- Laboratory of Food Microbiology, Research and Production Center for Microbiology and Virology, Almaty, Kazakhstan
Global climate change presents various challenges to agricultural biotechnology in developing crops with increased resilience to various adverse natural conditions. Given the importance of this problem, we explored the mechanisms of plant salt tolerance and the role of plant-associated microbes, in mediating important physiological and metabolic processes that increase plant resistance to salt stress. Understanding the physiological, metabolic, and molecular responses of the entire plant holobiont, primarily including microorganisms, to the combination of abiotic stresses may be the key to developing more effective methods of combating various stress conditions and increasing agricultural efficiency. This work encompassed 86 peer-reviewed articles focused on various aspects of plant development in saline conditions and especially on key mechanisms of mitigating stress conditions, including the role of rhizobiome and endophytic microorganisms. It is shown that host plants and various microorganisms can form complex relationships where each organism plays a specific role in forming tolerance to stress conditions. Our review proposes that studying microorganisms that are resistant to soil salinity can lead to the development of new strategies to combat salinization and improve crop stress resistance. The paper concludes that using salt-adapted biostimulant microorganisms, which are natural components of agricultural plant microbiomes, is a highly promising research area.
1 Introduction
Global climate change on the planet, combined with human activities that are often irrational, destabilizes the functioning of agroecosystems, causing irreparable damage (Sánchez-Bermúdez et al., 2022; Zandalinas et al., 2022; Bhusal et al., 2023) to food production. Abiotic stresses such as drought, salinity, flooding, extreme temperatures, potassium deficiency, UV radiation, soil pollution with heavy metals, disease outbreaks, and others, lead to alterations in the physiological, biochemical, cellular, and molecular mechanisms of plants (He et al., 2018) resulting in reduced yield and quality of plant products. Salinity stress is a widespread ecological problem, which tends to rise with increasing climate aridity. The accumulation of salts in groundwater, combined with prolonged periods of drought and other factors, leads to the deterioration of agricultural land, posing a threat to food security (Tahir et al., 2022). According to FAO, more than one billion hectares of soil across one hundred countries are impacted by salinity issues. Predictions indicate that 50% of arable land will suffer from salinization in the near future, potentially causing up to a 70% drop in grain crop yields, including wheat, rice, corn, and barley(Hussain et al., 2019).
In the situation of increasing population and growing food demand, it is necessary to find effective ways to intensify food production. The modern challenge of agricultural biotechnology is to identify an optimal development strategy for agricultural crops with increased resilience to various adverse natural conditions including salinity stress. The current decade, due to the drastic changes in climatic conditions, represents a critical time for taking measures in this regard (Eckardt et al., 2023).
Current research in this direction covers studies of key mechanisms of salt stress responses and resilience, as well as practical strategies for salt stress mitigation (Park et al., 2016; Pandey et al., 2020). In addition to using modern genetic engineering methods to obtain plant varieties (Shukla et al., 2018), biostimulants are considered a promising and environmentally friendly approach (Pandey et al., 2020; Ali et al., 2021; Ma et al., 2022). These biostimulants mainly consist of beneficial bacteria and/or fungi capable of modulating metabolic processes in plants, increasing their resistance to different abiotic stresses, and improving their productivity. In recent years, studies have shown that exploring the fine-tuning and integration of many signals generated by microbial interactions in plants, as well as manipulating beneficial microbes and plant regulators, serves as the basis for adapting agricultural crops to changing climatic conditions, forming the foundation of sustainable agriculture (Gul et al., 2023).
2 Overcoming salt stress by plants
In saline soils, water retention, structural stability, nutrient availability, pH, and microbial biodiversity are reduced. Increased soil salinity results in toxic Na+ accumulation in salt-sensitive plant cells, disrupting nutrient uptake, cell membrane function, and enzyme activities, and causing oxidative stress (Craig Plett and Møller, 2010; Baral et al., 2015; Jacoby et al., 2016). Potassium deficiency co-occurs along with an increase in sodium level and affects cell division, protein functions, photosynthesis, subsequent carbohydrate translocation, and other metabolic pathways, as well as sensitivity to other stresses, finally influencing crop yield and quality (Imtiaz et al., 2023; Zhang et al., 2023). Plants adapted to saline environments mitigate sodium damage by controlling uptake, transport, and exclusion (Munns and Tester, 2008) by such means as enhanced hydraulic conductivity, sequestration of toxic Na+ particles, osmolyte accumulation, and maintaining higher stomatal conductance (Parvaiz and Satyawati, 2008; Yamaguchi et al., 2013).
The ability of plants to retain potassium under stressful conditions is considered the most crucial mechanism for their salt tolerance(Shabala, 2000; Imtiaz et al., 2022). K+ ions are associated with reactive oxygen species (ROS) detoxification under various abiotic stresses (Anil Kumar et al., 2022). The key instrument for achieving cell homeostasis in saline stress conditions is maintaining Na+ and K+ transporters and channel activity (Saibi and Brini, 2021) and the coordinated work of several K+ channels and transporters (Mostofa et al., 2022). Transcription of genes related to ion homeostasis serves as the primary regulator under saline conditions (Parvaiz and Satyawati, 2008). H+, K+, and Na+ transporters play a critical role in plant mitigating ion stress by excluding toxic Na+ from the cytosol or preventing Na+ transport to photosynthetic organs while modulating osmotic balance. In response to unfavorable conditions, plants also produce small secretory peptides, which act as signaling molecules to start certain physiological processes (Xie et al., 2022). As a part of the adaptation strategy to salinity, plants synthesize osmoprotectants (Ashraf and Foolad, 2007), which mainly include amino compounds and sugars and help maintaining low water potential and enhancing water uptake and absorption (Slama et al., 2015).
The adaptation of plants to saline soil is a very slow process as it depends on the combined regulation of hundreds of genes. Manipulations with these genes in model plants yield promising results, but their application in real agricultural conditions is still limited.
