- Instituto de Biotecnología y Biología Molecular (IBBM), CCT CONICET La Plata, Departamento de Ciencias Biológicas, Facultad de Ciencias Exactas, Universidad Nacional de La Plata, La Plata, Buenos Aires, Argentina
The growth and persistence of alfalfa (Medicago sativa), a perennial legume capable of producing high yields of high-quality forage, is reduced in moderately acidic soils. The low performance of alfalfa at low pH is due to numerous factors that affect the host plant, their rhizobia, and the symbiotic interaction. Sinorhizobium meliloti LPU63 was isolated from acid topsoil (in Argentina) and showed to be a highly competitive and efficient N2-fixing rhizobium under both neutral and moderately acidic soil conditions. In this study, we obtained a draft of the LPU63 genome sequence using Illumina HiSeq4000. The whole genome phylogenetic analysis confirmed the taxonomic position of LPU63 as a S. meliloti strain and the multilocus sequence analysis confirmed that LPU63 is not related to the strains used in Argentina in bioformulations. The genomic analysis showed that beyond the canonical chromosome, pSymA, and pSymB, LPU63 strain has an accessory plasmid that codes for a repABC origin of replication and a conjugative T4SS, suggesting that this plasmid could be self-transmissible. In addition, the complete denitrification pathway (i.e., the gene clusters nap, nir, nor, and nos), including napC and nosZ, which could be used as an alternative respiration route under hypoxic conditions with moderate N2O emissions was found. Also, genes associated with plant growth-promoting activities (PGPR) and the degradation of phenylacetic acid (PAA) were identified. LPU63 is a highly melanogenic strain, a property that could enhance its survival under soil conditions, and the genome data showed a particular arrangement of the genes involved in melanin production. The information regarding LPU63 activities compatible with plant-growth promotion phenotypes, together with other characteristics mentioned here (melanin production, potential moderate N2O emissions), constitute the basis of future experiments toward the rational design of a novel bioinoculant for the environmentally sustainable production of alfalfa.
1 Introduction
Nitrogen is a crucial element for plant growth and production. Inadequate access to bioavailable nitrogen limits crop productivity and, consequently, food production. Several legume plants can fulfill their demand for nitrogen by establishing a symbiotic association with nitrogen-fixing soil bacteria known as rhizobia. An important goal of nitrogen-fixing symbiosis research is to understand how to increase plant productivity while achieving a parallel drastic reduction of greenhouse gas emissions. However, developing more productive and environmentally sustainable symbiosis is not simply a matter of choosing both best symbionts because symbiotic productivity can be limited by suboptimal soil conditions for their interaction and for nitrogen fixation. For example, soil’s acidity significantly reduce the productivity of legumes mainly because of the detrimental effects of hydrogen ions on the plants and their N2-fixing rhizobial symbionts (Munns, 1970; O'hara et al., 1989). Sinorhizobium meliloti and Sinorhizobium medicae (the symbionts of Medicago, Melilotus, and Trigonella spp.) are extremely sensitive to low pH (Glenn and Dilworth, 1994), slowing down and even stopping their growth at pH 5.5 or below (Howieson et al., 1992; Reeve et al., 1993). On the basis of empirical observations, the in vitro acid tolerance of rhizobia has been considered a positive trait for the bacteria to perform well under acidic conditions in the field (Howieson et al., 1988). Therefore, screening for acid-tolerant isolates and those that colonize or persist in acidic soils gave rise to novel strains with enhanced survival and/or symbiosis in moderately acid conditions (Thornton and Davey, 1984; Richardson and Simpson, 1989; Graham et al., 1994; Del Papa et al., 1999; Segundo et al., 1999; Del Papa et al., 2003).
S. meliloti LPU63 is an autochthonous strain isolated from an acid soil in Argentina (Castelar, Buenos Aires). It has a “mid-acid tolerant” phenotype (able to grow under laboratory conditions at pH 5.6), whereas the majority of strains isolated from this region were shown to be sensitive to acidic pH (not able to grow under laboratory conditions at pH 5.6) (Del Papa et al., 1999). This strain proved to be a competitive and effective nitrogen-fixing rhizobium in neutral and moderately acidic soil conditions (Segundo et al., 1999; Del Papa et al., 2003). Based on these results, strain LPU63 was proposed as an efficient inoculant for use in the cultivation of alfalfa both in acid soils and in optimal edaphic conditions. Although several phenotypic aspects of S. meliloti LPU63 have been described in relation to its tolerance of acid stress and symbiotic association with alfalfa (Segundo et al., 1999; Del Papa et al., 2003), the underlying molecular mechanisms that enable acid pH-tolerant inoculants to outperform other strains in acidic soils are still unclear.
Although reducing the use of synthetic nitrogen fertilizers due to biological nitrogen fixation (BNF) is considered a favorable alternative for the environment, the generation of greenhouse gases during alfalfa cultivation has been recognized as a possible negative effect. These gases are generated both by the plant and by the incomplete denitrification process carried out by the rhizobia (Horchani et al., 2011; Ruiz et al., 2022). In oxygen-limited conditions, many bacterial species can switch from O2 respiration to nitrate respiration (NO3−) to produce energy through a process called denitrification (Torres et al., 2018). The complete denitrification pathway comprises the reduction of nitrate (NO3-) or nitrite (NO2-) to molecular nitrogen (N2) with formation of the gaseous intermediates nitric oxide (NO) and nitrous oxide (N2O). NO contributes to the depletion of the ozone layer and N2O is a potent greenhouse gas (Solomon, 2007). In particular, S. meliloti B399, used for more than 50 years as a commercial inoculant in Argentina, produces high levels of N2O, due to the absence of N2O reductase genes (nos), involved in the conversion of N2O to N2 (Brambilla et al., 2018; Brambilla et al., 2019). For these reasons, priority should be given to the selection of inoculant strains efficient in BNF and nos+ to mitigate the emission of N2O in the cultivation of alfalfa (Brambilla et al., 2019). It is unknown whether strain LPU63 codes for the complete denitrification pathway. Recently, it has been shown that the overexpression of the nap operon (coding for the enzymes involved in the first step of the denitrification pathway), promotes nodulation when the host plant develops in a hypoxic environment (Pacheco et al., 2023).
In addition to the ability to establish a nitrogen-fixing symbiosis, other metabolic activities of rhizobia generate a beneficial effect on the host plant, called PGPR activities. These activities include the solubilization of phosphates, production of auxins, synthesis of siderophores and enzymatic activities such as chitinase, cellulase, protease, amylase, and pectinase (Paterson et al., 2017; López et al., 2018). The identification of PGPR activities in LPU63 would increase its value as a potential inoculant of alfalfa.
S. meliloti LPU63 was also described as a proficient melanin producing strain (Del Papa et al., 1999). The identification and analysis of the melanin biosynthesis gene cluster were carried out in the Sinorhizobium meliloti CA15-1 strain (Chizhevskaya et al., 2018). It was shown that the melanin production did not affect efficiency of symbiosis with Medicago sativa, most probably melanin is important at the stages of adaptation of the free-living cells in the environment. These pigments, mostly derived from aromatic amino acids such as tyrosine, are produced by several types of bacteria and contribute to numerous relevant biological roles: protection from UV radiation, oxidants, and free-radicals (Meredith and Sarna, 2006); and chelation of metals (Hong and Simon, 2007); among other roles which ultimately improve the survival of bacteria in stressful conditions (Pavan et al., 2020). In S. meliloti, copper-induced melanin production was linked to bacterial resistance to predation by the soil bacterium Myxococcus xanthus (Contreras-Moreno et al., 2020). Therefore, production of melanin is other trait that could enhance the survival of rhizobial strains in soil conditions.
To answers these outstanding questions, in this study, we obtained the S. meliloti LPU63 draft genome and presented an analysis of relevant genome properties and features. We also analyzed the roles of various genetic determinants in the development of symbiotic relationships with the host plant and in its survival in soil conditions.
2 Materials and methods
2.1 Bacterial strains and growth conditions
The strains used in this study are listed in Table 1. Rhizobial strains were grown at 28°C in tryptone yeast (TY) medium (Beringer, 1974). Gluconacetobacter diazotrophicus Pal5 was grown in LGI medium (Cavalcante and Dobereiner, 1988) at 28°C.
2.2 Genomic DNA preparation, sequencing, and assembly
Genomic DNA was extracted using the AccuPrep Genomic DNA Extraction Kit (Bioneer) following the manufacturer’s protocol. Insert libraries from S. meliloti LPU63 genomic DNA were performed using standard Illumina protocols and further sequenced at SNPsaurus (Eugene, USA) using an Illumina HiSeq 4000 instrument. All raw reads obtained from SNPsaurus were analyzed with FastQC (Andrews, 2010), and a high proportion of Nextera adapters was found. Adapters were removed and reads were quality filtered using Trimmomatic (Bolger et al., 2014) (at Galaxy Australia server). Next, filtered reads were assembled with SPAdes, Velvet Optimizer (Zerbino, 2010), and Unicycler. Medusa (Bosi et al., 2015), using Unicycler assembly as the target, Multi-CSAR (Chen et al., 2018) and CISA (at Orione galaxy portal, http://orione.crs4.it) were used to improve the assembly. A comparison of assembly quality was done with Quast and the Medusa (http://combo.dbe.unifi.it/medusa) refined assembly was selected. Mummer (Delcher et al., 2003) was used to look for artifacts produced during the scaffolding step. Misassembled Medusa scaffolds were manually broken.
2.3 Genome annotation and analysis of the codon usage profiles
The genome was annotated using the National Center for Biotechnology Information (NCBI) Prokaryotic Genome Annotation Pipeline (PGAP) (Tatusova et al., 2013). TXSScan was used to search for secretion systems or flagellar genes in the genome of LPU63 at the Galaxy Pasteur server (Abby et al., 2014).
For analysis of the codon usage profiles of S. meliloti LPU63 contigs, modal codon usages for different gene sets were calculated as previously described by (Davis and Olsen, 2009) with the details reported by López et al. (2019). Factorial correspondence analysis of Relative Synonymous Codon Usage frequencies (RSCU) of the individual genes and modal codon usage frequencies from S. meliloti genomes, S. meliloti accessory plasmids, and S. meliloti LUP63 contigs was calculated by the use of CodonW software (http://codonw.sourceforge.net/).
2.4 Taxonomic classification
LPU63 assembly was analyzed with MiGA to identify the most closely related genomes available in the NCBI Genome database (Rodriguez-R et al., 2018). A genome-based taxonomy was performed with TYGS (Meier-Kolthoff and Göker, 2019) comparing LPU63 genome with available genomes of type strains. Next, a whole-genome phylogenetic analysis was performed with EGDAR (Efficient Database framework for comparative Genome Analyses using BLAST score Ratios) (Blom et al., 2009) in order to include non-type strains of rhizobia. Genomes used for the analysis are listed in Table S1. A multilocus sequence analysis (MLSA) was conducted in MEGA X (Kumar et al., 2018) using the housekeeping genes aapJ, aapM, dnaK, fcl, gyrB, hydR, ndvB and rho as described in Brambilla et al. (2019). The concatenated DNA sequences of the housekeeping genes were aligned with ClustalW (Gap Opening Penalty: 15.00; Gap Extension Penalty: 6.66). Next, the evolutionary history was inferred by using a Neighbor-joining phylogenetic tree constructed using the Tamura-Nei model with 1,000 bootstrap replications.
2.5 Pan-genome
To determine the accessory genome of S. meliloti LPU63 a pan-genome analysis was done with Roary (Page et al., 2015), using default parameters and the genomes of S. meliloti strains selected following the MiGA ANI results (KH46, RMO17, BL225c, AK83, SM11, 2011, HM006, KH35c, WSM1022, CCMM_B554, GR4, and B401; Table S1).
2.6 Identification of phenylacetic acid degradation and denitrification pathway gene clusters
Genes involved in PAA degradation and the denitrification pathway were identified using BLASTp. Gene cluster comparison figures were created with Clinker (Gilchrist and Chooi, 2021). The final figures were edited with Inkscape.
2.7 PGPR activities
Homologous genes involved in plant growth promotion traits were identified by BLASTp as described by Bruto et al. (Bruto et al., 2014). The solubilization of mineral phosphate was tested on plates containing the National Botanical Research Institute’s phosphate growth medium (NBRIP), according to Nautiyal (Nautiyal, 1999). Gluconacetobacter diazotrophicus Pal5 was used as a positive control.
2.8 Melanin production
Genes involved in melanin production were identified using BLAST. Melanin production was determined by the method of Cubo et al. (1988), as modified by Zhang et al., (1991). Briefly, bacteria were cultured in TY media supplemented with 40 µg mL-1 CuSO4 and 100 µg mL-1 of L-tyrosine for 3 days at 28°C, then 10% (w/v) SDS was added, and plates were further incubated for 1 day at room temperature.
