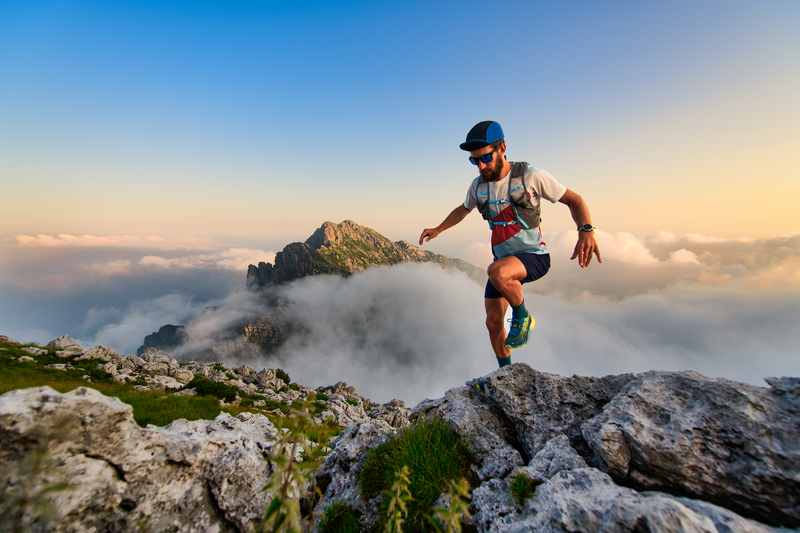
95% of researchers rate our articles as excellent or good
Learn more about the work of our research integrity team to safeguard the quality of each article we publish.
Find out more
REVIEW article
Front. Agron. , 09 June 2023
Sec. Pest Management
Volume 5 - 2023 | https://doi.org/10.3389/fagro.2023.1064041
The continuous growth of the world population has imposed major challenges on agriculture. Consequently, farmers generalized the overuse of synthetic fertilizers and pesticides to meet the global food demand. Although these products have helped many developing countries increase their crop yield, they have simultaneously resulted in many issues, mainly the decline of soil fertility and degradation of local ecosystems due to soil, water, and air contamination, combined with their non-renewable nature and increased costs. For agriculture to become more sustainable, the use of alternative biological products, with recognized beneficial effects on plant yield and health, must be expanded. In this context, microalgae and cyanobacteria are rich sources of nutrients and bioactive metabolites, which have been gaining attention from researchers and companies for their ability to improve plant nutrition, growth, and tolerance to stress. This review gives an overview of the research work that has been done in the last two decades, regarding the use of microalgae and cyanobacteria (blue-green algae) as biofertilizers, biostimulants, and biopesticides. This work identified trends and challenges and highlights the use of microalgae to recycle the nutrients from wastewater to improve plant productivity while reducing the fertilizer and water footprint for more sustainable agriculture practices.
Agriculture is facing significant pressure to meet the growing food demands of the increasing world population. Until the 20th century, nitrogen and phosphorus were the limiting factors for agricultural yield. Despite the abundance of atmospheric nitrogen, most crop plants cannot uptake it in this form and therefore need the application of fertilizers. Conventional fertilizers are obtained primarily from declining nonrenewable resources. Currently, more than 96% of ammonia is produced through the Haber-Bosch process, which requires fossil fuels as feedstock (natural gas, oil, and coal) (Smith et al., 2020). This process is one of the largest consumers of global energy, expending more than 1-2% of the annual world energy supply (Chatterjee et al., 2017). Furthermore, this process is also a large greenhouse gas emitter, responsible for 1.2% of global anthropogenic CO2 emissions (Smith et al., 2020).
Regarding phosphorus, all the produced fertilizers are derived from limited mineral deposits which are restricted to specific areas of the planet. Approximately 75% of the current reserves of phosphate minerals are in Morocco. China and the US also have significant reserves, but these are not sold on the global market. This problem of scarcity and the key role of phosphorus in agriculture makes it highly susceptible to unpredictable price fluctuations on the global market, which is also strongly influenced by geopolitical interests. Countries that do not have their reserves, including the European Union are more at risk (Zilio et al., 2022). These concerns are more intensified with the recent war triggered by the Russian invasion of Ukraine.
The productivity of crops has been negatively affected by the appearance of pests and diseases, and increase of water scarcity, due to the soil erosion and degradation of local ecosystems by the intensive use of synthetic fertilizers (Chatterjee et al., 2017). Despite the detrimental environmental impacts, the use of fertilizers is inevitable to meet the growing global food demand. As most environmental legislations worldwide become more rigorous, the emergence of organic farming practices appears as a promising solution to fulfill the growing demand for healthy foods that do not compromise long-term environmental sustainability, promote low pesticide inputs, and the use of natural bioproducts, such as biofertilizers, biostimulants, and biopesticides (Calvo et al., 2014; Bulgari et al., 2015; Colla and Rouphael, 2015; Chatterjee et al., 2017; Win et al., 2018). Still, when it comes to bioproducts, misinterpretations between these concepts can still occur, especially between biofertilizers and biostimulants (Figure 1). According to the new EU fertilizing products regulation (FPR) 2019/1009 that lays down rules on the making available on the market EU fertilising products, and was implemented on 16th of July of 2022, a biostimulant in “an EU fertilizing product whose function is to stimulate plant nutrition processes independently of the product’s nutrient content with the sole aim of improving one or more of the following characteristics of the plant or the plant rhizosphere: nutrient use efficiency, tolerance to abiotic stress, quality traits, or availability of confined nutrients in the soil or rhizosphere” (Regulation (EU) 2019/1009, 2019). Essentially, the main difference between both concepts is that biostimulants do not provide nutrients directly to crops as opposed to biofertilizers.
Figure 1 Summary of natural bioproducts (biofertilizer, biostimulant, and biocontrol) and their impact on the crop.
Biofertilizers are biological products that contain microorganisms, such as algae, fungi, or bacteria, or natural compounds derived from those microorganisms, which act at the soil level. These products promote plant growth and soil fertility by increasing the supply or availability of essential nutrients, including macro and micronutrients, and by improving physico-chemical and biological soil properties (Abdel-Raouf, 2012; Sahu et al., 2012; Ronga et al., 2019). On the other hand, biostimulants stimulate plant growth by modulating plant growth metabolisms, such as germination, respiration, photosynthetic activity, nutrient uptake, and flower and fruit production, amongst others (Górka et al., 2018). They also play a relevant role in extending plant tolerance and resistance to various environmental stress conditions (e.g., drought, heat, salinity, etc.) (Povero et al., 2016; Chiaiese et al., 2018). On the other hand, biopesticides promote antagonism and biological control of pathogenic organisms, such as bacteria, viruses, fungi, and insects (Carvajal-Muñoz and Carmona-Garcia, 2012).
One group of microorganisms that can act as bioproducts in Agriculture are microalgae. These organisms excrete organic acids, increase phosphorus availability and uptake, provide nitrogen fixation, and enhance the soil content of organic matter to build soil fertility (Górka et al., 2015; Coppens et al., 2016; Khan et al., 2019). Microalgae produce biologically active extracellular substances that influence plant growth, also known as Plant Growth Regulators (PGRs) which act at very low concentrations (i.e., phytohormones, amino acids, polypeptides, polysaccharides, and vitamins), whilst specific eukaryotic microalgae have also shown antibacterial and antifungal activity. (Chiaiese et al., 2018; Chanda et al., 2019). Several bioactive compounds are behind these antimicrobial mechanisms, such as fatty acids, and phenolic compounds (Renuka et al., 2018; Costa et al., 2019). Cyanobacteria have also been reported as stimulators of plant defense mechanisms and producers of metabolites with pesticidal action (Singh et al., 2016). Further research is required for green microalgae on the control of plant pathogens. Nevertheless, studies already reveal that green microalgae (e.g., Coccomyxa onubensis, Chlorella vulgaris, etc.) have antimicrobial properties, which makes them an interesting subject for research in the agricultural biotechnology field (Costa et al., 2019).
From the economic point of view, the growth of the European biostimulants sector has been driven by the increasing importance of organic and sustainable farming as well as the need for enhanced yields (Mordor Intelligence, 2020). Europe is currently the biggest market for biostimulants, with around 8.5 million acres of area treated in 2016 (Liebig et al., 2020). This has increased the need for harmonized European Regulation, concerning the placement of biostimulants in the market. Thus, the new Fertilizing Products Regulation (FPR) (EU) 2019/1009 includes biostimulants as CE-marked fertilizing products meaning that the producer can now officially evaluate and prove that these products meet EU safety, health, or environmental requirements, therefore receiving the official EU conformity certification to be sold in the markets belonging to the European Economic Area (CE stands for “Conformité Européenne,” which translates to “European Conformity.”) (Regulation (EU) 2019/1009, 2019). The Global Biostimulant Market was estimated to be valued at USD 2.6 billion, in 2019, and is expected to grow by 11.24% by 2025 (MarketsandMarkets, 2020). A recent event that has further aggravated the expectations and potential of the biostimulant market is the Russian invasion of Ukraine. The beginning of the war in March of 2022, has led to a shortage of fertilizers in the market. Both countries were reported to be responsible, altogether, for the exportation of 28% of fertilizers containing nitrogen, phosphorus, and potassium, according to Morgan Stanley (Domm, 2022). This has resulted in a significant increase in fertilizer prices, which have become worryingly scarce. For the farmers, these events translate to lower crop yields, and for the consumers, increased market prices of the final products. Although the duration of the conflict cannot be foretold with complete certainty, the negative effects on the global markets are expected to last for the coming years, with or without the prolongation of the war. Furthermore, a reshape of the global order is already at hand, with several world leaders working on decreasing the dependency on Russia’s supplies, such as fertilizers. This conflict has thus begun a new cycle in the world’s economy and the search for alternative suppliers is at its peak. In the case of fertilizers, the current crisis is further aggravated by the climate crisis as the chemicals associated with fertilizers, as well as the production of fertilizers, are highly pollutant. Hence, microalgae bioproducts such as biostimulants and biofertilizers may very well pose an opportunity to alleviate and overcome the current fertilizer crisis.
This manuscript gives an overview of the trends of microalgae, eukaryotic, and cyanobacteria, for agricultural applications, through a literature review of the most relevant studies in this field. The authors pretend to emphasize the relevance of microalgae biomass as an emerging application in agriculture, especially after the use of microalgae for the treatment of wastewater from various sources.
