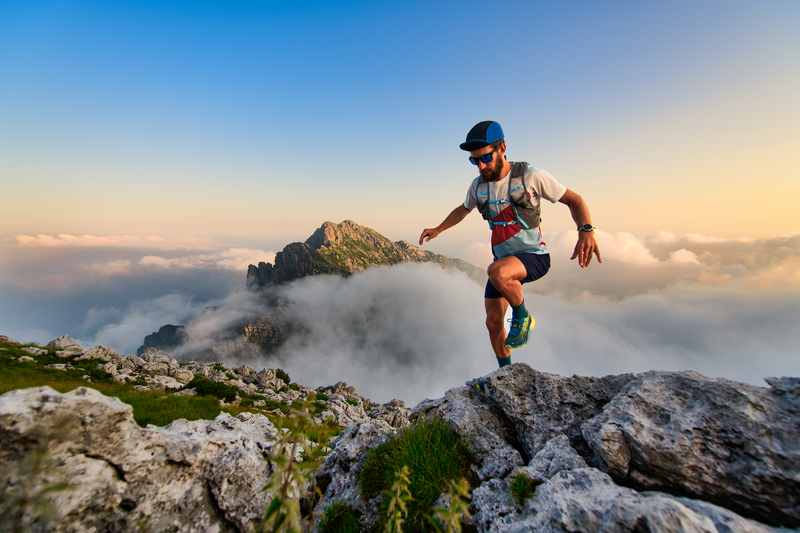
95% of researchers rate our articles as excellent or good
Learn more about the work of our research integrity team to safeguard the quality of each article we publish.
Find out more
REVIEW article
Front. Agron. , 15 January 2024
Sec. Plant-Soil Interactions
Volume 5 - 2023 | https://doi.org/10.3389/fagro.2023.1293555
The reuse of wastewater to meet irrigation requirements and slurries, sludges and manures as fertilisers to meet crop nutrient demands inadvertently introduces human and veterinary-use pharmaceuticals into the agro-ecosystem. This review synthesises recent research, which has observed sub-lethal effects, following pharmaceutical uptake by plants. Potential links between pharmaceutical mode of action and observed sub-lethal effects in the plant were then examined. Common receptors and biological pathways across species suggests a clear need to integrate plant cellular biology into our understanding of the impacts of pharmaceuticals on important plant functions and processes. To help prioritise future research efforts an analysis of shared mammalian and plant biochemical pathways was undertaken to identify classes of pharmaceuticals which may present a greater risk to key plant functions. These included sulfonylurea antihyperglycemics, steroids, opiods, antipsychotic phenothiazines and pharmaceuticals targeting several neurotransmitters shared between mammals and plants (including beta-blockers, antihistamines and benzodiazepines). Whilst a number of pharmaceutical induced sub-lethal effects have been observed, this review highlights the clear need to study a wider range of pharmaceuticals on a broader range of plant species, including cover crops and wild plants, under realistic exposure scenarios, to fully understand the wider implications of pharmaceutical exposure in agro-ecosystems. State-of-the art omics-techniques offer great potential to understand the mode of action of pharmaceuticals in plants and elucidate links between the pharmaceutical intended mode of action and observed plant effects. In addition, studies under co-stress from pharmaceutical exposure and other stressors such as increased temperatures, drought or pests are lacking and present an urgent research need in the face of feeding a growing population under the threats of climate change.
Pharmaceuticals are used for the treatment, cure and prevention of disease; whilst they offer many benefits, their use has resulted in them becoming ubiquitous global pollutants with numerous published reports documenting the presence of pharmaceuticals from human and veterinary origin in freshwater and marine systems (Gaw et al., 2014; Madikizela et al., 2017; Mezzelani et al., 2018; Peña-Guzmán et al., 2019; Khan et al., 2020; Branchet et al., 2021; Świacka et al., 2022; Mo et al., 2022). Pharmaceuticals can also enter the agricultural environment following the use of treated and untreated wastewater as a source of irrigation (Carter et al., 2019), and the use of organic soil amendments as fertilisers, such as manure, slurry and wastewater derived biosolids (Zheng and Guo, 2021). Faced with the need to meet increasing global food demands in a sustainable manner, the reuse of materials such as human and animal excreta offers many benefits within the context of circular economy, including the reuse of waste which would otherwise need to be disposed of, the recovery of valuable nutrients and a consistent irrigation source in water stressed regions (Jimenez-Cisneros, 2006; Thebo et al., 2017; Helmecke et al., 2020; Drechsel et al., 2022). However, the presence of human and veterinary pharmaceuticals in these resources creates a pathway by which these chemicals can enter into agro-ecosystems (Azanu et al., 2016; Carter et al., 2019; Rocha et al., 2021; Zheng and Guo, 2021). Pharmaceuticals are typically found at low concentrations in irrigation waters (ng-mg L-1) and in soil amendments (ng-mg kg-1), but their consistent application ensures their continual introduction into soils and allows for potential build up over time up to detectable levels. For example, a recent study observed concentrations ranging from 0.7 (diazepam) to 1004.7 ng L-1 (gabapentin), in treated wastewater used for agricultural irrigation in Israel (Ben Mordechay et al., 2021). Another study measured concentrations in the Tula Valley in Mexico, where wastewater is not always treated before use as a source of agricultural irrigation, ranging from 4.5 ng L-1 (estrone-3-sulfate) to 299.1 (acetaminophen) μg L-1 of (Garduño-Jiménez et al., 2023). Reported measured concentrations of pharmaceuticals in soil amendments used in agriculture include sludge concentrations ranging from 17.1 ± 0.6 (fluoxetine) to 4,410.9 ± 485.6 (ofloxacin) ng g -1 (Bourdat-Deschamps et al., 2017) and in chicken slurry pharmaceuticals ranging from 27 (salicylic acid) to 72 (sulfamethazine) ng g-1 (Wychodnik et al., 2020). It has been widely reported that once in the environment, pharmaceuticals can degrade, nevertheless their constant application makes them pseudo-persistent chemicals (Daughton, 2003). This presents a pressing challenge as it has been widely demonstrated that once in the agricultural environment pharmaceuticals can be taken up, and accumulated in plants (Carter et al., 2019; Fu et al., 2019; Gworek et al., 2021; Mansilla et al., 2021; Rocha et al., 2021; Zheng and Guo, 2021).
The uptake of pharmaceuticals by plants has been demonstrated across a range of scales from benchtop experiments to agriculturally relevant field scenarios. Maximum in-plant accumulation has been typically demonstrated in hydroponic studies, in the absence of soil as potential sorptive medium (Christou et al., 2019; Klampfl, 2019). For example, lettuce grown in a hydroponic system containing diclofenac (20, 000 ng L-1) observed diclofenac accumulation in root tissue (6,020 ng g-1 dry weight) but remained undetected in the lettuce (Lactuca sativa) leaves at the same time point (Bigott et al., 2021). Comparatively, studies including the soil compartment, have also demonstrated pharmaceutical uptake and accumulation. In an early study a controlled experiment with radish (R. sativus) and ryegrass (L. perenne), Carter et al., observed that five out of the six pharmaceuticals directly added to the soil were taken up by both plants, with pharmaceutical physicochemical properties (e.g log D) and soil parameters (e.g organic carbon content) observed to strongly influence the degree of uptake (Carter et al., 2014). Another study, where the set-up was a field divided into two sections, one irrigated with tertiary treated wastewater and the other with water fortified with 16 pharmaceuticals and personal care products, found caffeine, meprobamate, primidone, DEET, carbamazepine, dilantin, naproxen, and triclosan detected in edible tissues of a variety of root, stem and leaf vegetables, with total chemical concentrations of 0.01−3.87 and 0.15−7.3 ng g-1 (dry weight) for the first and second irrigation treatment respectively (Wu et al., 2014). Agricultural field studies have also demonstrated pharmaceutical uptake in crops. For example, in Spanish agricultural fields, tramadol, ofloxacin, tonalide, gemfibrozil, atenolol and caffeine, were detected at the highest levels in irrigation water samples (ranging from 11,000 to 44,000 ng L-1), leading to detectable concentrations in soil (e.g. tramadol, cetirizine and clarithromycin measured at 12.7 to 14.6 ng g-1) and finally detection in crops of carbamazepine (0.1 to 1.7 ng g-1) (García-Valverde et al., 2023). Comparatively a study conducted in agricultural fields irrigated with reclaimed wastewater in Israel found up to 46 pharmaceuticals and personal care products detected in irrigation water, leading to 21 chemicals detected in soil samples and a total of 23 detections at quantifiable levels in edible agricultural produce (Ben Mordechay et al., 2021). As an example, carbamazepine concentrations in irrigation water were 784.8 ± 1,378.6 ng L-1 and in agricultural soils 3.6 ± 7.1 ng g-1; leading to carbamazepine being the highest concentration reported in crops, specifically in parsley, with measured concentrations of up to 2,470 ng g-1 (Ben Mordechay et al., 2021).
The uptake of pharmaceuticals by plants mostly depends on the pollutant’s availability which is linked to the persistence and sorption of the chemical and the soil solution chemistry, the plant’s transpiration rate and physiology, the structure of the plant’s cell membrane and the physicochemical properties of the pollutant (Christou et al., 2019; Kodesova et al., 2019; Ben Mordechay et al., 2021) (Figure 1). In terms of soil properties, crops grown in predominantly sandy soils or soils with low organic matter, or clay content typically have a higher potential to accumulate pharmaceuticals compared to soils rich in clay or organic matter where the chemicals are less bioavailable due to strong sorption interactions (Christou et al., 2019). With respect to pharmaceutical physicochemical properties, pharmaceutical uptake has been shown to increase with increasing chemical lipophilicity (logkow, or pH corrected logDow), molecular size, weight and polarity (Fu et al., 2019). Lipophilic compounds can cross membranes through passive diffusion and enter the symplast pathway, enabling translocation via the xylem (Fu et al., 2019). Unionised chemicals (as opposed to anionic or cationic) typically demonstrate a greater potential for uptake. This is because negatively charged molecules are repelled by the cell walls of the root apoplast and the uptake and translocation of positively charged molecules is reduced by their attraction to the negatively charged cell walls (Christou et al., 2019; Kodesova et al., 2019). However, it is important to note that different uptake mechanisms such as organic cation transporters, a group of proteins in plant cell membranes, have been shown to be involved in the uptake of charged chemicals, such as metformin (Cui et al., 2015). Acidic pharmaceuticals (pka<7, logkow <3) tend to remain in the phloem and therefore accumulate more in fruits, whereas basic pharmaceuticals (pka > 7) which are hydrophilic (LogKow < 0) tend to move both in the xylem and phloem and those which are lipophilic (0 < LogKow < 4) tend to move in the xylem (Christou et al., 2019). It has been widely reported that moderately lipophilic neutral compounds (logKow 2 to 5) such as carbamazepine and diazepam can cross membranes through passive diffusion, enabling translocation via the xylem (Fu et al., 2019) (Figure 1). In addition to chemical properties and soil parameters, pharmaceutical accumulation has been shown to be influenced by plant physiology with accumulation typically in the order of leafy vegetables > root vegetables > cereals and fodder crops > fruit vegetables (Christou et al., 2019; Ben Mordechay et al., 2021).
Figure 1 A summary diagram demonstrating key mechanisms of pharmaceutical uptake and accumulation in plants and observed sub-lethal plant effects.
The uptake and accumulation of pharmaceuticals in plants, presents a risk to the health of the plant (i.e. ecotoxicity) (Mansilla et al., 2021; Rocha et al., 2021) and/or to human health following the consumption of contaminated produce (Keerthanan et al., 2021). Potential implications for human health have been comprehensively reviewed elsewhere (Keerthanan et al., 2021) and this review will focus specifically on the potential for pharmaceutical induced plant effects. Batchelder et al., provided early evidence of the potential for pharmaceuticals to induce negative effects on crop growth (pinto beans) following a hydroponic study at 160 ppm of oxytetracycline (Batchelder, 1981). Examples of other toxic effects on plant physiology include chlorosis and necrosis on zucchini (C. pepo) leaves, from soil concentrations of carbamazepine ranging from 1 to 20 mg kg-1 (Knight et al., 2017). Negative effects on lettuce (L. sativa) root morphology have also been observed following exposure to estradiol and ethinyl estradiol concentrations ranging from 0.1- 10,000 μg L-1 (Adeel et al., 2017). Other examples include significant decrease in germination of lettuce (L. sativa) seeds following exposure to ampicillin, colistin, amoxicillin, ampicillin and reduced biomass following amoxicillin exposure (Benassi-Borba et al., 2021). Reduced biomass has also been observed in lettuce (L. sativa) grown hydroponically exposed to a mixture of antiretrovirals at a concertation of 100 μgL-1 (Akenga et al., 2021). It is important to note that whilst measurable effects have been observed the concentrations at which these effects are typically observed are generally higher (i.e. mg kg-1) than those observed in real agricultural environments (i.e. ng kg-1 to low ug kg-1). Nevertheless, this does not preclude the potential for these biologically active pharmaceuticals to induce sub-lethal effects at much lower, environmentally relevant concentrations. Recently there has been a shift to study effects of pharmaceuticals beyond biomass and lethality, in order to understand sub-lethal effects which often occur at low-level chronic exposures (Saaristo et al., 2018). Sub-lethal effects include metabolism and/or behaviour which may have cascading effects through ecosystems (Mayer-Pinto et al., 2020).
Pharmaceuticals are mostly designed to be biologically active and interact with mammal biological receptors and this potency is not lost in the environment or when taken up by biota, such as plants. Importantly studies have also unveiled plants have more in common with mammals than previously thought, for example their ability to communicate with each other (Sharifi and Ryu, 2021; Brosset and Blande, 2022) as well as signalling in response to environmental stimuli (Akula and Mukherjee, 2020; Segundo-Ortin and Calvo, 2022). Evidence suggests several communication, and biochemical pathways in plants are shared with those in mammals (Segundo-Ortin and Calvo, 2022). Notable examples include the use of electrical action potentials, akin to neurons in mammals (Favre and Agosti, 2007; Felle and Zimmermann, 2007) and similar responses of plants to anaesthetics as those exhibited by mammals (Yokawa et al., 2017). For a majority of pharmaceuticals it has also been established that pharmaceutical potency is not lost when these chemicals enter the environment, including in the soil-plant continuum. Shared receptors between species (i.e. mammals and plants) provides a route by which response pathways can be initiated (Rocha et al., 2021; Krupka et al., 2022). For example anti-convulsant pharmaceuticals are designed to interact with cation channels in humans (Waszkielewicz et al., 2013) and similar ion channels are found in plants, which could be affected by these pharmaceuticals following accumulation by the plant (Carter et al., 2014). Given the widespread prevalence of human and veterinary pharmaceuticals in the environment, it is therefore necessary to examine whether these chemicals are responsible for unintended sub-lethal effects on plant biological functioning which, following exposure at higher concentrations have already been shown to result in the manifestation of responses such as plant lethality and reduced germination and growth. Impacts on plant functioning have the potential to result in wider ecosystem effects including for example our ability to meet the food demands of a growing population (Cheng and Cheng, 2015; Fu et al., 2019).
The aim of this review was therefore to present an up-to-date synthesis of published literature which has demonstrated the potential for pharmaceuticals to induce sub-lethal plant effects. We divide the review in two sections, in Section 2 sub-lethal effects that have been studied following pharmaceutical exposure are reviwed. This section is divided in 4 sub-sections of the four classes of pharmaceuticals for which sub-lethal effects in plants have been studied, namely antibiotics, non-steroidal antiinflamatory drugs, antipsychotics and antihyperglycemics. Section 3 reviews the known biomolecular pathways key to plant life and explores how pharmaceuticals in use or in development may affect these biomolecular pathways based on shared receptors of their mode of action in the intended organism and that of plants. The pharmaceutical classes which were found to potentially affect plant biomolecular pathways were neuro-active pharmaceuticals (antipsychotics, antidepressants, acetylcholinesterase inhibitors) imidiazoles, histamines, beta-blockers, antihistamines, diuretic agent, antiparasitics (including antifungals and antibiotics), anti-cancer pharmaceuticals, non-steroidal antiinflamatory drugs and antineoplastics. Our review underpins further analysis exploring the similarities between the mode of action of pharmaceuticals in the intended organism and the observed effect in plants, as well as highlighting plant receptors which are expected to be affected by pharmaceuticals given receptor similarities amongst different species.