2.9 Plasmid profiles
In order to compare bacterial plasmid profiles in situ lysis gel assay described by Eckhardt (1978) was used with the modifications described by Martini et al. (2015).
2.10 PCR
The mcoA gene was amplified using PCR with Pfu DNA polymerase (Embiotec, Quilmes, Argentina) and primers mcoA-Fw (GCACGTTTGGGAGGTTTG) and mcoA-Rv (CTAGTGATGGCCGGTAAC) designed using S. meliloti LPU63 sequence information, S. meliloti LPU63 total DNA as template and following standard procedures. The PCR product was purified and sequenced at Macrogen (South Korea).
3 Results and discussion
3.1 General characteristics and genome annotation
In this study, we performed a high-throughput sequencing of the whole genome of S. meliloti LPU63. The draft genome sequence and annotation of strain LPU63 is available at GenBank (BioProject accession number: JAFFTL000000000.1; BioSample: SAMN17951857; SRA accession: SRX10118759; Assembly: ASM2410269v1). The sequencing run (2 × 150 bp) resulted in 724,343 paired end reads yielding approximately 93.0 Mb of sequence information. A total of 710,276 paired end reads that passed the quality check were used for the assembly. The G+C content of the assembled genome of strain LPU63 was 62.00% and the size was estimated to be 6,931,924 bp (Table S2). The assembled genome (coverage of 15×) was composed of 160 large contigs (> 1,000 bp). The size of the contig N50 was 107,219 bp. The genome was annotated applying the NCBI Prokaryotic Genome Annotation Pipeline, which predicted 6,374 protein-coding sequences, 50 tRNA genes and 3 rRNA genes (one 5S, one 16S and one 23S) (Table S2).
3.2 Phylogenetic analysis
LPU63 was previously identified as a S. meliloti strain based only on 16S rRNA sequencing. To accurately identify its species, a genome-based taxonomy was performed with TYGS, comparing the LPU63 genome with available genomes of type strains. This study showed that LPU63 belongs to the same species cluster as S. meliloti NBRC 14782 type strain (deposited as USDA 1002), in agreement with previous results. The dDDH value between the two strains was 87.1% (TYGS formula d4, equivalent to GGDC formula 2), which is above the cut-off required to assign both strains as the same species (≥70% dDDH). The closest available genome deposited in NCBI was S. meliloti KH35c (NZ_CP021825), as identified by MiGA.
Next, we expanded this phylogenetic analysis in order to include non-type strains of rhizobia, to analyze the relatedness of strain LPU63 with previously characterized rhizobia. A whole-genome phylogenetic tree was constructed using EDGAR (Figure 1). Strain LPU63 and S. meliloti KH35c were found in a cluster that diverged from the one containing laboratory strains, such as 1021, and commercial strains like B399 and B401, the latter two extensively used in Argentina as alfalfa inoculants. This result suggests that LPU63 is not related to strains previously isolated from Argentina, but it is more closely related to strain KH35c, originally isolated from France.
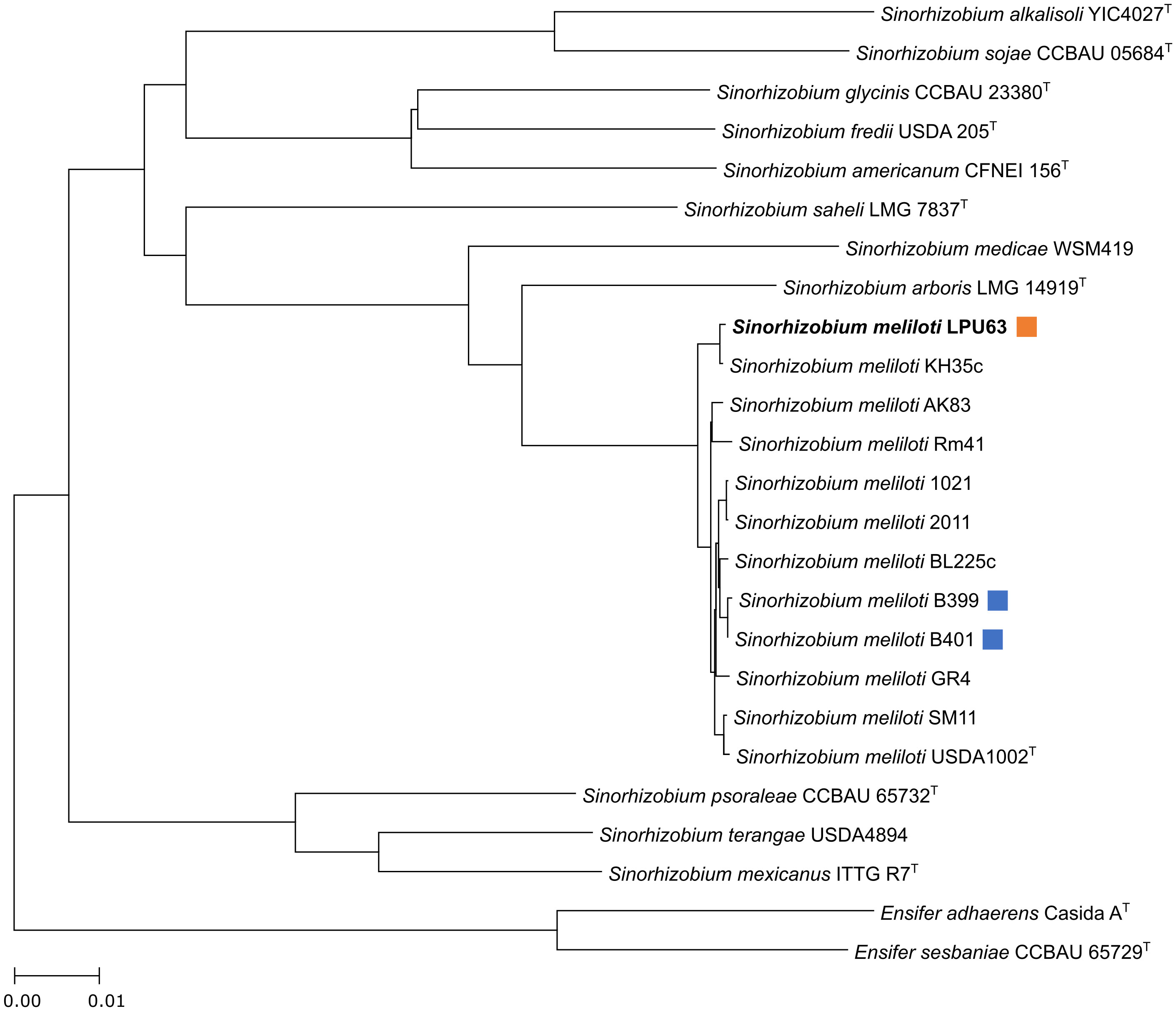
Figure 1 Whole genome phylogenetic analysis. Phylogenetic study based on whole genomic sequences of S. meliloti LPU63 and related rhizobia performed with EDGAR. Superscript T indicates that strains are type strains. The orange squares indicate the strain analyzed in this work; the light blue squares denote rhizobia that is used for commercial inoculant production in Argentina. S. meliloti LPU63 clusters together with S. meliloti KH35c.
The clustering of stain LPU63 and KH35c, and their divergence from other S. meliloti strains in the whole-genome phylogenetic tree is noteworthy (Figure 1). In a recent screening of 13 S. meliloti strains, KH35c was described as one of the most competitive for nodule occupancy against strain BL225C (Bellabarba et al., 2021). Interestingly, S. meliloti LPU63 was previously reported as a good competitor for nodule occupancy against the native rhizobia population and even the acid tolerant and highly competitive Rhizobium favelukesii LPU83T strain (Segundo et al., 1999), which is an acid tolerant alfalfa-nodulating rhizobia. Bellabarba et al. (2021) performed a k-mer-based GWAS analysis that linked a 26 kb region present in the KH35c genome putatively associated with its competition capability against BL225C. This region includes genes involved in the synthesis of the redox cofactor F420 (fbi operon) and several Bra/Liv type ABC transporters involved in uptake of branched-chain amino acids. The presence of F420 cofactor is linked to several important processes such as persistence, antibiotic biosynthesis (tetracyclines, lincosamides, and thiopeptides), and prodrug activation, possibly increasing the fitness of these strains (Greening et al., 2016; Ney et al., 2017). The S. meliloti genome encodes many ABC uptake and export systems (Greening et al., 2016; Ney et al., 2017), it has been reported an attenuated competitive phenotype in the livM mutant. Considering that livM gene encodes the permease subunit of the Bra/Liv complex, it was then proposed that this complex may provide a significant advantage in the competition, guaranteeing a higher supply of amino acids in rhizospheres’ environments and increasing strain competitiveness (van Dillewijn et al., 2001; Pobigaylo et al., 2008). We also found this 26 kb region present in LPU63, which is highly conserved in content and synteny (Figure S1), pointing to these genes as attractive candidates to study in the competition of rhizobia for the host plant.
Lastly, a MLSA based phylogenetic tree with chromosomal housekeeping genes was constructed in order to compare strain LPU63 with the recently described INTA strains isolated from different eco-regions of Argentina (Brambilla et al., 2019). The genomes of these strains have not been sequenced yet, however, Bambrilla et al., (2019) reported the sequence of several housekeeping genes, that we here use for the MLSA analysis. We included the orthologs from strain LPU63 of these housekeeping genes. This phylogenetic study showed that LPU63 diverges from the INTA-1 to INTA-6 strains (Figure 2). Altogether, these findings clearly indicate that strain LPU63 is not a re-isolation of a previously described S. meliloti strain.
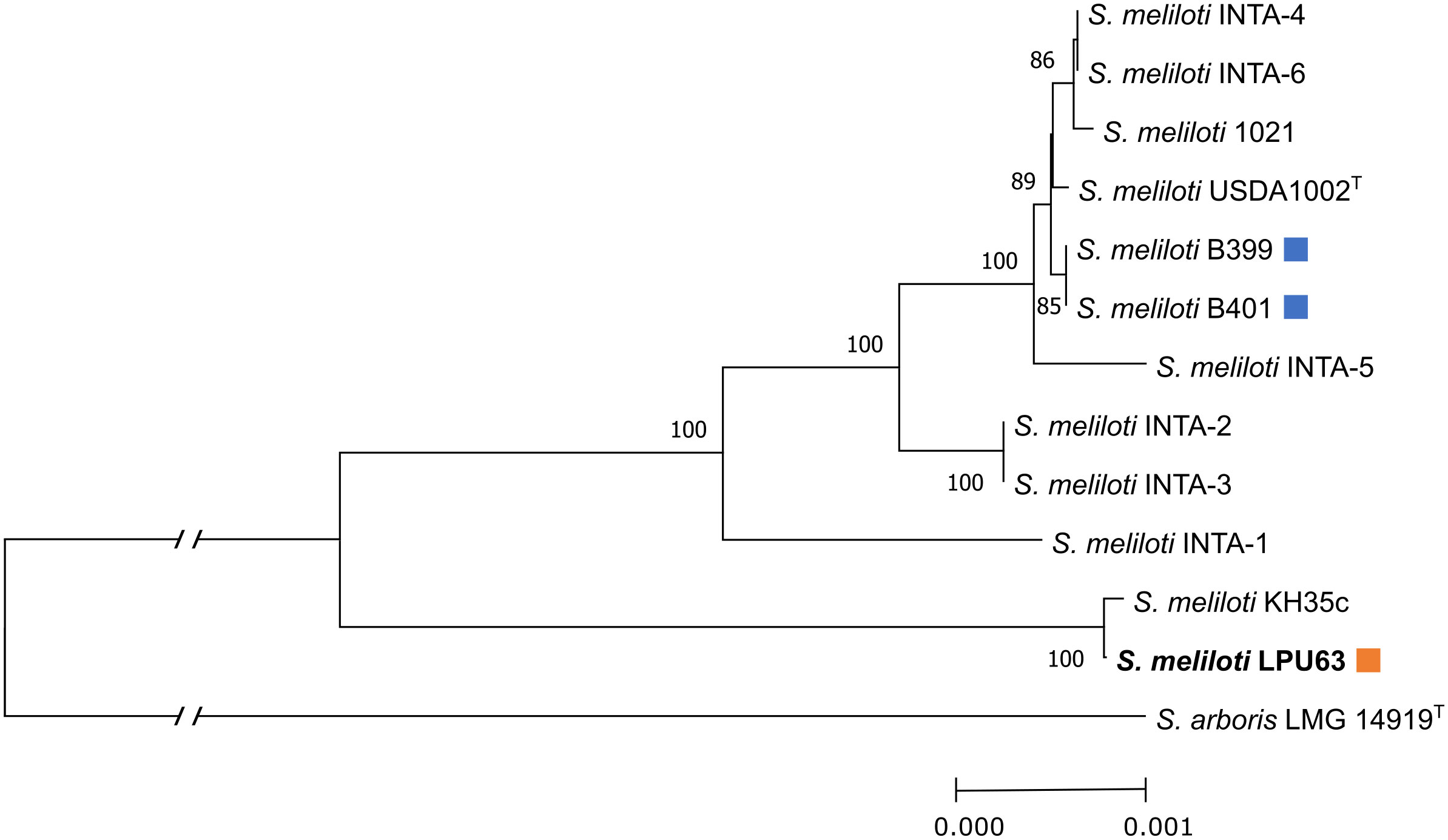
Figure 2 MLSA phylogenetic analysis. Phylogenetic study based on the DNA sequence of 8 chromosomal housekeeping genes from several S. meliloti strains. The percentage of trees in which the associated taxa clustered together is shown next to the branches. The evolutionary history was inferred using the Neighbor-Joining method (Saitou and Nei, 1987). The optimal tree is shown. The percentage of replicate trees in which the associated taxa clustered together in the bootstrap test (1000 replicates) are shown next to the branches (Felsenstein, 1985). The tree is drawn to scale, with branch lengths in the same units as those of the evolutionary distances used to infer the phylogenetic tree. The evolutionary distances were computed using the Tamura-Nei method (Tamura and Nei, 1993) and are in the units of the number of base substitutions per site. This analysis involved 13 nucleotide sequences. All ambiguous positions were removed for each sequence pair (pairwise deletion option). There were a total of 18017 positions in the final dataset. Evolutionary analyses were conducted in MEGA X (Kumar et al., 2018). Superscript T indicates that strains are type strains. The orange squares indicate the strain analyzed in this work; the light blue squares denote rhizobia that is used for commercial inoculant production in Argentina. S. meliloti LPU63 diverges from other strains isolated from Argentina (INTA1 to INTA6, B399, and B401).