The data was collected from the Web of Science database. The search was done using several combinations of keywords: (*microalga* OR *cyanobacteri*) AND (*biostimulant* OR *biofertilizer* OR *biopesticide* OR “germination” OR “plant growth” OR “bioprotection” OR “agriculture”). The search generated 1873 articles from the Science Citation Index (SCI) starting in 2001 until September 2022. From those articles, both reviews and viewpoint articles were removed, and only English language journals were considered (1458 articles). From these articles, we searched within the references for more articles that were not in our initial dataset. Among these, a selection was made to consider only articles which studied the effect of microalgae and cyanobacteria on target plants, soil, or pests. The final dataset included 330 original research papers for a 21-year period (2001 – 2022), which were synthesized in the sections below. Furthermore, an online page displaying the information within this manuscript was prepared at the following link: https://shorturl.at/klxD0.
In the last 5 years, the research on the agricultural application of microalgae has registered a steep growth, duplicating the number of studies in 2021 compared to 2018 (Figure 2A). This demonstrates the emergence of microalgae in the field of agriculture, which might develop to be one of the most relevant applications in microalgae technology in the following years (Figure 2B).
Figure 2 (A) Evolution of publications on the application of microalgae in agriculture worldwide; (B) Evolution of publications on the various applications of microalgae worldwide; and (C) Number of publications on the application of microalgae in agriculture by country, over 21 years (2001-2022).
Asia has led the research in this field, being the source of almost half the studies published between 2001 and 2022, with India standing out among them (87 publications). In the last decade, India has emerged as a major agricultural exporter, ranking first in the world with the highest net cropped area (USGS, 2021). However, the Indian agricultural sector has faced various challenges such as low farm yields, limited water availability, and environmental and soil degradation, amongst others (Chew and Soccio, 2016; Narain, 2020). These could be the major incentives behind extensive research on sustainable ways to overcome these challenges. In Europe, Spain has been the leading country in publications (18 publications), closely followed by Italy (17 publications). In Africa and America, Egypt and Brazil stood out, respectively, with 33 and 26 published studies each (Figure 2C). Spain and Italy have been valuable players in the European agriculture sector, however, Germany and France, represent 51% of the total European Union (EU) utilized agricultural area and 49% of the total EU arable land. Moreover, Italy cut down 20% of the use of pesticides between 2011 and 2018 by substituting them for more sustainable alternatives such as practices like crop rotation and technologies like precision farming making it the most sustainable agriculture sector in Europe (Eurostat, 2020).
There has been a predominance of studies addressing cyanobacteria species for agricultural purposes over microalgae (185 vs. 108 studies) (Figure 3A). 35 studies used both microalgae and cyanobacteria species, where 6 took advantage of mixed consortia. Among the microalgal species studied (Figure 3B, there was a clear predominance of the genus Chlorella (108), being that almost half of the studies used Chlorella vulgaris, followed by Scenedesmus (48) (including Tetrademus and Desmodesmus due to changes in taxonomy). Other microalgal genera have been studied, although to a much lesser extent, such as Dunaliella, Nannochloropsis, Porphyridium, and Tetraselmis, among others. Regarding cyanobacteria, Anabaena and Nostoc were dominant genera, especially in studies dealing with soil-borne cyanobacteria in rice paddy fields in India. In fact, throughout the years, many studies regarding the microbial communities of soils, included both genera as the most found species, due to their ability to fix atmospheric nitrogen. In addition, their significant effect on plant growth can be attributed to the ability of Anabaena and Nostoc strains to produce growth regulators (e.g. phytohormones like indole-acetic acid) and other secondary metabolites, linked to the biocontrol of diseases, as well as improving soil aggregation through secretion of mucilage and polysaccharides (Prasanna et al., 2011). Arthrospira has also been the dominant cyanobacterial genus in most recent studies (58), especially Arthrospira platensis (46). Moreover, in the literature, the higher abundance of cyanobacteria compared to microalgae was related to their identification within various consortia collected from soils, not to their isolated effect. The fact that Chlorella vulgaris and Arthrospira platensis were the most studied microalga and cyanobacteria species, respectively, was expected given their public acceptance and dominance in the global production market.
Figure 3 Distribution in agriculture application studies by (A) type of microorganism; (B) number and genus of the microalgae and cyanobacteria used in the studies; (C) cultivation medium used to grow the biomass.
Regarding the cultivation medium, most studies used synthetic media to produce the microalgal/cyanobacterial biomass (285), while only 40 publications used biomass that was produced in wastewater (Figure 3C).In terms of composition, these wastewaters are typically richer in nutrients such as nitrogen, phosphate, and potassium, as well as contain significant contents of solids, dissolved and particular matter, and microorganisms, when compared with synthetic media. It is also interesting to notice that most biomass produced from wastewater (WW) were microalgae, either in isolates or in consortium with other microalgal or cyanobacterial species. From the literature, only 3 studies used cyanobacterial isolates or consortia (Wuang et al., 2016; Rashad et al., 2019; Ferreira et al., 2021), while the remaining used consortia with both microalgae and cyanobacteria, dominated by the former (Mulbry et al., 2005; Mukherjee et al., 2016; Renuka et al., 2016; Castro et al., 2017; Ahn et al., 2020; Supraja et al., 2020). From these microalgae, the most common species that have been found in wastewater treatment plants are Chlorella and Scenedesmus/Tetradesmus, two highly robust and resilient strains. This has resulted in the generalized use of these species in studies involving wastewaters, either in the form of isolates or due to their domination of the cultures. (Ferreira et al., 2019; Navarro-López et al., 2020a; Ferreira et al., 2021; Ranglová et al., 2021; Viegas et al., 2021a; Viegas et al., 2021b; Viegas et al., 2021c). The potential of wastewater-grown microalgae for agriculture was further addressed in section 4.1.
Microalgae can be used either as pure extracts or as crude algal compost to enhance seed germination, and crop productivity at different levels, by improving soil quality, stimulating growth, and/or expanding protection against stress conditions. Figure 4A shows that most studies used microalgae/cyanobacteria as a biostimulant (162) rather than a biofertilizer (145). There seems to be some incorrect denomination of the effect due to the misconception between both concepts, as previously mentioned, being that some studies did not distinguish between them and considered only a biofertilizer effect. However, for of Figure 4A, a distinction between them was considered depending on their described effect in the respective studies. In comparison, the biocontrol effect has been less studied, with only 26 studies addressing it. Furthermore, being an emerging topic, most studies have been developed at the laboratory scale (154), especially in plant germination and early development stages. Nonetheless, there are a considerable number of studies performed in pots, both in a greenhouse (104) or outdoors (20), and in field trials (70) (Figure 4B).
Figure 4 Distribution of studies by (A) the agricultural purpose of the biomass; (B) the experimental scale of the trials; (C) the application mode of the biomass and (D) the growth conditions of the plant trials (stress or no stress).
The most common mode of application was via root (207) (Figure 4C). All studies that applied seed soaking (92) were performed at the laboratory scale. This can be explained by the fact that this method is mainly used for germination experiments, which are usually the fastest ones to perform when the goal is to first study the biostimulant/biofertilizer potential of a certain compound and microorganism. From the literature, only 2 studies were found regarding the biostimulant (Bayona-Morcillo et al., 2020) and biopesticide (Kim et al., 2020) effects of microalgae when applied via foliar and root in greenhouse trials. When comparing foliar spray to soil application, the former provides faster nutrient utilization and correction of nutrient deficiencies, with improvements of N, P and K contents (Dineshkumar et al., 2020a). The number of studies evaluating the potential utilization of microalgae and microalgae-derived compounds, such as polysaccharides and phytohormones, for foliar application was limited (45). This strategy is relatively new and is one of the most innovative agricultural practices, as it is environmentally safe and promotes agricultural sustainability (Ronga et al., 2019). Microalgae-derived extracts, even at low concentrations, can induce an array of physiological plant responses. Since the amount of natural substances in algae is relatively smaller than in mineral fertilizers, the foliar application could be a more suitable method, since plant responses to nutrients supplied via foliar sprays are normally more rapid than when applied in soil (Ronga et al., 2019).
Regarding growth conditions (Figure 4D), most studies were developed under controlled environments with optimal growth conditions (262). Still, 40 studies have already tested the potential of microalgae/cyanobacteria for improving plant growth under abiotic stress conditions, especially salinity (20), chemical contamination (9), drought (5), and heat (4). The studies considering biotic stress were related to the biopesticide effect (26).
Concerning the type of crop used in these studies, cereal plants were the most common, especially rice (Oryza sativa) and wheat (Triticum aestivum) given their rapid growths and thus possibility to obtain faster results (Figure 5). Garden cress (Vigna radiata) was also significantly studied for germination trials due to fast germination and development of only the primary root. Microalgal/cyanobacterial derived products are a relatively unexplored resource (when compared with seaweeds), therefore there is a great opportunity in this field to develop novel research, more field trials in crop productivity, in natural environmental conditions as well as in stress abiotic conditions (e.g., salinity, temperature, and drought).
Figure 5 Distribution of studies regarding the application of microalgae in agriculture, by the target plants.
Some microalgal and cyanobacterial species are also well-known for their positive effect on soil quality, given their ability to fix atmospheric nitrogen, increase the availability of essential nutrients or improve soil physical and chemical properties. Furthermore, as photosynthetic microorganisms, microalgae and cyanobacteria can recover nutrients from different sources, therefore reducing the use of traditional fertilizers.
As previously mentioned, most research on the agricultural applications of algae has focused on the use of cyanobacteria, given their ability to fix atmospheric nitrogen to organic nitrogen forms, that can be assimilated by higher plants (Nilsson et al., 2002; Pereira et al., 2009; Prasanna et al., 2014; Zayadan et al., 2014; Buenaventura and Barrientos, 2019; Shamim et al., 2020; Bao et al., 2021; Zhang et al., 2021) (Table 1). Altogether, the use of cyanobacteria in soils has been reported to promote nutrient recycling with increased availability of nitrogen, and to promote efficient C-N sequestration in the soil alongside the enrichment of the crop’s micronutrients fraction (Swarnalakshmi et al., 2013; Prasanna et al., 2015b; Prasanna et al., 2015c). When applied directly to the soil, cyanobacteria (alone or in consortia) can improve nitrogen availability and form soil crusts that avoid nitrogen leaching. This significantly improves plant growth whilst economizing fertilizers by 25-30% and minimizing the risks of water contamination (Pereira et al., 2009; Buenaventura and Barrientos, 2019; Ramírez-López et al., 2019; Bao et al., 2021; Zhang et al., 2021).
