- School of Water, Energy and Environment, Cranfield University, Bedford, United Kingdom
Biostimulants are gaining prominence in scientific research, with the potential to enhance plant productivity through benefits to crop yield/quality and tolerance to environmental stresses. Through possible improvements to nutrient use efficiency, they may also lessen the adverse environmental impacts of conventional inorganic fertilizer use in agriculture. The application of biostimulants is currently uncommon as a farming practice, with uncertain effectiveness in delivering these potential benefits. Current research focuses on biostimulant effects on plant physiological changes. There is little scientific evidence on the impact of biostimulants on soil properties (biological, physical, or chemical) or soil functions. This knowledge gap should be addressed considering the vital role of soil processes in the bioavailability of nutrients, as reflected in crop productivity. This review evaluates laboratory and field experimental work on the effectiveness of common, non-microbial biostimulants, with a focus on their modes of action within the soil matrix. Of 2,097 initial articles returned through the search strings, 10 were within the scope of this review. A common soil biostimulant mechanism emerges from this literature. This relates to the supply of nutrients provided by the biostimulants, which stimulate native soil microbiology in mineralizing organic material in the soil, thus producing more bioavailable nutrients for plant uptake. Additionally, some articles link biostimulant effects to soil physical and chemical changes, which in turn impact soil biology (and vice versa). However, there is inconsistent evidence to provide full support for these explanatory mechanisms. This review highlights the need for further research into the effect of biostimulants on the native soil microbiology and associated soil properties, to provide greater clarity on biostimulants’ modes of action and greater mechanistic insights into how they can be used to improve crop production.
1 Introduction
The global population is growing at an unprecedented rate, which is increasing the demand for safe, affordable, and available food supplies (Araújo et al., 2023). These pressures not only relate to the tonnage of staple foods such as wheat and maize, and fresh produce such as salad crops, but also on the nutritional value of these products. To meet food demands, agrochemicals, inorganic fertilizers, and pesticides have been manufactured and used to maximize production, but often with adverse environmental impacts on land, air, and water (Halpern et al., 2015; Tripathi et al., 2020). It is therefore important that future agricultural practices use products that cause less damage to natural capital while producing more food to meet the demand (Rouphael et al., 2020). Alternative products to inorganic agrochemicals, such as biostimulants, have gained commercial interest in recent years, with the biostimulant market size estimated to reach circa €2.66 billion in 2022 (Critchley et al., 2021). Biostimulants may provide a means of improving crop productivity while simultaneously addressing the economic, environmental, and sustainability concerns around conventional food production systems (del Buono, 2021).
Biostimulants were defined by (du Jardin, 2015, 2012) as substances or microorganisms that, when applied to plants, seeds, or growing substrates, have the capacity to enhance nutrition efficiency, stress response, and/or crop yield and quality traits, regardless of its nutrients content. The European Biostimulant Industry Council (EBIC) and European Commission (EC) further defined these benefits under a new regulation in 2022, “Regulation (EU) 2019/1009”. This included highlighting additional benefits of increased availability of nutrients confined in the soil or rhizosphere (The European Parliament and The Council of the European Union, 2019).
Biostimulants were categorized by du Jardin (2012) into groups such as humic substances and complex organic materials. However, it was quickly recognized that those categories were not mutually exclusive because they shared common active substances. Since 2012, there has been a continual effort to improve the categorization of biostimulants, with more specific categories. Currently, the most common groups of biostimulants recognized in the literature are the following:
● humic substances
● protein hydrolysates
● seaweed extracts
● plant extracts
● biopolymers (e.g., chitosan)
● inorganic compounds (e.g., aluminum, cobalt, sodium, selenium and silicon)
● plant-growth-promoting fungi and bacteria (PGPB) (Grammenou et al., 2023).
These biostimulant categories can be more broadly grouped into microbial or non-microbial substances (Ngoroyemoto et al., 2020). The latter includes humic substances, which are derived from the decomposition of plant, animal, and microbial residues, and are separated into humic and fulvic acids (Popa et al., 2022). Protein hydrolysates encompass free amino acids and small peptides (Hellequin et al., 2020). Seaweed extracts are derived from red, brown, or green macroalgae, such as Ascophyllum and primarily contain polysaccharides, phenolics, and osmolytes (Ali et al., 2021). Plant extracts include a wide range of naturally synthesized phytoextracts important in plant growth and development such as glycinebetaine, a hormone-like biochemical regulator (Akram et al., 2022). Biopolymers such as chitosan is a naturally occurring sugar formed in the outer skeleton of shellfish (Shahrajabian et al., 2021). Inorganic compounds include some essential elements necessary for plant growth and some non-essential elements that can promote additional growth factors (Grammenou et al., 2023). Microbial biostimulants are plant-growth-promoting fungi and bacteria including microbial genus such as Azotobacter spp., Rhizobium spp., Azospirillum spp., and Mycorrhizal fungi (Castiglione et al., 2021).
Research into the effects of these biostimulants has increased over the past decade, with outcomes such as increased crop tolerance to abiotic stress (such as salinity or temperature extremes) (Fang et al., 2020), increased nutrient content of leaves and/or fruit of the crop (Celiktopuz et al., 2021), and improved yield and nutrient use efficiency (Di Mola et al., 2020). More recently, there has been a greater focus on the different mechanisms behind these effects related to type of biostimulant. Widely reported biostimulant mechanisms relate to interactions between foliar-applied biostimulants and associated plant response (du Jardin et al., 2020). These include actions such as chelation and stress-induced responses by the plant. These plant responses may relate to abiotic stresses such as drought (Bulgari et al., 2019) and upregulation of genes coding for a wide range of nutrient transporters thus altering plant metabolism (Jindo et al., 2020a, 2020b; Yakhin et al., 2017; Moreno-Hernández et al., 2019).
However, the mechanisms of biostimulants, specifically non-microbial types, on soil properties and processes have been seldom reported. These mechanisms are highlighted in Figure 1, which presents an overview of biostimulant types, soil-based mechanisms, and beneficial provisions to the plant. This paper presents a semi-systematic review to evaluate previous research on the impacts of non-microbial biostimulants, when applied directly to soil, on native soil microbiology and associated soil properties and processes, e.g., nutrient cycling. The evidence presented in this review will be used to identify knowledge gaps and recommend future research that will advance the mechanistic understanding of soil-applied biostimulants. In turn, this will inform how biostimulants can improve crop productivity.
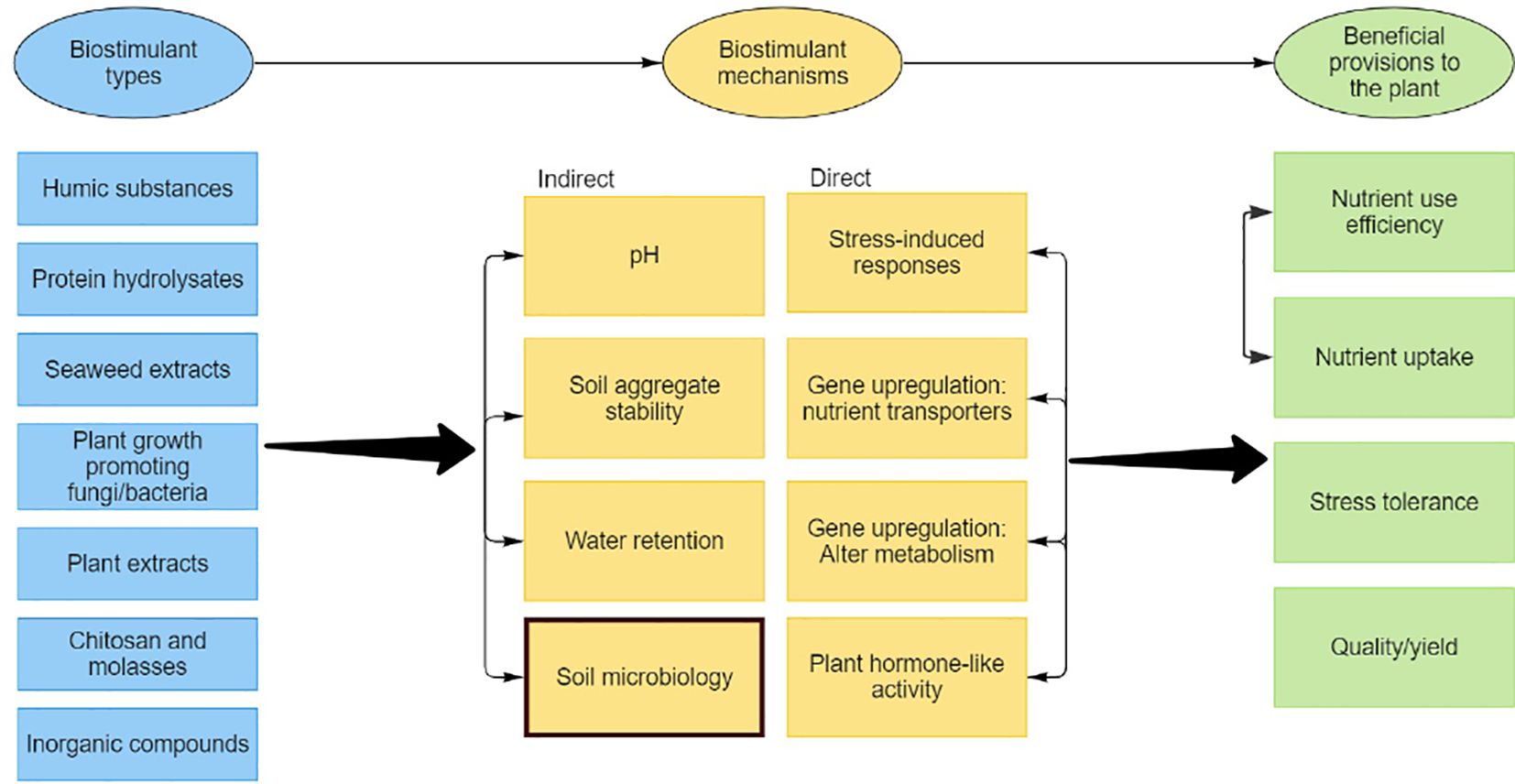
Figure 1 Biostimulant types, proposed mechanisms and beneficial provisions to the plant. Arrows between the variable boxes show multiple interconnections.