3.3 Genome overview: identification of replicons, syntenic regions, core and pan-genome
The S. meliloti 1021 reference genome has a chromosome and two megaplasmids, pSymA and the pSymB chromid, but no other small accessory plasmids (Galibert et al., 2001). However, several S. meliloti strains carry smaller accessory plasmids (also called cryptic plasmids), that code for genes implicated in adaptation to the specific niche they occupy, some of which have been shown to affect nodulation and metabolic potential (Crook et al., 2012; Mazur and Koper, 2012). This is the case of the accessory plasmid pSmeSM11a, that among other genes codes for acdS, suggested to modulate the levels of the phytohormone ethylene and potentially enhancing the nodulation capacity of the rhizobia (Stiens et al., 2006). Cryptic plasmids can also attenuate nitrogen fixation in alfalfa, as it is the case for S. meliloti SAF22 carrying the plasmid pRmSAF22c (Velazquez et al., 1995). Accessory plasmids are generally thought to be transient components (easily gained or lost) from the species pan-genome.
LPU63 contigs were mapped to the corresponding replicon of S. meliloti KH35c, the closest available strain with a complete sequenced genome (Table S3). Most of the contigs were related to the chromosome or the pSymA and pSymB megaplasmids. Also, several contigs mapped to the accessory plasmid A of strain KH35c (Table S3). This result is consistent with the plasmid profile shown in Figure 3, which indicates that LPU63 carries an accessory plasmid of approximately 200 kb. This accessory plasmid was named pLPU63a.
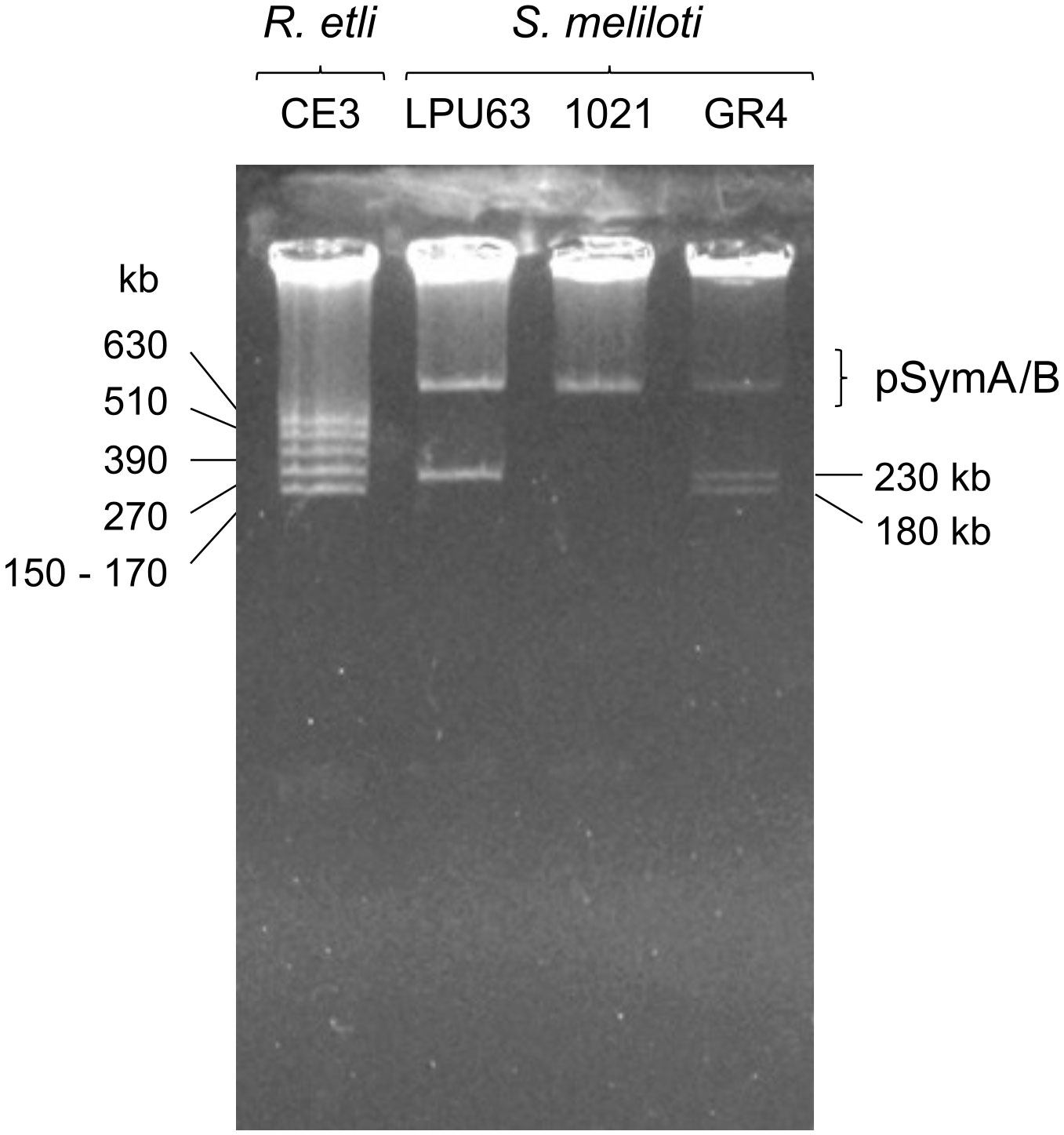
Figure 3 Plasmid profiles obtained in gels of in situ lysis. S. meliloti LPU63 carries an accessory plasmid of approximately 200 kb. Plasmid sizes were estimated from R. etli CE3 which carries plasmids of known sizes. S. meliloti GR4 harbors two accessory plasmid of sizes 176 kb and 226 kb. S. meliloti 1021 does not have any accessory plasmids.
Considering that LPU63 was isolated from acidic soil, it is tempting to speculate that genes coded in this accessory plasmid could provide functions to overcome this specific abiotic stress. Therefore, we tried to reconstruct the sequence of this accessory plasmid with cBar and PlasFlow. However, the results were unreliable, due to the presence of sequencing reads from the pSymA and pSymB megaplasmids. This shows that the assembly of the accessory plasmid with these methods is not possible as they are not able to distinguish the reads belonging to the megaplasmids or the cryptic plasmid. Therefore, we designed a strategy to look for which contigs potentially belong to the LPU63 accessory plasmid. All contigs were first used in a BLASTn search against sequences of accessory plasmids isolated from several S. meliloti strains (López et al., 2019). Contigs that showed a query coverage larger than 5 kb were selected. Next, considering that samples of purified cryptic plasmid are usually contaminated with pSym or chromosomal DNA, we discarded the contigs that mapped to these replicons of S. meliloti KH35c. Contigs that passed this test and putatively belong to plasmid pLPU63a are shown in Table S4. Genes that code for a conjugative T4SS, plasmid replication and a module of the repABC partitioning system were identified in the pLPU63a putative contigs suggesting that this plasmid could be self-transmissible (Table S5). These results should be interpreted with caution, as our analysis are unable to distinguish whether these contigs belong to only one or more than one accessory plasmid of the strain LPU63, since it is challenging to reconstruct plasmids from short read sequencing.
In addition, the BLAST analysis of gene content of the contigs putatively assigned to pLPU63a indicated that more genes found on the accessory genome were found only on other Sinorhizobium criptic plasmids or pSymA, rather than pSymB or the chromosome. Nelson et al. (2018) suggests that the accessory plasmids and pSymA have greater ability to exchange genetic elements than the other replicons. Whereas the pSymA is enriched in genes associated with signal transduction (COG-T) and energy production (COG-C) compared to pLPU63a, the latter is enriched in genes promoting trafficking and transport (COG-U).
Previous studies have shown that cryptic plasmids of S. meliloti have different codon usage compared to the chromosome or the pSyms (Lopez et al., 2019). To confirm the designation of LPU63 contigs to the accessory plasmid, we performed a correspondence analysis of RSCU. We included all the KH35c replicons (chromosome, pSyms, and accessory plasmid), all accessory plasmids of S. meliloti available on the NCBI database, and LPU63’s contigs. As shown in Figure 4, LPU63 contigs (big red dots) assigned as accessory plasmid had a modal codon usage that clustered together with other cryptic plasmids of S. meliloti (big turquoise dots).
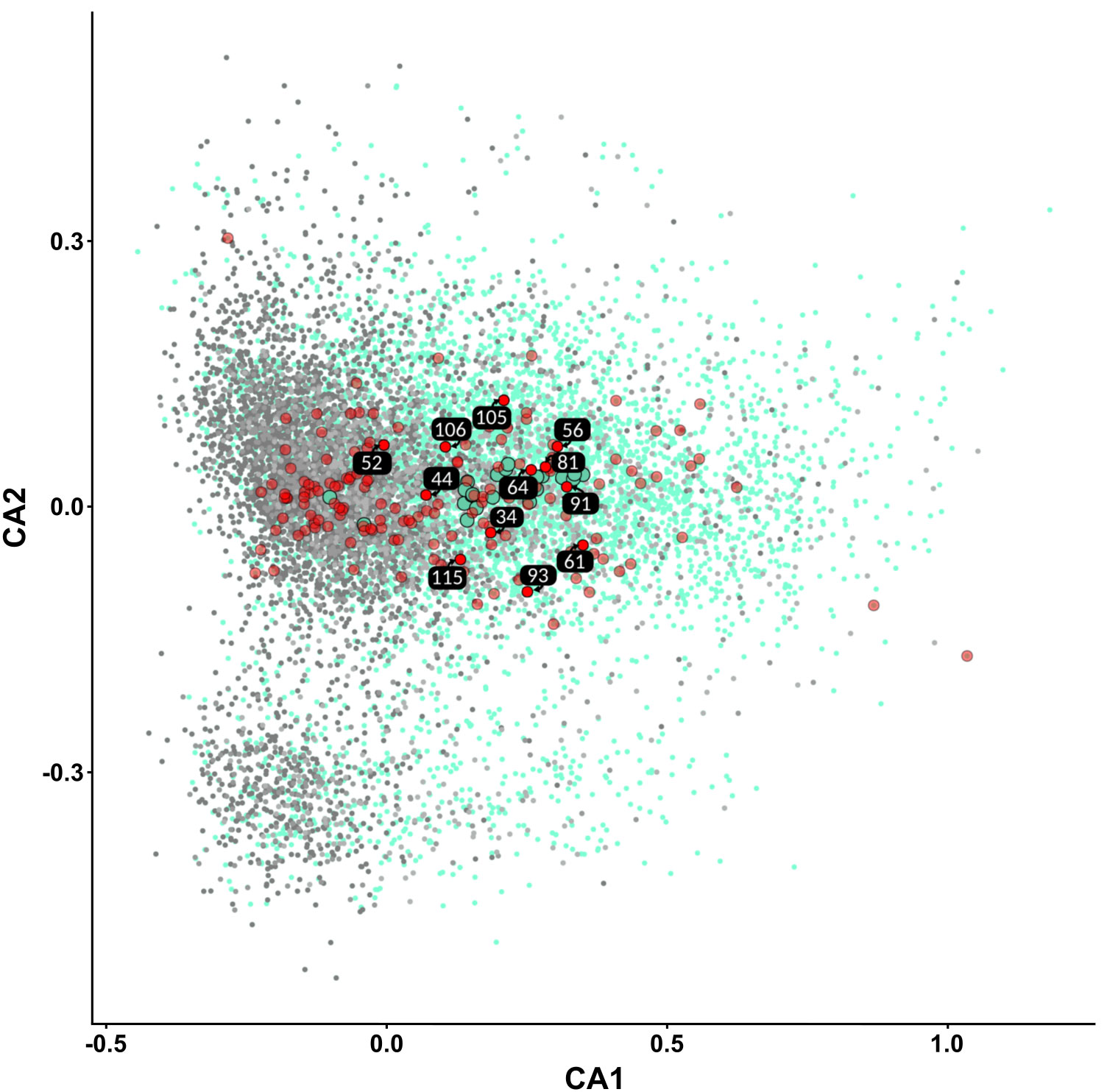
Figure 4 Analysis of the codon usage profiles of S. meliloti LPU63 contigs. Correspondence analysis of the Relative Synonymous Codon Usage (RSCU) frequencies of genes from S. meliloti KH35c genome, S. meliloti accessory plasmids and S. meliloti LPU63 contigs. KH35c chromosomal and pSyms genes (gray), S. meliloti accessory plasmid genes (turquoise), and LPU63 contigs (red). Small dots represent the RSCU of individual genes. Big dots represent modal codon usage frequencies.