Traditionally, ecotoxicology has assessed the risk posed by chemicals based on survival (or lethality), as an endpoint, however this has been criticised as sub-lethal effects with severe consequences are more likely in the context of environmental chemical pollution (Straub et al., 2020). Environmental pollution is not limited to short-term, acute exposures; long-term and low-level chronic exposures can be equally damaging to the environment (Saaristo et al., 2018). Sub-lethal effects reflect changes beyond population abundance and shed light on effects on their physiology, metabolism and/or behaviour which may affect ecosystem functioning by reducing the fitness of organisms and these impacts may cascade through ecosystems (Mayer-Pinto et al., 2020). For example, in recent years there has been a move in pesticide ecotoxicology to assess sub-lethal endpoints considering population implications and soil health as a whole (Vischetti et al., 2020). In the regulatory context, recent European Chemical Agency (ECHA) methodologies assess reproduction activity, morphological and behavioural changes of earthworms, collembola and mites and evaluate nitrogen and carbon transformation activity for microorganisms (Vischetti et al., 2020). In plants, sub-lethal effects going beyond physiological changes affecting yield, such as reduced biomass and germination, have been classified as oxidative stress, affecting the phytohormone regulatory network, toxic effect on metabolomic pathways, stimulation of heat shock protein production, affecting micro RNAs and the production of Phase I and II metabolites which can have further effects (Mansilla et al., 2021). These effects can result in detrimental consequences in plant fitness and ecosystem health, which ultimately has agricultural implications in terms of feeding the growing population as well as environmental health concerns.
In this section we review the latest research which has evaluated the potential for pharmaceuticals to elicit sub-lethal effects in plants, and focus on understanding the relationship between observed effects and pharmaceutical mode of action. Table 1 provides a summary of select endpoints which have been attributed to pharmaceutical exposure. However, it is important to note that pharmaceuticals may undergo in-plant metabolism, where effects may sometimes be elicited by metabolites, instead of the pharmaceutical parent compound. As pharmaceutical metabolites are rarely quantified it is not always possible to differentiate between effects induced by the parent compound or potential metabolites (Madikizela et al., 2022).
A promising approach to understanding the mechanistics behind the sub-lethal effects detailed in this section and Table 1, are omics techniques. These include genomics, transcriptomics and miRNAomics as well as metabolomics, proteomics and lipidomics, which have been developed and used for multiple applications, including understanding molecular changes at virtually all levels of an organisms biology in organisms in the context of ecotoxicity (Worley and Powers, 2013; Liu et al., 2019; Matich et al., 2019; Ebner, 2021; Jamla et al., 2021). For example proteomics have been used to elucidate the molecular pathways by which stressors such as salinity and heat stress affect plants (Sun et al., 2017; Zhao et al., 2018). Whilst studies utilising omics approaches to investigate sub-lethal impacts of pharmaceuticals on plant functioning are still in their infancy, multi-omic approaches unveiled that nanoplastics can modulate terpenoid and flavonoid biosynthesis pathways by regulating small RNA transcription and protein expression (Yu et al., 2022).
Only four classes of pharmaceuticals were found to have been studied in relation to their sub-lethal effects on plants, namely antibiotics, non-steroidal antiinflamatory drugs, antipsychotics and antihyperglycemics, as discussed in this section. Section 3 will thenexplore which other classes should be studied based on biological receptors affected by their mode of action which are the same in plants as in the intended organism.
Antibiotics are designed to reduce/inhibit bacterial cellular growth, exploiting the differences between bacterial prokaryotic cells and mammal eukaryotic cells. The four main mode of action pathways of antibiotics include inhibition of 1) bacterial cell wall synthesis; 2) bacterial deoxyribonucleic acid (DNA) synthesis; 3) bacterial protein synthesis; 4) folate synthesis (Kaufman, 2011). Penicillin and cephalosporins (beta-lactam antibiotics) are amongst the most commonly used antibiotics (Krupka et al., 2022) and their mode of action is to interrupt bacterial cell wall formation through covalent binding to essential penicillin-binding enzymes that are involved in the terminal steps of peptidoglycan (the polysaccharide forming the outside of bacterial cell membrane) cross-linking in bacteria (Bush and Bradford, 2016). Given that plant cells do not share the cell wall structure of prokaryotic bacterial cells, it would be expected that these antibiotics are less toxic towards plants cells. Published research does exist which supports this theory (Rede et al., 2019), for example, amoxicillin did not affect germination of lettuce (L. sativa), alfalfa (M. sativa) and carrot (D. carota), when other antibiotics such as sulfamethoxazole did under the same conditions (Hillis et al., 2011). However, it is important to point that this research has assessed non-sublethal end points such as effects on germination, root elongation, shoot and leaf length (Hillis et al., 2011; Rede et al., 2019). Comparatively, a study assessing antibiotic induced sub-lethal effects, observed that amoxicillin, ampicillin and penicillin G at concentrations of 0.5 and 1.5 mg L−1 reduced photosynthetic electron transport rate in wheat (T. aestivum) (Opriş et al., 2013). The same study also observed reduced stomatal conductance and photosynthetic electron transport upon wheat exposure to cephalosporins (Opriş et al., 2013), which clearly shows antibiotic uptake can elicit sub-lethal responses in a plant (further examples in Table 1), despite the fact the mode of action for these effects remains unknown.
Antibiotics targeting deoxyribonucleic acid (DNA) synthesis include fluroquinolones (e.g. ciprofloxacin), and other commonly used antibiotics such as metronidazole, nitrofurantoin and rifampicin (Kaufman, 2011). In plants fluroquinolone uptake has resulted in a suite of morphological effects, such as oxidative stress, affecting several aspects of photosynthesis, including reducing photosynthetic pigments, and altering cellular energy allocation, as can be seen in Table 1, on different plant species including common bean (P. vulgaris), cucumber (C. sativus), common wheat (T. aestivum), field mustard (B, campestris (L)) and alfalfa (M. sativa) (Sodhi and Singh, 2021). Fluoroquinolone exposure has also been shown to affect photosynthesis (Aristilde et al., 2010; Rocha et al., 2021; Sodhi and Singh, 2021). A range of different pathways have been proposed to describe this effect, including for example 1) inhibition of photosystem II (PS-II), a key enzyme in photosynthetic electron transport (Aristilde et al., 2010) 2) an effect on mitochondrial electron transport chain (Gomes et al., 2017), 3) diminished Rubisco activity resulting in smaller vascular bundles which affect the transportation of photosynthesis products (Gomes et al., 2020) 4) and a reduction in chlorophyll content (Sodhi and Singh, 2021). Quinolones, like fluoroquinolones, are another class of antibiotic which inhibits the DNA synthesis of bacteria. This mode of action appears conserved in plants with demonstrated effects on plant DNA gyrase, impacting DNA replication and repair (Rocha et al., 2021). Quinolones have also been shown to elicit an oxidative stress response, including levofloxacin, enrofloxacin, norfloxacin in rice (O. sativa.) (Jin et al., 2023) and ciprofloxacin in microalgae (R. subcapitata) (Aderemi et al., 2018).
Antibiotics that interfere with protein synthesis include aminoglycosides, tetracyclines and macrolides (Kaufman, 2011). Protein synthesis pathways in plants and bacteria are similar, and as such uptake and accumulation of these antibiotics by plants is expected to affect chloroplast and mitochondrial systems (Opriş et al., 2013). Interestingly recent research has demonstrated that exposure to tetracyclines decreased several photosynthetic parameters (as can be seen in Table 1), including reducing electron transfer and photosynthetic pigments across several plant species, providing evidence that plant protein synthesis pathways were inhibited to a degree following antibiotic exposure (Rocha et al., 2021). Folate synthesis is another key pathway in plants which is responsible for nitrogen metabolism, a crucial nutrient for plant growth and development (Rocha et al., 2021). Plants share the folate biosynthetic pathway with bacteria (Cheong et al., 2020), so antibiotics which inhibit folate synthesis including sulfonamides and trimethoprim (Kaufman, 2011), are expected to have an impact on plant growth and development. As expected, sulfonamide exposure has been shown to reduce plant growth and germination as well as reduce chlorophyll content (Cheong et al., 2020).
As can be seen from Table 1, several classes of antibiotics have been shown to affect photosynthesis in plants. This is believed to be linked to chloroplasts being descendants of cyanobacteria; specifically pathways responsible for chloroplast division originate from the bacterial peptidoglycan synthesis pathway, the same pathway targeted by beta-lactam antibiotics (Krupka et al., 2022). Tetracyclines appear to interfere with mitochondrial and chloroplast protein synthesis, as well as with diminishing plant antioxidant capacities (Rocha et al., 2021). The pathways by which other antibiotic classes affect photosynthesis are not yet fully clear, however it is known is that antibiotic accumulation in plants leads to hydrogen peroxide (H2O2) accumulation in chloroplasts, resulting in protein damage which coordinates electron flow during photosynthesis (Krupka et al., 2022).
It is important to note that hormesis, positive effects of a stressor at low concentrations, have been observed in relation to antibiotic exposure to plants, for example by increasing chlorophyll content due to the reduction in the activity of chlorophyllase. It is not fully understood why this occurs, but there seems to be a link between production of reactive oxygen species (ROS) species upon stressor interaction and the activation of adaptive/beneficial responses (Krupka et al., 2022).
NSAIDs are medicines that are widely used to relieve pain, reduce inflammation, and bring down a high temperature. NSAID mode of action occurs via the non-selective inhibition of the cyclooxygenase enzymes, including cyclooxygenase-1 (COX-1) and cyclooxygenase-2 (COX-2) isoenzymes, which then prevents the formation of prostaglandins and thromboxane from arachidonic acid (AA) (Abbas et al., 2021). Despite the fact this pathway is not shared with plants, NSAID exposure has been shown to result in a range of sub-lethal effects in plants including oxidative stress and impacts on photosynthesis (Kummerová et al., 2016; Bigott et al., 2021; Siemieniuk et al., 2021; Madikizela et al., 2022), as can be seen in Table 1. From a proteomic analysis of lettuce (L. sativa) leaves and roots grown hydroponically following exposure to metformin, acetaminophen and carbamazepine (at 1 mg L-1) over 8 days found 612 and 237 significantly different proteins to controls in roots and leaves respectively. Exposure to acetaminophen, a pharmaceutical sometimes classified as a NSAID, was linked to cell respiration protein species being affected, suggesting possible dysregulation of the Krebs cycle (Leitão et al., 2021). The pathway by which this group of pharmaceuticals affects plants is therefore not clear; however further analysis is provided in Section 3.3 to explore this concept further.
The mode of action of antipsychotic drugs is not fully elucidated, however the way in which they affect neurotransmitters, many of which are shared with plants, can be linked to reported negative responses in plants (Table 1). Stress responses such as effects on cell respiration, photosynthesis, nutrient composition and plant circadian rhythm have all been reported following plant uptake and accumulation of antipsychotic pharmaceuticals (Carter et al., 2015; Bigott et al., 2021; Leitão et al., 2021). Specifically, exposure of lettuce (L. sativa) to 0.1, 1 and 5 mg L-1 of carbamazepine in hydroponic conditions for 15 days, resulted in several oxidative stress responses such as increase in hydrogen peroxide in leaves and roots and the increase of antioxidant enzymes. From a proteomic analysis of lettuce (L. sativa) leaves and roots grown hydroponically following exposure to metformin, acetaminophen and carbamazepine (at 1 mg L-1) over 8 days found 612 and 237 significantly different proteins to controls in roots and leaves respectively. Exposure to the antiphyschotic carbamazepine was linked to expression of stress proteins and cell respiration protein species being affected, suggesting possible dysregulation of the Krebs cycle (Leitão et al., 2021). The wide suite of effects observed is likely linked to the many key plant pathways regulated by neurotransmitters (further details provided in Section 3.2).
With increasing cases of type 2 diabetes mellitus, the number of oral antihyperglycemic pharmaceuticals in use has increased 5-fold in the past 6 years (Silvio E., 2022). Antihyperglycemic pharmaceuticals fit into five different categories based on their mode of action, namely sulfonylurea, nonsulfonylurea insulin secretagogues, biguanides, α-glucosidase inhibitors, and thiazolidinediones (Silvio E., 2022). This class of pharmaceutical is understudied in plants (further details in section 4.2), with only data available for metformin (Table 1). Metformin is commonly found in the aquatic environment due to its high use, incomplete metabolism in humans and poor removal in wastewater treatment plants (Ambrosio-Albuquerque et al., 2021). Metformin targets the AMP-activated protein kinase (AMPK), which is orthologues to the plant enzyme, sucrose-non-fermenting enzyme-related protein kinase-1 (SnRK1). In algae, metformin has been shown to inhibit photosynthesis by activating the plant enzyme SnRK1 (Ambrosio-Albuquerque et al., 2021). Further research is therefore needed to explore the effects of metformin uptake in crops as the plant enzyme SnRK1 can regulate cellular energy and reduce light processing capacity, in plants as well as algae. From a proteomic analysis of lettuce (L. sativa) leaves and roots grown hydroponically following exposure to metformin, acetaminophen and carbamazepine (at 1 mg L-1) over 8 days found 612 and 237 significantly different proteins to controls in roots and leaves respectively. Exposure to the antihyperglycemic metformin was linked to cell respiration protein species being affected, suggesting possible dysregulation of the Krebs cycle (Leitão et al., 2021).
The global use of medicines is growing. On average 38 new drugs were approved per year from 2010 to 2019 in North America alone. (U.S. Congress, 2021). It is therefore unrealistic to evaluate the potential effects of all active pharmaceutical ingredients on all plant species despite the fact that there are multiple conserved biomolecular pathways in eukaryotes that control key response and development functions in both mammals and plants (Gallé et al., 2015; Akula and Mukherjee, 2020). One option to prioritise future research efforts would be to focus on plant-pharmaceutical combinations deemed most at risk. An evaluation of potential similarities between the pharmaceutical mode of action in the intended species and the unintended effect on plants offers a means to prioritise and direct future efforts. It is important to note that despite this section being broken down based on different biochemical pathways and signal molecules in plants, there is a strong interconnection between all pathways and an effect on a singular pathway is likely to result in a cascade of multiple effects on other biomolecular pathways, ultimately impacting on a suite of different plant processes.
Electrical, or voltage gated, signals have been studied in numerous species (Gallé et al., 2015), with the calcium ion (Ca2+) identified as acting as a signalling transducer in all eukaryotic organisms (Pirayesh et al., 2021). Voltage-gated signalling plays a key role in plants transmitting stimuli information such as touch, light, gravity and temperature and thus regulating their responses to biotic and abiotic stress (Gallé et al., 2015; Pirayesh et al., 2021). Ca2+ signalling can occur in the plant across long distances between different plant organs, through sieve tubes (Gallé et al., 2015). These cross-organ signals have been shown to influence critical plant processes such as water and nutrient uptake and follow-up steps after pollen has been received (Gallé et al., 2015). Signalling can also be intracellular across organelles to regulate growth and development process (Pirayesh et al., 2021). Any potential interaction of pharmaceuticals with Ca2+ signalling pathways could therefore affect a plant’s response to environmental stimuli, development and growth.