2 Method
2.1 Semi-systematic review
A semi-systematic review method was applied using principles from the Preferred Reporting Items for Systematic Review and Meta-Analysis (PRISMA) approach (Moher et al., 2009) and theory from Snyder (2019) to investigate the impacts of soil-applied, non-microbial biostimulants on native soil microbiology and associated soil properties. The semi-systematic review approach was applied to provide an overview of the research area and current state of knowledge, as opposed to a systematic review, which is used to strictly synthesize and compare empirical evidence between studies (Snyder, 2019). Three multidisciplinary databases were used: Scopus, Science Direct, and Google Scholar. Keywords such as “biostimulant” and “soil” were included in all searches. More specific search strings included “non-microbial biostimulants” AND “soil” AND (“biology” OR “microbial activity” OR “microorganisms”) to further refine the literature down to the specific topic.
2.2 Inclusion, exclusion criteria, and screening process
Articles applying microbial biostimulants were excluded so that the review could focus on (non-microbial) biostimulant impact on native soil microbiology, rather than interactions between the microbiology of the biostimulant and that of the native soil. The literature search results included only experimental articles that presented peer reviewed, primary data. Therefore, the search excluded review papers, book chapters, and books for example. Results were not limited by their country of origin, experimental design (i.e., field, glasshouse or incubation experiment), or timeframe of publication. Articles referring to non-soil applications, or primarily focusing on plant-related mechanisms or applications of a combination of a microbial and non-microbial biostimulant, were excluded because these scenarios were also out of scope. Articles that appeared across more than one database (i.e., duplicates) were removed. Articles were then screened in three stages via their title, abstract, and finally full-body text for relevance.
3 Results
3.1 Literature search results
The search strings resulted in an initial 2,097 related articles. Of these, 925 were excluded based on the type of article. The remaining 1,172 articles were screened at the title and abstract level, of which 872 articles were excluded because they were primarily concerned with the production of biostimulants, non-soil biostimulant applications (such as foliar sprays or seed treatments) or biostimulants used in bioremediation and waste studies. The full texts of the remaining 300 articles were screened. Of these, 289 were excluded because the paper either frequently referred to products named as a biostimulant in the title or abstract but were later deemed a fertilizer (i.e., applied nutrients that can be directly taken up by the plant) (The European Parliament and The Council of the European Union, 2019), concerned combinations of microbial and non-microbial biostimulants, or focused predominantly on plant-related biostimulant mechanisms (which was not explicitly stated in the title or abstract). One article was not open access and not available for full-text screening and therefore was excluded. Following these exclusions, 10 articles remained within scope. An overview of this process and corresponding search results are presented in Figure 2.
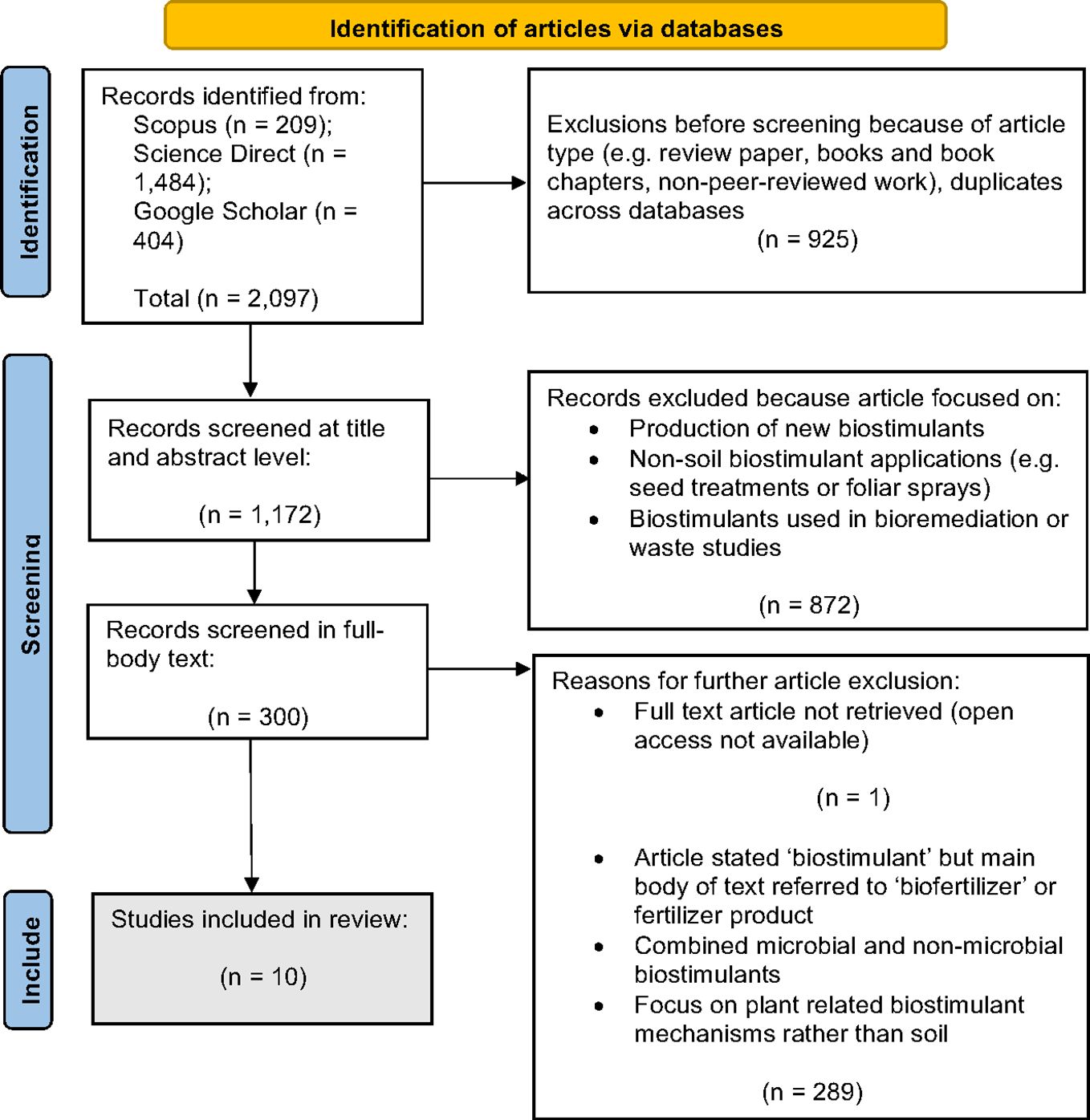
Figure 2 Flow chart using the Preferred Reporting Items for Systematic Review and Meta-Analysis (PRISMA) approach for selection of articles in the review. n denotes the number of articles found in each stage of the review method.
The 10 articles included in the review range from 2002 to 2023. The articles vary in experimental design used. For example, there were five laboratory microcosm experiments, four of which were carried out on soil only (Chen et al., 2002; Hellequin et al., 2020, 2018; Macias-Benitez et al., 2020). The other microcosm experiment used planted wheat (Chen et al., 2003). There were four field experiments (Alam et al., 2014; Yousfi et al., 2021; Wadduwage et al., 2023), one of which ran alongside a corresponding glasshouse trial (Alam et al., 2013). One experiment was solely based in a glasshouse (Renaut et al., 2019).
The articles reviewed investigated various non-microbial biostimulants. Five of the articles focused on a single ingredient, while the other five investigated a biostimulant containing multiple ingredients. For example, Renaut et al. (2019) applied a seaweed extract, Hellequin et al. (2020) applied a combination of seaweed extract with protein hydrolysates, and Wadduwage et al. (2023) investigated a biostimulant containing mineral trace elements, phytoproteins, vitamins, seaweed extracts, and plant hormones.
Biostimulant application dosage and frequency varied strongly between experiments. For example, within the field experiments, Wadduwage et al. (2023) applied 2.5 L ha−1 just once at the start of the experiment to different types of field sites (grassland and arable), whereas Yousfi et al. (2021) applied 10 L ha−1 every 20 days to ryegrass. There was no general consensus in these articles (or in the wider literature) as to why these application regimes were selected.
The following section critiques each of these 10 articles. The section is structured by the main biostimulant type applied in each study. However, there is overlap between sections, given the number of articles investigating the application of several biostimulants. Moreover, information detailing each experimental design including biostimulant application dosage and frequency are summarized in Table 1.
