- Department of Soil Science, University of Tehran, Tehran, Iran
The soil microbiome plays a pivotal role in the functioning and resilience of agricultural ecosystems, contributing to critical processes such as organic matter decomposition, nutrient cycling, and plant growth promotion. However, the soil microbiome is constantly challenged by various environmental stresses, including drought, heavy metal contamination, salinity, and climate change, which can significantly disrupt the delicate balance of the soil ecosystem. In this context, the application of silicon (Si) has emerged as a promising strategy to mitigate the adverse effects of these environmental stresses on the soil microbiome. This review paper synthesizes the current understanding of the impacts of environmental stresses on the soil microbiome and explores the potential of Si as a mitigating agent in enhancing the resilience of the soil microbial community. Silicon can enhance the resilience of the soil microbiome through several mechanisms, such as increasing soil pH, improving nutrient and water availability and uptake, altering root exudation patterns and plant physiology, and directly stimulating the abundance, diversity, and functional potential of key microbial groups. By enhancing the resilience of the soil microbiome, Si application can help maintain the critical ecosystem services provided by soil microorganisms, ultimately contributing to the sustainability and productivity of agricultural systems. The review also highlights future research aspects, including elucidating the precise mechanisms of Si-microbiome interactions, evaluating the long-term effects of Si on soil microbiome resilience, optimizing Si application strategies for specific crop-soil systems, integrating Si management with other sustainable soil practices, and assessing the impacts of Si on soil microbiome-mediated ecosystem services.
1 Introduction
The rising global demand for agricultural products is driven by population growth, leading to increased production rates that have resulted in topsoil depletion, diminished organic matter levels, and diminished soil ecological functions. Soil microbes play a crucial role in maintaining these functions, providing stability to the soil environment and enhancing resilience to disturbances. Poor land management practices have also contributed to problems such as groundwater contamination, plant disease outbreaks, and air pollution. The increasing recognition of the significance of sustainable and nutritious food reflects a growing awareness among the populace of the importance of environmental conservation (Abdul Rahman et al., 2021; Aguilar-Paredes et al., 2023; Vincze et al., 2024). Therefore, environmentally-friendly approaches to sustainable agriculture are gaining increasing popularity. De Corato proposes that sustainable agroecosystems demonstrate high levels of resilience, adaptability, and diversity (De Corato, 2020). These elements are interconnected, as diversity promotes adaptability, which is a crucial component in the resilience of agricultural ecosystems. The diversity of soil microbiota is essential for nutrient recycling and the formation of soil structure, and it plays a vital role in sustainable agriculture. Instead of solely focusing on the taxonomic diversity of soil microorganisms, the emphasis should be on their functional diversity (Gupta et al., 2022; Aguilar-Paredes et al., 2023). It is essential to have a thorough understanding of the function of microbes in agroecosystem processes, particularly in relation to crop growth and soil fertility, in order to achieve sustainable agricultural practices.
Soil microorganisms play a vital role in agricultural ecosystems by contributing to the decomposition of soil organic matter, maintaining soil fertility, facilitating nutrient cycling for plants, and supporting overall productivity (Etesami and Adl, 2020b). They are essential for the transformation and movement of nutrients from the soil to plants. The structure and composition of microbial communities in the soil are often used as a key indicator of soil quality (Jacoby et al., 2017). Moreover, soil microorganisms are indispensable for maintaining soil functions, including carbon and nitrogen cycles, as well as the metabolism of organic matter (Paul and Frey, 2023). The diversity of soil microbes serves as a significant indicator for evaluating ecosystem function (Luo et al., 2018). Numerous studies have highlighted the vital role of soil microbes as environmental indicators of soil quality, as they contribute to preserving the biological activities of soils, enhancing soil nutrient cycling, and promoting the formation of soil structure (Sharma et al., 2011; Schloter et al., 2018). However, the soil microbiome is constantly challenged by various environmental stresses, which can significantly alter its composition, diversity, and overall functionality (Chu, 2018; Etesami, 2018; Abdul Rahman et al., 2021).
Environmental stresses can have profound impacts on the soil microbiome (Khoshru et al., 2020; Abdul Rahman et al., 2021). These stresses can disrupt the delicate balance of the soil ecosystem, leading to shifts in microbial community structure, changes in metabolic activities, and reduced ecosystem services (Jansson and Hofmockel, 2020; Abdul Rahman et al., 2021; Vincze et al., 2024). For example, drought stress increases Gram-positive bacteria (e.g., Actinobacteria) while decreasing Gram-negative bacteria (e.g., Proteobacteria), reducing nutrient cycling and soil moisture (Ward et al., 2009; Barnard et al., 2013; Meisner et al., 2018; Flórián et al., 2019; Denardin et al., 2020). Salinity disrupts plant water relations and nutrient uptake, lowering microbial biomass and activity, with fungi being more vulnerable than bacteria (Zhang et al., 2019; Etesami and Adl, 2020a). Heavy metals like cadmium and lead select for tolerant taxa but impair microbial metabolism, leading to decreased diversity (Song et al., 2021b). Flooding compacts soil, reducing oxygen for aerobic organisms and favoring anaerobic bacteria, while altering pH and nutrient status (Bai et al., 2000; Graff and Conrad, 2005; Furtak et al., 2020; Grzyb et al., 2020). Temperature stress shifts microbial community structures, often decreasing alpha diversity and increasing beta diversity, impacting nutrient cycling and organic matter decomposition (Goicoechea et al., 2010; Frater et al., 2018; Jerbi et al., 2020). Agrochemicals in conventional farming reduce microbial diversity, disrupt soil health, and impact enzyme activities (Milošević and Govedarica, 2002; Santos et al., 2006; Kremer and Means, 2009). Changes in soil pH also influence microbial composition, with higher pH generally increasing bacterial diversity (Jones et al., 2009b; Malik et al., 2018; Smercina et al., 2019). Overall, these stresses lead to significant shifts in microbial community dynamics and soil health.
In recent years, the use of silicon (Si) has emerged as a promising strategy to mitigate the adverse effects of environmental stresses on the soil microbiome. Silicon is the second most abundant element in the Earth’s crust and has been shown to play a crucial role in soil parameters such as nutrient and water availability, as well as aggregate stability (Schaller et al., 2020, Schaller et al., 2021a, Schaller et al., 2022; Barbosa et al., 2024). It enhances plant growth, stress tolerance, and overall ecosystem resilience (Etesami and Jeong, 2018; Etesami and Jeong, 2020; Etesami et al., 2022a; Etesami and Jeong, 2023). Numerous studies have demonstrated that the application of Si can significantly alter the composition and diversity of the soil microbial community, even under stressful conditions (Leonard, 2019; Deng et al., 2021; Song et al., 2021b; Ahmad et al., 2024; Leite et al., 2024). However, the practical mechanisms through which Si can affect the soil microbiome are not well defined and are at the beginning of the research stages, requiring further investigation. Some of the potential mechanisms may include changes in soil pH, nutrient availability, and plant-microbe interactions (Etesami and Jeong, 2018; Etesami and Adl, 2020a; Putra et al., 2020; Etesami et al., 2021). Additionally, Si-induced changes in crop physiology and root exudation patterns can also have cascading impacts on the soil microbiome (Fan et al., 2016; Putra et al., 2020; Etesami et al., 2022a; Etesami and Glick, 2024). The aim of this review paper was to synthesize the current understanding of the effects of various environmental stresses on the soil microbiome and to explore the potential of Si as a mitigating agent in enhancing the resilience of the soil microbial community. Given the critical role of the soil microbiome in maintaining ecosystem function and agricultural productivity, and the growing challenges posed by environmental stresses, this review paper is essential in providing a comprehensive overview of the latest research and insights that can inform sustainable soil management practices and contribute to the development of more resilient and productive agricultural systems.
2 Importance of soil microbiome
Microorganisms are among the most abundant living organisms on Earth, accounting for approximately 17% of global biomass (Bar-On et al., 2018). Soil is considered the most complex habitat, harboring an immense abundance of microbial life, estimated to comprise around 4-5 × 1030 microbial cells. The soil microbiome consists mainly of soil archaea, bacteria, viruses, and fungi (Dubey et al., 2019). Mendes et al. (2013) estimate that 108–109 bacteria, 107–108 viruses, and 105–106 fungal cells occupy just one gram of soil. The soil microbiome plays a vital role in various aspects of crop growth, soil health and fertility, nutrient cycling, decomposition of organic matter, bioremediation of pollutants, and ecosystem functioning. These microorganisms are essential components of terrestrial ecosystems, contributing to overall ecosystem functioning and services (Sharma et al., 2011; Sahu et al., 2017; Banerjee and van der Heijden, 2023; Vincze et al., 2024). Soil also provides habitats for crop pathogenic microorganisms and opportunistic human pathogenic microorganisms (Mendes et al., 2013). Research related to soil microbiota has gained significant attention due to its pivotal role in the global carbon cycle and climate change and its importance for sustainable agricultural practices.
The rhizosphere, the region surrounding plant roots, serves as a critical interface for interactions between plants and the soil microbiome. It acts as a biological hotspot where complex interactions among plants, microbes, and other organisms take place. Plant roots secrete organic compounds that nourish and support the activity of microorganisms in the rhizosphere (Massalha et al., 2017). Rhizosphere soil contains 108–1011 cultivable cells in one gram of soil, corresponding to approximately 104 microbial species (Saleem et al., 2019). Among the microbes associated with plants, bacteria and fungi are crucial components of the plant microbiome, playing complementary roles in enhancing plant growth, nutrient acquisition, abiotic and biotic stress tolerance, and overall ecosystem functioning (Etesami and Maheshwari, 2018; Begum et al., 2019). The diversity and composition of the bacterial community in the rhizosphere vary among crop plants, showing greater disparities among plant types like legumes, forbs, and grasses (Vincze et al., 2024). The rhizosphere microbiome consists of a variety of bacterial groups belonging to phyla such as Actinobacteria, Acidobacteria, Ascomycota, Bacteroidetes, Basidiomycota, Euryarchaeota, Deinococcus-Thermus, Firmicutes, and Proteobacteria (Yadav et al., 2018). Among the bacterial phyla, Proteobacteria and Acidobacteria are the most abundant bacterial groups in rhizosphere soil, playing key roles in carbon and nutrient cycling (Islam et al., 2020). They promote plant growth (Gahan and Schmalenberger, 2014; Smercina et al., 2019), suppress plant pathogens, fix nitrogen (N2), decompose recalcitrant organic matter, and produce beneficial compounds such as antibiotics and auxins (Bhatti et al., 2017; Ebrahimi-Zarandi et al., 2023) (Figure 1). Plant growth-promoting rhizobacteria (PGPR), such as those from the Rhizobiaceae family, form symbiotic relationships with plants, directly improving nutrient availability, root growth, and stress tolerance while indirectly suppressing pathogens. PGPR can reduce fertilizer use by 50% while enhancing plant nutrient uptake and yield. Rhizosphere and endophytic bacteria can also produce plant hormones like auxin, gibberellin, and cytokinin, which regulate plant development, while also lowering plant ethylene levels to mitigate stress (Etesami, 2018; Etesami and Maheshwari, 2018; Etesami and Adl, 2020b; Etesami et al., 2023). Nitrogen-fixing diazotrophs, a group of PGPR, serve as a major nitrogen source in soils, accounting for 30-50% of the total nitrogen in crop fields (Rosenblueth et al., 2018; Smercina et al., 2019). The presence of these bacteria is crucial for plant health, facilitating growth and nutrient uptake. These bacteria can also enhance the resilience of plants against biotic and abiotic stresses, making them essential for sustainable agricultural practices. Archaea also contribute to soil function by playing roles in carbon, nitrogen, and sulfur cycling. Ammonia-oxidizing Thaumarchaeota are particularly abundant and versatile in soil environments. However, the nitrification carried out by archaea can lead to nitrate leaching and greenhouse gas emissions (Ren et al., 2018; Baker et al., 2020; Wang et al., 2020b).