Ca2+ signalling is comprised of: i) a stimulus induced transient or sustained increase in the free Ca2+ concentration [Ca2+], ii) the detection of changes in [Ca2+] by Ca2+ sensor proteins, iii) a down-stream response triggered by the action of the Ca2+ sensor proteins and iv) the removal of excess free Ca2+ (Pirayesh et al., 2021). In step two, Ca2+ detection and decoding occurs by different Ca2+ sensors, of which calmodulin is the most common detector protein, and it is shared among all eukaryotic cells. There are other detector proteins, which are plant-specific (Pirayesh et al., 2021). Although some of the plant-specific proteins may be affected by mammal intended pharmaceuticals, the fact that calmodulin is an evolutionary shared protein with conserved function across eukaryotic species, raises the specific need to better understand pharmaceuticals with the potential to inhibit this protein specifically. Phenothiazines, as one of the most common antipsychotic classes of pharmaceuticals, are known calmodulin inhibitors, and are also known to be persistent to conventional wastewater treatment and as such are often detected in wastewaters which can enter the environment (El-Azazy, 2023). Antipsychotic phenothiazines such as trifluoperazine and fluphenazine may become even more widely used as they are currently being studied for their potential in cancer treatment and as an anti-retroviral (Manoharan et al., 2022; Mao et al., 2022; Westerlund et al., 2022). Therefore, they should be further studied in terms of their potential to elicit sub-lethal effects in plants.
Imidazole’s are anti-fungal agents, whose primary mode of action is through the inhibition of ergosterol (a sterol found in fungi which maintains cell integrity) synthesis; however, it has recently been identified that they can also act as antagonists to calmodulin (Breitholtz et al., 2020). In the wider ecotoxicity literature, exposure to miconazole (a model imidazole drug) resulted in calmodulin inhibition in the water flea, (D. magna) (Breitholtz et al., 2020). On the basis of the shared role of calmodulin between species, there is therefore the potential for these pharmaceuticals to also interfere with Ca2+ signalling in plants. Imidiazoles are of particular concern as, in addition to human and veterinary therapeutic use, they are also widely used in the agricultural environment as a fungicide (Anagnostopoulou, 2022), and as such further research is needed to explore the potential for imidiazole uptake and potential effects in plants.
Plants possess most of the chemistry of the neuromotoric system in mammals, i.e. neurotransmitters and neuromodulators, such as acetylcholine, biogenic amines (dopamine, noradrenaline, adrenaline, histamine), indoleamines (e.g. melatonin, serotonin), catechoalmines (norepinephrine), alpha-aminoacid (glutamate) and γ-aminobutyric acid (GABA) (Frederick and Stanwood, 2009; Gallé et al., 2015; Akula and Mukherjee, 2020). Neurotransmitters in plants play important roles in organogenesis, flowering, ion permeability, photosynthesis, circadian rhythm, reproduction, fruit ripening, photomorphogenesis and adaptation to environmental changes (Akula and Mukherjee, 2020). Classes of pharmaceuticals which inhibit or enhance neurotransmitter action in mammals such as antidepressants (e.g. serotonin and norepinephrine reuptake inhibitors) could therefore affect these key plant processes. The cross-species effect of four different neuro-active pharmaceuticals (sertraline, venlafaxine, duloxetine and fluoxetine) has already been shown on the common water flea (D. magna) neurotramsitters (Gómez-Canela et al., 2019), and the fact that these chemical pathways are conserved in plants suggests the need to better understand neuro-active pharmaceutical interacts in plants too.
Pharmaceuticals such as citalopram and escitalopram are used to treat neurological disorders in humans, and recent research has shown that they downregulate serotonin (MahmoudianDehkordi et al., 2021). Serotonin is an important biogenic amine neurotransmitter in mammals which has been associated to controlling mood, sleep, anxiety and influencing the modulating of gastrointestinal motility (Jones et al., 2020). However, the roles serotonin plays in humans are not fully understood and recent evidence suggests lower serotonin concentration or activity may not be the cause of depression as previously thought (Moncrieff et al., 2022). In plants serotonin plays a key role in relation to long distance signalling, auxin-interaction, growth and morphogenesis, regulation of root architecture, biotic and abiotic stress (Akula and Mukherjee, 2020). Citalopram has been widely identified in effluent wastewaters entering the environment globally (Ziegler et al., 2020), in different regions such as South Africa (Assress et al., 2019), Portugal (Silva et al., 2014) and Germany (Gurke et al., 2015). The persistence of citalopram in the environment as well as the fact it has already been shown to induce effects on non-target species, such as brown trout (S. trutta f. fario) (Ziegler et al., 2020), indicates it is a priority pollutant to study in relation to key serotonin regulated plant processes. Biogenic amines, also include histamines (Akula and Mukherjee, 2020). As such, the effects of anti-histamines should be further studied in plants, given they have been detected in agricultural crops (Ben Mordechay et al., 2021). Similarly, beta-blockers, which are designed to inhibit the release of adrenaline and noradrenaline in humans (Hieble, 2000) are understudied in terms of their sub-lethal effects in plants, despite the uptake and translocation of atenolol being demonstrated in agricultural crops (Beltrán et al., 2020).
Antipsychotic pharmaceuticals have been widely used since the 50’s following the synthesis of chlorpromazine to treat diseases including schizophrenia, bipolar disease, depression, autism, attention deficit hyperactivity disorder and dementia (Escudero et al., 2021; Seeman, 2021). Antipsychotic pharmaceuticals are commonly found in the environment, due to their often-unchanged excretion and incomplete removal in wastewater treatment facilities (Escudero et al., 2021). Chlorpromazine, as the oldest and still commonly used antipsychotic, is an example commonly detected in wastewater effluents and surface waters (Reichert et al., 2019; Goswami et al., 2022). The mode of action of antipsychotic pharmaceuticals is not fully elucidated (Seeman, 2021), however as a group of drugs there is current agreement that they act on multiple receptors: dopaminergic, serotoninergic, histaminergic, adrenergic, and cholinergic (Escudero et al., 2021) or by affecting neurotransmitters, such as chlorpromazine binding to dopamine receptors in the brain (Seeman, 2021).
Further shared neurotransmitters across plants and mammals, include serotonin and melatonin, which in plants have been observed to effect organogenesis, seed germination and senescence in species such as coffee (C. canephora), thale cress (A. thaliana), shameplant (M. pudica), among others (Akula and Mukherjee, 2020). Dopamine has been observed to play an important role in fertilization as well as fruit and seed development in various plant species, including crops such as bananas (Akula and Mukherjee, 2020) and dopamine, norepinephrine, and normetanephrine have been observed to vary at different developmental stages in potatoes (Szopa et al., 2001). Similarly, l-Epinephrine, l-norepinephrine, and l-isoproterenol have been shown to promote flowering in duckweed (Khurana et al., 1987). Furthermore, biogenic amines in plants have been found to be involved in growth and development as well as stress response mechanisms (Kulma and Szopa, 2007; Akula and Mukherjee, 2020). The many unknowns which remain with respect to understanding the mode of action of antipsychotic pharmaceuticals (Seeman, 2021), as well as neurotransmitters biochemical pathways in plants (Akula and Mukherjee, 2020) means it is difficult to predict the exact effects this group of pharmaceuticals may have on plants; however, it is clear that due to the important role neurotransmitters have in plant development, growth, stress response and fertilization, antipsychotic pharmaceuticals induced effects in plants is an area which merits further study.
Neuro-active pharmaceuticals also include acetylcholinesterase inhibitors such as donepezil, rivastigmine and galantamine, which are chemicals that block the enzyme which breaks down acetylcholine, enabling a build-up of acetylcholine in patients from dementia or Alzheimer’s who have reduced levels of this chemical in their brain (Mukherjee et al., 2007; Colovic et al., 2013). In fact, plants themselves contain chemicals which act as acetylcholinesterase inhibitors (Mukherjee et al., 2007). A change in the levels of acetylcholinesterase inhibitors could therefore be detrimental to plant signalling by affecting the balance in the plant or inhibiting signals which need to be transmitted for plant response to stress. Acetylcholine in plants affects tropic response, cell division, and growth, likely by interacting with phytohormones; with these interactions requiring further study (Akula and Mukherjee, 2020). For example, acetylcholine has been observed to play an important role in mitigating the effect of osmotic stress in soybean (G. max) (Braga et al., 2017). Pharmaceuticals such as donepezil, rivastigmine and galantamine, which have the potential to interfere with this pathway are therefore of particular interest. Donepezil has already been identified as taken up by plankton and bioaccumulated by carp (C. carpino) (Grabicová et al., 2020) and rivastigmine has been identified as present in the environment and causing anti-cholinesterasic to non-target species (Rocha et al., 2014; Oboh et al., 2017).
The inhibitory neurotransmitter, GABA, plays an important role in plants and mammals. In plants, GABA has been linked to Ca2+ signalling and its interactions with calmodulin, carbon and nitrogen metabolism (key for metabolism, growth and development (Huang et al., 2016)), tricarboxylic acid cycling, signalling and stress tolerance (Akula and Mukherjee, 2020). In humans, GABA plays an important role in seizure episodes and as such pharmaceuticals which interfere with this neurotransmitter have been developed and used to treat several neurological disorders (Perucca et al., 2023). Pharmaceuticals targeting GABA, and currently in use for the treatment of neurological conditions include the benzodiazepine alprazolam, the α2/3/5 subtype-selective agents darigabat and ENX-101, and the neurosteroids ETX155 and LPCN 2101. Other pharmaceuticals which are currently used for other therapeutic purposes, but which have been observed to affect GABA are the diuretic agent bumetanide and the antiparasitic ivermectin (Perucca et al., 2023). Of these pharmaceuticals, alprazolam has been identified as persistent in the environment following wastewater and sludge reuse (Estrada-Arriaga et al., 2016; Ivanová et al., 2018; Pivetta et al., 2020), and benzodiazepines in general have been identified as having high uptake potential by plants (Carter et al., 2018). However, it is important to note that in a monitoring study of agricultural soils irrigated with wastewater it was not detected in soils or crops, despite being found in wastewater (Ben Mordechay et al., 2021). Bumetanide is used to reduce extra fluid in the body (edema) caused by conditions such as heart failure, liver disease, and kidney disease but has also been reported to alter synaptic excitation–inhibition balance by potentiating the action of GABA. As bumetanide has been identified as persistent to wastewater treatment and therefore likely to enter the environment (Zuo et al., 2022) the potential plant uptake of bumetanide other pharmaceuticals designed to interact with GABA presents a wider risk to GABA mediated pathways in plants. In fact, a study with ivermectin which has been widely detected in the aquatic and soil environment (Rath et al., 2016) and is also a chemical which has been observed to affect GABA, provides early evidence of pharmaceutical induced effects in plant plants as it has already been shown to decrease the number and weight of the soybeans (G. max) and change the activities of antioxidant enzymes (Navrátilová et al., 2020).
Lastly, actin is a highly adaptable and conserved protein which acts as an neuromotor in eukaryotic organisms (Lockett et al., 2014; Gallé et al., 2015). In mammals it is a key component of the cytoskeleton and its functions include motility, cell division and cytokinesis, vesicle and organelle movement, and cell signalling (Lockett et al., 2014). Similarly in plants it is involved in cell growth, cell division, cytokinesis, and regulating movement of various molecules across cells (Diao and Huang, 2021). Currently no pharmaceuticals actively target actin, but some studies for anti-cancer drugs targeting actin as a promising way to reduce metastasis have been undertaken (Hayot et al., 2006; Lockett et al., 2014); it is important that these developments are monitored in terms of the potential environmental effects these pharmaceuticals could have on non-target species.
Plant hormones, or phytohormones, are key in regulating plant’s development and reproduction (Bennett, 2021). They are different from animal hormones in that they can be produced in all plant cells, unlike in mammals were they are produced in specialised organs (Koo et al., 2020). Plants have a highly sophisticated cross-talk between phytohormones (Koo et al., 2020) and other biochemicals to control key life processes (Akula and Mukherjee, 2020) and respond to stresses such as drought (Jogawat et al., 2021). Phytohormones can elicit different effects and act with an antagonist or synergistic action in response to a stressor (Ghorbel et al., 2021). Phytohormones such as, abscisic acid (ABA), cytokinins (CKs), auxins (IAA), gibberellins (GA), ethylene (ET), salicylic acid (SA), brassinosteroids (BRs), jasmonic acid (JA) and strigolactones are also involved in ROS-Ca2+ mediated signals (Pottosin et al., 2014). Therefore pharmaceuticals affecting the synthesis or detection of any of these biomolecules in plants can have effects on their response to environmental stimuli, development and growth.
Examples of functions associated to different phytohormones, include jasmonic acid and its derivatives (JAs) as lipid-derived signalling molecules that control many plant developmental processes. Salicylic acid mediates the phenylpropanoid pathway, which plays an important role in defence against pathogens, some insect pests and abiotic stresses (War et al., 2011). Salicylic acid in plants also plays an important role in thermogenesis, abiotic stress tolerance, DNA damage/repair, fruit yield, seed germination (Koo et al., 2020). Auxin plays an essential role in almost every aspect of plant growth and development processes, including cell division and differentiation, as well as biotic and abiotic stress responses (Koo et al., 2020). Ethylene is involved in seed germination, fruit ripening, senescence, and abscission, as well as responses to stress (e.g. flooding, high salt, and soil compaction) (Binder, 2020). ABA is a key hormone for coping with environmental stress (Ng et al., 2014), an important agricultural role in terms of changing climatic patterns. ABA also plays an important role in seed development, dormancy, germination, and water stress responses (Koo et al., 2020), it is key in tolerance to environmental stress such as water shortage, high salinity and temperature extremes (Ng et al., 2014). Cytokinins play a key role in plant cell growth and development, impeding senescence and alleviating abiotic stress damage (Liu et al., 2020).
One of the key roles of phytohormones is controlling plant flowering and reproduction. Plants have evolved to time flowering to coincide with pollinators’ life cycles in order to maximise their mating potential (Bennett, 2021). This process is regulated by the expression of the FLOWERING LOCUS T (FT) gene, resulting in the FT protein moving around the phloem to the shoot appex to promote flowering. FT gene expression is triggered by signals received by the plant based on environmental factors such as the day length, light quality, temperature and vernalisation, as well as internal factors such as the plant’s age. The hormone gibberellin also promotes flowering, independently from FT (Bennett, 2021). Interestingly, some fungi and bacteria have independently developed the ability to produce gibberellins, through convergent evolution to interact with plants as pathogens or symbionts (Hedden, 2020), however gibberellins are not produced in mammals and as such not targeted by the mode of action of a specific pharmaceutical. Given the symbiotic relationship between some plants and fungi or bacteria it would be pertinent to study antifungals and antibacterial in the agro-environment in relation to sub-lethal effects these may have by affecting phytohormone communication between plants and symbiotic fungi or bacteria.
Ways in which pharmaceuticals may interact with phytohormones and thus have a detrimental effect on plants can be identified based on similarities across chemical structures or biomolecular pathways. Jasmonates (JAs) are synthesised by the activity of lipoxygenases enzymes or alpha-dioxygenases enzymes (Ghorbel et al., 2021). Jasmonic acids are structurally similar to prostaglandins (Ghorbel et al., 2021), so prostaglandin pharmaceuticals may affect the different pathways they regulate, however these chemicals have not been widely studied in the environment. Lipoxygenases enzymes are involved in the synthesis of JAs (Ghorbel et al., 2021), these enzymes are also present in mammals (Mashima and Okuyama, 2015) and have been shown to be inhibited by NSAIDs (Gan, 2010; Shahid et al., 2021). Inhibition of JA production by NSAIDs could be one of the mode of action by which increased stress is observed in plants exposed to NSAIDs (as observed in Table 1). However, until recently it was believed NSAIDs acted by inhibiting cyclooxygenase enzymes, a recent study showed they inhibit soybean lipoxygenases (Shahid et al., 2021), which hints at how unknown modes of action of human and veterinary pharmaceuticals can have unintended consequences in the environment. JAs are implicated in plant response to biotic and abiotic stress and they are detected in almost all plants (Ghorbel et al., 2021). Jasmonates along with ethylene, are key in plant immune response to pathogens (Wasternack and Song, 2016) Jasmonate signalling has also been suggested to be involved in C:N balancing mechanism under high C:low N conditions (Huang et al., 2016). Pharmaceutical exposure studies taking plants to the flowering and reproductive stage are lacking, as well as studies looking at the effect of pharmaceutical uptake plus an added biotic or abiotic stress. Therefore, effects on plant reproductive health and stress response, regulated by JAs may go unnoticed. These may have significant knock-on effects on plant biodiversity, in particular with hedgerow plant biodiversity (which is not dependent on seed sowing).