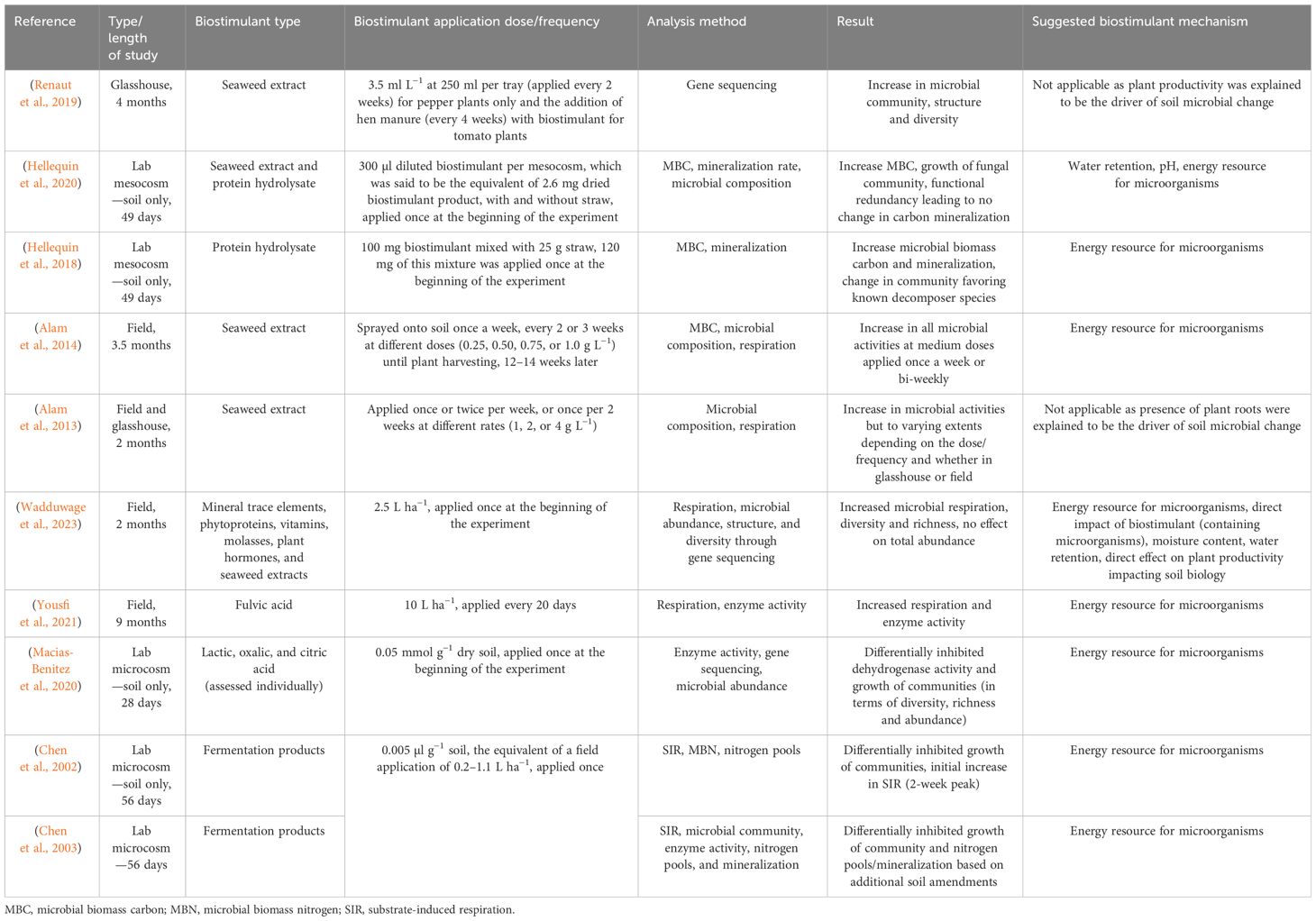
Table 1 Literature review articles investigating the link between biostimulants and native soil microbiology as a potential mechanism for improving soil properties and processes, and associated plant growth.
3.2 Discussion of articles identified in the literature review
3.2.1 Seaweed extracts and protein hydrolysates
Renaut et al. (2019) investigated the effect of a commercial seaweed extract (Acadian Seaplants Ltd, Canada) on tomato (Solanum lycopersicum L.) and pepper (Capsicum annuum L.) plants. The authors identified small but significant changes in the soil microbial community structure in the rhizosphere across both plant types in the biostimulant amended treatments (using gene sequencing techniques). This correlated with an increase in all crop growth parameters in both plant species in those treatments (root and shoot growth, fruit biomass, and number of fruits). There were greater increases in crop parameters of the pepper plants, but this was explained by the combined treatment of the biostimulant and hen manure. More specifically, there was a greater diversity of bacterial genera (for example, Bacillaceae, Rhizobiaceae, Sphingomonadaceae, and Pseudomonadaceae) present in the biostimulant-amended treatments than the control (across both plant species). A large proportion of the total diversity of fungi were of the Nectriaceae family in the biostimulant-amended soils. Nectriaceae and other fungal families identified in the study such as Mortierellaceae are saprotrophs, critical for the decomposition of organic matter and nutrient cycling within the soil. This is because they are the primary agents of plant litter decomposition and their hyphal networks readily distribute nutrients in the soil (Crowther et al., 2012). These changes in the microbial community in both the biostimulant (and hen manure)-amended treatments in comparison to the control suggest that the biostimulant may be at least partly responsible for shaping the microbial community. However, Renaut et al. (2019) were unable to explain the driving mechanism for this shift in microbial structure and could not disentangle the effects of the biostimulant from the hen manure in the tomato plant trials.
Hellequin et al. (2020) researched the potential role of a biostimulant containing both seaweed extracts and protein hydrolysates on soil microbiology. The focus was on carbon (C) mineralization, which is a general function of multiple decomposers in the soil and is commonly used to indicate overall microbial productivity (Banerjee et al., 2016). The authors measured an immediate reduction in the abundance of active fungi and bacteria where the biostimulant had been applied (determined by quantitative polymerase chain reaction to determine phylogenetic microbial groups). This was explained by physical disturbances: either the polysaccharides within the seaweed extract immobilizing soil microorganisms, or from physically mixing the soil with the biostimulant. Although the abundance of active microorganisms reduced, the diversity indexes (measured by gene sequencing techniques) were either maintained or increased in the biostimulant treatments compared with the untreated soil control (Hellequin et al., 2020). After 7 days, the total abundance of microbial phylogenetic groups had increased from Day 0, and this increase was greater with the biostimulant compared to the treatments without. On the final sampling date (Day 49), the soil microbial community in the biostimulant treatments was significantly richer and more diverse in fungi (when compared with bacteria) than in the control.
The sustained abundance of microbial species in the biostimulant treatments on Day 49 was explained by multiple factors. First, carrageenans (naturally occurring sulfated polysaccharides) contained in seaweed extracts are comprised of hydrophilic colloids that attract water molecules, thereby promoting better soil water-holding capacity (WHC) and aggregate stability, two properties that are closely linked to microbial activity (Hellequin et al., 2020). For example, soil moisture affects the mobility and the structural and functional diversity of microorganisms (Tecon and Or, 2017). Moreover, water availability is essential for the activity of soil enzymes, which contribute to the process of nutrient mineralization, by breaking down organic matter that is too large or insoluble for direct absorption by microorganisms (Dick and Kandeler, 2004; Bailey et al., 2013).
Second, soil aggregate stability is fundamental in shaping microbial communities by supporting their ability to disperse and reach additional energy resources (Liu and Han, 2020). This link between physical and biological soil properties was used to partially explain the sustained higher abundance of microbial species at the end of the experiment (Hellequin et al., 2020). However, although aggregate stability was used to explain the relationships between biostimulant application and soil microorganisms, it was not a variable that was measured. It is difficult to determine whether the observed changes in microbiological community affected aggregate stability and/or vice versa.
Third, the biostimulant had a higher pH (6.4) than the soil (5.5), measured at the beginning of the experiment, which was explained to induce a neutralizing effect on biostimulant-treated soils (increasing the pH to neutral values of between 6.6 and 7.5), as observed by Hellequin et al. (2020). This change in pH would induce changes in the soil bacterial and fungal communities (Rousk et al., 2009). However, pH was not measured at the end of the experiment, and therefore, this is a speculative explanation by the authors. Earlier, Hellequin et al. (2018) showed that the addition of a protein hydrolysate-based biostimulant increased soil pH from moderately acidic to near-neutral values. This was positively correlated with the abundance of Bacteroidetes, a phylum of bacteria commonly abundant in neutral to low alkaline pH soils. These are key regulators in C cycling due to their ability to degrade complex plant-derived carbohydrate molecules and thus play a vital role in soil functioning (Bauer et al., 2006). While a correlation does not explain the mechanism, it does provide some evidence of protein hydrolysate effects on soil pH, which might influence the soil microbial community. Moreover, it is common that soil microorganisms have optimum pH levels in which they thrive, and so biostimulants may directly support their preferred pH environment (Lauber et al., 2009). This may have positive implications on plant growth, assuming that the pH promotes “beneficial” soil microorganisms in terms of increasing nutrient mineralization and providing bioavailable nutrients in the soil for plants to utilize (Rousk et al., 2009).
Lastly, Hellequin et al. (2020) suggested that amino acids (which make up a significant proportion of the biostimulants in their study) were used by microorganisms as an alternative energy source in which to mineralize organic materials in the soil (in this case, straw). This energy supply was needed to sustain the microbial population over the 49 experimental days. Amino acids are more easily available for soil microorganisms in comparison to humic acids for example because they have a lower number of carboxyl groups (Adeleke et al., 2017; Macias-Benitez et al., 2020). Molecules with fewer carboxyl groups have a smaller molecular weight and are therefore easier for soil microorganisms to digest (García-Martínez et al., 2010). It is well known that molecule size is an important parameter for soil microbial stimulation (Yousfi et al., 2021). Moreover, biostimulants made from seaweed extracts have a high polysaccharide content, which may also provide an energy source for microorganisms (Renaut et al., 2019). As polysaccharides break down over time, glucose and galactose are made available from the laminarins and carrageenans found in seaweeds. This breakdown occurs over a longer period of time than for amino acids that are already more accessible to soil microorganisms. The former may therefore provide available resources to the longer-lasting or slower-growing populations within the microbial community, such as fungi (Yu et al., 2022). This theory is supported by the results of Hellequin et al. (2020) where the species richness and long-lasting microbial population were dominated by fungi.
