- 1Wageningen Plant Research, Wageningen University & Research, Wageningen, Netherlands
- 2Institute for Sugar Beet Research (IRS), Dinteloord, Netherlands
Crop residues colonized saprophytically by necrotrophic plant pathogens are an important primary inoculum source for epidemics of foliar diseases. Residues of crops, weeds, and litter were systematically sampled in a complex crop rotation experiment. Concentrations of DNA of major pathogens of the grown crops, Alternaria solani in potato and Cercospora beticola, Ramularia beticola, and Stemphylium beticola in sugar beet, were quantified in the residues using newly developed qPCR assays. Repeated field trials gave additional insights into the dynamics of A. solani in potato foliage residues for 2 years. The overall results demonstrate that the A. solani and C. beticola colonized crop residues of their host crops initially after harvest at high densities whereas R. beticola and S. beticola were almost absent in the field. Within several months, amounts of available host residues decreased substantially and concentrations of pathogens in the remaining host residues decreased steeply. Alternative substrates, residues of non-host crops including cover crops and weeds, were colonized saprophytically by the necrotrophic pathogens A. solani and C. beticola. It can be concluded that residues of non-hosts can potentially serve as an important bridge for pathogen populations during host-free cropping seasons in crop rotation systems. These findings contribute to the development of rational crop residue management strategies aiming at disease prevention by lowering the inoculum potential in crop rotation systems.
1 Introduction
Foliar diseases of potato caused by Alternaria solani Sorauer and of sugar beet caused by Cercospora beticola Sacc., Ramularia beticola Fautr. & Lamb, or Stemphylium beticola Woudenb. & Hanse regularly lead to significant yield losses (Abuley et al., 2018; Shane and Teng, 1992; Hanse et al., 2015). Multiple fungicide applications per season are common to control the diseases (Jindo et al., 2021; Khan and Smith, 2005; Vereijssen et al., 2007). Alternaria solani infecting Solanaceae including common weeds such as Solanum nigrum but also non-solanaceous host species of Asteraceae and Fabaceae (Woudenberg et al., 2014), as well as wild cabbage (Brassica oleracea), cucumber (Cucumis sativus), and zinnia (Zinnia elegans) (reviewed by van der Waals et al., 2001), has been described. Host ranges of the pathogens C. beticola, R. beticola, and S. beticola are also narrow with sugar beet and other Chenopodiaceae including the common weed Chenopodium album, but for C. beticola and S. beticola, several other plant genera have also been described as host (Hanse et al., 2015; Knight et al., 2019). Common to all four pathogens is their necrotrophic lifestyle and life cycles that include survival on crop residues in and on soil during host-free periods.
The role of residues of host crops for survival of pathogen populations and as potential inoculum sources in subsequent host crops has been studied for many pathogen–host combinations. Examples are Venturia inaequalis in apple (MacHardy et al., 2001), toxigenic Fusarium spp. in maize and wheat (Cotton and Munkvold, 1998; Köhl et al., 2007; Mourelos et al., 2024), and A. brassicicola, A. brassicae, Mycosphaerella brassicicola, and Xanthomonas campestris pv. campestris in cabbage (Humpherson-Jones, 1989; Köhl et al., 2011). This knowledge can be used to develop crop residue management strategies to reduce inoculum survival and thus to reduce the amounts of primary pathogen inoculum in subsequent cropping seasons. For example, physical, chemical, or biological treatments of overwintering apple leaves have been investigated in apple orchards to reduce the survival of V. inaequalis in the leaf residues during winter and to delay the onset of apple scab epidemics in spring (Gomez et al., 2007).
Crop residues of infected crops can be considered as the main substrate for pathogen populations, allowing survival during host-free periods. However, other saprophytic microorganisms will compete with the pathogens for nutrients and space in the residues, and residues will be degraded during time. Saprophytic colonization of residues of non-host crops or weeds present in the field may allow an additional route for pathogen survival. An example for such a survival strategy is the colonization of residues of non-host weeds and grasses by pear-pathogenic S. vesicarium, resulting in significant contributions of such alternative inoculum sources in brown spot epidemics in pear orchards (Rossi et al., 2008; Köhl et al., 2013). Mourelos et al. (2024) also observed that soybean residues were an inoculum reservoir for Fusarium graminearum causing Fusarium head blight in wheat. They found correlations between the pathogen DNA quantified in soybean residues compared to the amount of inoculum found in the previous cereal crop, suggesting that the inoculum is perpetuating in the fields and increases the chances of infection development.
The objective of our study was to contribute to the development of rational crop residue management strategies aiming at disease prevention in crop rotation systems. The colonization of residues of host crops by A. solani, C. beticola, R. beticola, and S. beticola was quantified using species-specific qPCR assays. Residues of non-host crops and cover crops grown in rotation as well as weeds and organic litter on the soil surface were also assessed to elucidate their role in pathogen population dynamics during host-free periods in arable systems with crop rotation. Pathogen populations were measured in field plots with various crop sequences during three winter seasons without growing a main crop (except winter barley) with sampling breaks during the two corresponding growing seasons. This was executed in replicated field plots of a crop rotation field trial with potato (1), winter or spring barley, sugar beet (late harvest), carrots, potato (2), maize, sugar beet (early harvest), and spring sown onion grown in rotation in two different crop management systems, comparing common practice in the Southern parts of the Netherlands with an integrated crop management (ICM) strategy based on minimizing chemical crop control (Riemens et al., 2022).
2 Materials and methods
2.1 Fungal isolates
Fungal isolates used in the study are listed in Table 1. Isolates are from the collection of Wageningen University & Research unless indicated otherwise.
2.2 Crop rotation experiment
2.2.1 Experimental design
The field experiment started in 2020 at Wageningen University & Research, Vredepeel (51.54 N; 5.85 E) on sandy soil with an organic matter content, varying from 4.4% to 4.8%. Crops were grown according to two plant protection strategies. The reference strategy (REF) controls weeds, pests, and diseases according to common practice in the southeast region of the Netherlands. The ICM strategy was based on minimizing chemical crop protection and refraining from pesticides, which are listed by the European Union as candidates for substitution (CfS) (European Commission, 2015). For example, potato varieties with better resistance against late blight (Phytophthora infestans) were grown in ICM to minimize pesticide input and to avoid fluopicolide (CfS). Since difenoconazole as a CfS was not available for early blight control, options were limited in the first years. To control Cercospora leaf spot, no synthetic fungicides were available, since these all contained an active ingredient listed as CfS. Details of the fungicide applications in potato aiming at early blight control and in sugar beet aiming at the control of foliar diseases are given in Table 2.

Table 2. Application dates of fungicides and dose rates in (A) potato and (B) sugar beet crops in the reference cropping system (REF) and the integrated crop management (ICM) system.
The crops grown were potato, winter or spring barley, sugar beet, carrots, maize, and spring sown onion. The crop rotation was one in eight except for potato and sugar beet which was one in four. The rotation order of the crops in both strategies was potato (1), winter or spring barley, sugar beet (late harvest), carrots, potato (2), maize, sugar beet (early harvest), and spring sown onion (Figure 1; Supplementary Table 1). Cover crops depended on main crops and the crop protection strategy (Supplementary Table 1). In total, the experiment consisted of four main blocks (replicates), each split into two plots with the different cropping systems, each plot with eight sub-plots for randomly distributed crops (REF1 to REF8; ICM 1 to ICM 8). Sub-plot size was 24 m × 24 m. Disease severity of early blight in potato and Cercospora leaf spot in sugar beet was weekly estimated visually on a scale of 0%–100% of leaf area affected. The area under the disease progress curve (AUDPC) was calculated according to Madden et al. (2007) and standardized by dividing by the duration of the epidemic (days). The resulting StAUDPC values for potato and sugar beet crops grown in the different cropping systems are shown in Table 3. Symptoms of infections by R. beticola were not observed in sugar beet plots and only few symptoms typical for S. beticola infections were found.
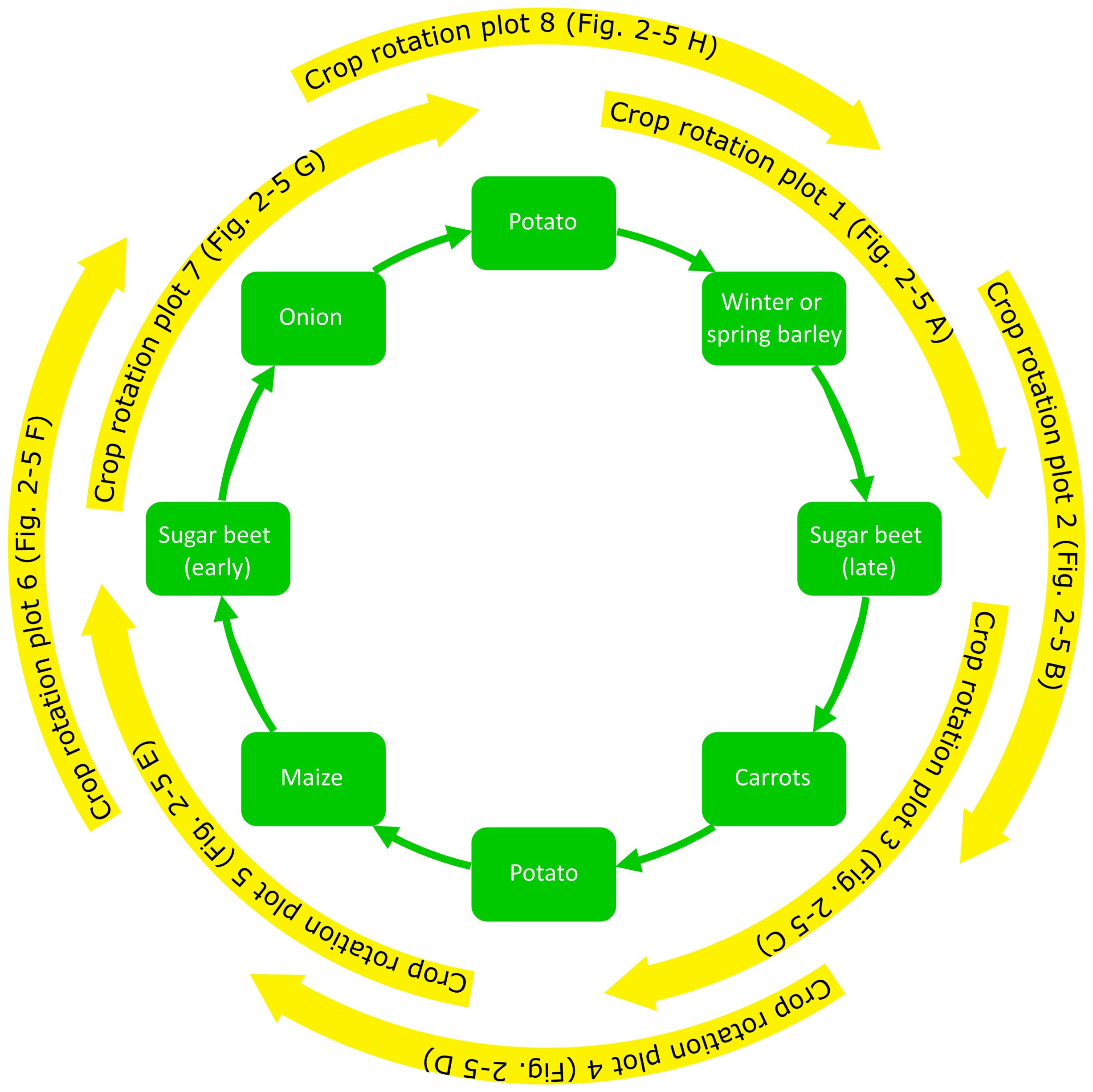
Figure 1. Diagram showing crop rotation and sampling periods in the eight crop rotation plots. Corresponding results are shown in Figures 2–5.