Finally, to determine the accessory genome of S. meliloti LPU63 a pan-genome analysis was done using the genomes of S. meliloti strains listed in Table S1. The pangenome is a collection of all DNA sequences of a species that contains sequences shared by all individuals (core genome) and is also able to display sequence information unique to each individual (variable genome). LPU63 singletons (unique, exclusive genes) found are listed on Table S6.
3.4 Analysis of symbiosis-related and nitrogen fixing proteins of LPU63
LPU63 genes involved in nodulation and nitrogen fixation were identified by BLAST search using the already characterized S. meliloti 1021 genes. Nod factor biosynthesis genes (nod, noe, and nol) are involved in the synthesis of hosts specific lipochitooligosaccharides which are indispensable for symbiosis initiation. All the fundamental genes involved in the synthesis of the Nod factor and genes that codified enzymes involved in nitrogen fixation and their corresponding regulators were identified (Table S7 and Table S8). Most of the genes were mapped to the putative pSymA replicon in strain LPU63. This result is consistent with the location of the symbiotic-related genes directly involved in symbiotic nitrogen fixation (such as the nod, nif and fix genes) to the pSymA megaplasmid in other S. meliloti strains. By examining the genomic data of S. meliloti strains 1021 and LPU63, we can locate these Nod factor biosynthesis genes (nodM, nolFG, nodN, nodD1ABCIJ, nodQPGEFH, syrM, nodD3, noeBA, nodL) into their correct order and they present a syntenic organization. The conservation of blocks of order within these two chromosomes is one of the most reliable criteria for establishing the orthology of genomic regions and can reflect important functional relationships between genes. As expected, also LPU63 contain orthologs of the key nitrogen fixation genes (nifH, nifD, nifK, nifA, nifBEN, fixABCX, fixNOPQ, fixLJ, fixK, fixGHIS, and fdxN), mainly co-located on megaplasmid pSymA, with the Nod factor biosynthesis gene clusters. As shown in Table S7 and Table S8, Nod and nitrogen fixation proteins of LPU63 present more than 96% and 92% sequence identity respectively with their homologous. The exception with lower protein identity are NodQ2 and FixT2 (57.4% and 76.5% protein identity, respectively).
Other genes putatively involved in competition or interaction with their host plant, such as genes involved in secretion systems and flagella, were identified with TXSScan (Table S9). Several chromosomally encoded flagellum genes were found in LPU63. In S. meliloti flagellum motility has been associated with competitiveness for nodule occupation (Bernabéu-Roda et al., 2015). Several T1SS coding genes were identified in the chromosome and in pSymB. We also found putative conjugative bacterial type IV secretion systems (T4SSs), one located in pSymA and another in pLPU63a.The T4SSs are the specific nano machine for bacterial horizontal gene transfer. These devices mediate the dissemination of genes via horizontal gene transfer and contribute to the bacteria’s evolution. In addition, Nelson et al. (2017) showed that Sinorhizobium uses a T4SS during the initiation of symbiosis with Medicago spp. and alters Medicago cells in planta during symbiosis. Previous studies have shown that the S. meliloti 1021 megaplasmid pSymA is self‐transmissible (Pérez-Mendoza et al., 2005). Therefore, the pSymA and pLPU63a of LPU63 could be also conjugated via a T4SS.
Two putative Tight adherence (Tad) gene clusters were identified in the genome of LPU63, one encoded in the chromosome and the other on pSymA. S. meliloti 1021 also contains two gene clusters coding for Tad: flp-1, which is chromosomally-encoded, and flp-2, located on the pSymA megaplasmid (Zatakia et al., 2014). These gene clusters are homologous to the Tad loci present in Caulobacter crescentus (Skerker and Shapiro, 2000) and Aggregatibacter actinomycetemcomitans (Kachlany et al., 2000). Flp-1 was found to be important for the plant nodulation of S. meliloti 1021, indicating that this pilus might enhance the attachment of the bacteria to plant roots (Zatakia et al., 2014). The reason for S. meliloti to possess two distinct Tad loci is still unclear, but the maintenance of the flp-2 locus on pSymA suggests a specific role during the free-living or symbiotic state. Further studies are required to understand the role of flp-2 in the early stages of the symbiotic process.
3.5 Genes involved in acid tolerance
The study of rhizobial strains under acidic conditions showed that acid-tolerant related traits are multigenic or strain-dependant (Glenn et al., 1999; Draghi et al., 2016). As a first step to try to unravel the molecular determinants involved in the acidic tolerance of strain LPU63, we searched for orthologs of genes already characterized in other rhizobia that showed to play a role in the resistance to low pH or that were upregulated in this condition (Table S10). LPU63 codes for several genes putatively involved in acid tolerance, such as the two-component systems (TCS) ActSR (Tiwari et al., 1996) and ActJK (Albicoro et al., 2021; Albicoro et al., 2023). Most of the genes found to be important for acid tolerance in Rhizobium strains had orthologs in S. meliloti LPU63.
3.6 Denitrification pathway
Rhizobia can use nitrate as the final electron acceptor, in a process known as denitrification. Although this metabolic pathway is crucial for the survival of rhizobia in anoxic environments, it is considered one of the main drawbacks in the use of these bacteria as inoculants, because this process involves the generation of greenhouse gases. Particularly, the use of S. meliloti strains that have the nap-nir-nor gene cluster, which can start the denitrification process, but lack the nos gene cluster (involved in the last step of this pathway, converting the greenhouse N2O into the inert N2) is not desirable. This is the case of the S. meliloti B399 strain, extensively used for decades in Argentina as alfalfa inoculant, which has recently been shown to produce high levels of N2O during symbiosis (Brambilla et al., 2020). We were therefore interested in determining the integrity of the denitrification pathway in strain LPU63. Our results show that LPU63 codes the complete denitrification pathway, in an almost identical gene arrangement as the reference strain 1021 (Figure 5, Table S11). The genome sequence revealed the presence of the napEFDABC, nirK, norECBQD and nosRZDFYLX denitrification genes (Torres et al., 2014). As such, it is possible to speculate that the N2O emission of this strain could be comparable to the levels of strain 1021, which are significantly lower than strain B399 (Brambilla et al., 2020).
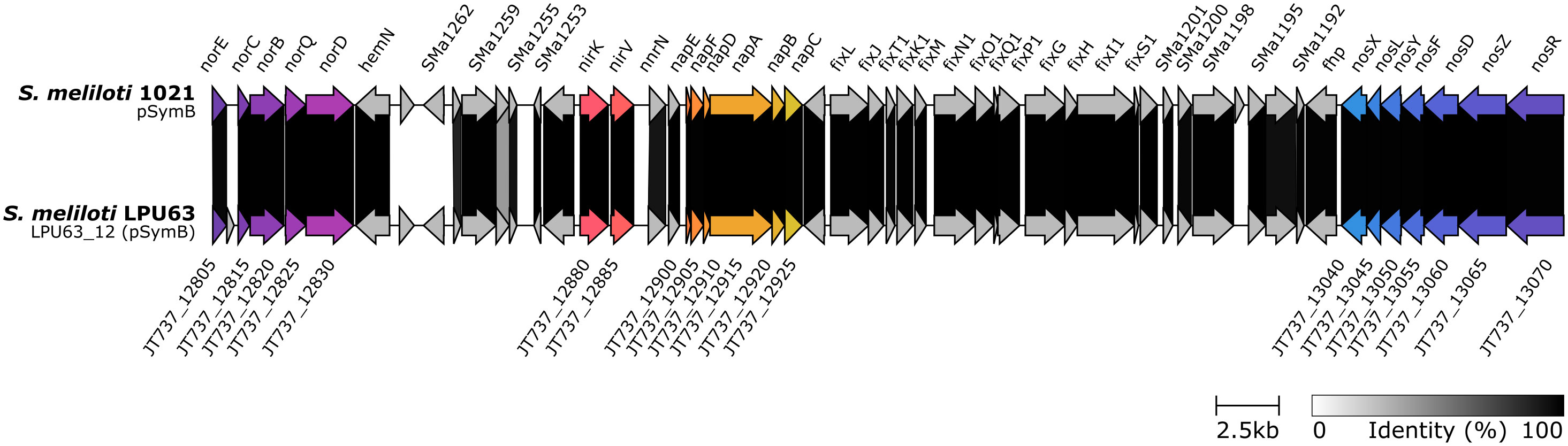
Figure 5 S. meliloti LPU63 genome codes for the complete denitrification pathway. Synteny plot of the genomic region coding for the denitrification pathway (nor, nir, nap and nos gene clusters) performed with Clinker.
Alfalfa root nodules produce N2O and Nap has an important role on its release from nodules in response to nitrate and flooding (Pacheco et al., 2023). Recently, a novel strategy to avoid these undesirable metabolic pathways was the screening of S. meliloti strains with spontaneous mutations in napC, which encodes the nitrate reductase structural protein NapC involved in the first step of N2O production via the denitrification process. These napC minus strains are consequently unable to start the denitrification pathway (Brambilla et al., 2020). While these strains are regarded as eco-friendly, due to the extremely low N2O production in the symbiosis with alfalfa, it remains to be proven whether they are able to survive in field conditions, especially when O2 is limited.
Altogether, strain LPU63 could be regarded as a compromise solution, emitting low N2O levels but capable, under low O2 environments, of utilizing nitrate or nitrate as final electron acceptors, a useful trait for the survival of this bacterium in adverse soil conditions.
3.7 Phenylacetic acid degradation pathway
For the aerobic degradation route of phenylacetic acid (PAA), in a first stage, PAA is activated to PAA-CoA in the presence of ATP and Mg2+ by a CoA-ligase. Subsequently, it was postulated that the enzymatic complex composed of the proteins encoded in the paaABCDE operon would catalyze the hydroxylation of the aromatic ring of PAA-CoA. This complex would give rise to a non-aromatic product that is subsequently metabolized in a complex sequence of reactions.
Comparative genomic studies of numerous bacterial species involved in the nodulation of legume plants have positioned the paaABCDE cluster as a possible molecular determinant of the interaction between plants and rhizobia (Seshadri et al., 2015). This cluster belongs to a group of genes involved in the degradation of PAA. Therefore, the presence of the catabolic cluster could promote some adaptive advantages for the rhizobium. PAA is the auxin produced in greatest quantity by many plant species (Cook, 2019), and has been detected in root exudates in different plants, including legumes (Rovira, 1969). Thus, the available PAA levels imply the possible use of this aromatic compound as an alternative carbon source for rhizobia, consequently comprising an adaptive advantage in the colonization of the rhizosphere. Alternatively, the metabolism of PAA by rhizobia could intervene in the modulation of responses from the host plant. In addition, PAA is one of the degradation products of plant-derived flavonoids by S. meliloti (Rao and Cooper, 1994) and the expression of the paaABCDE gene cluster was found to be increased in nodule bacteria (Barnett et al., 2004). S. meliloti PAA degradation genes were also found to be induced by oxidative stress (Lehman et al., 2018) and by the entry into stationary phase (Sauviac et al., 2007). PAA catabolism ultimately serves as a carbon source that provides intermediates for the TCA cycle.