Despite these changes in microbial community structure over the course of the experiment, Hellequin et al. (2020) did not observe any associated increase in the mineralization rate of organic soil C in the biostimulant and straw amended treatment (as shown by cumulative C–CO2 emissions). This might be explained by the fact that the changing microbial community (because of the biostimulant treatment) may still function in the same way as the original community. This is because of the variety of microbial clades that exhibit similar functions (Louca et al., 2018; Hellequin et al., 2020). This suggestion is also supported by the fact that the biostimulant treatment had no effect on total microbial biomass C (i.e., the size of the community). Thus, although the biostimulant did not increase the total community size, it affected different sections of the community differently. Where a changing microbial community is able to carry out similar functions to the original community is known as functional redundancy (Sadeghinia et al., 2023). The idea of functional redundancy suggested by Hellequin et al. (2020) is also supported by Rousk et al. (2009). The latter discuss how changes in pH may change microbial community structure, but the overall function of the microorganisms can remain unchanged. Functional redundancy may be more beneficial in creating a stable and resilient community that maintains its function throughout adverse environmental changes, rather than improve the overall outputs (e.g., C mineralization), which benefit plant growth (Sadeghinia et al., 2023).
To summarize the study by Hellequin et al. (2020), the addition of a biostimulant made of seaweed extract and protein hydrolysates resulted in an increase in soil microbial abundance after 49 days compared to an unamended control treatment. However, by incorporating a straw and biostimulant soil treatment into the experimental design, the authors were able to conclude that the change in microbial community did not elicit any associated increase in C mineralization, explained by functional redundancy. The observed changes in the microbial community were explained by physical soil properties such as moisture retention and aggregate stability (although these were not directly measured) and chemical/biological characteristics including pH and available energy resources (as a result of the biostimulants), which stimulated the microbial community.
However, an earlier study by Hellequin et al. (2018) found opposite effects when using a biostimulant made from protein hydrolysates (predominantly amino acids), with a similar experimental design to that of Hellequin et al. (2020). Hellequin et al. (2018) found an enhancement of organic C mineralization of the straw, which coincided with a change in the microbial community. The conditions favored microorganisms that are affiliated with known microbial decomposers such as Pseudomonas sp., and Mortierella sp. Moreover, the total microbial biomass increased, correlating to the increase in C mineralization. This contradicts Hellequin et al. (2020), who found no impact of the biostimulant on microbial biomass.
The difference in microbial stimulation identified in these two studies may be due to the higher number of amino acids within the protein hydrolysate biostimulant in the 2018 study than when combined with seaweed extract in the 2020 study. Amino acids have a low molecular weight and are therefore more easily assimilated by soil microorganisms (García-Martínez et al., 2010). These amino acids are used as an alternative energy resource to mineralize soil organic materials, such as C. This explanation is common among the literature and supports Hellequin et al. (2020), despite conflicting results in terms of the amount of C mineralization. The wider implications for plant growth (although not measured in these studies) could suggest a positive response due to a stimulated microbial community that can produce bioavailable nutrients for plants to utilize. However, there is currently a lack of supporting evidence for this mechanism.
Alam et al. (2014) investigated the application of seaweed extract on carrot. This paper focused on soil microbial measurements including colony counts, respiration, and metabolic activity. In comparison to the control treatment (with no biostimulant), seaweed extract applied at 0.50 or 0.75 g L−1 once a week or bi-weekly created the highest mean response in both microbial parameters and root yield. Bacterial genus of Pseudomonas and Bacillus were very responsive to this biostimulant application. These genera are commonly associated with carrot roots, so the authors explained that the close association between plant and soil microorganisms make it difficult to determine whether the seaweed extract stimulated root growth, which encourages the bacteria, or vice versa. This mechanistic challenge is discussed by Renaut et al. (2019), who also identified the difficulty in differentiating cause and effect between root and soil. Moreover, the Biolog method relies on extracting microbial populations before measuring the response to different C substrates (Preston-Mafham et al., 2002). Thus, the method excludes measurement and characterization of the complete microbial community. As a result, it is unclear how the biostimulant impacted other areas of the community outside of the pre-selected community (Stefanowicz, 2006).
Alam et al. (2013) also followed a similar experimental design and microbial measurements to Alam et al. (2014), but investigated the use of seaweed extract both with and without the presence of strawberry plants. Microbial activity (colony counts, metabolic activity, and respiration) tended to increase following the highest biostimulant application rate in the glasshouse trials. In the field trial however, the authors found successive increases in microbial activity at 1 and 2 g L−1, but a reduced effect at the highest application. The authors suggested that the higher biostimulant application rate had a suppressive effect on the soil microbial activity, but with no further explanation of the reasons for this. Importantly, by comparing the biostimulant effect with and without the plant, Alam et al. (2013) demonstrated that the biostimulant did not increase soil microbial respiration (measured through CO2 evolution) when applied to the soil without plants. This may help explain the results seen in the study by Alam et al. (2014) in which the biostimulant stimulated root growth first, which in turn stimulated microbial activity. Even so, Alam et al. (2014) conclude that the interdependence of biostimulant actions on soil and plant roots means it remains speculative as to whether the seaweed extract, microbial community, or both had an impact on subsequent carrot root growth. These studies highlight the need for a better mechanistic understanding to connect seaweed extract-induced changes in the soil microbial community with increased plant growth.
In another field trial, Wadduwage et al. (2023) investigated the effects of a biostimulant containing seaweed extracts, mineral trace elements, phytoproteins, vitamins, molasses, and plant hormones. The authors measured biostimulant effects on microbial activity at two soil depths (0–10 and 10–20 cm). Microbial activity was characterized by basal and multiple substrate-induced respiration (SIR) using MicroResp (colorimetric CO2 detection method, specific calculation of basal respiration versus multiple SIR was not stated), bacterial and fungal abundances, community structure, and diversity through gene sequencing techniques. They also measured soil chemical [total C and nitrogen (N)] and physical (soil moisture content and WHC) properties. Measurements were taken across five field sites; three were on arable land growing wheat and two were on grasslands. Both land use types had control plots with no biostimulant application. The fields’ regular fertilizer regimes were continued (e.g., wheat cultivation received one application of mono-ammonium phosphate at sowing and two urea applications through the growing period; the grassland sites received no fertilizer).
Wadduwage et al. (2023) reported an increase in basal microbial respiration in the biostimulant-treated soils of up to 40.1% and 36.4% in the surface and deeper soil layers, respectively, across all of the fields when compared with the controls. However, this was largely attributed to the larger statistical differences in basal respiration between the control and biostimulant-treated soils at the grassland sites. Moreover, statistical analysis of individual fields showed that soil microbial respiration responses to the biostimulant were strongly field specific. For example, basal respiration did not significantly increase in two of the arable fields following biostimulant application. The authors reported that these two sites had been intensively used, i.e., “under wheat cultivation with intensive management”. They suggest that this management may have contributed to lower background soil microbial biomass, which may take longer to respond to biostimulant effects. However, this does not explain why the microbial respiration of the third arable field did not follow the same pattern. In relation to field specificity, there were two fields that had similar conditions and land use (i.e., grassland). Both of these fields resulted in significant basal respiration responses in biostimulant-treated soils at surface soil layers, but had different multiple SIR responses. Biostimulant-treated soils at one of the grassland fields had significantly higher multiple SIR in surface soil layers, whereas the other field had significantly higher multiple SIR at the greater soil depth, when compared to their respective control (Wadduwage et al., 2023). The individual respiration responses to the substrates were not given, so presumably the “higher multiple SIR” was in response to all of the substrates used in the MicroResp method. Moreover, the authors did not explain the specific site differences in response to the biostimulant, but they did suggest that an increase in some microbial activity supported their hypothesis that biostimulants can stimulate soil biological activity (Wadduwage et al., 2023).
Total bacteria and fungi abundance was not significantly affected by biostimulants at either depth at any of the field sites. Wadduwage et al. (2023) explained that this could be because of the relatively short length of the experiment (2 months) and the repeated biostimulant applications required to evoke changes to microbial abundances. However, a number of studies in this review found significant changes to microbial abundances with only one biostimulant application over similar short experimental time frames. For example, Hellequin et al. (2018) measured significant increases in microbial abundance following biostimulant application within 49 days. However, their biostimulant was predominantly protein hydrolysates in comparison to the complex biostimulant applied by Wadduwage et al. (2023). These differences in biostimulant types and effects may offer some insight into specific biostimulant ingredients, which may affect specific areas of soil microbial structure and activity.
Additionally, species richness and diversity of both bacterial and fungal communities were increased by the biostimulant application across both types of field sites (Wadduwage et al., 2023). For example, common fungal species including Mortierlla, Techisopra, Auricularia, Lycoperdon, Helotiales, and Chaetothyriales increased. Bacterial species that increased as a result of biostimulant application were primarily Proteobacteria, Actinobacteria, and Acidobacteria (Wadduwage et al., 2023). This result suggests that the biostimulant application was sufficient to impact the microbial population beyond just total community abundance, primarily in their composition and diversity. This provides support for the hypothesis that different ingredients of a biostimulant may impact different characteristics of the soil microbial community.