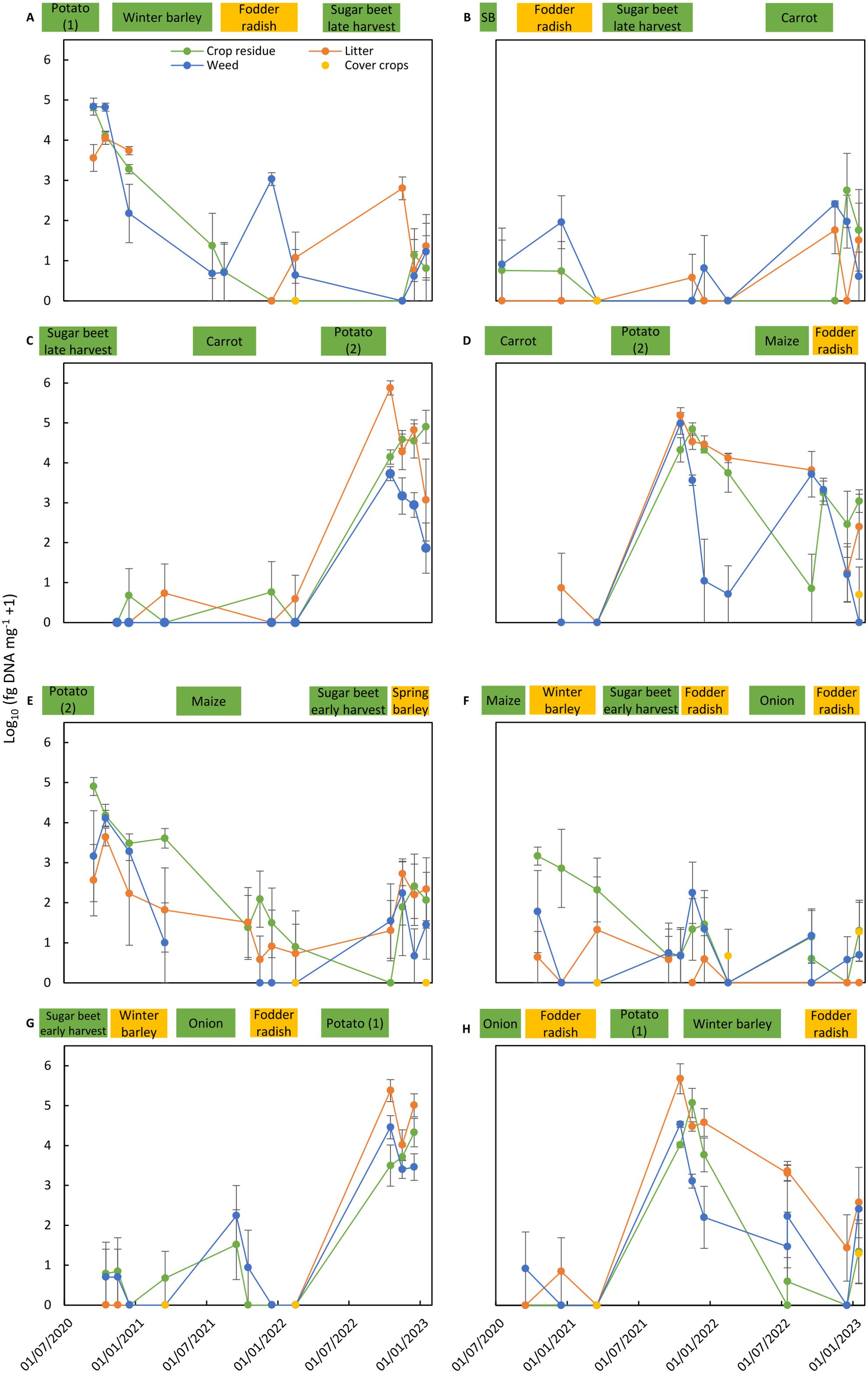
Figure 2. Colonization of residues of main crops, cover crops, and weeds and of litter by Alternaria solani in the eight crop rotation plots (A–H) of the reference cropping system (REF). Alternaria solani colonization is expressed as the mean of log10-transformed data from four replicate sub-plots (fg of DNA of A. solani mg−1 dry material − 1). Bars correspond to the standard error of the means. Cropping periods of main crops (in green) and cover crops (in yellow) in the crop rotation plot are shown on top of each graph. SB in graph B means spring barley.
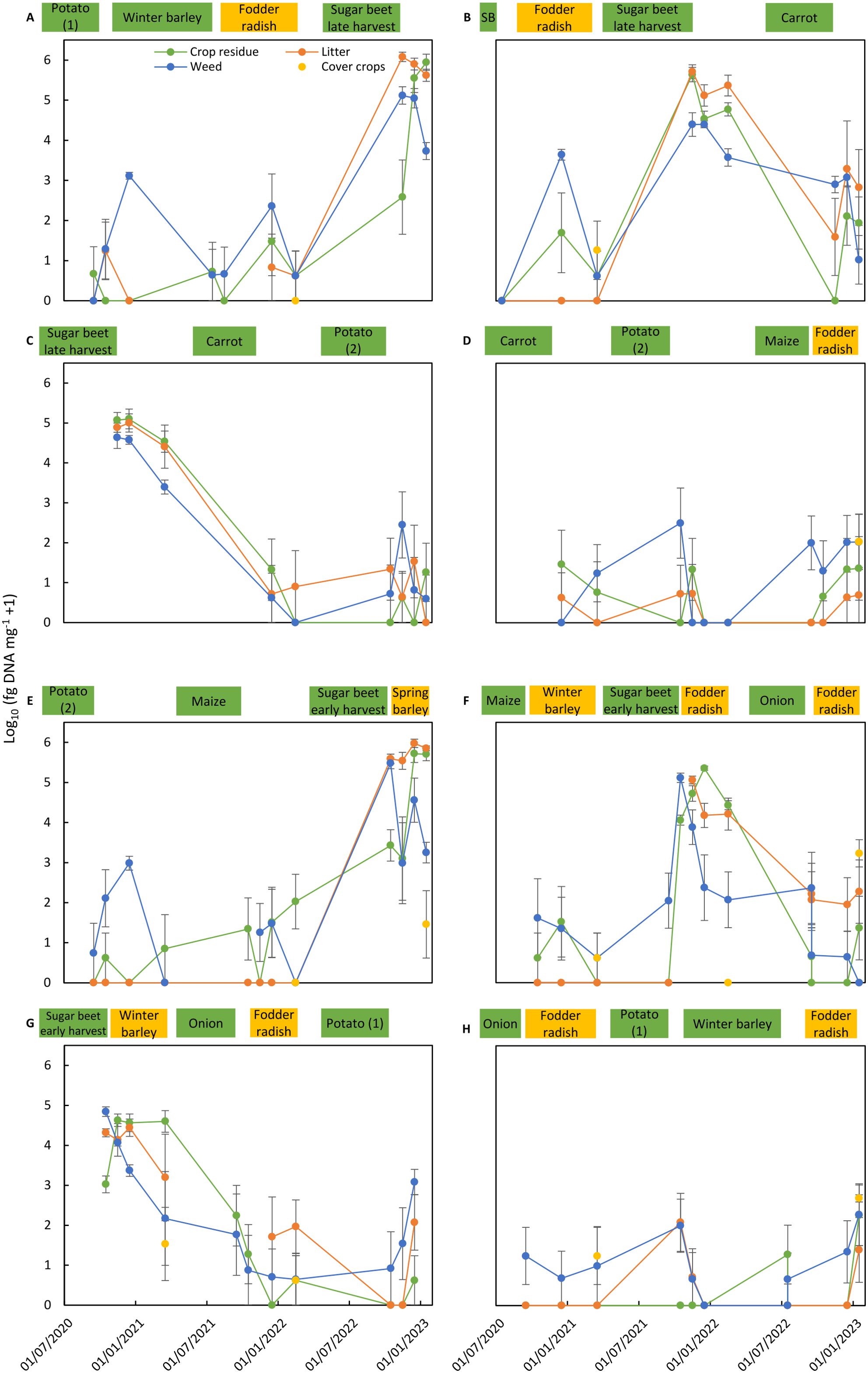
Figure 3. Colonization of residues of main crops, cover crops, and weeds and of litter by Cercospora beticola in the eight crop rotation plots (A–H) of the reference cropping system (REF). Cercospora beticola colonization is expressed as the mean of log10-transformed data from four replicate sub-plots (log10 fg of DNA of C. beticola mg−1 dry material + 1). Bars correspond to the standard error of the means. Cropping periods of main crops (in green) and cover crops (in yellow) in the crop rotation plot are shown on top of each graph. SB in graph B means spring barley.
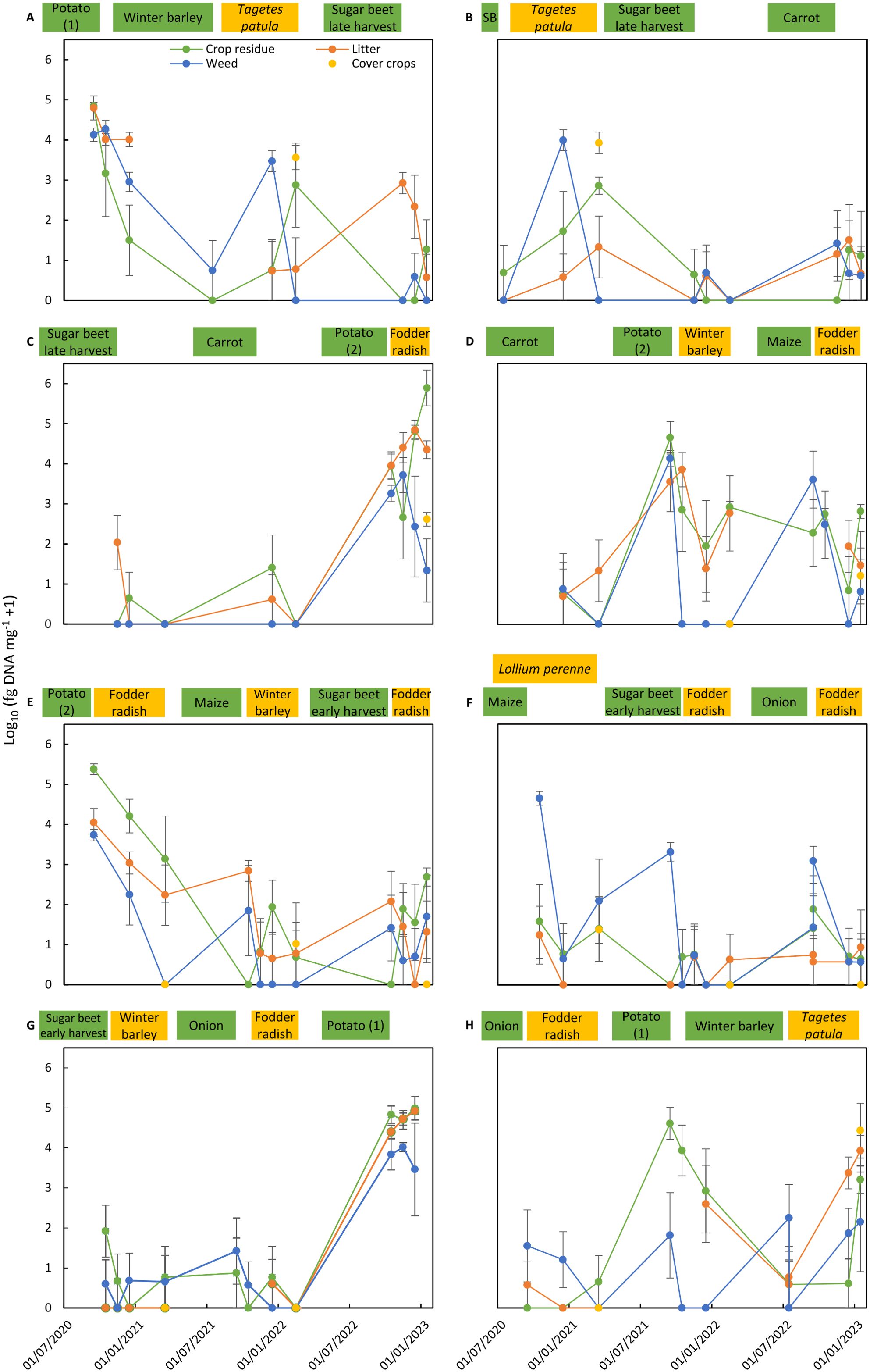
Figure 4. Colonization of residues of main crops, cover crops, and weeds and of litter by Alternaria solani in the eight crop rotation plots (A–H) of the integrated crop management system (ICM). Alternaria solani colonization is expressed as the mean of log10-transformed data from four replicate sub-plots (log10 fg of DNA of A. solani mg−1 dry material + 1). Bars correspond to the standard error of the means. Cropping period of main crops (in green) and cover crops (in yellow) in the crop rotation plot are shown on top of each graph. SB in graph B means spring barley.
