- Institute of Urban Water Management and Environmental Engineering, Ruhr-Universität Bochum, Universitätsstraβe, Bochum, Germany
The traditional linear model in agriculture based on the so-called ‘take-make-waste’ has created many problems such as resource scarcity, waste generation, climate change and biodiversity loss. Recently, with the increase in public awareness, the attentiveness in developing a circular economy model was doubled with a focus on proper waste management to bring some benefits to the agricultural sector. Although the increasing acceptance of biochar as a carbon-based material capable of playing a multidimensional role in reducing waste, mitigating climate change, and creating a closed-loop agricultural system, it is still far to move to a final conclusion that biochar application in agriculture could bring attractive environmental and economic benefits. Research conducted so far has led to many insights into how to enhance agricultural sustainability through biochar application, as the impact of biochar is strongly interrelated to their inherent properties, which vary deeply with the nature of biomass and the preparation conditions. In the present study, a systematic literature review was performed to investigate the state- of-the-art research related to the application of biochar in agriculture and its contribution in the establishment of circular economy concept. The interlinking between biochar application in agriculture with energy-water systems and its contribution to successfully build up a circular economy model has also been investigated.
1 Introduction
Today’s world is facing great challenges related to the increase in anthropogenic activities threatening the environment and the sustainability of various ecosystems. Agricultural sector is the major consumer of water and energy, and it accounts for more than 90% of the global environmental impacts (Ellen MacArthur Foundation, 2019). It has become more urgent than ever to introduce new alternative strategies that encourage the establishment of more sustainable agricultural practices aiming the mitigation of emissions and the proper management of agricultural wastes, while improving the agricultural productivity to respond to the continuous increase in food demand without negatively affecting ecosystems and natural resources. In the same green economy framework, the concept of circular economy (CE) is gaining momentum as an environmentally sustainable model that promote the regeneration, restoration and the rational use of natural resources (Sarkar et al., 2022b). The main objective of this strategy is to close the loops on the previous linear processes by considering wastes as alternative resources that could be recycled as value-added products and consumed (Sarkar et al., 2022a). Among many scenarios, the conversion of biomass into biochar is considered as a valuable and sustainable option for waste management within the recently promoted CE concept (Figure 1). The conversion of biomass into biochar implies a series of complex chemical reactions including decomposition, depolymerization and condensation under high temperatures (Wang and Wang, 2019; Hu et al., 2021). In addition to be cheap and simple to produce, biochar chemical and physical properties including its cation exchange capacity (CEC), its hydrophobicity and its porosity and surface area, reveal its broad application prospects, which is continuing to expand (Zornoza et al., 2016). Thus, in addition to its use as construction material and as an adsorbent for wastewater treatment, the expanded application of biochar in agriculture has been recently growing (Enaime et al., 2020a; Hu et al., 2021). Biochar application in soil could improve its water retention capacity, increase nutrients availability and reduce greenhouse gas emissions. Biochar could also be used to alleviate environmental issues due to its adsorption capacity towards pollutants in soil, this could be due to the high content of oxygen containing groups on its surface (Song et al., 2021). The reincorporation of biomass in the soil in the form of biochar is considered as a blocking pathway for the natural carbon cycle, allowing the sequestration of atmospheric CO2 in a long-term stable holder (Woolf et al., 2016). Biochar produced from agricultural wastes may also be used for energy production at local level, which helps in creating a certain energy independence particularly in rural agricultural regions (Ahsan et al., 2022). Although the numerous reports currently available on the production of biochar and the benefits of its application in agriculture from different and specific aspects (Allohverdi et al., 2021; Enaime and Lübken, 2021), people still consider that biochar specific impact on agriculture productivity is not enough demonstrated to spend for it. This review aims to highlight the CE-based environment management aspect of biochar. Biochar properties and their specific benefits when applied in agriculture have been discussed based on previous studies in a CE context. The main challenges affecting the commercialization of biochar and its wide spread application in agriculture are also provided.
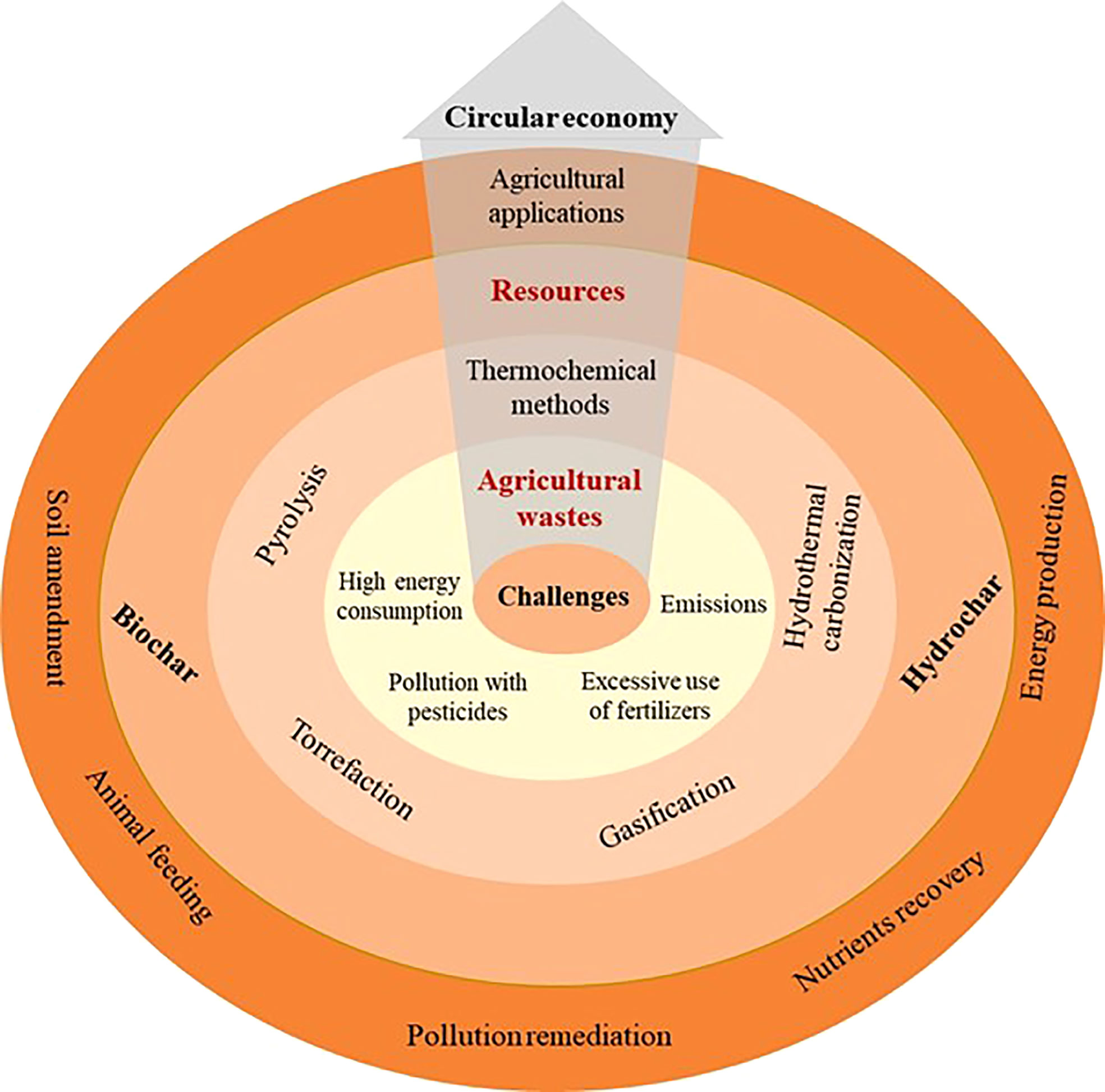
Figure 1 Biochar application in agriculture based on the waste-to-resource model within the CE context.
2 Role of CE for sustainable agriculture
To responds to the forecasted increase in world population and then to the projected increasing demand for food, agricultural and food world production must increase by 70% by 2050 (Food and Agriculture Organization of the United Nations, 2009). This urgent increase will certainly create an unbalance in the long-term availability of natural resources (Velasco-Muñoz et al., 2021). The magnitude of this unbalance is doubled by the impacts of climate change; especially the increase in temperature and the change in global precipitation patterns (Nelson et al., 2009). The increase in agricultural and food production is also accompanied by an inevitable increase in the production of waste. For instance, Europe is annually producing 1.3 billion tons of waste, of which 700 million tons are generated from the agricultural activities (Pavwelczyk, 2005). It is believed that agriculture is an important contributor to global pollution, natural resources stress, loss of biodiversity and ecosystems toxicity due to the excessive use of inorganic fertilizers, which contributed immensely to soil and water pollution (Ellen MacArthur Foundation, 2019; Gogoi et al., 2019). A degradation of soil quality (soil acidity, depletion of soil organic matter stock, soil erosion) could also be claimed after a long-term cultivation, which can affect the agricultural productivity (De Meyer et al., 2011). Agriculture is also the main contributor to the anthropogenic greenhouse gases (GHGs) (about 25%) and to the total global anthropogenic methane and nitrous oxide emissions (52 and 84%) (Smith et al., 2007; Smith et al., 2008). Out of the above challenges can emerge an opportunity to decouple the agronomic development from the traditional linear production models essentially based on the extraction of natural resources and their conversion into products and wastes (Murray et al., 2015), and to move into CE models aiming especially in saving resources and reducing environmental impacts of the agricultural sector while ensuring a satisfying economic performance (Kuisma and Kahiluoto, 2017). Development of CE models can also provide value through the creation of new industries and jobs. CE as described by Ellen MacArthur Foundation (2013) is “an economic system of closed loops in which raw materials, components and products keep their quality and value for the longest possible and systems are fueled by renewable energy sources”. In other words, CE aims to develop a model that can effectively reduce the use of natural resources and the production of wastes, while designing the use of these latter as valuable co-products in various systems (Toopa et al., 2017). The CE is recently promoted in the agriculture for its ability to transform challenges into solution. This concept could be established by the conversion of agriculture residues or wastes from other sectors into valuable products that could be utilized for multiple agricultural and environmental purposes, leading to improved processes and to the creation of new businesses as well.
In the framework of CE, several technologies either mature or under development are suggested to convert food wastes into organic fertilizers, medicines, and bioenergy. Thermochemical conversion of wastes into biochar has been suggested as a sustainable strategy for the management of the agricultural wastes annually generated in farms (Enaime and Lübken, 2021). Sustainable agriculture involving biochar application can be performed in smaller scale considering the farming system as a closed area that should exploit their self-wastes and convert them into biochar to sustain itself over a long period of time. This enables them to protect their productive area by conserving soil fertility, reducing the impact of agricultural activities on surface and groundwater resources, developing new sustainable energy sources, and reliving challenges related to climate change (Semida et al., 2019). The reintegration of agricultural wastes in the form of biochar in different agricultural activities is perfectly in line with the principles of CE in creating of closed-loop agricultural systems (Ellen MacArthur Foundation, 2012; World Economic Forum, 2014). This will allow increasing the value of agricultural wastes, reducing their impacts while enhancing the agricultural production. The second approach considers that agriculture should also contribute to the establishment of sustainability in wider level. This approach does not separate rural and urban areas and considers that agriculture should help urban areas to manage their wastes by converting and reintegrating them in different agricultural activities.