Fungi exhibit high plasticity and can thrive in diverse environmental conditions. They act as decomposers, producing extracellular enzymes to convert organic matter to CO2, and can mitigate metal toxicity by absorbing heavy metals (Islam et al., 2020). Fungi are recognized as biological controllers, helping to manage phytopathogenic fungal diseases (Iqbal et al., 2021; Yan and Khan, 2021). Additionally, fungal communities are pivotal in nutrient cycling and uptake (Khan et al., 2010; Vergara et al., 2017). Mycorrhizal fungi form symbiotic relationships with crop roots, enhancing nutrient and water uptake and increasing tolerance to biotic and abiotic stresses (Sun et al., 2018; Islam et al., 2020; Abdul Rahman et al., 2021) (Figure 2). They also play a critical role in solubilizing and enhancing the availability of phosphorus, a key nutrient for plant growth (Begum et al., 2019; Abdul Rahman et al., 2021).
A diverse and balanced soil microbiome is essential for sustaining soil fertility, productivity, and resilience to environmental stresses. Disruption of the soil microbiome can result in soil degradation, reduced crop yields, and heightened susceptibility to pests and diseases. Therefore, understanding and managing the soil microbiome is crucial for sustainable agriculture and for preserving the ecosystem services provided by soils.
3 Abiotic drivers of soil microbial diversity and function
Soil microbiomes are highly sensitive to various abiotic factors that can significantly influence their composition and functions (Abdul Rahman et al., 2021) (Figure 3). Soil pH is a primary driver, as beneficial microbes and plants prefer a neutral pH range of 6-7 (Sullivan et al., 2017). Changes in soil acidity or alkalinity often lead to shifts in the microbial community, with acidic soils reducing the diversity of nitrogen-fixing bacteria (Smercina et al., 2019), and different bacterial groups exhibiting varying pH tolerances (Jones et al., 2009b; Malik et al., 2018; Smercina et al., 2019). Soil temperature also plays a crucial role, as it affects the growth and activity of mesophilic, psychrophilic, and thermophilic microbes (Frater et al., 2018; Keenleyside, 2019; Jerbi et al., 2020). Increased temperatures can favor certain pathogens and alter mycorrhizal associations (Goicoechea et al., 2010; Frater et al., 2018; Jerbi et al., 2020). Soil aeration is another important factor, as hypoxic or waterlogged conditions disrupt aerobic microbes and nitrogen fixation (Smercina et al., 2019), prompting diazotrophs to employ various strategies to maintain nitrogen fixation under oxygen stress (Smercina et al., 2019). The soil’s physico-chemical properties—such as soil texture, structure, and organic matter content—play a crucial role in shaping microbial communities and their functions (Gupta and Roper, 2010; Smercina et al., 2019; Mosaffaei et al., 2020; Scudeletti et al., 2021). Soil compaction and depth can negatively affect fungal populations. Soil moisture levels significantly influence soil microbial respiration, biomass, and community structure (Sorensen et al., 2013; Yan et al., 2019; Grzyb et al., 2020; Siebielecet al., 2020). Excess soil moisture can be particularly harmful to aerobic microbes, as it limits oxygen availability (Yan et al., 2019; Sales Da Silva et al., 2020; Siebielec et al., 2020).
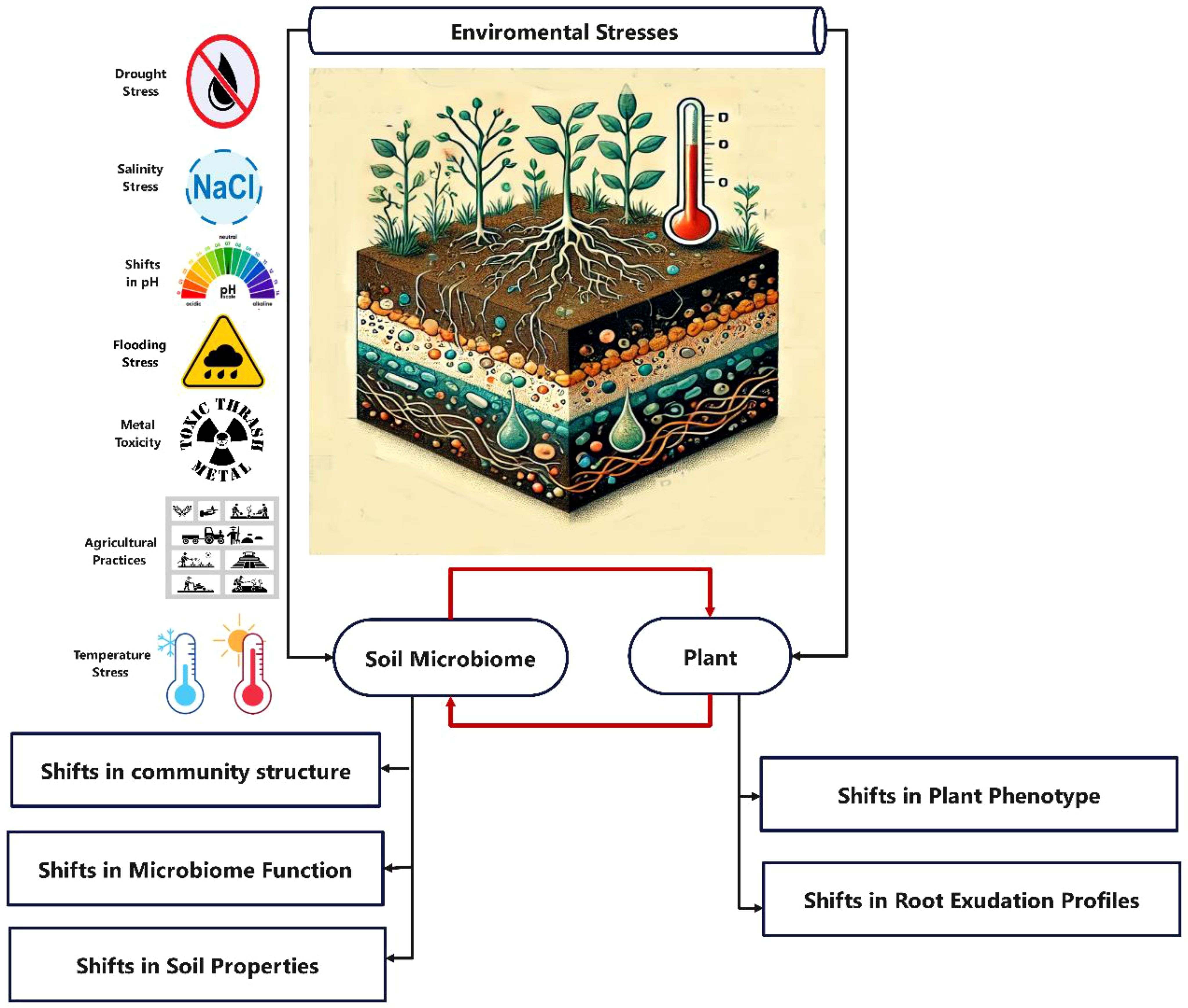
Figure 3. Effects of environmental stress on plant and soil microbiome. Environmental stress impacts the soil microbiome both directly and indirectly through changes in plant root secretions. For example, drought stress increases Gram-positive bacteria (e.g., Actinobacteria and Firmicutes) while decreasing Gram-negative ones (e.g., Proteobacteria and Bacteroidetes). It also leads to reduced nutrient cycling, lower soil moisture, and increased solute concentrations. Salinity disrupts plant growth and reduces soil microbial activity by creating ion imbalances and high osmotic pressures. This lowers microbial biomass, particularly affecting fungi more than bacteria, thus increasing the bacterium/fungi ratio. Heavy metals (Cd, Pb, Cr, and Hg) can damage microbial cells, impair metabolism, and reduce diversity. Polluted areas often show a prevalence of resistant taxa, such as Firmicutes and Actinobacteria, while arbuscular mycorrhizal fungi (AMF) may adapt to metal-contaminated soils. Flooding restricts oxygen exchange and alters soil pH, favoring anaerobic bacteria while declining aerobic ones. It may reduce fungal populations but can increase AMF density under some conditions. Certain bacteria can survive submergence, showcasing microbial resilience. Temperature stress causes shifts in microbial community structures, typically decreasing alpha diversity while increasing beta diversity. It can enhance enzyme activities at optimal temperatures but may denature them at higher extremes, affecting nutrient cycling. Warmer temperatures can facilitate AMF colonization, while colder temperatures hinder it. Agrochemical use in conventional farming often reduces microbial diversity, leading to ecological homogenization and impaired soil health. Long-term mineral fertilizer use can lower soil pH and disrupt microbial communities. Similarly, herbicides and fungicides can harm beneficial microbes and alter community dynamics. Soil pH greatly influences microbial composition and function. Generally, bacterial diversity increases with pH, while acidic conditions can reduce it, impacting key soil functions like nutrient cycling and organic matter decomposition. These stresses can also alter carbon exudation from plants, impacting substrate availability and significantly changing microbial community composition.
Drought can significantly impact microbial growth, activity, and community composition, favoring the proliferation of drought-tolerant taxa like Actinobacteria and Firmicutes that can produce spores and osmolytes to withstand desiccation (Ward et al., 2009; Barnard et al., 2013; Meisner et al., 2018; Flórián et al., 2019; Denardin et al., 2020). Conversely, flooding and waterlogged conditions promote the dominance of anaerobic bacteria while suppressing aerobic microbes and fungal communities (Bai et al., 2000; Graff and Conrad, 2005; Furtak et al., 2020; Grzyb et al., 2020). The reduced oxygen availability and changes in soil pH and nutrient status during submergence induce major shifts in the microbial community structure (Rosenblueth et al., 2018; Sánchez-Rodríguez et al., 2018; Hartman and Tringe, 2019). Heavy metal contamination significantly affects soil microbiomes, with Firmicutes, Proteobacteria, and Actinobacteria often dominating polluted soils. Arbuscular mycorrhizal fungi can colonize nutrient-poor, metal-laden environments, but high levels of metals like cadmium can hinder key life cycle stages, such as sporulation and mycelium expansion (Fomina et al., 2005; Abdu et al., 2017; Ma et al., 2021; Etesami and Glick, 2023). Heavy metals inhibit soil enzyme activities, reducing biological activity and potentially leading to the selection of metal-resistant microbial strains (Song et al., 2021b). Microbes adapt to metal toxicity through strategies like producing metal-chelating organic acids and developing metal-tolerant enzymes (Khan et al., 2000; Fomina et al., 2005; Seneviratne et al., 2017).