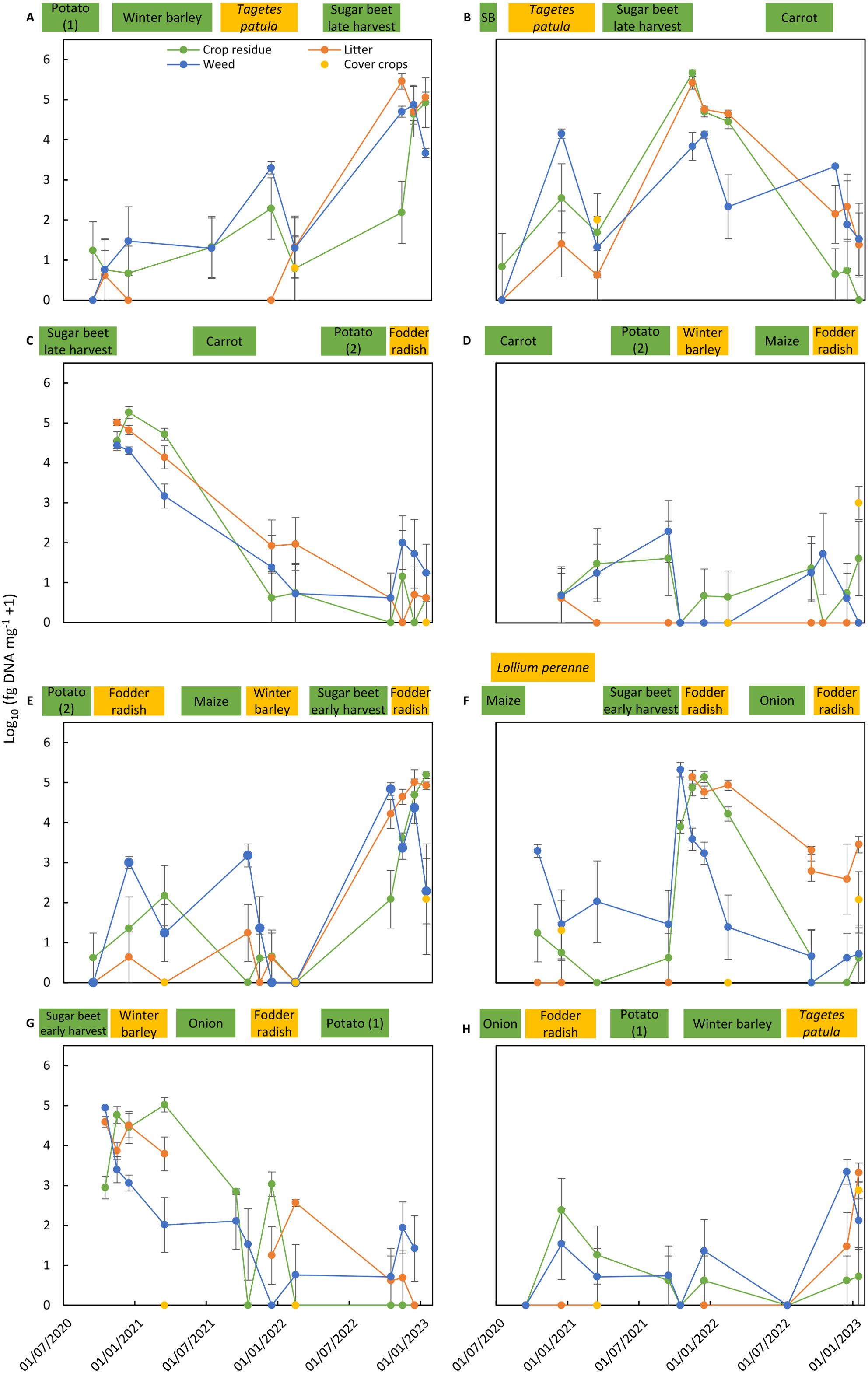
Figure 5. Colonization of residues of main crops, cover crops, and weeds and of litter by Cercospora beticola in the eight crop rotation plots (A–H) of the integrated crop management system (ICM). Cercospora beticola colonization is expressed as the mean of log10-transformed data from four replicate sub-plots (log10 fg of DNA of C. beticola mg−1 dry material + 1). Bars correspond to the standard error of the means. Cropping periods of main crops (in green) and cover crops (in yellow) in the crop rotation plot are shown on top of each graph. SB in graph B means spring barley.
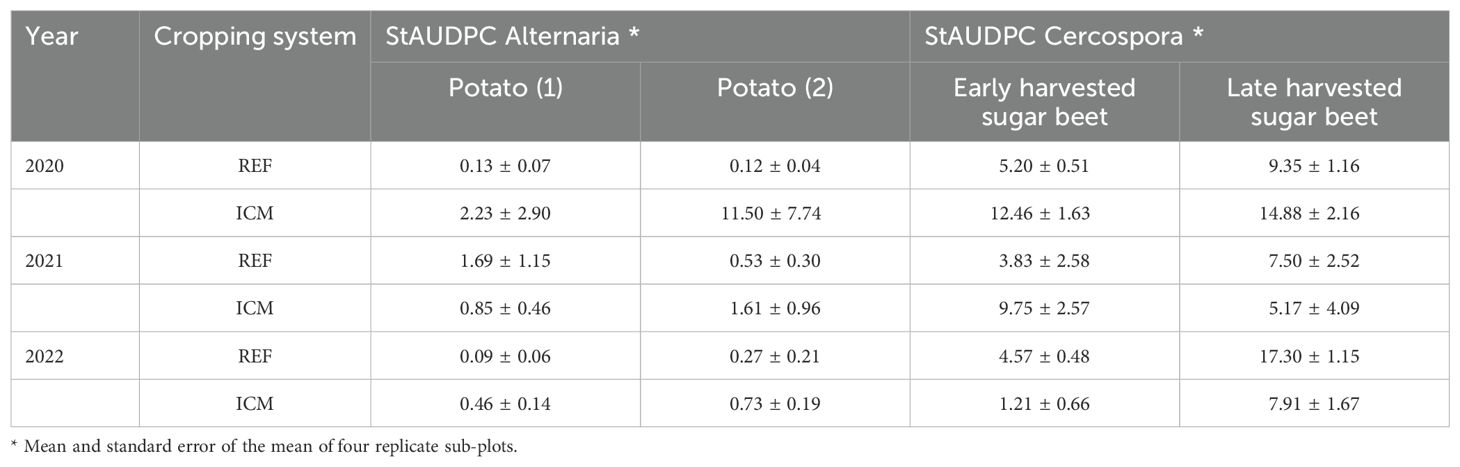
Table 3. Disease rating (StAUDPC) for early blight caused by Alternaria solani in potato crops and Cercospora leaf spot caused by Cercospora beticola in sugar beet crops grown in rotation plots in 2020–2023.
Weeds were controlled in the various crops by a combination of mechanical control and use of herbicides, depending on crop, crop stage, and control strategy (REF or ICM). Nevertheless, weed development was never completely controlled such that it always remained possible to take senescent weed samples.
2.2.2 Sampling of crop residues
Residues were sampled three or four times in a season depending on the grown crop. Samples were taken (1) directly after harvest; (2) approximately 3 weeks after harvest but before tillage or seeding of the subsequent crop; (3) at the end of the year just before the onset of winter (if possible); and (4) in spring before tillage, planting, or sowing of the subsequent crop. In some cases, 3 weeks after crop harvest coincided with the end of the year. In that case, only one sample was taken per plot at that time. Figure 1 illustrates crop rotation and sampling periods in the eight different crop rotation plots and Supplementary Table 1 gives an overview of sowing dates, harvesting dates, and sampling dates in the various crops.
Three different types of crop residues were sampled from the soil surface at each sampling time: (1) clearly recognizable debris of the grown main crop or the cover crop, (2) weed debris, and (3) litter scraped using a small blade together with some top soil from the ground surface. Only plant material that was already senescent was taken. Plant material, which was still alive, was not sampled. Samples were taken from each plot at approximately 20 different sites randomly distributed over the plot. Between sampling of the plots, the scissors, knives, and small spades used were cleaned and disinfected with 70% alcohol to prevent contamination of the samples between plots. Sample size per plot varied but was generally at least 10 g fresh weight. The samples were stored at −18°C in plastic bags.
2.2.3 Crop residue management experiment
A potato crop cv. Fontane showing early blight symptoms at the end of the season was selected as the source of potato canopy residues for the experiment. The percentage leaf surface affected by early blight (disease severity) was 7.5%. Potato canopy residues were collected at growth stage BBCH 95-97 on 19 August 2020. Residues were treated as follows: no treatment, cut in 2-cm pieces with a pruning shears, and cut in 10-cm pieces. For a fourth treatment, canopy residues were collected on a stroke of the same field where the canopy had been treated by a flailing machine, Grimme KS 3000 (Damme, Germany), 30 min before collecting the samples. Net bags (size 25 × 30 cm) were filled with subsamples of residues of each of the four treatments with approximately 250 g fresh weight per bag. In total, 40 bags per treatment were prepared and placed in a field not grown with a crop at Wageningen University & Research, Vredepeel.
There were two main plots sized 3.1 × 4.8 m, one with net bags buried horizontally in the soil at 10 cm depth and one with net bags placed on top of the soil. Within each main plot, there were five blocks (replicates), each consisting of four plots with net bags belonging to the four different treatments, arranged fully randomized within the block. In each of the plots, four bags with the same treatment were placed with 0.1-m distance between bags. One of the bags was selected randomly per sampling time, approximately every 6 months on 15 April 2021, 23 September 2021, 29 April 2022, and 23 September 2022. Ten additional samples, each with approximately 250 g fresh weight, were taken at the beginning of the experiments on 19 August 2020 from the canopy residues used for treatments 1–3 and 10 samples from the flailing-treated field stroke. All samples were stored at −20°C before processing.
The experiment was repeated in the same field. Canopy residue samples were collected on 23 September 2021 from a potato crop cv. Cammeo at growth stage BBCH 99. Early blight disease symptoms were found on 5% of the canopy surface on 15 September 2021. Sampling dates of bags containing canopy residues were 23 April 2022, 29 September 2022, 1 May 2023, and 20 September 2023.
2.3 Sample processing
Frozen field samples of plant residues of main crops, cover crops, and weed debris collected in the crop rotation experiment were shredded and subsamples of approximately 150 mL were freeze-dried using the Alpha 1-4 LSC basic (Martin Christ, Osterode am Harz, Germany). Thereafter, the dried material was pulverized using a CT 293 Cyclotec laboratory mill with a 1-mm mesh sieve (Foss, Hillerød, Denmark) and stored at −20°C.
The frozen litter samples were transferred to 1-L plastic beakers and 600 mL of tap water was added. After complete thawing, the water with the sample was vigorously stirred with a disinfected metal spoon to create an aqueous mixture and left for 1 h to completely saturate and allow sand to settle at the bottom. The supernatant, including most of the litter, was carefully poured through a funnel containing a monodur filter (200 µm). Pouring of the supernatant was stopped before sand particles could flow out. To increase the yield of litter, this process was repeated once, this time with a settling time of 10 s. The litter residues in the monodur filter were carefully rinsed with tap water to ensure all small particles were removed. The remaining litter was collected in a 15-mL Precellys tube (Bertin Technologies, Montigny-le-Bretonneux, France) and two 6.35-mm RVS beads (Vanem, Hoek van Holland, the Netherlands) were added. The tubes including the litter samples were freeze-dried using the Epsilon 1-4 LSC plus (Martin Christ, Osterorde am Harz, Germany). The freeze-dried samples were pulverized using the Precellys Evolution Homogenizer (Bertin Technologies, Montigny-le-Bretonneux, France) at 6,000 rpm for 2 × 15 s with a 10-s pause. Milled samples were stored at −20°C.
Frozen samples of the potato canopy residues collected from the crop residue management experiments were transferred to 1-L plastic beakers and 600 mL of tap water was added. After complete thawing, the water with the sample was vigorously stirred with a disinfected metal spoon to create an aqueous mixture. The complete mixture was poured on a metal sieve (mesh size, 2.8 mm) and carefully rinsed with tap water to ensure all small particles were removed. The residue was sorted out and contaminants such as weeds, insects, or material other than potato residue were removed. The remaining potato residue was collected, freeze-dried using the Alpha 1-4 LSC basic and the total dry weight was noted. Finally, the samples were pulverized in the CT 293 Cycloteca laboratory mill with a 1-mm mesh sieve and stored at −20°C.
2.4 DNA extraction
2.4.1 DNA extraction from fungal isolates
Fungal isolates were grown on potato dextrose agar (PDA) for 2 weeks at 20°C in the dark except for R. beticola isolates, which were grown for 4 weeks (Table 1). Mycelium and spores of cultured isolates were scraped from the agar surface, freeze-dried, and disrupted using the TissueLyser II (Qiagen) and one stainless bead (3.2 mm diameter) for 30 s at 30 Hz. After disruption, 200 μL of lysis buffer from the Sbeadex maxi plant kit (LGC, 175 Teddington, UK) was added to each sample and further DNA extraction was done automatically according to the protocol supplied by the manufacturer with the KingFisher™ Flex Purification System pipetting robot (Thermo Fisher Scientific, Waltham, MA, USA). DNA concentration was measured using PicoGreen in a fluorescence plate reader (TECAN).
2.4.2 DNA extraction from crop residue samples
DNA was extracted from weighted freeze-dried and pulverized crop residue samples (approximately 50 mg) using the Sbeadex maxi plant kit according to the manufacturer’s protocol with the following modifications: lysis was done at 65°C for 1 h with 700 µL of lysis buffer, and after centrifugation, 200 µL of the supernatant was used in the subsequent protocol. The KingFisher™ Flex Purification System pipetting robot was used to automate the extraction. DNA extracts were stored at −20°C.
2.5 Development of species-specific Taqman qPCR assays
Four different Taqman qPCR assays were developed for the detection and quantification of A. solani, C. beticola, R. beticola, and S. beticola. For primer and probe design, alignments were made with CLC genomics workbench 12.0 (CLC bio, Denmark) based on target and non-target Alt a 1 sequences from Woudenberg et al. (2014) for A. solani, gapdh sequences from Bakhshi et al. (2018) for C. beticola, ITS sequences from Videira et al. (2016) for R. beticola, and cmdA sequences from Woudenberg et al. (2017) for S. beticola. The species-specific primers and probe were designed with CLC genomic workbench and primer express v.3.0 (Thermo Fisher Scientific, USA).
The specificity of developed Taqman qPCR assays was first tested in silico using blastn search in NCBI and, if specific, the designed primers and probe were assessed with synthetic DNA and with 1 ng of DNA of all target and non-target fungi listed in Table 1. The test was considered as specific if, for the non-target fungi, no reactions or Ct values were measured that were higher than the Ct value for the target fungus at the lowest concentration of the dynamic range of the TaqMan PCR. The sensitivity was determined by a 10-fold dilution series from 1 ng to 10 fg for five replicates of A. solani isolate 2020Vr001, C. beticola isolate 18-539-2, R. beticola isolate 11rr04, and S. beticola isolate 14-250-1.