3 Biochar production and properties
Biochar is a solid, carbon-rich material produced by thermochemical decomposition of biomass in oxygen-depleted atmosphere (Tripathi et al., 2016). Biochar properties are directly related to its chemical composition in organic and inorganic constituents. The organic matter of biochar is dominated by carbon, oxygen, hydrogen, sulfur and nitrogen, while the inorganic fraction, which is in the form of ash, is essentially composed of silica, aluminum, calcium, magnesium, phosphorus, sodium and potassium (Jindo et al., 2020). Hydroxyl, carbonyl, epoxy, carboxyl, ether, ester, sulphonic, aliphatic, phenolic and aromatic C groups are the main functional groups identified on biochar surface (Yuan et al., 2019). Mekuria and Noble (2013) reported that biochar with high content of carboxyl and phenolic C groups exhibits high CEC and then a high affinity for the adsorption of nutrients. Biochar properties vary significantly depending on the raw biomass, the thermochemical conversion method used and the preparation conditions, especially temperature, reaction time, heating rate, and ventilation conditions (Tang et al., 2013). The selection of the process and process parameters is performed based on the nature and properties of biomass and the desired final-products like biochar, biogas and bio-oil (Singh et al., 2022). Pyrolysis, torrefaction, gasification and hydrothermal carbonization (HTC) are generally used for the conversion of biomass into biochar (Enaime et al., 2020a). Pyrolysis is the most common method used to convert biomass into biochar (Qian et al., 2015). The effect of pyrolysis temperature is critical in designing specific biochar for specific agricultural purposes (Jindo et al., 2020). The increase in pyrolysis temperature induces an increase in biochar aromatic C content (ranging from 17 to 85%) (Domingues et al., 2017; Solaiman et al., 2019), whilst other properties such as O/C and H/C ratios and the content of carboxyl groups decrease (Yuan et al., 2019; Jindo et al., 2020). Biochars produced at high pyrolysis temperatures have lower CEC than low temperature biochars (Gai et al., 2014). The nature of feedstock is also affecting biochar CEC. For instance, biochars produced from woody exhibited lower CEC than those derived from manure waste (Jindo et al., 2020). Other parameters such as reaction time and heating rate are reported influencing biochar decomposition rate when applied to soil. Based on these two parameters, pyrolysis can be subdivided into slow pyrolysis (long residence time; > 1 h and low heating rates; 5 to 10° C/min) and fast pyrolysis (residence time less than 10 s and heating rates over 200°C/min) (Qian et al., 2015; Zhang et al., 2019; Zhang et al., 2019). Biochar produced via fast pyrolysis has a slower rate of decomposition in soil than biochar formed through slow pyrolysis and could provide a beneficial effect of amendment that can for long lasted (Jacobs et al., 2015; Ok et al., 2016). Similar to slow pyrolysis, torrefaction is usually carried out at a slow heating rates and temperatures ranging from 200 to 300°C under an inert atmosphere by involving various decomposition reactions. However, torrefaction is generally applied to upgrade the thermochemical properties of the biomass allowing the production of biochars with high calorific values since this process induces only 30% weight loss, with only 10% of the energy initially present in the biomass is released in the form of gas (Kambo and Dutta, 2015; Yu et al., 2017). In other hand, gasification is principally used to convert biomass into a mixture of combustible gases (mainly carbon monoxide and hydrogen and small amounts of carbon dioxide, methane, water and hydrocarbon derivatives) under a controlled flow of oxidizing agent at high temperatures (>700°C) (Qian et al., 2015; Weber and Quicker, 2018). Tay et al. (2013) reported that the treatment of Victorian brown coal by gasification at 800°C in the presence of steam as an oxidating agent decreased the relative ratio of small and large aromatic ring structures in biochar and reduced the volatization of Mg and Ca ions during the thermal process. Fewer attention has been, however, dedicated to the mechanism involved in the formation of biochar by gasification and its application in agriculture, considering that biochar is produced as a secondary product with a lower yield (about 10% (w/w)) as compared to that of gaseous by-product. In comparison to other conventional technologies typically used for low-moisture biomass, HTC is an effective and economical method in processing high-moisture biomass (Wilk and Magdziarz, 2017; Lee et al., 2018; Enaime et al., 2020b). Under HTC conditions, biomass is pressurized in aqueous medium at temperatures ranging from 180 to 250°C and do not need an intensive predrying step of the feedstock, which decreases the cost and energy of the process (Libra et al., 2011; Yu et al., 2017). Hydrochar is the desired product in the HTC process, recovered with a yield of about 40-70%, which is higher than that of pyrolysis biochar (Chen et al., 2017). Liu and Balasubramanian (2014) assumed that with the same mass yield, the densification ratio achieved by HTC is higher than that of pyrolysis. Even though that both biochar and hydrochar can be utilized in agriculture for similar purposes and can act as multi-functional soil amendments affecting structural, physico-chemical and microbiological properties of soil, their physical and chemical properties significantly differ according to the nature of biomass and the preparation condition (Hitzl et al., 2015; Yihunu et al., 2020). While biochar is a pyrogenic carbonaceous material rich in aromatic compounds and exhibiting a high degree of porosity, hydrochar is hydrophobic, more energy dense and rich in functional groups (Wiedner et al., 2013; Yihunu et al., 2019). The abundance of oxygen-containing groups on hydrochar surface improves its CEC and affinity to water, which affect positively the water holding capacity of the soil (Zhang et al., 2019). Hydrochar is generally considered less stable and more easily decomposable compared to biochar due to its high labile carbon content and its low content in aromatic compounds (Gascó et al., 2018). The lower temperature used in the HTC process simulates the natural process of humification and the conversion of biomass into materials with properties similar to those of peat (Álvarez et al., 2017). Although biochar is recognized for its resistance to biodegradation, the presence of bioavailable organic components in its structure was also reported (Rombolà et al., 2016). Biochars/hydrochars produced from different precursor and by different production methods were found to contain adsorbed volatile organic compounds, polycyclic aromatic hydrocarbons and numerous potentially toxic organic and inorganic elements (Spokas et al., 2011; Becker et al., 2013; Buss et al., 2015; Chakrabarti et al., 2015). The content of these compounds in most chars have been however reported under the threshold values recommended by International Biochar Initiative (IBI) and European Biochar Certificate (EBC) (Wiedner et al., 2013).
4 Contribution of biochar in agriculture sustainability
As described above, agriculture is facing serious environmental and economic challenges. The rising concerns about these global issues exerted more pressure on agricultural actors to make key changes in the management of their activities to achieve sustainability and economic improvement. The interest in searching naturally derived and/or organic materials and its application in agriculture has notably increased in the past few years. Manures and sewage sludge composts have been used as alternative organic biofertilizer that could effectively substitute inorganic fertilizers. These residues contain, however, pathogens and its improper application in soil may induce negative effects such as soil acidification, release of GHGs and contamination of surface water and groundwater (Lehmann et al., 2011). Biochar application in agriculture is alternatively receiving increasing interest for its beneficial effect from both agronomical and environmental prospects (Calvo et al., 2014; Akhtar et al., 2015). As reviewed by Enaime and Lübken (2021), biochar can be a multi-functional player in the CE through its application in agriculture as a soil amendment, for animal feeding and for remediation purposes.
The lifecycle of biochar in soil depends strongly on the rate of biochar degradation and on the degree of soil fertility (Allohverdi et al., 2021). Carbon sequestration rate increases at lower decomposition rates (Roy and Dias, 2017). Xu et al. (2018) amended four paddy rice fields and 3 maize fields in mainland China with biochar. Authors observed a reduction in carbon footprint by 20.37–41.29 t CO2 equivalent per ha for paddy rice and 28.58–39.49 t CO2 equivalent per ha for maize production, as compared to the experiment without biochar addition. In another investigation, Spokas and Reicosky (2009) studied the effect of the addition of 16 different types of biochar on GHG release. Most chars evaluated reduced the rate of net CH4 oxidation in soil, decreased CH4 production in an initial CH4 producing soil. Biochar can use soils as a receptacle not only to sequester CO2 but also other GHG such as nitrous oxide. Nitrogen-rich-feedstock such as chicken litter, animal manure and municipal sewage sludge allow the transfer of nitrogen to the plants (Solaiman and Anawar, 2015). In a study performed by Liu et al. (2020), a low organic matter sandy-loam soils were amended with straw derived biochar. Authors observed a great decrease in N2O emissions, a slight increase in crop yields but no GWP decrease after biochar treatment for over five rotation year. In addition to its contribution to carbon sequestration, the carbon footprint of biochar must also include biochar production process. Spokas and Reicosky (2009) reported that the GHG released during biochar production are compensated when biochar is applied as soil amendment. Global warming potential (GWP) measures how much energy the emissions of one ton of carbon dioxide will absorb over a given period of time (Woolf, 2008). The source of the feedstock and its growth conditions are important factors affecting the overall GWP of biochar. Feedstocks originated from forests and energy crops cultivated on marginal land could create a carbon debt (Roy and Dias, 2017). Additional GHG impacts could also be unintendedly produced as a result of the expansion of bioenergy crops on productive agricultural lands (Sanscartier et al., 2014).
Besides carbon sequestration, the application of biochar in agricultural soils improves several soil characteristics such as water and nutrients retention capacity, soil structure, soil organic matter and the activity of soil microbial communities, which also increases crop yields (Table 1). Application of biochar to soils can decrease fertilizers leaching to surface and groundwater, allowing a promising soil’s fertility management (Glaser et al., 2002; Lone et al., 2015). Furthermore, biochar could be used as a filler in nitrogen-rich compost processes, improving then the degradation rate and reducing odor emissions and loss of nitrogen (Dias et al., 2010). Liu et al. (2017) reported that the addition of biochar to poultry manure at a rate of 10% during composting allowed the highest OM degradation rate and the lowest ammonia and GHG emissions with an ideal cost. Biochar also gained attention for its higher capability to reduce pesticide bioavailability, due to their polar and non-polar groups (Khorram et al., 2016; O’Connor et al., 2018). The improvement in soil characteristics and the remediation of soil will definitely induce an increase in crops production. For instance, Srinivasarao et al., 2014) observed a significant increase in the rain fed crops yield following the addition of 1000 kg/ha of organic matter in the form of carbon in the root zone. The beneficial effect of biochar application on crop productivity also includes its contribution in protecting the environmental system. For example, raising crop yields within the existing agricultural lands without expansion to new lands allows protecting natural ecosystems from intensive agricultural activities (Allohverdi et al., 2021).