The extensive use of chemical fertilizers and pesticides in agriculture can be detrimental to the ecosystem and human health. Heavy metal contamination is a widespread issue in agricultural fields and farms as a result of these chemical inputs (Ali et al., 2019; Alengebawy et al., 2021; Nadarajah et al., 2021). However, the impact on the soil microbiome is complex and varied. Soil microbes are highly sensitive to fertilization practices, and their reactions to organic and inorganic fertilizers have received considerable attention (Hartmann et al., 2015; Fu et al., 2017). Fertilization can influence soil microbial diversity by altering the nutrient concentration in the soil (Bell et al., 2015). The long-term use of mineral fertilizers, especially nitrogen, can decrease microbial biomass and shift community composition, potentially due to soil acidification and metal contaminants (Holík et al., 2019; Wu et al., 2020). Emerging nano-fertilizer technologies can also impact the soil microbiome, with both positive and negative effects reported (Rajput et al., 2018; Gupta and Prakash, 2020). Pesticides, including herbicides, insecticides, and fungicides, are widely used to control weeds, pests, and pathogens. However, these chemicals can have detrimental impacts on soil microbes, reducing microbial abundance, diversity, and key functional processes like nitrogen fixation (Milošević and Govedarica, 2002; Santos et al., 2006; Kremer and Means, 2009). The specific effects depend on the pesticide type, dose, and interactions with other soil factors. Organic pesticides are generally less harmful than synthetic ones, but concerns remain about their environmental impacts (Bahlai et al., 2010; Biondi et al., 2012). It is important to note that the environmental stresses mentioned can have an indirect impact on the soil microbiome through their effects on plants. Plants play a significant function in shaping the composition and diversity of the soil microbiome in the rhizosphere through mechanisms such as altering root architecture, releasing root exudates, and forming symbiotic relationships (Abdul Rahman et al., 2021). Overall, the environmental stresses chemicals pose risks to the soil microbiome and ecosystem health. Across these stresses, the ability of the soil microbiome to adapt and maintain functional diversity is crucial for sustaining soil health and ecosystem processes in the face of environmental change.
4 Silicon
Silicon is the second most abundant element in the Earth’s crust, after oxygen, and plays a crucial role in the growth and development of many plant species, particularly grasses and cereals. Although Si is not considered an essential nutrient for plants, it is now recognized as a beneficial element that can enhance crop tolerance to both biotic and abiotic stresses (Epstein, 1994). In soils, Si exists in various forms, including soluble silicic acid, amorphous silica, and crystalline silicates, and its availability for plant uptake depends on factors such as soil pH, moisture, and the presence of other elements (Epstein, 1994; Ma and Yamaji, 2006). For example, amorphous silica has recently been shown to be an important factor influencing several soil traits, such as nutrient availability (Schaller et al., 2022), and water availability (Schaller et al., 2020; Zarebanadkouki et al., 2022), which depend on soil texture (Zarebanadkouki et al., 2024). It also improves aggregate stability (Barbosa et al., 2024). All of these parameters can significantly affect soil microbial community structure (Lewin et al., 2024). The availability of silicic acid for microbes is influenced by mineralogy and the content of amorphous silica, which serves as the primary source of silicic acid (Schaller et al., 2021a).
Once absorbed, Si can confer numerous benefits to plants, including structural support through deposition in cell walls and other plant tissues, improved tolerance to stresses such as water deficit, salinity, heavy metal toxicity, extreme temperatures, and fungal diseases, regulation of nutrient uptake and utilization, stimulation of antioxidant activity, and enhancement of crop yields and quality (Epstein, 1994; Yongchao et al., 2015; Etesami and Jeong, 2018; Etesami and Jeong, 2020; Etesami et al., 2022a; Etesami and Jeong, 2023). The beneficial effects of Si on plants have led to increased interest in the use of Si-based fertilizers and amendments in sustainable agriculture, but their impact on soil biological properties is still not clear. Understanding the mechanisms by which Si interacts with plants, soil, and microorganisms is crucial for developing effective strategies to optimize Si management and harness its potential for improved crop performance and environmental resilience.
5 Modulation of the soil microbiome by Si application
The application of Si has emerged as a promising approach to alleviate the negative impacts of environmental stresses on soil microbiomes (Table 1). Research indicates that Si can significantly stimulate beneficial soil bacteria. For instance, Karunakaran et al. (2013) demonstrated that nanosilica application doubled the total soil bacterial population, enhancing microbial biomass and activity. Specifically, the viability of PGPR, including Bacillus megaterium, B. brevis, P. fluorescens, and Azotobacter vinelandii, increased by over 20% in the presence of nanosilica. Silicon not only boosts bacterial abundance but also alters microbial community diversity and composition. Lin et al. (2020) found that Si application influenced 63.7% of the operational taxonomic units (OTUs) in the soil, highlighting a direct effect on microorganisms independent of crop presence. This alteration has been linked to improved soil biological properties and overall ecosystem functioning, including enhanced carbon/nitrogen cycling genes and metal detoxification in contaminated soils. In various studies, Si application has been shown to enhance microbial populations in crops like tomatoes (Wang et al., 2013) and in environments with heavy metal contamination (Wang et al., 2020a). Moreover, Si-rich amendments, such as rice husk and calcium silicate, have been reported to modify specific microbial communities tied to soil processes like arsenic metabolism (Dykes et al., 2021).
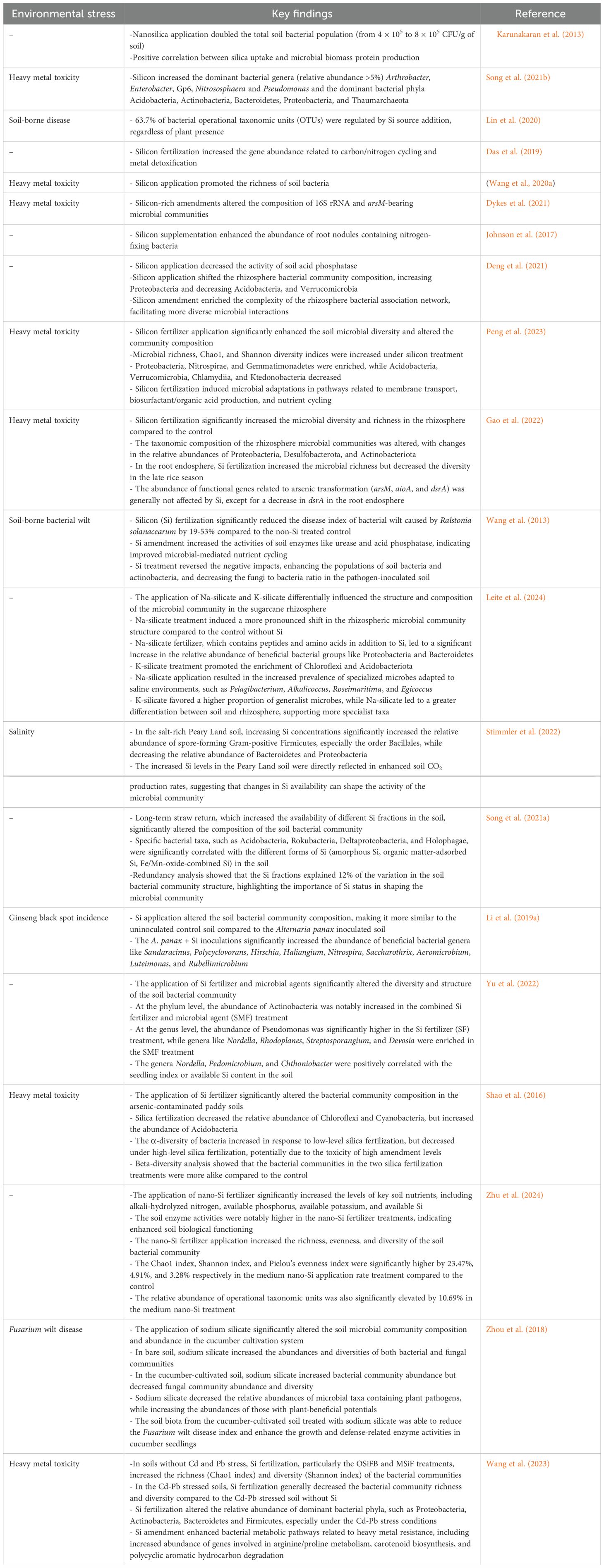
Table 1. The summarization of key findings on the changes in microbial community composition and enzyme activities in response to silicon (Si) application.
The beneficial effects of Si extend to legume root-microbe symbiosis, where Medicago sativa showed a 44% increase in nitrogen-fixing root nodules upon Si supplementation (Johnson et al., 2017). This improvement may arise from Si stimulating signaling compounds that enhance nodulation or increasing the abundance of nitrogen-fixing bacteria in root nodules. Silicon applications also favor certain bacterial groups capable of producing plant growth-promoting compounds. For example, Pseudomonas species that produce indole-3-acetic acid and solubilize phosphorus were found to increase following Si treatment (Song et al., 2021b). California-based studies showed that Si helped microbial communities thrive in heavy metal-contaminated soils by improving overall soil properties and nutrient availability while mitigating heavy metal toxicity (Zhang et al., 2019). Studies noted shifts in soil biological properties in response to Si addition. For example, one study observed decreased soil acid phosphatase activity, potentially due to increased phosphorus availability. The application of Si altered microbial composition, increasing Proteobacteria while decreasing Acidobacteria and Verrucomicrobia, driven by changes in soil chemistry including pH and nutrient levels (Deng et al., 2021). Silicon fertilization significantly impacts microbial richness and diversity, correlating positively with lower cadmium (Cd) accumulation in rice plants. Analysis revealed that Si treatment prompted microbial adaptations beneficial for nutrient cycling and Cd immobilization, with factors like soil pH and nutrient levels influencing community variations (Peng et al., 2023). In the rhizosphere of rice plants, long-term Si application enhanced microbial diversity and richness, particularly favoring groups like Proteobacteria and Actinobacteriota (Gao et al., 2022). It also reduced the bioavailability of arsenic, demonstrating potential for mitigating toxic influences on plants. Furthermore, Si application has been shown to enhance disease resistance in various crops. For example, in tomato plants, Si treatment reduced the incidence of soil-borne diseases caused by Ralstonia solanacearum, aiding nutrient cycling and microbial community activity (Wang et al., 2013). The type of Si source utilized also influences microbial community structure. For instance, sodium silicate was found to promote beneficial bacterial groups while inhibiting disease-related fungal taxa in cucumber cultivation, thus enhancing growth and defense against Fusarium wilt (Zhou et al., 2018). A field plot study on wheat cultivation found that the application of amorphous silica to the soil not only increased wheat yield during drought (Schaller et al., 2021b) but also altered the microbial community structure, resulting in an increase in beneficial microbes and a reduction in pathogenic microbes (Lewin et al., 2024).
Silicon’s role in modulating microbial communities extends to ginseng cultivation, where it reduced disease severity from Alternaria panax by restructuring soil bacterial community composition (Li et al., 2019a). Additionally, the combination of Si fertilizer with microbial agents significantly improved plant growth and microbial dynamics in lily crops, indicating a synergistic effect (Yu et al., 2022). Results from arsenic-contaminated soils show that Si application can alter bacterial communities, reducing harmful taxa while enhancing beneficial ones, suggesting that Si treatment has significant implications for soil health and arsenic detoxification (Shao et al., 2016). In another investigation, Si significantly increased soil microbial activity and diversity (Shamshiripour et al., 2022). The addition of Si and phosphorus not only improved rice productivity but also enhanced key soil biological properties, such as enzyme activity (Jinger et al., 2020). Nanotechnology has further enhanced potential applications of Si; nano-Si fertilizers notably improved soil properties and microbial diversity in wheat cultivation, suggesting substantial benefits for soil health and productivity (Zhu et al., 2024). Overall, Si’s application can effectively reshape soil microbial communities across various contexts—from agricultural settings to contaminated environments—indicating its utility in enhancing ecosystem resilience to stressors. Understanding the mechanisms by which Si influences soil microbiomes, such as changes in pH, nutrient dynamics, and plant-microbe interactions (Figure 4), is key for harnessing its full potential. Future research should aim to clarify these mechanisms and explore practical applications for sustainable soil management, underscoring Si’s role in promoting agricultural sustainability. Further research is needed to elucidate the precise mechanisms underlying these Si-mediated changes in the soil microbial community and to explore the practical implications for sustainable soil management practices.