The presence of paa genes in the strain LPU63, including the genes that code for catalytic enzymes involved in PAA degradation, transport, and transcriptional regulation, is currently unknown. Due to the emerging role of this pathway in rhizobia-legume interactions, we performed a BLAStp search in the LPU63 genome and related rhizobia for gene homologues involved in PAA metabolism previously characterized in Escherichia coli (Table S12). As shown in Figure 6, the analysed rhizobia have a conserved gene cluster for the degradation of PAA. Our analysis also indicate similarity at protein level and the presence of syntheny between the paa-cluster found in LPU63 and WSM419, despite them belonging to different Sinorhizobium species. Particularly, S. meliloti and S. medicae have genes that code for the PaaABCDE, PaaG, and PaaZ proteins, involved in the enzymatic steps required for the hydroxylation and rupture of the aromatic ring present in PAA, a crucial step for the degradation of this molecule. These strains also code for a homologue of the paaX gene, a transcriptional repressor that responds to intracellular PAA-CoA levels, and two ABC transporters that could be involved in the uptake of PAA. S. fredi and S. americanum have a more complete PAA degradation gene cluster, including PaaK, which catabolizes the first step in this pathway by generating the PAA-CoA intermediate, and PaaI. Interestingly, in these strains paaX seems to have been replaced by a different transcriptional regulator (SAMCCGM7_Ch2788 in S. americanum CCGM7).
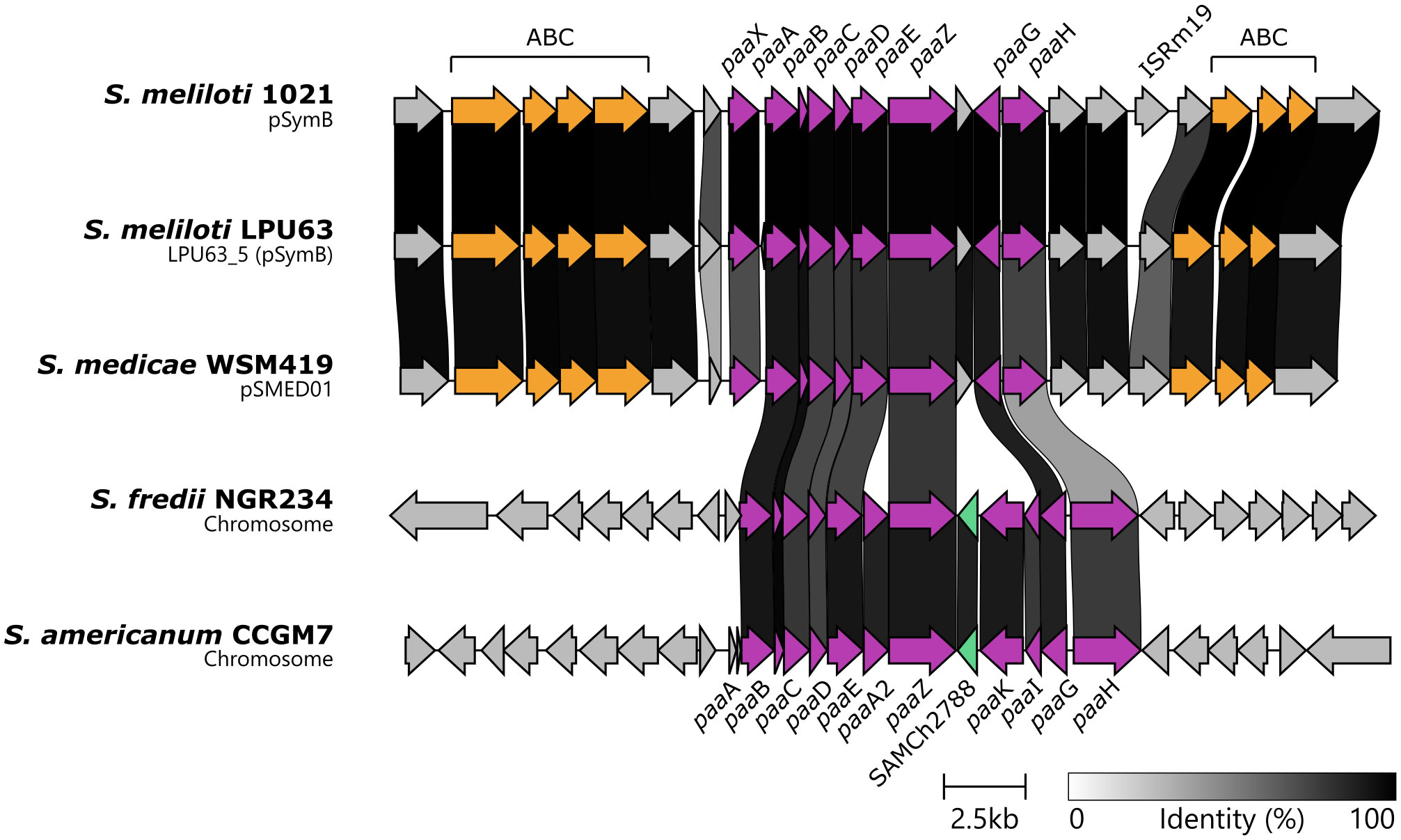
Figure 6 Conservation of the PAA catabolic gene cluster in rhizobia. Synteny plot of the PAA degradation gene cluster (violet arrows) of several rhizobia performed with Clinker. Two ABC transporter gene clusters putatively involved in PAA transport are depicted with orange arrows.
The identification of a clear paaK homolog remains elusive in the S. meliloti strains analyzed. In this species, either there is another protein that catabolizes the CoA activation of PAA, or this step is not required. However, the conservation of the PAA catabolic gene cluster and its respective expression in S. meliloti implies that it confers some adaptive advantage on the rhizobium, even in the absence of paaK.
The ability to degrade PAA is emerging as a relevant feature in host-bacteria interactions. For example, the PAA degradation gene cluster is the largest region acquired by horizontal gene transfer in the Mycobacterium abscessus genome (Ripoll et al., 2009). PAA degradation was found involved in the pathogenicity of Burkholderia cenocepacia (Law et al., 2008). Both M. abscessus and B. cenocepacia are opportunistic pathogens in cystic fibrosis patients. To our knowledge the functional role of PAA degradation in the rhizobia-legume symbiosis has not yet been investigated.
3.8 Melanin production
S. meliloti LPU63 was described as a melanin producing strain (Del Papa et al., 1999). The production of melanin is involved in overcoming abiotic and biotic stress, a trait that could enhance the survival of this strain in soil. In rhizobia, the synthesis of melanin is linked to two genes, the mcoA (encode a multicopper oxidase) and mepA (encode a melanin production protein with tyrosinase activity)(Mercado-Blanco et al., 1993; Chizhevskaya et al., 2018). In our genomic sequencing of LPU63, the 5’ end of mcoA was found at the end of contig LPU63_25 and the 3’ end at the beginning of contig LPU63_173. We designed primers to PCR-amplify the complete mcoA gene and the amplicon sequencing confirmed the proposed junction of contigs LPU63_25 with LPU63_173. Interestingly, in strain LPU63 there is a frameshift in mcoA producing two separate ORFs, designed as mcoA1 and mcoA2 (Figure 7A) which may be functional because melanin production is observed in strain LPU63 (Figure 7B). The same genetic arrangement is found in S. meliloti HM006.
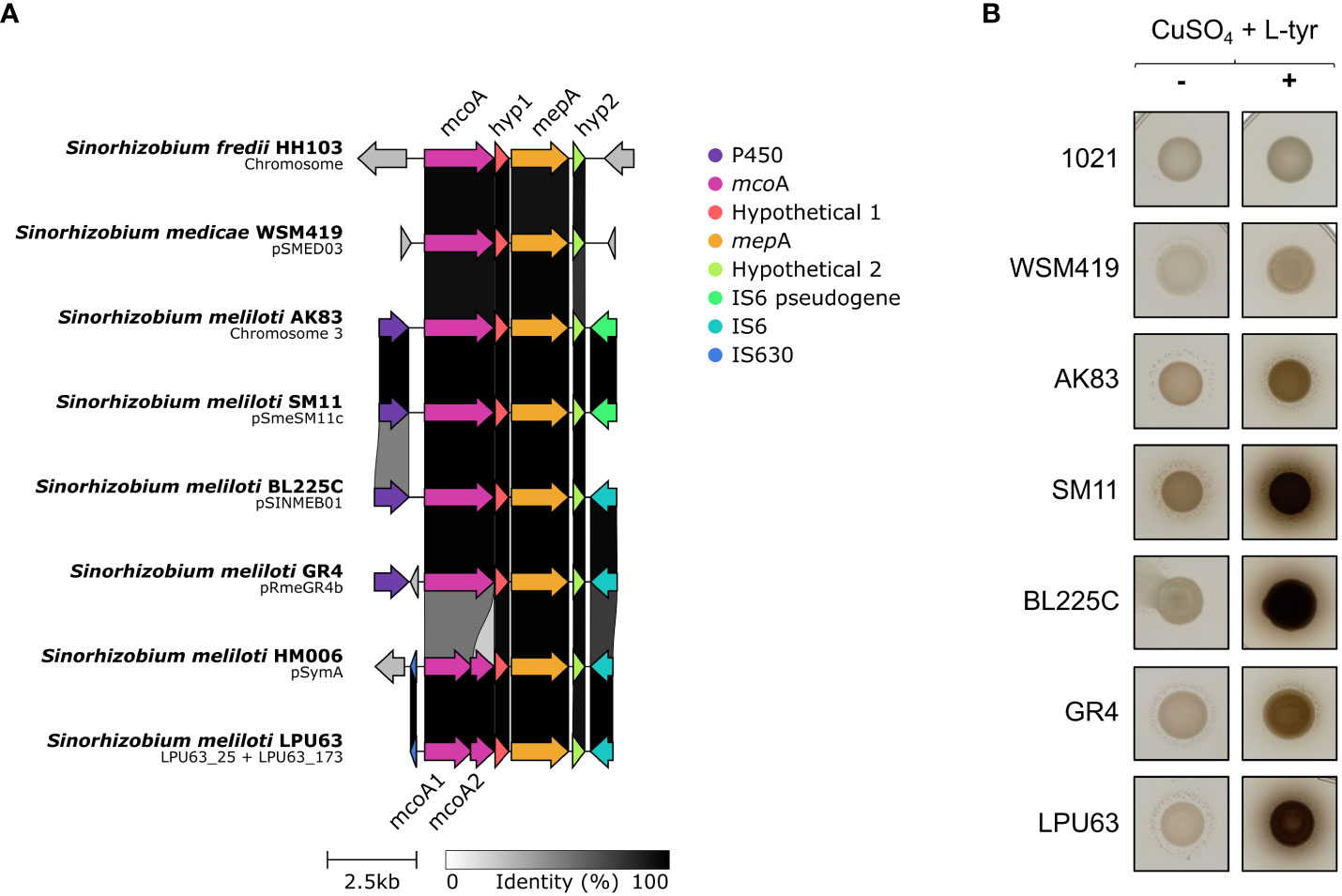
Figure 7 Melanin production by S. meliloti LPU63 and related rhizobia. (A) Analysis of the gene cluster involved in melanin production. In strains LPU63 and HM006 the mcoA gene is split in two genes by a frameshift mutation. Two genes coding for hypothetic proteins (Hyp1 and Hyp2) are highly conserved in rhizobia. (B) Melanin production was evidenced by culturing strains in TY media supplemented with 40 µg mL-1 CuSO4 and 100 µg mL-1 L-tyrosine for 3 days at 28°C and then 10% (w/v) SDS was added. Positive strains show a dark brown pigment.
The production of melanin by LPU63 was compared with other previously reported melanin producing strains with an intact mcoA gene (such as S. meliloti GR4) (Mercado-Blanco et al., 1993; Castro-Sowinski et al., 2002) following the protocols previously described. The medium was supplemented with CuSO4 and L-tyrosine to attain the maximum melanin yield because previous reports had shown that absence of the tyrosinase cofactor Cu in culture medium, results in the synthesis of inactive tyrosinase (Cabrera-Valladares et al., 2006). LPU63 produced high amounts of melanin in the presence of CuSO4 and L-tyrosine, with levels similar to those of strain BL225C and SM11 (Figure 7B). Thus, the split of mcoA into two different genes does not seem to have a detrimental effect on melanin production. The proficient melanin production by strains LPU63, BL225C and SM11 could be due to a high level of mcoA and mepA transcripts or to the presence of additional genes involved in melanin synthesis. The genomic comparisons revealed that, in rhizobia, two hypothetical proteins are conserved in the mcoA-mepA region, suggesting a putative role in melanin production.