Table 1 Impacts of microalgae and cyanobacteria on soil quality regarding their nitrogen fixation ability.
One mode of application that has been considered an economically attractive option, was the use of cyanobacteria as a matrix to develop biofilms in the soil (Prasanna et al., 2011; Prasanna et al., 2020). Prasanna et al (2013a; 2014; 2015a; 2015b; 2015c). and Swarnalakshmi et al. (2013) have extensively used the cyanobacterium Anabaena torulosa to develop biofilms, due to its inherent characteristics, such as the presence of an extensive nutrient-rich mucilage and its facultative mode of nutrition, which can improve the supply of N, P and C to the plant. Moreover, the cyanobacterial biofilms can further enhance plant growth through the production of phytohormones (i.e. auxins, gibberellins, cytokinins), bioactive metabolites (i.e. aurilide, barbamide, garbamide), and increased content of glomalin-related soil protein in the soil, as observed for several crops (Prasanna et al., 2013a; Prasanna et al., 2013b; Prasanna et al., 2014; Prasanna et al., 2015b).
Another cyanobacteria that was widely used as a nitrogen-fixing biofertilizer was Nostoc (Table 1). Nilsson et al. (2002) screened various Nostoc isolates for their ability to associate with rice, which promoted higher N2 fixation rates compared to free-living cyanobacteria. In another study, N. muscorum and N. rivulare, either alone or in combination with N-fertilizer, were also shown to associate with soybean, resulting in a significant increase in plant height, leaf area, and fresh weight (Sholkamy et al., 2015). Ultimately, Buenaventura and Barrientos (2019) demonstrated that the supplementation with N. commune allowed for the reduction of the recommended dose of inorganic fertilizer by half since it provided a similar nitrogen content to the inorganic fertilizer, where similar plant height and harvest index were achieved.
The use of microalgae as nitrogen-fixing biofertilizers has also been studied. Different methods of application of microalgal monocultures of Microcystis aeruginosa and Chlorella sp. were studied on maize (Grzesik and Romanowska-Duda, 2014) and willow plants (Grzesik et al., 2017). The authors concluded that applying the microalgae to grains prior to sowing, was more profitable than continuously applying them to roots via the substrate. In addition, Grzesik et al. (2017) studied the foliar application of the referred monocultures, from which the physiological performances and growths of plants were significantly enhanced. In both studies, the increased height, number of shoots, and length of plants, could be related to the accumulation of higher quantities of active compounds from the microalgae strains. Moreover, the applied monocultures improved the NPK content of plants, the enzyme activity (dehydrogenases, RNase, acid or alkaline phosphatase and nitrate reductase), as well as the overall photosynthesis process (stability of cytomembranes, chlorophyll content, transpiration, stomatal conductance and reduced intercellular CO2 concentration) (Grzesik and Romanowska-Duda, 2014; Grzesik et al., 2017).
Finally, the use of consortia with both cyanobacteria and microalgae has also been explored. Ramírez-López et al. (2019) showed that it was possible to reduce 75% the chemical fertilization for wheat (Triticum aestivum L.), due to the complementary effect of a photosynthetic and N-fixing microbial consortium, including cyanobacteria and Chlorophyta species, that had beneficial effects on plant growth and the soil. The same was evidenced by Dineshkumar et al. (2018), where reducing the chemical N fertilizer up to 50 or 75% of the recommended dose while complementing with the application of microalgae (C. vulgaris and Arthrospira platensis), showed better results than merely using the recommended N dose. In conclusion, the use of microalgae and cyanobacteria as biofertilizers has shown promising results in promoting nutrient recycling, improving nitrogen availability, and enhancing plant growth while reducing the need for inorganic fertilizers, therefore holding great potential for sustainable agriculture.
In the past three decades there have been secondary micronutrient deficiencies in the soils, due to the imbalanced use of macronutrient fertilizers, decreased use of organic manure, reduced recycling of crop residues, and bumper harvests. Microalgae and cyanobacteria are photosynthetic microorganisms that can uptake and store essential nutrients (N and P) in their biomass, even from sites where they are scarce. In addition, they can accumulate other important micronutrients like potassium, magnesium, sulfur, and iron, which are involved in redox reactions in the plant metabolism (Ronga et al., 2019; Gonçalves, 2021). Thus, as it has been reported in the literature (Table 2), microalgae or cyanobacteria can be considered as an alternative source of essential macro- and micronutrients for plants’ growth.
In addition to their nitrogen-fixation capacity, cyanobacteria-bacteria biofilms or consortia can improve nutrient availability in the soils (Rana et al., 2012; Manjunath et al., 2016; Simranjit et al., 2019). Simranjit et al. (2019) showed that the application of these biofilms improved the composition of the soil, not only in N (50-90%) but also in P and organic C by 40-60%. Moreover, these biofilms improved the bioavailability of micronutrients such as Cu, Fe, Mn, and Zn, which reflected a positive effect on the leaves photosynthetic pigments. Manjunath et al. (2016) also observed improvements in the bioavailability of soil macro/micronutrients, by applying Anabaena in microbial biofilms or consortia, and Calothrix sp. The synergistic action of bacteria and cyanobacteria increased Fe and Zn contents, contributing to an improved root yield and weight of okra plants. These two micronutrients are amongst the most limiting nutrients for plant growth. However, since several cyanobacteria can sequester Fe or Zn from metallothioneins, they can be used for biofortification to cover these crop deficiencies (Rana et al., 2012; Manjunath et al., 2016).
Regarding the application of microalgal species, Chlorella vulgaris has been a widely studied species on different plants (Shaaban, 2001b; Faheed and Fattah, 2008; Dineshkumar et al., 2020c). In a study involving the use of both cyanobacteria and microalgae, Dineshkumar et al (2019; 2020b). tested C. vulgaris and A. platensis mixed with cow manure as a biofertilizer for maize and onion. For maize, all the treatments recorded significantly improved the levels of N, Mn, P, and K. In contrast, low levels were observed for Ca, Zn, Fe, and CO,3 and Na contents. The combination of manure with microalgae allowed for the best growth of maize during the early stages, specifically up to 51.1% after 60 days of planting (Dineshkumar et al., 2019). The same trend was observed when applying identical conditions to onion plants. The microalgal treatments mixed with cow manure allowed for the maximum micro and macronutrient availability, which yielded the best growth parameters in onion plants. All treatments originated onions larger than the marketable size, with improved biochemical (e.g., soluble sugars, phenols, free amino acids, and indoles) and mineral compositions (e.g., Na, K, P, Ca, Fe, Mg, and Mn), that result in increased market value. The same group also tested the foliar application of both species in mung bean plants (Dineshkumar et al., 2020a). Likewise, plants treated with C. vulgaris and A. platensis generated superior levels on the composition of mung bean leaves and soil and improved the physical characteristics of green gram including water absorption index, water solubility index, and water and oil absorption. Recently, Dineshkumar et al. (2020c) concluded that C. vulgaris extracts could be used as an eco-friendly and potentially economically viable foliar spray treatment for black gram (Vigna mungo L.). The soil properties and production yields were improved, as well as fortified the seed flour with beneficial minerals for consumers, such as Na, K, Ca, Mg, and P.
Nutrient depletion is a major problem for crop production, given the insufficiency of direct sunlight and continuous cultivation with intensive use of fertilizers and pesticides. As previously stated, microalgae can recover nutrients from sites where nutrient availability is limited. Schreiber et al. (2018) compared the application of mineral and Chlorella vulgaris fertilizers in nutrient-poor soil substrates. For all experimental conditions, the biofertilization enhanced the growth of wheat plants in comparison to the unfertilized controls (nutrient limitation). No significant differences were observed between mineral and Chlorella fertilizers. When compared with wheat plants grown without nutrient limitation, the fertilization with Chlorella vulgaris facilitated the growth of wheat with similar nitrogen contents, but lower phosphorus levels in plant tissues. This suggests that microalgae cells can cover the nutrient deficiency of the soils, though, while N can be released in a form that wheat roots can easily uptake, P might not, or is released slowly. Nonetheless, the root-hair properties of plants were similar among treatments, which confirms the conversion of microalgal P to plant-available forms in the soil. These results validate that microalgal biomass can support crop growth on marginal soils, with similar performance to mineral fertilizers.
The use of other microalgal species has also been evaluated, as is the case of Acutodesmus dimorphus, whose culture, extracts, and dry biomass were tested in seed priming, foliar spraying, and biofertilization of tomato plants (Garcia-Gonzalez and Sommerfeld, 2016). The authors were able to achieve early germination with the seed treatment, as well as longer plants, a higher number of flowers, and braces per plant, with foliar application of the extract. Furthermore, the application of dry A. dimorphus biomass 22 days prior to transplant, significantly enhanced plant growth, when compared to the application at the time of transplant. These results suggest that a preliminary application is required for the biomass to break down and increase the nutrient availability for plant uptake. In another case, Saadaoui et al. (2019) studied the effects of fertilizing date palms with Tetraselmis sp. The authors observed that after 3 months of treatment, the supplementation with 0.5 g of Tetraselmis sp. increased the NPK content of soil and maintained a safe level of heavy metals. This led to higher plant growth rates of date palm when compared to conventional fertilizer, with 100% survival rates, greater number of leaves (3.17 ± 0.14 vs. 3 ± 0.66), high ability to root largest stem thickness, longer shoot, and higher chlorophyll content.
Microalgae and cyanobacteria can also enhance soil properties (aggregation, porosity, permeability, ventilation, and humidity) (Sharma et al., 2012; Baweja et al., 2019; Pan et al., 2019). As previously stated, some microalgae and cyanobacteria species can excrete polysaccharides that form an adhesive and gelatinous mesh, which binds soil particles on their surface, therefore improving water retention and soil aeration (Xu et al., 2013). This ability plays a significant role in pH and temperature regulation, as well as protection against erosion (Sharma et al., 2012; Baweja et al., 2019). These improvements positively affect crop productivity since they stimulate root growth and soil microbial activity. Therefore, microalgae and cyanobacteria can help build soil fertility (Nisha et al., 2007; Saadatnia and Riahi, 2009; Prasanna et al., 2016a; Marks et al., 2017; Barone et al., 2019b; Lv et al., 2020), prevent erosion (Hu et al., 2002; Issa et al., 2007; Kheirfam et al., 2017), recover damaged soil crusts (Acea et al., 2001), or sequester toxic pollutants from soil (Tripathi et al., 2008; Priya et al., 2014; Decesaro et al., 2016) (Table 3). Furthermore, microalgae and cyanobacteria can form associations with plant roots which are mutually beneficial. They benefit plants by producing extracellular enzymes that break down organic matter in the soil, and releasing nutrients that are essential for plant growth, such as nitrogen and phosphorus. They can also produce growth-promoting hormones that stimulate plant growth (Prasanna et al., 2011). In turn, plant roots release exudates (e.g., simple sugars, amino acids, organic acids, and other compounds) that provide a food source for the microorganisms. These microorganisms can then form colonies around the plant roots, creating a biofilm that can help protect the roots from pathogens and other stressors, while also improving the soil structure. The improved soil structure allows for better water and nutrient retention, which further supports plant growth (Nisha et al., 2018).