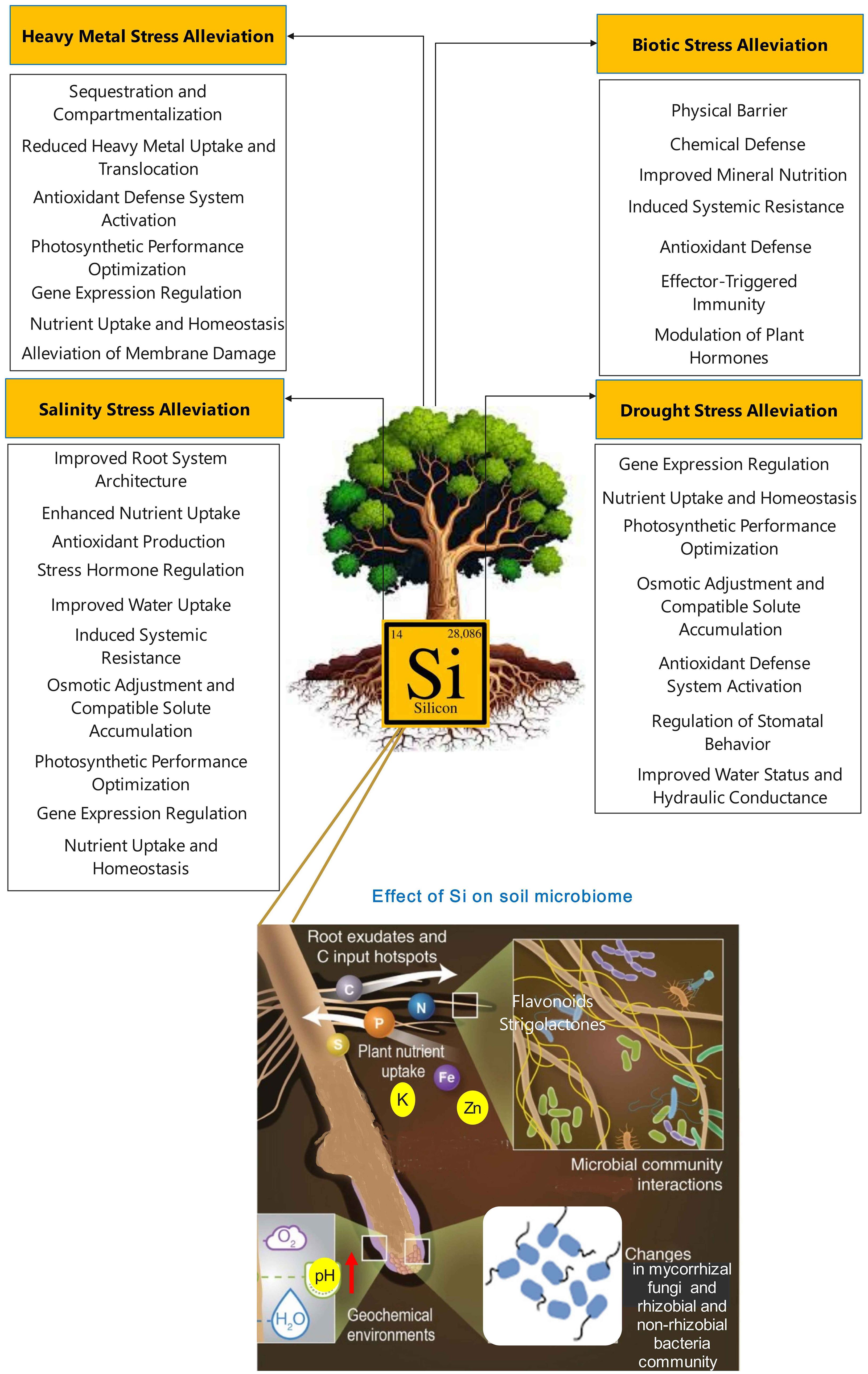
Figure 4. Some possible effects of silicon on the soil microbiome. Environmental stresses can impact the soil microbiome both directly and indirectly, particularly through their effects on plants (e.g., a decrease in labile carbon root exudates, an increase in carbon exudation per gram root, an increase in osmolyte synthesis, an increase in complex organic acids, a decrease in photosynthetic rate, a decrease in labile carbon production, a change in secondary metabolism, and a change in rooting architecture and depth). Similarly, silicon not only directly influences the soil microbiome but also mitigates the impact of environmental stresses on plants, thereby benefiting the microbiome indirectly.
6 Possible mechanisms of Si on the soil microbiome
6.1 An increase in soil pH
One of the key mechanisms by which Si enhances the soil microbiome is through an increase in soil pH. The toxicity of heavy metals is well known to have inhibitory effects on the soil microbial community (Etesami, 2018; Etesami, 2020). Soil pH is a critical factor affecting heavy metal bioavailability, as there is an inverse relationship between soil pH and heavy metal bioavailability (Govarthanan et al., 2016; Song et al., 2021b). Specifically, as soil pH increases, heavy metal accumulation in crops and microbes decreases. Silicon is an alkaline amendment that can contribute to a greater increase in soil pH levels. This enhancement in soil pH reduces the bioavailability of heavy metals in the soil, leading to a decrease in heavy metal uptake by plants and microbes (Liang et al., 2005; Etesami and Jeong, 2018; Wang et al., 2020a). The increase in soil pH alters the speciation of heavy metals, shifting them from the more bioavailable acid-soluble fraction to less bioavailable reducible, oxidizable, and residual forms (Liang et al., 2005; Song et al., 2009). For example, in a previous study, Si application increased soil pH from 5.15 to 6.13, which in turn reduced the bioavailability of cadmium (Cd) (Peng et al., 2023). By increasing soil pH, Si can reduce the bioavailability of heavy metals that are toxic to rhizobia and other beneficial soil microorganisms, creating a more favorable environment for rhizobial and non-rhizobial growth and activity (Putra et al., 2020). This reduction in heavy metal bioavailability due to the Si-induced increase in soil pH is a key mechanism by which Si enhances the soil microbial community, as it reduces the inhibitory effects of heavy metals on soil microorganisms.
6.2 Changes in plant root exudation patterns
One of the key mechanisms by which Si improves the soil microbiome is through changes in plant root exudation patterns. Root exudates contain a diverse array of primary metabolites (carbohydrates, amino acids, and organic acids) and secondary metabolites (flavonoids, glucosinolates, and auxins) that play crucial roles in mediating plant-microbe interactions within the rhizosphere (Vives-Peris et al., 2020; Etesami and Glick, 2024). These metabolites secreted by plant roots act as signaling compounds that attract and recruit beneficial microorganisms, such as mycorrhizal fungi and PGPR (Jones et al., 2009a). The specific composition of root exudates, including primary metabolites like sugars, organic acids, carboxylic acids, and amino acids, as well as secondary metabolites like flavonoids and strigolactones, can differentially shape the soil bacterial community structure and determine the types of microbes that are able to colonize the rhizosphere (Badri and Vivanco, 2009; Strehmel et al., 2014; Wen et al., 2022).
The application of Si has been shown to significantly improve root growth, increasing volume, secondary roots, and biomass (Guo et al., 2006; Etesami and Jeong, 2018). This enhancement of the root system leads to an increase in root secretions, as Si can stimulate the root-level exudation of organic compounds (Kidd et al., 2001). Importantly, Si can alter the composition of root exudates, potentially leading to increased secretion of metal-chelating organic acids such as acetic, tartaric, and maleic acid (Fan et al., 2016). Studies have reported that Si nanoparticles (SiO2 NPs) can further modulate root exudation patterns. SiO2 NPs have been shown to significantly increase the concentration of oxalic acid while decreasing levels of malic and citric acids in root exudates (Ghoto et al., 2020). This change in organic acid composition may be linked to the observed decrease in rhizosphere pH, as the solubility and speciation of these organic acids can be influenced by pH. Additionally, SiO2 NPs were found to increase superoxide anion production in roots, indicating they can induce oxidative stress, which could trigger changes in the antioxidant defense system and the production of organic compounds in root exudates (Ghoto et al., 2020). Silicon can enhance plant growth, photosynthetic efficiency, and overall productivity, leading to increased carbon inputs to the soil through root exudates and plant residues (Etesami et al., 2022b).
Conversely, the release of root secretions stimulated by Si can enhance the availability of additional Si in the rhizosphere. This released Si can, in turn, promote further root secretions, creating a cyclic process. For example, root-released exudates (e.g., carboxylates), such as those stimulated by Si, can play a key role in the weathering of soil minerals, including amorphous phases and phyllosilicates like smectite and mobilizing Si from soil minerals, thereby influencing the dynamics of Si in the rhizosphere (Gattullo et al., 2016; De Tombeur et al., 2021).
The available evidence indicates that Si can regulate soil organic carbon stabilization, influence carbon cycling in grasslands, and enhance carbon sequestration through phytolith formation and cellulose biosynthesis (Song et al., 2018; Liu et al., 2020). This suggests that Si-induced changes in soil carbon inputs and in root exudation patterns can influence the mobilization and cycling of soil nutrients, which in turn impacts the composition and function of the rhizosphere microbiome.
It is understood that Si boosts the performance of mycorrhizal fungi by enhancing nutrient absorption (such as through a rise in root exudates and root system activity), elevating carbon provision (such as through an augmentation in photosynthetic activity), overseeing phenolic metabolic routes (such as by aiding mycorrhizal fungi in their connection with host plants), and escalating soluble sugars in roots (such as by aiding in the attachment of mycorrhizal fungi onto the roots of host plants) (Etesami et al., 2022b, Etesami et al., 2022c; Etesami and Glick, 2023). Silicon can also play a multifaceted role in enhancing the legume-rhizobia symbiosis (Etesami and Adl, 2020a; Putra et al., 2020). Studies have shown that Si supplementation can significantly increase the number of root nodules formed (Nelwamondo and Dakora, 1999; Johnson et al., 2017). This suggests Si can promote the signaling and interactions between the legume host and its rhizobial partners (Putra et al., 2020). Additionally, Si supply has been found to elevate the concentrations of key flavonoids within the root nodules, including liquiritigenin, 2’-O-methylliquiritigenin, formononetin, and glycitein (Putra et al., 2021). Flavonoids are crucial signaling molecules that regulate the legume-rhizobia symbiosis by inducing rhizobial Nod genes (Cooper, 2004; Maj et al., 2010), and the Si-mediated increase in nodule flavonoids could enhance the chemical dialogue between the host and its microbial partners (Nelwamondo and Dakora, 1999; Johnson et al., 2017). In summary, Si-induced changes in root exudation patterns can have a significant impact on the composition and function of the rhizosphere microbiome, including both beneficial fungi (mycorrhizae) and bacteria (rhizobia). The altered exudation of organic compounds, including organic acids and phenolics, can shape the soil microbial community structure and influence the availability of soil nutrients, such as Si, Fe, and P, to support the growth and activity of these symbiotic microorganisms. This highlights the importance of understanding the complex interplay between plant, soil, and microbial components in the rhizosphere, and the role of Si in mediating these interactions.