3 Input of microorganisms in plant salt tolerance
Plant-associated microorganisms, such as plant growth-promoting bacteria (PGPB) and arbuscular mycorrhizal fungi (AMF) can increase crop productivity and provide stress resistance. Microorganisms isolated from stress-prone plant habitats can regulate the production and accumulation of osmoprotectants and signaling molecules to enhance plant stress tolerance, increase nutrient availability, and enhance the antioxidant system (Finkel et al., 2017; Mhlongo et al., 2018; Inbaraj, 2021) (Table 1). The genetic diversity of microorganisms and their metabolic capabilities contribute to their adaptation to extreme conditions, including soil salinity.
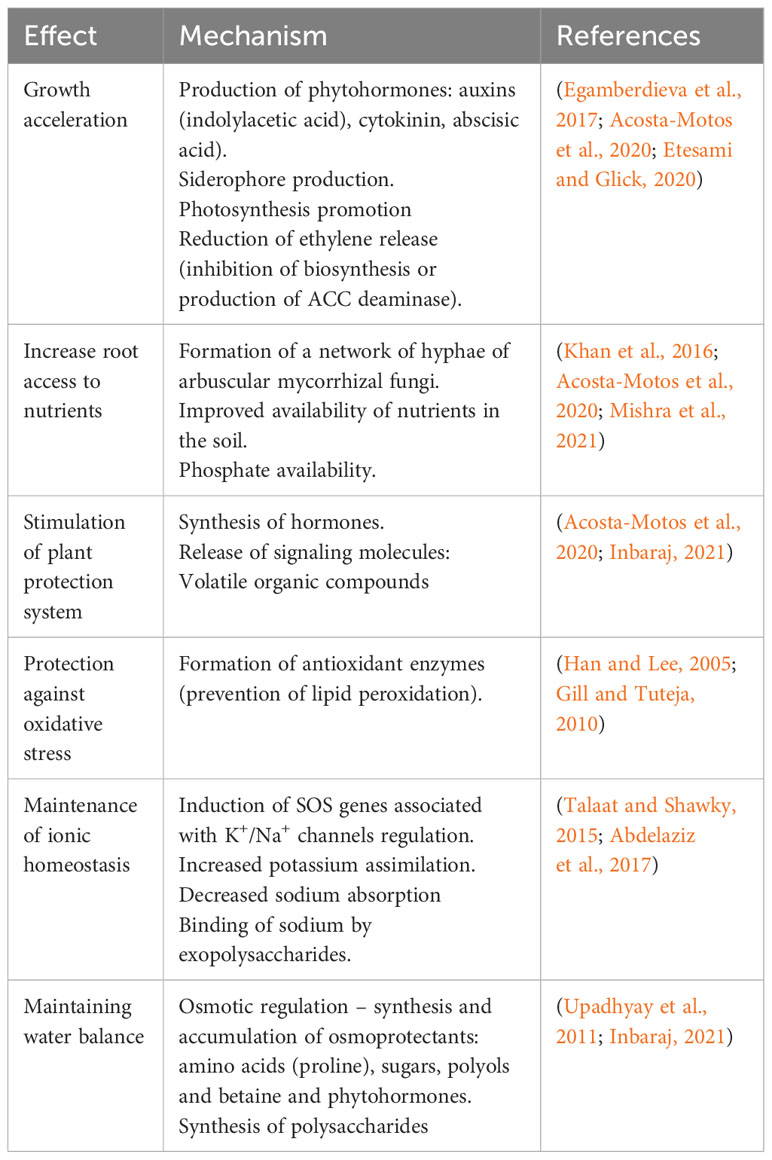
Table 1 The main mechanisms of the effect of microorganisms on the experience of salt stress by plants.
The data of Table 1 show that microorganisms can activate salt stress defensive mechanisms that are similar to plant responses, and produce some plant-specific compounds like phytohormones. This allows them to promote and enhance the plant response to stress and increase plant non-specific resistance.
3.1 PGPB
PGPB are an important group, which can play a pivotal role in mitigating plant stress from salinity and other abiotic stresses by facilitating plant growth. PGPB help to mitigate sodium damage by producing phytohormones, controlling uptake, transport, and exclusion of ions, osmotic regulation, and antioxidant defense; thus these mechanisms enhance plants’ natural ability to cope with stress (Seguel et al., 2016; Arora et al., 2021; Baltazar et al., 2021; Jalal et al., 2023).
PGPB can enhance plant tolerance to salt stress by modulating the expression of antioxidant enzymes, including the enzyme that removes ROS (Xia et al., 2020). They induce peroxide-reduction systems and express ACC deaminase, which enhances plant growth by reducing the internal concentration of stress-induced ethylene. Due to the inoculation with these bacteria, the content of malondialdehyde and H2O2 decreases, and indicators of antioxidant capacity, proline content, and antioxidant enzymes increase. Bacteria can mitigate the effect of salt stress by providing plants with phytohormones involved in the regulation of growth and general stress mitigation such as auxin, cytokinin, and abscisic acid (Egamberdieva et al., 2017; Acosta-Motos et al., 2020; Etesami and Glick, 2020; Kumawat et al., 2023). Additionally, most rhizobacteria can also produce various compounds, modulating the solubility of nutrients and binding Na+, while symbiotic endophytes promote the synthesis of protective cell components such as amino acids, sugars, polyols, and betaines, therefore regulating osmotic balance and ion homeostasis (Egamberdieva et al., 2017; Acosta-Motos et al., 2020; Etesami and Glick, 2020).
Using PGPB as biofertilizers can be a powerful tool for sustainable agriculture in saline soils. Nevertheless, many molecular functions and signaling pathways of PGPB used to stimulate the growth of agricultural crops during plant-microbe interactions have yet to be fully understood.
3.2 Fungi
The use of biotechnological approaches with AMF is another environmentally friendly option for sustainable agriculture. AMF form a network of hyphae with plant roots, significantly increasing root access to a larger soil surface area and enhancing nutrient uptake (Bowles et al., 2016; Khan et al., 2016). They also improve soil quality by influencing its structure and texture and enhancing the uptake of nutrients such as K, N, P, Cu, Fe, and Zn (Khan et al., 2016; Thirkell et al., 2017). This prevents disruption of normal cellular enzymatic processes. AMF can regulate the movement of excess Na+ out of cells through the Na+/H+ antiporter of the plasma membrane by modulating the expression of genes responsible for maintaining ion homeostasis (Al-Khaliel, 2010). Some common AMF species include Rhizophagus, Septoglycus viscosum, Claroideoglomus etunicatum, and Claroideoglomus claroideum (Bitterlich et al., 2020).