In a study, a multi-strain biofertilizer (dried paste) consisting of three indigenous cyanobacterial isolates (Anabaena doliolum, Cylindrospermum sphaerica, and Nostoc calcicole), was applied to an organically poor semi-arid soil under limited-water conditions (Nisha et al., 2007). The native strains improved carbon and nitrogen mineralization, by promoting soil microbial activities and decreasing the C:N ratio. Also, a decline in bulk density and an increase in water holding capacity and hydraulic conductivity of soil were observed. Plant growth and yield of pearl millet and wheat increased in response to cyanobacterial biofertilizer, which was more pronounced at lower water levels (6%). In another study, microalgal suspensions of alive S. quadricauda and Anabaena circinalis were evaluated in cucumber cultivation. The application of a high concentration of S. quadricauda, in the soil, boosted the diversity of rhizosphere fungi of cucumber, especially of growth-promoting bacteria and fungi (Azotobacter, Bacillus, Pseudomonas, Cryptococcus, Fusarium, Penicillium, and Trichoderma), which resulted in increased height, number of leaves, flower buds, and stem diameter of cucumber (Lv et al., 2020).
Enriching the biological properties of soil crusts, by increasing its microbial population, can be an effective strategy to improve soil chemical properties. Moreover, because of their filamentous structure and their ability to secrete polysaccharides and other cementing substances (amongst others), cyanobacteria can generate a prosperous environment for microbial populations to proliferate and form a crust, which has a significant role in preventing soil erosion, improving water kinetics and plant growth) (Nisha et al., 2018).
Accordingly, Acea et al. (2001) investigated the potential value of cyanobacterial suspensions (Nostoc, Oscillatoria and Scytonema species) for accelerating soil recolonization and promoting microbiotic crust formation after a fire. The heated soils inoculated with cyanobacteria were quickly colonized and, after 2 months, the cyanobacterial filaments, and associated fungal hyphae, built up a matrix that aggregated surface soil particles, therefore improving crust formation. These crusts were not observed in the uninoculated soils. In another study, Kheirfam et al. (2017) showed that the inoculation of cyanobacteria suspensions (Nostoc, Oscillatoria, and Lyngbya species) had a more effective role in N fixing (240%) and organic matter storing (40%), compared to the other inoculation treatments, which ultimately improves soil quality to prevent soil degradation.
Finally, microalgae and cyanobacteria can perform the remediation of soils by removing heavy metals (e.g., cadmium, lead, and chromium), trace elements (e.g., iron, zinc, copper, and manganese), hydrocarbons, and others, which helps build soil quality and fertility (Priya et al., 2015; Suresh Kumar et al., 2015). Heavy metals can be transformed, detoxified, and volatilized by microalgae, and since microalgae are non-pathogenic there is no risk of accidental release into the atmosphere (Priya et al., 2015). Microalgae degrade the pollutants enzymatically but can also absorb them onto their surfaces due to the high metal binding capacity of polysaccharides, proteins, or lipids present on their cell walls (Suresh Kumar et al., 2015). This capacity is crucial in rice production, since an increase in land contamination has been reported, which not only reduces arable land but could negatively impact human health due to heavy metal accumulation in rice grains (Tripathi et al., 2008; Srivastava et al., 2018). The inoculation of cyanobacteria slightly declined Cd, Ni and As in the content of rice plants, while reducing the nitrogen requirements by 30 kg/ha. Aside from enhancing growth, it also fortified the composition of essential micronutrients while maintaining the level of toxic metals under safe limits (Tripathi et al., 2008). A combination of C. vulgaris and Pseudomonas putida mitigated the As stress during P-enriched conditions by reducing As availability, as well and modulating its uptake and detoxification mechanisms in rice plants (Srivastava et al., 2018).
The use of microalgae and cyanobacteria (and/or their extracts) can directly stimulate plants’ growth and development by improving germination rates and plant characteristics, such as shoot and root length, leaf area, and nutrient contents. These enhancements are accomplished due to microalgal/cyanobacterial metabolites (e.g., phytohormones, amino acids, vitamins, polysaccharides, polyamines, etc.), which can trigger several metabolic responses, such as respiration, photosynthesis, nucleic acid synthesis, chlorophyll production and ions uptake (Chiaiese et al., 2018; Chanda et al., 2019). Table 4 demonstrates examples of microalgae and cyanobacteria (biomass and/or extracts) that have been studied for their direct stimulation of higher plants growth.
The potential of C. sorokiniana to be used as substitute for chemical fertilizers, and its capacity to stimulate wheat plants was studied by Kholssi et al. (2019). Apart from the harvested biomass, resuspended in fresh and spent medium, the authors also analyzed the filtered medium where the microalga was cultivated and compared it with fresh medium alone (control). All treatments resulted in a higher germination percentage than the control, however, only the treatments containing spent medium (with or without microalga) were statistically different from the control. A general enhancement of plant growth was observed with the microalga treatments, although the most significant increase in plant length (30%) and fresh weight (77%) was obtained with the filtrate of C. sorokiniana (spent medium), which suggests that the microalga excreted beneficial compounds for plant growth into the medium. From a biorefinery perspective, these results are extremely interesting, since the supernatant has the potential to be used for the biostimulation of plants, whilst the harvested biomass can be used for other high-value applications like food and/or feed (Ferreira et al., 2022).
The biostimulant potential of Chlorella vulgaris (auto and heterotrophic) was addressed by Uysal et al. (2015), where an increase in height was observed for wheat and barley plants when compared with the control. Barone et al. (2019a) reported a positive effect on the biomass productivity of tomato plants and microalgae in a co-cultivation system, especially using Scenedesmus quadricauda biomass in the presence of digestates from the waste of an agro-livestock farm. The highest weight values of fresh plant shoot and dry whole plant were observed in the co-cultivation system with only C. vulgaris, where a 2.5-fold increase was obtained over the control. Barone et al. (2018) also found that C. vulgaris and S. quadricauda extracts had biostimulant effects on the expression of root traits and genes related to the nutrient acquisition in sugar beet (genes putatively involved in sulfate starvation). In a similar study, Puglisi et al. (2020) tested S. quadricauda extracts, demonstrating a beneficial impact on the growth of lettuce seedlings, especially at the shoot level, along with increased contents of dry matter, chlorophylls, carotenoids, and protein. Moreover, these extracts were also proven to positively influence the activities of various enzymes involved in the carbon and nitrogen primary metabolisms.
Plaza et al. (2018) evaluated the effects of foliar application of Scenedesmus sp. and A. platensis hydrolysates in petunia plants. The application of Scenedesmus improved the plant nutrient status, and accelerated plant development and the flowering process, while Arthrospira improved the root dry matter, the number of flowers, and the water content.
The application of various dosages of Arthrospira in radish plants was also explored by Godlewska et al. (2019). An increase in length and fresh weight was observed for homogenate treatments of seeds and of filtrate as a foliar spray. The highest increase in chlorophyll was observed for lower concentrations. It is also relevant to acknowledge that the lowest dosages of Arthrospira always achieved better results compared with the commercial biostimulant. Akgül (2019) demonstrated that up to 75% microalgal cell extract promoted an increase in germination and seedling of wheat and barley plants and that higher concentrations had an inhibitory effect. Michalak et al. (2016) performed field trials to evaluate the effect of supercritical extracts of A. platensis in winter wheat plants. The best results were achieved by A. platensis extracts (higher number of grains in ear and shank length). Moreover, these extracts showed similar biostimulant properties to commercial products, with the additional advantage of being a natural and environmentally friendly source of biologically active compounds.
In conclusion, the use of microalgae and cyanobacteria as biostimulants for higher plants has shown promising results in enhancing growth and development, with their metabolites triggering several metabolic responses. These findings suggest a potential for these organisms to replace chemical fertilizers and serve as a sustainable and environmentally friendly source of biologically active compounds.
Under non-stress conditions, plants use the most energy in processes necessary for maintenance, and vegetative, and generative growth. However, when exposed to extreme environmental conditions (e.g., cold, heat, drought, salinity), plant resources are allocated towards stress mitigation, which decreases plant growth and final yield (Table 5).
Table 5 Impacts of microalgae and cyanobacteria on the enhancement of tolerance to abiotic stress (heat, temperature, drought).
Extremely low or high temperatures negatively affect the metabolic activity of plants, and damage cell membranes, therefore affecting the nutritional composition of plants and the rates of photosynthesis and transpiration. To improve the plant’s response to these harmful environmental conditions, a very promising option can be the use of microalgal biostimulants, as they are a rich source of antioxidants and other bioactive compounds that can modulate plant response mechanisms to cope with adverse conditions. The use of algal-based products as biostimulants has already been successfully applied in crops under temperature stress conditions (Figure 4D; Table 5).
Heat-stressed Arabidopsis thaliana treated with Nostoc muscorum exhibited significantly lower root hair death when compared to untreated seedlings (Chua et al., 2020). Moreover, they identified proline as a compound of interest responsible for stress response. Kopta et al. (2018) showed that the application of a bacterial-algal biostimulant enhanced the fresh weight of two lettuce varieties in the spring and summer seasons. The summer crop of romaine lettuce treated with the biostimulant presented enhanced antioxidant capacity and carotenoid contents. Nonetheless, it is essential to highlight the vital role that the biostimulant application had in mitigating the temperature stress, which positively impacted the harvesting yield during the summer season.