Other than their biological role and, due to their particular chemical structure, microbial melanins are used in diverse industrial applications such as: X-ray, γ-ray and UV absorption; semiconduction; cation exchange; bioremediation, among other applications (Singh et al., 2021). Interestingly, LPU63 produced melanin even in a medium with CuSO4 as low as 4 µg mL-1 in the absence of L-tyrosine, suggesting that this strain could be used in the cost-effective industrial production of melanin.
3.9 Plant growth promoting rhizobacteria activities
In addition to nitrogen fixation, the presence of additional bacterial activities that promote plant growth present in LPU63 could enhance its value as a potential bioinoculant of alfalfa. Therefore, we searched for PGPR coding genes following the method of Bruto et al. (Bruto et al., 2014).
Solubilizing bacteria convert unavailable forms of P (both inorganic and organic) into available nutrients that can be absorbed. The phosphate solubilisation process helps meet the P requirements of plants. Soil-dwelling bacteria solubilize mineral phosphates by secreting gluconic acid. This compound is produced from glucose by a periplasmic glucose dehydrogenase (GDH) that uses pyrroloquinoline quinone (PQQ) as a redox coenzyme. We found that LPU63 codes for the pqqBCDE gene cluster necessary for the PQQ cofactor biosynthesis, involved in phosphate solubilization. Then, we confirmed the in vitro phosphate solubilization capacity of strain LPU63 (Figure 8), which shows a clear halo around the colony in NBRIP media, as the G. diazotrophicus Pal5 positive control and S. meliloti AK83 used as the reference strain for the pqq gene cluster identification.
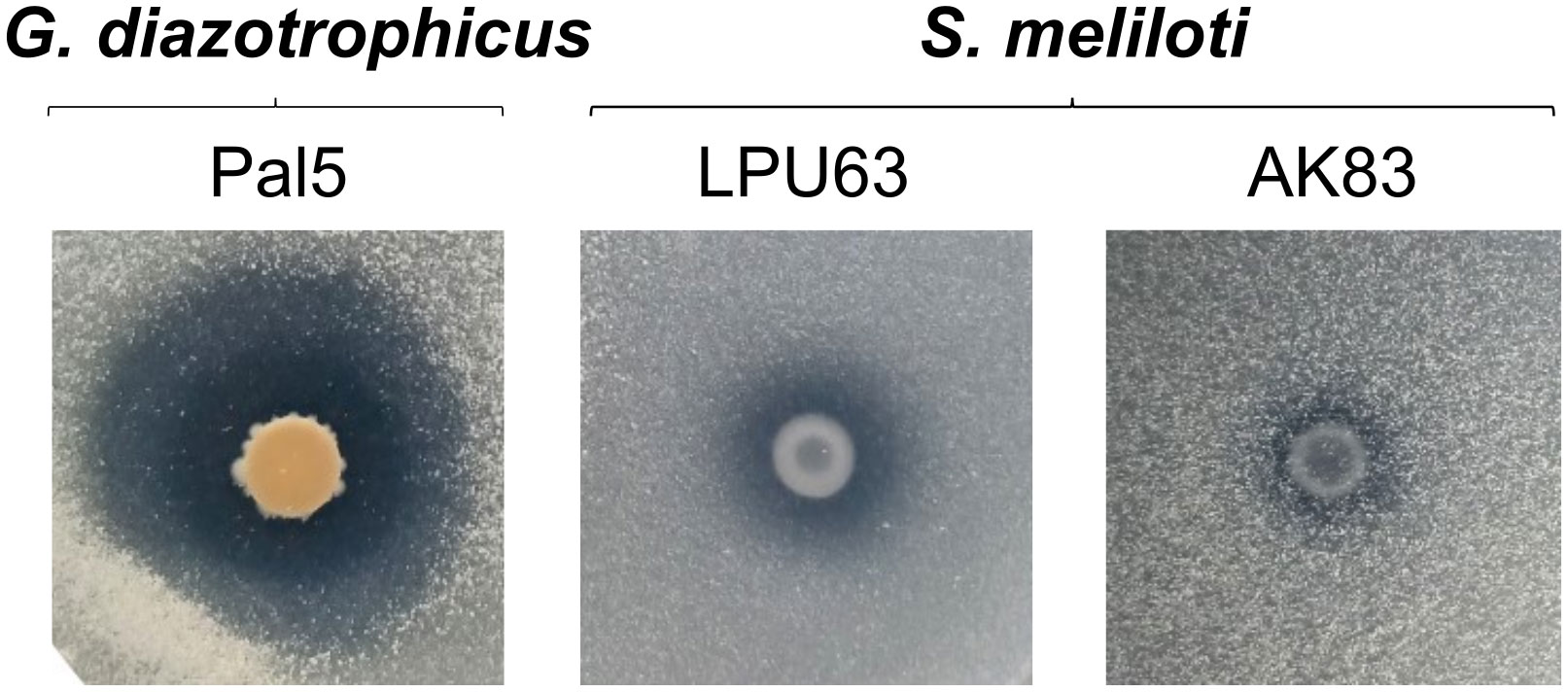
Figure 8 Mineral phosphate solubilization. Solubilization of mineral phosphate was tested on plates containing NBRIP growth medium. Gluconacetobacter diazotrophicus Pal5 was used as a positive control. The presence of a transparent halo is considered as a positive test. S. meliloti LPU63 and AK83 (pqq gene cluster reference strain) solubilize mineral phosphate.
Some soil bacteria produce the enzyme 1-aminocyclopropane-1-carboxylate (ACC) deaminase which promotes plant growth by sequestering and cleaving plant-generated ACC, thereby reducing the level of ethylene in the plant. This allows the plant to be more resistant to a wide variety of environmental stresses. In addition, acdS was detected in LPU63 strain (Table 2). These PGPR activities are consistent with those found in other S. meliloti strains (Bruto et al., 2014). Interestingly, it was recently reported that the transfer of an ACC deaminase gene from S. medicae WSM419 to S. meliloti 1021 increases nodulation and improves plant growth in the symbiosis with Medicago truncatula (Ghosh et al., 2021). In this study, it was also shown the expression of acdS in symbiotic and in free-living bacteria confer improvements to nodule formation and symbiotic productivity. The above results indicate the relevance and potential benefits of the acdS in LPU63 strain.
We also searched for genes homologs to phlABCD (required for 2,4-diacetylphloroglucinol synthesis), idpC (required for auxin synthesis) and budABC (required for acetoine/2,3-butanediol synthesis). None of these PGPR-related genes were found. A study based on ancestral character reconstruction has proposed that PGPR-plant cooperation may have been established separately in various taxa, yielding PGPR strains that use different gene assortments (Bruto et al., 2014). According to our study, LPU63 strain (like many PGPR strains) is multifunctional, i.e., it harbors more than one phyto-beneficial trait, then all PGPR activities detected make strain LPU63 an even more attractive candidate for use as an alfalfa inoculant.
4 Conclusion
Inoculant selection should be viewed as a continuous process, that complements the choice and implementation of new plant cultivars, as well as to changes in the cropping areas, in the soil and in environmental conditions. The genetic stability of the bacterial strains should also be considered since long-term storage could involve genotypic and phenotypic changes that could alter the rhizobium´s capacity to fix nitrogen, among other relevant characteristics.
This work presents an in-depth analysis of the genome of an economically and eco-friendly promising strain LPU63. The genome of LPU63 is represented by a chromosome, two megaplasmids, and also has an accessory plasmid, pLPU63a. This potentially conjugative replicon contained traA, the T4SS gene cluster, and type IV coupling protein. Our analyses based on ANIb and core phylogenetic trees supported the taxonomy of the strain LPU63 as S. meliloti.
In addition to the previously reported outstanding competitiveness, persistence and efficiency of strain LPU63 in acidic and neutral conditions, the information regarding LPU63 activities compatible with plant-growth promotion phenotypes, together with other characteristics examined here (presence of paa cluster, high-level production of melanin and the presence of the complete denitrification pathway that could be related with moderate N2O emissions), constitute the basis of future experiments tending toward the rational design of a novel bioinoculant for environmentally sustainable alfalfa production. This could impact by increasing the stability of forage production and could lead to an increase in the extension of the cultivation area.
The detailed genome annotation of strain LPU63 provides a rewarding resource, allowing the use of comparative genomics as a powerful tool to figure out the genetic mechanisms that could contribute to the phenotype of this strain and sustain the rhizobium–Medicago symbiotic interaction.
Further analysis of the genomic features in beneficial rhizobia could clarify how some bacterial strains establish a highly efficient symbiosis or present superior performance in certain edaphic environments.
Data availability statement
The datasets presented in this study can be found in online repositories. The names of the repository/repositories and accession number(s) can be found in the article/Supplementary Material.
Author contributions
The authors confirm their contribution to the paper as follows: Study conception and design: MD. Methodology: JC, MS, CV, SL. Software and formal analysis: JC, ML and AL. Investigation: JC, MS, CV, WD. Data curation: ML. Writing and original draft preparation: JC and MD. All authors contributed to the discussion, provided comments on the manuscript, and approved the submitted manuscript version.
Funding
This work was partially funded by the MinCyT, Argentina Grant PICT2020-1475 provided to MD. ML, AL and MD are members of the Research Career of CONICET, Argentina.
Acknowledgments
The authors are grateful to Susan Rogers, a native English speaker, who edited the final version of the manuscript. We thank Jochen Blom for helping with EDGAR platform. EDGAR is financially supported by the BMBF within the de.NBI network.
Conflict of interest
The authors declare that the research was conducted in the absence of any commercial or financial relationships that could be construed as a potential conflict of interest.
Publisher’s note
All claims expressed in this article are solely those of the authors and do not necessarily represent those of their affiliated organizations, or those of the publisher, the editors and the reviewers. Any product that may be evaluated in this article, or claim that may be made by its manufacturer, is not guaranteed or endorsed by the publisher.
Supplementary material
The Supplementary Material for this article can be found online at: https://www.frontiersin.org/articles/10.3389/fagro.2023.1175524/full#supplementary-material
References
Abby S. S., Néron B., Ménager H., Touchon M., Rocha E. P. C. (2014). MacSyFinder: A Program to mine genomes for molecular systems with an application to CRISPR-Cas systems. PloS One 9 (10), e110726. doi: 10.1371/journal.pone.0110726
Albicoro F. J., Draghi W. O., Martini M. C., Salas M. E., Torres Tejerizo G. A., Lozano M. J., et al. (2021). The two-component system ActJK is involved in acid stress tolerance and symbiosis in Sinorhizobium meliloti. J. Biotechnol. 10;329, 80–91. doi: 10.1016/j.jbiotec.2021.01.006
Albicoro F. J., Vacca C., Cafiero J. H., Draghi W. O., Martini M. C., Goulian M., et al. (2023). Comparative proteomic analysis revealing actJ-regulated proteins in Sinorhizobium meliloti. J. Proteome Res. 22 (6), 1682–1694. doi: 10.1021/acs.jproteome.2c00731
Andrews S. (2010). FastQC: a quality control tool for high throughput sequence data (Babraham Institute, Cambridge, United Kingdom: Babraham Bioinformatics).