6.3 An increase in plant tolerance to environmental stresses
It is known that microbes can thrive in various stressed areas, primarily owing to their host crops’ ability to survive in such conditions (Wang, 2017; Etesami and Glick, 2023). Silicon has been widely reported to alleviate various non-biological stresses (e.g., salinity, heavy metal toxicity, drought, nutritional imbalance) and biotic stresses (e.g., pathogens) in plants (Etesami and Jeong, 2018; Etesami and Jeong, 2020; Etesami et al., 2022a), which can indirectly benefit the plant-microbe interactions by maintaining plant health and vigor. Numerous studies are available that show how Si can increase plant resistance to biotic and abiotic stress, which need not be repeated in detail here. For example, After being taken up by the crop through the root system, Si can improve crop survival under heavy metal stress by diminishing metal absorption, limiting root-to-shoot translocation, chelating metals, and stimulating antioxidant systems (Li et al., 2019b; Seyfferth et al., 2019; Song et al., 2021b; Zhou et al., 2021; Etesami et al., 2022a). Moreover, Si-induced changes in root exudation patterns can lead to the increased secretion of metal-chelating organic compounds, such as acetic, tartaric, and maleic acids, which can help alleviate heavy metal toxicity (Fan et al., 2016). Silicon has also been found to stimulate the production of phenolic compounds in the plant rhizosphere, which can chelate and detoxify heavy metals (Guo et al., 2016). In general, Si helps to boost plant resistance to various environmental stresses by several mechanisms: stimulation of antioxidant systems, mitigation of photosynthesis inhibition, improvement of root morphology, stimulation of root exudation, complexation of heavy metals, up-regulation of nutrient transporter genes, and increase in soil nutrient bioavailability (Kidd et al., 2001; Fan et al., 2016; Kostic et al., 2017; Etesami and Jeong, 2018; Schaller et al., 2019; Pastore et al., 2020; Deng et al., 2021; Etesami et al., 2022a; Etesami and Schaller, 2023). By enhancing the plant’s tolerance to biotic and abiotic stresses, Si can indirectly support the growth and activity of beneficial microorganisms in the rhizosphere, as the plant maintains its vigor and provides a more favorable environment for microbial communities.
7 Conclusions and future research aspects
The review has highlighted the critical importance of the soil microbiome in maintaining the overall health and functioning of agricultural ecosystems. Soil microorganisms play pivotal roles in nutrient cycling, organic matter decomposition, crop growth promotion, and enhancing tolerance to biotic and abiotic stresses. However, the soil microbiome is constantly challenged by different environmental stresses, such as drought, salinity, heavy metal contamination, and climate change, which can significantly disrupt the delicate balance of the soil ecosystem. In this context, the application of Si has emerged as a promising strategy to mitigate the adverse effects of environmental stresses on the soil microbiome. Silicon can enhance the resilience of the soil microbial community through several mechanisms, including increasing soil pH, improving nutrient availability and uptake, altering root exudation patterns and plant physiology, and directly stimulating the abundance, diversity, and functional potential of key microbial groups. By enhancing the resilience of the soil microbiome, Si application can help maintain the critical ecosystem services provided by soil microorganisms, ultimately contributing to the sustainability and productivity of agricultural systems. Future research aspects include elucidating the precise mechanisms of Si-microbiome interactions under various environmental stresses, evaluating the long-term effects of Si on soil microbiome resilience, optimizing Si application strategies for specific crop-soil systems, integrating Si management with other sustainable soil practices, and assessing the impacts of Si on soil microbiome-mediated ecosystem services. By addressing these future research aspects, we can deepen our understanding of the mechanisms underlying Si-microbiome interactions and leverage this knowledge to develop more effective and resilient agricultural systems that can withstand the challenges posed by environmental stresses.
Author contributions
HE: Writing – original draft, Writing – review & editing.
Funding
The author(s) declare that no financial support was received for the research, authorship, and/or publication of this article.
Acknowledgments
Author wishes to appreciate University of Tehran, Iran, for making provision the needful facilities for the research.
Conflict of interest
The author declares that the research was conducted in the absence of any commercial or financial relationships that could be construed as a potential conflict of interest.
Publisher’s note
All claims expressed in this article are solely those of the author and do not necessarily represent those of their affiliated organizations, or those of the publisher, the editors and the reviewers. Any product that may be evaluated in this article, or claim that may be made by its manufacturer, is not guaranteed or endorsed by the publisher.
References
Abdu N., Abdullahi A. A., Abdulkadir A. (2017). Heavy metals and soil microbes. Environ. Chem. Lett. 15, 65–84. doi: 10.1007/s10311-016-0587-x
Abdul Rahman N. S. N., Abdul Hamid N. W., Nadarajah K. (2021). Effects of abiotic stress on soil microbiome. Int. J. Mol. Sci. 22.
Aguilar-Paredes A., Valdés G., Araneda N., Valdebenito E., Hansen F., Nuti M. (2023). Microbial community in the composting process and its positive impact on the soil biota in sustainable agriculture. Agronomy 13, 542. doi: 10.3390/agronomy13020542
Ahmad W., Coffman L., Weerasooriya A. D., Crawford K., Khan A. L. (2024). The silicon regulates microbiome diversity and plant defenses during cold stress in Glycine max L. Front. Plant Sci. 14, 1280251. doi: 10.3389/fpls.2023.1280251
Alengebawy A., Abdelkhalek S. T., Qureshi S. R., Wang M.-Q. (2021). Heavy metals and pesticides toxicity in agricultural soil and plants: Ecological risks and human health implications. Toxics 9, 42. doi: 10.3390/toxics9030042
Ali H., Khan E., Ilahi I. (2019). Environmental chemistry and ecotoxicology of hazardous heavy metals: environmental persistence, toxicity, and bioaccumulation. J. Chem. 2019, 6730305. doi: 10.1155/2019/6730305
Badri D. V., Vivanco J. M. (2009). Regulation and function of root exudates. Plant Cell Environ. 32, 666–681. doi: 10.1111/j.1365-3040.2009.01926.x
Bahlai C. A., Xue Y., Mccreary C. M., Schaafsma A. W., Hallett R. H. (2010). Choosing organic pesticides over synthetic pesticides may not effectively mitigate environmental risk in soybeans. PloS One 5, e11250. doi: 10.1371/journal.pone.0011250
Bai Q., Gattinger A., Zelles L. (2000). Characterization of microbial consortia in paddy rice soil by phospholipid analysis. Microbial Ecol. 39, 273–281.
Baker B. J., De Anda V., Seitz K. W., Dombrowski N., Santoro A. E., Lloyd K. G. (2020). Diversity, ecology and evolution of Archaea. Nat. Microbiol. 5, 887–900. doi: 10.1038/s41564-020-0715-z
Banerjee S., van der Heijden M. G. A. (2023). Soil microbiomes and one health. Nat. Rev. Microbiol. 21, 6–20. doi: 10.1038/s41579-022-00779-w
Barbosa L., Stein M., Gerke H. H., Schaller J. (2024). Synergistic effects of organic carbon and silica in preserving structural stability of drying soils. Sci. Rep. 14.
Barnard R. L., Osborne C. A., Firestone M. K. (2013). Responses of soil bacterial and fungal communities to extreme desiccation and rewetting. ISME J. 7, 2229–2241. doi: 10.1038/ismej.2013.104
Bar-On Y. M., Phillips R., Milo R. (2018). The biomass distribution on Earth. Proc. Natl. Acad. Sci. 115, 6506–6511. doi: 10.1073/pnas.1711842115
Begum N., Qin C., Ahanger M. A., Raza S., Khan M. I., Ashraf M., et al. (2019). Role of arbuscular mycorrhizal fungi in plant growth regulation: implications in abiotic stress tolerance. Front. Plant Sci. 10. doi: 10.3389/fpls.2019.01068
Bell C. W., Asao S., Calderon F., Wolk B., Wallenstein M. D. (2015). Plant nitrogen uptake drives rhizosphere bacterial community assembly during plant growth. Soil Biol. Biochem. 85, 170–182. doi: 10.1016/j.soilbio.2015.03.006
Bhatti A. A., Haq S., Bhat R. A. (2017). Actinomycetes benefaction role in soil and plant health. Microbial pathogenesis 111, 458–467. doi: 10.1016/j.micpath.2017.09.036
Biondi A., Desneux N., Siscaro G., Zappalà L. (2012). Using organic-certified rather than synthetic pesticides may not be safer for biological control agents: selectivity and side effects of 14 pesticides on the predator Orius laevigatus. Chemosphere 87, 803–812. doi: 10.1016/j.chemosphere.2011.12.082
Chu D. (2018). Effects of heavy metals on soil microbial community in IOP Conference Series: Earth and environmental science. (Bristol, United Kingdom: IOP Publishing), 012009.
Cooper J. E. (2004). “Multiple responses of rhizobia to flavonoids during legume root infection,” in Advances in botanical research (Amsterdam, Netherlands: Elsevier), 1–62.
Das S., Gwon H. S., Khan M. I., Van Nostrand J. D., Alam M. A., Kim P. J. (2019). Taxonomic and functional responses of soil microbial communities to slag-based fertilizer amendment in rice cropping systems. Environ. Int. 127, 531–539. doi: 10.1016/j.envint.2019.04.012
De Corato U. (2020). Towards new soil management strategies for improving soil quality and ecosystem services in sustainable agriculture: Editorial overview (Basel, Switzerland: MDPI).
Denardin L. G. D. O., Alves L. A., Ortigara C., Winck B., Coblinski J. A., Schmidt M. R., et al. (2020). How different soil moisture levels affect the microbial activity. Ciec. Rural 50, e20190831. doi: 10.1590/0103-8478cr20190831
Deng Q., Yu T., Zeng Z., Ashraf U., Shi Q., Huang S., et al. (2021). Silicon application modulates the growth, rhizosphere soil characteristics, and bacterial community structure in sugarcane. Front. Plant Sci. 12. doi: 10.3389/fpls.2021.710139
De Tombeur F., Cornelis J.-T., Lambers H. (2021). Silicon mobilisation by root-released carboxylates. Trends Plant Sci. 26, 1116–1125. doi: 10.1016/j.tplants.2021.07.003
Dubey A., Malla M. A., Khan F., Chowdhary K., Yadav S., Kumar A., et al. (2019). Soil microbiome: a key player for conservation of soil health under changing climate. Biodiversity Conserv. 28, 2405–2429. doi: 10.1007/s10531-019-01760-5
Dykes G. E., Limmer M. A., Seyfferth A. L. (2021). Silicon-rich soil amendments impact microbial community composition and the composition of arsM bearing microbes. Plant Soil 468, 147–164. doi: 10.1007/s11104-021-05103-8
Ebrahimi-Zarandi M., Etesami H., Glick B. R. (2023). Fostering plant resilience to drought with Actinobacteria: unveiling perennial allies in drought stress tolerance. Plant Stress 100242. doi: 10.1016/j.stress.2023.100242
Epstein E. (1994). The anomaly of silicon in plant biology. Proc. Natl. Acad. Sci. 91, 11–17. doi: 10.1073/pnas.91.1.11
Etesami H. (2018). Bacterial mediated alleviation of heavy metal stress and decreased accumulation of metals in plant tissues: Mechanisms and future prospects. Ecotoxicology Environ. Saf. 147, 175–191. doi: 10.1016/j.ecoenv.2017.08.032
Etesami H. (2020). “Plant–microbe interactions in plants and stress tolerance,” in Plant life under changing environment (Amsterdam, Netherlands: Elsevier), 355–396.
Etesami H., Adl S. M. (2020a). Can interaction between silicon and non–rhizobial bacteria help in improving nodulation and nitrogen fixation in salinity–stressed legumes? A review. Rhizosphere 15, 100229. doi: 10.1016/j.rhisph.2020.100229
Etesami H., Adl S. M. (2020b). Plant growth-promoting rhizobacteria (PGPR) and their action mechanisms in availability of nutrients to plants. Phyto-Microbiome Stress Regul. 147-203.
Etesami H., Al Saeedi A. H., El-Ramady H., Fujita M., Pessarakli M., Hossain M. A. Eds. (2022a). Silicon and nano-silicon in environmental stress management and crop quality improvement: progress and prospects. (1st ed.). (Amsterdam, Netherlands: Elsevier).