Despite the inconsistent and therefore unclear biostimulant effects on the soil microbial activity in the study by Wadduwage et al. (2023), they offered some general explanations as to why the biostimulant positively impacted some of the microbial factors (i.e., increased respiration, richness, and diversity of community composition) at some of the sites. For example, the composition of the biostimulant provided multiple benefits. Some components, such as the molasses, provided easily degradable C sources to the microbial community. Fulvic acids were said to provide C and energy to the microbial population, explanations commonly used elsewhere in the literature (Hellequin et al., 2020; Macias-Benitez et al., 2020; Yousfi et al., 2021). Furthermore, some of the dominant microbial taxa were suggested to have increased because the seaweed extract component of the biostimulant was itself rich in some of these microbial taxa. However, despite associations between bacterial phyla such as Proteobacteria and Firmicutes, and seaweed surfaces (Selvarajan et al., 2019), the suggestion that seaweed extract biostimulants are applied as a microbial inoculant has not been reported in the literature elsewhere.
In terms of the physiochemical soil properties, Wadduwage et al. (2023) found that biostimulant-treated soils had increased soil moisture content in surface soil layers, as much as 88% in one of the grassland fields when compared with the control. WHC was also significantly increased by the biostimulant in the deeper soil layer in three of the sites (two arable, one grassland). The seaweed extract part of the biostimulant was used to explain the changes in these soil characteristics. The seaweeds can increase soil moisture directly by absorbing water, indirectly by altering soil micro- and macropores, and reducing evaporation losses (Wadduwage et al., 2023). Hellequin et al. (2020) also suggested that the hydrophilic colloids within seaweed extracts attract water molecules and can therefore directly impact soil WHC.
In the same study, application of the biostimulant significantly increased total C and N in both soil depths across most sites except for two; these were the intensively used arable fields that corresponded to the non-significant difference in microbial respiration found in the biostimulant-treated soils at these sites (Wadduwage et al., 2023). The authors did not provide a specific explanation for why the third wheat cultivation field site resulted in significant increases in total C and N where it might be expected to follow the same pattern as the other two wheat fields. Wadduwage et al. (2023) suggested that the general increase in total C and N, however, was due to the biostimulant enhancing plant growth across the field sites, rhizodeposition, and litter inputs, so likely leading to increased soil organic C. Specific mechanisms for such a response were not provided. The suggested mechanism for increased soil organic C was also thought to promote microbial activity (by providing an energy resource for microorganisms), which was evidenced earlier by the correlation between microbial respiration, and total soil C and N across the sampled fields. Additionally, the authors suggested that the biostimulants’ potential to enhance root and plant growth may lead to increased aeration and moisture content of the soil, which further supports microbial growth and activity. However, these metrics were not measured in the experiment to support these hypotheses.
In summary of Wadduwage et al. (2023), a complex biostimulant product differently influenced microbial activity (in terms of abundance, respiration, diversity, and richness) depending on field conditions (land use and management) and soil depth. Increases in soil microbial activity (as a result of the biostimulant) generally corresponded to increases in total soil C and N. Physical soil properties (soil moisture content and WHC) were also increased by the biostimulant. The mechanistic understanding of these biostimulant/soil interactions was focused primarily on the role of the seaweed extract within the biostimulant product, rather than the other ingredients. The challenge of partitioning different biostimulant properties to different modes of action results in an ongoing lack of clarity of biostimulant mechanisms on soil properties and processes. Moreover, this study highlights the interdependencies between microbial activity and physiochemical soil properties, which remain unclear.
3.2.2 Organic acids
Yousfi et al. (2021) investigated the effect of a fulvic-acid-based biostimulant on ryegrass. Fulvic acids are part of the “humic substances” biostimulant category. They are low molecular weight molecules that are easily assimilated and utilized by soil microorganisms (Braziene et al., 2021). Yousfi et al. (2021) measured soil microbial activity (respiration methods and dehydrogenase activity), soil chemical composition (e.g., calcium and magnesium) and root growth of ryegrass. The study demonstrated that the biostimulant significantly increased microbial activity [i.e., respiration and dehydrogenase activity, the latter commonly used as an indicator of soil microbial activity because of its role in organic matter decomposition (Navnage et al., 2018)]. It also increased root length of the ryegrass. These results reinforce the theory that low molecular weight resources (e.g., fulvic acids, small peptides, amino acids) can be easily assimilated by soil microorganisms, as suggested frequently throughout the reviewed literature García-Martínez et al. (2010); Hellequin et al. (2020) and Wang et al. (2018).
Macias-Benitez et al. (2020) examined the potential of biostimulants composed of three different organic acids (lactic, oxalic, and citric acid) naturally present in the soil rhizosphere. Soil enzyme activity (dehydrogenase and phosphomonoesterase), sequencing of bacterial DNA, and bacterial community composition (taxonomic groups, diversity and abundance) were measured. Results showed that after 28 days, all three organic acids were mineralized completely by the soil microbial community (evidenced by the organic acid content of the soil). This was explained by the biostimulants providing C and energy to the microbial community. The dehydrogenase activity increased significantly after lactic and citric acid application, with the latter promoting the highest dehydrogenase activity. Citric acid was said to be the richest C source, thus producing the highest microbial metabolism (as indicated by dehydrogenase activity). Oxalic acid, however, while completely metabolized, did not increase dehydrogenase activity significantly. The authors claim that this was because the acid was utilized by only a small part of the microbial community that had the capability to metabolize it (Macias-Benitez et al., 2020).
All three organic acids decreased the diversity and richness of the microbial community in comparison to the control by Day 28. Macias-Benitez et al. (2020) suggested this was because the C sources provided by the organic acids could only be utilized by specialist microorganisms that would then dominate the population. This explanation concurs with Yousfi et al. (2021) who discussed the utilization ability of different soil microorganisms depending on the size of the biostimulant molecules, in terms of their molecular weight. Moreover, the results by Macias-Benitez et al. (2020) specifically show examples of microbial taxa being outcompeted by those favored by the application of the biostimulants. For example, taxonomic compositions of Proteobacteria, Acitnobacteria, Acidobacteria, and Firmicutes were the predominant phyla in all samples (including the control) at the beginning of the experiment. However, lactic acid stimulated increases in the abundance of Actinobacteria and Firmicutes phyla, while citric acid stimulated the Firmicutes phylum. Oxalic acid increased the Proteobacteria phylum. On Day 28, the effect of lactic and citric acid on the microbial diversity had returned to similar levels measured at the beginning of the experiment. An exception to this was PGPB such as the Pseudomonaceae family, which were maintained for the duration of the experiment. Oxalic acid also promoted long-lasting effects but on specific microorganisms associated with oxalic acid mineralization, such as oxalotrophic bacteria (Palmieri et al., 2019). In summary, Macias-Benitez et al. (2020) provide further evidence of the mechanisms linking biostimulant application to resource utilization by native soil microorganisms. Their work supports others such as Hellequin et al. (2020) in suggesting that biostimulants may impact different areas of the community without increasing the size of the community overall.
3.2.3 Fermentation products and trace minerals
Chen et al. (2002) investigated the effects of a biostimulant containing solutions of fermentation products and trace minerals on soil microbial activity, with a focus on N dynamics. They characterized the effects of these biostimulants on soil microbial SIR, dehydrogenase activity, microbial biomass nitrogen (MBN), and N pools in treatments containing either bare soil (control) or soils amended with alfalfa (readily available N) or straw (low available N). By comparing treatments of high and low available N materials with a biostimulant, Chen et al. (2002) were able to investigate how the biostimulant affected the rates of mineralization and immobilization of soil organic materials by soil microorganisms. Soil microbial activity governs the bioavailability of N for plant uptake in the soil, so impacting plant growth (Van Der Heijden et al., 2008).
The biostimulant significantly increased SIR in the alfalfa- and straw-amended soils compared to the bare soil over the first 2 weeks of the incubation. This suggests that the biostimulant enhanced microbial utilization of the additional amendments. This was most prominent in the alfalfa-amended treatment because of its readily available N content (Chen et al., 2002). This result contrasts with others such as Hellequin et al. (2020) who describe greater biostimulant effects on soil microbial activity under low resource availability, suggesting that the biostimulant acts as an alternative energy resource. Following the peak SIR at 2 weeks, SIR was consistently low across all biostimulant treated soils. While the authors did not explain this, it is hypothesized that the biostimulant stimulated early utilization of the amendments, which led to the peak SIR in those treatments in the first 2 weeks. This result also contrasts with Hellequin et al. (2020) who measured a delayed response from the microbial community to the biostimulant application.
Alongside SIR, Chen et al. (2002) measured dehydrogenase activity, which was greatest in the first 3 days of the incubation and decreased steadily thereafter across all treatments. Dehydrogenase activity was most prevalent in the control and alfalfa-amended treatments and less so in the straw-amended treatment. It was unclear as to why dehydrogenase activity and SIR were not well-correlated. This could be because dehydrogenase activity depends on multiple dehydrogenase enzymes, which are fundamental in microbial respiratory metabolism, hence the expected correlation with SIR (Wolinska and Stepniewsk, 2012). However, the authors suggested that dehydrogenase activity may be primarily a measure of the active bacterial biomass, as opposed to the active fungal biomass. Thus, while SIR increased in the straw treatment over the first 2 weeks for example, the lower dehydrogenase activity in that treatment suggested a smaller stimulation of the bacterial population and prominence of the fungal population, thus maintaining the respiration rate (Chen et al., 2002). However, the hypothesis that dehydrogenase activity is primarily a measure of bacterial biomass is not supported in other studies (Casida, 1977; Wolinska and Stepniewsk, 2012). Moreover, it was expected that there would be less dehydrogenase activity in the straw treatment because of the straw’s high C:N ratio (i.e., less available N), which favors fungal communities (Chen et al., 2002). The evidence presented here suggests that biostimulants stimulate microbial community respiration, which will contribute towards the mobilization of key nutrients in the soil that are crucial for plant growth. The evidence suggests that biostimulants may also impact areas of the community differently (i.e., fungi vs. bacteria), depending on the quality of the amendment (in terms of resource availability) (Chen et al., 2002).