As described above, soil amendment with biochar is a win–win strategy, allowing both carbon sequestration and crop production increase. However, it might not be realistic to simultaneously have both benefits in their maximum level. Biochar intended to carbon sequestration has less impact on soil quality, while biochar designed to maximize crop yield present a limited carbon sequestration potential (Jeffery et al., 2015). The biochar in the soil undergoes a natural aging process inducing an increase in its surface area and ability to adsorb nutrients. Prior chemical oxidation can further accelerate the natural aging process of biochar, which can further improve cation adsorption capacity and reduce nutrient leaching. However, the oxidation process simultaneously results in carbon loss and therefore reduces the potential for carbon storage in soil (Jeffery et al., 2015). The oxidation of biochar may also reduce the resistance of biochar to decomposition, which can further reduce the potential of carbon storage in soil (Nguyen et al., 2010).
Agricultural sector could be then considered as an area where biochar can be used in huge quantities to sequester carbon and to simultaneously creates an economic and sustainable strategy for agricultural wastes management. In the same line, biochar application could be a promising method to restore degraded lands and maintain their long-term fertility. Adding biochar can also help improve crop yield by improving water and nutrient retention in soils without the need of intensive addition of chemicals and synthetic fertilizers. All these aspects could contribute to ensuring the sustainability of agriculture and supporting the circular economy model, especially in regions characterized by limited natural resources, water scarcity and restricted access to fertilizers. However, it is still difficult to fully understand and evaluate the specific role of biochar in the sustainability model and to measure the magnitude of its impact on agriculture in long term prospects.
5 Coupling biochar application in agriculture with energy-water systems in CE perspectives
Further improvements of biochar application are suggested to better build up the principles of the CE. This will open the door for more sustainable management of agricultural wastes by combining different technique and innovative strategic approaches (Singh et al., 2022). Anaerobic digestion (AD) of agricultural by-products contributes not only to the reduction of GHG emitted by agricultural activities, but also allows the production of biogas that can be subsequently used as a biofuel with high energy content. The application of AD for the management of agricultural wastes enhances then the contribution of the agricultural sector in producing clean energy and protecting ecological systems (Monlau et al., 2013; Sambusiti et al., 2013; Enaime et al., 2020c). There is currently considerable potential for biogas technology using a variety of digestible residues; including agricultural wastes such as straw, animal and poultry manure and livestock farming (Lübken et al., 2007; Wichern et al., 2008). When considering the global valorization of all side-streams, AD contributes only partially to the resolution of agricultural waste management problem, as a significant fraction of waste organic content, such as polysaccharides and lignin, remains in the produced digestate (Santi et al., 2015; González et al., 2020). Although the increasing efforts made to promote the implementation of AD on broader scale, clear and consistent directions on the subsequent use of the obtained digestate are still not sufficiently presented (Saveyn and Edder, 2014). Digestate is rich in valuable nutrients such as nitrogen, phosphorous and potassium. The conventional recycling model lying in the direct utilization of digestate as soil conditioner in agriculture and horticulture and/or as fertilizer. Although it can promote the growth of crops, the direct use of digestate may also cause secondary pollution generally related to its odor and its content in pathogens and heavy metals (Hung et al., 2017). Nutrients and heavy metals contained in the digestate could be easily leached from the soil due to their relatively high mobility (Hsu and Lo, 2001; Monlau et al., 2014). The agronomic application of digestates may also be restricted in some countries, where composts produced from biowaste or biological sludge are alternatively well commercialized (Taurino et al., 2016; Hung et al., 2017). In addition, excessive production of digestate in some intensive farming regions where biogas plants are more centralized can lead to an oversupply of digestate, which should be transported to regions with nutrients deficit if the original producing areas cannot fully manage these large amounts of digestate (Kuligowski and Luostarinen, 2011; Lacroix et al., 2014). Therefore, to enhance the role of biogas plants in solving environmental issues and ensure an effective energy transition, it is necessary to develop new combination of technologies to allow at the same time the management of the secondary pollution associated with biogas digestate and promote its commercialization (Song et al., 2021).
Based on the recycling model that couples biogas and biochar technologies, many investigations have been performed to evaluate the effect of their combination on SOC, soil pH and nutrients and crops production. Greenberg et al. (2019) showed that the simultaneous application of biochar and digestate was effective in improving SOC. Similarly, Roberto et al. (2018) reported that the application of digestate combined to biochar allowed an increase in SOC content by more than 82% of the initial amount and a decrease in the pH to 6.5 assuring an alkaline environment of the soil. Ge et al. (2016) and Wang et al. (2018) also showed that the addition of biochar and biogas slurry significantly reduced soil nutrient loss and enhanced the content of active organic carbon and the fertility of soil. In another study, Zhang et al. (2015) reported that the application of biochar and biogas slurry allowed an average increase in apple fruit yield by 58.5%, vitamin C content by 47.8%, and soluble sugar content by 17.4%, which was significantly higher than using biochar and biogas slurry in single applications. Considering the aforementioned findings, the benefit effect of applying biochar together with biogas residues on soil quality can be clearly established. Another approach was also suggested considering the large surface area and porosity of biochar that could be used for nutrient recovery from digestate and the retained nutrients could be slowly available for plant after biochar application to soil. In this context, Oh et al. (2014) impregnated biochar with anaerobically digested slurry and successfully produced a nutrient-enriched biochar, which was subsequently used as a slow-release fertilizer. This sustainable concept could assure the supply of all essential nutrients for plant growth while maintaining soil fertility and preventing nutrient leaching.
The conversion of digestate into a solid stable carbon material was also suggested as a promising solution to enhance their properties before its application to soil. The technological methods used to enhance digestate properties should be low-cost and capable of dealing with a variety of inputs to achieve significant market penetration. Thermal processes, such as pyrolysis, torrefaction and gasification have been largely used as a complementary to AD for the valorization of anaerobic digestate (Monlau et al., 2015a; Sheets et al., 2015). Many investigations have used pyrolysis for the conversion of solid digestate into biofuels such as bio-oil, gases, and biochar (Troy et al., 2013; Li et al., 2014; Monlau et al., 2015a). In addition to biofuel application, pyrolytic biochar could also be utilized as a complement to mineral fertilizers, or as soil amendment (Monlau et al., 2015a). In isolated agricultural areas with intensive activities, the implementation of AD plants combined with thermochemical processes, can guarantee partial reuse of excess heat (Monlau et al., 2015b). The use of digestate-based biochar provides several benefits to soil including the increase in CEC and soil pH, the increase in water holding capacity, the reduction of GHG emissions and the reduction in nitrogen and other nutrients leaching into groundwater, which also enhances crop productivity (Andert and Mumme, 2015; Dicke et al., 2015; Antoniou et al., 2019). These beneficial effects are mainly related to biochar properties. Inyang et al. (2010) showed that biochar produced from bagasse digestate had basic pH, higher surface area and CEC and more negative surface charge as compared to biochar derived from raw bagasse. Although the previously reported benefits, there some investigations reporting some problematic effects of digestate-derived biochar application. The high content of digestate in ash and nitrogen results in high ash biochar (Neumann et al., 2015; Opatokun et al., 2015; Monlau et al., 2016). Moreover, there is uncertainty with some digestate-derived biochars, if the mineral matter and plant nutrients are crop available when added to soils (Monlau et al., 2016). Low temperature gasification assays of anaerobically digested materials, performed at pilot scale, also allowed the production of chars with high nutrient content (Judex et al., 2012; Pecchi and Baratieri, 2019), but also enriched with high levels of ash (Antoniou et al., 2019). Some authors also reported the potential existence of toxic elements such as polycyclic aromatic hydrocarbons (PAH), which make of its application a challenging issue (Rollinson, 2016). This effect could be, however, not generalized and the bioavailability of these elements and their effect on crops growth are so far uncertain. Within the innovative recycling model, digestate-based biochar could also be returned to anaerobic reactor; biochar added to anaerobic systems was shown to enhance biogas production and improve the reactor performances. Some previous investigations demonstrated that biochar produced from digestate is superior to that directly produced from biomass, in term of physico-chemical properties (pH, CEC, hydrophobicity) and adsorption behavior (Song et al., 2021).
One of the barriers limiting the adoption of pyrolysis for the treatment of digestate on an industrial scale include its high moisture content. Alternatively, HTC is a potential route in upgrading digestate properties. The high content of HTC liquid phase in solubilized organic material initially contained in the digestate creates an opportunity in solving the black beast of the HTC process by recycling it back into HTC or treating it with AD permitting a potential increase in the biogas yield (Parmar and Ross, 2019). Recently, more focus has been dedicated to the hydrothermal conversion of solid digestate into hydrochar and its use for energy production or for agronomic purposes. Reza et al. (2015) proceeded to the hydrothermal treatment of wheat straw digestate at temperatures ranging 180–260°C. Authors reported that 220°C-hydrochar contained primarily crystalline cellulose and lignin, while that produced at 260°C exhibited less crystalline cellulose and more aliphatic carbon and lignin contents. In another study, Mumme et al. (2011) showed that hydrothermal treatment of digested maize silage allowed the production of hydrochars with distinct physico-chemical properties than hydrochars from undigested maize silage. The application of HTC for the treatment of anaerobic digestate has the ability to bring several benefits at once. The sanitation of digestate from bacterial pathogens and spores could be occurred during the HTC process. Moreover, an enhancement of digestate hydrophobicity is also observed, which makes of the dewatering step less energy intensive (Wang et al., 2014). Stutzenstein et al. (2018) showed that a high level of nutrient recovery could be reached when applying HTC at optimal conditions on anaerobic digestate. Authors also reported that cellulose initially contained in the digestate could be completely degraded at high temperatures and low pHs. Even the several advantages of HTC for the management of digestate and the upgrading of their properties for its further agricultural application, the environmental impact of digestate-derived hydrochar on soil can be in some cases discouraging, due to their content on some phytotoxic compounds such as PAH and phenols formed during the HTC process (Stutzenstein et al., 2018). Bargmann et al. (2013) observed an inhibition of spring barley and cress germination when hydrochar or process water were applied. Authors suggested a pre-treatment of hydrochars (washing, storage) to reduce their inhibitory effect before their addition. So, the key challenges are related to how overcome inhibition and ensure that the levels of hydrochar phytotoxicity are not exceeded.