Etesami H., Glick B. R. (2023). Exploring the potential: Can mycorrhizal fungi and hyphosphere silicate-solubilizing bacteria synergistically alleviate cadmium stress in plants. Curr. Res. Biotechnol. 6, 100158. doi: 10.1016/j.crbiot.2023.100158
Etesami H., Glick B. R. (2024). Bacterial indole-3-acetic acid: A key regulator for plant growth, plant-microbe interactions, and agricultural adaptive resilience. Microbiological Res. 281, 127602. doi: 10.1016/j.micres.2024.127602
Etesami H., Jeong B. R. (2018). Silicon (Si): Review and future prospects on the action mechanisms in alleviating biotic and abiotic stresses in plants. Ecotoxicology Environ. Saf. 147, 881–896. doi: 10.1016/j.ecoenv.2017.09.063
Etesami H., Jeong B. R. (2020). “Importance of silicon in fruit nutrition: Agronomic and physiological implications,” in Fruit crops (Amsterdam, Netherlands: Elsevier), 255–277.
Etesami H., Jeong B. R. (2023). “How does silicon help alleviate biotic and abiotic stresses in plants? Mechanisms and future prospects,” in Plant stress mitigators (Amsterdam, Netherlands: Elsevier), 359–402.
Etesami H., Jeong B. R., Glick B. R. (2021). Contribution of arbuscular mycorrhizal fungi, phosphate–solubilizing bacteria, and silicon to P uptake by plant. Front. Plant Sci. 12,699618. doi: 10.3389/fpls.2021.699618
Etesami H., Jeong B. R., Glick B. R. (2023). Potential use of Bacillus spp. as an effective biostimulant against abiotic stresses in crops—A review. Curr. Res. Biotechnol. 5, 100128.
Etesami H., Li Z., Maathuis F. J. M., Cooke J. (2022b). The combined use of silicon and arbuscular mycorrhizas to mitigate salinity and drought stress in rice. Environ. Exp. Bot. 201, 104955. doi: 10.1016/j.envexpbot.2022.104955
Etesami H., Maheshwari D. K. (2018). Use of plant growth promoting rhizobacteria (PGPRs) with multiple plant growth promoting traits in stress agriculture: Action mechanisms and future prospects. Ecotoxicology Environ. Saf. 156, 225–246. doi: 10.1016/j.ecoenv.2018.03.013
Etesami H., Schaller J. (2023). Improving phosphorus availability to rice through silicon management in paddy soils: A review of the role of silicate-solubilizing bacteria. Rhizosphere 100749. doi: 10.1016/j.rhisph.2023.100749
Etesami H., Shokri E., Jeong B. R. (2022c). “The combined use of silicon/nanosilicon and arbuscular mycorrhiza for effective management of stressed agriculture: Action mechanisms and future prospects,” in Silicon and nano-silicon in environmental stress management and crop quality improvement (Amsterdam, Netherlands: Elsevier), 241–264.
Fan X., Wen X., Huang F., Cai Y., Cai K. (2016). Effects of silicon on morphology, ultrastructure and exudates of rice root under heavy metal stress. Acta Physiologiae Plantarum 38, 197. doi: 10.1007/s11738-016-2221-8
Flórián N., Ladányi M., Ittzés A., Kröel-Dulay G., Ónodi G., Mucsi M., et al. (2019). Effects of single and repeated drought on soil microarthropods in a semi-arid ecosystem depend more on timing and duration than drought severity. PloS One 14, e0219975. doi: 10.1371/journal.pone.0219975
Fomina M., Hillier S., Charnock J., Melville K., Alexander I. J., Gadd G. (2005). Role of oxalic acid overexcretion in transformations of toxic metal minerals by Beauveria caledonica. Appl. Environ. Microbiol. 71, 371–381. doi: 10.1128/AEM.71.1.371-381.2005
Frater P. N., Borer E. T., Fay P. A., Jin V., Knaeble B., Seabloom E., et al. (2018). Nutrients and environment influence arbuscular mycorrhizal colonization both independently and interactively in Schizachyrium scoparium. Plant Soil 425, 493–506. doi: 10.1007/s11104-018-3597-6
Fu L., Penton C. R., Ruan Y., Shen Z., Xue C., Li R., et al. (2017). Inducing the rhizosphere microbiome by biofertilizer application to suppress banana Fusarium wilt disease. Soil Biol. Biochem. 104, 39–48. doi: 10.1016/j.soilbio.2016.10.008
Furtak K., Grządziel J., Gałązka A., Niedźwiecki J. (2020). Prevalence of unclassified bacteria in the soil bacterial community from floodplain meadows (fluvisols) under simulated flood conditions revealed by a metataxonomic approachss. Catena 188, 104448. doi: 10.1016/j.catena.2019.104448
Gahan J., Schmalenberger A. (2014). The role of bacteria and mycorrhiza in plant sulfur supply. Front. Plant Sci. 5, 723. doi: 10.3389/fpls.2014.00723
Gao Z., Jiang Y., Yin C., Zheng W., Nikolic N., Nikolic M., et al. (2022). Silicon fertilization influences microbial assemblages in rice roots and decreases arsenic concentration in grain: A five-season in-situ remediation field study. J. Hazardous Materials 423, 127180. doi: 10.1016/j.jhazmat.2021.127180
Gattullo C. E., Allegretta I., Medici L., Fijan R., Pii Y., Cesco S., et al. (2016). Silicon dynamics in the rhizosphere: Connections with iron mobilization. J. Plant Nutr. Soil Sci. 179, 409–417. doi: 10.1002/jpln.201500535
Ghoto K., Simon M., Shen Z.-J., Gao G.-F., Li P.-F., Li H., et al. (2020). Physiological and root exudation response of maize seedlings to TiO 2 and SiO 2 nanoparticles exposure. BioNanoScience 10, 473–485. doi: 10.1007/s12668-020-00724-2
Goicoechea N., Garmendia I., Sanchez-Diaz M., Aguirreolea J. (2010). Arbuscular mycorrhizal fungi (AMF) as bioprotector agents against wilt induced by Verticillium spp. in pepper: a review. Spanish J. Agric. Res. 8, 25–42. doi: 10.5424/sjar/201008S1-5300
Govarthanan M., Mythili R., Selvankumar T., Kamala-Kannan S., Rajasekar A., Chang Y.-C. (2016). Bioremediation of heavy metals using an endophytic bacterium Paenibacillus sp. RM isolated from the roots of Tridax procumbens. 3 Biotech. 6, 1–7. doi: 10.1007/s13205-016-0560-1
Graff A., Conrad R. (2005). Impact of flooding on soil bacterial communities associated with poplar (Populus sp.) trees. FEMS Microbiol. Ecol. 53, 401–415. doi: 10.1016/j.femsec.2005.01.009
Grzyb A., Wolna-Maruwka A., Niewiadomska A. (2020). Environmental factors affecting the mineralization of crop residues. Agronomy 10. doi: 10.3390/agronomy10121951
Guo B., Liu C., Ding N., Fu Q., Lin Y., Li H., et al. (2016). Silicon alleviates cadmium toxicity in two cypress varieties by strengthening the exodermis tissues and stimulating phenolic exudation of roots. J. Plant Growth Regul. 35, 420–429. doi: 10.1007/s00344-015-9549-y
Guo Z. G., Liu H. X., Tian F. P., Zhang Z. H., Wang S. M. (2006). Effect of silicon on the morphology of shoots and roots of alfalfa (Medicago sativa). Aust. J. Exp. Agric. 46, 1161–1166. doi: 10.1071/EA05117
Gupta C., Prakash D. (2020). Effect of nano-fertilizers on soil microflora. Ann. Plant Sci. 9, 3846–3859.
Gupta V., Roper M. (2010). Protection of free-living nitrogen-fixing bacteria within the soil matrix. Soil Tillage Res. 109, 50–54. doi: 10.1016/j.still.2010.04.002
Gupta A., Singh U. B., Sahu P. K., Paul S., Kumar A., Malviya D., et al. (2022). Linking soil microbial diversity to modern agriculture practices: a review. Int. J. Environ. Res. Public Health 19. doi: 10.3390/ijerph19053141
Hartman K., Tringe S. G. (2019). Interactions between plants and soil shaping the root microbiome under abiotic stress. Biochem. J. 476, 2705–2724. doi: 10.1042/BCJ20180615
Hartmann M., Frey B., Mayer J., Mäder P., Widmer F. (2015). Distinct soil microbial diversity under long-term organic and conventional farming. ISME J. 9, 1177–1194. doi: 10.1038/ismej.2014.210
Holík L., Hlisnikovský L., Honzík R., Trögl J., Burdová H., Popelka J. (2019). Soil microbial communities and enzyme activities after long-term application of inorganic and organic fertilizers at different depths of the soil profile. Sustainability 11. doi: 10.3390/su11123251
Iqbal M., Jamshaid M., Zahid M. A., Andreasson E., Vetukuri R. R., Stenberg J. A. (2021). Biological control of strawberry crown rot, root rot and grey mould by the beneficial fungus Aureobasidium pullulans. BioControl 66, 535–545. doi: 10.1007/s10526-021-10083-w
Islam W., Noman A., Naveed H., Huang Z., Chen H. Y. (2020). Role of environmental factors in shaping the soil microbiome. Environ. Sci. pollut. Res. 27, 41225–41247. doi: 10.1007/s11356-020-10471-2
Jacoby R., Peukert M., Succurro A., Koprivova A., Kopriva S. (2017). The role of soil microorganisms in plant mineral nutrition—current knowledge and future directions. Front. Plant Sci. 8. doi: 10.3389/fpls.2017.01617
Jansson J. K., Hofmockel K. S. (2020). Soil microbiomes and climate change. Nat. Rev. Microbiol. 18, 35–46. doi: 10.1038/s41579-019-0265-7
Jerbi M., Labidi S., Lounes-Hadj Sahraoui A., Chaar H., Ben Jeddi F. (2020). Higher temperatures and lower annual rainfall do not restrict, directly or indirectly, the mycorrhizal colonization of barley (Hordeum vulgare L.) under rainfed conditions. PloS One 15, e0241794. doi: 10.1371/journal.pone.0241794
Jinger D., Dhar S., Dass A., Sharma V., Shukla L., Parihar M., et al (2020). Crop productivity, grain quality, water use efficiency, and soil enzyme activity as influenced by silicon and phosphorus application in aerobic rice (Oryza sativa). Communications Soil Sci. Plant Anal. 51, 2147–2162.
Johnson S. N., Hartley S. E., Ryalls J. M., Frew A., Degabriel J. L., Duncan M., et al. (2017). Silicon-induced root nodulation and synthesis of essential amino acids in a legume is associated with higher herbivore abundance. Funct. Ecol. 31, 1903–1909. doi: 10.1111/1365-2435.12893
Jones D. L., Nguyen C., Finlay R. D. (2009a). Carbon flow in the rhizosphere: carbon trading at the soil–root interface (Dordrecht, Netherlands: Springer).