AMF are not the only form of fungal biostimulants. Various endophytic fungi, such as Trichoderma spp and the order Sebacinales, are widely used to aid sustainable agricultural production (López-Bucio et al., 2015; Mishra et al., 2021). Endophytic fungus Piriformospora indica increases salt tolerance of a host plant by regulating ion channels, which play an essential role in potassium transportation and regulation of Na+/K+ homeostasis (Abdelaziz et al., 2017). Other mechanisms, employed by endophytic fungi, may include enhancing the accumulation of soluble polyamines, sugars, and organic acids in salt-stressed plants (Bouzouina et al., 2021). These compounds play the role of osmoprotectants and signaling molecules to modify the plant rhizosphere (Souza et al., 2015).
3.3 Protection against multiple stress by plant-microbe interaction
As various stress conditions could trigger similar biochemical responses in plants, it is important to understand the overlapping mechanisms providing abiotic stress tolerance. Since the oxidative stress is a common response to abiotic stresses (Vadez et al., 2012; Harshavardhan et al., 2018), the protection against multiple stresses can be achieved by cultivating plants resistant to oxidative stress. Recognizing the potential for adaptation to ROS stress could yield organisms capable of tolerating various abiotic stresses simultaneously.
Noteworthy instances of enhanced resistance to various types of stress after exposure to another stress type are illustrated in Table 2.
Microbial cross-tolerance can stem from shared stimulation targets or the ability to secrete the same substances under diverse stress conditions. Additionally, microorganisms utilize membrane transport and absorb extracellular organic compounds to bolster stress resistance across various stress environments (Tang et al., 2014; Huang et al., 2016; Wijesundara and Rupasinghe, 2019).
Experiments involving such “adaptive laboratory evolution” (Sandberg et al., 2019) are an effective approach for studying evolutionary forces influencing microbial phenotypes, ability to promote plant productivity, and field treatment reliability, as well as obtaining industrial strains with beneficial mutations. Gene regulation shapes microbial phenotypes, and stress conditions can favor adaptive gene combinations. This leads to mutual coordination between genes and the environment, altering gene interactions due to genetic variability under stress, a mechanism observed in yeast’s global genetic network under various stresses (Carlson et al., 2012). A comparable stress resistance mechanism might occur in various microorganisms.
Analyzing a comprehensive dataset from diverse sources reveals that bioinoculants’ interaction efficacy with plants was improved after sublethal stress exposure (lyophilization, desiccation, and prolonged storage). This enhanced development, disease control, and stress resistance in plants compared to non-prepared inoculants (da Cunha et al., 2023) underscore that microbial adaptation, both short- and long-term, universally raises adaptability to stress conditions (Tan et al., 2022).
As shown in Table 2, plant genes can affect yeast cross-resistance. Attention should be given to the impact of adapted microorganisms on plant cross-resistance. The use of wheat endophytic actinomycetes adapted to high levels of sodium chloride improved plant resistance to salinity (Yermekbay et al., 2022). The adapted microorganisms simultaneously protect sprouts from fungal infection. Investigating the input of experimental adaptation of local microbiome to increased salinity can be a promising approach to solving the problem.
3.4 Current application and future prospects
The most common implementation of stress-reducing PGPB and/or AMF involves the introduction of rhizosphere or endophytic strains that have been previously isolated from different saline environments. This practice proved to be effective for some plants, reducing the stress of the host plant and noticeably increasing crop yields. However, it has been mentioned that this approach could have some significant reliability issues (Souza et al., 2015; Liu et al., 2022), mainly caused by potential conflicts and a lack of symbiotic relations between invasive (introduced) species and native plants and microbiota (Hart et al., 2018; Magan, 2020; Mitter et al., 2021). Studies show that the microbiomes of the rhizosphere of various plants are highly species-specific (Leach et al., 2017), which can be a serious obstacle to the successful use of microorganisms isolated from other plant species. Moreover, each halophytic plant in different geographical zones has its own unique bacterial and fungal communities (Yamamoto et al., 2020), determined not only by the plant’s specificity and the set of microorganisms existing in these conditions but also by various soil and climatic conditions of that geographic zone.
Promisingly, several research (Gouda et al., 2018; Mishra et al., 2018; Kumar et al., 2019) indicate that local strains can be adapted to various stress conditions and re-introduced into the original environment as biofertilizers. The main idea of this approach is that native PGPB and AMF will develop stress tolerance while retaining most ecological connections in a given biome thus enhancing chances of successful assimilation while taking advantage of the existing microbiome. It has been shown that native AMF can adapt and employ all the same mechanisms of salt tolerance as those fungi that had been isolated from different saline locations (Ben-Laouane et al., 2020).
Understanding local microbiota adaptation mechanisms enhances insights into high-salinity ecosystem reactions (Kim et al., 2019). Stress during the plant’s growth induces the production of secondary metabolites in developing cells, fortifying them against future abiotic stressors, and illustrating a memory of prior stress exposure (Sharma et al., 2022). Acclimation and stress memory to a specific type of stress also confer resistance against other stressors, granting cross-stress memory and tolerance (Walter et al., 2013). Currently, researchers are delving into the mechanisms behind transcriptional stress memory (Nguyen et al., 2022). Adaptive responses under stresses like heat, cold, acidity, osmotic changes, nutrient deprivation, and oxidative stress encompass the synthesis of stress-induced proteins, membrane fatty acid regulation, accumulation of compatible solutes, and other cross-protective factors (Gao et al., 2022). The selection of stress-tolerant plant genotypes requires a long time. However, the adaptation of the natural plant microbiota can be carried out in a short time due to the high rate of microbial reproduction. Numerous studies show that salt-adapted bacteria from halophytic plants have undergone some genetic changes that may involve over-expression of specific genes (Binod Kumar et al., 2023) or plasmids, improving ion transport and homeostasis, production of osmoprotectans, and antioxidants (Domínguez-Ferreras et al., 2006); they can also successfully up-regulate numerous relevant plant genes conferring salt tolerance to the host and even can induce salt responsive genes expression through the intervention of several transcription factors (Roy et al., 2021).