Chen et al. (2002) measured a significant increase in MBN when the biostimulant was added to both the control and alfalfa treatments, while in the straw-amended treatment, the MBN was consistently lower (than the control and alfalfa-amended treatments). In the alfalfa treatment (where there were already readily available N sources), the biostimulant provided an additional resource for the microbial community, which could have been converted into greater MBN (Chen et al., 2002). This does not explain the increase in the control treatments’ MBN however, considering that this treatment would be expected to have fewer available N sources than the treatments with additional amendments. Furthermore, in the straw treatment, the authors proposed that the biostimulant inhibited the growth of at least some of the microbial biomass, leading to consistently lower MBN than the other treatments. However, a mechanism for this hypothesis was not provided. A possible explanation could be that the biostimulant did not inhibit the MBN growth as such, but rather the initial amendment (straw) did not allow for enough N to be incorporated into the MBN and the biostimulant needed to be utilized immediately as a N source. This demonstrates the lack of transparency in soil processes when biostimulants are used, especially when applied alongside soil amendments [in the case of Chen et al. (2002), straw].
With regard to soil nitrate (plant available form of N), Chen et al. (2002) found no significant differences in the straw treatments (with and without biostimulant) on Day 56. At the same time point in the alfalfa-amended soils, there were substantially lower concentrations of nitrate in the biostimulant-amended soils than without. The authors proposed this result was because of decreased N mineralization or increased microbial uptake of N (immobilization), as the N source depletes over time. However, if this was true, this would have been evidenced by the straw treatment with low available N, but this was not the case. Chen et al. (2002) proposed another possible explanation for the results of the alfalfa-amended soils in which the biostimulant may have stimulated soil microorganisms involved in denitrification, leading to reduced nitrate levels when compared to the control without biostimulant. The process of denitrification removes plant available forms of N, which could have an adverse effect on crop yield: the opposite effect of intended biostimulant applications (Skiba, 2008). However, this mechanism of biostimulant-induced soil microbial denitrification is unclear and unreported in the literature elsewhere.
To briefly synthesize the work by Chen et al. (2002), the addition of a biostimulant was suggested to both inhibit and promote the growth of different microbial communities within the soil, which can affect the rates of mineralization and immobilization of soil organic materials. Overall, the addition of a biostimulant increased microbial SIR in the short term (2 weeks) when amended with either alfalfa or straw, which would often be a good indicator for an associated increase in soil function in terms of nutrient bioavailability for plant growth (Comerford, 2005). However, when measuring a particular available nutrient for plant uptake (nitrate), there were some conflicting interpretations. For example, the nitrate concentrations were lower in the alfalfa-amended soil in comparison with the control soil by the final sampling date. This was hypothesized to be the result of the biostimulant-stimulating microorganisms involved in denitrifying soil processes (Chen et al., 2002). The multiple and contradictory interpretations of these biostimulant effects contribute to a poor mechanistic understanding and explanation in terms of soil nutrient bioavailability. In turn, understanding the mechanisms by which biostimulants affect plant productivity remains unclear.
A later study by Chen et al. (2003) followed the same experimental design as that by Chen et al. (2002), but they investigated the biostimulant effects on wheat growth (shoot length, root and shoot biomass, and concentration of N in shoot tissues), in addition to the soil parameters that were measured previously. The authors also used an isotopic tracer (15N) and an ion-exchange resin in the base of the microcosm to further understand the rates of N mineralization. Briefly, soil microbial activity (SIR and dehydrogenase activity) was significantly lower in the biostimulant-treated soils than the control soils, regardless of amendment type. This effect was most pronounced on Day 56 (Chen et al., 2003).
N pools were differentially affected by biostimulant application. For example, biostimulant effects on ammonium-N concentrations were not significant, regardless of amendment type. Biostimulant effects on nitrate-N were significant only in the biostimulant- and alfalfa-amended treatment. In this soil, there were delayed effects in comparison to the untreated control in which nitrate-N concentrations decreased rapidly within the first 2 weeks and then remained low for the remainder of the experiment. The authors suggest that the general reduction in nitrate-N was presumably a result of the germinating wheat seeds having an increasing demand and uptake of nitrate-N through the early growth stages (Chen et al., 2003).
The biostimulant led to significant increases in mineral N concentrations in the alfalfa-amended soil, but not in the straw-amended or control soils. In terms of the mineral N pool dynamics (measured by the isotopic tracer N), the biostimulant had the most pronounced effect on Day 15 in the alfalfa-amended soil where the isotopic tracer N portion increased (i.e., applied N increase and not native soil N). The authors explain that the biostimulant in the alfalfa-amended soil suppressed mineralization at this time point. This was because of the simultaneous processes of ammonium and nitrate consumption by microbial immobilization, plant uptake, denitrification and possible leaching, and the production of ammonium through the mineralization of soil organic materials by soil microorganisms. The authors admit that it was not possible to stipulate the relative contributions of these different factors with a single measurement of the isotopic N tracer, however.
Net N mineralization rates were significantly higher within the first 3 days of the experiment with biostimulant application, regardless of the amendment type. By Day 56, net N accumulations (ammonium and nitrate-N) on the ion-exchange resin had increased significantly with biostimulant application, regardless of amendment type when compared to the controls. This indicated that the biostimulant application promoted longer-term N availability (Chen et al., 2003).
Plant biomass measured on Day 56 significantly decreased in the biostimulant-treated soil amended with alfalfa. On the other hand, the biostimulant-treated soil amended with straw promoted the opposite effect. Chen et al. (2003) suggested that the wheat plant responded differently to the biostimulant, depending on the soil amendment. In this case, plant growth was enhanced by the biostimulant when C was abundant, but the N supply was limited (i.e., straw treatment with low available N). Conversely, the biostimulant reduced total shoot lengths of wheat, irrespective of soil amendment when compared to the control. However, Chen et al. (2003) observed that this result was most likely related to the different number of germinated plants in each microcosm, thus introducing a factor of plant competition.
Chen et al. (2003) concluded that the soil N dynamics were highly influenced by the soil amendments (i.e., straw and alfalfa) rather than any effect of the biostimulant on the microbial community, particularly because microbial activity was reduced in biostimulant-treated soils. The authors also suggested that in addition to nutrient pools such as N, other possible mechanisms could affect the native soil microbial community and crop growth. These included other chemical growth factors such as micronutrients, extracellular enzymes, and plant growth regulators. Chen et al. (2003) were unable to confirm the precise biostimulant mechanisms operating, especially with regard to their effect on microbial activity. However, the authors stated that they remained confident that biostimulants do have the capability of inhibiting or stimulating particular areas of the microbial community, which influence N transformations in the soil and N uptake by the crop (Chen et al., 2003). Their uncertainty regarding biostimulant mechanisms reflects the overall ambivalence of biostimulant effects on soil properties in general and soil microbiology and nutrient cycling in particular.
A summary of all the evidence reviewed above, in addition to details of each study’s experimental design, is presented in Table 1. This table highlights the common factors that have been used to explain the link between biostimulants and the changes in native soil microbiology. The primary mechanism that was discussed related to the stimulation of the native soil microbiology, producing more bioavailable nutrients for plant uptake. Figure 3 illustrates this linear sequence. Specifically, this mechanism was related to the ingredients of the biostimulants. These ingredients are comprised of accessible components for soil microbial utilization, similar to the nutrients (e.g., C and N) required by soil microorganisms to mineralize soil organic material. The mineralization of soil organic matter by microorganisms provides benefits to plant growth in the form of bioavailable nutrients (Glick, 1995). However, this review also highlights many conflicting and unclear results and contradictory interpretations of biostimulants’ impacts on soil microbial processes. This may have resulted from the variable experimental designs [in terms of spatial scale (i.e., field, glasshouse, laboratory), biostimulant type, and application dose and frequency] of the 10 articles relevant for review in this paper. This indicates the need for further work in understanding how these non-microbial biostimulants impact the native soil microbial community.
4 Concluding remarks
Current research into the mechanistic understanding of both microbial and non-microbial biostimulants focuses predominantly on plant-related mechanisms. There is a paucity of research investigating the influence of non-microbial biostimulants on soil biological, chemical, and physical properties and processes. It is well understood that the soil microbial community is critical in soil nutrient turnover cycles, which govern plant nutrient uptake and subsequent growth and productivity. Yet, there is a significant lack of research into how biostimulants impact the native soil microbial community.
The evidence reviewed in this paper suggests that by stimulating the native soil microbial community directly, non-microbial biostimulants may enhance the production of bioavailable nutrients in the soil, necessary for plant uptake and subsequent growth. The few articles that have investigated this mode of action describe varying changes in the soil microbial community characteristics, such as the community structure, total C/N biomass, and respiration rates. This is explained by the individual ingredients of biostimulant products, which are proposed to be the source of energy required by soil microorganisms to mineralize soil organic material into plant available nutrient forms. However, there are mixed results on how these biostimulants affect the overall function of these microbial communities in terms of C and N mineralization. Additionally, some explanations link the biostimulant impact to soil physical and chemical properties, which in turn impact soil microbiology (and vice versa). The interdependence of soil biological, physical, and chemical properties make these relationships challenging to decipher.