Plant synthesis of abscisic acid has been recently better understood (Ng et al., 2014) and includes process which are specific to plants such as carotenoid synthesis (Metibemu and Ogungbe, 2022). No pharmaceutical mode of action could be identified to affect the synthesis of this plant hormone, but some of the chemicals involved in its synthesis, such as zeaxanthin are being currently studied for their antioxidant health benefits in humans (Wong et al., 2022). Similarly, cytokinin’s are being studied for their potential as human pharmaceuticals (Fathy et al., 2022). This again points at the similar biochemical pathways between humans and plants and how chemicals found or used by either can affect the other. Salicylic acid is already commonly used as a pharmaceutical, persistent to conventional wastewater treatment (Wang et al., 2021; Köktaş and Gökkuş, 2022) and found in agricultural soils and to be taken up by plants such as lettuce (Sunyer-Caldú et al., 2022).
Despite several phytohormones themselves not being shared with mammals, including auxins and gibberllins and thus not targeted by pharmaceuticals, their cross-talk with other plant biochemical pathways means their synthesis or signalling may be indirectly affected through other pathways. Therefore, as they are vital for plant development, growth, reproduction and symbiotic relationships with bacteria and fungi they should remain studied in terms of sub-lethal effects on plants from pharmaceuticals.
Reactive oxygen species (ROS) include hydroxyl radical (·OH), alkoxyl radical (RO·), superoxide anion (·O2–) and oxygen-centered non-radicals: singlet oxygen (1O2), hydrogen peroxide (H2O2) and various forms of organic and inorganic peroxides (-ROO·) (Oyinloye et al., 2015; Mittler et al., 2022) and are produced in response to stressors in plants and mammals (Oyinloye et al., 2015; Mittler et al., 2022). In mammals these include exposure to harmful chemicals and irradiation (Oyinloye et al., 2015). In plants these include excessive lighting or viruses, and they interact with other plant signalling networks, such as phytohormones or Ca2+. They can impact on many different signalling pathways at once because they do not have specific sensors, like phytohormones. As such, ROS are key in response and acclimatization of plants to extreme weather patterns (Mittler et al., 2022).
In all cells a balance exists between ROS and the cellular antioxidant capacity which prevents excessive damage of lipids, proteins, RNA, DNA and other small molecules (Oyinloye et al., 2015; Mittler et al., 2022). Oxidative stress is a common response and endpoint assessed in plants exposed to pharmaceuticals (Kummerová et al., 2016; Siemieniuk et al., 2021). Abiotic stress in plants, such as salinity and heat, results in the accumulation of ROS which can eventually lead to cellular death (de Dios Alaché, 2019; Hasanuzzaman and Fujita, 2022), this has potential negative effects for agricultural yield and in the context of climate change. Plant bioengineering has been looking for ways to increase crop’s resistance to oxidative stress in order to increase crops tolerance to stressors such as heat and salinity (Hasanuzzaman and Fujita, 2022). In mammals long term uncontrolled oxidative stress has been linked to several inflammatory diseases such as hepatitis, asthma, rheumatoid arthritis and cancer (Oyinloye et al., 2015). Methotrexate is a pharmaceutical used since the 1980’s for the treatment of rheumatoid arthritis (Cronstein and Aune, 2020) and is one of the most frequently detected antineoplastics in the environment (Wormington et al., 2020). Different pathways have been linked to its mode of action, which are linked to ROS signalling in mammal cells, the most closely linked pathway to plants is that related to nitric oxide synthase uncoupling (Cronstein and Aune, 2020; Mittler et al., 2022). Due to this conserved pathway in plants pharmaceuticals, such as methotrexate, targetting ROS-related functions in mammals may interact with plant’s ROS balance in different ways. It is important to further research to understand the remaining unknowns in the biochemical pathways of ROS production and suppression in both mammals and plants, as these will help elucidate how existing and new pharmaceuticals may affect plant responses.
It has recently been shown that the source of energy adenosine triphosphate (ATP) can also play an important role in plant signalling (Pietrowska-Borek et al., 2020). ATP is conserved in all cellular live forms (Pietrowska-Borek et al., 2020). Pharmaceuticals, including diphenhydramine, a common over‐the‐counter antihistamine, and fluoxetine, an antidepressant, have been shown to affect mitochondrial activity and therefore ATP in aquatic organisms (Yeh et al., 2017; Brew et al., 2020). Exposure to these pharmaceuticals has been linked to a reduction in total mitochondrial glutathione, suggesting reductions in ATP synthesis, metabolic capacity, and oxidative stress (Yeh et al., 2017; Brew et al., 2020). Similar effects could be observed in plants, as these biomolecular pathways are conserved, and these could affect plant energy levels as well as signalling, indicating these pharmaceuticals should be further studied in plants.
In recent years different signalling molecules have been identified in both plants and humans, namely Mitogen Activated protein Kinases (MAPKs); reactive nitrogen species (RNS); Cyclic adenosine monophosphate (cAMP) and Guanosine 3’,5’-cyclic monophosphate (cGMP). In humans these have been linked to different diseases and although current pharmaceutics are not designed to target them; there is ongoing research to develop therapies targeting these common pathways. It is therefore important to understand their role in plants and humans and how new pharmaceuticals entering health care and potentially the environment could affect plants. MAPKs in plants are key signalling molecules which are vital for immunity, response to environmental stresses, and normal growth and development (Zhang and Zhang, 2022). There are three main families of MAPKs in mammals, namely ERK, JNK and p38 family, no obvious JNK or p38 MAPK homolog have been identified in plants (Taj et al., 2010). In humans, the ERK MAPK signalling pathway is affected by several cancers and has therefore been targeted by pharmaceuticals development, however those specifically targeting ERK MAPKs are not yet approved for clinical use (Yuan et al., 2020), but these efforts should be monitored in relation to their entering the environment and potentially impact plants. Reactive nitrogen species (RNS) have been recently identified as an important plant signalling molecule, for growth, development, and senescence (Del Río, 2015), with particular importance in responding to salt stress (Saddhe et al., 2019). In humans uncontrolled RNS have been linked to diseases such as cancer (Pandey and Khan, 2021). Currently research is being carried out in the development of pharmaceuticals to treat tuberculosis (Tuyiringire et al., 2018) or in anti-cancer therapies (Pandey and Khan, 2021) which target RNS pathways in humans, again these efforts should be monitored from the perspective of these pharmaceuticals reaching the environment and having unintended effects on plants. cAMP and cGMP are signalling molecules in both mammals and plants, which are recently being further researched (Schlossmann and Schinner, 2012; Jarratt-Barnham et al., 2021; Mangmool et al., 2023). cGMP has been identified to be linked to important signalling pathways in humans related to heart failure, and as such new drugs are being developed to target this biomolecular pathway (Mangmool et al., 2023), it is important to monitor these efforts for the potential effects they could have on plants if they reach the agricultural environment.
Callose synthesis plays an important role in plant cellular defence from pathogens (Wang et al., 2021; German et al., 2023) however, knowledge gaps in terms of callose synthesis and degradation and the crosstalk between these pathways exist (German et al., 2023). Callose is not present in mammals and there are currently no pharmaceuticals which mode of action targets callose. However, callose synthesis is conserved in fungi and some novel pharmaceuticals being developed due to increase antimicrobial resistance target callose synthesis in fungi (Jujjavarapu and Dhagat, 2018). It is therefore important that researchers looking at effects of pharmaceuticals’ on plants remain updated in terms of new pharmaceuticals being introduced into the market which target callose.
In the previous sub-sections we have presented an analysis of plant biomolecular pathways which may be affected by pharmaceuticals based on their mode of action. However, this was done mostly from the perspective of an individual plant, in reality plants have complex communication systems with other plants, microorganisms and insects around them which allow them to better respond to biotic and abiotic stress (Cheng and Cheng, 2015; Sharifi and Ryu, 2021). This phenomenon is known as plant allelopathy and is based on an organism producing non-nutritive biochemicals which influence the growth, survival, development and reproduction of other organisms (microorganisms, viruses and fungi) (Cheng and Cheng, 2015). These have been divided into two categories, first wired communication involving plant to plant signalling through microbial structures or hyper-plastic plant organs and the second are termed wireless communication, involving sending a signal through the space separating plants. (Sharifi and Ryu, 2021). The biochemiclas eliciting allelopathy are known as allelochemicals and have been divided into 14 categories based on their chemical structures, for example water-soluble organic acids and straight chain alcohols (Cheng and Cheng, 2015).
In addition, phytohormones: salicylic acid, gibberellic acid, dopamine, jasmonates and ethylene, are also considered to be allelochemicals (Cheng and Cheng, 2015; Akula and Mukherjee, 2020; Wang et al., 2021). As has been discussed in Section 3.3 phytohormones can influence symbiotic and pathogenic plant-microorganism relationships. An important example is their role in mediating the symbiotic relationship with mycorrhizal fungi, with some hormones promoting mycorrhizal colonisation and growth and others hindering them (Pozo et al., 2015). Salicylic acid is known to have negative fungal penetration on root colonization steps (Pozo et al., 2015) and as a pharmaceutical found in the environment (Wychodnik et al., 2020; Wang et al., 2021; Köktaş and Gökkuş, 2022; Sunyer-Caldú et al., 2022) could negatively impact this symbiotic plant relationship. Jasmonates are also involved in the regulation of mycorrhizal symbiotic relationship (Pozo et al., 2015) and as described in section 3.4, the production of this hormones is likely affected by NSAID pharmaceuticals, their environmental presence could also affect this symbiotic relationship.
In terms of plant wireless signals, volatile organic compounds are one of the most widely researched and have been shown to play a key role in warning plants to prepare in defence against herbivorous predators, pathogens, and environmental stresses (Brilli et al., 2019). Recent studies have also shown that volatile organic compound (VOC) signalling can also play a role in areas other than defence such as potentially affecting gas exchange and nutrient assimilation in plants receiving the signal, this would influence resource allocation to primary and secondary metabolism, and ultimately affecting plant growth, reproduction, and defence (Brosset and Blande, 2022). The biochemical pathways for synthesis and reception of VOC compounds by emitting and receiver plants are plant specific (Brosset and Blande, 2022) and no common pathways could be found with mammals. However, to elicit VOC warnings, plants need to be able to detect and transmit the stressor signal, and these processes could be affected by pharmaceuticals as previously discussed. Similarly, once the VOC signal is received by a plant this triggers a cascade of biomolecular responses, using pathways reviewed in Sections 3.1-3.5 (Brosset and Blande, 2022)., which if affected by pharmaceuticals would mean that plants are not able to prepare defence mechanisms for threats other plants are sending warnings for. As such biotic and abiotic stress could have wider plant population implications than only to individual plant.
Root exudates are another plant-plant signalling mechanism which has a role in mediating kin recognition, flowering and production, in agricultural settings these processes facilitate mixed-species plantations (Wang et al., 2021). The secretion of root signals is mainly mediated by ATP-binding cassette transporters (Wang et al., 2021), another important reason why ATP-affecting pharmaceuticals such as diphenhydramine (Yeh et al., 2017; Brew et al., 2020), as detailed in section 3.5, should be monitored in terms of plant effects. These would have wider ecosystem implications as the relationship between plant root apparatus and soil microbial communities is strictly linked with soil fertility at a chemical, biochemical, and microbiological level (Vischetti et al., 2020).
Understanding of plant allelopathy has been used in agriculture since ancient times, through the use of cover crops or companion crops to improve soil microbial health, nutrient exchange or control pests and weeds (Cheng and Cheng, 2015). This points to the fact that the sub-lethal effects pharmaceuticals can have on plant biochemical pathways can have cascading effects on the wider agricultural ecosystem, which could have implications on agricultural yields, potentially by making plants more susceptible to biotic and abiotic stress.
On the whole there is a lack of studies which have documented sub-lethal effects in plants using realistic scenarios. A majority of published studies are carried out using pharmaceutical concentrations which are higher than those typically observed in the environment (i.e. mg L-1 in irrigation water or mg kg-1 in soil) and study exposure to a single chemical at a time (Benassi-Borba et al., 2021; Bigott et al., 2021; Leitão et al., 2021; Mansilla et al., 2021; Siemieniuk et al., 2021). While these studies have unveiled a suite of biomolecular pathways in which pharmaceuticals can affect plants, the importance of studying sub-lethal effects at realistic environmental concentrations and mixture scenarios remains. For example, a study using lettuce (L. sativa) and cucumber (C. sativus) seedlings found increased root length following exposure to 10 pharmaceuticals as a mixture at realistic environmental concentrations. An opposite trend was observed in other studies whereby exposure to a single pharmaceutical, resulted in decreased root length. This specific effect was attributed to either root stress leading to root growth to access nutrients or water elsewhere or hormesis (McGinnis et al., 2019).
A number of studies which have observed plant effects are carried out in a hydroponic setting (Akenga et al., 2021; Bigott et al., 2021; Leitão et al., 2021; Mansilla et al., 2021; Nikzad and Parastar, 2021; Siemieniuk et al., 2021). Hydroponics settings eliminate confounding variables such as soil sorption and thus facilitate the investigation of mechanistic ways in which pharmaceuticals have the potential to result in a plant stress response. However, soil is important component and plays a key role in determining the bioavailability of pharmaceuticals; soil studies are also more representative of a typical agricultural exposure. Incorporating soil into the study design is also important as research has shown that pharmaceuticals can indirectly affect crops by affecting other receptors in the soil ecosystem. For example, a study exposing lettuce (L. sativa) grown in Brazilian soil observed a significant decrease in soil enzyme activity following exposure to antibiotics (amoxicillin and norfloxacin at 0.1, 1, 10 mg kg-1; oxytetracycline and Neomycin at 5, 10, 20 mg kg-1) (Benassi-Borba et al., 2021). Other examples include avoidance behaviour of earthworms (E. andrei) following exposure to 50 g kg-1 of enrofloxacin and ciprofloxacin in poultry litter (Parente et al., 2021). These two examples highlight the ways in which pharmaceutical exposure could affect crop health or yield indirectly following an observed negative effect on wider soil health metrics.
It remains undetermined whether plants can recover from a stress response following exposure to a pharmaceutical. Further study is required to understand whether a plant stressed under realistic pharmaceutical exposure could recover from additional stresses such as other types of pollutants present in the agro-environment (e.g. nano plastics (Yu et al., 2022)) or increased temperatures or drought (Sun et al., 2017; Gaffney et al., 2021). In a bid to minimise potential implications of heavy metal uptake by plants, approaches to assess effects across different cultivars have been undertaken (Yang et al., 2021) and this may be an approach that could be used to identify crops which are resistant to pharmaceutical exposure, in order to maximise benefits from waste reuse and food production. Further studies are needed to explore how pharmaceutical exposure can affect plant signalling with other plants, for example in terms of early warning signalling between plants for biotic and abiotic stressors. This is very important in terms of population survival, as early warning of a stressor allows plants to prepare and better survive biotic and abiotic stress (Cheng and Cheng, 2015; Sharifi and Ryu, 2021). Similarly, pharmaceuticals have the potential to affect plant communication with other organisms such as fungi with which they have important symbiotic relationships and this warrants further investigation.
Pharmaceutical uptake and effect studies to date have focused on agriculturally relevant crops such as lettuce, cabbage, carrot, radish, maize, wheat alfalfa, tomato and cucumber (Mansilla et al., 2021). However, it is important to note that effects cannot always be generalised across species, even following exposure to the same chemical. For example, a study looking at maize (Z. mays) and tomato (Solanum lycopersicum L) found that effects on pigments form 2 mg L-1 exposure to diclofenac and naproxen were species dependent, with diclofenac exposure reducing chlorophyll (linked to energy production) and carotenoid content (linked defence mechanism against oxidative stress) in tomato leaves. Comparatively in maize (Z. mays), pigment content actually increased under the same conditions (Siemieniuk et al., 2021). This supports the need for a targeted assessment of mode of action linked effects across a wide range of agriculturally relevant crop species, for example fruit trees (Mansilla et al., 2021), cover crops such as red clover (McKenna et al., 2018), grasses and wild flowers.