It is worth mentioning a few drawbacks of this method: mainly possible higher costs of resulting fertilizer and time needed for such development. However, a more efficient result, by reducing the time and cost of selecting microorganisms that are not characteristic of this biome, will contribute to a higher final result. Occasionally, it is possible that existing soil microbiome already contains AMF and PGPB not fully expressing their beneficial effects. It is noted that microbial activity decreases as a result of soil disturbance (excessive tilling) and application of mineral fertilizers, and rises after application of various organic fertilizers and continuous crop rotation practice (Chernov and Semenov, 2021). Thus, the beneficial effects of soil microbes (either native or artificially amended) are strictily conditioned by global soil management practices. The use of endophytic microorganisms that do not live in the soil but in the plant itself is a good way to bypass the problem of incompatibility with the soil conditions. For example, endophytic Fusarium sp. from salt-adapted Pokkali rice could colonize a salt-sensitive rice variety and make it salt tolerant (Sampangi-Ramaiah et al., 2020).
4 Conclusions
Studying microorganisms’ resistance to soil salinity opens up possibilities for developing and implementing new strategies for elevating crop stress resistance (Dodd and Perez-Alfocea, 2012; Praveen and Singh, 2023) and imparting induced systemic tolerance to plants (Kumawat et al., 2023). Rhizosphere microorganisms modulate nutrient availability in the surrounding soil or modify the root system itself thus effectively changing soil conditions for the host plant, while endophytic microorganisms regulate plants’ own defense systems, or produce various compounds meant to protect host plants from various stresses.
Inoculating seeds with halotolerant bacteria and/or fungi shows significant improvement in crop production in drought and saline conditions, however, new research shows that pre-conditioning of either commercial strains or locally selected microbes to certain stressing or cultural conditions may enhance their efficacy. The optimal technological solution for pre-adaptation (salt dose, number of passages, etc.) and return of adapted microbiota to a host plant is a direction for further research.
Successful development of methods to enhance the salt resistance of microorganisms would broaden the range of bioinoculants able not only to increase the salt tolerance of crops but also address many issues related to worsening climate conditions and other stresses. The high rate of growth and reproduction of microorganisms allows them to adapt faster than plants to changing conditions and has a protective and stimulating effect on plant physiology. The use of plant endophytic microorganisms that inhabit the internal parts of plants and are not strictly influenced by soil conditions may be the promising perspective. Increasing the adaptation of native endophytic and rhizosphere microorganisms can be a very productive and time-saving approach to solving the problem of plant salt tolerance. In general, it should be noted that the increase in plant resistance to salinity is highly interconnected and intertwined with the response of plant microbiome es; therefore, an effective impact can be achieved only if the entire holobiont of a particular plant is taken into account.
Author contributions
SM: Writing – original draft. MS: Writing – review & editing. OY: Writing – review & editing. YM: Writing – review & editing.
Funding
The author(s) declare financial support was received for the research, authorship, and/or publication of this article. This research has been funded by the Science Committee of the Ministry of Education and Science of the Republic of Kazakhstan (Grant No. AP09258751).
Conflict of interest
The authors declare that the research was conducted in the absence of any commercial or financial relationships that could be construed as a potential conflict of interest.
Publisher’s note
All claims expressed in this article are solely those of the authors and do not necessarily represent those of their affiliated organizations, or those of the publisher, the editors and the reviewers. Any product that may be evaluated in this article, or claim that may be made by its manufacturer, is not guaranteed or endorsed by the publisher.
References
Abdelaziz M. E., Kim D., Ali S., Fedoroff N. V., Al-Babili S. (2017). The endophytic fungus Piriformospora indica enhances Arabidopsis thaliana growth and modulates Na+/K+ homeostasis under salt stress conditions. Plant Sci. 263, 107–115. doi: 10.1016/j.plantsci.2017.07.006
Acosta-Motos J. R., Penella C., Hernández J. A., Díaz-Vivancos P., Sánchez-Blanco M. J., Navarro J. M., et al. (2020). Towards a sustainable agriculture: Strategies involving phytoprotectants against salt stress. Agronomy 10 (2), 194. doi: 10.3390/agronomy10020194
Ali A., Raddatz N., Pardo J. M., Yun D. J. (2021). HKT sodium and potassium transporters in Arabidopsis thaliana and related halophyte species. Physiol. Plantarum 171 (4), 546–558. doi: 10.1111/ppl.13166
Al-Khaliel A. (2010). Effect of salinity stress on mycorrhizal association and growth response of peanut infected by Glomus mosseae. Plant Soil Environ. 56 (7), 318–324. doi: 10.17221/204/2009-PSE
Anil Kumar S., Kaniganti S., Hima Kumari P., Sudhakar Reddy P., Suravajhala P., S. P and P. K. (2022). Functional and biotechnological cues of potassium homeostasis for stress tolerance and plant development. Biotechnol. Genet. Eng. Rev., 1–44. doi: 10.1080/02648725.2022.2143317
Arora N. K., Egamberdieva D., Mehnaz S., Li W.-J., Mishra I. (2021). Salt tolerant Rhizobacteria: for better productivity and remediation of saline soils. Front. Media SA 12, 660075. doi: 10.3389/978-2-88966-880-9
Ashraf M., Foolad M. R. (2007). Roles of glycine betaine and proline in improving plant abiotic stress resistance. Environ. Exp. Bot. 59 (2), 206–216. doi: 10.1016/j.envexpbot.2005.12.006
Baltazar M., Correia S., Guinan K. J., Sujeeth N., Bragança R., Gonçalves B. (2021). Recent advances in the molecular effects of biostimulants in plants: An overview. Biomolecules 11 (8), 1096. doi: 10.3390/biom11081096
Banti V., Loreti E., Novi G., Santaniello A., Alpi A., Perata P. (2008). Heat acclimation and cross-tolerance against anoxia in Arabidopsis. Plant Cell Environ. 31 (7), 1029–1037. doi: 10.1111/j.1365-3040.2008.01816.x
Baral A., Shruthi K., Mathew M. (2015). Vesicular trafficking and salinity responses in plants. IUBMB Life 67 (9), 677–686. doi: 10.1002/iub.1425
Ben-Laouane R., Baslam M., Ait-El-Mokhtar M., Anli M., Boutasknit A., Ait-Rahou Y., et al. (2020). Potential of native arbuscular mycorrhizal fungi, rhizobia, and/or green compost as alfalfa (Medicago sativa) enhancers under salinity. Microorganisms 8 (11), 1695. doi: 10.3390/microorganisms8111695
Bhusal N., Sharma P., Kumar R. R., Sareen S. (2023). Multiple abiotic stresses: Molecular, physiological, and genetic responses and adaptations in cereals. Front. Plant Sci. 14, 1146326. doi: 10.3389/fpls.2023.1146326
Binod Kumar S., Kalwasińska A., Swiontek Brzezinska M., Wróbel M. (2023). Application of halotolerant Azotobacter chroococcum W4ii isolated from technosoils to mitigate salt stress in wheat plant. Open Res. Europe 3, 76. doi: 10.12688/openreseurope.15821.2
Bitterlich M., Mercy L., Arato M., Franken P. (2020). Arbuscular mycorrhizal fungi as biostimulants for sustainable crop production. Biostimulants for sustainable crop production (Cambridge UK: Burleigh Dodds Science Publishing), 227–271.