Furthermore, with only 10 articles investigating non-microbial biostimulants effects on soil properties and processes, comparing results from significantly different experimental designs [e.g., biostimulant type (often including multiple combinations), dose, and frequency of application] remains challenging. This has resulted in no common mechanistic understanding of biostimulants’ impact on soil properties. Unanswered questions persist of how biostimulants impact the microbial community generally and attributing individual modes of action.
This review highlights the need for further research into the influence of biostimulants in stimulating the native soil microbiology and related soil properties as a mechanism for improved plant productivity. More specifically in the short term, investigations using biostimulant treatments that incorporate both combination and singular biostimulants will help to attribute more direct impacts to different biostimulant types. In the long term, future work should investigate how the proposed mechanisms of stimulation of soil microbiology by biostimulants affect measurable plant yield and quality, primarily through nutrient uptake from the soil. Future research in this area will provide clarity on biostimulants’ modes of action and more transparent linkages between biostimulant use and improved plant growth and development.
Author contributions
DR: Conceptualization, Formal Analysis, Investigation, Methodology, Writing – original draft, Writing – review & editing. JR: Conceptualization, Writing – review & editing. MP: Conceptualization, Writing – review & editing.
Funding
The author(s) declare financial support was received for the research, authorship, and/or publication of this article. This research was funded by UKRI BBSRC FoodBioSystems Doctoral Training Partnership (DTP) and Sainsbury’s, grant number BB/T008776/1.
Conflict of interest
The authors declare that the research was conducted in the absence of any commercial or financial relationships that could be construed as a potential conflict of interest.
Publisher’s note
All claims expressed in this article are solely those of the authors and do not necessarily represent those of their affiliated organizations, or those of the publisher, the editors and the reviewers. Any product that may be evaluated in this article, or claim that may be made by its manufacturer, is not guaranteed or endorsed by the publisher.
References
Adeleke R., Wangburuka N., Boirien O. (2017). Origins, roles and fate of organic acids in soils: A review. South Afr. J. Bot. 108, 393–406. doi: 10.1016/j.sajb.2016.09.002
Akram N. A., Saleem M. H., Shafiq S., Naz H., Farid-ul-Haq M., Ali B., et al. (2022). Phytoextracts as crop biostimulants and natural protective agents—A critical review. Sustainability 14, 14498. doi: 10.3390/su142114498
Alam M. Z., Braun G., Norrie J., Hodges D. M. (2013). Effect of Ascophyllum extract application on plant growth, fruit yield and soil microbial communities of strawberry. Can. J. Plant Sci. 93, 23–36. doi: 10.4141/cjps2011-260
Alam M. Z., Braun G., Norrie J., Hodges D. M. (2014). Ascophyllum extract application can promote plant growth and root yield in carrot associated with increased root-zone soil microbial activity. Can. J. Plant Sci. 94, 337–348. doi: 10.4141/cjps2013-135
Ali O., Ramsubhag A., Jayaraman J. (2021). Biostimulant properties of seaweed extracts in plants: implications towards sustainable crop production. Plants 10, 531. doi: 10.3390/plants10030531
Araújo R. A.Parra-Saldívar R.Melchor-Marínez E. M.Iqbal C. (2023). Agro-food systems and environment: Sustaining the unsustainable. Current Opinion in Environmental Science & Health, Vol. 1325. 100413
Bailey V. L., McCue L. A., Fansler S. J., Boyanov M. I., DeCarlo F., Kemner K. M., et al. (2013). Micrometer-scale physical structure and microbial composition of soil macroaggregates. Soil Biol. Biochem. 65, 60–68. doi: 10.1016/j.soilbio.2013.02.005
Banerjee S., Kirkby C. A., Schmutter D., Bissett A., Kirkegaard J. A., Richardson A. E. (2016). Network analysis reveals functional redundancy and keystone taxa amongst bacterial and fungal communities during organic matter decomposition in an arable soil. Soil Biol. Biochem. 97, 188–198. doi: 10.1016/j.soilbio.2016.03.017
Bauer M., Kube M., Teeling H., Richter M., Lombardot T., Allers E., et al. (2006). Whole genome analysis of the marine Bacteroidetes’Gramella forsetii’ reveals adaptations to degradation of polymeric organic matter. Environ. Microbiol. 8, 2201–2213. doi: 10.1111/j.1462-2920.2006.01152.x
Braziene Z., Paltanavicius V., Avizienytė D. (2021). The influence of fulvic acid on spring cereals and sugar beets seed germination and plant productivity. Environ. Res. 195, 1–5. doi: 10.1016/j.envres.2021.110824
Bulgari R., Franzoni G., Ferrante A. (2019). Biostimulants application in horticultural crops under abiotic stress conditions. Agronomy 9, 1–30. doi: 10.3390/agronomy9060306
Casida L. E. (1977). Microbial metabolic activity in soil as measured by dehydrogenase determinations. Appl. Environ. Microbiol. 34, 630–636. doi: 10.1128/aem.34.6.630-636.1977
Castiglione A. M., Mannino G., Contartese V., Bertea C. M., Ertani A. (2021). Microbial biostimulants as response to modern agriculture needs: composition, role and application of these innovative products. Plants 10, 1533. doi: 10.3390/plants10081533
Celiktopuz E., Kapur B., Sarıdas M. A., Kargı S. P. (2021). Response of strawberry fruit and leaf nutrient concentrations to the application of irrigation levels and a biostimulant. J. Plant Nutr. 44, 153–165. doi: 10.1080/01904167.2020.1806310
Chen S. K., Edwards C. A., Subler S. (2003). The influence of two agricultural biostimulants on nitrogen transformations, microbial activity, and plant growth in soil microcosms. Soil Biol. Biochem. 35, 9–19. doi: 10.1016/S0038-0717(02)00209-2
Chen S. K., Subler S., Edwards C. A. (2002). Effects of agricultural biostimulants on soil microbial activity and nitrogen dynamics. Appl. Soil Ecol. 19, 249–259. doi: 10.1016/S0929-1393(02)00002-1
Comerford N. (2005). Soil factors affecting nutrient bioavailability in Nutrient acquisition by plants (Springer, Berlin, Heidelberg), 1–14.
Critchley A. T., Critchley J. S. C., Norrie J., Gupta S., Van Staden J. (2021). Perspectives on the global biostimulant market: applications, volumes, and values 2016 data and projections to 2022 in Biostimulants for crops from seed germination to plant development: A practical approach (London, UK: Academic Press), 289–296. doi: 10.1016/B978-0-12-823048-0.00012-5
Crowther T. W., Boddy L., Hefin Jones T. (2012). Functional and ecological consequences of saprotrophic fungus–grazer interactions. ISME J. 6, 1992–2001. doi: 10.1038/ismej.2012.53
del Buono D. (2021). Can biostimulants be used to mitigate the effect of anthropogenic climate change on agriculture? It is time to respond. Sci. Total Environ. 751, 2–9. doi: 10.1016/j.scitotenv.2020.141763
Dick R. P., Kandeler E. (2004). Enzymes in soils in Encyclopedia of soils in the environment (Oxford, UK: Elsevier Inc.), 448–456.