Barnett M. J., Toman C. J., Fisher R. F., Long S. R. (2004). A dual-genome symbiosis chip for coordinate study of signal exchange and development in a prokaryote–host interaction. Proc. Natl. Acad. Sci. 101 (47), 16636–16641. doi: 10.1073/pnas.0407269101
Bellabarba A., Bacci G., Decorosi F., Aun E., Azzarello E., Remm M., et al. (2021). Competitiveness for nodule colonization in Sinorhizobium meliloti: combined in vitro-tagged strain competition and genome-wide association analysis. Msystems 6 (4), e00550–e00521. doi: 10.1128/mSystems.00550-21
Beringer J. (1974). R factor transfer in Rhizobium leguminosarum. Microbiology 84, 1, 188–198. doi: 10.1099/00221287-84-1-188
Bernabéu-Roda L., Calatrava-Morales N., Cuéllar V., Soto M. J. (2015). Characterization of surface motility in Sinorhizobium meliloti: regulation and role in symbiosis. Symbiosis 67 (1), 79–90. doi: 10.1007/s13199-015-0340-4
Bertalan M., Albano R., de Pádua V., Rouws L., Rojas C., Hemerly A., et al. (2009). Complete genome sequence of the sugarcane nitrogen-fixing endophyte Gluconacetobacter diazotrophicus Pal5. BMC Genomics 10 (1), 450. doi: 10.1186/1471-2164-10-450
Biondi E. G., Tatti E., Comparini D., Giuntini E., Mocali S., Giovannetti L., et al. (2009). Metabolic capacity of Sinorhizobium (Ensifer) meliloti strains as determined by phenotype microarray analysis. Appl. Environ. Microbiol. 75 (16), 5396–5404. doi: 10.1128/AEM.00196-09
Blom J., Albaum S. P., Doppmeier D., Pühler A., Vorhölter F.-J., Zakrzewski M., et al. (2009). EDGAR: a software framework for the comparative analysis of prokaryotic genomes. BMC Bioinf. 10 (1), 1–14. doi: 10.1186/1471-2105-10-154
Bolger A. M., Lohse M., Usadel B. (2014). Trimmomatic: a flexible trimmer for Illumina sequence data. Bioinformatics 30 (15), 2114–2120. doi: 10.1093/bioinformatics/btu170
Bosi E., Donati B., Galardini M., Brunetti S., Sagot M.-F., Lió P., et al. (2015). MeDuSa: a multi-draft based scaffolder. Bioinformatics 31 (15), 2443–2451. doi: 10.1093/bioinformatics/btv171
Brambilla S., Frare R., Soto G., Jozefkowicz C., Ayub N. (2018). Absence of the nitrous oxide reductase gene cluster in commercial alfalfa inoculants is probably due to the extensive loss of genes during rhizobial domestication. Microbial Ecol. 76 (2), 299–302. doi: 10.1007/s00248-018-1145-9
Brambilla S., Soto G., Odorizzi A., Arolfo V., McCormick W., Primo E., et al. (2020). Spontaneous mutations in the nitrate reductase gene napC drive the emergence of eco-friendly low-N2O-emitting alfalfa rhizobia in regions with different climates. Microbial Ecol. 79, 1044–1053. doi: 10.1007/s00248-019-01473-w
Bruto M., Prigent-Combaret C., Muller D., Moënne-Loccoz Y. (2014). Analysis of genes contributing to plant-beneficial functions in plant growth-promoting rhizobacteria and related Proteobacteria. Sci. Rep. 4 (1), 6261. doi: 10.1038/srep06261
Cabrera-Valladares N., Martinez A., Piñero S., Lagunas-Muñoz V. H., Tinoco R., de Anda R., et al. (2006). Expression of the melA gene from Rhizobium etli CFN42 in Escherichia coli and characterization of the encoded tyrosinase. Enzyme Microb. Technol. 38, 772–779. doi: 10.1016/j.enzmictec.2005.08.004
Casadesús J., Olivares J. (1979). Rough and fine linkage mapping of the Rhizobium meliloti chromosome. Mol. Gen. genetics: MGG 174 (2), 203–209. doi: 10.1007/BF00268356
Castro-Sowinski S., Martinez-Drets G., Okon Y. (2002). Laccase activity in melanin-producing strains of Sinorhizobium meliloti. FEMS Microbiol. Lett. 209 (1), 119–125. doi: 10.1111/j.1574-6968.2002.tb11119.x
Cavalcante V. A., Dobereiner J. (1988). A new acid-tolerant nitrogen-fixing bacterium associated with sugarcane. Plant Soil 108, 23–31. doi: 10.1007/BF02370096
Chen K.-T., Shen H.-T., Lu C. L. (2018). Multi-CSAR: a multiple reference-based contig scaffolder using algebraic rearrangements. BMC Syst. Biol. 12 (9), 69–75. doi: 10.1186/s12918-018-0654-y
Chizhevskaya E. P., Naidenova E. A., Onishchuk O. P., Andronov E., Simarov B. V. (2018). The Melanin biosynthesis gene from the CA15-1 strain of alfalfa nodule bacteria: Molecular Analysis and Phylogeny. Russ J. Genet. 54, 925–932. doi: 10.1134/S1022795418080045
Contreras-Moreno F. J., Muñoz-Dorado J., García-Tomsig N. I., Martínez-Navajas G., Pérez J., Moraleda-Muñoz A. (2020). Copper and melanin play a role in Myxococcus xanthus predation on Sinorhizobium meliloti. Front. Microbiol. 11 (94). doi: 10.3389/fmicb.2020.00094
Cook S. D. (2019). An historical review of phenylacetic acid. Plant Cell Physiol. 60 (2), 243–254. doi: 10.1093/pcp/pcz004
Crook M. B., Lindsay D. P., Biggs M. B., Bentley J. S., Price J. C., Clement S. C., et al. (2012). Rhizobial plasmids that cause impaired symbiotic nitrogen fixation and enhanced host invasion. Mol. Plant Microbe Interact. 25 (8), 1026–1033. doi: 10.1094/MPMI-02-12-0052-R
Cubo M. T., Buendia-Claveria A. M., Beringer J. E., Ruiz-Sainz J. E. (1988). Melanin production by Rhizobium strains. Appl. Environ. Microbiol. 54 (7), 1812–1817. doi: 10.1128/aem.54.7.1812-1817.1988
Davis J. J., Olsen G. J. (2009). Modal codon usage: assessing the typical codon usage of a genome. Mol. Biol. Evol. 27 (4), 800–810. doi: 10.1093/molbev/msp281
Delcher A. L., Salzberg S. L., Phillippy A. M. (2003). Using MUMmer to identify similar regions in large sequence sets. Curr. Protoc. Bioinf. 1), 10.13. doi: 10.1002/0471250953.bi1003s00
Del Papa M. F., Balagué L. J., Sowinski S. C., Wegener C., Segundo E., Abarca F. M., et al. (1999). Isolation and characterization of alfalfa-nodulating rhizobia present in acidic soils of central Argentina and Uruguay. Appl. Environ. Microbiol. 65 (4), 1420–1427. doi: 10.1128/AEM.65.4.1420-1427.1999
Del Papa M. F., Pistorio M., Balague L., Draghi W., Wegener C., Perticari A., et al. (2003). A microcosm study on the influence of pH and the host-plant on the soil persistence of two alfalfa-nodulating rhizobia with different saprophytic and symbiotic characteristics. Biol. fertility soils 39 (2), 112–116. doi: 10.1007/s00374-003-0690-6
Draghi W. O., Del Papa M. F., Hellweg C., Watt S. A., Watt T. F., Barsch A., et al. (2016). A consolidated analysis of the physiologic and molecular responses induced under acid stress in the legume-symbiont model-soil bacterium Sinorhizobium meliloti. Sci. Rep. 6, 29278. doi: 10.1038/srep29278
Eckhardt T. (1978). A rapid method for the identification of plasmid deoxyribonucleic acid in bacteria. Plasmid 1, 584–588. doi: 10.1016/0147-619X(78)90016-1
Felsenstein J. (1985). Confidence limits on phylogenies: An approach using the bootstrap. Evolution 39, 783–791. doi: 10.2307/2408678
Galardini M., Mengoni A., Brilli M., Pini F., Fioravanti A., Lucas S., et al. (2011). Exploring the symbiotic pangenome of the nitrogen-fixing bacterium Sinorhizobium meliloti. BMC Genomics 12 (1), 235. doi: 10.1186/1471-2164-12-235
Galibert F., Finan T. M., Long S. R., Pühler A., Abola P., Ampe F., et al. (2001). The composite genome of the legume symbiont. Sinorhizobium meliloti Sci. 293 (5530), 668–672. doi: 10.1126/science.1060966
Ghosh P., Adolphsen K. N., Yurgel S. N., Kahn M. L. (2021). Sinorhizobium medicae WSM419 genes that improve symbiosis between Sinorhizobium meliloti Rm1021 and Medicago truncatula Jemalong A17 and in other symbiotic systems. Appl. Environ. Microbiol. 87 (15), e03004–e03020. doi: 10.1128/AEM.03004-20
Gilchrist C. L. M., Chooi Y. H. (2021). Clinker & clustermap.js: Automatic generation of gene cluster comparison figures. Bioinformatics 37 (16), 2473–2475. doi: 10.1093/bioinformatics/btab007
Glenn A., Dilworth M. (1994). The life of root nodule bacteria in the acidic underground. FEMS Microbiol. Lett. 123 (1-2), 1–9. doi: 10.1111/j.1574-6968.1994.tb07193.x
Glenn A. R., Reeve W. G., Tiwari R. P., Dilworth M. J. (1999). Acid tolerance in root nodule bacteria. Novartis Found Symp 221, 112–126. doi: 10.1002/9780470515631.ch8
Graham P. H., Draeger K. J., Ferrey M. L., Conroy M. J., Hammer B. E., Martinez E., et al. (1994). Acid pH tolerance in strains of Rhizobium and Bradyrhizobium, and initial studies on the basis for acid tolerance of Rhizobium tropici UMR1899. Can. J. Microbiol. 40 (3), 198–207. doi: 10.1139/m94-033
Greening C., Ahmed F. H., Mohamed A. E., Lee B. M., Pandey G., Warden A. C., et al. (2016). Physiology, biochemistry, and applications of F420 - and Fo-dependent redox reactions. Microbiol. Mol. Biol. Rev. 80, 451–493. doi: 10.1128/MMBR.00070-15
Hong L., Simon J. D. (2007). Current understanding of the binding sites, capacity, affinity, and biological significance of metals in melanin. The Journal of Physical Chemistry B, 111 (28), 7938–7947.