An Arthrospira platensis hydrolysate was tested for its capacity to mitigate the negative effect of salt on Petunia x hybrida (Plaza et al., 2018; Bayona-Morcillo et al., 2020) and Pelargonium hortorum (Tejada-Ruiz et al., 2020). Both studies observed an increase in plant growth and flower development, under high salinity. Dunaliella salina extracts were also shown to alleviate the salt stress in tomatoes (El Arroussi et al., 2018) and pepper plants (Guzmán-Murillo et al., 2013). According to GC-MS metabolomics analysis, the exopolysaccharides of D. salina triggered the activation or inhibition of the metabolic pathways involved in the plant’s response to stress, such as jasmonic acid-dependent pathways. Root growth of pepper plants was favorably influenced by the microalgae treatments (D. salina and Phaeodactylum tricornutum), which increased the content of antioxidant enzymes and reduced the oxidative stress imposed by the increasing salt concentrations (Guzmán-Murillo et al., 2013). Cyanobacteria, particularly Nostoc, were also shown to mitigate the saline stress by decreasing the soil’s electrical conductivity (El-Sheekh et al., 2018). Other studies showed that the integrative application of cyanobacteria with other microbes or antioxidant substances could also significantly improve growth characteristics under saline conditions (Zaki et al., 2019; El Semary et al., 2020).
Drought stress has a negative impact on several cultures. Tomatoes are particularly very sensitive to this type of stress, which strongly affects photosynthesis and, consequently, plant growth and yield. To mitigate drought stress, Barsanti et al. (2019) explored the effects of β-(1,3)-glucan (paramylon) from the microalga Euglena gracilis. Paramylon-treated plants showed no signs of wilting compared to untreated plants, and while the density and length of the root system were drastically reduced, there was an increase in lateral rootlets. The water stress imposed on tomato plants negatively affected the main ecophysiological parameters of leaves (water potential, CO2 assimilation, internal concentration, and stomatal conductance). However, the paramylon treatment allowed the tomato plants to recover to the values of control plants (non-stressed), after 1-2 weeks. The fruit size of paramylon-treated plants was like the ones under the optimal water regime, although the former reached the first ripening stage two weeks earlier than the latter. Moreover, the content of antioxidant compounds (carotenoids, phenolic acid, and vitamins), and soluble carbohydrates (glucose, fructose, and sucrose) of fruits from treated plants doubled concerning untreated plants.
Drought stress was also addressed by Kusvuran and Kusvuran (2019) in guar plants. There was a significant increase in antioxidant activity (total phenolic and flavonoid contents, superoxide dismutase, catalase, ascorbate peroxidase, and glutathione reductase), and reduction of oxidative stress (malondialdehyde) in guar plants treated with Chlorella vulgaris. Furthermore, the application of A. platensis in grape berries allowed the stomata to be open under water-stress conditions, without negatively impacting the water potential of vines, and promoted an increase in berry weight in both optimal and stress conditions. In addition, improved berry composition (sugar content) was also observed in treated vines under drought conditions (Salvi et al., 2020).
Pathogen organisms, such as insects, nematodes, bacteria, and fungi, strongly affect agricultural productivity. Some plants have their defense and resistance mechanisms, which include the regulation of signaling pathways, gene expression, and induction/inhibition of specific metabolic pathways, to produce secondary metabolites with antioxidant and antimicrobial activities. However, the application of external protection agents is crucial to achieve the ambitious target productivity, to fulfill the growing food demands. Considering the wide variety of bioactive compounds that can be found in microalgae and cyanobacteria, the use of these microorganisms (or their extracts) can promote adequate crops protection against these biotic factors. Although the biocontrol effect was not so widely studied as the biofertilizer or biostimulant effect (Figure 4A), Table 6 provides published examples of the application of microalgae and cyanobacteria (biomass and/or extracts), on the biological control and protection of crops.
Table 6 Impacts of microalgae and cyanobacteria on the enhancement of tolerance to biotic stress (biopesticide effect).
The fungicidal effect of microalgae against plant pathogens has been most widely evidenced than the antimicrobial effect. Only 3 recent studies from the last 2 years addressed the in vitro antibacterial effect of microalgae, or cyanobacteria, against plant pathogens (Al dayel et al., 2020; Bao et al., 2021; Ranglová et al., 2021). The potential of Chlorella vulgaris extracts as antibacterial was studied by Al dayel et al. (2020) and Ranglová et al. (2021), while Bao et al. (2021) evaluated the same effect from cyanobacterium Anabaena variabilis. Al dayel et al. (2020) obtained inhibition zones for Escherichia coli, Streptococcus sp., Bacillus sp., and Staphylococcus aureus, while Ranglová et al. (2021) showed that C. vulgaris grown in the synthetic medium were only active against Clavibacter michiganensis. A. variabilis extracts resulted in inhibition rates of 75.3 and 83.6% against Xanthomonas oryzae and Rhizoctonia solani, respectively, in in vitro trials (Bao et al., 2021). Moreover, in vivo trials were done by Bao et al. (2021) in rice fields infected with Rhizoctonia solani. The seedlings treated with A. variabilis were significantly longer than the control, achieving a disease control efficacy of 62.3%.
Kim (2006) evaluated different cyanobacteria isolates and Prasanna et al. (2008) evaluated several Anabaena isolates for biocidal activity against a set of phytopathogenic fungi. Nine cyanobacteria showed promising results. Nostoc commune and Oscillatoria tenuis showed strong antifungal activity against Phytophthora capsica (Kim, 2006). Among the Anabaena isolates, more than half showed inhibition zones of varying diameter against one or more fungi. The strains were characterized in terms of hydrolytic enzymes, proteins, and IAA (Indole-3-acetic acid), revealing a positive correlation between the presence of these metabolites and the fungicidal activity (Prasanna et al., 2008). Abdel-Hafez et al. (2015) also tested several cyanobacteria species and obtained a lower growth of Alternaria porri, using Nostoc muscorum and Oscillatoria sp. (20.4 and 36.3%, respectively). The culture filtrates of both species also contained high concentrations of phenolics compounds and alkaloids. Furthermore, their application in greenhouse conditions allowed for a reduction of 55.1-66.5% in the severity of purple blotch disease (Abdel-Hafez et al., 2015).
Ecological management of manure on farms is vital to minimize losses of valuable plant nutrients and to prevent nutrient contamination of the surrounding watershed and soils. During the storage and land application of manure effluents, large amounts of N are lost to the atmosphere due to the volatilization of ammonia or nitrate runoff by rain to water streams and deep waters. An alternative to the land spreading of manure is to grow microalgae, where the pollutant from the effluents turns into nutrients from the microalgae point of view, converting them into biomass (Khan et al., 2019). Using microalgae for wastewater treatment (WWT) can provide a dual role, where bioremediation of wastewater occurs with the simultaneous production of valuable biomass, rich in essential nutrients and other bioactive compounds, which are beneficial for optimal seed germination and plant growth (amongst other applications). When microalgae are used for wastewater treatment, they are produced using open ponds due to their capacity to process large volumes of wastewater at lower construction and operation costs, and easier scale-up. Acién et al. (2017) estimated the overall costs of microalgae-based WWT to be 30% lower than conventional activated sludge treatment, without even taking into account the revenues from potential commercial agricultural products.
Given the microalgal potential for WWT, there has been an increased interest in the use and research of these organisms, especially regarding the treatment of WW originating from livestock (i.e., piggeries, poultries, aquaculture), humans (i.e., urban), industrial ones (i.e. breweries, wineries) and others such as foods and surface waters. Furthermore, with the scope of transforming WWTs into more circular processes, the biomass that is obtained is also being studied for application in agriculture (Table 7). The WW source will influence the biomass composition and, thus, the quality of the final product. The percentage of N and P recovered by the biomass will depend largely on the initial concentration in the WW. Wastewaters generally have a low content of P, and its limitation has also been demonstrated to lead to an increased content of antioxidants (e.g., carotenoids, ascorbic acid, and tocopherols) (Gauthier et al., 2020). The presence of heavy metals, which can be accumulated by microalgae, also needs to be considered for the safety of agricultural microalgae-derived products (Ronga et al., 2019). The exposure of microalgae to heavy metals can trigger the synthesis of valuable compounds such as ascorbate peroxidase, catalase, superoxide dismutase, or ascorbate (Gauthier et al., 2020).
Table 7 Summary of results for microalgae grown in wastewater and used for agricultural applications.
The effect of unicellular and filamentous microalgal-cyanobacterial consortia, grown in urban wastewater, on the soil composition and grain quality of a wheat crop was evaluated (Renuka et al, 2016). Besides an increase in micronutrient availability in the soil (mainly zinc, iron, copper, and manganese), the studied consortia also enhanced their organic carbon content. This improvement in soil quality resulted in increased grain yield and higher product quality, as the obtained grains presented an improved nutritional composition. Silambarasan et al. (2021) studied the urban (at 75%) wastewater treatment capacity of a microalgal consortium composed of Chlorella sp. and Scenedesmus sp. The following use of deoiled microalgal biomass, combined with inorganic fertilizer, improved the tomato plant yield by 174%, as well as nutrient contents regarding nitrate (61%), phosphate (179%), potassium (71%), calcium (38%), magnesium (26%) and iron (11%). In another study, González et al. (2020) evaluated the formulation of microalgal biofertilizers from Scenedesmus sp. biomass obtained after the treatment of secondary urban wastewater. The application of solid-state formulations (2% microalgae) resulted in a DW increase of 220% and 180% for ryegrass and barley plants, respectively. Additionally, the authors observed an inhibitor effect on plant growth, for formulations that contained more than 2% microalgae. In another study, Ranglová et al. (2021) compared the biostimulant potential of two C. vulgaris cultures, grown in different media, inorganic BG11 medium, and centrate from municipal WW, by evaluating the germination index of cress seeds, the auxin-like activity in mung bean and cytokinin-like activity in wheat growth tests. The biostimulant activity related to seed germination was found in the biomass cultivated in synthetic medium, but not for the one grown in WW, which could be related to the presence of inhibitory substances. The highest activity was obtained for C. vulgaris biomass harvested in the morning, at the lowest extract concentration. Both auxin- cytokinin-like activities were found in 2.0 and 3.0 g DW/L of C. vulgaris biomass harvested in the afternoon, which was equivalent to 0.5 mg DW/L of indole-3-butyric acid (auxin) and 0.3 mg DW/L of kinetin (cytokinin).