Jones R. T., Robeson M. S., Lauber C. L., Hamady M., Knight R., Fierer N. (2009b). A comprehensive survey of soil acidobacterial diversity using pyrosequencing and clone library analyses. ISME J. 3, 442–453. doi: 10.1038/ismej.2008.127
Karunakaran G., Suriyaprabha R., Manivasakan P., Yuvakkumar R., Rajendran V., Prabu P., et al. (2013). Effect of nanosilica and silicon sources on plant growth promoting rhizobacteria, soil nutrients and maize seed germination. IET Nanobiotechnology 7, 70–77. doi: 10.1049/iet-nbt.2012.0048
Khan A. G., Kuek C., Chaudhry T., Khoo C. S., Hayes W. J. (2000). Role of plants, mycorrhizae and phytochelators in heavy metal contaminated land remediation. Chemosphere 41, 197–207. doi: 10.1016/S0045-6535(99)00412-9
Khan M. S., Zaidi A., Ahemad M., Oves M., Wani P. A. (2010). Plant growth promotion by phosphate solubilizing fungi–current perspective. Arch. Agron. Soil Sci. 56, 73–98. doi: 10.1080/03650340902806469
Khoshru B., Moharramnejad S., Gharajeh N. H., Asgari Lajayer B., Ghorbanpour M. (2020). Plant microbiome and its important in stressful agriculture. Plant microbiome paradigm, 13–48.
Kidd P. S., Llugany M., Poschenrieder C., Gunsé B., Barceló J. (2001). The role of root exudates in aluminium resistance and silicon-induced amelioration of aluminium toxicity in three varieties of maize (Zea mays L.). J. Exp. Bot. 52, 1339–1352.
Kostic L., Nikolic N., Bosnic D., Samardzic J., Nikolic M. (2017). Silicon increases phosphorus (P) uptake by wheat under low P acid soil conditions. Plant Soil 419, 447–455. doi: 10.1007/s11104-017-3364-0
Kremer R. J., Means N. E. (2009). Glyphosate and glyphosate-resistant crop interactions with rhizosphere microorganisms. Eur. J. Agron. 31, 153–161. doi: 10.1016/j.eja.2009.06.004
Leite M. R. L., De Alcântara Neto F., Dutra A. F., Mendes L. W., Miranda R. D. S., Melo V. M. M., et al. (2024). Distinct sources of silicon shape differently the rhizospheric microbial community in sugarcane. Appl. Soil Ecol. 193, 105131. doi: 10.1016/j.apsoil.2023.105131
Leonard J. (2019). Effects of silicon and beneficial bacteria on sheath blight of rice and the microbial community of rice rhizosphere (Baton Rouge, Louisiana, USA: Louisiana State University and Agricultural & Mechanical College).
Lewin S., Schaller J., Kolb S., Francioli D. (2024). Amorphous silica fertilization ameliorated soil properties and promoted putative soil beneficial microbial taxa in a wheat field under drought. Appl. Soil Ecol. 105286. doi: 10.1016/j.apsoil.2024.105286
Li Z., Unzué-Belmonte D., Cornelis J.-T., Linden C. V., Struyf E., Ronsse F., et al. (2019b). Effects of phytolithic rice-straw biochar, soil buffering capacity and pH on silicon bioavailability. Plant Soil 438, 187–203. doi: 10.1007/s11104-019-04013-0
Li M., Wang Q., Liu Z., Pan X., Zhang Y. (2019a). Silicon application and related changes in soil bacterial community dynamics reduced ginseng black spot incidence in Panax ginseng in a short-term study. BMC Microbiol. 19, 263. doi: 10.1186/s12866-019-1627-z
Liang Y., Wong J., Wei L. (2005). Silicon-mediated enhancement of cadmium tolerance in maize (Zea mays L.) grown in cadmium contaminated soil. Chemosphere 58, 475–483. doi: 10.1016/j.chemosphere.2004.09.034
Lin W.-P., Jiang N.-H., Li P., Fan X.-Y., Yang G., Wang G.-P., et al. (2020). Silicon impacts on soil microflora under Ralstonia Solanacearum inoculation. J. Integr. Agric. 19, 251–264. doi: 10.1016/S2095-3119(18)62122-7
Liu L., Song Z., Yu C., Yu G., Ellam R. M., Liu H., et al. (2020). Silicon effects on biomass carbon and phytolith-occluded carbon in grasslands under high-salinity conditions. Front. Plant Sci. 11. doi: 10.3389/fpls.2020.00657
Luo G., Rensing C., Chen H., Liu M., Wang M., Guo S., et al. (2018). Deciphering the associations between soil microbial diversity and ecosystem multifunctionality driven by long-term fertilization management. Funct. Ecol. 32, 1103–1116. doi: 10.1111/1365-2435.13039
Ma J., Ullah S., Niu A., Liao Z., Qin Q., Xu S., et al. (2021). Heavy metal pollution increases CH4 and decreases CO2 emissions due to soil microbial changes in a mangrove wetland: Microcosm experiment and field examination. Chemosphere 269, 128735. doi: 10.1016/j.chemosphere.2020.128735
Ma J. F., Yamaji N. (2006). Silicon uptake and accumulation in higher plants. Trends Plant Sci. 11, 392–397. doi: 10.1016/j.tplants.2006.06.007
Maj D., Wielbo J., Marek-Kozaczuk M., Skorupska A. (2010). Response to flavonoids as a factor influencing competitiveness and symbiotic activity of Rhizobium leguminosarum. Microbiological Res. 165, 50–60. doi: 10.1016/j.micres.2008.06.002
Malik A. A., Puissant J., Buckeridge K. M., Goodall T., Jehmlich N., Chowdhury S., et al. (2018). Land use driven change in soil pH affects microbial carbon cycling processes. Nat. Commun. 9. doi: 10.1038/s41467-018-05980-1
Massalha H., Korenblum E., Tholl D., Aharoni A. (2017). Small molecules below-ground: the role of specialized metabolites in the rhizosphere (Hoboken, New Jersey, USA: Wiley Online Library).
Meisner A., Jacquiod S., Snoek B. L., Ten Hooven F. C., van der Putten W. H. (2018). Drought legacy effects on the composition of soil fungal and prokaryote communities. Front. Microbiol. 9,294. doi: 10.3389/fmicb.2018.00294
Mendes R., Garbeva P., Raaijmakers J. M. (2013). The rhizosphere microbiome: significance of plant beneficial, plant pathogenic, and human pathogenic microorganisms. FEMS Microbiol. Rev. 37, 634–663. doi: 10.1111/1574-6976.12028
Milošević N. A., Govedarica M. M. (2002). Effect of herbicides on microbiological properties of soil. Zbornik Matice srpske za prirodne nauke, 5–21. doi: 10.2298/ZMSPN0201005M
Mosaffaei Z., Jahani A., Chahouki M., Goshtasb H., Etemad V., Saffariha M. (2020). Soil texture and plant degradation predictive model (STPDPM) in national parks using artificial neural network (ANN). Modeling Earth Syst. Environ. 6, 715–729. doi: 10.1007/s40808-020-00723-y
Nadarajah K., Abdul Hamid N. W., Abdul Rahman N. S. N. (2021). SA-mediated regulation and control of abiotic stress tolerance in rice. Int. J. Mol. Sci. 22. doi: 10.3390/ijms22115591
Nelwamondo A., Dakora F. (1999). Silicon promotes nodule formation and nodule function in symbiotic cowpea (Vigna unguiculata). New Phytol. 142, 463–467. doi: 10.1046/j.1469-8137.1999.00409.x
Pastore G., Kernchen S., Spohn M. (2020). Microbial solubilization of silicon and phosphorus from bedrock in relation to abundance of phosphorus-solubilizing bacteria in temperate forest soils. Soil Biol. Biochem. 151, 108050. doi: 10.1016/j.soilbio.2020.108050
Paul E., Frey S. (2023). Soil microbiology, ecology and biochemistry. (Amsterdam, Netherlands: Elsevier).
Peng H., Deng K., Shi Y., Liu S., Jian Z., Li C., et al. (2023). Alleviation of Cd-polluted paddy soils through Si fertilizer application and its effects on the soil microbial community. Sci. Total Environ. 855, 158735. doi: 10.1016/j.scitotenv.2022.158735
Putra R., Powell J. R., Hartley S. E., Johnson S. N. (2020). Is it time to include legumes in plant silicon research? Funct. Ecol. 34, 1142–1157.
Putra R., Vandegeer R. K., Karan S., Powell J. R., Hartley S. E., Johnson S. N. (2021). Silicon enrichment alters functional traits in legumes depending on plant genotype and symbiosis with nitrogen-fixing bacteria. Funct. Ecol. 35, 2856–2869. doi: 10.1111/1365-2435.13912
Rajput V. D., Minkina T., Sushkova S., Tsitsuashvili V., Mandzhieva S., Gorovtsov A., et al. (2018). Effect of nanoparticles on crops and soil microbial communities. J. Soils Sediments 18, 2179–2187. doi: 10.1007/s11368-017-1793-2
Ren M., Zhang Z., Wang X., Zhou Z., Chen D., Zeng H., et al. (2018). Diversity and contributions to nitrogen cycling and carbon fixation of soil salinity shaped microbial communities in Tarim Basin. Front. Microbiol. 9, 431. doi: 10.3389/fmicb.2018.00431
Rosenblueth M., Ormeño-Orrillo E., López-López A., Rogel M. A., Reyes-Hernández B. J., Martínez-Romero J. C., et al. (2018). Nitrogen fixation in cereals. Front. Microbiol. 9. doi: 10.3389/fmicb.2018.01794
Sahu N., Vasu D., Sahu A., Lal N., Singh S. (2017). Strength of microbes in nutrient cycling: a key to soil health. Agriculturally Important Microbes Sustain. Agriculture: Volume I: Plant-soil-microbe nexus, 69–86.
Saleem M., Hu J., Jousset A. (2019). More than the sum of its parts: microbiome biodiversity as a driver of plant growth and soil health. Annu. Rev. ecology evolution systematics 50, 145–168. doi: 10.1146/annurev-ecolsys-110617-062605
Sales Da Silva I. G., Gomes De Almeida F. C., Padilha Da Rocha E Silva N. M., Casazza A. A., Converti A., Asfora Sarubbo L. (2020). Soil bioremediation: Overview of technologies and trends. Energies 13. doi: 10.3390/en13184664
Sánchez-Rodríguez A. R., Chadwick D. R., Tatton G. S., Hill P. W., Jones D. L. (2018). Comparative effects of prolonged freshwater and saline flooding on nitrogen cycling in an agricultural soil. Appl. Soil Ecol. 125, 56–70. doi: 10.1016/j.apsoil.2017.11.022
Santos J., Jakelaitis A., Silva A., Costa M., Manabe A., Silva M. (2006). Action of two herbicides on the microbial activity of soil cultivated with common bean (Phaseolus vulgaris) in conventional-till and no-till systems. Weed Res. 46, 284–289. doi: 10.1111/j.1365-3180.2006.00510.x
Schaller J., Cramer A., Carminati A., Zarebanadkouki M. (2020). Biogenic amorphous silica as main driver for plant available water in soils. Sci. Rep. 10.
Schaller J., Faucherre S., Joss H., Obst M., Goeckede M., Planer-Friedrich B., et al. (2019). Silicon increases the phosphorus availability of Arctic soils. Sci. Rep. 9, 449. doi: 10.1038/s41598-018-37104-6
Schaller J., Puppe D., Kaczorek D., Ellerbrock R., Sommer M. (2021a). Silicon cycling in soils revisited. Plants 10, 295. doi: 10.3390/plants10020295
Schaller J., Scherwietes E., Gerber L., Vaidya S., Kaczorek D., Pausch J., et al. (2021b). Silica fertilization improved wheat performance and increased phosphorus concentrations during drought at the field scale. Sci. Rep. 11, 20852. doi: 10.1038/s41598-021-00464-7
Schaller J., Wu B., Amelung W., Hu Z., Stein M., Lehndorff E., et al. (2022). Silicon as a potential limiting factor for phosphorus availability in paddy soils. Sci. Rep. 12, 16329. doi: 10.1038/s41598-022-20805-4
Schloter M., Nannipieri P., Sørensen S. J., Van Elsas J. D. (2018). Microbial indicators for soil quality. Biol. Fertility Soils 54, 1–10. doi: 10.1007/s00374-017-1248-3
Scudeletti D., Crusciol C., Bossolani J. W., Moretti L. G., Momesso L., Servaz Tubana B., et al. (2021). Trichoderma asperellum inoculation as a tool for attenuating drought stress in sugarcane. Front. Plant Sci. 12, 645542. doi: 10.3389/fpls.2021.645542
Seneviratne M., Seneviratne G., Madawala H., Vithanage M. (2017). Role of rhizospheric microbes in heavy metal uptake by plants. Agro-Environmental Sustainability: Volume 2: Managing Environ. pollut., 147–163.