Horchani F., Prévot M., Boscari A., Evangelisti E., Meilhoc E., Bruand C., et al. (2011). Both plant and bacterial nitrate reductases contribute to nitric oxide production in Medicago truncatula nitrogen-fixing nodules. Plant Physiol. 155 (2), 1023–1036. doi: 10.1104/pp.110.166140
Howieson J., Ewing M. (1986). Acid tolerance in the Rhizobium meliloti–Medicago symbiosis. Aust. J. Agric. Res. 37 (1), 55–64. doi: 10.1071/AR9860055
Howieson J., Ewing M., D'antuono M. (1988). Selection for acid tolerance in Rhizobium meliloti. Plant Soil 105 (2), 179–188. doi: 10.1007/BF02376781
Howieson J., Robson A., Abbott L. (1992). Acid-tolerant species of Medicago produce root exudates at low pH which induce the expression of nodulation genes in Rhizobium meliloti. Funct. Plant Biol. 19 (3), 287–296. doi: 10.1071/PP9920287
Kachlany S. C., Planet P. J., Bhattacharjee M. K., Kollia E., DeSalle R., Fine D. H., et al. (2000). Nonspecific adherence by Actinobacillus actinomycetemcomitans requires genes widespread in Bacteria and Archaea. J. bacteriology 182 (21), 6169–6176. doi: 10.1128/JB.182.21.6169-6176.2000
Kumar S., Stecher G., Li M., Knyaz C., Tamura K. (2018). MEGA X: molecular evolutionary genetics analysis across computing platforms. Mol. Biol. Evol. 35 (6), 1547–1549. doi: 10.1093/molbev/msy096
Law R. J., Hamlin J. N. R., Sivro A., McCorrister S. J., Cardama G. A., Cardona S. T. (2008). A functional phenylacetic acid catabolic pathway is required for full pathogenicity of Burkholderia cenocepacia in the Caenorhabditis elegans host model. J. Bacteriology 190 (21), 7209–7218. doi: 10.1128/JB.00481-08
Lehman A. P., Long S. R., Silhavy T. J. (2018). OxyR-dependent transcription response of Sinorhizobium meliloti to oxidative stress. J. Bacteriology 200 (7), e00622–e00617. doi: 10.1128/JB.00622-17
López J. L., Alvarez F., Príncipe A., Salas M. E., Lozano M. J., Draghi W. O., et al. (2018). Isolation, taxonomic analysis, and phenotypic characterization of bacterial endophytes present in alfalfa (Medicago sativa) seeds. J. Biotechnol. 267, 55–62. doi: 10.1016/j.jbiotec.2017.12.020
López J. L., Lozano M. J., Lagares A. Jr., Fabre M. L., Draghi W. O., Del Papa M. F., et al. (2019). Codon usage heterogeneity in the multipartite prokaryote genome: selection-based coding bias associated with gene location, expression level, and ancestry. MBio 10 (3), e00505–e00519. doi: 10.1128/mbio.00505-19
Martini M. C., Albicoro F. J., Nour E., Schlüter A., van Elsas J. D., Springael D., et al. (2015). Characterization of a collection of plasmid-containing bacteria isolated from an on-farm biopurification system used for pesticide removal. Plasmid 80, 16–23. doi: 10.1016/j.plasmid.2015.05.001
Mazur A., Koper P. (2012). Rhizobial plasmids — replication, structure and biological role. Open Life Sci. 7 (4), 571–586. doi: 10.2478/s11535-012-0058-8
Meade H. M., Long S. R., Ruvkun G. B., Brown S. E., Ausubel F. M. (1982). Physical and genetic characterization of symbiotic and auxotrophic mutants of Rhizobium meliloti induced by transposon Tn5 mutagenesis. J. bacteriology 149 (1), 114–122. doi: 10.1128/jb.149.1.114-122.1982
Meier-Kolthoff J. P., Göker M. (2019). TYGS is an automated high-throughput platform for state-of-the-art genome-based taxonomy. Nat. Commun. 10 (1), 1–10. doi: 10.1038/s41467-019-10210-3
Mercado-Blanco J., García F., Fernández-López M., Olivares J. (1993). Melanin production by Rhizobium meliloti GR4 is linked to nonsymbiotic plasmid pRmeGR4b: cloning, sequencing, and expression of the tyrosinase gene mepA. J. Bacteriol. 175 (17), 5403–5410. doi: 10.1128/jb.175.17.5403-5410.1993
Meredith P., Sarna T. (2006). The physical and chemical properties of eumelanin. Pigment Cell Res. 19 (6), 572–594. doi: 10.1111/j.1600-0749.2006.00345.x
Munns D. (1970). Nodulation of Medicago sativa in solution culture. Plant Soil 32 (1), 90–102. doi: 10.1007/BF01372849
Nautiyal C. S. (1999). An efficient microbiological growth medium for screening phosphate solubilizing microorganisms. FEMS Microbiol. Lett. 170 (1), 265–270. doi: 10.1111/j.1574-6968.1999.tb13383.x
Nelson M. S., Chun C. L., Sadowsky M. J. (2017). Type IV effector proteins involved in the medicago-sinorhizobium symbiosis. Mol. Plant Microbe Interact. 30 (1), 28–34. doi: 10.1094/MPMI-10-16-0211-R
Nelson M., Guhlin J., Epstein B., Tiffin P., Sadowsky M. J. (2018). The complete replicons of 16 Ensifer meliloti strains offer insights into intra- and inter-replicon gene transfer, transposon-associated loci, and repeat elements. Microbial Genomics 4 (5), e000174. doi: 10.1099/mgen.0.000174
Ney B., Ahmed F. H., Carere C. R., Biswas A., Warden A. C., Morales S. E., et al. (2017). The methanogenic redox cofactor F420 is widely synthesized by aerobic soil bacteria. ISME J. 11, 125–137. doi: 10.1038/ismej.2016.100
Noel K. D., Sanchez A., Fernandez L., Leemans J., Cevallos M. A. (1984). Rhizobium phaseoli symbiotic mutants with transposon Tn5 insertions. J. Bacteriology 158 (1), 148–155. doi: 10.1128/jb.158.1.148-155.1984
O'hara G. W., Goss T. J., Dilworth M. J., Glenn A. R. (1989). Maintenance of intracellular pH and acid tolerance in Rhizobium meliloti. Appl. Environ. Microbiol. 55 (8), 1870–1876. doi: 10.1128/aem.55.8.1870-1876.1989
Pacheco P. J., Bedmar E. J., Mesa S., Tortosa G., Delgado M. J. (2023). Ensifer meliloti denitrification is involved in infection effectiveness and N2O emissions from alfalfa root nodules. Plant Soil 486, 519–534. doi: 10.1007/s11104-023-05946-3
Page A. J., Cummins C. A., Hunt M., Wong V. K., Reuter S., Holden M. T., et al. (2015). Roary: rapid large-scale prokaryote pan genome analysis. Bioinformatics 31 (22), 3691–3693. doi: 10.1093/bioinformatics/btv421
Paterson J., Jahanshah G., Li Y., Wang Q., Mehnaz S., Gross H. (2017). The contribution of genome mining strategies to the understanding of active principles of PGPR strains. FEMS Microbiol. Ecol. 93 (3), fiw249. doi: 10.1093/femsec/fiw249
Pavan M. E., López N. I., Pettinari M. J. (2020). Melanin biosynthesis in bacteria, regulation and production perspectives. Appl. Microbiol. Biotechnol. 104 (4), 1357–1370. doi: 10.1007/s00253-019-10245-y
Pérez-Mendoza D., Sepúlveda E., Pando V., Muñoz S., Nogales J., Olivares J., et al. (2005). Identification of the rctA gene, which is required for repression of conjugative transfer of rhizobial symbiotic megaplasmids. J. Bacteriology 187 (21), 7341–7350. doi: 10.1128/JB.187.21.7341-7350.2005
Pobigaylo N., Szymczak S., Nattkemper T. W., Becker A. (2008). Identification of genes relevant to symbiosis and competitiveness in Sinorhizobium meliloti using signature-tagged mutants. Mol. Plant Microbe Interact. 2), 219–231. doi: 10.1094/MPMI-21-2-0219
Rao J. R., Cooper J. E. (1994). Rhizobia catabolize nod gene-inducing flavonoids via C-ring fission mechanisms. J. Bacteriology 176 (17), 5409–5413. doi: 10.1128/jb.176.17.5409-5413.1994
Reeve W., Tiwari R., Dilworth M., Glenn A. (1993). Calcium affects the growth and survival of Rhizobium meliloti. Soil Biol. Biochem. 25 (5), 581–586. doi: 10.1016/0038-0717(93)90197-J
Richardson A., Simpson R. (1989). Acid-tolerance and symbiotic effectiveness of Rhizobium trifolii associated with a Trifolium subterraneum L.-based pasture growing in an acid soil. Soil Biol. Biochem. 21 (1), 87–96. doi: 10.1016/0038-0717(89)90016-3
Ripoll F., Pasek S., Schenowitz C., Dossat C., Barbe V., Rottman M., et al. (2009). Non Mycobacterial virulence genes in the genome of the emerging pathogen Mycobacterium abscessus. PloS One 4 (6), e5660. doi: 10.1371/journal.pone.0005660
Rodriguez-R L. M., Gunturu S., Harvey W. T., Rosselló-Mora R., Tiedje J. M., Cole J. R., et al. (2018). The Microbial Genomes Atlas (MiGA) webserver: taxonomic and gene diversity analysis of Archaea and Bacteria at the whole genome level. Nucleic Acids Res. 46 (W1), W282–W288. doi: 10.1093/nar/gky467
Ruiz B., Sauviac L., Brouquisse R., Bruand C., Meilhoc E. (2022). Role of nitric oxide of bacterial origin in the Medicago truncatula-Sinorhizobium meliloti symbiosis. Mol. Plant Microbe Interact. 35 (10), 887–892. doi: 10.1094/MPMI-05-22-0118-SC
Saitou N., Nei M. (1987). The neighbor-joining method: A new method for reconstructing phylogenetic trees. Mol. Biol. Evol. 4, 406–425. doi: 10.1093/oxfordjournals.molbev.a040454
Sauviac L., Philippe H., Phok K., Bruand C. (2007). An extracytoplasmic function Sigma Factor acts as a general stress response regulator in Sinorhizobium meliloti. J. Bacteriology 189 (11), 4204–4216. doi: 10.1128/JB.00175-07
Segundo E., Martinez-Abarca F., Dillewijn P. V., Fernández-López M., Lagares A., Martinez-Drets G., et al. (1999). Characterisation of symbiotically efficient alfalfa-nodulating rhizobia isolated from acid soils of Argentina and Uruguay. FEMS Microbiol. Ecol. 28 (2), 169–176. doi: 10.1111/j.1574-6941.1999.tb00572.x
Seshadri R., Reeve W. G., Ardley J. K., Tennessen K., Woyke T., Kyrpides N. C., et al. (2015). Discovery of novel plant interaction determinants from the genomes of 163 root nodule bacteria. Sci. Rep. 5, 16825. doi: 10.1038/srep16825
Singh S., Nimse S. B., Mathew D. E., Dhimmar A., Sahastrabudhe H., Gajjar A., et al. (2021). Microbial melanin: Recent advances in biosynthesis, extraction, characterization, and applications. Biotechnol. Adv. 53, 107773. doi: 10.1016/j.biotechadv.2021.107773
Skerker J. M., Shapiro L. (2000). Identification and cell cycle control of a novel pilus system in Caulobacter crescentus. EMBO J. 19 (13), 3223–3234. doi: 10.1093/emboj/19.13.3223
Solomon S. (2007). IPCC 2007. Climate Change 2007: The Physical Science Basis. Intergovernmental Panel on Climate Change (Cambridge University Press).
Stiens M., Schneiker S., Keller M., Kuhn S., Pühler A., Schlüter A. (2006). Sequence analysis of the 144-kilobase accessory plasmid pSmeSM11a, isolated from a dominant Sinorhizobium meliloti strain Identified during a long-term field release experiment. Appl. Environ. Microbiol. 72 (5), 3662–3672. doi: 10.1128/aem.72.5.3662-3672.2006
Tamura K., Nei M. (1993). Estimation of the number of nucleotide substitutions in the control region of mitochondrial DNA in humans and chimpanzees. Mol. Biol. Evol. 10, 512–526. doi: 10.1093/oxfordjournals.molbev.a040023
Tatusova T., DiCuccio M., Badretdin A., Chetvernin V., Ciufo S., Li W. (2013). “Prokaryotic genome annotation pipeline,” in The NCBI Handbook, 2nd edition (National Center for Biotechnology Information (US).
Thornton F. C., Davey C. (1984). Saprophytic competence of acid tolerant strains of Rhizobium trifolii in acid soil. Plant Soil 80 (3), 337–344. doi: 10.1007/BF02140040
Tiwari R. P., Reeve W. G., Dilworth M. J., Glenn A. R. (1996). Acid tolerance in Rhizobium meliloti strain WSM419 involves a two-component sensor-regulator system. Microbiology 142 (Pt 7), 1693–1704. doi: 10.1099/13500872-142-7-1693
Torres M. J., Avila S., Bedmar E. J., Delgado M. J. (2018). Overexpression of the periplasmic nitrate reductase supports anaerobic growth by Ensifer meliloti. FEMS Microbiol. Lett. 365 (7). doi: 10.1093/femsle/fny041
Torres M. J., Rubia M. I., de la Peña T. C., Pueyo J. J., Bedmar E. J., Delgado M. J. (2014). Genetic basis for denitrification in Ensifer meliloti. Bmc Microbiology 14 (1).
van Dillewijn P., Soto M. J., Villadas P. J., Toro N. (2001). Construction and environmental release of a Sinorhizobium meliloti strain genetically modified to be more competitive for alfalfa nodulation. Appl. Environ. Microbiol. 67, 3860–3865. doi: 10.1128/AEM.67.9.3860-3865.2001
Velazquez E., Mateos P. F., Pedrero P., Dazzo F. B., Martinez-Molina E. (1995). Attenuation of symbiotic effectiveness by rhizobium meliloti SAF22 related to the presence of a cryptic plasmid. Appl. Environ. Microbiol. 61 (5), 2033–2036. doi: 10.1128/aem.61.5.2033-2036.1995
Zatakia H. M., Nelson C. E., Syed U. J., Scharf B. E. (2014). ExpR coordinates the expression of symbiotically important, bundle-forming Flp pili with quorum sensing in Sinorhizobium meliloti. Appl. Environ. Microbiol. 80 (8), 2429–2439. doi: 10.1128/AEM.04088-13
Zerbino D. R. (2010). Using the Velvet de novo Assembler for Short-Read Sequencing Technologies. Curr. Protoc. Bioinf. 31 (1), 11.15.11–11.15.12. doi: 10.1002/0471250953.bi1105s31
Keywords: rhizobia, Sinorhizobium meliloti, accessory genome, denitrification pathway, PGPR-related genes, symbiosis
Citation: Cafiero JH, Salvetti Casasco M, Lozano MJ, Vacca C, López García SL, Draghi WO, Lagares A and Del Papa MF (2023) Genomic analysis of Sinorhizobium meliloti LPU63, an acid-tolerant and symbiotically efficient alfalfa-nodulating rhizobia. Front. Agron. 5:1175524. doi: 10.3389/fagro.2023.1175524
Received: 27 February 2023; Accepted: 07 July 2023;
Published: 27 July 2023.
Edited by:
Ashwani Kumar, University of Allahabad, IndiaReviewed by:
Olga Onishchuk, All-Russian Research Institute of Agricultural Microbiology of the Russian Academy of Agricultural Sciences, RussiaManda Yu, The Forsyth Institute, United States
Gayathri Ilangumaran, McGill University, Canada
Copyright © 2023 Cafiero, Salvetti Casasco, Lozano, Vacca, López García, Draghi, Lagares and Del Papa. This is an open-access article distributed under the terms of the Creative Commons Attribution License (CC BY). The use, distribution or reproduction in other forums is permitted, provided the original author(s) and the copyright owner(s) are credited and that the original publication in this journal is cited, in accordance with accepted academic practice. No use, distribution or reproduction is permitted which does not comply with these terms.
*Correspondence: María Florencia Del Papa, ZmxvcHB5QGJpb2wudW5scC5lZHUuYXI=