The research on the agricultural use of microalgal biomass applied in the treatment of animal-farming wastewater has also been gaining momentum. For instance, Ferreira et al. (2021) were able to improve the GI and root length of various plant seeds (tomato, watercress, cucumber, soybean, wheat, and barley seeds), after applying the biomass of a microalgal consortium composed of Tetradesmus obliquus, Chlorella protothecoides, Chlorella vulgaris, and the cyanobacterium Synechocystis sp., at 0.5g/L. In addition, Ferreira et al. (2022) studied the effect of Tetradesmus obliquus grown in 5% piggery effluent on wheat seed germination. The authors obtained three main conclusions: the use of biomass homogenized by high pressure (HPH) at 100-bar, or the use of non-treated biomass resulted in similar improvements of GI, up to 40-45% and 40%, respectively; and the use of filtrate from the initial culture also promoted shoot development up to 21%. These results demonstrate that all fractions of the microalgae culture have the potential to be utilized, including the supernatant as an enriched (in nutrients and bioactive compounds) irrigation solution and the microalga biomass in other high-value applications, as previously acknowledged by Kholssi et al. (2019). On another hand, Coppens et al. (2016) evaluated the application of microalgal-bacterial flocs (Ulothrix sp. and Klebsormidium sp.) in the treatment of brown crab’s aquaculture wastewater, as well as the application of Nannochloropsis oculata on soil composition and fruit development in tomato plants. The microalgae biomass increased the availability of NPK in the soil, resulting in a plant growth comparable to the organic fertilizer treatment. Although a lower tomato yield was achieved than the treatment using inorganic fertilizer, the fruit quality was highly superior in terms of sugar and carotenoid contents.
In another study, Viegas et al. (2021a) analyzed the biostimulant effect of Chlorella vulgaris and Tetradesmus obliquus, used in the treatment of raw wastewater from brown crab aquaculture, on the germination of wheat and watercress seeds. The promising results obtained represented a GI improvement of 175 and 48% in watercress, and 84 and 98% in wheat, treated with C. vulgaris and T. obliquus, respectively.
Likewise, the agricultural potential of microalgal biomass obtained from the treatments of other wastewaters is also being explored. For example, Zarezadeh et al. (2019) improved the N retention in a common pasture of ryegrass, after the application of dried biomass from Chlorella sp. and Scenedesmus sp. grown in anaerobically digested wastewater from food waste. The authors observed a slow release of nutrients which ultimately impacted the N cycling through the modulation of N mineralization and N pathways. In addition, de Souza et al. (2019) performed a life cycle analysis of the application on soil, of 1kg of N from microalgal biomass grown in food waste effluent. The authors suggested the alternative uses of photovoltaic energy and nitrogen-richer effluents, as well as the consideration of environmental compensation for the treatment of effluent, to improve the economic and environmental viability of the use of microalgal N biofertilizer. In another case, Ferreira et al. (2019) were able to increase the germination percentage of barley and wheat seeds, by 143 and 100%, respectively, with the application of Tetradesmus obliquus used in the treatment of anaerobically digested wastewater from a brewery. Finally, Ahn et al. (2020) evaluated the effects of the use of biomass of microalgae-cyanobacterium consortia, obtained in eutrophic freshwater from three wastewater treatment facilities, on Perilla sp. plants. Positive results were registered, with the improvement of plant signaling to abiotic stress, plant growth, and biomass, thus setting forth one more high potential application of microalgal biomass obtained with the treatment of wastewaters.
The practical applications of microalgae and cyanobacteria in agriculture are still very limited since the focus on these microorganisms has been on their functional activities in food and feed (as nutraceuticals and additives), pharmaceutical, and cosmetic products. However, their interest has been increasing given their potential role in enriching soil quality and plant nutrition, stimulating plant growth, and expanding plant tolerance to biotic and abiotic stress.
Although cyanobacteria, commonly known as blue-green algae, have already been recognized for their fertilizing role on the soil and rhizosphere, the research on the application of microalgae for biostimulants and biopesticides has drastically increased in the last two years. Most works have shown evidence of microalgae improving plant productivity and resistance to adverse conditions, thus supporting them as a viable biological-based alternative to chemical fertilizers, pesticides, and growth stimulants. Moreover, some studies highlight the added benefit of microalgae in enhancing the biometric and nutritional quality of leaves and fruits.
Despite the recognized benefits, the mode of action of microalgal compounds on plant response mechanisms is still not fully understood, given the high diversity of species and their products. Hence, metabolomics’ studies focusing on the effect of each metabolite/biomass on crops are essential to identify the most adequate for agricultural activities. Furthermore, only a small set of studies have been developed using microalgae grown in wastewaters, although with promising results. Nevertheless, until now no data have been reported on the specific composition of an algal culture medium that could help to understand its positive biofertilizing effect; therefore, the characterization of compounds with biofertilizing capability and enzymatic activity profiles in the extracellular medium of algal cultures also needs to be investigated.
The use of microalgae in sustainable agriculture is expected to be one of the most important applications of microalgae biomass in short term, especially for biomass cultivated in wastewater. This will not only bring benefits from an environmental perspective, concerning the recovery of nutrients and recycling of water in wastewater treatments and agriculture but will also improve the economic feasibility of microalgal production and downstream processing.
With increasing climate change, global warming, water scarcity, degradation of soils, and emerging crisis such as the war in Ukraine, innovations will be needed to enhance and protect crops throughout the world. The challenge to produce more food with limited resources makes microalgae a suitable alternative for enhancing and protecting agricultural production and delivering economic and environmental benefits to farmers and algae producers.
Conceptualization, AF. Writing—original draft preparation, AF. Writing—review and editing, AF, CB, CM-D-S, FA-F and LG. Visualization, AF, and CB. Data curation, CB. Supervision, CM-D-S, FA-F and LG. Project administration, LG. Funding acquisition, LG. All authors contributed to the article and approved the submitted version.
This work was supported by the project ALGAVALOR - Lisboa-01-0247-FEDER-035234, supported by Operational Programme for Competitiveness and Internationalization (COMPETE2020), by Lisbon Portugal Regional Operational Programme (Lisboa 2020) and by Algarve Regional Operational Programme (Algarve 2020) under the Portugal 2020 Partnership Agreement, through the European Regional Development Fund (ERDF); Biomass and Bioenergy Research Infrastructure (BBRI)- LISBOA-01-0145-FEDER-022059, supported by Operational Programme for Competitiveness and Internationalization (PORTUGAL2020), by Lisbon Portugal Regional Operational Programme (Lisboa 2020) and by North Portugal Regional Operational Programme (Norte 2020) under the Portugal 2020 Partnership Agreement, through the European Regional Development Fund (ERDF); project Performalgae, from the European Union’s Horizon 2020 research and innovation programme (grant agreement n° ALG-01-0247-FEDER-069961); Red CYTED P319RT0025 - RENUWAL - Red Iberoamericana para el Tratamiento de Efluentes con Microalgas; Bilateral Portugal-India DRI/India/0609/2020 Project WCAlgaeKIT+- Combination of Vertical Wetlands, Microalgae Photobioreactor, and Microbial Fuel Cell (KIT) for wastewater treatment in small pig production farms. AF and CB are pleased to acknowledge their PhD grants SFRH/BD/144122/2019 and FCT 2022.14298.BDANA, respectively, awarded by Fundação para a Ciência e Tecnologia.
The authors declare that the research was conducted in the absence of any commercial or financial relationships that could be construed as a potential conflict of interest.
All claims expressed in this article are solely those of the authors and do not necessarily represent those of their affiliated organizations, or those of the publisher, the editors and the reviewers. Any product that may be evaluated in this article, or claim that may be made by its manufacturer, is not guaranteed or endorsed by the publisher.
Abdel-Hafez S. I. I., Abo-Elyousr K. A. M., Abdel-Rahim I. R. (2015). Fungicidal activity of extracellular products of cyanobacteria against alternaria porri. Eur. J. Phycol. 50, 239–245. doi: 10.1080/09670262.2015.1028105
Abdel-Raouf N. (2012). Agricultural importance of algae. Afr. J. Biotechnol. 11, 11648–11658. doi: 10.5897/AJB11.3983
Acea M. J., Diz N., Prieto-Fernández A. (2001). Microbial populations in heated soils inoculated with cyanobacteria. Biol. Fertil. Soils. 33, 118–125. doi: 10.1007/s003740000298
Acién F. G., Molina E., Fernández-Sevilla J. M., Barbosa M., Gouveia L., Sepúlveda C., et al. (2017). “20 - Economics of microalgae production,” in Microalgae-Based Biofuels and Bioproducts: From Feedstock Cultivation to End-products. Eds. Gonzalez-Fernandez C., Muñoz R. (Duxford, UK: Woodhead Publishing), 485–503.
Ahn C. H., Lee S., Park J. R., Hwang T.-M., Joo J. C. (2020). Harvested microalgal biomass from different water treatment facilities–its characteristics and potential use as renewable sources of plant biostimulation. Agronomy 10, 1882. doi: 10.3390/agronomy10121882
Akgül F. (2019). Effect of Spirulina platensis (Gomont) geitler extract on seed germination of wheat and barley. Alınteri. Zirai. Bilim. Derg. 34, 148–153. doi: 10.28955/alinterizbd.639000
Al dayel M. F., El Semary N. A., Al Amer K., Al Ali K. M. (2020). Investigating the applications of Chlorella vulgaris in agriculture and nanosilver production. J. Environ. Biol. 41, 1099–1104. doi: 10.22438/jeb/41/5/MRN-1395
Atzori G., Nissim W. G., Rodolfi L., Niccolai A., Biondi N., Mancuso S., et al. (2020). Algae and bioguano as promising source of organic fertilizers. J. Appl. Phycol. 32, 3971–3981. doi: 10.1007/s10811-020-02261-7
Bao J., Zhuo C., Zhang D., Li Y., Hu F., Li H., et al. (2021). Potential applicability of a cyanobacterium as a biofertilizer and biopesticide in rice fields. Plant Soil 463, 1–16. doi: 10.1007/s11104-021-04899-9
Barone V., Baglieri A., Stevanato P., Broccanello C., Bertoldo G., Bertaggia M., et al. (2018). Root morphological and molecular responses induced by microalgae extracts in sugar beet (Beta vulgaris l.). J. Appl. Phycol. 30, 1061–1071. doi: 10.1007/s10811-017-1283-3
Barone V., Puglisi I., Fragalà F., Lo Piero A. R., Giuffrida F., Baglieri A. (2019a). Novel bioprocess for the cultivation of microalgae in hydroponic growing system of tomato plants. J. Appl. Phycol. 31, 465–470. doi: 10.1007/s10811-018-1518-y
Barone V., Puglisi I., Fragalà F., Stevanato P., Baglieri A. (2019b). Effect of living cells of microalgae or their extracts on soil enzyme activities. Arch. Agron. Soil Sci. 65, 712–726. doi: 10.1080/03650340.2018.1521513
Barsanti L., Coltelli P., Gualtieri P. (2019). Paramylon treatment improves quality profile and drought resistance in Solanum lycipersicum l. cv. micro-tom. Agronomy 9, 394. doi: 10.3390/agronomy9070394
Baweja P., Kumar S., Kumar G. (2019). “Organic fertilizer from algae: a novelapproach towards sustainable agriculture,”Biofertilizers for Sustainable Agriculture and Environment. Soil Biology. Eds. Giri B., Prasad R., Wu Q. S., Varma A. (Cham, Switzerland: Springer), 353–370.