2.6 Taqman qPCR assays
Separate qPCR assays were performed in a 384-well format in CFX 384 (Bio-Rad) Real-Time PCR Detection System to quantify A. solani, C. beticola, R. beticola, S. beticola, and green fluorescent protein (GFP) serving as an amplification control (AC) (Klerks et al., 2004). For each TaqMan PCR, 1 μL of sample was mixed with 9 μL of reaction mix containing 5 μL of 2× PerfeCTa Toughmix no ROX (Quantabio), 100 nM probe, and 300 nM of each forward and reverse primer (Table 4). For C. beticola, 150 nM probe was used. The reaction conditions were as follows: 95°C for 2 min, 40 cycles of 95°C for 15 s, followed by 60°C for 60 s.
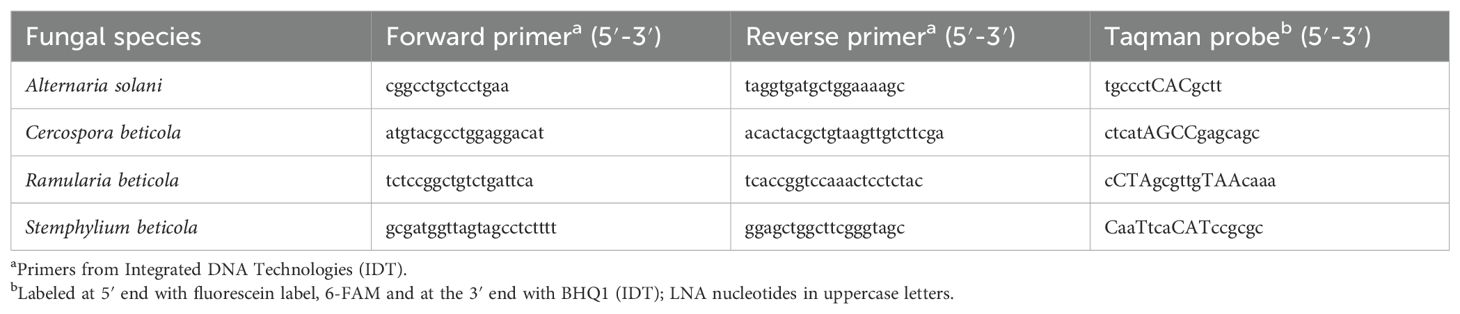
Table 4. Taqman qPCR primers and probes used for species-specific quantification of Alternaria solani, Cercospora beticola, Ramularia beticola, and Stemphylium beticola in environmental samples.
A 10-fold serial dilution range of genomic DNA was included in each 384-well plate for reference. The concentrations of extracted pathogen DNA in the samples were calculated from the derivative cycle threshold values (Ct values) of TaqMan PCRs for the DNA dilution series and for DNA extracts of plant samples, and expressed as fg of DNA of pathogen DNA per mg of plant residue (dry weight).
2.7 Statistical analysis
For the crop rotation experiment, means and standard errors of the means of the four replicates were calculated for log10-transformed DNA concentrations [fg of DNA of pathogen DNA per mg of plant residue (dry weight)] of A. solani, C. beticola, R. beticola, and S. beticola per sampling date for the different types of crop residues obtained in each crop–cropping system combination of the crop rotation experiment.
For the crop residue management experiment, means and standard errors of the means of the replicates (10 replicates at the beginning of the experiments and 5 replicates for each sampling date) were calculated for log10-transformed DNA concentrations of A. solani (expressed as fg of DNA of A. solani mg−1 dry material +1) on each experiment per sampling date, treatment, and soil surface/buried placement. DNA of A. solani was also calculated as the log10-transformed DNA concentrations remaining in the original samples placed in the bag (expressed as pg of DNA of A. solani in the remains of 1 g of dry material at the beginning of the experiment + 1). Decomposition was expressed as the means and standard errors of the means of the percent dry weight decomposed at each sampling date compared to the beginning of the experiments.
Statistical analysis was performed for the crop residue management experiment using the R software version 4.0.3 (R Core Team, 2020). For the analysis, linear mixed models as implemented in the R package version 3.1-3 lmerTest (Kuznetsova et al., 2017) were used. The responses in the analyses were respectively percent dry weight decomposed, the log10 transformation of A. solani DNA (fg of DNA of A. solani mg−1 dry material +1), and the log10 transformation of A. solani DNA present in the remains of the original samples placed in the bags [expressed as pg of A.s. DNA initial g−1 canopy residue (dw)]. The assumptions of the underlying model were checked with diagnostic plots. In order to include the initial amount of both DNA responses at the beginning of the experiments, the responses were transformed into the difference between the mean amount at the beginning and the actual measured amount at the four sampling dates. Percent dry weight decomposed was analysed as is. The model included the treatment as well as the placement as nominal variables in the fixed part of the model. Additionally, the four sampling times were included as a factor in the model. Initially, the model(s) also comprised the interactions between these 3 factors. However, they were reduced to a more parsimonious model when (some or all of) the interactions were not significantly contributing to the model. The random part of the model(s) accommodates corrections for the experimental design and controls for the variation associated with the experiments and the blocks nested within the experiments.
3 Results
3.1 Specificity and sensitivity of Taqman qPCR assays
Primers and probes developed for the quantification of A. solani, C. beticola, R. beticola, or S. beticola are given in Table 4. The specificities of the four qPCR assays were evaluated in silico by blastn research (https://www.ncbi.nlm.nih.gov/BLAST). The DNA of the host plants Solanum tuberosum (biosample SAMN02981305) and Beta vulgaris subsp. vulgaris (biosample SAMN23167344) could not be detected by the different primers and probes. Blast search of R. beticola and S. beticola-specific primers and probes gave a 100% match with the respective target and no match with the closest related species. In contrast, primers and probe for the detection of the A. solani were not specific to the targeted species but also detected A. alternariacida sp. nov, A. catananches sp. nov., A. cichorii, A. conidiophora, A. dichondrae, A. grandis, A. linicola, and A. protenta. For C. beticola, there was also a 100% match with C. plantaginis.
The specificities of the four developed Taqman qPCR assays were confirmed with synthetic DNA of the targets and the phylogenetically closest non-targets and genomic DNA (1 ng) of isolates listed in Table 1. Positive Taqman qPCR results were found for the respective assay with all targeted eight A. solani, six C. beticola, five R. beticola, or five S. beticola isolates. For non-targeted hyphal fungi and yeasts listed in Table 1, Ct values were higher than 40 or higher than Ct values of the lowest target concentration (see below). Calibration curves each consisting of five replicate dilution series of DNA of its target were used to determine the sensitivity. Linearity, efficiency, and limit of detection were estimated from the standard curves of A. solani 2020VR001, C. beticola 18-539-2, and S. beticola GV14-250-1 ranging from 1,000 to 0.1 pg of genomic DNA per reaction and from 1,000 to 0.01 pg for R. beticola 11rr04 (Supplementary Figure 1).
3.2 Population dynamics of Alternaria solani, Cercospora beticola, Ramularia beticola, and Stemphylium beticola in crop residues in crop rotation systems
Alternaria solani was consistently detected in 80% to 100% of the assessed samples of residues of the two potato crops at high concentrations above 10,000 fg of A.s. DNA mg−1 (Tables 5, 6). The pathogen was also consistently found in the litter on the soil surface after potato crops. Residue samples of weeds collected after potato crops contained for 50%–80% A. solani, but colonization was less compared to pathogen concentrations present in potato residues. The incidence of A. solani, defined as percentage of samples with positive detection of the targeted pathogen, in crop residue samples of barley, sugar beet, carrot, maize, or onion plots ranged between 10% and 60%, and mean concentrations of the pathogen were three to four orders of magnitudes lower compared to concentrations in potato residues collected during the first months after potato harvest. A similar pattern was observed in residues of weeds and in litter samples obtained from the same plots. Maximum concentrations indicate that in exceptional cases, crop residues, weed residues, or litter from plots not grown with potato but other crops contained A. solani at high concentrations at 1,000 to 100,000 fg of A.s. DNA mg−1. In residues of fodder radish, winter barley, and Lolium perenne grown as cover crops, the incidence of A. solani in samples was 25% or lower with only few cases of concentrations above 100 fg of A.s. DNA mg−1. A distinct exception was found for Tagetes patula, grown as a cover crop after winter or spring barley in the ICM cropping system. A. solani was detected in all samples of T. patula residues at a high mean concentration of 13,000 fg of A.s. DNA mg−1, similar to concentrations measured in residues of potato.
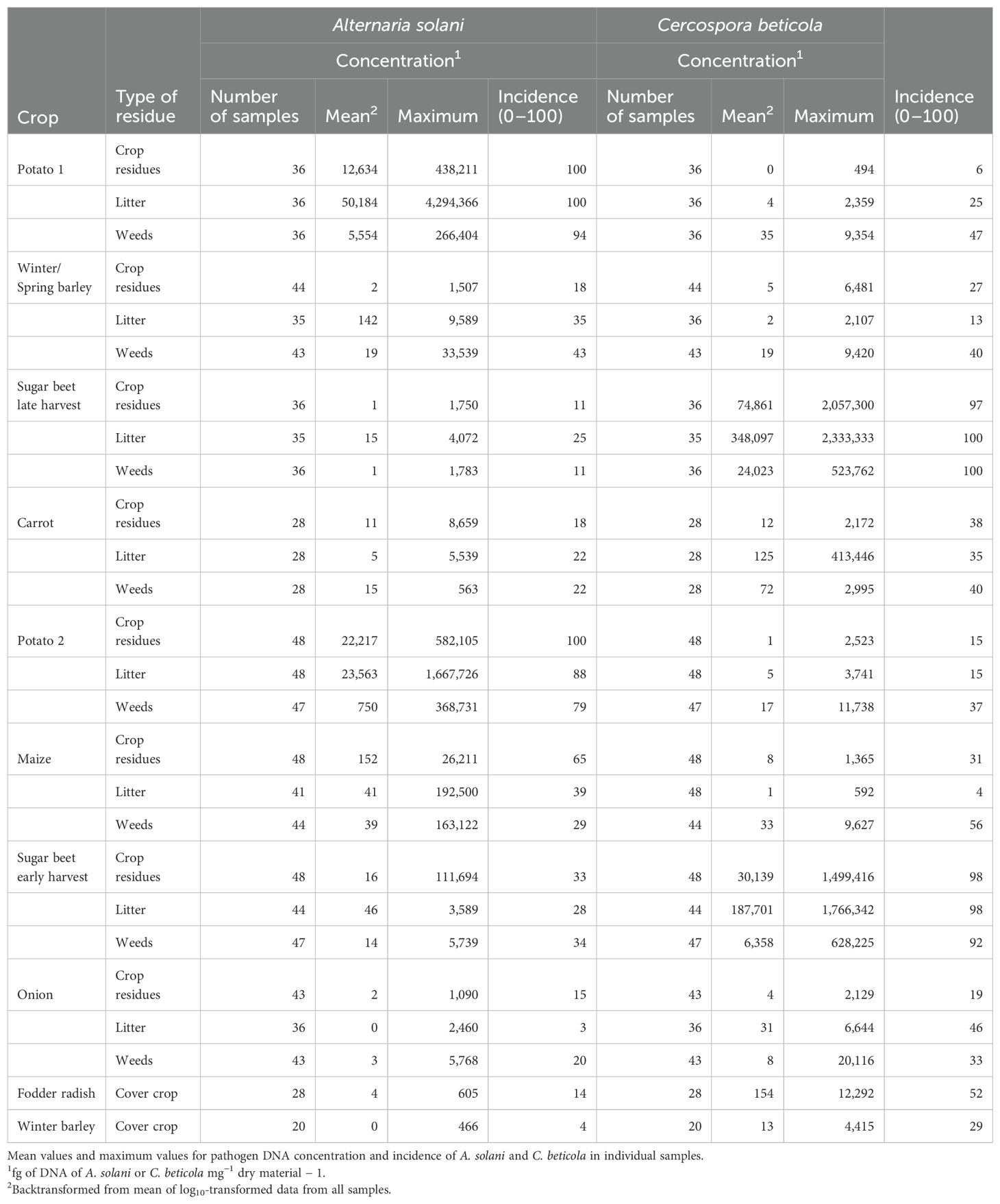
Table 5. Colonization of residues of main crops, cover crops, and weeds and of litter by Alternaria solani and Cercospora beticola in crops grown with the reference cropping system strategy (REF).