Bouzouina M., Kouadria R., Lotmani B. (2021). Fungal endophytes alleviate salt stress in wheat in terms of growth, ion homeostasis and osmoregulation. J. Appl. Microbiol. 130 (3), 913–925. doi: 10.1111/jam.14804
Bowles T. M., Barrios-Masias F. H., Carlisle E. A., Cavagnaro T. R., Jackson L. E. (2016). Effects of arbuscular mycorrhizae on tomato yield, nutrient uptake, water relations, and soil carbon dynamics under deficit irrigation in field conditions. Sci. Total Environ. 566, 1223–1234. doi: 10.1016/j.scitotenv.2016.05.178
Çakar Z. P., Alkım C., Turanlı B., Tokman N., Akman S., Sarıkaya M., et al. (2009). Isolation of cobalt hyper-resistant mutants of Saccharomyces cerevisiae by in vivo evolutionary engineering approach. J. Biotechnol. 143 (2), 130–138. doi: 10.1016/j.jbiotec.2009.06.024
Carlson R. P., Oshota O. J., Taffs R. L. (2012). Systems analysis of microbial adaptations to simultaneous stresses. Reprogramming Microbial Metab. Pathways, 64 139–157. doi: 10.1007/978-94-007-5055-5_7
Cheng Z., Chi M., Li G., Chen H., Sui Y., Sun H., et al. (2016). Heat shock improves stress tolerance and biocontrol performance of Rhodotorula mucilaginosa. Biol. Control 95, 49–56. doi: 10.1016/j.biocontrol.2016.01.001
Chernov T., Semenov M. (2021). Management of soil microbial communities: opportunities. Eurasian Soil Sci. (54), 1888–1902. doi: 10.1134/S1064229321120024
Craig Plett D., Møller I. S. (2010). Na+ transport in glycophytic plants: what we know and would like to know. Plant Cell Environ. 33 (4), 612–626. doi: 10.1111/j.1365-3040.2009.02086.x
da Cunha E. T., Pedrolo A. M., Arisi A. C. M. (2023). Effects of sublethal stress application on the survival of bacterial inoculants: a systematic review. Arch. Microbiol. 205 (5), 190. doi: 10.1007/s00203-023-03542-8
Dodd I. C., Perez-Alfocea F. (2012). Microbial amelioration of crop salinity stress. J. Exp. Bot. 63 (9), 3415–3428. doi: 10.1093/jxb/ers033
Domínguez-Ferreras A., Pérez-Arnedo R., Becker A., Olivares J., Soto M., Sanjuán J. (2006). Transcriptome profiling reveals the importance of plasmid pSymB for osmoadaptation of Sinorhizobium meliloti. J. Bacteriol. 188 (21), 7617–7625. doi: 10.1128/JB.00719-06
Eckardt N. A., Ainsworth E. A., Bahuguna R. N., Broadley M. R., Busch W., Carpita N. C., et al. (2023). Climate change challenges, plant science solutions. Plant Cell 35 (1), 24–66. doi: 10.1093/plcell/koac303
Egamberdieva D., Wirth S. J., Alqarawi A. A., Abd_Allah E. F., Hashem A. (2017). Phytohormones and beneficial microbes: essential components for plants to balance stress and fitness. Front. Microbiol. 8, 2104. doi: 10.3389/fmicb.2017.02104
Etesami H., Glick B. R. (2020). Halotolerant plant growth–promoting bacteria: Prospects for alleviating salinity stress in plants. Environ. Exp. Bot. 178, 104124. doi: 10.1016/j.envexpbot.2020.104124
Finkel O. M., Castrillo G., Paredes S. H., González I. S., Dangl J. L. (2017). Understanding and exploiting plant beneficial microbes. Curr. Opin. Plant Biol. 38, 155–163. doi: 10.1016/j.pbi.2017.04.018
Gangadhar B. H., Yu J. W., Sajeesh K., Park S. W. (2014). A systematic exploration of high-temperature stress-responsive genes in potato using large-scale yeast functional screening. Mol. Genet. Genomics 289, 185–201. doi: 10.1007/s00438-013-0795-z
Gao X., Kong J., Zhu H., Mao B., Cui S., Zhao J. (2022). Lactobacillus, Bifidobacterium and Lactococcus response to environmental stress: Mechanisms and application of cross-protection to improve resistance against freeze-drying. J. Appl. Microbiol. 132 (2), 802–821. doi: 10.1111/jam.15251
Gill S. S., Tuteja N. (2010). Reactive oxygen species and antioxidant machinery in abiotic stress tolerance in crop plants. Plant Physiol. Biochem. 48 (12), 909–930. doi: 10.1016/j.plaphy.2010.08.016
Gouda S., Kerry R. G., Das G., Paramithiotis S., Shin H.-S., Patra J. K. (2018). Revitalization of plant growth promoting rhizobacteria for sustainable development in agriculture. Microbiol. Res. 206, 131–140. doi: 10.1016/j.micres.2017.08.016
Gul N., Wani I. A., Mir R. A., Nowshehri J. A., Aslam S., Gupta R., et al. (2023). Plant growth promoting microorganisms mediated abiotic stress tolerance in crop plants: A critical appraisal. Plant Growth Regul. 100 (1), 7–24. doi: 10.1007/s10725-022-00951-5
Han H., Lee K. (2005). Plant growth promoting rhizobacteria effect on antioxidant status, photosynthesis, mineral uptake and growth of lettuce under soil salinity. Res. J. Agric. Biol. Sci. 1 (3), 210–215.