Bayona-Morcillo P. J., Plaza B. M., Gómez-Serrano C., Rojas E., Jiménez-Becker S. (2020). Effect of the foliar application of cyanobacterial hydrolysate (Arthrospira platensis) on the growth of Petunia x hybrida under salinity conditions. J. Appl. Phycol. 32, 4003–4011. doi: 10.1007/s10811-020-02192-3
Boopathi T., Balamurugan V., Gopinath S., Sundararaman M. (2013). Characterization of IAA production by the mangrove cyanobacterium Phormidium sp. MI405019 and its influence on tobacco seed germination and organogenesis. J. Plant Growth Regul. 32, 758–766. doi: 10.1007/s00344-013-9342-8
Buenaventura M. K. P., Barrientos D. S. (2019). Response of Oryza sativa CL1 (Basmati 370) to Nostoc commune vauch. as fertilizer supplement. Mindanao. J. Sci. Technol. 17, 242–256.
Bulgari R., Cocetta G., Trivellini A., Vernieri P., Ferrante A. (2015). Biostimulants and crop responses: a review. Biol. Agric. Hortic. 31, 1–17. doi: 10.1080/01448765.2014.964649
Calvo P., Nelson L., Kloepper J. W. (2014). Agricultural uses of plant biostimulants. Plant Soil 383, 3–41. doi: 10.1007/s11104-014-2131-8
Carvajal-Muñoz J. S., Carmona-Garcia C. E. (2012). Benefits and limitations of biofertilization in agricultural practices. Livest. Res. Rural Dev. 24.
Castro J., de S., Calijuri M. L., Assemany P. P., Cecon P. R., de Assis I. R., et al. (2017). Microalgae biofilm in soil: greenhouse gas emissions, ammonia volatilization and plant growth. Sci. Total. Environ. 574, 1640–1648. doi: 10.1016/j.scitotenv.2016.08.205
Castro J., de S., Calijuri M. L., Mattiello E. M., Ribeiro V. J., Assemany P. P. (2020). Algal biomass from wastewater: soil phosphorus bioavailability and plants productivity. Sci. Total. Environ. 711, 135088. doi: 10.1016/j.scitotenv.2019.135088
Chanda M., Merghoub N., EL Arroussi H. (2019). Microalgae polysaccharides: the new sustainable bioactive products for the development of plant bio-stimulants? World J. Microbiol. Biotechnol. 35, 1–10. doi: 10.1007/s11274-019-2745-3
Chatterjee A., Singh S., Agrawal C., Yadav S., Rai R., Rai L. C. (2017). “Role of algae as a biofertilizer,” Algal Green Chemistry: Recent Progress in Biotechnology. Eds. Rastogi R. P., Pandey A., Madamwar D. (Amesterdam, Netherlands: Elsevier), 189–200.
Chaudhary V., Prasanna R., Nain L., Dubey S. C., Gupta V., Singh R., et al. (2012). Bioefficacy of novel cyanobacteria-amended formulations in suppressing damping off disease in tomato seedlings. World J. Microbiol. Biotechnol. 28, 3301–3310. doi: 10.1007/S11274-012-1141-Z
Chew P., Soccio M. (2016). Asia-Pacific: agricultural perspectives. Available at: https://economics.rabobank.com/publications/2016/february/asia-pacific-agricultural-perspectives/ (Accessed July 6, 2021).
Chiaiese P., Corrado G., Colla G., Kyriacou M. C., Rouphael Y. (2018). Renewable sources of plant biostimulation: microalgae as a sustainable means to improve crop performance. Front. Plant Sci. 871. doi: 10.3389/fpls.2018.01782
Chua A., Sherwood O. L., Fitzhenry L., Ng C. K. Y., McCabe P. F., Daly C. T. (2020). Cyanobacteria-derived proline increases stress tolerance in arabidopsis thaliana root hairs by suppressing programmed cell death. Front. Plant Sci. 11. doi: 10.3389/fpls.2020.490075
Colla G., Rouphael Y. (2015). Biostimulants in horticulture. Sci. Hortic. (Amsterdam). 196, 1–2. doi: 10.1016/j.scienta.2015.10.044
Coppens J., Grunert O., Van Den Hende S., Vanhoutte I., Boon N., Haesaert G., et al. (2016). The use of microalgae as a high-value organic slow-release fertilizer results in tomatoes with increased carotenoid and sugar levels. J. Appl. Phycol. 28, 2367–2377. doi: 10.1007/s10811-015-0775-2
Corbellini J. R., Ribas L. L. F., de Maia F. R., Corrêa D., de O., Noseda M. D., et al. (2020). Effect of microalgae Messastrum gracile and Chlorella vulgaris on the in vitro propagation of orchid Cattleya labiata. J. Appl. Phycol. 32, 4013–4027. doi: 10.1007/s10811-020-02251-9
Costa J. A. V., Freitas B. C. B., Cruz C. G., Silveira J., Morais M. G. (2019). Potential of microalgae as biopesticides to contribute to sustainable agriculture and environmental development. J. Environ. Sci. Heal. - Part B. Pestic. Food Contam. Agric. Wastes. 54, 366–375. doi: 10.1080/03601234.2019.1571366
Decesaro A., Rampel A., Machado T. S., Thomé A., Reddy K., Margarites A. C., et al. (2016). Bioremediation of soil contaminated with diesel and biodiesel fuel using biostimulation with microalgae biomass. J. Environ. Eng. 143, 04016091. doi: 10.1061/(ASCE)EE.1943-7870.0001165
Deepika P., MubarakAli D. (2020). Production and assessment of microalgal liquid fertilizer for the enhanced growth of four crop plants. Biocatal. Agric. Biotechnol. 28, 101701. doi: 10.1016/j.bcab.2020.101701
de Souza M. H. B., Calijuri M. L., Assemany P. P., Castro J., de S., de Oliveira A. C. M. (2019). Soil application of microalgae for nitrogen recovery: a life-cycle approach. J. Clean. Prod. 211, 342–349. doi: 10.1016/j.jclepro.2018.11.097
Dias G. A., Rocha R. H. C., Araújo J. L., De Lima J. F., Guedes W. A. (2016). Growth, yield, and postharvest quality in eggplant produced under different foliar fertilizer (Spirulina platensis) treatments. Semin. Agrar. 37, 3893–3902. doi: 10.5433/1679-0359.2016v37n6p3893
Dineshkumar R., Duraimurugan M., Sharmiladevi N., Lakshmi L. P., Rasheeq A. A., Arumugam A., et al. (2020a). Microalgal liquid biofertilizer and biostimulant effect on green gram (Vigna radiata l) an experimental cultivation. Biomass Convers. Biorefinery. 12, 3007–3027. doi: 10.1007/s13399-020-00857-0
Dineshkumar R., Kumaravel R., Gopalsamy J., Sikder M. N. A., Sampathkumar P. (2018). Microalgae as bio-fertilizers for rice growth and seed yield productivity. Waste. Biomass Valorization. 9, 793–800. doi: 10.1007/s12649-017-9873-5
Dineshkumar R., Subramanian J., Arumugam A., Ahamed Rasheeq A., Sampathkumar P. (2020b). Exploring the microalgae biofertilizer effect on onion cultivation by field experiment. Waste. Biomass Valorization. 11, 77–87. doi: 10.1007/s12649-018-0466-8
Dineshkumar R., Subramanian J., Gopalsamy J., Jayasingam P., Arumugam A., Kannadasan S., et al. (2019). The impact of using microalgae as biofertilizer in maize (Zea mays l.). Waste. Biomass Valorization. 10, 1101–1110. doi: 10.1007/s12649-017-0123-7
Dineshkumar R., Subramanian J., Sampathkumar P. (2020c). Prospective of chlorella vulgaris to augment growth and yield parameters along with superior seed qualities in black gram, Vigna mungo (L.). Waste. Biomass Valorization. 11, 1279–1287. doi: 10.1007/s12649-018-0465-9
Do T. C., Van, Tran D. T., Le T. G., Nguyen Q. T. (2020). Characterization of endogenous auxins and gibberellins produced by Chlorella sorokiniana TH01 under phototrophic and mixtrophic cultivation modes toward applications in microalgal biorefinery and crop research. J. Chem. 2020, 4910621. doi: 10.1155/2020/4910621
Domm P. (2022). A fertilizer shortage, worsened by war in Ukraine, is driving up global food prices and scarcity (CNBC). Available at: https://www.cnbc.com/2022/04/06/a-fertilizer-shortage-worsened-by-war-in-ukraine-is-driving-up-global-food-prices-and-scarcity.html (Accessed July 4, 2022).
Ekinci K., Erdal I., Uysal Ö., Uysal F. Ö., Tunce H., Doğan A. (2019). Anaerobic digestion of three microalgae biomasses and assessment of digestates as biofertilizer for plant growth. Environ. Prog. Sustain. Energy 38, 1–9. doi: 10.1002/ep.13024
El Arroussi H., Benhima R., Elbaouchi A., Sijilmassi B., EL Mernissi N., Aafsar A., et al. (2018). Dunaliella salina exopolysaccharides: a promising biostimulant for salt stress tolerance in tomato (Solanum lycopersicum). J. Appl. Phycol. 30, 2929–2941. doi: 10.1007/s10811-017-1382-1
El Arroussi H., El Mernissi N., Benhima R., El Kadmiri I. M., Bendaou N., Smouni A. (2016). Microalgae polysaccharides a promising plant growth biostimulant. J. Algal. Biomass Utln. 7, 55–63.