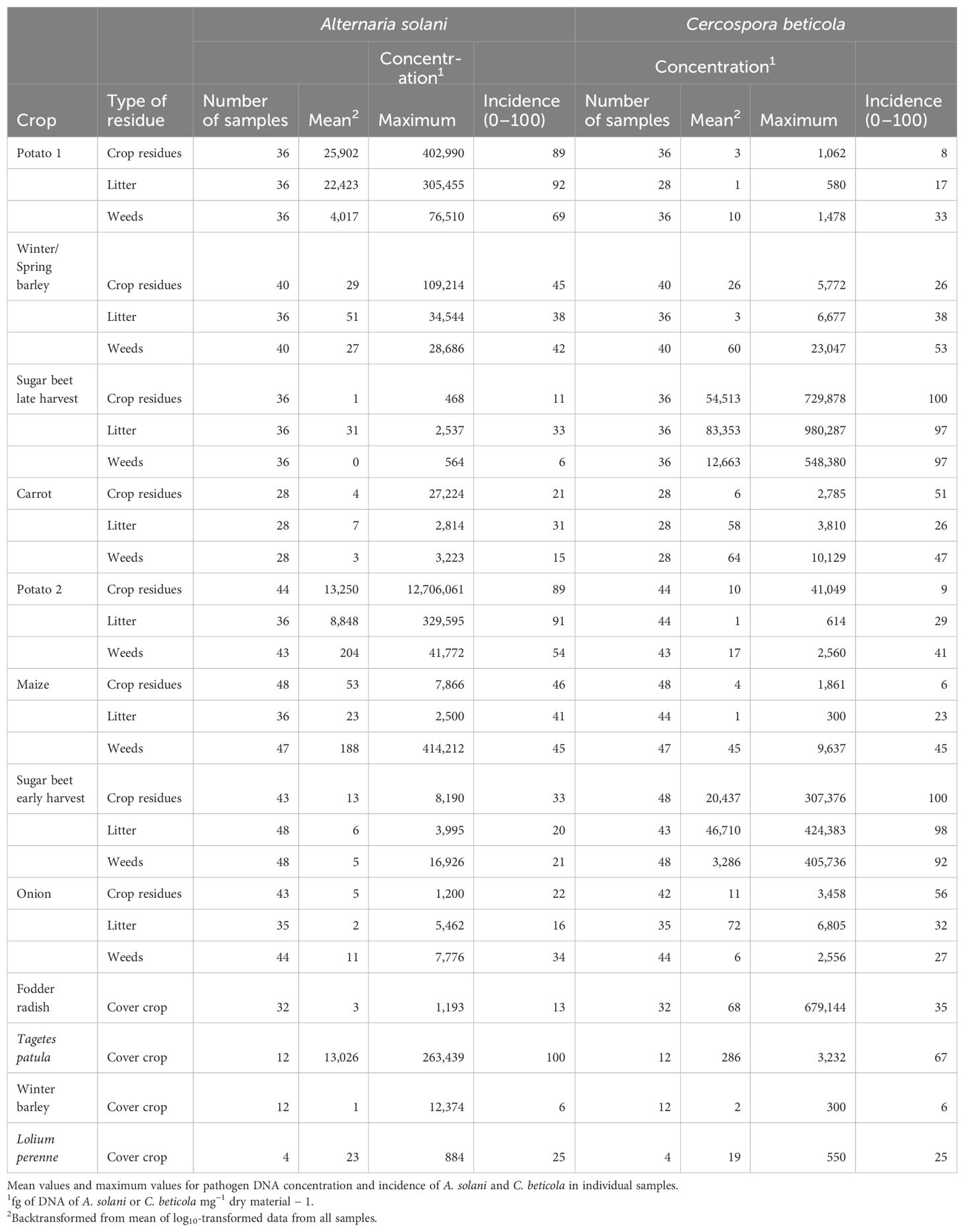
Table 6. Colonization of residues of main crops, cover crops, and weeds and of litter by Alternaria solani and Cercospora beticola in crops grown with the integrated crop management (ICM) strategy.
A similar pattern as found for A. solani in potato was found for C. beticola in early or late harvested sugar beet crops (Tables 5, 6). The incidence of C. beticola was >90% in sugar beet residues, weed residues, and litter collected in plots after harvest of sugar beet. Pathogen DNA concentrations were generally two times higher in plots where sugar beets had been harvested late compared to early harvested sugar beet. The concentrations in litter reached levels similar to or above the levels in sugar beet residues. In samples obtained from plots grown with barley, carrot, potato, maize, or onion, the incidence of C. beticola ranged between 4% and 56% and concentrations were three to four orders of magnitudes lower compared to the concentrations measured in residues from sugar beet plots. Exceptional high concentrations of C. beticola DNA were found in individual samples of weeds collected in crops grown in rotation with sugar beets. Concentrations above 10,000 fg of DNA of C. beticola mg−1 dry material were also found in individual crop residue samples collected in plots grown with fodder radish as cover crop.
Ramularia beticola was detected in 64 of 168 samples of sugar beet residues at average concentrations of 94 fg of R.b. DNA mg−1 (backtransformed values) and in 60 of 577 samples of other crop residues at on average 1 fg of R.b. DNA mg−1. Stemphylium beticola was detected in 39 of 168 samples of sugar beet residues at an average concentration of 14 fg of S.b. DNA mg−1 and in 25 of 612 samples of other crop residues at an average concentration of 3 fg of S.b. DNA mg−1. In litter, R. beticola was detected in 65 of 659 samples (average concentration of 28 fg of R.b. DNA mg−1) and S. beticola was detected in 64 of 659 samples (average concentration of 35 fg of S.b. DNA mg−1). In weeds, R. beticola was detected in 66 of 701 samples (average concentration of 13 fg of R.b. DNA mg−1) and S. beticola was detected in 39 of 701 samples (average concentration of 2 fg of S.b. DNA mg−1).
The dynamics of pathogen colonization during 2.5 years in residues of main crops, cover crops, and weeds and in litter present in the four replicated sub-plots are shown for all rotation plots of both crop management systems for A. solani (Figures 2, 4) and C. beticola (Figures 3, 5). Figures also include information on cropping periods of main crops and cover crops before and during the sampling period of 2.5 years and time points of residue sampling (for details, see Supplementary Table 1).
Main trends as summarized in Tables 5, 6 are shown, such as high concentrations of the pathogens in residues of their host crops and of weeds and in litter collected in plots during the months after harvest of the host crop. A decrease of A. solani concentration of up to two orders of magnitude for a few months after potato harvest was found in most cases, whereas no decrease or even an increase in colonization by one to two orders of magnitude during the months after harvest was observed in two cases (Figures 2C, G). These patterns in plots after potato cropping were similar for potato residues as well as for weed residues and litter. A similar rapid decrease was also found for C. beticola in residues of sugar beet and weeds and litter collected in plots during the months after harvest of the host crop.
During sampling periods following non-host crops, concentrations of A. solani were magnitudes lower than found in samples collected after potato growing, and the observed pattern was inconsistent. Values fluctuated between sampling dates, between replicate plots (resulting in larger standard error of the means), and between substrate types. Despite the inconsistent results for the various individual assessments, a common pattern occurred for all sampling periods following cropping periods of non-host crops: A. solani was present in all plots (at low levels) during periods without host crops. The highest variation including occasionally higher values of A. solani concentrations was found for residues of weeds, indicating that some weeds present in non-host crops may function as a host for A. solani. A similar pattern was found for C. beticola concentrations in non-host plots with fluctuating values between sampling dates, replicate plots, and substrate types, with the highest variation and highest values in weed samples.
Pathogens showed the same behavior in both crop management systems (Figures 2–5). Similar levels of colonization and similar patterns in population dynamics were observed except for the periods with T. patula growth in the ICM system. A. solani reached high concentrations not only in residues of this cover crop, but also in residues of the preceding main crop and weeds and in litter present in plots with T. patula cover crops.
3.3 Effect of crop residue treatment on decomposition and dynamics of Alternaria solani in potato crop residues
Flailed potato canopy residues buried in soil decomposed for 95% for 6 months in the first experiment started after potato harvest in 2020 (Figure 6A). Canopy residues left untreated or cut into 10-cm or 2-cm pieces decomposed for 92% to 93%. After the rapid decomposition during the initial 6 months of the experiment, further decomposition was slower. After 24 months, 1%–2% of the originally canopy residues were recovered. Residues were found in 75% of the buried bags (representing all four treatments), and in the remaining 25% of the bags, no residues could be recovered. Canopy residues that had been exposed in the field on the soil surface decomposed slower (Figure 6C). During 6 months, 93% of flailed residues were decomposed, whereas untreated residues or residues cut into 10-cm or 2-cm pieces decomposed less by 84%–87%. After 24 months, 3% of the flailed residues and 7%–9% of the residues cut into 10-cm or 2-cm pieces were still present. In the second experiment, started after harvest in 2021, decomposition of potato canopy residues in the soil was initially slower (Figure 6B) than in the first experiment. After 6 months, 80% of the untreated residues were decomposed. Higher decomposition was found for the other three treatments with 85%–88% decomposition. Further decomposition was slower with no significant differences between treatments, and after 24 months, only 1%–4% of the residues were still present in the buried bags. In addition, residues placed on the soil surface decomposed slower compared to the first experiment (Figure 6D). After 6 months, 80% of the residues were decomposed without differences between treatments. Further decomposition was slow, but flailed residues tended to decompose faster than untreated or cut residues. After 24 months, 4% of the flailed residues were present, whereas a higher proportion of 10% of the residues cut into 2-cm pieces was still found. Statistically significant treatment effects were found only in a few cases for the two experiments. Flailed residues decomposed significantly faster compared to the untreated residues (p = 0.0045) and the residues cut in 2-cm pieces (p = 0.0090). However, there was a significant difference between bags buried in soil versus bags placed on the soil surface (p < 2.2e-16). Decomposition was significantly higher during the first two sampling dates (p < 0.0001), compared to the decomposition rates after the first year. In summary, decomposition of potato canopy residues showed similar pattern in both experiments for all “cutting” treatments.

Figure 6. Decomposition (%) of potato canopy residues treated by flailing or cutting tissues in 2-cm or 10-cm pieces in comparison to untreated residues. Residues were buried in soil (A, B) or exposed to soil surface (C, D). Two experiments started after potato harvest in 2020 (A, C) and in 2021 (B, D). Decomposition was expressed as the percent dry weight decomposed at each sampling date compared to the beginning of the experiments.
The concentration of A. solani DNA steeply decreased in canopy residues buried in soil during the initial 6 months by three to four orders of magnitude with no differences between the treatments in the first experiment (Figure 7A). A. solani DNA concentration further decreased during the following months and no A. solani could be detected on the remaining residues after 24 months. Similar patterns were found in the second experiment (Figure 7B). However, higher concentrations of A. solani DNA were found in residues cut into 2-cm pieces after 12-month field exposition with 3,568 fg of A.s. DNA mg−1 (dw) (backtransformed values) compared to <50 fg of A.s. DNA mg−1 (dw) in the other treatments. At the end of the experiment after 24 months, traces of A. solani DNA were found in few residue samples. For residues on the field surface, different patterns compared to residues buried in soil and between the two experiments were observed (Figures 7C, D). In the first experiment, A. solani DNA concentration decreased by two orders of magnitude in flailed residues and residues cut into 2-cm pieces, whereas for the other treatments, such a decrease in concentration was not found during the initial 6 months. DNA concentration decreased in flailed residues during the following months, and after 24 months, A. solani could not be detected in such residues. In untreated residues and residues cut into 10-cm pieces, higher concentrations were found at the subsequent sampling dates, and finally after 24 months, low concentrations of 55–225 fg of A.s. DNA mg−1 (dw) were detected. In the second experiment, no treatment effect was observed during the first year (Figure 7D). Flailed residues still contained 3,568 fg of A.s. DNA mg−1 (dw) after 18 months of field exposition on the soil surface and 87 fg of A.s. DNA mg−1 (dw) after 24 months. At the end of the experiment, traces of A. solani were also still found in residues of the other treatments. Statistical analysis of both experiments showed no significant differences in any of the treatments (p = 0.2338). However, the decomposition of the canopy samples left on the soil surface was significantly slower in all sampling periods, compared to the samples buried in the soil (p = 2.369e−08). In summary, the A. solani DNA concentrations decreased in all treatments by two to four orders of magnitude during the initial 6 months and down to traces or undetectable concentrations after 24 months in the remaining residues. Decrease in concentrations in residues on soil surfaces was generally slower but reached similar low levels after 24 months as found in buried residues.