Due to its large surface area and hydrophobic interactions, several researchers also proposed the use of biochar for the recovery of nutrients. Compared to other adsorbents such as the widely used activated carbon, biochar produced at relatively lower pyrolysis temperature have lower standard enthalpy of formations, while activated carbon requiring high temperatures (600-1200°C) and a secondary chemical or physical activation to improve their textural characteristics, demands significant energy during its production (Barber et al., 2018). Within the wastewater treatment context, biochar has been largely suggested as media in water filtration systems (Enaime et al., 2020a). However, fewer studies have investigated the subsequent use of the ‘nutrient loaded’ biochar to enhance soil nutrient content. For instance, biochar has shown high affinity for phosphorus adsorption, the application of phosphorus-enriched biochar as a slow-release fertilizer could be therefore a promising solution to improve soil quality and productivity. An et al. (2020) proceeded with the adsorption of phosphorus onto bentonite-modified biochar and its subsequent use as a controlled-release fertilizer, enabling the establishment of sustainable phosphorus circulation routes. The innovative approach of recovering biochar originally designed for a particular use and its application for another purpose is emerging. Interestingly, the use of biochar in wastewater filtration systems to produce phosphorus-enriched biochar that can replace fertilizers. The use of biochar as both a filter media and subsequently as a nutrient carrying soil amendment, makes it possible the sustainable and the circular integration of different food growing, processing and disposal activities (Barber et al., 2018). So instead of discharging nutrients-rich wastewater in ecosystems, leading to a state of eutrophication, these nutrients could be recovered by filtration on biochar and turn back to soil, which allows the development of more efficient soil fertility management practices (Tilman et al., 2013). Streubel et al. (2012) reported a phosphor recovery of about 1.9 g per kg biochar by filtration of anaerobic digest lagoon on biochar. In another study, Sarkhot et al. (2013) used biochar as a carrier media for the recovery of nutrients from dairy manure effluent, achieving a recovery of 5.3 mg/g NH4 and 0.24 g/g PO4, expecting a release of nutrients to crops after biochar application as soil amendment. Additionally, Kammann et al. (2015) observed a plant growth improvement and a slow release of nutrients retained in biochar pores during composting. Indeed, this approach is an improved sustainable opportunity for wastewater treatment on the one hand and for recycling wastewater nutrients on the other hand. The bioavailability of recovered nutrients on biochar to crops is however not yet certain; further research studies are needed to fully understand the mechanisms controlling the uptake of wastewater nutrients on biochar and its release when the nutrients-loaded biochar is applied to soil. In a study performed by Werner et al. (2018), authors reported that the application of biochar previously used as a filter media for the filtration of raw wastewater did not show any enrichment of biochar with nutrients during filtration. Authors suggested that the used wastewater was not sufficiently loaded to enrich the biochar with nutrients. Instead, authors observed a loss of nutrients from rice husk biochar during the filtration process. Nevertheless, the application of untreated biochar and biochar-filter to soil allowed an increase in crop production but no significant difference was observed between both samples. The results also showed that both treated and untreated biochars can immobilize nitrogen in soil and make it unavailable for plant. This effect is reduced by the prior use of biochar in wastewater filtration system, which allow reducing biochar sorption affinity towards mineral nitrogen compounds most likely through reducing free sorption sites.
In addition to nutrient recovery, biochar was also used as a sustainable filter media for the removal of pathogens for a safer irrigation water production (Kätzl et al., 2018; Kätzl et al., 2020). Rice husk biochar was used by Kätzl et al. (2019) in a low-cost anaerobic biofiltration system for the treatment of wastewater in Sub-Saharan Africa. A high reduction of fecal indicator bacteria and bacteriophages as well as chemical oxygen demand and turbidity (up to 97%) was observed, which led to a significantly lower contamination of soil and plants irrigated with the prefiltered wastewater.
6 Economic feasibility of biochar integration in agroecosystem
Moving toward circular models is highly required, due to the significant resource footprint and GHG emissions of the agricultural sector. The introduction of CE in agriculture is especially encouraged for the sustainable development of the sector from both environmental and economic sides. Indeed, economic benefits are always related to the prevention of environmental impacts. For instance, when soil is amended with fast pyrolyzed biochar for remediation purposes, the resulting beneficial effects could last longer (Allohverdi et al., 2021). Economic benefits could be then gained, in addition to environmental one, related to earning carbon credits by selling offsets. Scholz et al. (2014) reported that 10–70% of carbon from the original biomass could be retained in soil for decades. Van Beilen (2016) also reported that a net carbon storage of 20% could be achieved when biomass is returned to soil in the form of biochar (Van Beilen, 2016). The economic and sustainable viability of biochar production in industrial scale is strongly depending on the availability of feedstock, its transportation costs and on the infrastructure (Ahsan et al., 2022). Much of the biomass produced by photosynthesis that could be converted and commercialized as biochar is inaccessible from economic, practical or environmental considerations. These resources could stabilize around 600,000 kilotons of carbon dioxide equivalent (Kt CO2e) in the world (Van Beilen, 2016). The symbiosis of the pyrolysis facility with on-farm producing agricultural wastes and its in-site application will allow eliminating supply chain logistics costs, which could significantly improve the economic balance of biochar production and, in addition, provide a readily adaptable alternative for crop residue burning in the field (Ahsan et al., 2022). Another factor that considerably affect the energy balance, the economic efficacy of biochar production and its environmental impact is the thermochemical conversion method used. Pyrolysis has been defined as a cost-effective, energy-efficient and ecofriendly thermochemical process (Ning et al., 2013). The pyrolysis temperature influences the cost of biochar production as the latter is reduced at lower pyrolysis temperatures. Shabangu et al. (2014) showed that about 70% and 30% of the production revenue of a biochar-methanol system at 300°C pyrolysis temperature comes from selling biochar and methanol, respectively, from 30% and 70% at 450°C pyrolysis temperature and from 10% and 90% at 800°C.
The energy balance of HTC was also investigated. It was estimated that one-third of the combustion energy stored in carbohydrates is released during the hydrothermal treatment, an exothermic process, following the dehydration reaction. The recovery of this energy and its reuse could significantly reduce the input energy of the HTC process (Singh et al., 2022). Zhao et al. (2014) found that HTC of solid sludge could be an energy-self-sustainable system when performed at a temperature of 200°C during a residence time of 30 min. Authors reported that about 52.4% of heat from hydrochar combustion is sufficient to operate the HTC process including drying process and dewatering and the remaining 47.6% can be recovered as heat and/or electricity, etc. The techno-economical assessment of the HTC process was also performed by Lucian and Fiori (2017) from 20,000 tons/year capacity plant of grape marc, considering both investment and production costs. The cost of pelletized hydrochar production was estimated to be 157 €/ton and the hydrochar break-even value for a plant repayment period of 10 years was equal to 200 €/ton, which is competitive with the price of wood pellets (150–200 €/ton). In general, biochar production is economically beneficial when waste biomass is used as feedstock (Marshall et al., 2019). Beyond the costs related to biochar production, the application rate is also significantly affecting the profitability of biochar application in agriculture. The most promising results obtained under field conditions in term of crop yield improvements, were achieved at high biochar application rates (> 2.5 tons/ha) (Joseph et al., 2013). Regarding the profitability of biochar application in agriculture, Keske et al. (2020) showed that the agricultural application of biochar could be profitable until a 99% of probability. When applying 10,000 kg/ha of biochar derived from black spruce to grow beets, authors observed an increase in beet yields from 2900 kg/ha to 11,004 kg/ha, consequently, a net return of up to $4953/ha was achieved. Authors also reported that the recovery of costs could not be possible for all crops. As biochar is proved for its long-term stability, no continuous biochar application is needed. In this regard, if in-site agricultural areas cannot completely process large quantities of biochar, the excess biochar could be in-situ used for energy production or exported to regions with nutrients deficit, therefore, farmer’s income is raised. Here it is, however, necessary to consider transport costs and carefully analyze the competitiveness of the final product in relation to its final price.
The combination of energy production and biochar application in agriculture could also be used to establish the CE model. The implementation of a hydride system regrouping AD and thermochemical processes could have many benefits either for energetic or agronomic considerations. However, the overall costs of this hybrid system are still in question, especially that no clear strategy has been adopted so far to go further in its implementation on an industrial scale. The evaluation of the profitability of such a process is based on many variables including the readily supply of the digestate, the char selling price, the selling price of electricity, the transport costs, etc. However, according to González et al. (2020) the combination of digestion and pyrolysis still not profitable enough, even its benefits of improving the efficiency of electricity generation and ensuring sustainable waste management.
Even the fact that biochar application in soil could provide long-term economic benefits, biochar still seems costly for agricultural actors whose waste management is intrinsically outside the scope of their focus (Ahsan et al., 2022). More efforts are still needed to assess economic and environmental benefits of biochar implementation within the context of the CE, but especially to increase the awareness of third-party testing and the government and to go into on developing a strong collaboration to concretely develop and sustain the biochar market (Singh et al., 2022). Moreover, it is neither financially nor energetically feasible to widespread the use of biochar without guidelines or regulations for its production and rational application (Hu et al., 2021). These regulations will help reducing the health and the environmental risks related to biochar production and agronomic use and allow meeting market standards. The agricultural application of biochar is currently in a legally grey area in many countries, while in other countries more information are still needed to clearly make biochar producing industries in their legal framework and establish clear criteria for the safe use of biochar by policy makers (Wiedner et al., 2013). Currently, IBI and EBC are the most widely used international regulations. As the interest in using biochar is continually growing, many countries developed their own biochar standards aligned with those of IBI and EBC, while other countries are regulating biochar application with fertilizer or compost standards (Table 2). A version 2.1 of the Standardized Product definition and Product Testing Guidelines for Biochar application in soil was published by the IBI in 2015 (IBI, 2015), which was then recognized as an international standard (Hu et al., 2021). However, as biochar has great potential to be used in different industries, the existing standards are apparently insufficient to make relevant recommendations for biochar use in various applications. Moreover, biochars and hydrochars are defined as different materials, due to the different parameters and thermochemical reactions involved in their production (Cao et al., 2011; Wiedner et al., 2012). A specific standard for hydrochar is also necessary for the sustainable development of char industry’s.
7 Conclusion
The conversion of organic wastes to biochar and its application in agriculture gives a treatment alternative for wastes and contributes to improve the sustainability of agricultural sector and the establishment of the CE model. Biochar manufacturing system can be linked to other systems like water treatment system, by using the output of one process as an input for another. Although the practical applicability of biochar is facilitated by its simple production method and the low cost and availability of feedstock, its real application in agriculture could face some limitations. Biochar is not a standardized material; due to the large variety of feedstock and the different parameters used during the thermochemical conversion processes, biochar properties as well as its effect may differ from one composition to another. It is then necessary to investigate the connection between biochar properties and the purpose of its applications and the best combination of type of biochar and its application rate. It is also important to consider the big difference between biochar and hydrochar and that these materials may probably have a complementary reaction during their applications. Hence, specific standards and recommendation for hydrochar application are still needed. The modification of biochar to design their properties for specific agricultural applications is also still need to be further investigated to improve their performances. The comprehension of biochar production process and biochar modification to design their properties for specific agricultural applications is also still needed to be further investigated to improve biochar performances and build up a trustworthy circular economic model. Moreover, biochar’s impact on agriculture has yet to be fully understood as the majority of biochar research studies performed so far are being executed in laboratory scale. The real world is more complex, and the actual environmental impact of biochar should be further investigated based on field and site-specific research on a large-scale. Beyond the aspects related to biochar production and application, the identification of indicators that could be used to measure its effect is of great importance. The transition from linear to CE in large scale is still challenging and still require the establishment of new knowledge that should be adopted by different actors to make this transition more flexible.
Author contributions
Conceptualization, GE and ML; methodology, ML and GE; writing—review and editing, GE, MW, and ML; visualization, GE; supervision, ML and MW; funding acquisition, ML. All authors have read and agreed to the published version of the manuscript.
