doi: 10.1146/annurev-earth-082517-010120
Olkowicz S., Kocourek M., Luèan R. K., Porteš M., Fitch W. T., Herculano-Houzel S., et al. (2016). Birds have primate-like numbers of neurons in the forebrain. Proc. Natl. Acad. Sci. U.S.A. 113, 7255–7260. doi: 10.1073/pnas.1517131113
Papet L., Raymond M., Boyer N., Mathevon N., Grimault N. (2020). Crocodiles use both interaural level differences and interaural time differences to locate a sound source. J. Acoust Soc. Am. 148, EL307–EL313. doi: 10.1121/10.0001979
Pyron R. A., Burbrink F. T., Wiens J. J. (2013). A phylogeny and revised classification of Squamata, including 4161 species of lizards and snakes. BMC Evol. Biol. 13. doi: 10.1186/1471-2148-13-93
Ramón y Cajal S. (1908). Les ganglions terminaux du nerf acoustique des oiseaux. Travaux Du Laboratorio Investgationes Biológica (Madrid) 6, 195–225.
Rieppel O. (1988). “A review of the origin of snakes,” in Evolutionary biology, vol. 22 . Eds. Hecht M. K., Wallace B., Prance G. T. (Springer, Boston), 37–130. doi: 10.1007/978-1-4613-0931-4_2
Robinson J., Ahlberg P. E., Koentges G. (2005). The braincase and middle ear region of Dendrerpeton acadianum (Tetrapoda: Temnospondyli). Zool J. Linn Soc. 143, 577–597. doi: 10.1111/j.1096-3642.2005.00156.x
Ryugo D. K., Parks T. N. (2003). Primary innervation of the avian and mammalian cochlear nucleus. Brain Res. Bull. 60, 435–456. doi: 10.1016/S0361-9230(03)00049-2
Sigurdsen T. (2008). The otic region of Doleserpeton (Temnospondyli) and its implications for the evolutionary origin of frogs. Zool J. Linn Soc. 154, 738–751. doi: 10.1111/j.1096-3642.2008.00459.x
Sigurdsen T., Bolt J. R. (2010). The Lower Permian amphibamid Doleserpeton (Temnospondyli: Dissorophoidea), the interrelationships of amphibamids, and the origin of modern amphibians. J. Vertebr Paleontol 30, 1360–1377. doi: 10.1080/02724634.2010.501445
Sobral G., Reisz R., Neenan J. M., Müller J., Scheyer T. M. (2016a). “Basal Reptilians, marine Diapsids, and turtles: The flowering of reptile diversity,” in Evolution of the vertebrate ear: evidence from the fossil record. Springer handbook of the vertebrate ear, vol. 59 . Eds. Clack J. A., Fay R. R., Popper A. N. (Springer, Cham), 207–243. doi: 10.1007/978-3-319-46661-3_8
Sobral G., Sookias R. B., Bhullar B. A. S., Smith R., Butler R. J., Müller J. (2016b). New information on the braincase and inner ear of euparkeria capensis broom: Implications for diapsid and archosaur evolution. R Soc. Open Sci. 3. doi: 10.1098/rsos.160072
Song Z., Griesser M., Schuppli C., van Schaik C. P. (2023). Does the expensive brain hypothesis apply to amphibians and reptiles? BMC Ecol. Evol. 23. doi: 10.1186/s12862-023-02188-w
Szpir M. R., Sento S., Ryugo D. K. (1990). Central projections of cochlear nerve fibers in the alligator lizard. J. Comp. Neurol. 295, 530–547. doi: 10.1002/cne.902950403
Tang Y., Christensen-Dalsgaard J., Carr C. E. (2012). Organization of the auditory brainstem in a lizard, Gekko gecko. I. Auditory nerve, cochlear nuclei, and superior olivary nuclei. J. Comp. Neurol. 520, 1784–1799. doi: 10.1002/cne.23013
van Hemmen J. L., Christensen-Dalsgaard J., Carr C. E., Narins P. M. (2016). Animals and ICE: meaning, origin, and diversity. Biol. Cybern 110, 237–246. doi: 10.1007/s00422-016-0702-x
Walton P. L., Christensen-Dalsgaard J., Carr C. E. (2017). Evolution of sound source localization circuits in the nonmammalian vertebrate brainstem. Brain Behav. Evol. 90, 131–153. doi: 10.1159/000476028