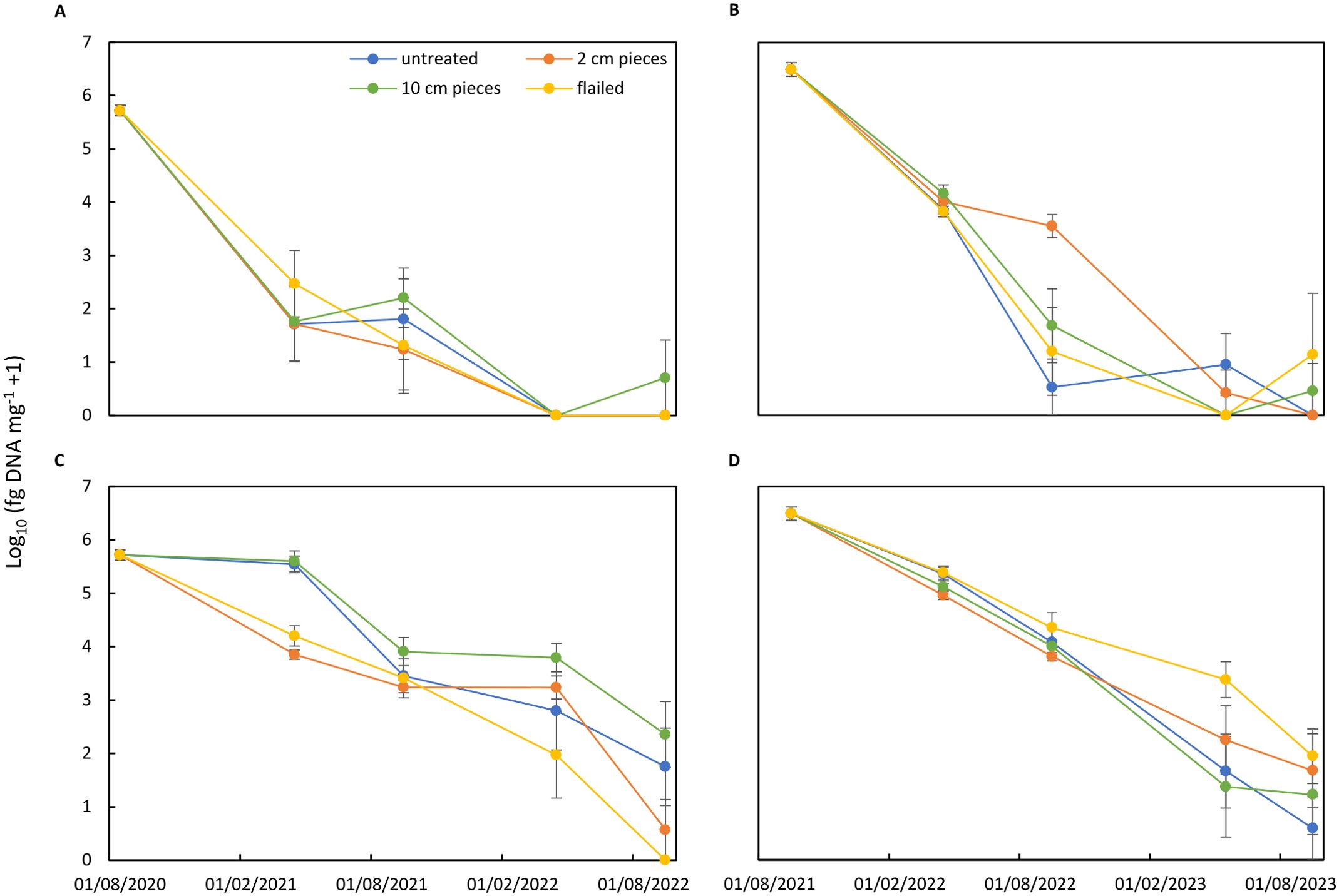
Figure 7. Concentration of Alternaria solani (log10 fg of DNA of A. solani mg−1 dry material + 1) in potato canopy residues treated by flailing or cutting tissues in 2-cm or 10-cm pieces in comparison to untreated residues. Residues were buried in soil (A, B) or exposed to soil surface (C, D). Two experiments started after potato harvest in 2020 (A, C) and in 2021 (B, D). Alternaria solani DNA concentration is expressed as the mean of log10-transformed data from 10 replicates at the beginning of the experiments and five replicates for each sampling date. Bars correspond to the standard error of the means.
Combining the data on residue decomposition and the DNA concentration of A. solani in the remaining residues resulted in the amounts of A. solani DNA being present in the remains of the original samples placed in the bags (expressed as pg of DNA of A. solani in the remains of 1 g of dry material at the beginning of the experiment − 1) (Figure 8). Amounts of A. solani DNA present in the remains of canopy residues decreased during the initial 6 months by five orders of magnitude in residues buried in soil in the first experiment and two orders of magnitude in the second experiment (Figures 8A, B) without consistent treatment effects. In residues exposed to field surface, treatment effect was found in the first experiment, which was mainly caused by the treatment effects on DNA concentration of A. solani rather than treatment effects on decomposition (Figure 8C). In the second experiment, the decrease of A. solani DNA amounts followed the same pattern (Figure 8D). Statistical analysis showed no significant differences between any of the treatments (p = 0.5428). Significant differences were found between soil surface/buried placement (p < 2e−16) and between sampling periods (p < 2e−16).
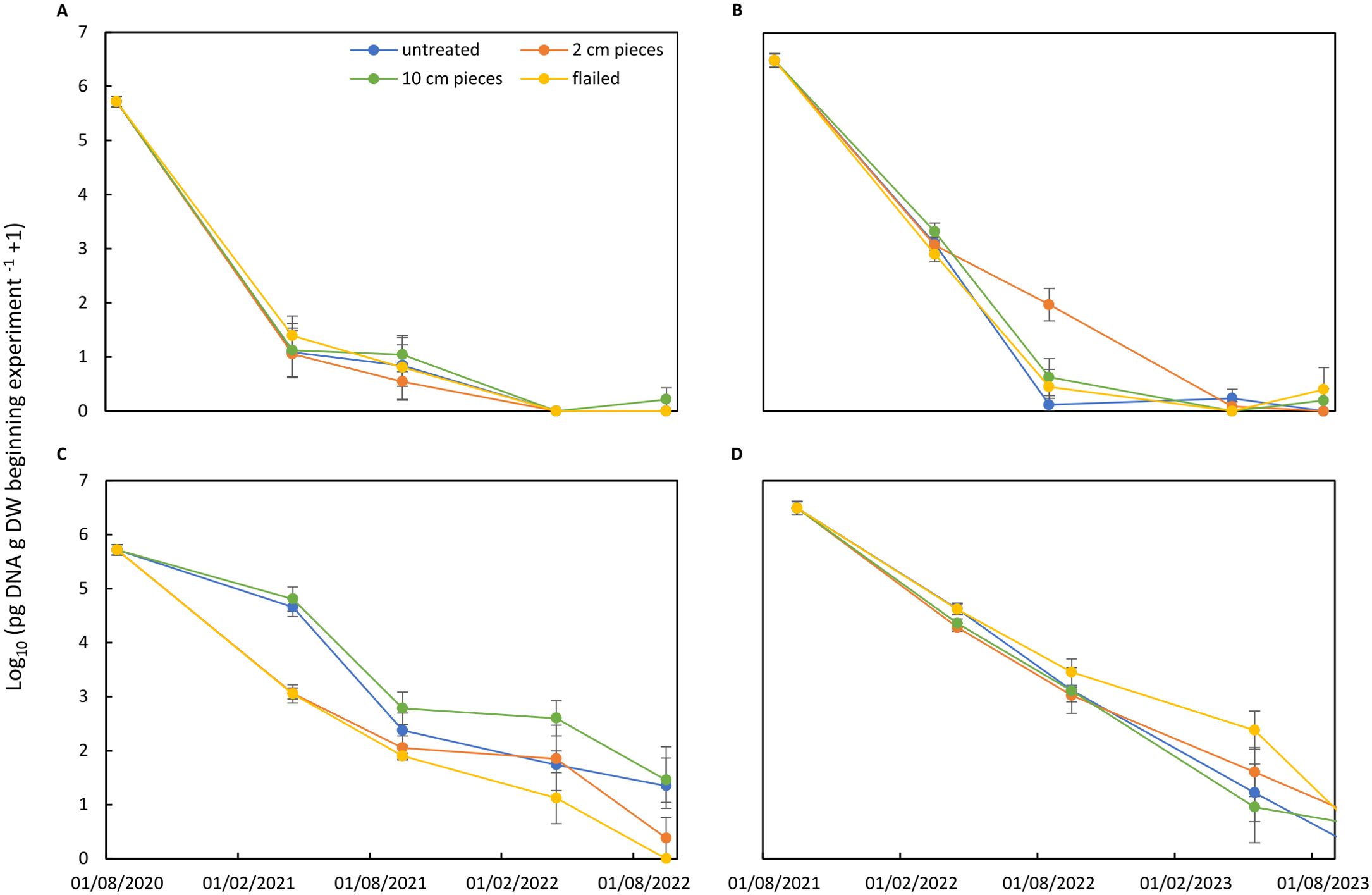
Figure 8. Amount of Alternaria solani log10 pg of DNA of A. solani in remains of 1 g of dry material at the beginning of the experiment +1 in potato canopy residues treated by flailing or cutting tissues in 2-cm or 10-cm pieces in comparison to untreated residues. Residues were buried in soil (A, B) or exposed to soil surface (C, D). Two experiments started after potato harvest in 2020 (A, C) and in 2021 (B, D). Values were calculated by considering residue decomposition (Figure 6) and A. solani concentration (Figure 7).
4 Discussion
Residues of crops, weeds, and litter were systematically sampled in a complex crop rotation experiment. Concentrations of DNA of major pathogens of the grown crops, A. solani, C. beticola, R. beticola, and S. beticola, were quantified in the residues using newly developed qPCR assays. Alternaria solani and C. beticola were consistently detected, whereas R. beticola and S. beticola were found only a few samples at low concentrations. Repeated field trials gave additional insights into the dynamics of A. solani in potato foliage residues during 2 years. The overall results demonstrated that A. solani and C. beticola colonized crop residues of their host crops initially after harvest at high densities. Within several months, amounts of available host residues decreased substantially (as found for potato canopy residues) and concentrations of pathogens in the remaining host residues decreased steeply. Alternative substrates, residues of non-host crops including cover crops and weeds, were colonized saprophytically by necrotrophic pathogens. It can be concluded that such residues can potentially serve as a bridge for pathogen populations during host-free cropping seasons in crop rotation systems.
Quantitative Taqman PCR assays are powerful tools for such assessments because they can potentially quantify the concentration of the targeted DNA in environmental samples reliably within a large dynamic range. Original samples and extracted DNA samples can be stored, allowing the organization of workflows with high numbers of assessments in cost-effective formats, e.g., automated DNA extraction in combination with a 384-well format for real-time PCR measurements. Furthermore, storage of DNA samples allows future measurement of additional important pathogens, e.g., of toxigenic Fusarium spp. as pathogens of maize and barley, A. dauci and A. radicina as pathogens of carrots, Botrytis squamosa and B. aclada/allii as pathogens of onions, and C. apii as a pathogen of sugar beet, to enhance the view on the role of crop residues in pathogen population dynamics.
Several PCR assays for the quantification of A. solani have been described. The previously reported qPCR assay by Leiminger et al. (2015) showed a weak cross-reactivity with P. infestans and several other potato pathogens as well as with fungi not pathogenic in potato. The assay reported by Lees et al. (2019) shows cross-reaction with A. dauci, which is a pathogen in carrots present in our rotation experiment. The Syber green-based assay developed by Khan et al. (2018) for the detection of A. solani needs extra run and analyses time for melting curve analysis. Our new qPCR assay includes a Taqman probe, eliminating the use of a melting curve analyses. It also has a higher sensitivity with 100 fg per reaction compared to Khan et al. (2018), which has a sensitivity of 1,000 fg per reaction. However, our Taqman-PCR assay developed for quantification of A. solani also detects the closely related A. alternariacida sp. nov, A. catananches sp. nov., A. cichorii, A. conidiophora, A. dichondrae, A. grandis, A. linicola, and A. protenta. A. grandis and A. protenta are causing early blight in potato and are members of the European Alternaria population on potato (Landschoot et al., 2017). In addition, A. alternariacida sp. nov, newly described by Woudenberg et al. (2014) for an isolate from a fruit of Solanum lycopersicum in UK, has recently been reported as a causal agent of potato leaf blight in Russia (Kokaeva and Elansky, 2023). The other Alternaria species have distinct host ranges and have not been associated with potato or other crops grown in the rotation experiment. The recently newly described species A. catananches sp. nov. has been found on Catananche caerulea (Cupid’s dart, Asteraceae) in the Netherlands, and A. conidiophora has been found on an unspecified host (Woudenberg et al., 2014). A. cichorii is causing leaf spot in endive and other Asteraceae (Barreto et al., 2008). A. dichondrae is a leaf spot pathogen of Dichondra repens (Convolvulaceae) (Cardin et al., 2005). A. linicola is causing seedling blight in flax (Corlett and Corlett, 1999). It is thus not likely that DNA of A. catananches, A. conidiophore, A. cichorii, A. dichondrae, or A. linicola was present in the investigated residue samples in amounts interfering with results obtained for A. solani. For members of the Alternaria complex on potato causing early blight, A. grandis and A. protenta, and possibly A. alternariacida, it is expected that they are present in the Netherlands, but this has not been confirmed yet.
Unexpected high concentrations of DNA of A. solani were detected in residues of Tagetes patula cover crops. Tagetes is not a known host of A. solani. However, A. tagetica has been described as a pathogen of T. patula causing foliar necrotic lesions (Tomioka et al., 2000). Sequence information on A. tagetica revealed that this pathogen is not detected by the applied Taqman-PCR assay. Another pathogen of T. patula, A. patula has been described as a new species in 2005 (Wu and Wu, 2005, 2019). Published information on this species closely related to A. tagetica is limited and sequence information is not available. Alternaria spp. were frequently isolated from residues of T. patula in three samples collected in February 2023 from the rotation experiment (data not presented). The majority of the isolates as identified by alta 1 and gpd1 DNA sequence information belonged to A. alternata, a species that is not detected by the applied Taqman-PCR assay. Isolates of A. solani were also isolated from two of the three samples. Only one of the obtained various Alternaria spp. isolates belonged to another species, A. cinerariae.