Harshavardhan V. T., Govind G., Kalladan R., Sreenivasulu N., Hong C.-Y. (2018). Cross-protection by oxidative stress: improving tolerance to abiotic stresses including salinity. Salinity Responses Tolerance Plants 1, 283–305. doi: 10.1007/978-3-319-75671-4_11
Hart M. M., Antunes P. M., Chaudhary V. B., Abbott L. K. (2018). Fungal inoculants in the field: Is the reward greater than the risk? Funct. Ecol. 32 (1), 126–135. doi: 10.1111/1365-2435.12976
He M., He C.-Q., Ding N.-Z. (2018). Abiotic stresses: general defenses of land plants and chances for engineering multistress tolerance. Front. Plant Sci. 9, 1771. doi: 10.3389/fpls.2018.01771
Huang M., Khan J., Kaur M., Vanega J. D. T., Patiño O. A. A., Ramasubramanian A. K., et al. (2019). CgSTE11 mediates cross tolerance to multiple environmental stressors in Candida glabrata. Sci. Rep. 9 (1), 17036. doi: 10.1038/s41598-019-53593-5
Huang R., Pan M., Wan C., Shah N. P., Tao X., Wei H. (2016). Physiological and transcriptional responses and cross protection of Lactobacillus plantarum ZDY2013 under acid stress. J. Dairy Sci. 99 (2), 1002–1010. doi: 10.3168/jds.2015-9993
Hussain S., Shaukat M., Ashraf M., Zhu C., Jin Q., Zhang J. (2019). Salinity stress in arid and semi-arid climates: Effects and management in field crops. Climate Change Agric. 13, 201–226. doi: 10.5772/intechopen.87982
Imtiaz H., Mir A. R., Corpas F. J., Hayat S. (2023). Impact of potassium starvation on the uptake, transportation, photosynthesis, and abiotic stress tolerance. Plant Growth Regul. 99 (3), 429–448. doi: 10.1007/s10725-022-00925-7
Imtiaz H., Shiraz M., Mir A. R., Hayat S. (2022). Green synthesis of nanoparticles and their effect on plant growth and development: a review. Plant Arch. 22 (1) 343–350. doi: 10.51470/PLANTARCHIVES.2022.v22.no1.054
Inbaraj M. P. (2021). Plant-microbe interactions in alleviating abiotic stress—a mini review. Front. Agron. 3, 667903. doi: 10.3389/fagro.2021.667903
Jacoby R., Che-Othman M., Millar A., Taylor N. (2016). Analysis of the sodium chloride-dependent respiratory kinetics of wheat mitochondria reveals differential effects on phosphorylating and non-phosphorylating electron transport pathways. Plant Cell Environ. 39 (4), 823–833. doi: 10.1111/pce.12653
Jalal A., da Silva Oliveira C. E., Galindo F. S., Rosa P. A. L., Gato I. M. B., de Lima B. H., et al. (2023). Regulatory mechanisms of plant growth-promoting rhizobacteria and plant nutrition against abiotic stresses in Brassicaceae family. Life 13 (1), 211. doi: 10.3390/life13010211
Kalburge S. S., Whitaker W. B., Boyd E. F. (2014). High-salt preadaptation of Vibrio parahaemolyticus enhances survival in response to lethal environmental stresses. J. Food Prot. 77 (2), 246–253. doi: 10.4315/0362-028X.JFP-13-241
Khan M. A., Gemenet D. C., Villordon A. (2016). Root system architecture and abiotic stress tolerance: current knowledge in root and tuber crops. Front. Plant Sci. 7, 1584. doi: 10.3389/fpls.2016.01584
Kim K., Samaddar S., Chatterjee P., Krishnamoorthy R., Jeon S., Sa T. (2019). Structural and functional responses of microbial community with respect to salinity levels in a coastal reclamation land. Appl. Soil Ecol. 137, 96–105. doi: 10.1016/j.apsoil.2019.02.011
Kumar A., Patel J., Meena V., Srivastava R. (2019). Recent advances of PGPR based approaches for stress tolerance in plants for sustainable agriculture. Biocatal Agric. Biotechnol. 20, 101271. doi: 10.1016/j.bcab.2019.101271
Kumawat K. C., Sharma B., Nagpal S., Kumar A., Tiwari S., Nair R. M. (2023). Plant growth-promoting rhizobacteria: Salt stress alleviators to improve crop productivity for sustainable agriculture development. Front. Plant Sci. 13, 1101862. doi: 10.3389/fpls.2022.1101862
Leach J. E., Triplett L. R., Argueso C. T., Trivedi P. (2017). Communication in the phytobiome. Cell 169 (4), 587–596. doi: 10.1016/j.cell.2017.04.025
Liu Y., Xun W., Chen L., Xu Z., Zhang N., Feng H., et al. (2022). Rhizosphere microbes enhance plant salt tolerance: Toward crop production in saline soil. Comput. Struct. Biotechnol. J. 20, 6543–655. doi: 10.1016/j.csbj.2022.11.046
López-Bucio J., Pelagio-Flores R., Herrera-Estrella A. (2015). Trichoderma as biostimulant: exploiting the multilevel properties of a plant beneficial fungus. Scientia Hortic. 196, 109–123. doi: 10.1016/j.scienta.2015.08.043
Ma Y., Freitas H., Dias M. C. (2022). Strategies and prospects for biostimulants to alleviate abiotic stress in plants. Front. Plant Sci. 13, 1024243. doi: 10.3389/fpls.2022.1024243
Magan N. (2020). Importance of ecological windows for efficacy of biocontrol agents. How Res. Can. Stimulate Dev. Commercial Biol. Control Against Plant Dis., 21 1–14. doi: 10.1007/978-3-030-53238-3_1
Mhlongo M. I., Piater L. A., Madala N. E., Labuschagne N., Dubery I. A. (2018). The chemistry of plant–microbe interactions in the rhizosphere and the potential for metabolomics to reveal signaling related to defense priming and induced systemic resistance. Front. Plant Sci. 9, 112. doi: 10.3389/fpls.2018.00112
Mishra J., Fatima T., Arora N. K. (2018). Role of secondary metabolites from plant growth-promoting rhizobacteria in combating salinity stress. Plant Microbiome: Stress Response, 5 127–163. doi: 10.1007/978-981-10-5514-0_6