Funding
We gratefully acknowledge the financial support of the Alexander von Humboldt Foundation (Georg Forster Research Fellowship). We also acknowledge support by the Open Access Publication Funds of the Ruhr-Universität Bochum.
Conflict of interest
The authors declare that the research was conducted in the absence of any commercial or financial relationships that could be construed as a potential conflict of interest.
Publisher’s note
All claims expressed in this article are solely those of the authors and do not necessarily represent those of their affiliated organizations, or those of the publisher, the editors and the reviewers. Any product that may be evaluated in this article, or claim that may be made by its manufacturer, is not guaranteed or endorsed by the publisher.
References
Agegnehu G., Bass A., Nelson P., Bird M. (2016). Benefits of biochar, compost and biochar–compost for soil quality, maize yield and greenhouse gas emissions in a tropical agricultural soil. Sci. Total Environ. 543, 295–306. doi: 10.1016/j.scitotenv.2015.11.054
Ahsan M., Singh M., Singh R. P., Yadav V., Tandon S., Saikia B. K., et al. (2022). An innovative circular model for recycling the wastes into biochar using distillation units. J. Clean. Prod. 361, 132258. doi: 10.1016/j.jclepro.2022.132258
Akhtar S. S., Andersen M. N., Liu F. (2015). Residual effects of biochar on improving growth, physiology and yield of wheat under salt stress. Agric. Water Manage. 158, 61–68. doi: 10.1016/j.agwat.2015.04.010
Allohverdi T., Mohanty A. K., Roy P., Misra M. (2021). A review on current status of biochar uses in agriculture. Molecules 26, 5584. doi: 10.3390/molecules26185584
Álvarez M. L., Gascó G., Plaza C., Paz-Ferreiro J., Méndez A. (2017). Hydrochars from biosolids and urban wastes as substitute materials for peat. Land Degrad. Dev. 28, 2268–2276. doi: 10.1002/ldr.2756
An X., Wu Z., Yu J., Ge L., Li T., Liu X., et al. (2020). High-efficiency reclaiming phosphate from an aqueous solution by bentonite modified biochars: a slow release fertilizer with a precise rate regulation. ACS Sustain. Chem. Eng. 8, 6090–6099. doi: 10.1021/acssuschemeng.0c01112
Andert J., Mumme J. (2015). Impact of pyrolysis and hydrothermal biochar on gas-emitting activity of soil microorganisms and bacterial and archaeal community composition. Appl. Soil Ecol. 96, 225–239. doi: 10.1016/j.apsoil.2015.08.019
Antoniou N., Monlau F., Sambusiti C., Ficara E., Barakat A., Zabaniotou A. (2019). Contribution to circular economy options of mixed agricultural wastes management: Coupling anaerobic digestion with gasification for enhanced energy and material recovery. J. Clean. Prod. 209, 505–514. doi: 10.1016/j.jclepro.2018.10.055
Adekiya A. O., Agbede T. M., Olayanju A., Ejue W. S., Adekanye T.A., Adenusi T. T., et al. (2020). Effect of biochar on soil properties, soil loss, and cocoyam yield on a tropical Sandy loam alfisol. Scientific World J. 2020, 1–9. doi: 10.1016/j.agwat.2020.106263
Barber S. T., Yin J., Draper K., Trabold T. A. (2018). Closing nutrient cycles with biochar- from filtration to fertilizer. J. Clean. Prod. 197, 1597–1606. doi: 10.1016/j.jclepro.2018.06.136
Bargmann I., Rillig M. C., Buss W., Kruse A., Kuecke M. (2013). Hydrochar and biochar effects on germination of spring barley. J. Agron. Crop Sci. 199, 360–373. doi: 10.1111/jac.12024
Becker R., Dorgerloh U., Helmis M., Mumme J., Diakité M., Nehls I. (2013). Hydrothermally carbonized plant materials: patterns of volatile organic compounds detected by gas chromatography. Bioresour. Technol. 130, 621–628. doi: 10.1016/j.biortech.2012.12.102
Bian R., Zhang Z., Zhang A., Zheng J., Li L., Joseph S., et al. (2013). Effect of municipal biowaste biochar on greenhouse gas emissions and metal bioaccumulation in a slightly acidic clay rice paddy. BioResources 9, 685–703. doi: 10.15376/biores.9.1.685-703
Borchard N., Wolf A., Laabs V., Aeckersberg R., Scherer H., Moeller A., et al. (2012). Physical activation of biochar and its meaning for soil fertility and nutrient leaching–a greenhouse experiment. Soil Use Manage. 28, 177–184. doi: 10.1111/j.1475-2743.2012.00407.x
Buss W., Mašek O., Graham M., Wüst D. (2015). Inherent organic compounds in biochar–their content, composition and potential toxic effects. J. Environ. Manage. 156, 150–157. doi: 10.1016/j.jenvman.2015.03.035
Calvo P., Nelson L., Kloepper J. W. (2014). Agricultural uses of plant biostimulants. Plant Soil 383, 3–41. doi: 10.1007/s11104-014-2131-8
Cao X., Ro K. S., Chappell M., Li Y., Mao J. (2011). Chemical structures of swine manure chars produced under different carbonization conditions investigated by advanced solid-state 13C nuclear magnetic resonance (NMR) spectroscopy. Energ. Fuel 25, 388–397. doi: 10.1021/ef101342v
Chakrabarti S., Dicke C., Kalderis D., Kern J. (2015). Rice husks and their hydrochars cause unexpected stress response in the nematode caenorhabditis elegans: reduced transcription of stress-related genes. Environ. Sci. pollut. Res. Int. 22, 12092–12103. doi: 10.1007/s11356-015-4491-x
Chen J., Zhang L., Yang G., Wang Q., Li R., Lucia L. A. (2017). Preparation and characterization of activated carbon from hydrochar by phosphoric acid activation and its adsorption performance in prehydrolysis liquor. BioRes 12, 5928–5941. doi: 10.15376/biores.12.3.5928-5941
De Meyer A., Poesen J., Isabirye M., Deckers J., Raes D. (2011). Soil erosion rates in tropical villages: a case study from lake victoria basin, Uganda. Catena 84, 89–98. doi: 10.1016/j.catena.2010.10.001
Dias B. O., Silva C. A., Higashikawa F. S., Roig A., Sanchez-Monedero M. A. (2010). Use of biochar as bulking agent for the composting of poultry manure: effect on organic matter degradation and humification. Bioresour. Technol. 101, 1239–1246. doi: 10.1016/j.biortech.2009.09.024
Dicke C., Lühr C., Ellerbrock R., Mumme J., Kern J. (2015). Effect of hydrothermally carbonized hemp dust on the soil emissions of CO2 and N2O. Bioresources 10, 3210–3223. doi: 10.15376/biores.10.2.3210-3223
Domingues R. R., Sánchez-Monedero M. A., Spokas K. A., Melo L. C. A., Trugilho P. F., Valenciano M. N., et al. (2020). Enhancing cation exchange capacity of weathered soils using biochar: feedstock, pyrolysis conditions and addition rate. Agronomy 10, 824. doi: 10.3390/agronomy10060824
Domingues R. R., Trugilho P. F., Silva C. A., de Melo I. C. N. A., Melo L. C. A., Magriotis Z. M., et al. (2017). Properties of biochar derived from wood and high-nutrient biomasses with the aim of agronomic and environmental benefits. PloS One 12, 1–19. doi: 10.1371/journal.pone.0176884
EBC (2012). European biochar certificate-Guidelines for a sustainable production of biochar (Arbaz, Switzerland: European Biochar Foundation (EBC). doi: 10.13140/RG.2.1.4658.7043
Ellen MacArthur Foundation (EMF) (2012). Towards the circular economy: Economic and business rationale for an accelerated transition (Cowes: MacArthur Foundation).
Ellen MacArthur Foundation (EMF) (2013) Towards the circular economy: Opportunities for the consumers goods sector. Available at: www.ellenmacarthurfoundation.org/publications (Accessed 06-30-2020).
Ellen MacArthur Foundation (EMF) (2019) Completing the picture: How the circular economy tackles climate change. Available at: www.ellenmacarthurfoundation.org/publications (Accessed 06-30-2020).
El-Naggar A., Awad Y. M., Tang X. Y., Liu C., Niazi N. K., Jien S. H., et al. (2018). Biochar influences soil carbon pools and facilitates interactions with soil: A field investigation. Land Degrad. Dev. 29, 2162–2171. doi: 10.1002/ldr.2896
Enaime G., Baçaoui A., Yaacoubi A., Lübken M. (2020a). Biochar for wastewater treatment-conversion technologies and applications. Appl. Sci. 10, 3492. doi: 10.3390/app10103492
Enaime G., Baçaoui A., Yaacoubi A., Wichern M., Lübken M. (2020b). Hydrothermal carbonization of the filter bed remained after filtration of olive mill wastewater on olive stones for biofuel application. Biomass Conv. Bioref. 12, 1237–1247. doi: 10.1007/s13399-020-00743-9
Enaime G., Lübken M. (2021). Agricultural waste-based biochar for agronomic applications. Appl. Sci. 11, 8914. doi: 10.3390/app11198914
Enaime G., Nettmann E., Berzio S., Baçaoui A., Yaacoubi A., Wichern M., et al. (2020c). Performance and microbial analysis during long-term anaerobic digestion of olive mill wastewater in a packed-bed biofilm reactor. J. Chem. Technol. Biotechnol. 95, 850–861. doi: 10.1002/jctb.6275
Food and Agriculture Organization of the United Nations (2009). High level expert forum—how to feed the world in 2050; office of the director (Rome, Italy: Agricultural Development Economics Division).
Gai X., Wang H., Liu J., Zhai L., Liu S., Ren T., et al. (2014). Effects of feedstock and pyrolysis temperature on biochar adsorption of ammonium and nitrate. PloS One 9, e113888. doi: 10.1371/journal.pone.0113888
Gamage D. N., Mapa R. B., Dharmakeerthi R. S., Biswas A. (2016). Effect of rice husk biochar on selected soil properties in tropical alfisols. Soil Res. 54, 302–310. doi: 10.1071/SR15102
Gascó G., Paz-Ferreiro J., Álvarez M., Saa A., Méndez A. (2018). Biochars and hydrochars prepared by pyrolysis and hydrothermal carbonisation of pig manure. Waste Manage. 79, 395–403. doi: 10.1016/j.wasman.2018.08.015
Ge Z., Ling Z., Rong B., Qian Z., Hua R., Hua C. (2016). Effects of biogas slurry and biochar application on active organic carbon in the topsoil of poplar plantation. J. Nanjing For Univ. (Nat. Sci. Ed.) 40, 9–14. doi: 10.3969/j.issn.1000-2006.2016.06.002
Glaser B., Lehmann J., Zech W. (2002). Ameliorating physical and chemical properties of highly weathered soils in the tropics with charcoal—A review. Biol. Fertil. Soils 35, 219–230. doi: 10.1007/s00374-002-0466-4
Gogoi N., Sarma B., Mondal S. C., Kataki R., Garg A. (2019). Use of Biochar in Sustainable Agriculture (Cham: Springer International Publishing), 501–528.