Seyfferth A. L., Amaral D., Limmer M. A., Guilherme L. R. (2019). Combined impacts of Si-rich rice residues and flooding extent on grain As and Cd in rice. Environ. Int. 128, 301–309. doi: 10.1016/j.envint.2019.04.060
Shao J., He Y., Zhang H., Chen A., Lei M., Chen J., et al. (2016). Silica fertilization and nano-MnO2 amendment on bacterial community composition in high arsenic paddy soils. Appl. Microbiol. Biotechnol. 100, 2429–2437. doi: 10.1007/s00253-015-7131-y
Sharma S. K., Ramesh A., Sharma M. P., Joshi O. P., Govaerts B., Steenwerth K. L., et al. (2011). Microbial community structure and diversity as indicators for evaluating soil quality. Biodiversity biofuels Agroforestry Conserv. Agric., 317–358.
Shamshiripour M., Motesharezadeh B., Rahmani H. A., Alikhani H. A., Etesami H. (2022). Optimal concentrations of silicon enhance the growth of soybean (Glycine Max L.) cultivars by improving nodulation, root system architecture, and soil biological properties. Silicon 14, 5333–5345.
Siebielec S., Siebielec G., Klimkowicz-Pawlas A., Gałązka A., Grządziel J., Stuczyński T. (2020). Impact of water stress on microbial community and activity in sandy and loamy soils. Agronomy 10. doi: 10.3390/agronomy10091429
Smercina D. N., Evans S. E., Friesen M. L., Tiemann L. K. (2019). To fix or not to fix: controls on free-living nitrogen fixation in the rhizosphere. Appl. Environ. Microbiol. 85, e02546–e02518. doi: 10.1128/AEM.02546-18
Song A., Li Z., Liao Y., Liang Y., Wang E., Wang S., et al. (2021a). Soil bacterial communities interact with silicon fraction transformation and promote rice yield after long-term straw return. Soil Ecol. Lett. 3, 395–408. doi: 10.1007/s42832-021-0076-4
Song A., Li Z., Wang E., Xu D., Wang S., Bi J., et al. (2021b). Supplying silicon alters microbial community and reduces soil cadmium bioavailability to promote health wheat growth and yield. Sci. Total Environ. 796, 148797. doi: 10.1016/j.scitotenv.2021.148797
Song A., Li Z., Zhang J., Xue G., Fan F., Liang Y. (2009). Silicon-enhanced resistance to cadmium toxicity in Brassica chinensis L. @ is attributed to Si-suppressed cadmium uptake and transport and Si-enhanced antioxidant defense capacity. J. Hazardous Materials 172, 74–83. doi: 10.1016/j.jhazmat.2009.06.143
Song Z., Liu C., Müller K., Yang X., Wu Y., Wang H. (2018). Silicon regulation of soil organic carbon stabilization and its potential to mitigate climate change. Earth-Science Rev. 185, 463–475. doi: 10.1016/j.earscirev.2018.06.020
Sorensen P. O., Germino M. J., Feris K. P. (2013). Microbial community responses to 17 years of altered precipitation are seasonally dependent and coupled to co-varying effects of water content on vegetation and soil C. Soil Biol. Biochem. 64, 155–163. doi: 10.1016/j.soilbio.2013.04.014
Stimmler P., Priemé A., Elberling B., Goeckede M., Schaller J. (2022). Arctic soil respiration and microbial community structure driven by silicon and calcium. Sci. Total Environ. 838, 156152. doi: 10.1016/j.scitotenv.2022.156152
Strehmel N., Böttcher C., Schmidt S., Scheel D. (2014). Profiling of secondary metabolites in root exudates of Arabidopsis thaliana. Phytochemistry 108, 35–46. doi: 10.1016/j.phytochem.2014.10.003
Sullivan T. S., Barth V. P., Lewis R. W. (2017). Soil acidity impacts beneficial soil microorganisms (Pullman, Washington, USA: Washington State University Extension).
Sun Z., Song J., Xin X. A., Xie X., Zhao B. (2018). Arbuscular mycorrhizal fungal 14-3-3 proteins are involved in arbuscule formation and responses to abiotic stresses during AM symbiosis. Front. Microbiol. 9, 91. doi: 10.3389/fmicb.2018.00091
Vergara C., Araujo K. E., Urquiaga S., Schultz N., Balieiro F. D. C., Medeiros P. S., et al. (2017). Dark septate endophytic fungi help tomato to acquire nutrients from ground plant material. Front. Microbiol. 8. doi: 10.3389/fmicb.2017.02437
Vincze É.-B., Becze A., Laslo É., Mara G. (2024). Beneficial soil microbiomes and their potential role in plant growth and soil fertility. Agriculture 14, 152. doi: 10.3390/agriculture14010152
Vives-Peris V., De Ollas C., Gómez-Cadenas A., Pérez-Clemente R. M. (2020). Root exudates: from plant to rhizosphere and beyond. Plant Cell Rep. 39, 3–17. doi: 10.1007/s00299-019-02447-5
Wang F. (2017). Occurrence of arbuscular mycorrhizal fungi in mining-impacted sites and their contribution to ecological restoration: Mechanisms and applications. Crit. Rev. Environ. Sci. Technol. 47, 1901–1957. doi: 10.1080/10643389.2017.1400853
Wang H., Bier R., Zgleszewski L., Peipoch M., Omondi E., Mukherjee A., et al. (2020b). Distinct distribution of Archaea from soil to freshwater to estuary: implications of archaeal composition and function in different environments. Front. Microbiol. 11, 576661. doi: 10.3389/fmicb.2020.576661
Wang L., Cai K., Chen Y., Wang G. (2013). Silicon-mediated tomato resistance against Ralstonia solanacearum is associated with modification of soil microbial community structure and activity. Biol. Trace element Res. 152, 275–283. doi: 10.1007/s12011-013-9611-1
Wang B., Chu C., Wei H., Zhang L., Ahmad Z., Wu S., et al. (2020a). Ameliorative effects of silicon fertilizer on soil bacterial community and pakchoi (Brassica chinensis L.) grown on soil contaminated with multiple heavy metals. Environ. pollut. 267, 115411. doi: 10.1016/j.envpol.2020.115411
Wang B., Xiao L., Xu A., Mao W., Wu Z., Hicks L. C., et al. (2023). Silicon fertilization enhances the resistance of tobacco plants to combined Cd and Pb contamination: Physiological and microbial mechanisms. Ecotoxicology Environ. Saf. 255, 114816. doi: 10.1016/j.ecoenv.2023.114816
Ward N. L., Challacombe J. F., Janssen P. H., Henrissat B., Coutinho P. M., Wu M., et al. (2009). Three genomes from the phylum Acidobacteria provide insight into the lifestyles of these microorganisms in soils. Appl. Environ. Microbiol. 75, 2046–2056. doi: 10.1128/AEM.02294-08
Wen T., Yu G.-H., Hong W.-D., Yuan J., Niu G.-Q., Xie P.-H., et al. (2022). Root exudate chemistry affects soil carbon mobilization via microbial community reassembly. Fundam. Res. 2, 697–707. doi: 10.1016/j.fmre.2021.12.016
Wu L., Jiang Y., Zhao F., He X., Liu H., Yu K. (2020). Increased organic fertilizer application and reduced chemical fertilizer application affect the soil properties and bacterial communities of grape rhizosphere soil. Sci. Rep. 10.
Yadav A. N., Kumar V., Dhaliwal H. S., Prasad R., Saxena A. K. (2018). “Microbiome in crops: diversity, distribution, and potential role in crop improvement,” in Crop improvement through microbial biotechnology (Amsterdam, Netherlands: Elsevier), 305–332.
Yan Z., Dyck M., Cai H.-J., Song L.-B., Hui C. (2019). The effects of aerated irrigation on soil respiration, oxygen, and porosity. J. Integr. Agric. 18, 2854–2868. doi: 10.1016/S2095-3119(19)62618-3
Yan L., Khan R. (2021). Biological control of bacterial wilt in tomato through the metabolites produced by the biocontrol fungus, Trichoderma harzianum. Egyptian J. Biol. Pest Control 31, 1–9. doi: 10.1186/s41938-020-00351-9
Yongchao L., Miroslav N., Richard B., Haijun G., Alin S. (2015). Silicon in agriculture. From theory to practice (Netherlands: Springer).
Yu Y., Zhang L., Li Y., Hou L., Yang H., Shi G. (2022). Silicon fertilizer and microbial agents changed the bacterial community in the consecutive replant soil of lilies. Agronomy 12. doi: 10.3390/agronomy12071530
Zarebanadkouki M., Al Hamwi W., Abdalla M., Rahnemaie R., Schaller J. (2024). The effect of amorphous silica on soil–plant–water relations in soils with contrasting textures. Sci. Rep. 14, 10277. doi: 10.1038/s41598-024-60947-1
Zarebanadkouki M., Hosseini B., Gerke H. H., Schaller J. (2022). Amorphous silica amendment to improve sandy soils’ hydraulic properties for sustained plant root access under drying conditions. Front. Environ. Sci. 10, 935012. doi: 10.3389/fenvs.2022.935012
Zhang W.-W., Chong W., Rui X., Wang L.-J. (2019). Effects of salinity on the soil microbial community and soil fertility. J. Integr. Agric. 18, 1360–1368. doi: 10.1016/S2095-3119(18)62077-5
Zhou X., Shen Y., Fu X., Wu F. (2018). Application of sodium silicate enhances cucumber resistance to fusarium wilt and alters soil microbial communities. Front. Plant Sci. 9. doi: 10.3389/fpls.2018.00624
Zhou J., Zhang C., Du B., Cui H., Fan X., Zhou D., et al. (2021). Soil and foliar applications of silicon and selenium effects on cadmium accumulation and plant growth by modulation of antioxidant system and Cd translocation: Comparison of soft vs. durum wheat varieties. J. Hazardous Materials 402, 123546. doi: 10.1016/j.jhazmat.2020.123546
Keywords: soil microbiome, silicon, environmental stresses, agroecosystem resilience, sustainable agriculture
Citation: Etesami H (2024) Enhancing soil microbiome resilience: the mitigating role of silicon against environmental stresses. Front. Agron. 6:1465165. doi: 10.3389/fagro.2024.1465165
Received: 15 July 2024; Accepted: 12 September 2024;
Published: 04 October 2024.
Edited by:
Antonio Rafael Sánchez-Rodríguez, University of Cordoba, SpainReviewed by:
Qaiser Javed, Jiangsu University, ChinaCopyright © 2024 Etesami. This is an open-access article distributed under the terms of the Creative Commons Attribution License (CC BY). The use, distribution or reproduction in other forums is permitted, provided the original author(s) and the copyright owner(s) are credited and that the original publication in this journal is cited, in accordance with accepted academic practice. No use, distribution or reproduction is permitted which does not comply with these terms.
*Correspondence: Hassan Etesami, aGFzc2FuZXRlc2FtaUB1dC5hYy5pcg==