Mishra D., Kumar A., Tripathi S., Chitara M. K., Chaturvedi P. (2021). Endophytic fungi as biostimulants: An efficient tool for plant growth promotion under biotic and abiotic stress conditions. Biostimulants Crops Seed Germination to Plant Development Elsevier, 365–391. doi: 10.1016/B978-0-12-823048-0.00019-8
Mitter E. K., Tosi M., Obregón D., Dunfield K. E., Germida J. J. (2021). Rethinking crop nutrition in times of modern microbiology: innovative biofertilizer technologies. Front. Sustain. Food Syst. 5, 606815. doi: 10.3389/fsufs.2021.606815
Mostofa M. G., Rahman M. M., Ghosh T. K., Kabir A. H., Abdelrahman M., Khan M. A. R., et al. (2022). Potassium in plant physiological adaptation to abiotic stresses. Plant Physiol. Biochem. 186, 279–289. doi: 10.1016/j.plaphy.2022.07.011
Munns R., Tester M. (2008). Mechanisms of salinity tolerance. Annu. Rev. Plant Biol. 59, 651–681. doi: 10.1146/annurev.arplant.59.032607.092911
Nguyen N. H., Vu N. T., Cheong J.-J. (2022). Transcriptional stress memory and transgenerational inheritance of drought tolerance in plants. Int. J. Mol. Sci. 23 (21), 12918. doi: 10.3390/ijms232112918
Pandey P., Srivastava S., Pandey A. K., Dubey R. S. (2020). Abiotic-stress tolerance in plants-system biology approach. Plant Life Under Changing Environ., 577–609. doi: 10.1016/B978-0-12-818204-8.00025-4
Park H. J., Kim W.-Y., Yun D.-J. (2016). A new insight of salt stress signaling in plant. Molecules Cells 39 (6), 447. doi: 10.14348/molcells.2016.0083
Parvaiz A., Satyawati S. (2008). Salt stress and phyto-biochemical responses of plants-a review. Plant Soil Environ. 54 (3), 89. doi: 10.17221/2774-PSE
Praveen A., Singh S. (2023). The role of potassium under salinity stress in crop plants. Cereal Res. Commun., 1–8. doi: 10.1007/s42976-023-00393-3
Roetzer A., Gabaldón T., Schüller C. (2011). From Saccharomyces cerevisiae to Candida glabrata in a few easy steps: important adaptations for an opportunistic pathogen. FEMS Microbiol. Lett. 314 (1), 1–9. doi: 10.1111/j.1574-6968.2010.02102.x
Roy S., Chakraborty A. P., Chakraborty R. (2021). Understanding the potential of root microbiome influencing salt-tolerance in plants and mechanisms involved at the transcriptional and translational level. Physiol. Plantarum 173 (4), 1657–1681. doi: 10.1111/ppl.13570
Saibi W., Brini F. (2021). Ion transporters and their molecular regulation mechanism in plants. J. Plant Sci. Phytopath 5, 28–43. doi: 10.29328/journal.jpsp.1001058
Sampangi-Ramaiah M. H., Jagadheesh P.D., Jambagi S., Vasantha Kumari M., Oelmueller R., Nataraja K. N., et al. (2020). An endophyte from salt-adapted Pokkali rice confers salt-tolerance to a salt-sensitive rice variety and targets a unique pattern of genes in its new host. Sci. Rep. 10 (1), 3237. doi: 10.1038/s41598-020-59998-x
Sánchez-Bermúdez M., Del Pozo J. C., Pernas M. (2022). Effects of combined abiotic stresses related to climate change on root growth in crops. Front. Plant Sci. 13, 918537. doi: 10.3389/fpls.2022.918537
Sandberg T. E., Salazar M. J., Weng L. L., Palsson B. O., Feist A. M. (2019). The emergence of adaptive laboratory evolution as an efficient tool for biological discovery and industrial biotechnology. Metab. Eng. 56, 1–16. doi: 10.1016/j.ymben.2019.08.004
Seguel A., Castillo C., Morales A., Campos P., Cornejo P., Borie F. (2016). Arbuscular Mycorrhizal symbiosis in four Al-tolerant wheat genotypes grown in an acidic Andisol. J. Soil Sci. Plant Nutr. 16 (1), 164–173. doi: 10.4067/S0718-95162016005000013
Shabala S. (2000). Ionic and osmotic components of salt stress specifically modulate net ion fluxes from bean leaf mesophyll. Plant Cell Environ. 23 (8), 825–837. doi: 10.1046/j.1365-3040.2000.00606.x
Sharma M., Kumar P., Verma V., Sharma R., Bhargava B., Irfan M. (2022). Understanding plant stress memory response for abiotic stress resilience: Molecular insights and prospects. Plant Physiol. Biochem. 179, 10–24. doi: 10.1016/j.plaphy.2022.03.004
Shukla P. S., Borza T., Critchley A. T., Hiltz D., Norrie J., Prithiviraj B. (2018). Ascophyllum nodosum extract mitigates salinity stress in Arabidopsis thaliana by modulating the expression of miRNA involved in stress tolerance and nutrient acquisition. PloS One 13 (10), e0206221. doi: 10.1371/journal.pone.0206221
Slama I., Abdelly C., Bouchereau A., Flowers T., Savouré A. (2015). Diversity, distribution and roles of osmoprotective compounds accumulated in halophytes under abiotic stress. Ann. Bot. 115 (3), 433–447. doi: 10.1093/aob/mcu239
Souza R. D., Ambrosini A., Passaglia L. M. (2015). Plant growth-promoting bacteria as inoculants in agricultural soils. Genet. Mol. Biol. 38, 401–419. doi: 10.1590/S1415-475738420150053
Tabassum T., Farooq M., Ahmad R., Zohaib A., Wahid A. (2017). Seed priming and transgenerational drought memory improves tolerance against salt stress in bread wheat. Plant Physiol. Biochem. 118, 362–369. doi: 10.1016/j.plaphy.2017.07.007
Tahir J., Belgacem A. O., Jibran R. (2022). Realizing food security in saline environments in a changing climate: mitigation technologies. Food Security Biodivers. Climate Nexus, 383–403. doi: 10.1007/978-3-031-12586-7_20
Talaat N. B., Shawky B. T. (2015). “Plant-microbe interaction and salt stress tolerance in plants,” in Managing salt tolerance in plants: molecular and genomic perspectives (Boca Raton: CRC Press/Taylor & Francis Group), 267–289.