Studies to date mostly assess pharmaceutical effects on endpoints at a single time-point. There is a notable lack of studies at different time points and stages in plant development, in particular the seeding and fruiting stages. A kinetic study which assessed gene expression and oxidation stress at several time points found that diclofenac and lamotrigine might act as signals or zeitgebers affecting the circadian expression of stress related genes in lettuce (L. sativa) (Bigott et al., 2021). Effects on the circadian rhythm of plants should be further studied as these could have knock-on effects in the environment by affecting the synchronisation between flowering times and pollinators life-cycles. Ultimately, effects across different stages of plant development could result in wider negative implications on agricultural yield as well as overall environmental health.
Finally, studies are usually short-term (usually ending priori to flowering) and there are no inter-generational studies. In ecotoxicology in general the importance of using multi-generation reproductive studies has been highlighted as an important aspect for future ERAs (Straub et al., 2020). The fact that effects on genes have been observed (Bigott et al., 2021) and that negative effects on reproduction, such as affecting fruiting has been observed (Knight et al., 2018) points towards the need to understand inter-generational effects, especially for plants such as fruiting trees and wild plants, which are self-reliant on seed/fruit production for the next generation or for agricultural yield.
Our review highlighted several understudied classes of pharmaceuticals which possess a mode of action with the potential to affect key plant biomolecular pathways. From section 2 we can see there are some pharmaceutical classes which have not been studied for their sub-lethal effects on plants.
These include antihyperglycemics, in particular the sulfonylurea class, steroids and opiods. In humans, sulfonylurea pharmaceuticals act by binding to the sulfonylurea receptors in pancreatic cells, leading to a closure of the voltage-dependent potassium adenosine trisphosphate (KATP), which facilitates depolarization of the cell membrane, calcium entry into the cell and insulin secretion (Silvio E., 2022). A commonly used herbicide, nicosulfuron, is also a sulfonylurea (Dimaano and Iwakami, 2021). The mode of action of this herbicide in plants is to inhibit the plant enzyme acetolactate synthase, which belongs to the group of P450 monooxygenases (P450s) and is among the major enzymes targeted by herbicides (Dimaano and Iwakami, 2021). In plants P450 enzymes are key in the biosynthesis of alkaloids, which are key chemicals for a wide range of plant functions (Nguyen and Dang, 2021). Despite these links, the effects of antihyperglycemic sulfonylurea pharmaceuticals on plants has not been widely studied. The uptake of glyburide in several edible crops following municipal biosolid application has been demonstrated (Sabourin et al., 2012), as well as the uptake of chlorpropamide in three types of sprouts (radish, buckwheat, and okra) (Zeng et al., 2022); indicating the need to further study the potential effects this exposure may result in. The mechanism of action of the nonsulfonylurea insulin secretagogues (repaglinide and nateglinide) is similar to that of sulfonylurea: interaction with voltage dependent KATP channels on beta cells (Silvio E., 2022). However, despite research also showing that repaglinide can be taken up by crops (Zeng et al., 2022), an assessment of the potential for nonsulfonylurea insulin secretagogues to result in sub-lethal plant responses remains elusive. Two other classes of antihyperglycemics remain understudied (α-glucosidase inhibitors and thiazolidinediones). α-glucosidase inhibitors act by targeting the α-glucosidase enzyme, which breaks down disaccharides and more complex carbohydrates (Silvio E., 2022). α-glucosidase can be found naturally in plants and controls polysaccharide composition (Tomasik and Horton, 2012), which indicates these pharmaceuticals may affect plant polysaccharide control. Thiazolidinediones (rosiglitazone and pioglitazone) alter the transcription of several genes that regulate carbohydrate and lipid metabolism (Silvio E., 2022), these could be similarly affected in plants. Thiazolidinediones have not been widely studied in the environment (Bain et al., 2014), so their presence in the agricultural environment or uptake to plants is not documented. It would be necessary to first determine the prevalence of these two classes of antihyperglycemics in the environment before studying their effects on plants.
Steroid hormones exist both in mammals and plants, controlling key growth and development processes (Tarkowská, 2019) and 17β-estradiol uptake and translocation has been demonstrated in wheat (T. acstivnm L.) (Chen et al., 2021), indicating a need to further study the sub-lethal effects of these pharmaceuticals in plants. Opioids, such as morphine and codeine, have been used for centuries and are derived from plants. More recently synthetic and semi-synthetic opioids have also been developed. They act by their presynaptic action to limit neurotransmitter release (Al-Onizi et al., 2023). As chemicals produced by plants and with effects on neurotransmitters (Section 3.2), these deserve further attention in terms of plant sub-lethal effects.
From Section 3 we identified biomolecular pathways shared across plants and mammals which may be affected by existing pharmaceuticals which are currently understudied. These include antipsychotic phenothiazines and anti-fungal pharmaceuticals given their intended mode of action affects calmodulin in mammals, and this protein is conserved in plants. In plants, calmodulin is the main sensor protein to Ca2+ changes (Pirayesh et al., 2021). Given Ca2+ signalling is key for transmitting environmental stimuli information across the plant to respond to biotic or abiotic stress, as well as regulating growth and development processes (Gallé et al., 2015; Pirayesh et al., 2021), the effect of antipsychotic phenothiazines and anti-fungal pharmaceuticals on calmodulin Ca2+ signalling should be studied.
Similarly pharmaceutical classes which target mammalian neurotransmitters as their mode of action, such as antihistamines (targeting histamines) (Brew et al., 2020) and benzodiazepines (targeting GABA) (Carter et al., 2018) should be further studied. This is because histamine and GABA are common neurotransmitters across mammals and plants and play key roles in plant growth and development (Section 3.2). Furthermore, benzodiazepines have been identified as having high uptake potential by plants (Carter et al., 2018) and antihistamines such as diphenhydramine are commonly detected in the agricultural environment (Ben Mordechay et al., 2021). Beta-blockers, designed to inhibit the release of adrenaline and noradrenaline (Hieble, 2000) should also be further studied and in particular atenolol, given its uptake and translocation in agricultural crops having been demonstrated (Beltrán et al., 2020). Acetylcholinesterase inhibitors such as donepezil, rivastigmine and galantamine, (Mukherjee et al., 2007; Colovic et al., 2013) should also be studied, given that in plants acetylcholine affects tropic response, cell division, and growth, likely by interacting with phytohormones (Akula and Mukherjee, 2020). In particular donepezil and rivastigmine should be further studied given their prevalence in the environment (Rocha et al., 2014; Oboh et al., 2017; Grabicová et al., 2020). Specific neuro-active pharmaceuticals which should be further studied include citalopram, chlorpromazine, ivermectin and alprazolam. Citalopram targets serotonin (MahmoudianDehkordi et al., 2021), and its widespread presence in the environment has been reported(Silva et al., 2014; Gurke et al., 2015; Assress et al., 2019; Ziegler et al., 2020). Chlorpromazine, is commonly detected in wastewater effluents and surface waters (Reichert et al., 2019; Goswami et al., 2022) and it acts by binding to dopamine receptors in the brain (Seeman, 2021). Given plants share dopamine as a neurotransmitter playing a key role in fertilization as well as fruit and seed development (Akula and Mukherjee, 2020), this pharmaceutical merits further attention. Ivermectin and alaprazolam have both been recently identified to affect GABA (Perucca et al., 2023), and given their environmental persistence should be further studied.(Estrada-Arriaga et al., 2016; Rath et al., 2016; Ivanová et al., 2018; Navrátilová et al., 2020; Pivetta et al., 2020).
Methotrexate was a pharmaceutical specifically identified for further study due to its prevalence in the environment (Wormington et al., 2020) and because its mode of action has been linked to nitric oxide synthase uncoupling, which also occurs in plants (Cronstein and Aune, 2020; Mittler et al., 2022). This pathway is linked to ROS-related functions in mammals and may interact with plant’s ROS balance in different ways. Fluoxetine was another specific pharmaceutical identified due to its environmental prevalence (Fekadu et al., 2019) and potential to affect ATP, which is conserved in all cellular live forms and plays a key signalling role in plants (Pietrowska-Borek et al., 2020). Salicylic acid is another specific pharmaceutical meriting further attention. It is both an important phytohormone regulating thermogenesis, abiotic stress tolerance, DNA damage/repair, fruit yield, seed germination (Koo et al., 2020), and a prevalent environmental pollutant which can be taken up by plants (Wychodnik et al., 2020; Wang et al., 2021; Köktaş and Gökkuş, 2022; Sunyer-Caldú et al., 2022).
It is important to keep an eye on drug development pipelines and pharmaceuticals coming to market which target shared biomolecular pathways between mammals and plants. One example is for pharmaceuticals targeting actin as anti-cancer drugs (Hayot et al., 2006; Lockett et al., 2014), given this signalling molecule in plants is involved in cell growth, cell division, cytokinesis, and regulating movement of various molecules across cells (Diao and Huang, 2021). Furthermore, there has been recent research into biomolecular pathways involving signalling molecules, which are conserved in plants and mammals, such as ERK MApK, RNS and cAMP and cGMP (Zhang and Zhang, 2022). These molecules play key plant roles such as immunity, response to environmental stresses, senescence and normal growth and development (Schlossmann and Schinner, 2012; Del Río, 2015; Saddhe et al., 2019; Jarratt-Barnham et al., 2021; Zhang and Zhang, 2022; Mangmool et al., 2023). In humans ERK MApK pathways are currently being researched as targets of anti-cancer drugs (Yuan et al., 2020). Similarly, research is being carried out to develop pharmaceuticals targeting RON formation to treat tuberculosis (Tuyiringire et al., 2018) and cancer (Pandey and Khan, 2021). Finally, cGMP biomolecular pathways are being studied as targets to treat heart failure (Mangmool et al., 2023). It is important to monitor these efforts for the potential effects they could have on plants if they reach the agricultural environment.
Given the symbiotic relationship between some plants and fungi or bacteria it would be pertinent to study antifungals and antibacterial in the agro-environment in relation to sub-lethal effects as these chemicals have the potential to affect phytohormone communication between plants and symbiotic fungi or bacteria. Mycorrhizal symbiosis aids in plant’s response to stress factors, such as increasing nutrient availability or antagonizing pathogens (Pozo et al., 2015) and bacterial symbiosis includes nitrogen-fixing (Hedden, 2020), all key processes for plant’s growth and development.
As highlighted, plant uptake and accumulation of NSAIDs has been reported to result in a range of sub-lethal plant responses (Table 1). However, studies are yet to link these reported effects to a specific mode of action-mediated response. It has been suggested that effects may result from NSAIDs affecting the biomolecular pathways in relation to jasmonate phytohormones (Shahid et al., 2021), and further research is needed to confirm this.
On the whole, there is a general lack of data in the literature documenting sub-lethal effects of pharmaceuticals in the agricultural environment. To date, research has largely centred on plant uptake of pharmaceuticals, with a focus on human health effects. However, sub-lethal effects of pharmaceuticals on plants can have detrimental consequences in terms of agricultural yield and negative effects cascading throughout the ecosystem. Addressing these knowledge gaps would help achieve a holistic understanding of pharmaceuticals as plants stressors, in the context of reaping the benefits of wastewater reuse for irrigation and the use of manures, slurries and biosolids to meet crop nutrient demands in the context of feeding a growing population under pressure from climate change.
AG: Writing – original draft. LC: Writing – review & editing.
The author(s) declare financial support was received for the research, authorship, and/or publication of this article. All authors would like to thank UK Research and Innovation (UKRI) for the funding from a Future Leaders Fellowship (grant no.MR/S032126/1).
The authors declare that the research was conducted in the absence of any commercial or financial relationships that could be construed as a potential conflict of interest.
All claims expressed in this article are solely those of the authors and do not necessarily represent those of their affiliated organizations, or those of the publisher, the editors and the reviewers. Any product that may be evaluated in this article, or claim that may be made by its manufacturer, is not guaranteed or endorsed by the publisher.