The Taqman-PCR developed for the quantification of C. beticola described by Knight and Pethybridge (2020) also detects C. cf. flagellaris albeit with low sensitivity. The presence of C. cf. flagellaris on B. vulgaris has been reported by Groenewald et al. (2006) and Vaghefi et al. (2018), respectively. Our newly developed Taqman-PCR is not detecting C. cf. flagellaris and achieves a 10-fold increased sensitivity compared to the qPCR described by Knight and Pethybridge (2020). However, our Taqman-PCR assays developed for quantification of C. beticola also detect C. plantaginis. C. plantaginis has been associated with Plantago (Groenewald et al., 2006) and reported on Plantago lanceolata (Bakhshi et al., 2018). P. lanceolata (buckhorn plantain) is a ubiquitous species in the Netherlands that is commonly not growing in arable fields. There are no reports of C. plantaginis on sugar beet or other crops grown in the rotation experiment or other plant genera. It is thus not very likely that DNA of C. plantaginis was present in the investigated residue samples in amounts interfering with obtained for C. beticola. Besides C. beticola, C. apii is causing Cercospora leaf spot in sugar beet (Groenewald et al., 2006). This species is not detected by the used Taqman PCR assay. C. apii has recently been isolated from leaf spots on sugar beet leaves in Dutch fields including the experimental field in Vredepeel. In 2021, C. beticola was mainly isolated from leaf spots and only few isolates of C. apii were obtained from cv. Reforma KWS. However, in 2022, approximately 50% of isolates obtained from cv. Reforma KWS grown at the experimental field or from various cultivars grown at different locations in the Netherlands belonged to C. apii (Hanse, 2023). A new detection assay for quantification of C. apii is thus needed to complete the study on the role of crop residues on Cercospora leaf spot.
Quantifying the DNA concentration of a targeted fungal pathogen in environmental samples for a certain duration gives insights into its population dynamics. However, a careful interpretation should consider that such measurements do not distinguish between DNA derived from living or dead cells. Especially in situations when populations are decreasing, the decrease may thus be underestimated since the DNA of dead cells may be detectable for certain time spans. Furthermore, fungi can produce different cell types with different importance in population survival, e.g., mycelial cells, spores, resting spore such chlamydospores, sclerotia, and fruiting bodies. Knowledge on the biology of the pathogen is thus essential to allow adequate conclusions. Alternaria solani generally survives as mycelium and conidia, protected by dark pigmentation from environmental stress factors. Chlamydospores have been reported, but found infrequently (Kemmit, 2002). For C. beticola, it is reported that the pathogen survives in infested crop debris through formation of pseudostromata and that the pathogen can also survive on dead plant tissue as a necrotroph regardless of host status (Sharma et al., 2022).
The assessments of pathogen DNA in plant residues including litter present in the upper soil layer in the rotation experiment revealed a common pattern for both pathogens A. solani and C. beticola. Pathogen DNA concentrations were high in crop residues, weed residues, and residues in litter after harvest of the host crop. Concentrations decreased substantially during the following months. Pathogen DNA was found at low concentration in plant residues from plots grown with non-host crops. However, such results varied in time and between replicate plots. Specific variation and occasionally high concentrations were measured in residues of weeds, most likely because particular weeds occurring in the rotation plots acted as hosts, e.g., C. album for C. beticola or S. nigrum for A. solani. The risk that residues of weeds acting as hosts of the studied pathogens contribute to pathogen survival in a rotation system may increase in future cropping systems with reduced input of herbicides. Although not yet observed in the comparison between the reference cropping system and the ICM cropping system during the first years of the experiment, it is expected that occurrence of certain weeds will increase after several years of implementation of ICM systems with reduced herbicide use.
Our results suggest that residues of non-host crops may play an important role, allowing pathogens to bridge periods in the field without host crops and rapidly decaying residues of host crops. The amount of particular types of residues was not quantified when residues in the crop rotation experiment were sampled in our study. The relative importance of residues for colonization by pathogens depends on the amount and concentration of pathogens in the particular substrate. Residues will be present at harvest of a crop at high amounts and decrease in subsequent months as demonstrated for potato canopy residues in the additional experiments on residue treatments. This fluctuation of substrate availability will result in considerable fluctuations of pathogen populations. Cover crops included in crop rotation schemes may play a particular role since they produce biomass that entirely remains in the field and converts into plant residues. Differences in pathogen colonization between cover crops were observed in our study with unexpected high concentrations of A. solani in tagetes residues. Tagetes residues may be a particular suitable substrate for A. solani. Possibly, the senescence of the Tagetes crop coincidenced in certain periods with high A. solani densities on other residues leads to a rapid and intense colonization of Tagetes residues. Since cover crops produce huge amounts of residues, their particular role in the dynamics of pathogen populations needs attention in further studies.
The hypothesis for our field trial with treatments of potato canopy residues was that A. solani concentrations decrease faster in smaller canopy pieces since smaller pieces may be faster colonized by saprophytes outcompeting the pathogen. Effects of canopy residue treatments on A. solani during time could not be found. Further experiments are needed to better understand the colonization of residue pieces by saprophytes and how to support such microbiome functions. A clear main effect was demonstrated in the experiments. Canopy residues buried in soil decayed significantly faster and the concentration of A. solani also decreased faster compared to residues on the soil surface. This may be due to different environmental conditions with higher and more constant water availability in buried substrates in combination with different composition of the invading microbiome developing in the residues. Burying crop residues, e.g., by ploughing, may not only enhance decomposition and reduce pathogen colonization but will also block transfer of pathogen from host residues to residues present after harvest of the subsequent non-host crop. This possible advantage of tillage in pathogen management needs further attention in future studies on tillage systems (Kerdraon et al., 2019; Wang et al., 2020).
Different inoculum sources can potentially initiate new epidemics once a susceptible host crop is established in the field. Pathogen populations may be present, colonizing necrotic plant tissues saprophytically as assessed in our study. Another source within a field can be volunteer plants of the host and host weeds that survived in subsequent crops and harbor the pathogen actively infecting the volunteer plants and weeds as pathogen (Thompson et al., 2015). Sources from outside the field can also play a role, e.g., pathogen spores present on seeds or airborne spores originating from fields where epidemics are already ongoing, or from saprophytically colonized plants and infected volunteer plants in the neighborhood of the field. The relative importance of the different potential sources depends on the biology of pathogen as well as on regional cropping systems and crop management. Experimental data on the source of spores initiating epidemics are limited and hard to obtain in field studies (Suffert and Sache, 2011). Experimental manipulations, e.g., by removal of known inoculum sources, that result in delayed epidemics give insight into the roles of certain sources (Köhl et al., 1995).
Future reduced tillage and non-tillage systems aiming at reduced CO2 emissions, increase of organic matter in soil, and improved soil structure will result in increasing amounts of crop residues. However, there is evidence, including the results of this study, that crop residues within the field are a major source of pathogen inoculum, e.g., in cereal production (Kerdraon et al., 2019). Crop residue management—not interfering with the general goals of increasing organic matter in arable systems—is thus an option to lower the inoculum potential and subsequently delay or even prevent disease outbreaks. Crop residue treatments like shredding, application of urea, or physical removal have been reported for fruit production (Gomez et al., 2007). For prevention of early blight caused by A. solani, the removal of potentially infected materials such as wines and fruits (in case of tomato) is considered (Kemmit, 2002). Scientific reports on the effects of such sanitation measures for arable crops are limited, and reports considering the dynamics of pathogens in residues of subsequently grown crops including non-host crops in crop rotation systems are missing. Understanding the temporal dynamics of substrate quantities and quality will open opportunities for disease prevention through crop residue management in arable rotation systems. Understanding the biotic mechanisms will be important. Plant debris is colonized by different types of organisms in succession, depending on nutrient availability in degrading tissues, the nutritional needs of the potential colonizers, and their ability to utilize the remaining more complex organic compounds (Hudson, 1971; Hudson and Webster, 1958; Köhl and Fokkema, 1998; Kerdraon et al., 2019). Pathogens, being present already at senescence of the tissue, have an advantage in the early stages of decomposition during competitive substrate colonization. Substrate composition changes caused by nutrient consumption by initial colonizers result in more complex organic substrates, favoring specialists with appropriate physiological properties and leading to typical microbial successions in substrate colonization. Pathogens in this situation may be outcompeted and need to survive through the formation of resting structures or the colonization of alterative plant debris.
Crop residue management aiming at disease prevention thus needs to focus on the early stage of substrate colonization. Collection and physical removal of crop residues from the field is a realistic option if crop residues such as straw can be used as side products with an economic value. Field management will always focus on pathogens outcompeting during the early stages of decomposition, enhancement of early decomposition, or burying residues into the soil to block the transfer to alternative substrates and additionally to create a microbial buffer preventing substrate colonization by pathogens at later stages. Decomposition processes are the result of microbial activity, determined by the microbiome present at the beginning of decomposition processes and microbiome dynamics during decomposition processes (Kerdraon et al., 2019). Knowledge on microbiome functions and options for shaping microbiomes in the “residue sphere”, defined as the microhabitat consisting of all crop residues on and in soil (Kerdraon et al., 2019), is essential to develop rational strategies for crop residue management including microbiome-based biocontrol solutions. Additionally, the role of the mesofauna in early decomposition of necrotic plant tissues, often neglected by plant pathologists, needs particular attention.
Crop residues of non-host crops grown in rotation as alternative routes for pathogens make pathogen life cycles more complex and considerations of sanitation more difficult. On the other hand, sanitation measures targeting crop debris shortly after harvest will not only block the development of populations of pathogens of this particular crop but also affect pathogens of other crops grown in the rotation. This increases the value of targeted crop residue management and may allow an integral sanitation of the crop rotation system rather than sanitation directed at a single pathogen.
Data availability statement
The raw data supporting the conclusions of this article will be made available by the authors, without undue reservation.
Author contributions
JK: Conceptualization, Formal Analysis, Funding acquisition, Investigation, Methodology, Project administration, Writing – original draft, Writing – review & editing. GE: Data curation, Formal Analysis, Investigation, Visualization, Writing – original draft, Writing – review & editing. BH: Conceptualization, Funding acquisition, Investigation, Writing – original draft, Writing – review & editing. IH: Data curation, Investigation, Methodology, Writing – original draft. LG: Data curation, Investigation, Methodology, Writing – original draft. EL: Data curation, Investigation, Methodology, Writing – original draft. HV: Data curation, Investigation, Methodology, Writing – original draft. AE: Conceptualization, Funding acquisition, Investigation, Methodology, Writing – original draft, Writing – review & editing.
Funding
The author(s) declare financial support was received for the research, authorship, and/or publication of this article. The projects “Crop residue management for disease control” (LWV193), “Role of crop residues of leaf pathogens of sugar beet in crop rotation systems” (LWV20.167), and “Integrated crop protection management for arable farming on sand” (LWV19093) received financial support from the Top Sector Horticulture and Propagation Materials. Within the Top Sector, the business community, knowledge institutions, and the government work together on innovations in the field of sustainable production of healthy and safe food and the development of a healthy, green living environment. We acknowledge funding by Brancheorganisatie Akkerbouw and Consun Beet Company.
Acknowledgments
The authors acknowledge their colleagues at Wageningen University & Research—Geert Kessel, Marie Wesselink, and Marleen Riemens—for collaboration and allowing sampling from plots of their crop rotation field experiment; Jos Wilms, Rik Peters, and Marc Kroonen for their technical support during the execution of the field experiments; Dafydd Timmerman and Wim van der Slikke for their help in sample processing; and Sabine Schnabel for the statistical analysis of the crop residue management experiment.
Conflict of interest
The authors declare that the research was conducted in the absence of any commercial or financial relationships that could be construed as a potential conflict of interest.
Publisher’s note
All claims expressed in this article are solely those of the authors and do not necessarily represent those of their affiliated organizations, or those of the publisher, the editors and the reviewers. Any product that may be evaluated in this article, or claim that may be made by its manufacturer, is not guaranteed or endorsed by the publisher.
Supplementary material
The Supplementary Material for this article can be found online at: https://www.frontiersin.org/articles/10.3389/fagro.2024.1470598/full#supplementary-material
References
Abuley I. K., Nielsen B. J., Hansen H. H. (2018). The influence of crop rotation on the onset of early blight (Alternaria solani). J. Phytopathol. 167, 35–40. doi: 10.1111/jph.12771
Bakhshi M., Arzanlou M., Babai-ahari A., Groenewald J. Z., Crous P. W. (2018). Novel primers improve species delimitation in Cercospora. IMA FUNGUS 9, 299–332. doi: 10.5598/imafungus.2018.09.02.06
Barreto R. W., Santin A. M., Vieira B. S. (2008). Alternaria cichorii in Brazil on Cichorium spp. seeds and cultivated and weedy hosts. J. Phytopathol. 156, 425–430. doi: 10.1111/j.1439-0434.2007.01380.x
Cardin L., Delecolle B., Moury B. (2005). Occurrence of Alternaria dichondrae, Cercospora sp., and Puccinia sp. on Dichondra repens in France and Italy. Plant Dis. 89, 1012. doi: 10.1094/PD-89-1012A
Corlett M., Corlett M. E. (1999). Alternaria linicola. Can. J. Plant Pathol. 21, 55–57. doi: 10.1080/07060661.1999.10600134
Cotton T. K., Munkvold G. P. (1998). Survival of Fusarium moniliforme, F. proliferatum, and F. subglutinans in maize stalk residue. Phytopathology 88, 550–555. doi: 10.1094/PHYTO.1998.88.6.550
European Commission (2015). Commission Implementing Regulation (EU) No. 2015/408 of 11 March 2015 implementing Article 80(7) of Regulation (EC) No 1107/2009 of the European Parliament and of the Council concerning the placing of plant protection products on the market and establishing a list of candidates for substitution. O.J.E.U., L Official J. European Union (O.J.E.U.) 67, 18–22.