Di Mola I., Cozzolino E., Ottaiano L., Nocerino S., Rouphael Y., Colla G., et al. (2020). Nitrogen use and uptake efficiency and crop performance of baby spinach (Spinacia oleracea L.) and Lamb’s Lettuce (Valerianella locusta L.) grown under variable sub-optimal N regimes combined with plant-based biostimulant application. Agronomy 10, 1–15. doi: 10.3390/agronomy10020278
du Jardin P. (2012). The Science of Plant Biostimulants–A bibliographic analysis in Ad hoc study report to the european commission DG ENTR (Luxemborg: Publications Office of the European Union), vol. 2012
du Jardin P. (2015). Plant biostimulants: Definition, concept, main categories and regulation. Sci. Hortic. 196, 3–14. doi: 10.1016/j.scienta.2015.09.021
du Jardin P., Xu L., Geelen D. (2020). Agricultural functions and action mechanisms of plant biostimulants (PBs) in The chemical biology of plant biostimulants (United States: Wiley), 1–30
Fang Z., Wang X., Zhang X., Zhao D., Tao J. (2020). Effects of fulvic acid on the photosynthetic and physiological characteristics of Paeonia ostii under drought stress. Plant Signal Behav. 15, 1–11. doi: 10.1080/15592324.2020.1774714
García-Martínez A. M., Díaz A., Tejada M., Bautista J., Rodríguez B., Santa María C., et al. (2010). Enzymatic production of an organic soil biostimulant from wheat-condensed distiller solubles: Effects on soil biochemistry and biodiversity. Process Biochem. 45, 1127–1133. doi: 10.1016/j.procbio.2010.04.005
Glick B. R. (1995). The enhancement of plant growth by free-living bacteria. Can. J. Microbiol. 41, 109–117. doi: 10.1139/m95-015
Grammenou A., Petropoulos S. A., Thalassinos G., Rinklebe J., Shaheen S. M., Antoniadis V. (2023). Biostimulants in the soil–Plant interface: agro-environmental implications—A review. Earth Syst. Environ. 7, 3 7, 583–600. doi: 10.1007/s41748-023-00349-x
Halpern M., Bar-Tal A., Ofek M., Minz D., Muller T., Yermiyahu U. (2015). The use of biostimulants for enhancing nutrient uptake. Adv. Agron. 130, 141–174. doi: 10.1016/bs.agron.2014.10.001
Hellequin E., Monard C., Chorin M., Le Bris N., Daburon V., Klarzynski O., et al. (2020). Responses of active soil microorganisms facing to a soil biostimulant input compared to plant legacy effects. Sci. Rep. - Nat. 10, 1–12. doi: 10.1038/s41598-020-70695-7
Hellequin E., Monard C., Quaiser A., Henriot M., Klarzynski O., Binet F. (2018). Specific recruitment of soil bacteria and fungi decomposers following a biostimulant application increased crop residues mineralization. PloS One 13, 1–19. doi: 10.1371/journal.pone.0209089
Jindo K., Olivares F. L., Malcher D. J., da P., Sánchez-Monedero M. A., Kempenaar C., et al. (2020a). From lab to field: role of humic substances under open-field and greenhouse conditions as biostimulant and biocontrol agent. Front. Plant Sci. 11, 426. doi: 10.3389/fpls.2020.00426
Jindo K., Pasqualoto Canellas L., Albacete A., Figueiredo dos Santos L., Luiz Frinhani Rocha R., Carvalho Baia D., et al. (2020b). Interaction between humic substances and plant hormones for phosphorous acquisition. Agronomy 10, 2–18. doi: 10.3390/agronomy10050640
Lauber C. L., Hamady M., Knight R., Fierer N. (2009). Pyrosequencing-based assessment of soil pH as a predictor of soil bacterial community structure at the continental scale. Appl. Environ. Microbiol. 75, 5111–5120. doi: 10.1128/AEM.00335-09
Liu M., Han G. (2020). Assessing soil degradation under land-use change: Insight from soil erosion and soil aggregate stability in a small karst catchment in southwest China. PeerJ 8, e8908. doi: 10.7717/peerj.8908
Louca S., Polz M. F., Mazel F., Albright M. B. N., Huber J. A., O’Connor M. I., et al. (2018). Function and functional redundancy in microbial systems. Nat. Ecol. Evol. 2, 936–943. doi: 10.1038/s41559-018-0519-1
Macias-Benitez S., Garcia-Martinez A. M., Caballero Jimenez P., Gonzalez J. M., Tejada Moral M., Parrado Rubio J. (2020). Rhizospheric organic acids as biostimulants: monitoring feedbacks on soil microorganisms and biochemical properties. Front. Plant Sci. 11, 1–11. doi: 10.3389/fpls.2020.00633
Moher D., Liberati A., Tetzlaff J., Altman D. G. (2009). Preferred reporting items for systematic reviews and meta-analyses: the PRISMA statement. BMJ 339, 332–336. doi: 10.1136/bmj.b2535
Moreno-Hernández J. M., Benítez-García I., Mazorra-Manzano M. A., Ramírez-Suárez J. C., Sánchez E. (2019). Strategies for production, characterization and application of protein-based biostimulants in agriculture: A review. Chil J. Agric. Res. 80, 274–285. doi: 10.4067/S0718-58392020000200274
Navnage N., Patle P., Ramteke P. (2018). Dehydrogenase activity (DHA): Measure of total microbial activity and as indicator of soil quality. Int. J. Chem. Stud. 6, 456–458. doi: 10.37273/chesci.cs205205338
Ngoroyemoto N., Kulkarni M. G., Stirk W. A., Gupta S., Finnie J. F., Van Staden J. (2020). Interactions between microorganisms and a seaweed-derived biostimulant on the growth and biochemical composition of amaranthus hybridus L. Nat. Prod Commun. 15, 1–11. doi: 10.1177/1934578X 20934228
Palmieri F., Estoppey A., House G. L., Lohberger A., Bindschedler S., Chain P. S. G., et al. (2019). Oxalic acid, a molecule at the crossroads of bacterial-fungal interactions. Adv. Appl. Microbiol. 106, 49–77. doi: 10.1016/bs.aambs.2018.10.001
Popa D. G., Lupu C., Constantinescu-Aruxandei D., Oancea F. (2022). Humic substances as microalgal biostimulants—Implications for microalgal biotechnology. Mar. Drugs 20, 327. doi: 10.3390/md20050327
Preston-Mafham J., Boddy L., Randerson P. F. (2002). Analysis of microbial community functional diversity using sole-carbon-source utilisation profiles - a critique. FEMS Microbiol. Ecol. 42, 1–14. doi: 10.1111/j.1574-6941.2002.tb00990.x
Renaut S., Masse J., Norrie J. P., Blal B., Hijri M. (2019). A commercial seaweed extract structured microbial communities associated with tomato and pepper roots and significantly increased crop yield. Microb. Biotechnol. 12, 1346–1358. doi: 10.1111/1751-7915.13473
Rouphael Y., Cardarelli M., Bonini P., de Pascale S., Colla G. (2020). Implications of microbial and non-microbial biostimulatory action on the quality of leafy and fruit vegetables. Acta Hortic. 1268, 13–17. doi: 10.17660/ActaHortic.2020.1268.2
Rousk J., Brookes P. C., Bååth E. (2009). Contrasting soil pH effects on fungal and bacterial growth suggest functional redundancy in carbon mineralization. Appl. Environ. Microbiol. 75, 1589–1596. doi: 10.1128/AEM.02775-08
Sadeghinia M., Kargar M., Jafarian Z. (2023). The relationship between the functional diversity, functional redundancy and community stability in mountain rangelands. Community Ecol. 24, 1–8. doi: 10.1007/s42974-022-00128-0
Selvarajan R., Sibanda T., Venkatachalam S., Ogola H. J. O., Christopher Obieze C., Msagati T. A. (2019). Distribution, interaction and functional profiles of epiphytic bacterial communities from the rocky intertidal seaweeds, South Africa. Sci. Rep. 9, 1 9, 1–1 9,13. doi: 10.1038/s41598-019-56269-2
Shahrajabian M. H., Chaski C., Polyzos N., Tzortzakis N., Petropoulos S. A. (2021). Sustainable agriculture systems in vegetable production using chitin and chitosan as plant biostimulants. Biomolecules 11, 819. doi: 10.3390/biom11060819
Skiba U. (2008). Denitrification. Encyclopedia Ecol. 5, 866–871. doi: 10.1016/B978-008045405-4.00264-0
Snyder H. (2019). Literature review as a research methodology: An overview and guidelines. J. Bus Res. 104, 333–339. doi: 10.1016/j.jbusres.2019.07.039
Stefanowicz A. (2006). The biolog plates technique as a tool in ecological studies of microbial communities. Pol. J. Environ. Stud. 15, 669–676
Tecon R., Or D. (2017). Biophysical processes supporting the diversity of microbial life in soil. FEMS Microbiol. Rev. 41, 599–623. doi: 10.1093/femsre/fux039
The European Parliament, The Council of the European Union (2019). Regulation (EU) 2019/1009 (Luxemborg: The Publications Office of the European Union). Available at: http://data.europa.eu/eli/reg/2019/1009/oj.
Tripathi S., Srivastava P., Devi R. S., Bhadouria R. (2020). Influence of synthetic fertilizers and pesticides on soil health and soil microbiology. Agrochemicals Detection Treat Remediation 1, 25–54. doi: 10.1016/B978-0-08-103017-2.00002-7
Van Der Heijden M. G. A., Bardgett R. D., Van Straalen N. M. (2008). The unseen majority: soil microbes as drivers of plant diversity and productivity in terrestrial ecosystems. Ecol. Lett. 11, 296–310. doi: 10.1111/j.1461-0248.2007.01139.x
Wadduwage J., Liu H., Egidi E., Singh B. K., Macdonald C. A. (2023). Effects of biostimulant application on soil biological and physicochemical properties: A field study. J. Sustain. Agric. Environ. 2, 285–300. doi: 10.1002/sae2.12057
Wang M., Lei C., Li Y., Lin C., Liu Z., Wang X., et al. (2018). Responses of soil microbial communities to a short-term application of seaweed fertilizer revealed by deep amplicon sequencing. Appl. Soil Ecol. 125, 288–296. doi: 10.1016/j.apsoil.2018.02.013
Wolinska A., Stepniewsk Z. (2012). Dehydrogenase activity in the soil environment in Dehydrogenases (London, UK: InTech), 184–202.
Yakhin O. I., Lubyanov A. A., Yakhin I. A., Brown P. H. (2017). Biostimulants in plant science: A global perspective. Front. Plant Sci. 7, 2049. doi: 10.3389/fpls.2016.02049
Yousfi S., Marín J., Parra L., Lloret J., Mauri P. V., Boldú P. (2021). A rhizogenic biostimulant effect on soil fertility and roots growth of turfgrass. Agronomy 11, 573. doi: 10.3390/agronomy11030573
Keywords: humic substances, protein hydrolysates, seaweed extracts, soil functions, soil microbiology
Citation: Roche D, Rickson JR and Pawlett M (2024) Moving towards a mechanistic understanding of biostimulant impacts on soil properties and processes: a semi-systematic review. Front. Agron. 6:1271672. doi: 10.3389/fagro.2024.1271672
Received: 02 August 2023; Accepted: 25 April 2024;
Published: 13 May 2024.
Edited by:
Garima Singh, Pachhunga University College, IndiaReviewed by:
Federica Caradonia, University of Modena and Reggio Emilia, ItalyYoussef Rouphael, University of Naples Federico II, Italy
Dr. Bendangchuchang Longchar, Pachhunga University College, India
Copyright © 2024 Roche, Rickson and Pawlett. This is an open-access article distributed under the terms of the Creative Commons Attribution License (CC BY). The use, distribution or reproduction in other forums is permitted, provided the original author(s) and the copyright owner(s) are credited and that the original publication in this journal is cited, in accordance with accepted academic practice. No use, distribution or reproduction is permitted which does not comply with these terms.
*Correspondence: Dannielle Roche, ZGFubmllbGxlLnJvY2hlQGNyYW5maWVsZC5hYy51aw==