Świacka K., Maculewicz J., Kowalska D., Caban M., Smolarz K., Świeżak J. (2022). Presence of pharmaceuticals and their metabolites in wild-living aquatic organisms – Current state of knowledge. J. Hazard. Mater. 424, 127350. doi: 10.1016/j.jhazmat.2021.127350
Abbas S., Noorimotlagh Z., Ahmadi M., Rahim F. (2021). The possible oxidative stress and DNA damage induced in Diclofenac-exposed Non-target organisms in the aquatic environment: A systematic review. Ecol. Indic. 131, 108172. doi: 10.1016/j.ecolind.2021.108172
Adeel M., Song X., Wang Y., Francis D., Yang Y. (2017). Environmental impact of estrogens on human, animal and plant life: A critical review. Environ. Int. 99, 107–119. doi: 10.1016/j.envint.2016.12.010
Aderemi A. O., Novais S. C., Lemos M. F. L., Alves L. M., Hunter C., Pahl O. (2018). Oxidative stress responses and cellular energy allocation changes in microalgae following exposure to widely used human antibiotics. Aquat. Toxicol. 203, 130–139. doi: 10.1016/j.aquatox.2018.08.008
Akenga P., Gachanja A., Fitzsimons M. F., Tappin A., Comber S. (2021). Uptake, accumulation and impact of antiretroviral and antiviral pharmaceutical compounds in lettuce. Sci. Total Environ. 766, 144499. doi: 10.1016/j.scitotenv.2020.144499
Akula R., Mukherjee S. (2020). New insights on neurotransmitters signaling mechanisms in plants. Plant Signal. Behav. 15, 1737450. doi: 10.1080/15592324.2020.1737450
Al-Onizi A. D., Abu-hasabu A., Al-Hwaiti D. N., Al-Ali R. M., Al-Madani N. M., El Rabey H. A. (2023). General review on opioids. J. Biomed. Res. Environ. Sci. 4, 1046–1056. doi: 10.37871/jbres1767
Ambrosio-Albuquerque E. P., Cusioli L. F., Bergamasco R., Sinópolis Gigliolli A. A., Lupepsa L., Paupitz B. R., et al. (2021). Metformin environmental exposure: A systematic review. Environ. Toxicol. Pharmacol. 83, 103588. doi: 10.1016/j.etap.2021.103588
Anagnostopoulou K. (2022). Overarching issues on relevant pesticide transformation products in the aquatic environment: A review. Sci. Total Environ. 815, 152863. doi: 10.1016/j.scitotenv.2021.152863
Aristilde L., Melis A., Sposito G. (2010). Inhibition of photosynthesis by a fluoroquinolone antibiotic. Environ. Sci. Technol. 44, 1444–1450. doi: 10.1021/es902665n
Assress H. A., Nyoni H., Mamba B. B., Msagati T. A. M. (2019). Target quantification of azole antifungals and retrospective screening of other emerging pollutants in wastewater effluent using UHPLC –QTOF-MS. Environ. pollut. 253, 655–666. doi: 10.1016/j.envpol.2019.07.075
Azanu D., Mortey C., Darko G., Weisser J. J., Styrishave B., Abaidoo R. C. (2016). Uptake of antibiotics from irrigation water by plants. Chemosphere 157, 107–114. doi: 10.1016/j.chemosphere.2016.05.035
Bain P. A., Williams M., Kumar A. (2014). Assessment of multiple hormonal activities in wastewater at different stages of treatment: Changes in endocrine potency during wastewater treatment. Environ. Toxicol. Chem. 33, 2297–2307. doi: 10.1002/etc.2676
Batchelder A. R. (1981). Chlortetracycline and oxytetracycline effects on plant growth and development in liquid cultures. J. Environ. Qual. 10, 515–518. doi: 10.2134/jeq1981.00472425001000040019x
Beltrán E. M., Pablos M. V., Fernández Torija C., Porcel M.Á., González-Doncel M. (2020). Uptake of atenolol, carbamazepine and triclosan by crops irrigated with reclaimed water in a Mediterranean scenario. Ecotoxicol. Environ. Saf. 191, 110171. doi: 10.1016/j.ecoenv.2020.110171
Benassi-Borba L., Dal’Lin C. M. P., Testolin R. C., Batista Vieira N. M., Tagliari Corrêa C. V., Bianchi I., et al. (2021). Assessment of phytotoxicity and impact on the enzymatic activity of soil microorganisms caused by veterinary antibiotics used in Brazilian farms. J. Environ. Sci. Health - Part B Pestic. Food Contam. Agric. Wastes 56, 675–684. doi: 10.1080/03601234.2021.1938480
Ben Mordechay E., Mordehay V., Tarchitzky J., Chefetz B. (2021). Pharmaceuticals in edible crops irrigated with reclaimed wastewater: Evidence from a large survey in Israel. J. Hazard. Mater. 416, 126184. doi: 10.1016/j.jhazmat.2021.126184
Bennett T. (2021). The other sexual revolution: hormonal control of family planning in plants. Biochemist 43, 20–24. doi: 10.1042/bio_2021_133
Bigott Y., Chowdhury S. P., Pérez S., Montemurro N., Manasfi R., Schröder P. (2021). Effect of the pharmaceuticals diclofenac and lamotrigine on stress responses and stress gene expression in lettuce (Lactuca sativa) at environmentally relevant concentrations. J. Hazard. Mater. 403, 123881. doi: 10.1016/j.jhazmat.2020.123881
Binder B. M. (2020). Ethylene signaling in plants. J. Biol. Chem. 295, 7710–7725. doi: 10.1074/jbc.REV120.010854
Bourdat-Deschamps M., Ferhi S., Bernet N., Feder F., Crouzet O., Patureau D., et al. (2017). Fate and impacts of pharmaceuticals and personal care products after repeated applications of organic waste products in long-term field experiments. Sci. Total Environ. 607–608, 271–280. doi: 10.1016/j.scitotenv.2017.06.240
Braga I., Pissolato M. D., Souza G. M. (2017). Mitigating effects of acetylcholine supply on soybean seed germination under osmotic stress. Braz. J. Bot. 40, 617–624. doi: 10.1007/s40415-017-0367-2
Branchet P., Arpin-Pont L., Piram A., Boissery P., Wong-Wah-Chung P., Doumenq P. (2021). Pharmaceuticals in the marine environment: What are the present challenges in their monitoring? Sci. Total Environ. 766, 142644. doi: 10.1016/j.scitotenv.2020.142644
Breitholtz M., Ivanov P., Ek K., Gorokhova E. (2020). Calmodulin inhibition as a mode of action of antifungal imidazole pharmaceuticals in non-target organisms. Toxicol. Res. 9, 425–430. doi: 10.1093/toxres/tfaa039
Brew D. W., Black M. C., Santos M., Rodgers J., Henderson W. M. (2020). Metabolomic investigations of the temporal effects of exposure to pharmaceuticals and personal care products and their mixture in the eastern oyster ( Crassostrea virginica ). Environ. Toxicol. Chem. 39, 419–436. doi: 10.1002/etc.4627
Brilli F., Loreto F., Baccelli I. (2019). Exploiting plant volatile organic compounds (VOCs) in agriculture to improve sustainable defense strategies and productivity of crops. Front. Plant Sci. 10. doi: 10.3389/fpls.2019.00264
Brosset A., Blande J. D. (2022). Volatile-mediated plant–plant interactions: volatile organic compounds as modulators of receiver plant defence, growth, and reproduction. J. Exp. Bot. 73, 511–528. doi: 10.1093/jxb/erab487
Bush K., Bradford P. A. (2016). β-lactams and β-lactamase inhibitors: an overview. Cold Spring Harb. Perspect. Med. 6, a025247. doi: 10.1101/cshperspect.a025247
Carter L. J., Chefetz B., Abdeen Z., Boxall A. B. A. (2019). Emerging investigator series: Towards a framework for establishing the impacts of pharmaceuticals in wastewater irrigation systems on agro-ecosystems and human health. Environ. Sci. Process. Impacts 21, 605–622. doi: 10.1039/c9em00020h
Carter L. J., Harris E., Williams M., Ryan J. J., Kookana R. S., Boxall A. B. A. (2014). Fate and uptake of pharmaceuticals in soil-plant systems. J. Agric. Food Chem. 62, 816–825. doi: 10.1021/jf404282y
Carter L. J., Williams M., Böttcher C., Kookana R. S. (2015). Uptake of pharmaceuticals influences plant development and affects nutrient and hormone homeostases. Environ. Sci. Technol. 49, 12509–12518. doi: 10.1021/acs.est.5b03468
Carter L. J., Williams M., Martin S., Kamaludeen S. P. B., Kookana R. S. (2018). Sorption, plant uptake and metabolism of benzodiazepines. Sci. Total Environ. 628–629, 18–25. doi: 10.1016/j.scitotenv.2018.01.337
Chen X., Li Y., Jiang L., Hu B., Wang L., An S., et al. (2021). Uptake, accumulation, and translocation mechanisms of steroid estrogens in plants. Sci. Total Environ. 753, 141979. doi: 10.1016/j.scitotenv.2020.141979
Cheng F., Cheng Z. (2015). Research progress on the use of plant allelopathy in agriculture and the physiological and ecological mechanisms of allelopathy. Front. Plant Sci. 6. doi: 10.3389/fpls.2015.01020
Cheong M. S., Seo K. H., Chohra H., Yoon Y. E., Choe H., Kantharaj V., et al. (2020). Influence of sulfonamide contamination derived from veterinary antibiotics on plant growth and development. Antibiotics 9, 456. doi: 10.3390/antibiotics9080456
Christou A., Papadavid G., Dalias P., Fotopoulos V., Michael C., Bayona J. M., et al. (2019). Ranking of crop plants according to their potential to uptake and accumulate contaminants of emerging concern. Environ. Res. 170, 422–432. doi: 10.1016/J.ENVRES.2018.12.048
Colovic M. B., Krstic D. Z., Lazarevic-Pasti T. D., Bondzic A. M., Vasic V. M. (2013). Acetylcholinesterase inhibitors: pharmacology and toxicology. Curr. Neuropharmacol. 11, 315–335. doi: 10.2174/1570159X11311030006
Cronstein B. N., Aune T. M. (2020). Methotrexate and its mechanisms of action in inflammatory arthritis. Nat. Rev. Rheumatol. 16, 145–154. doi: 10.1038/s41584-020-0373-9
Cui H., Hense B. A., Müller J., Schröder P. (2015). Short term uptake and transport process for metformin in roots of Phragmites australis and Typha latifolia. Chemosphere 134, 307–312. doi: 10.1016/j.chemosphere.2015.04.072
Daughton C. G. (2003). Cradle-to-cradle stewardship of drugs for minimizing their environmental disposition while promoting human health. I. Rationale for and avenues toward a green pharmacy. Environ. Health Perspect. 111, 757–774. doi: 10.1289/ehp.5947
de Dios Alaché J. (2019). A concise appraisal of lipid oxidation and lipoxidation in higher plants. Redox Biol. 23, 101136. doi: 10.1016/j.redox.2019.101136
Del Río L. A. (2015). ROS and RNS in plant physiology: an overview. J. Exp. Bot. 66, 2827–2837. doi: 10.1093/jxb/erv099
Diao M., Huang S. (2021). An update on the role of the actin cytoskeleton in plasmodesmata: A focus on formins. Front. Plant Sci. 12. doi: 10.3389/fpls.2021.647123
Dimaano N. G., Iwakami S. (2021). Cytochrome P450mediated herbicide metabolism in plants: current understanding and prospects. Pest Manage. Sci. 77, 22–32. doi: 10.1002/ps.6040
Dordio A. V., Belo M., Martins Teixeira D., Palace Carvalho A. J., Dias C. M. B., Picó Y., et al. (2011). Evaluation of carbamazepine uptake and metabolization by Typha spp., a plant with potential use in phytotreatment. Bioresour. Technol. 102, 7827–7834. doi: 10.1016/j.biortech.2011.06.050
Drechsel P., Qadir M., Baumann J. (2022). Water reuse to free up freshwater for higher-value use and increase climate resilience and water productivity. Irrig. Drain. 71, 100–109. doi: 10.1002/ird.2694
Ebner J. N. (2021). Trends in the application of “Omics” to ecotoxicology and stress ecology. Genes 12, 1481. doi: 10.3390/genes12101481
El-Azazy M. (2023). Competitive adsorptive removal of promazine and promethazine from wastewater using olive tree pruning biochar: operational parameters, kinetics, and equilibrium investigations. Environ. Sci. pollut. Res. 30, 82387–82405. doi: 10.1007/s11356-023-27688-6
Escudero J., Muñoz J. L., Morera-Herreras T., Hernandez R., Medrano J., Domingo-Echaburu S., et al. (2021). Antipsychotics as environmental pollutants: An underrated threat? Sci. Total Environ. 769, 144634. doi: 10.1016/j.scitotenv.2020.144634
Estrada-Arriaga E. B., Cortés-Muñoz J. E., González-Herrera A., Calderón-Mólgora C. G., de Lourdes Rivera-Huerta M., Ramírez-Camperos E., et al. (2016). Assessment of full-scale biological nutrient removal systems upgraded with physico-chemical processes for the removal of emerging pollutants present in wastewaters from Mexico. Sci. Total Environ. 571, 1172–1182. doi: 10.1016/j.scitotenv.2016.07.118
Fathy M., Saad Eldin S. M., Naseem M., Dandekar T., Othman E. M. (2022). Cytokinins: wide-spread signaling hormones from plants to humans with high medical potential. Nutrients 14, 1495. doi: 10.3390/nu14071495
Favre P., Agosti R. D. (2007). Voltage-dependent action potentials in Arabidopsis thaliana. Physiol. Plant 131, 070719024921004. doi: 10.1111/j.1399-3054.2007.00954.x
Fekadu S., Alemayehu E., Dewil R., van der Bruggen B. (2019). Pharmaceuticals in freshwater aquatic environments: A comparison of the African and European challenge. Sci. Total Environ. 654, 324–337. doi: 10.1016/J.SCITOTENV.2018.11.072
Felle H. H., Zimmermann M. R. (2007). Systemic signalling in barley through action potentials. Planta 226, 203. doi: 10.1007/s00425-006-0458-y
Frederick A. L., Stanwood G. D. (2009). Drugs, biogenic amine targets and the developing brain. Dev. Neurosci. 31, 7–22. doi: 10.1159/000207490
Fu Q., Malchi T., Carter L. J., Li H., Gan J., Chefetz B. (2019). Pharmaceutical an personal care proucts: from wastewater treatment into agro-foo systems. Environ. Sci. Technol. 53, 14083–14090. doi: 10.1021/acs.est.9b06206
Gaffney I., Sallach J. B., Wilson J., Bergström E., Thomas-Oates J. (2021). Metabolomic approaches to studying the response to drought stress in corn (Zea mays) cobs. Metabolites 11, 438. doi: 10.3390/metabo11070438
Gallé A., Lautner S., Flexas J., Fromm J. (2015). Environmental stimuli and physiological responses: The current view on electrical signalling. Environ. Exp. Bot. 114, 15–21. doi: 10.1016/j.envexpbot.2014.06.013
Gan T. J. (2010). Diclofenac: an update on its mechanism of action and safety profile. Curr. Med. Res. Opin. 26, 1715–1731. doi: 10.1185/03007995.2010.486301
García-Valverde M., Aragonés A. M., Andújar J. A. S., García M. D. G., Martínez-Bueno M. J., Fernández-Alba A. R. (2023). Long-term effects on the agroecosystem of using reclaimed water on commercial crops. Sci. Total Environ. 859, 160462. doi: 10.1016/j.scitotenv.2022.160462
Garduño-Jiménez A.-L., Durán-Álvarez J.-C., Ortori C. A., Abdelrazig S., Barrett D. A., Gomes R. L. (2023). Delivering on sustainable development goals in wastewater reuse for agriculture: Initial prioritization of emerging pollutants in the Tula Valley, Mexico. Water Res. 238, 119903. doi: 10.1016/j.watres.2023.119903
Gaw S., Thomas K. V., Hutchinson T. H. (2014). Sources, impacts and trends of pharmaceuticals in the marine and coastal environment. Philos. Trans. R. Soc B Biol. Sci. 369, 20130572. doi: 10.1098/rstb.2013.0572
German L., Yeshvekar R., Benitez-Alfonso Y. (2023). Callose metabolism and the regulation of cell walls and plasmodesmata during plant mutualistic and pathogenic interactions. Plant Cell Environ. 46, 391–404. doi: 10.1111/pce.14510
Ghorbel M., Brini F., Sharma A., Landi M. (2021). Role of jasmonic acid in plants: the molecular point of view. Plant Cell Rep. 40, 1471–1494. doi: 10.1007/s00299-021-02687-4
Gomes M. P., Gonçalves C. A., De Brito J. C. M., Souza A. M., Da Silva Cruz F. V., Bicalho E. M., et al. (2017). Ciprofloxacin induces oxidative stress in duckweed (Lemna minor L.): Implications for energy metabolism and antibiotic-uptake ability. J. Hazard. Mater. 328, 140–149. doi: 10.1016/j.jhazmat.2017.01.005
Gomes M. P., Rocha D. C., Moreira De Brito J. C., Tavares D. S., Marques R. Z., Soffiatti P., et al. (2020). Emerging contaminants in water used for maize irrigation: Economic and food safety losses associated with ciprofloxacin and glyphosate. Ecotoxicol. Environ. Saf. 196, 110549. doi: 10.1016/j.ecoenv.2020.110549
Gómez-Canela C., Rovira García X., Martínez-Jerónimo F., Marcé R. M., Barata C. (2019). Analysis of neurotransmitters in Daphnia magna affected by neuroactive pharmaceuticals using liquid chromatography-high resolution mass spectrometry. Environ. pollut. 254, 113029. doi: 10.1016/j.envpol.2019.113029
Goswami P., Guruge K. S., Tanoue R., Tamamura Y. A., Jinadasa K. B. S. N., Nomiyama K., et al. (2022). Occurrence of pharmaceutically active compounds and potential ecological risks in wastewater from hospitals and receiving waters in Sri Lanka. Environ. Toxicol. Chem. 41, 298–311. doi: 10.1002/etc.5212
Grabicová K., Grabic R., Fedorova G., Vojs Staňová A., Bláha M., Randák T., et al. (2020). Water reuse and aquaculture: Pharmaceutical bioaccumulation by fish during tertiary treatment in a wastewater stabilization pond. Environ. pollut. 267, 115593. doi: 10.1016/j.envpol.2020.115593
Guo A., Pan C., Su X., Zhou X., Bao Y. (2022). Combined effects of oxytetracycline and microplastic on wheat seedling growth and associated rhizosphere bacterial communities and soil metabolite profiles. Environ. pollut. 302, 119046. doi: 10.1016/j.envpol.2022.119046
Gurke R., Rößler M., Marx C., Diamond S., Schubert S., Oertel R., et al. (2015). Occurrence and removal of frequently prescribed pharmaceuticals and corresponding metabolites in wastewater of a sewage treatment plant. Sci. Total Environ. 532, 762–770. doi: 10.1016/j.scitotenv.2015.06.067
Gworek B., Kijeńska M., Wrzosek J., Graniewska M. (2021). Pharmaceuticals in the soil and plant environment: a review. Water. Air. Soil pollut. 232, 145–162. doi: 10.1007/s11270-020-04954-8
Hasanuzzaman M., Fujita M. (2022). Plant oxidative stress: biology, physiology and mitigation. Plants 11, 1185. doi: 10.3390/plants11091185
Hayot C., Debeir O., Van Ham P., Van Damme M., Kiss R., Decaestecker C. (2006). Characterization of the activities of actin-affecting drugs on tumor cell migration. Toxicol. Appl. Pharmacol. 211, 30–40. doi: 10.1016/j.taap.2005.06.006
Hedden P. (2020). The current status of research on gibberellin biosynthesis. Plant Cell Physiol. 61, 1832–1849. doi: 10.1093/pcp/pcaa092
Helmecke M., Fries E., Schulte C. (2020). Regulating water reuse for agricultural irrigation: risks related to organic micro-contaminants. Environ. Sci. Eur. 32, 4. doi: 10.1186/s12302-019-0283-0
Hieble J. P. (2000). Adrenoceptor subclassification: an approach to improved cardiovascular therapeutics. Pharmaceutica Acta Helvetiae 74, 163–171. doi: 10.1016/S0165-7208(00)80014-8
Hillis D. G., Fletcher J., Solomon K. R., Sibley P. K. (2011). Effects of ten antibiotics on seed germination and root elongation in three plant species. Arch. Environ. Contam. Toxicol. 60, 220–232. doi: 10.1007/s00244-010-9624-0
Huang A., Sang Y., Sun W., Fu Y., Yang Z. (2016). Transcriptomic analysis of responses to imbalanced carbon: nitrogen availabilities in rice seedlings. PloS One 11, e0165732. doi: 10.1371/journal.pone.0165732
Ivanová L., Mackuľak T., Grabic R., Golovko O., Koba O., Staňová A. V., et al. (2018). Pharmaceuticals and illicit drugs – A new threat to the application of sewage sludge in agriculture. Sci. Total Environ. 634, 606–615. doi: 10.1016/j.scitotenv.2018.04.001
Jamla M., Khare T., Joshi S., Patil S., Penna S., Kumar V. (2021). Omics approaches for understanding heavy metal responses and tolerance in plants. Curr. Plant Biol. 27, 100213. doi: 10.1016/j.cpb.2021.100213
Jarratt-Barnham E., Wang L., Ning Y., Davies J. M. (2021). The complex story of plant cyclic nucleotide-gated channels. Int. J. Mol. Sci. 22, 874. doi: 10.3390/ijms22020874
Jimenez-Cisneros B. (2006). Irrigation in developing countries using wastewater. IGES Int. Rev. Environ. Strateg. 6, 229–250.