González R., González J., Rosas J. G., Smith R., Gómez X. (2020). Biochar and energy production: Valorizing swine manure through coupling co-digestion and pyrolysis. C — J. Carbon Res. 6, 43. doi: 10.3390/c6020043
Greenberg I., Kaiser M., Gunina A., Ledesma P., Polifka S., Wiedner K., et al. (2019). Substitution of mineral fertilizers with biogas digestate plus biochar increases physically stabilized soil carbon but not crop biomass in a field trial. Sci. Total Environ. 680, 181–189. doi: 10.1016/j.scitotenv.2019.05.051
Hitzl M., Corma A., Pomares F., Renz M. (2015). The hydrothermal carbonization (HTC) plant as a decentral biorefinery for wet biomass. Catalysis Today 257, 154–159. doi: 10.1016/j.cattod.2014.09.024
Hsu J. H., Lo S. L. (2001). Effect of composting on characterization and leaching of copper, manganese, and zinc from swine manure. Environ. pollut. 114, 119–127. doi: 10.1016/S0269-7491(00)00198-6
Hu Q., Jung J., Chen D., Leong K., Song S., Li F., et al. (2021). Biochar industry to circular economy. Sci. Total Environ. 757, 143820. doi: 10.1016/j.scitotenv.2020.143820
Hung C. Y., Tsai W. T., Chen J. W., Lin Y. Q., Chang Y. M. (2017). Characterization of biochar prepared from biogas digestate. Waste Manage. 66, 53–60. doi: 10.1016/j.wasman.2017.04.034
IBI Standard, I. (2015) IBI Biochar Certification Program Manual: requirements and procedures for IBI biochar certification. Available at: https://www.biocharinternational.org/wpcontent/uploads/2018/05/IBI_Biochar_Certification_Program_Manual_V2.1_Final.pdf.
Inyang M., Gao B., Pullammanappallil P., Ding W., Zimmerman A. (2010). Biochar from anaerobically digested sugarcane bagasse. Bioresour. Technol. 101, 8868–8872. doi: 10.1016/j.biortech.2010.06.088
Ippolito J., Ducey T., Cantrell K., Novak J., Lentz R. (2016). Designer, acidic biochar influences calcareous soil characteristics. Chemosphere 142, 184–191. doi: 10.1016/j.chemosphere.2015.05.092
Jacobs J., Work T., Paré D., Bergeron Y. (2015). Paludification of boreal soils reduces wood decomposition rates and increases wood-based carbon storage. Ecosphere 6, 1–20. doi: 10.1890/ES14-00063.1
Jeffery S., Bezemer T. M., Cornelissen G., Kuyper T. W., Lehmann J., Mommer L., et al. (2015). The way forward in biochar research: Targeting trade-offs between the potential wins. GCB Bioenergy 7, 1–13. doi: 10.1111/gcbb.12132
Jindo K., Audette Y., Higashikawa F. S., Silva C. A., Akashi K., Mastrolonardo G., et al. (2020). Role of biochar in promoting circular open access economy in the agriculture sector. Part 1: A review of the biochar roles in soil n, p and k cycles. Chem. Biol. Technol. Agric. 7, 15. doi: 10.1186/s40538-020-00182-8
Joseph S., Graber E., Chia C., Munroe P., Donne S., Thomas T., et al. (2013). Shifting paradigms: development of high-efficiency biochar fertilizers based on nano-structures and soluble components. Carbon Manage. 4, 323–343. doi: 10.4155/cmt.13.23
Judex J., Gaiffi M., Burgbacher H. (2012). Gasification of dried sewage sludge: Status of the demonstration and the pilot plant. Waste Manage. 32, 719–723. doi: 10.1016/j.wasman.2011.12.023
Kambo H., Dutta A. (2015). A comparative review of biochar and hydrochar in terms of production, physico-chemical properties and applications. Renewable Sustain. Energy Rev. 45, 359–378. doi: 10.1016/j.rser.2015.01.050
Kammann C., Schmidt H. P., Messerschmidt N., Linsel S., Steffens D., Müller C., et al. (2015). Plant growth improvement mediated by nitrate capture in co-composted biochar. Sci. Rep. 5, 11080. doi: 10.1038/srep11080
Kätzl K., Lübken M., Gehring T., Wichern M. (2018). Efficient low-cost anaerobic treatment of wastewater using biochar and woodchip filters. Water 10, 818. doi: 10.3390/w10070818
Kätzl K., Lübken M., Nettmann E., Krimmler S., Wichern M. (2020). Slow sand fltration of raw wastewater using biochar as an alternative fltration media. Sci. Rep. 10, 1229. doi: 10.1038/s41598-020-57981-0
Kätzl K., Lübken M., Uzuna G., Gehring T., Nettmann E., Stenchly K., et al. (2019). On-farm wastewater treatment using biochar from local agroresidues reduces pathogens from irrigation water for safer food production in developing countries. Sci. Total Environ. 682, 601–610. doi: 10.1016/j.scitotenv.2019.05.142
Keske C., Godfrey T., Hoag D., Abedin J. (2020). Economic feasibility of biochar and agriculture coproduction from canadian black spruce forest. Food Energy Secur. 9, 118. doi: 10.1002/fes3.188
Khan S., Chao C., Waqas M., Peter H., Arp H., Zhu Y. (2013). Sewage sludge biochar influence upon rice (oryza sativa l.) yield, metal bioaccumulation and greenhouse gas emissions from acidic paddy soil. Environ. Sci. Technol. 47, 8624–8632. doi: 10.1021/es400554x
Khorram M. S., Zheng Y., Lin D., Zhang Q., Fang H., Yu Y. (2016). Dissipation of fomesafen in biochara- mended soil and its availability to corn (Zea mays L.) and earthworm (Eisenia fetida). J. Soils Sediments 16, 2439–2448. doi: 10.1007/s11368-016-1407-4
Kuisma M., Kahiluoto H. (2017). Biotic resource loss beyond food waste: Agriculture leaks worst. Resour. Conserv. Recycl. 124, 129–140. doi: 10.1016/j.resconrec.2017.04.008
Kuligowski K., Luostarinen S. (2011). Thermal gasification of manure. Baltic Forum for Innovative Technologies for Sustainable Manure Management. (Poland: University of Gdánsk–POMCERT)
Lacroix N., Rousse D., Hausler R. (2014). Anaerobic digestion and gasification coupling for wastewater sludge treatment and recovery. Waste Manage. Res. 32, 608–613. doi: 10.1177/0734242X14538308
Laird D., Fleming P., Wang B., Horton R., Karlen D. (2010). Biochar impact on nutrient leaching from a midwestern agricultural soil. Geoderma 158, 436–442. doi: 10.1016/j.geoderma.2010.05.012
Lee J., Lee K., Sohn D., Kim Y., Park K. (2018). Hydrothermal carbonization of lipid extracted algae for hydrochar production and feasibility of using hydrochar as a solid fuel. Energy 153, 913–920. doi: 10.1016/j.energy.2018.04.112
Lehmann J., Rillig M., Thies J., Masiello C., Hockaday W., Crowley D. (2011). Biochar effects on soil biota – A review. Soil Biol. Biochem. 43, 1812–1836. doi: 10.1016/j.soilbio.2011.04.022
Li Y., Zhang R., He Y., Zhang C., Liu X., Chen C., et al. (2014). Anaerobic co-digestion of chicken manure and corn stover in batch and continuously stirred tank reactor (CSTR). Bioresour. Technol. 156, 342–347. doi: 10.1016/j.biortech.2014.01.054
Li C., Zhao C., Zhao X., Wang Y., Lv X., Zhu X., et al. (2023). Beneficial effects of biochar application with nitrogen fertilizer on soil nitrogen retention, absorption and utilization in maize production. Agronomy 13, 113. doi: 10.3390/agronomy13010113
Libra J. A., Ro K. S., Kammann C., Funke A., Berge N. D., Neubauer Y., et al. (2011). Hydrothermal carbonization of biomass residuals: a comparative review of the chemistry, processes and applications of wet and dry pyrolysis. Biofuels 2, 71–106. doi: 10.4155/bfs.10.81
Liu Y., Bi Y., Xie Y., Zhao X., He D., Wang S., et al. (2020). Successive straw biochar amendments reduce nitrous oxide emissions but do not improve the net ecosystem economic benefit in an alkaline sandy loam under a wheat–maize cropping system. Land Degrad. Dev. 31, 868–883. doi: 10.1002/ldr.3495
Liu Z., Balasubramanian R. (2014). Upgrading of waste biomass by hydrothermal carbonization (HTC) and low temperature pyrolysis (LTP): A comparative evaluation. Applied Energy 114, 857–864.
Liu C., Wang H., Tang X., Guan Z., Reid B. J., Rajapaksha A. U., et al. (2016). Biochar increased water holding capacity but accelerated organic carbon leaching from a sloping farmland soil in China. Environ. Sci. pollut. Res. 23, 995–1006. doi: 10.1007/s11356-015-4885-9
Liu X., Ye Y., Liu Y., Zhang A., Zhang X., Li L., et al. (2014). Sustainable biochar effects for low carbon crop production: a 5-crop season field experiment on a low fertility soil from Central China. Agric. Syst. 129, 22–29. doi: 10.1016/j.agsy.2014.05.008
Liu N., Zhou J., Han L., Ma S., Sun X., Huang G. (2017). Role and multiscale characterization of bamboo biochar during poultry manure aerobic composting. Bioresour. Technol. 241, 190–199. doi: 10.1016/j.biortech.2017.03.144
Lone A. H., Najar G. R., Ganie M. A., Sofi J. A., Tahir A. T. (2015). Biochar for sustainable soil health: a review of prospects and concerns. Pedosphere 25, 639–653. doi: 10.1016/S1002-0160(15)30045-X
Lübken M., Wichern M., Schlattmann M., Gronauer A., Horn H. (2007). Modelling the energy balance of an anaerobic digester fed with cattle manure and renewable energy crops. Water Res. 41, 4085–4096. doi: 10.1016/j.watres.2007.05.061
Lucian M., Fiori L. (2017). Hydrothermal carbonization of waste biomass: Process design, modeling, energy efficiency and cost analysis. Energies 10, 211. doi: 10.3390/en10020211
Marshall J., Muhlack R., Morton B., Dunnigan L., Chittleborough D., Kwong C. (2019). Pyrolysis temperature effects on biochar–water interactions and application for improved water holding capacity in vineyard soils. Soil Syst. 3, 27. doi: 10.3390/soilsystems3020027
Mekuria W., Noble A. (2013). The role of biochar in ameliorating disturbed soils and sequestering soil carbon in tropical agricultural production systems. Appl. Environ. Soil Sci. 10, 354965, 10. doi: 10.1155/2013/354965
Monlau F., Barakat A., Trably E., Dumas C., Steyer J. P., Carrère H. (2013). Lignocellulosic materials into biohydrogen and biomethane: Impact of structural features and pretreatment. Crit. Rev. Environ. Sci. Technol. 43, 260–322. doi: 10.1080/10643389.2011.604258
Monlau F., Francavilla M., Sambusiti C., Antoniou N., Solhy A., Libutti A., et al. (2014). Toward a functional integration of anaerobic digestion and pyrolysis for a sustainable resource management. Comparison between solid-digestate and its derived pyrochar as soil amendment. Appl. Energy 169, 652–662. doi: 10.1016/j.apenergy.2016.02.084
Monlau F., Francavilla M., Sambusiti C., Antoniou N., Solhy A., Libutti A., et al. (2016). Toward a functional integration of anaerobic digestion and pyrolysis for a sustainable resource management. comparison between solid-digestate and its derived pyrochar as soil amendment. Appl. Energy 169, 652–662.