Wang Z., Pascual-Anaya J., Zadissa A., Li W., Niimura Y., Huang Z., et al. (2013). The draft genomes of soft-shell turtle and green sea turtle yield insights into the development and evolution of the turtle-specific body plan. Nat. Genet. 45, 701–706. doi: 10.1038/ng.2615
West M. J., Slomianka L., Gundersen H. J. G. (1991). Unbiased stereological estimation of the total number of neurons in the subdivisions of the rat hippocampus using the optical fractionator. Anat. Rec. 231, 482–497. doi: 10.1002/ar.1092310411
Wever E. G., Vernon J. A. (1956). Sound transmission in the turtle’s ear. Proc. Natl. Acad. Sci. 42, 292–299. doi: 10.1073/pnas.42.5.292
Wilczynski W. (1984). Central neural systems subserving a homoplasous periphery. Integr. Comp. Biol. 24, 755–763. doi: 10.1093/icb/24.3.755
Willis K. L., Carr C. E. (2017). A circuit for detection of interaural time differences in the nucleus laminaris of turtles. J. Exp. Biol. 220, 4270–4281. doi: 10.1242/jeb.164145
Willis K. L., Christensen-Dalsgaard J., Ketten D. R., Carr C. E. (2013). Middle ear cavity morphology is consistent with an aquatic origin for Testudines. PloS One 8. doi: 10.1371/journal.pone.0054086
Winter P. (1963). Vergleichende qualitative und quantitative untersuchungen an der hörbahn von vögeln. Z. für Morphologie und Ökologie der Tiere 52, 365–400. doi: 10.1007/BF00408568
Winter P., Schwartzkopff J. (1961). Form und Zellzahl der akustischen Nervenzentren in der Medulla oblongata von Eulen (Striges). Experientia 17, 515–516. doi: 10.1007/BF02158630
Witmer L. M. (1990). The craniofacial air sac system of Mesozoic birds (Aves). Zool J. Linn Soc. 100, 327–378. doi: 10.1111/j.1096-3642.1990.tb01865.x
Witmer L. M., Ridgely R. C. (2009). New insights into the brain, braincase, and ear region of tyrannosaurs (Dinosauria, Theropoda), with implications for sensory organization and behavior. Anat. Rec. 292, 1266–1296. doi: 10.1002/ar.20983
Womack M. C., Christensen-Dalsgaard J., Coloma L. A., Chaparro J. C., Hoke K. L. (2017). Earless toads sense low frequencies but miss the high notes. Proc. R. Soc. B: Biol. Sci. 284, 20171670. doi: 10.1098/rspb.2017.1670
Wylie D. R., Gutierrez-Ibanez C., Iwaniuk A. N. (2015). Integrating brain, behaviour and phylogeny to understand the evolution of sensory systems in birds. Front. Neurosci. 9. doi: 10.3389/fnins.2015.00281
Yan K., Tang Y., Carr C. E. (2010). Calcium-binding protein immunoreactivity characterizes the auditory system of Gekko gecko. J. Comp. Neurol. 518, 3409–3426. doi: 10.1002/cne.22428
Keywords: audition, brain evolution, lizards, snakes, turtles, crocodilians
Citation: Han D, Fuquen RW, Willis KL, Christensen-Dalsgaard J and Carr CE (2024) Sound localization circuits in reptiles. Front. Amphib. Reptile Sci. 2:1429172. doi: 10.3389/famrs.2024.1429172
Received: 07 May 2024; Accepted: 16 August 2024;
Published: 04 September 2024.
Edited by:
Shaun Collin, La Trobe University, AustraliaReviewed by:
Leo Fleishman, Union College, United StatesJacob Engelmann, Bielefeld University, Germany
Copyright © 2024 Han, Fuquen, Willis, Christensen-Dalsgaard and Carr. This is an open-access article distributed under the terms of the Creative Commons Attribution License (CC BY). The use, distribution or reproduction in other forums is permitted, provided the original author(s) and the copyright owner(s) are credited and that the original publication in this journal is cited, in accordance with accepted academic practice. No use, distribution or reproduction is permitted which does not comply with these terms.
*Correspondence: Jakob Christensen-Dalsgaard, amNkQGJpb2xvZ3kuc2R1LmRr; Catherine E. Carr, Y2VjYXJyQHVtZC5lZHU=