Jin J., Xu L., Zhang S., Jin M., Zhang P., Shen L., et al. (2023). Oxidative response of rice (Oryza sativa L.) seedlings to quinolone antibiotics and its correlation with phyllosphere microbes and antibiotic resistance genes. Sci. Total Environ. 867, 161544. doi: 10.1016/j.scitotenv.2023.161544
Jogawat A., Yadav B., Lakra N., Singh A. K., Narayan O. P. (2021). Crosstalk between phytohormones and secondary metabolites in the drought stress tolerance of crop plants: A review. Physiol. Plant 172, 1106–1132. doi: 10.1111/ppl.13328
Jones L. A., Sun E. W., Martin A. M., Keating D. J. (2020). The ever-changing roles of serotonin. Int. J. Biochem. Cell Biol. 125, 105776. doi: 10.1016/j.biocel.2020.105776
Jujjavarapu S. E., Dhagat S. (2018). In silico discovery of novel ligands for antimicrobial lipopeptides for computer-aided drug design. Probiotics Antimicrob. Proteins 10, 129–141. doi: 10.1007/s12602-017-9356-9
Kaufman G. (2011). Antibiotics: mode of action and mechanisms of resistance. Nurs. Stand. 25, 49–55. doi: 10.7748/ns.25.42.49.s52
Keerthanan S., Jayasinghe C., Biswas J. K., Vithanage M. (2021). Pharmaceutical and Personal Care Products (PPCPs) in the environment: Plant uptake, translocation, bioaccumulation, and human health risks. Crit. Rev. Environ. Sci. Technol. 51, 1221–1258. doi: 10.1080/10643389.2020.1753634
Khan H. K., Rehman M. Y. A., Malik R. N. (2020). Fate and toxicity of pharmaceuticals in water environment: An insight on their occurrence in South Asia. J. Environ. Manage. 271, 111030. doi: 10.1016/j.jenvman.2020.111030
Khurana J. P., Tamot B. K., Maheshwari N., Maheshwari S. C. (1987). Role of catecholamines in promotion of flowering in a short-day duckweed, lemna paucicostata 6746. Plant Physiol. 85, 10–12. doi: 10.1104/pp.85.1.10
Klampfl C. W. (2019). Metabolization of pharmaceuticals by plants after uptake from water and soil: A review. TrAC - Trends Anal. Chem. 111, 13–26. doi: 10.1016/j.trac.2018.11.042
Knight E., Carter L. J., McLaughlin M. J. (2017). Bioaccumulation, uptake, and toxicity of carbamazepine in soil–plant systems. Environ. Toxicol. Chem. 37, 1122–1130. doi: 10.1002/etc.4053
Knight E. R., Carter L. J., McLaughlin M. J. (2018). Sorption, plant uptake and metabolism of benzodiazepines. Environ. Toxicol. Chem. 37, 1122–1130. doi: 10.1002/etc.4053
Kodesova R., Klement A., Golovko O., Fer M., Nikodem A., Kocarek M., et al. (2019). Root uptake of atenolol, sulfamethoxazole and carbamazepine, and their transformation in three soils and four plants. Environ. Sci. pollut. Res. 26, 9876–9891. doi: 10.1007/s11356-019-04333-9
Köktaş İ.Y., Gökkuş Ö. (2022). Removal of salicylic acid by electrochemical processes using stainless steel and platinum anodes. Chemosphere 293, 133566. doi: 10.1016/j.chemosphere.2022.133566
Koo Y. M., Heo A. Y., Choi H. W. (2020). Salicylic acid as a safe plant protector and growth regulator. Plant Pathol. J. 36, 1–10. doi: 10.5423/PPJ.RW.12.2019.0295
Krupka M., Piotrowicz-Cieślak A. I., Michalczyk D. J. (2022). Effects of antibiotics on the photosynthetic apparatus of plants. J. Plant Interact. 17, 96–104. doi: 10.1080/17429145.2021.2014579
Kulma A., Szopa J. (2007). Catecholamines are active compounds in plants. Plant Sci. 172, 433–440. doi: 10.1016/j.plantsci.2006.10.013
Kummerová M., Zezulka Š., Babula P., Tříska J. (2016). Possible ecological risk of two pharmaceuticals diclofenac and paracetamol demonstrated on a model plant Lemna minor. J. Hazard. Mater. 302, 351–361. doi: 10.1016/j.jhazmat.2015.09.057
Leitão I., Leclercq C. C., Ribeiro D. M., Renaut J., Almeida A. M., Martins L. L., et al. (2021). Stress response of lettuce (Lactuca sativa) to environmental contamination with selected pharmaceuticals: A proteomic study. J. Proteomics 245, 45920–45932. doi: 10.1016/j.jprot.2021.104291
Liu Y., Lu S., Liu K., Wang S., Huang L., Guo L. (2019). Proteomics: a powerful tool to study plant responses to biotic stress. Plant Methods 15, 135. doi: 10.1186/s13007-019-0515-8
Liu X., Lv Y., Xu K., Xiao X., Xi B., Lu S. (2018). Response of ginger growth to a tetracycline-contaminated environment and residues of antibiotic and antibiotic resistance genes. Chemosphere 201, 137–143. doi: 10.1016/j.chemosphere.2018.02.178
Liu Y., Zhang M., Meng Z., Wang B., Chen M. (2020). Research progress on the roles of cytokinin in plant response to stress. Int. J. Mol. Sci. 21, 6574. doi: 10.3390/ijms21186574
Lockett S., Verma C., Brafman A., Gudla P., Nandy K., Mimaki Y., et al. (2014). Quantitative analysis of F-actin redistribution in astrocytoma cells treated with candidate pharmaceuticals: Quantifying F-Actin Redistribution in Cells. Cytometry A 85, 512–521. doi: 10.1002/cyto.a.22442
Madikizela L. M., Botha T. L., Kamika I., Msagati T. A. M. (2022). Uptake, occurrence, and effects of nonsteroidal anti-inflammatory drugs and analgesics in plants and edible crops. J. Agric. Food Chem. 70, 34–45. doi: 10.1021/acs.jafc.1c06499
Madikizela L. M., Tavengwa N. T., Chimuka L. (2017). Status of pharmaceuticals in African water bodies: Occurrence, removal and analytical methods. J. Environ. Manage. 193, 211–220. doi: 10.1016/J.JENVMAN.2017.02.022
MahmoudianDehkordi S., Ahmed A. T., Bhattacharyya S., Han X., Baillie R. A., Arnold M., et al. (2021). Alterations in acylcarnitines, amines, and lipids inform about the mechanism of action of citalopram/escitalopram in major depression. Transl. Psychiatry 11, 153. doi: 10.1038/s41398-020-01097-6
Mangmool S., Duangrat R., Parichatikanond W., Kurose H. (2023). New therapeutics for heart failure: focusing on cGMP signaling. Int. J. Mol. Sci. 24, 12866. doi: 10.3390/ijms241612866
Manoharan G. B., Okutachi S., Abankwa D. (2022). Potential of phenothiazines to synergistically block calmodulin and reactivate PP2A in cancer cells. PloS One 17, e0268635. doi: 10.1371/journal.pone.0268635
Mansilla S., Portugal J., Bayona J. M., Matamoros V., Leiva A. M., Vidal G., et al. (2021). Compounds of emerging concern as new plant stressors linked to water reuse and biosolid application in agriculture. J. Environ. Chem. Eng. 9, 105198. doi: 10.1016/j.jece.2021.105198
Mao Y., Wang Z., Yao C., Zeng Q., Cheng W., Zhang S., et al. (2022). The Food and Drug Administration-approved antipsychotic drug trifluoperazine, a calmodulin antagonist, inhibits viral replication through PERK-eIF2α axis. Front. Microbiol. 13. doi: 10.3389/fmicb.2022.979904
Margas M., Piotrowicz-Cieślak A. I., Michalczyk D. J., Głowacka K. (2019). A strong impact of soil tetracycline on physiology and biochemistry of pea seedlings. Scientifica 2019, 1–14. doi: 10.1155/2019/3164706
Mashima R., Okuyama T. (2015). The role of lipoxygenases in pathophysiology; new insights and future perspectives. Redox Biol. 6, 297–310. doi: 10.1016/j.redox.2015.08.006
Matich E. K., Chavez Soria N. G., Aga D. S., Atilla-Gokcumen G. E. (2019). Applications of metabolomics in assessing ecological effects of emerging contaminants and pollutants on plants. J. Hazard. Mater. 373, 527–535. doi: 10.1016/J.JHAZMAT.2019.02.084
Mayer-Pinto M., Ledet J., Crowe T. P., Johnston E. L. (2020). Sublethal effects of contaminants on marine habitat-forming species: a review and meta-analysis. Biol. Rev. 95, 1554–1573. doi: 10.1111/brv.12630
McGinnis M., Sun C., Dudley S., Gan J. (2019). Effect of low-dose, repeated exposure of contaminants of emerging concern on plant development and hormone homeostasis. Environ. pollut. 252, 706–714. doi: 10.1016/j.envpol.2019.05.159
McKenna P., Cannon N., Conway J., Dooley J. (2018). The use of red clover (Trifolium pratense) in soil fertility-building: A Review. Field Crops Res. 221, 38–49. doi: 10.1016/j.fcr.2018.02.006
Metibemu D. S., Ogungbe I. V. (2022). Carotenoids in drug discovery and medicine: pathways and molecular targets implicated in human diseases. Molecules 27, 6005. doi: 10.3390/molecules27186005
Mezzelani M., Gorbi S., Regoli F. (2018). Pharmaceuticals in the aquatic environments: Evidence of emerged threat and future challenges for marine organisms. Mar. Environ. Res. 140, 41–60. doi: 10.1016/j.marenvres.2018.05.001
Mittler R., Zandalinas S. I., Fichman Y., Van Breusegem F. (2022). Reactive oxygen species signalling in plant stress responses. Nat. Rev. Mol. Cell Biol. 23, 663–679. doi: 10.1038/s41580-022-00499-2
Mo J., Guo J., Iwata H., Diamond J., Qu C., Xiong J., et al. (2022). What approaches should be used to prioritize pharmaceuticals and personal care products for research on environmental and human health exposure and effects? Environ. Toxicol. Chem., etc.5520. doi: 10.1002/etc.5520
Moncrieff J., Cooper R. E., Stockmann T., Amendola S., Hengartner M. P., Horowitz M. A. (2022). The serotonin theory of depression: a systematic umbrella review of the evidence. Mol. Psychiatry 28, 3243–3256. doi: 10.1038/s41380-022-01661-0
Mukherjee P. K., Kumar V., Mal M., Houghton P. J. (2007). Acetylcholinesterase inhibitors from plants. Phytomedicine 14, 289–300. doi: 10.1016/j.phymed.2007.02.002
Navrátilová M., Stuchlíková L. R., Moťková K., Szotáková B., Skálová L., Langhansová L., et al. (2020). The uptake of ivermectin and its effects in roots, leaves and seeds of soybean (Glycine max). Molecules 25, 3655. doi: 10.3390/molecules25163655
Ng L. M., Melcher K., Teh B. T., Xu H. E. (2014). Abscisic acid perception and signaling: structural mechanisms and applications. Acta Pharmacol. Sin. 35, 567–584. doi: 10.1038/aps.2014.5
Nguyen T.-D., Dang T.-T. T. (2021). Cytochrome P450 enzymes as key drivers of alkaloid chemical diversification in plants. Front. Plant Sci. 12. doi: 10.3389/fpls.2021.682181
Nikzad N., Parastar H. (2021). Evaluation of the effect of organic pollutants exposure on the antioxidant activity, total phenolic and total flavonoid content of lettuce (Lactuca sativa L.) using UV–Vis spectrophotometry and chemometrics. Microchem. J. 170, 106632. doi: 10.1016/j.microc.2021.106632
Oboh G., Ogunsuyi O. B., Olonisola O. E. (2017). Does caffeine influence the anticholinesterase and antioxidant properties of donepezil? Evidence Vitro Vivo Stud. Metab. Brain Dis. 32, 629–639. doi: 10.1007/s11011-017-9951-1
Opriş O., Copaciu F., Loredana Soran M., Ristoiu D., Niinemets Ü., Copolovici L. (2013). Influence of nine antibiotics on key secondary metabolites and physiological characteristics in Triticum aestivum: Leaf volatiles as a promising new tool to assess toxicity. Ecotoxicol. Environ. Saf. 87, 70–79. doi: 10.1016/j.ecoenv.2012.09.019
Oyinloye B., Adenowo A., Kappo A. (2015). Reactive oxygen species, apoptosis, antimicrobial peptides and human inflammatory diseases. Pharmaceuticals 8, 151–175. doi: 10.3390/ph8020151
Pandey P., Khan F. (2021). A mechanistic review of the anticancer potential of hesperidin, a natural flavonoid from citrus fruits. Nutr. Res. 92, 21–31. doi: 10.1016/j.nutres.2021.05.011
Parente C. E. T., Oliveira da Silva E., Sales Júnior S. F., Hauser-Davis R. A., Malm O., Correia F. V., et al. (2021). Fluoroquinolone-contaminated poultry litter strongly affects earthworms as verified through lethal and sub-lethal evaluations. Ecotoxicol. Environ. Saf. 207, 111305. doi: 10.1016/j.ecoenv.2020.111305
Peña-Guzmán C., Ulloa-Sánchez S., Mora K., Helena-Bustos R., Lopez-Barrera E., Alvarez J., et al. (2019). Emerging pollutants in the urban water cycle in Latin America: A review of the current literature. J. Environ. Manage. 237, 408–423. doi: 10.1016/J.JENVMAN.2019.02.100
Perucca E., White H. S., Bialer M. (2023). New GABA-targeting therapies for the treatment of seizures and epilepsy: II. Treatments in clinical development. CNS Drugs 37, 781–795. doi: 10.1007/s40263-023-01025-4
Pietrowska-Borek M., Dobrogojski J., Sobieszczuk-Nowicka E., Borek S. (2020). New insight into plant signaling: extracellular ATP and uncommon nucleotides. Cells 9, 345. doi: 10.3390/cells9020345
Pirayesh N., Giridhar M., Ben Khedher A., Vothknecht U. C., Chigri F. (2021). Organellar calcium signaling in plants: An update. Biochim. Biophys. Acta BBA - Mol. Cell Res. 1868, 118948. doi: 10.1016/j.bbamcr.2021.118948
Pivetta R. C., Rodrigues-Silva C., Ribeiro A. R., Rath S. (2020). Tracking the occurrence of psychotropic pharmaceuticals in Brazilian wastewater treatment plants and surface water, with assessment of environmental risks. Sci. Total Environ. 727, 138661. doi: 10.1016/j.scitotenv.2020.138661
Pottosin I., Velarde-Buendía A. M., Bose J., Zepeda-Jazo I., Shabala S., Dobrovinskaya O., et al. (2014). Cross-talk between reactive oxygen species and polyamines in regulation of ion transport across the plasma membrane: implications for plant adaptive responses. J. Exp. Bot. 65, 1271–1283. doi: 10.1093/jxb/ert423
Pozo M. J., López-Ráez J. A., Azcón-Aguilar C., García-Garrido J. M. (2015). Phytohormones as integrators of environmental signals in the regulation of mycorrhizal symbioses. New Phytol. 205, 1431–1436. doi: 10.1111/nph.13252