Tan Y.-S., Zhang R.-K., Liu Z.-H., Li B.-Z., Yuan Y.-J. (2022). Microbial adaptation to enhance stress tolerance. Front. Microbiol. 13, 888746. doi: 10.3389/fmicb.2022.888746
Tang W., Zhang H., Wang L., Qian H. (2014). New cationic antimicrobial peptide screened from boiled-dried anchovies by immobilized bacterial membrane liposome chromatography. J. Agric. Food Chem. 62 (7), 1564–1571. doi: 10.1021/jf4052286
Thirkell T. J., Charters M. D., Elliott A. J., Sait S. M., Field K. J. (2017). Are mycorrhizal fungi our sustainable saviours? Considerations for achieving food security. J. Ecol. 105 (4), 921–929. doi: 10.1111/1365-2745.12788
Upadhyay S., Singh J., Singh D. (2011). Exopolysaccharide-producing plant growth-promoting rhizobacteria under salinity condition. Pedosphere 21 (2), 214–222. doi: 10.1016/S1002-0160(11)60120-3
Vadez V., Berger J. D., Warkentin T., Asseng S., Ratnakumar P., Rao K. P. C., et al. (2012). Adaptation of grain legumes to climate change: a review. Agron. Sustain. Dev. 32, 31–44. doi: 10.1007/s13593-011-0020-6
Walter J., Jentsch A., Beierkuhnlein C., Kreyling J. (2013). Ecological stress memory and cross stress tolerance in plants in the face of climate extremes. Environ. Exp. Bot. 94, 3–8. doi: 10.1016/j.envexpbot.2012.02.009
Wang D., Zhang M., Huang J., Zhou R., Jin Y., Zhao D., et al. (2021). Heat preadaptation improved the ability of Zygosaccharomyces rouxii to salt stress: a combined physiological and transcriptomic analysis. Appl. Microbiol. Biotechnol. 105, 259–270. doi: 10.1007/s00253-020-11005-z
Wijesundara N. M., Rupasinghe H. V. (2019). Bactericidal and anti-biofilm activity of ethanol extracts derived from selected medicinal plants against Streptococcus pyogenes. Molecules 24 (6), 1165. doi: 10.3390/molecules24061165
Xia Y., Farooq M. A., Javed M. T., Kamran M. A., Mukhtar T., Ali J., et al. (2020). Multi-stress tolerant PGPR Bacillus xiamenensis PM14 activating sugarcane (Saccharum officinarum L.) red rot disease resistance. Plant Physiol. Biochem. 151, 640–649. doi: 10.1016/j.plaphy.2020.04.016
Xie H., Zhao W., Li W., Zhang Y., Hajný J., Han H. (2022). Small signaling peptides mediate plant adaptions to abiotic environmental stress. Planta 255 (4), 72. doi: 10.1007/s00425-022-03859-6
Yamaguchi T., Hamamoto S., Uozumi N. (2013). Sodium transport system in plant cells. Front. Plant Sci. 4, 410. doi: 10.3389/fpls.2013.00410
Yamamoto K., Matsutani M., Shiwa Y., Ishige T., Sakamoto H., Saitoh H., et al. (2020). Comparative analysis of bacterial diversity and community structure in the rhizosphere and root endosphere of two halophytes, Salicornia europaea and Glaux maritima, collected from two brackish lakes in Japan. Microbes Environ. 35 (3), ME20072. doi: 10.1264/jsme2.ME20072
Yang H., Yao S., Zhang M., Wu C. (2021). Heat Adaptation Induced Cross Protection Against Ethanol Stress in Tetragenococcus halophilu s: Physiological Characteristics and Proteomic Analysis. Front. Microbiol. 12, 686672. doi: 10.3389/fmicb.2021.686672
Yermekbay Z. H., Oleynikova Y., Saubenova M., Daugaliyeva S., Kerdyashkin A., Yelubaeva M., et al. (2022). The influence of endophytic microorganisms on salt tolerance of wheat. Microbiol. Virol. 4 (39), 88–103. doi: 10.53729/MV-AS.2022.04.07
Zandalinas S. I., Balfagón D., Gómez-Cadenas A., Mittler R. (2022). Plant responses to climate change: metabolic changes under combined abiotic stresses. J. Exp. Bot. 73 (11), 3339–3354. doi: 10.1093/jxb/erac073
Keywords: agricultural biotechnology, plant growth-promoting (PGP) traits, salt tolerance, salt-adapted biostimulant microorganisms, endophytes salinity stress, mitigation strategies, microbiome, adaptation
Citation: Margarita S, Sviatoslav M, Yelena O and Makhpal Y (2023) Can salt-adapted microorganisms alleviate salt stress in plants and enhance their non-specific resilience? Front. Agron. 5:1287108. doi: 10.3389/fagro.2023.1287108
Received: 01 September 2023; Accepted: 09 October 2023;
Published: 24 October 2023.
Edited by:
Antonio Rafael Sánchez-Rodríguez, University of Cordoba, SpainReviewed by:
Antonio Cellini, University of Bologna, ItalyCopyright © 2023 Margarita, Sviatoslav, Yelena and Makhpal. This is an open-access article distributed under the terms of the Creative Commons Attribution License (CC BY). The use, distribution or reproduction in other forums is permitted, provided the original author(s) and the copyright owner(s) are credited and that the original publication in this journal is cited, in accordance with accepted academic practice. No use, distribution or reproduction is permitted which does not comply with these terms.
*Correspondence: Maksimovich Sviatoslav, c2V2ZWdAeWEucnU=