Gomez C., Brun L., Chauffour D., Vallée D. D. L. (2007). Effect of leaf litter management on scab development in an organic apple orchard. Agric. Ecosyst. Environ. 118, 249–255. doi: 10.1016/j.agee.2006.05.025
Groenewald M., Groenewald J. Z., Braun U., Crous P. W. (2006). Host range of Cercospora apii and C. beticola and description of C. apiicola, a novel species from celery. Mycologia 98, 275–285. doi: 10.3852/mycologia.98.2.27
Hanse B. (2023). “IRS project 12-14 stemphylium en cercospora in suikerbieten,” in IRS jaarverslag 2022 (Stichting IRS, Dinteloord, NL), 107–111.
Hanse B., Raaijmakers E. E. M., Schoone A. H. L., Oorschot, van P. M. S. (2015). Stemphylium sp., the cause of yellow leaf spot disease in sugar beet (Beta vulgaris L.) in the Netherlands. Eur. J. Plant Pathol. 142, 319–330. doi: 10.1007/s10658-015-0617-8
Hudson H. J. (1971). The development of the saprophytic fungal flora as leaves senesce and fall. In: Ecology of Leaf Surface Microorganisms (Preece T. F., Dickinson C. H. eds.), Academic Press, London, pp. 447-455
Hudson H. J., Webster J. (1958). Sucession of fungi on decaying stems of Agropyron repens. Trans. Br. Myco. Soc. 41, 165-177.
Humpherson-Jones F. M. (1989). Survival of Alternaria brassicae and Alternaria brassicicola on crop debris of oilseed rape and cabbage. Ann. Appl. Biol. 115, 45–50. doi: 10.1111/j.1744-7348.1989.tb06810.x
Jindo K., Evenhuis A., Kempenaar C., Pombo Sudré C., Zhan X., Goitom Teklu M., et al. (2021). Review: Holistic pest management against early blight disease towards sustainable agriculture. Pest Manage. Sci. 77, 3871–3880. doi: 10.1002/ps.6320
Kemmit G. (2002). “Early blight of potato and tomato,” in The Plant Health Instructor. The American Phytopathological Society (APS). vol. 2. doi: 10.1094/PHI-I-2002-0809-01
Kerdraon L., Laval V., Suffert F. (2019). Microbiomes and pathogen survival in crop residues, an ecotone between plant and soil. Phytobiomes J. 3, 246–255. doi: 10.1094/PBIOMES-02-19-0010-RVW
Khan M. F. R., Smith L. J. (2005). Evaluating fungicides for controlling Cercospora leaf spot on sugar beet. Crop Prot. 24, 79–86. doi: 10.1016/j.cropro.2004.06.010
Khan M., Wang R., Li B., Liu P., Weng Q., Chen Q. (2018). Comparative evaluation of the LAMP assay and PCR-based assays for the rapid detection of. Alternaria solani. Front. Microbiol. 9, 2089. doi: 10.3389/fmicb.2018.02089
Klerks M. M., Zijlstra C., van Bruggen A. H. C. (2004). Comparison of realtime PCR methods for detection of Salmonella enterica and Escherichia coli O157:H7, and introduction of a general internal amplification control. J. Microbiol. Methods 59, 337–349. doi: 10.1016/j.mimet.2004.07.011
Knight N. L., Pethybridge S. J. (2020). An improved PCR assay for species-specific detection and quantification of Cercospora beticola. Can. J. Plant Pathol. 42, 72–83. doi: 10.1080/07060661.2019.1621380
Knight N. L., Vaghefi N., Kikkert J. R., Pethybridge S. J. (2019). Alternative hosts of Cercospora beticola in field surveys and inoculation trials. Plant Dis. 103, 1983–1990. doi: 10.1094/PDIS-01-19-0229-RE
Köhl J., de Jong P.–F., Kastelein P., Groenenboom-de Haas B. H., Anbergen R. H. N., Balkhoven H., et al. (2013). Dynamics of pear-pathogenic Stemphylium vesicarium in plant residues in Dutch pear orchards. Eur. J. Plant Pathol. 137, 609–619. doi: 10.1007/s10658-013-0274-8
Köhl J., Fokkema N. J. (1998). “Biological control of necrotrophic foliar fungal pathogens,” in Plant-Microbe Interactions and Biological control. Eds. Boland G. J., Kuykendall L. V. (Marcel Dekker, New York), 49–88.
Köhl J., Haas B. H., Kastelein P., Burgers S. L. G. E., Waalwijk C. (2007). Population dynamics of Fusarium spp. and Microdochium nivale in crops and crop residues of winter wheat. Phytopathology 97, 971–978. doi: 10.1094/PHYTO-97-8-0971
Köhl J., van der Plas C. H., Molhoek W. M. L., Fokkema N. J. (1995). Suppression of sporulation ofBotrytis spp. as a valid biocontrol strategy. Eur. J. Plant Pathol. 101, 251–259. doi: 10.1007/BF01874781
Köhl J., Vlaswinkel M., Groenenboom-de Haas B. H., Kastelein P., van Hoof R. A., van der Wolf J. M., et al. (2011). Survival of pathogens of Brussels sprouts (Brassica oleracea Gemmifera group) in crop residues. Plant Pathol. 60, 661–670. doi: 10.1111/j.1365-3059.2010.02422.x
Kokaeva L. Y., Elansky S. N. (2023). First report of Alternaria alternariacida causing potato leaf blight in the Far East, Russia. Plant Dis. 107, 938. doi: 10.1094/PDIS-02-22-0291-PDN
Kuznetsova A., Brockhoff P., Christensen R. H. B. (2017). lmerTest package: tests in linear mixed effects models. J. Stat. Software 82, 1–26. doi: 10.18637/jss.v082.i13
Landschoot S., Vandecasteele M., De Baets B., Höfte M., Audenaert K., Haesaert G. (2017). Identification of A. arborescens, A. grandis, and A. protenta as new members of the European Alternaria population on potato. Fungal Biol. 121, 172–188. doi: 10.1016/j.funbio.2016.11.005
Lees A. K., Roberts D. M., Lynott J., Sullivan L., Brierley J. L. (2019). Real-Time PCR and LAMP assays for the detection of spores of Alternaria solani and sporangia of Phytophthora infestans to inform disease risk forecasting. Plant Dis. 103, 3172–3180. doi: 10.1094/PDIS-04-19-0765-RE
Leiminger J. H., Bäßler E., Knappe C., Bahnweg G., Hausladen H. (2015). Quantification of disease progression of Alternaria spp. on potato using real-time PCR. Eur. J. Plant Pathol. 141, 295–309. doi: 10.1007/s10658-014-0542-2
MacHardy W. E., Gadoury D. M., Gessler C. (2001). Parasitic and biological fitness of Venturia inaequalis: Relationship to disease management strategies. Plant Dis. 85 1036–1051. doi: 10.1094/PDIS.2001.85.10.1036
Madden L. V., Hughes G., van den Bosch F. (2007). The Study of Plant Disease Epidemics (St. Paul, MN: American Phytopathological Society).
Mourelos C. A., Malbrán I., Mengual Gómez D., Ghiringhelli P. D., Lori G. A. (2024). Dynamics of Fusarium graminearum inoculum on residues of naturally infected winter and summer crops. Eur. J. Plant Pathol. 169, 543–553. doi: 10.1007/s10658-024-02850-z
R Core Team (2020). R: A language and environment for statistical computing (Vienna, Austria: R Foundation for Statistical Computing). Available at: https://www.R-project.org/.
Riemens M., Sønderskov M., Moonen A. C., Storkey J., Kudsk P. (2022). An Integrated Weed framework: A pan-European perspective. Eur. J. Agron. 133, 126443. doi: 10.1016/j.eja.2021.126443
Rossi V., Pattori E., Bugiani R. (2008). Sources and seasonal dynamics of inoculum for brown spot disease of pear. Eur. J. Plant Pathol. 121, 147–159. doi: 10.1007/s10658-007-9258-x
Shane W. W., Teng P. S. (1992). Impact of Cercospora leaf spot on root weight, sugar yield, and purity of Beta vulgaris. Plant Dis. 76, 812–820. doi: 10.1094/PD-76-0812
Sharma S., Heck D. W., Branch E., Kikkert J. E., Pethybridge S. J. (2022). “Cercospora leaf spot of table beet,” in The Plant Health Instructor. The American Phytopathological Society (APS). vol. 22. doi: 10.1094/PHI-P-2022-02-0101
Suffert F., Sache I. (2011). Relative importance of different types of inoculum to the establishment of Mycosphaerella graminicola in wheat crops in north-west Europe: Wheat debris as local source of M. graminicola inoculum. Plant Pathol. 60, 878–889. doi: 10.1111/j.1365-3059.2011.02455.x
Thompson S., Tan Y., Shivas R., Neate S., Morin L., Bissett A., et al. (2015). Green and brown bridges between weeds and crops reveal novel Diaporthe species in Australia. Persoonia Mol. Phylogeny Evol. Fungi 35, 39–49. doi: 10.3767/003158515X687506
Tomioka K., Sato T., Koganezawa H. (2000). Marigold leaf spot caused by Alternaria tagetica new to Japan. J. Gen. Plant Pathol. 66, 294–298. doi: 10.1007/PL00012967
Vaghefi N., Kikkert J. R., Hay F. S., Carver G. D., Koenick L. B., Bolton M. D., et al. (2018). Cryptic diversity, pathogenicity, and evolutionary species boundaries in Cercospora populations associated with Cercospora leaf spot of Beta vulgaris. Fungal Biol. 122, 264–282. doi: 10.1016/j.funbio.2018.01.008
Vereijssen J., Schneider J. H. M., Jeger M. J. (2007). Supervised control of Cercospora leaf spot in sugar beet. Crop Prot. 26, 19–28. doi: 10.1016/j.cropro.2006.03.012
Videira S. I. R., Groenewald J. Z., Braun U., Shin H. D., Crous P. W. (2016). All that glitters is not Ramularia. Stud. Mycol. 83, 49–163. doi: 10.1016/j.simyco.2016.06.001
Waals v. d. J.E., Korsten L., Aveling T. A. S. (2001). A review of early blight of potato. Afr Plant Prot. 7, 91–102.
Wang H., Li X., Li X., Wang J., Li X., Guo Q., et al. (2020). Long-term no-tillage and different residue amounts alter soil microbial community composition and increase the risk of maize root rot in northeast China. Soil Tillage Res. 196. doi: 10.1016/j.still.2019.104452
Woudenberg J. H. C., Hanse B., van Leeuwen G. C. M., Groenewald J. Z., Crous P. W. (2017). Stemphylium revisited. Stud. Mycol. 87, 77–103. doi: 10.1016/j.simyco.2017.06.001
Woudenberg J. H. C., Truter M., Groenewald J. Z., Crous P. W. (2014). Large-spored Alternaria in section Porri disentangled. Stud. Mycol. 79, 1–47. doi: 10.1016/j.simyco.2014.07.003
Wu W.-S., Wu H.-C. (2005). A new species of Alternaria on seeds of French marigold. Mycotaxon 91, 21–25. doi: 10.1111/ppa.12982
Keywords: foliar pathogens, Alternaria solani, Cercospora beticola, crop residues, crop rotation, inoculum sources, potato, sugar beet
Citation: Köhl J, Elena G, Hanse B, Houwers I, Groenenboom-de Haas L, de Lange E, Verstegen H and Evenhuis A (2024) Population dynamics of Alternaria solani, Cercospora beticola, Ramularia beticola, and Stemphylium beticola in residues of host crops, non-host crops, and weeds in Dutch rotation systems. Front. Agron. 6:1470598. doi: 10.3389/fagro.2024.1470598
Received: 25 July 2024; Accepted: 10 October 2024;
Published: 07 November 2024.
Edited by:
Kevin King, Rothamsted Research, United KingdomReviewed by:
Sudhir Navathe, Agharkar Research Institute, IndiaPavel Matusinsky, Palacký University, Olomouc, Czechia
Copyright © 2024 Köhl, Elena, Hanse, Houwers, Groenenboom-de Haas, de Lange, Verstegen and Evenhuis. This is an open-access article distributed under the terms of the Creative Commons Attribution License (CC BY). The use, distribution or reproduction in other forums is permitted, provided the original author(s) and the copyright owner(s) are credited and that the original publication in this journal is cited, in accordance with accepted academic practice. No use, distribution or reproduction is permitted which does not comply with these terms.
*Correspondence: Jürgen Köhl, jurgen.kohl@wur.nl