Rath S., Pereira L. A., Bosco S. M. D., Maniero M. G., Fostier A. H., Guimarães J. R. (2016). Fate of ivermectin in the terrestrial and aquatic environment: mobility, degradation, and toxicity towards Daphnia similis. Environ. Sci. pollut. Res. 23, 5654–5666. doi: 10.1007/s11356-015-5787-6
Rede D., Santos L.H.M.L.M., Ramos S., Oliva-Teles F., Antão C., Sousa S. R., et al. (2019). Individual and mixture toxicity evaluation of three pharmaceuticals to the germination and growth of Lactuca sativa seeds. Sci. Total Environ. 673, 102–109. doi: 10.1016/j.scitotenv.2019.03.432
Reichert J. F., Souza D. M., Martins A. F. (2019). Antipsychotic drugs in hospital wastewater and a preliminary risk assessment. Ecotoxicol. Environ. Saf. 170, 559–567. doi: 10.1016/j.ecoenv.2018.12.021
Rocha D. C., Da Silva Rocha C., Tavares D. S., De Morais Calado S. L., Gomes M. P. (2021). Veterinary antibiotics and plant physiology: An overview. Sci. Total Environ. 767, 144902. doi: 10.1016/j.scitotenv.2020.144902
Rocha R., Gonçalves F., Marques C., Nunes B. (2014). Environmental effects of anticholinesterasic therapeutic drugs on a crustacean species, Daphnia magna. Environ. Sci. pollut. Res. 21, 4418–4429. doi: 10.1007/s11356-013-2339-9
Saaristo M., Brodin T., Balshine S., Bertram M. G., Brooks B. W., Ehlman S. M., et al. (2018). Direct and indirect effects of chemical contaminants on the behaviour, ecology and evolution of wildlife. Proc. R. Soc B Biol. Sci. 285, 20181297. doi: 10.1098/rspb.2018.1297
Sabourin L., Duenk P., Bonte-Gelok S., Payne M., Lapen D. R., Topp E. (2012). Uptake of pharmaceuticals, hormones and parabens into vegetables grown in soil fertilized with municipal biosolids. Sci. Total Environ. 431, 233–236. doi: 10.1016/j.scitotenv.2012.05.017
Saddhe A. A., Malvankar M. R., Karle S. B., Kumar K. (2019). Reactive nitrogen species: Paradigms of cellular signaling and regulation of salt stress in plants. Environ. Exp. Bot. 161, 86–97. doi: 10.1016/j.envexpbot.2018.11.010
Schlossmann J., Schinner E. (2012). cGMP becomes a drug target. Naunyn. Schmiedebergs Arch. Pharmacol. 385, 243–252. doi: 10.1007/s00210-012-0730-6
Seeman M. V. (2021). History of the dopamine hypothesis of antipsychotic action. World J. Psychiatry 11, 355–364. doi: 10.5498/wjp.v11.i7.355
Segundo-Ortin M., Calvo P. (2022). Consciousness and cognition in plants. WIREs Cogn. Sci. 13, e1578. doi: 10.1002/wcs.1578
Shahid W., Ejaz S. A., al-Rashida M., Saleem M., Ahmed M., Rahman J., et al. (2021). Identification of NSAIDs as lipoxygenase inhibitors through highly sensitive chemiluminescence method, expression analysis in mononuclear cells and computational studies. Bioorganic Chem. 110, 104818. doi: 10.1016/j.bioorg.2021.104818
Sharifi R., Ryu C. (2021). Social networking in crop plants: Wired and wireless cross-plant communications. Plant Cell Environ. 44, 1095–1110. doi: 10.1111/pce.13966
Siemieniuk A., Ludynia M., Rudnicka M. (2021). Response of two crop plants, zea mays L. and solanum lycopersicum L., to diclofenac and naproxen. Int. J. Mol. Sci. 22, 8856. doi: 10.3390/ijms22168856
Silva L. J. G., Pereira A. M. P. T., Meisel L. M., Lino C. M., Pena A. (2014). A one-year follow-up analysis of antidepressants in Portuguese wastewaters: Occurrence and fate, seasonal influence, and risk assessment. Sci. Total Environ. 490, 279–287. doi: 10.1016/j.scitotenv.2014.04.131
Silvio E. I. (2022). Oral antihyperglycemic therapy for type 2 diabetes. Clin. Corner 287, 360–372. doi: 10.1001/jama.287.3.379
Sodhi K. K., Singh D. K. (2021). Insight into the fluoroquinolone resistance, sources, ecotoxicity, and degradation with special emphasis on ciprofloxacin. J. Water Process Eng. 43, 102218. doi: 10.1016/j.jwpe.2021.102218
Straub L., Strobl V., Neumann P. (2020). The need for an evolutionary approach to ecotoxicology. Nat. Ecol. Evol. 4, 895–895. doi: 10.1038/s41559-020-1194-6
Sun X., Wang Y., Xu L., Li C., Zhang W., Luo X., et al. (2017). Unraveling the root proteome changes and its relationship to molecular mechanism underlying salt stress response in radish (Raphanus sativus L.). Front. Plant Sci. 8. doi: 10.3389/fpls.2017.01192
Sunyer-Caldú A., Sepúlveda-Ruiz P., Salgot M., Folch-Sánchez M., Barcelo D., Diaz-Cruz M. S. (2022). Reclaimed water in agriculture: A plot-scale study assessing crop uptake of emerging contaminants and pathogens. J. Environ. Chem. Eng. 10, 108831. doi: 10.1016/j.jece.2022.108831
Szopa J., Wilczyński G., Fiehn O., Wenczel A., Willmitzer L. (2001). Identification and quantification of catecholamines in potato plants (Solanum tuberosum) by GC–MS. Phytochemistry 58, 315–320. doi: 10.1016/S0031-9422(01)00232-1
Taj G., Agarwal P., Grant M., Kumar A. (2010). MAPK machinery in plants: Recognition and response to different stresses through multiple signal transduction pathways. Plant Signal. Behav. 5, 1370–1378. doi: 10.4161/psb.5.11.13020
Tarkowská D. (2019). Plants are capable of synthesizing animal steroid hormones. Molecules 24, 2585. doi: 10.3390/molecules24142585
Thebo A. L., Drechsel P., Lambin E. F., Nelson K. L. (2017). A global, spatially-explicit assessment of irrigated croplands influenced by urban wastewater flows. Environ. Res. Lett. 12, 074008. doi: 10.1088/1748-326/aa75d1
Tomasik P., Horton D. (2012). “Chapter 2 - Enzymatic conversions of starch,” in Advances in Carbohydrate Chemistry and Biochemistry. Ed. Horton D. (Academic Press) 68, 59–436. doi: 10.1016/B978-0-12-396523-3.00001-4
Tuyiringire N., Tusubira D., Munyampundu J., Tolo C. U., Muvunyi C. M., Ogwang P. E. (2018). Application of metabolomics to drug discovery and understanding the mechanisms of action of medicinal plants with anti-tuberculosis activity. Clin. Transl. Med. 7, 29. doi: 10.1186/s40169-018-0208-3
U.S. Congress (2021). Research and Development in the Pharmaceutical Industry. Available at: https://www.cbo.gov/publication/57025.
Vischetti C., Casucci C., De Bernardi A., Monaci E., Tiano L., Marcheggiani F., et al. (2020). Sub-lethal effects of pesticides on the DNA of soil organisms as early ecotoxicological biomarkers. Front. Microbiol. 11. doi: 10.3389/fmicb.2020.01892
Wahman R., Cruzeiro C., Graßmann J., Schröder P., Letzel T. (2022). The changes in Lemna minor metabolomic profile: A response to diclofenac incubation. Chemosphere 287, 132078. doi: 10.1016/j.chemosphere.2021.132078
Wang N., Kong C., Wang P., Meiners S. J. (2021). Root exudate signals in plant–plant interactions. Plant Cell Environ. 44, 1044–1058. doi: 10.1111/pce.13892
Wang Y., Li X., Fan B., Zhu C., Chen Z. (2021). Regulation and function of defense-related callose deposition in plants. Int. J. Mol. Sci. 22, 2393. doi: 10.3390/ijms22052393
Wang J., Xu M., Liang X., Zhang Y., Yang D., Pan L., et al. (2021). Development of a novel 2D Ni-MOF derived NiO@C nanosheet arrays modified Ti/TiO2NTs/PbO2 electrode for efficient electrochemical degradation of salicylic acid wastewater. Sep. Purif. Technol. 263, 118368. doi: 10.1016/j.seppur.2021.118368
War A. R., Paulraj M. G., War M. Y., Ignacimuthu S. (2011). Role of salicylic acid in induction of plant defense system in chickpea ( Cicer arietinum L.). Plant Signal. Behav. 6, 1787–1792. doi: 10.4161/psb.6.11.17685
Wasternack C., Song S. (2016). Jasmonates: biosynthesis, metabolism, and signaling by proteins activating and repressing transciption. J. Exp. Bot. 68, erw443. doi: 10.1093/jxb/erw443
Waszkielewicz A. M., Gunia A., Szkaradek N., Sloczynska K., Krupińska S., Marona H. (2013). Ion channels as drug targets in central nervous system disorders. Curr. Med. Chem. 20, 1241–1285. doi: 10.2174/0929867311320100005
Westerlund A. M., Sridhar A., Dahl L., Andersson A., Bodnar A.-Y., Delemotte L. (2022). Markov state modelling reveals heterogeneous drug-inhibition mechanism of Calmodulin. PloS Comput. Biol. 18, e1010583. doi: 10.1371/journal.pcbi.1010583
Wishart D., Feunang Y., Guo A., Lo E., Marcu A., Grant J., et al. (2017). DrugBank 5.0: a major update to the DrugBank database for 2018. Nucleic Acids Res. doi: 10.1093/nar/gkx1037
Wong K.-H., Nam H.-Y., Lew S.-Y., Naidu M., David P., Kamalden T. A., et al. (2022). Discovering the potential of natural antioxidants in age-related macular degeneration: A review. Pharmaceuticals 15, 101. doi: 10.3390/ph15010101
Worley B., Powers R. (2013). Multivariate analysis in metabolomics. Curr. Metabolomics 1, 92–107. doi: 10.2174/2213235x11301010092
Wormington A. M., De María M., Kurita H. G., Bisesi J. H., Denslow N. D., Martyniuk C. J. (2020). Antineoplastic agents: environmental prevalence and adverse outcomes in aquatic organisms. Environ. Toxicol. Chem. 39, 967–985. doi: 10.1002/etc.4687
Wu X., Conkle J. L., Ernst F., Gan J. (2014). Treated wastewater irrigation: uptake of pharmaceutical and personal care products by common vegetables under field conditions. Environ. Sci. Technol. 48, 11286–11293. doi: 10.1021/es502868k
Wychodnik K., Gałęzowska G., Rogowska J., Potrykus M., Plenis A., Wolska L. (2020). Poultry farms as a potential source of environmental pollution by pharmaceuticals. Molecules 25, 1031. doi: 10.3390/molecules25051031
Yang F., Li G., Sang N. (2021). The phytotoxicities of agricultural soil samples from a coal gangue stacking area to several maize cultivars ( Zea mays L . ). Environ Sci Pollut Res Int 28 (37), 52319–52328. doi: 10.1007/s11356-021-14250-5
Yeh A., Marcinek D. J., Meador J. P., Gallagher E. P. (2017). Effect of contaminants of emerging concern on liver mitochondrial function in Chinook salmon. Aquat. Toxicol. 190, 21–31. doi: 10.1016/j.aquatox.2017.06.011
Yokawa K., Kagenishi T., Pavlovič A., Gall S., Weiland M., Mancuso S., et al. (2017). Anaesthetics stop diverse plant organ movements, affect endocytic vesicle recycling and ROS homeostasis, and block action potentials in Venus flytraps. Ann. Bot. 122,: 747–756. doi: 10.1093/aob/mcx155
Yu C., Zeng H., Wang Q., Chen W., Chen W., Yu W., et al. (2022). Multi-omics analysis reveals the molecular responses of Torreya grandis shoots to nanoplastic pollutant. J. Hazard. Mater. 436, 129181. doi: 10.1016/j.jhazmat.2022.129181
Yuan J., Dong X., Yap J., Hu J. (2020). The MAPK and AMPK signalings: interplay and implication in targeted cancer therapy. J. Hematol. Oncol.J Hematol. Oncol. 13, 113. doi: 10.1186/s13045-020-00949-4
Zeng Y., Zhang Y., Zhang H., Wang J., Lian K., Ai L. (2022). Uptake and transport of different concentrations of PPCPs by vegetables. Int. J. Environ. Res. Public. Health 19, 15840. doi: 10.3390/ijerph192315840
Zhang M., Zhang S. (2022). Mitogen-activated protein kinase cascades in plant signaling. J. Integr. Plant Biol. 64, 301–341. doi: 10.1111/jipb.13215
Zhao Q., Chen W., Bian J., Xie H., Li Y., Xu C., et al. (2018). Proteomics and phosphoproteomics of heat stress-responsive mechanisms in spinach. Front. Plant Sci. 9. doi: 10.3389/fpls.2018.00800
Zheng W., Guo M. (2021). Soil–plant transfer of pharmaceuticals and personal care products. Curr. pollut. Rep. 7, 510–523. doi: 10.1007/s40726-021-00207-2
Ziegler M., Knoll S., Köhler H.-R., Tisler S., Huhn C., Zwiener C., et al. (2020). Impact of the antidepressant citalopram on the behaviour of two different life stages of brown trout. PeerJ 8, e8765. doi: 10.7717/peerj.8765
Keywords: plant, sub-lethal effect, pharmaceutical, mode of action (MOA), waste valorisation, agriculture
Citation: Garduño-Jiménez A-L and Carter LJ (2024) Insights into mode of action mediated responses following pharmaceutical uptake and accumulation in plants. Front. Agron. 5:1293555. doi: 10.3389/fagro.2023.1293555
Received: 13 September 2023; Accepted: 26 December 2023;
Published: 15 January 2024.
Edited by:
Helen Catherine Glanville, Loughborough University, United KingdomReviewed by:
Jian Wu, Guizhou University, ChinaCopyright © 2024 Garduño-Jiménez and Carter. This is an open-access article distributed under the terms of the Creative Commons Attribution License (CC BY). The use, distribution or reproduction in other forums is permitted, provided the original author(s) and the copyright owner(s) are credited and that the original publication in this journal is cited, in accordance with accepted academic practice. No use, distribution or reproduction is permitted which does not comply with these terms.
*Correspondence: Andrea-Lorena Garduño-Jiménez, YS5sLmdhcmR1bm9qaW1lbmV6QGxlZWRzLmFjLnVr; Laura J. Carter, bC5qLmNhcnRlckBsZWVkcy5hYy51aw==
Disclaimer: All claims expressed in this article are solely those of the authors and do not necessarily represent those of their affiliated organizations, or those of the publisher, the editors and the reviewers. Any product that may be evaluated in this article or claim that may be made by its manufacturer is not guaranteed or endorsed by the publisher.
Research integrity at Frontiers
Learn more about the work of our research integrity team to safeguard the quality of each article we publish.