Monlau F., Sambusiti C., Antoniou N., Barakat A., Zabaniotou A. (2015a). A new concept for enhancing energy recovery from agricultural residues by coupling anaerobic digestion and pyrolysis process. Appl. Energy 148, 32–38. doi: 10.1016/j.apenergy.2015.03.024
Monlau F., Sambusiti C., Ficara E., Aboulkas A., Barakat A., Carrere H. (2015b). New opportunities for agricultural digestate valorization: current situation and perspectives. Energy Environ. Sci. 8, 2600–2621. doi: 10.1039/C5EE01633A
Mukome F. N. D., Buelow M. C., Shang J., Peng J., Rodriguez M., Mackay D. M., et al. (2020). Biochar amendment as a remediation strategy for surface soils impacted by crude oil. Environ. Pollut. 265, Part B, 115006. doi: 10.1016/j.envpol.2020.115006
Mumme J., Eckervogt L., Pielert J., Diakité M., Rupp F., Kern J. (2011). Hydrothermal carbonization of anaerobically digested maize silage. Bioresour. Technol. 102, 9255–9260. doi: 10.1016/j.biortech.2011.06.099
Murray M., Skene K., Haynes K. (2015). The circular economy: An interdisciplinary exploration of the concept and application in a global context. J. Bus. Ethics 140, 369–380. doi: 10.1007/s10551-015-2693-2
Nelson G. C., Rosegrant M. W., Koo J., Robertson R., Sulser T., Zhu T., et al. (2009). Climate Change: Impact on Agriculture and Costs of Adaptation. (Washington: International Food Policy Research Institute).
Neumann J., Binder S., Apfelbacher A., Gasson J., Ramírez García P., Hornung A. (2015). Production and characterization of a new quality pyrolysis oil, char and syngas from digestate—introducing the thermo-catalytic reforming process. J. Anal. Appl. Pyrolysis 113, 137–142. doi: 10.1016/j.jaap.2014.11.022
Nguyen B. T., Lehmann J., Hockaday W. C., Joseph S., Masiello C. A. (2010). Temperature sensitivity of black carbon decomposition and oxidation. Environ. Sci. Technol. 44, 3324–3331. doi: 10.1021/es903016y
Ning S., Hung M., Chang Y., Wan H., Lee H., Shih R. (2013). Benefit assessment of cost, energy, and environment for biomass pyrolysis oil. J. Clean. Prod. 59, 141–149. doi: 10.1016/j.jclepro.2013.06.042
O’Connor D., Peng T., Zhang J., Tsang D. C., Alessi D. S., Shen Z., et al. (2018). Biochar application for the remediation of heavy metal polluted land: a review of in situ field trials. Sci. Total Environ. 619, 815–826. doi: 10.1016/j.scitotenv.2017.11.132
Oh T., Shinogi Y., Lee S.-J., Cho B. (2014). Utilization of biochar impregnated with anaerobically digested slurry as slow-release fertilizer. J. Plant Nutr. Soil Sci. 177, 97–103. doi: 10.1002/jpln.201200487
Ok Y. S., Uchimiya S. M., Chang S. X., Bolan N. (2016). Biochar: Production, Characterization and Applications (Boca Raton, FL, USA: CRC Press).
Opatokun S., Strezov V., Kan T. (2015). Product based evaluation of pyrolysis of food waste and ist digestate. Energy 92, 349–354. doi: 10.1016/j.energy.2015.02.098
Parmar K. R., Ross A. B. (2019). Integration of hydrothermal carbonisation with anaerobic digestion; opportunities for valorisation of digestate. Energies 12, 1586. doi: 10.3390/en12091586
Pavwelczyk A. (2005). Eu policy and legislation on recycling of organic wastes to agriculture. International Society for Animal Hygiene (ISAH) 1, 64–71.
Pecchi M., Baratieri M. (2019). Coupling anaerobic digestion with gasification, pyrolysis or hydrothermal carbonization: A review. Renew. Sustain. Energy Rev. 105, 462–475. doi: 10.1016/j.rser.2019.02.003
Qian K., Kumar A., Zhang H., Bellmer D., Huhnke R. (2015). Recent advances in utilization of biochar. Renew Sustain. Energy Rev. 42, 1055–1064. doi: 10.1016/j.rser.2014.10.074
Reza M., Mumme J., Ebert A. (2015). Characterization of hydrochar obtained from hydrothermal carbonization of wheat straw digestate. Biomass Convers. Biorefin. 5, 425–435. doi: 10.1007/s13399-015-0163-9
Roberto C., Gabriele G., Fausto M. (2018). Research sajs. short-term effects on soil of biogas digestate, biochar and their combinations. Soil Res 56, 623–631.
Rollinson A. (2016). Gasification reactor engineering approach to understanding the formation of biochar properties. Proc. R. Soc A Math. Phys. Eng. Sci. 472, 20150841. doi: 10.1098/rspa.2015.0841
Rombolà A. G., Fabbri D., Baronti S., Vaccari F. P., Genesio L., Miglietta F. (2019). Changes in the pattern of polycyclic aromatic hydrocarbons in soil treated with biochar from a multiyear field experiment. Chemosphere 219, 662–670. doi: 10.1016/j.chemosphere.2018.11.178
Rombolà A. G., Fabbri D., Meredith W., Snape C. E., Dieguez-Alonso A. (2016). Molecular characterization of the thermally labile fraction of biochar by hydropyrolysis and pyrolysis-GC/MS. J. Anal. Appl. Pyrolysis 121, 230–239. doi: 10.1016/j.jaap.2016.08.003
Roy P., Dias G. (2017). Prospects for pyrolysis technologies in the bioenergy sector: A review. Renew. Sustain. Energy Rev. 77, 59–69. doi: 10.1016/j.rser.2017.03.136
Sambusiti C., Monlau F., Ficara E., Carrère H., Malpei F. (2013). A comparison of different pretreatments to increase methane production from two agricultural substrates. Appl. Energy 104, 62–70. doi: 10.1016/j.apenergy.2012.10.060
Sanscartier D., Deen B., Dias G., MacLean H. L., Dadfar H., McDonald I., et al. (2014). Implications of land class and environmental factors on life cycle ghg emissions of miscanthus as a bioenergy feedstock. GCB Bioenergy 6, 401–413. doi: 10.1111/gcbb.12062
Santi G., Proietti S., Moscatello S., Stefanoni W., Battistelli A. (2015). Anaerobic digestion of corn silage on a commercial scale: Differential utilization of its chemical constituents and characterization of the solid digestate. Biomass Bioenergy 83, 17–22. doi: 10.1016/j.biombioe.2015.08.018
Sarkar B., Debnath A., Chiu A. S. F., Ahmed W. (2022a). Circular economy-driven two-stage supply chain management for nullifying waste. J. Clean. Prod. 339, 130513. doi: 10.1016/j.jclepro.2022.130513
Sarkar B., Ullah M., Sarkar M. (2022b). Environmental and economic sustainability through innovative green products by remanufacturing. J. Clean. Prod. 332, 129813. doi: 10.1016/j.jclepro.2021.129813
Sarkhot D., Ghezzehei T., Berhe A. (2013). Effectiveness of biochar for sorption of ammonium and phosphate from dairy effluent. J. Environ. Qual. 42, 1545–1554. doi: 10.2134/jeq2012.0482
Saveyn H., Edder P. (2014). End-of-waste criteria for biodegradable waste subjected to biological treatment (compost digestate): Technical proposal (Seville, Spain: IPTS, EC).
Scholz S., Sembres T., Roberts K., Whitman T., Wilson K., Lehmann J. (2014). Biochar Systems for Smallholders in Developing Countries: Leveraging Current Knowledge and Exploring Future Potential for Climate-Smart Agriculture (Washington, D.C.: World Bank Publications). doi: 10.1596/978-0-8213-9525-7
Semida W. M., Beheiry H. R., Setamou M., Simpson C. R., El-Mageedc T. A. A., Radyd M. M., et al. (2019). Biochar implications for sustainable agriculture and environment: A review. S. Afr. J. Bot. 127, 333–347. doi: 10.1016/j.sajb.2019.11.015
Shabangu S., Woolf D., Fisher E., Angenent L., Lehmann J. (2014). Technoeconomic assessment of biomass slow pyrolysis into different biochar and methanol concepts. Fuel 117, 742–748. doi: 10.1016/j.fuel.2013.08.053
Shackley S., Ibarrola Esteinou R., Hopkins D., Hammond J. (2014). Biochar Quality Mandate (BQM) version 1.0. (British Biochar Foundation)
Sheets J., Yang L., Ge X., Wang Z., Li Y. (2015). Beyond land application: Emerging technologies for the treatment and reuse of anaerobically digested agricultural and food waste. Waste Manage. (Oxford) 44, 94–115. doi: 10.1016/j.wasman.2015.07.037
Shetty R., Prakash N. B. (2020). Effect of different biochars on acid soil and growth parameters of rice plants under aluminium toxicity. Sci. Rep. 10, 1–10. doi: 10.1038/s41598-020-69262-x
Singh E., Mishra R., Kumar A., Shukla S. K., Lo S. L., Kumar S. (2022). Circular economy-based environmental management using biochar: Driving towards sustainability. Process Saf. Environ. Prot. 163, 585–600. doi: 10.1016/j.psep.2022.05.056
Smith P., Martino D., Cai Z., Gwary D., Janzen H., Kumar P., et al. (2007). “Agriculture,” in Climate change 2007: mitigation. contribution of working group III to the fourth assessment report of the intergovernmental panel on climate change (Cambridge, United Kingdom: Cambridge University Press), 497–540.
Smith P., Martino D., Cai Z., Gwary D., Janzen H., Kumar P., et al. (2008). Greenhouse gas mitigation in agriculture. Philos. Trans. R. Soc. B Biol. Sci. 363, 789–813. doi: 10.1098/rstb.2007.2184
Solaiman Z. M., Abbott L. K., Murphy D. V. (2019). Biochar phosphorus concentration dictates mycorrhizal colonisation, plant growth and soil phosphorus cycling. Sci. Rep. 9, 1–11. doi: 10.1038/s41598-019-41671-7
Solaiman Z., Anawar H. (2015). Application of biochars for soil constraints: Challenges and solutions. Pedosphere 25, 631–638. doi: 10.1016/S1002-0160(15)30044-8
Song J., Wang Y., Zhang S., Song Y., Xue S., Liu L., et al. (2021). Coupling biochar with anaerobic digestion in a circular economy perspective: A promising way to promote sustainable energy, environment and agriculture development in China. Renew. Sustain. Energy Rev. 144, 110973. doi: 10.1016/j.rser.2021.110973
Spokas K., Novak J., Stewart C., Cantrell K., Uchimiya M., DuSaire M., et al. (2011). Qualitative analysis of volatile organic compounds on biochar. Chemosphere 85, 869–882. doi: 10.1016/j.chemosphere.2011.06.108
Spokas K., Reicosky D. (2009). Impacts of sixteen different biochars on soil greenhouse has production. Ann. Environ. Sci. 3, 179–193.
Srinivasarao C., Lal R., Kundu S., Babu M., Venkateswarlu B., Singh A. (2014). Soil carbon sequestration in rainfed production systems in the semiarid tropics of India. Sci. Total Environ. 487, 587–603. doi: 10.1016/j.scitotenv.2013.10.006
Streubel J., Collins H., Tarara J., Cochran R. (2012). Biochar produced from anaerobically digested fiber reduces phosphorus in dairy lagoons. J. Environ. Qual. 41, 1166–1174. doi: 10.2134/jeq2011.0131
Stutzenstein P., Bacher M., Rosenau T., Pfeifer C. (2018). Optimization of nutrient and carbon recovery from anaerobic digestate via hydrothermal carbonization and investigation of the influence of the process parameters. Waste Biomass Valor 9, 1303–1318. doi: 10.1007/s12649-017-9902-4
Tang J., Zhu W., Kookana R., Katayama A. (2013). Characteristics of biochar and its application in remediation of contaminated soil. J. Biosci. Bioeng. 116, 653–659. doi: 10.1016/j.jbiosc.2013.05.035
Taurino R., Lancellotti I., Tatano F., Carchesio M., Pozzi P. (2016). Mechanical and chemical resistance of composite materials with addition of anaerobic digestate. Compos. Part B 92, 259–264. doi: 10.1016/j.compositesb.2016.02.012
Tay H. L., Kajitani S., Zhang S., Li C. Z. (2013). Effects of gasifying agent on the evolution of char structure during the gasification of victorian brown coal. Fuel 103, 22–28. doi: 10.1016/j.fuel.2011.02.044
Tilman D., Balzer C., Hill J., Befort B. L. (2013). Global food demand and the sustainable intensification of agriculture. Proc. Natl. Acad. Sci. U. S. A. 108, 20260–20264. doi: 10.1073/pnas.1116437108
Toopa T. A., Ward S., Oldfield T., Hull M., Kirby M. E., K.Theodorou M. (2017). Agrocycle – developing a circular economy in agriculture. Energy Proc. 123, 76–80. doi: 10.1016/j.egypro.2017.07.269
Tripathi M., Sahu J., Ganesan P. (2016). Effect of process parameters on production of biochar from biomass waste through pyrolysis: a review. Renew Sustain. Energy Rev. 55, 467–481. doi: 10.1016/j.rser.2015.10.122
Troy S., Nolan T., Leahy J., Lawlor P., Healy M., Kwapinski W. (2013). Effect of sawdust addition and composting of feedstock on renewable energy and biochar production from667 pyrolysis of anaerobically digested pig manure. Biomass Bioenergy 49, 1–9. doi: 10.1016/j.biombioe.2012.12.014
Van Beilen N. (2016). Commercialization of biochar and the benefits for climate change and agriculture. Inq. J. 8, 1–3. Available online: http://www.inquiriesjournal.com/articles/1509/3/commercialization-of-biochar-and-the-benefits-for-climate-change-and-agriculture (Accessed on 7 December 2021).
Velasco-Muñoz J. F., Mendoza J. M. F., Aznar-Sánchez J. A., Gallego-Schmid A. (2021). Circular economy implementation in the agricultural sector: Definition, strategies and indicators. Resour. Conserv. Recycl. 170, 105618. doi: 10.1016/j.resconrec.2021.105618
Wang J., Wang S. (2019). Preparation, modification and environmental application of biochar: a review. J. Clean. Prod. 227, 1002–1022. doi: 10.1016/j.jclepro.2019.04.282
Wang L., Zhang L., Li A. (2014). Hydrothermal treatment coupled with mechanical expression at increased temperature for excess sludge dewatering: influence of operating conditions and the process energetics. Water Res. 65, 85–97. doi: 10.1016/j.watres.2014.07.020
Wang Z., Zheng Z., Zhuo L., Li W., Long S., Zhen C. (2018). Effects of biochar combined with biogas slurry on soil nutrients in leaching state [in chinese]. Trans. Chin. Soc. Agric. Mach. 49, 260–267. doi: 10.6041/j.issn.1000-1298.2018.11.030
Weber K., Quicker P. (2018). Properties of biochar. Fuel 217, 240–261. doi: 10.1016/j.fuel.2017.12.054
Werner S., Kätzl K., Wichern M., Buerkert A., Steiner C., Marschner B. (2018). Agronomic benefits of biochar as a soil amendment after its use as waste water filtration medium. Environ. pollut. 233, 561–568. doi: 10.1016/j.envpol.2017.10.048
Wichern M., Lübken M., Schlattmann M., Gronauer A., Horn H. (2008). Investigations and mathematical simulation on decentralized anaerobic treatment of agricultural substrate from livestock farming. Water Sci. Technol. 58, 67–72. doi: 10.2166/wst.2008.332
Wiedner K., Naisse C., Rumpel C., Pozzi A., Wieczorek P., Glaser B. (2012). Chemical modification of biomass residues during hydrothermal carbonizationewhat makes the difference, temperature or feedstock? Org. Geochem. 54, 91–100. doi: 10.1016/j.orggeochem.2012.10.006
Wiedner K., Rumpel C., Steiner C., Pozzi A., Maas R., Glaser B. (2013). Chemical evaluation of chars produced by thermochemical conversion (gasification, pyrolysis and hydrothermal carbonization) of agro-industrial biomass on a commercial scale. Biomass Bioenergy 59, 264–278. doi: 10.1016/j.biombioe.2013.08.026
Wilk M., Magdziarz A. (2017). Hydrothermal carbonization, torrefaction and slow pyrolysis of miscanthus giganteus. Energy 140, 1292–1304. doi: 10.1016/j.energy.2017.03.031
Woolf D. (2008) Biochar as a soil amendment: A review of the environmental implications. Available at: https://orgprints.org/id/eprint/13268/1/Biocharasasoilamendment–areview.pdf.
Woolf D., Lehmann J., Lee D. (2016). Optimal bioenergy power generation for climate change mitigation with or without carbon sequestration. Nat. Commun. 7, 13160. doi: 10.1038/ncomms13160
World Economic Forum (2014). Towards the circular economy: Accelerating the scale-up across global supply chains (Geneva: World Economic Forum.).
Xu X., Cheng K., Wu H., Sun J., Yue Q., Pan G. (2018). Greenhouse gas mitigation potential in crop production with biochar soil amendment-a carbon footprint assessment for cross-site field experiments from China. GCB Bioenergy 11, 592–605. doi: 10.1111/gcbb.12561
Yihunu E. W., Minale M., Abebe S., Limin M. (2019). Preparation, characterization and cost analysis of activated biochar and hydrochar derived from agricultural waste: a comparative study. SN Appl. Sci. 1, 873. doi: 10.1007/s42452-019-0936-z
Yihunu E. W., Yu H., Junhe W., Kai Z., Teffera Z. L., Weldegebrial B., et al. (2020). A comparative study on defuoridation capabilities of biosorbents: isotherm, kinetics, thermodynamics, cost estimation and regeneration study. Environ. Eng. Res. 25, 384–392. doi: 10.4491/eer.2019.097
Yu K. L., Lau B. F., Show P. L., Ong H. C., Ling T. C., Chen W. H., et al. (2017). Recent developments on algal biochar production and characterization. Bioresour. Technol. 246, 2–11. doi: 10.1016/j.biortech.2017.08.009
Yuan P., Wang J., Pan Y., Shen B., Wu C. (2019). Review of biochar for the management of contaminated soil: preparation, application and prospect. Sci. Total Environ. 659, 473–490. doi: 10.1016/j.scitotenv.2018.12.400
Zhang A., Cui L., Pan G., Li L., Hussain Q., Zhang X., et al. (2010). Effect of biochar amendment on yield and methane and nitrous oxide emissions from a rice paddy from tai lake plain, China. Agric. Ecosyst. Environ. 139, 469–475. doi: 10.1016/j.agee.2010.09.003
Zhang L., Li T., Wei G., Cai C., Fan Z., Yu H. A. (2015). Effects of biochar and biogas slurry on soil and leaf nutrition and fruit yield quality of apple orchard [in chinese]. China Fruits, 10–13.
Zhang A., Liu Y., Pan G., Hussain Q., Li L., Zheng J., et al. (2012). Effect of biochar amendment on maize yield and greenhouse gas emissions from a soil organic carbon poor calcareous loamy soil from central China plain. Plant Soil 351, 263–275. doi: 10.1007/s11104-011-0957-x
Zhang Z., Zhu Z., Shen B., Liu L. (2019). Insights into biochar and hydrochar production and applications: A review. Energy 171, 581–598. doi: 10.1016/j.energy.2019.01.035
Zhao P., Shen Y., Ge S., Chen Z., Yoshikawa K. (2014). Clean solid biofuel production from high moisture840 content waste biomass employing hydrothermal treatment. Appl. Energy 131, 345–367. doi: 10.1016/j.apenergy.2014.06.038
Keywords: biochar, agriculture, circular economy, energy-water systems, waste-to-resource concept
Citation: Enaime G, Wichern M and Lübken M (2023) Contribution of biochar application to the promotion of circular economy in agriculture. Front. Agron. 5:1214012. doi: 10.3389/fagro.2023.1214012
Received: 28 April 2023; Accepted: 02 August 2023;
Published: 21 August 2023.
Edited by:
Muhammad Bilal Khan, Ayub Agriculture Research Institute, PakistanReviewed by:
Owais Ali Wani, Sher-e-Kashmir University of Agricultural Sciences and Technology of Kashmir, IndiaYuxue Liu, Zhejiang Academy of Agricultural Sciences, China
Copyright © 2023 Enaime, Wichern and Lübken. This is an open-access article distributed under the terms of the Creative Commons Attribution License (CC BY). The use, distribution or reproduction in other forums is permitted, provided the original author(s) and the copyright owner(s) are credited and that the original publication in this journal is cited, in accordance with accepted academic practice. No use, distribution or reproduction is permitted which does not comply with these terms.
*Correspondence: Manfred Lübken, bWFuZnJlZC5sdWVia2VuQHJ1Yi5kZQ==