El-Naggar A. H., Osman M. E. H. E.-S., Gheda S. F. (2005). Influence of the aqueous extracts of Ulva lactuca and Chlorella kessleri on growth and yield of Vicia faba. Algol. Stud. für. Hydrobiol. Suppl. Vol. 116, 213–229. doi: 10.1127/1864-1318/2005/0116-0213
El Semary N. A. H., Alouane M. H. H., Nasr O., Aldayel M. F., Alhaweti F. H., Ahmed F. (2020). Salinity stress mitigation using encapsulated biofertilizers for sustainable agriculture. Sustainability 12, 9218. doi: 10.3390/su12219218
El-Sheekh M. M., Zayed M. A., Elmossel F. K. A., Hassan R. S. A. (2018). Effect of cyanobacteria isolates on rice seeds germination in saline soil. Baghdad. Sci. J. 15, 16–21. doi: 10.21123/bsj.2018.15.1.0016
Eurostat (2020). Agri-environmental indicator - consumption of pesticides. In: Eurostat - stat. explain. Available at: https://ec.europa.eu/eurostat/statistics-explained/index.php/Agri-environmental_indicator_-_consumption_of_pesticides#Analysis_at_EU_and_country_level (Accessed April 8, 2021).
Faheed F. A., Fattah Z. A.-E. (2008). Effect of Chlorella vulgaris as bio-fertilizer on growth parameters and metabolic aspects of lettuce plant. J. Agric. Soc Sci. 4, 165–169.
Farid R., Mutale-joan C., Redouane B., Mernissi Najib E., Abderahime A., Laila S., et al. (2019). Effect of microalgae polysaccharides on biochemical and metabolomics pathways related to plant defense in Solanum lycopersicum. Appl. Biochem. Biotechnol. 188, 225–240. doi: 10.1007/s12010-018-2916-y
Ferreira A., Figueiredo D., Ferreira F., Ribeiro B., Reis A., Silva T. L., et al. (2022). Impact of high-pressure homogenization on the cell integrity of tetradesmus obliquus and seed germination. Molecules 27, 2275. doi: 10.3390/MOLECULES27072275
Ferreira A., Melkonyan L., Carapinha S., Ribeiro B., Figueiredo D., Avetisova G., et al. (2021). Biostimulant and biopesticide potential of microalgae growing in piggery wastewater. Environ. Adv. 4, 100062. doi: 10.1016/j.envadv.2021.100062
Ferreira A., Ribeiro B., Ferreira A. F., Tavares M. L. A., Vladic J., Vidović S., et al. (2019). Scenedesmus obliquus microalga-based biorefinery – from brewery effluent to bioactive compounds, biofuels and biofertilizers – aiming at a circular bioeconomy. Biofuels. Bioprod. Biorefining. 13, 1169–1186. doi: 10.1002/bbb.2032
Garcia-Gonzalez J., Sommerfeld M. (2016). Biofertilizer and biostimulant properties of the microalga Acutodesmus dimorphus. J. Appl. Phycol. 28, 1051–1061. doi: 10.1007/s10811-015-0625-2
Gauthier M. R., Senhorinho G. N. A., Scott J. A. (2020). Microalgae under environmental stress as a source of antioxidants. Algal. Res. 52, 102104. doi: 10.1016/J.ALGAL.2020.102104
Gayathri M., Shunmugam S., Thajuddin N., Muralitharan G. (2017). Phytohormones and free volatile fatty acids from cyanobacterial biomass wet extract (BWE) elicit plant growth promotion. Algal. Res. 26, 56–64. doi: 10.1016/j.algal.2017.06.022
Gemin L. G., Mógor Á.F., De Oliveira Amatussi J., Mógor G. (2019). Microalgae associated to humic acid as a novel biostimulant improving onion growth and yield. Sci. Hortic. (Amsterdam). 256, 108560. doi: 10.1016/j.scienta.2019.108560
Godlewska K., Michalak I., Pacyga P., Baśladyńska S., Chojnacka K. (2019). Potential applications of cyanobacteria: spirulina platensis filtrates and homogenates in agriculture. World J. Microbiol. Biotechnol. 35, 80. doi: 10.1007/s11274-019-2653-6
Gonçalves A. L. (2021). The use of microalgae and cyanobacteria in the improvement of agricultural practices: a review on their biofertilising, biostimulating and biopesticide roles. Appl. Sci. 11, 1–21. doi: 10.3390/app11020871
González I., Herrero N., Siles J. Á., Chica A. F., Ángeles Martín M., Izquierdo C. G., et al. (2020). Wastewater nutrient recovery using twin-layer microalgae technology for biofertilizer production. Water Sci. Technol. 82, 1044–1061. doi: 10.2166/wst.2020.372
Górka B., Korzeniowska K., Lipok J., Wieczorek P. P. (2018). “The biomass of algae and algal extracts in agricultural production,” in Algae biomass: characteristics and applications. Eds. Chojnacka K., Wiezorek P., Schroeder G., Michalak I. (Cham, Switzerland: Springer International Publishing) 8, 103–114.
Górka B., Lipok J., Wieczorek P. P. (2015). “Biologically active organic compounds, especially plant promoters, in algae extracts and their potential application in plant cultivation,” in Marine algae extracts (Weinheim, Germany: Wiley-VCH Verlag GmbH & Co. KGaA), 659–680. doi: 10.1002/9783527679577.ch37
Grzesik M., Romanowska-Duda Z. (2014). Improvements in germination, growth, and metabolic activity of corn seedlings by grain conditioning and root application with cyanobacteria and microalgae. Polish. J. Environ. Stud. 23, 1147–1153.
Grzesik M., Romanowska-Duda Z., Kalaji H. M. (2017). Effectiveness of cyanobacteria and green algae in enhancing the photosynthetic performance and growth of willow (Salix viminalis l.) plants under limited synthetic fertilizers application. Photosynthetica 55, 510–521. doi: 10.1007/s11099-017-0716-1
Guzmán-Murillo M. A., Ascencio F., Larrinaga-Mayoral J. A. (2013). Germination and ROS detoxification in bell pepper (Capsicum annuum l.) under NaCl stress and treatment with microalgae extracts. Protoplasma 250, 33–42. doi: 10.1007/s00709-011-0369-z
Hu C., Liu Y., Song L., Zhang D. (2002). Effect of desert soil algae on the stabilization of fine sands. J. Appl. Phycol. 14, 281–292. doi: 10.1023/A:1021128530086
Hussain A., Hasnain S. (2011). Phytostimulation and biofertilization in wheat by cyanobacteria. J. Ind. Microbiol. Biotechnol. 38, 85–92. doi: 10.1007/s10295-010-0833-3
Issa O. M., Défarge C., Le Bissonnais Y., Marin B., Duval O., Bruand A., et al. (2007). Effects of the inoculation of cyanobacteria on the microstructure and the structural stability of a tropical soil. Plant Soil 290, 209–2019. doi: 10.1007/s11104-006
Karthikeyan N., Prasanna R., Nain L., Kaushik B. D. (2007). Evaluating the potential of plant growth promoting cyanobacteria as inoculants for wheat. Eur. J. Soil Biol. 43, 23–30. doi: 10.1016/j.ejsobi.2006.11.001
Khan Z., Kim Y. H., Kim S. G., Kim H. W. (2007). Observations on the suppression of root-knot nematode (Meloidogyne arenaria) on tomato by incorporation of cyanobacterial powder (Oscillatoria chlorina) into potting field soil. Bioresour. Technol. 98, 69–73. doi: 10.1016/J.BIORTECH.2005.11.029
Khan S. A., Sharma G. K., Malla F. A., Kumar A., Rashmi, Gupta N. (2019). Microalgae based biofertilizers: a biorefinery approach to phycoremediate wastewater and harvest biodiesel and manure. J. Clean. Prod. 211, 1412–1419. doi: 10.1016/j.jclepro.2018.11.281
Kheirfam H., Sadeghi S. H., Homaee M., Zarei Darki B. (2017). Quality improvement of an erosion-prone soil through microbial enrichment. Soil Tillage. Res. 165, 230–238. doi: 10.1016/j.still.2016.08.021
Kholssi R., Marks E. A. N., Miñón J., Montero O., Debdoubi A., Rad C. (2019). Biofertilizing effect of chlorella sorokiniana suspensions on wheat growth. J. Plant Growth Regul. 38, 644–649. doi: 10.1007/s00344-018-9879-7
Kim J.-D. (2006). Screening of cyanobacteria (Blue-green algae) from rice paddy soil for antifungal activity against plant pathogenic fungi. Mycobiology 34, 138. doi: 10.4489/MYCO.2006.34.3.138
Kim M. J., Shim C. K., Ko B. G., Kim J. (2020). Effect of the microalga chlorella fusca CHK0059 on strawberry PGPR and biological control of fusarium wilt disease in non-pesticide hydroponic strawberry cultivation. J. Microbiol. Biotechnol. 30, 708–716. doi: 10.4014/jmb.2001.01015
Kopta T., Pavlíková M., Sękara A., Pokluda R., Maršálek B. (2018). Effect of bacterial-algal biostimulant on the yield and internal quality of lettuce (Lactuca sativa l.) produced for spring and summer crop. Not. Bot. Horti. Agrobot. Cluj-Napoca. 46, 615–621. doi: 10.15835/nbha46211110
Kusvuran A., Kusvuran S. (2019). Using of microbial fertilizer as biostimulant alleviates damage from drought stress in guar (Cyamopsis tetragonoloba (L.) taub.) seedlings. Int. Lett. Nat. Sci. 76, 147–157. doi: 10.18052/www.scipress.com/ilns.76.147
Liebig N., Salaun M., Monnier C. (2020). Development of standards and guidance documents for biostimulants approval under European fertilizer regulation (EU) 2019/1009. Eurofins. Agrosci. Serv. 2019–2021.
Loganathan B. G., Orsat V., Lefsrud M. (2020). Utilizing the microalgal biomass of chlorella variabilis and scenedesmus obliquus produced from the treatment of synthetic dairy wastewater as a biofertilizer. J. Plant Nutr. 44, 1486–1497. doi: 10.1080/01904167.2020.1862191
Lv J., Liu S., Feng J., Liu Q., Guo J., Wang L., et al. (2020). Effects of microalgal biomass as biofertilizer on the growth of cucumber and microbial communities in the cucumber rhizosphere. Turk. J. Bot. 44, 167–177. doi: 10.3906/bot-1906-1
Manjunath M., Kanchan A., Ranjan K., Venkatachalam S., Prasanna R., Ramakrishnan B., et al. (2016). Beneficial cyanobacteria and eubacteria synergistically enhance bioavailability of soil nutrients and yield of okra. Heliyon 2, e00066. doi: 10.1016/J.HELIYON.2016.E00066
Manjunath M., Prasanna R., Nain L., Dureja P., Singh R., Kumar A., et al. (2010). Biocontrol potential of cyanobacterial metabolites against damping off disease caused by Pythium aphanidermatum in solanaceous vegetables. Arch. Phytopathol. Plant Prot. 43, 666–677. doi: 10.1080/03235400802075815
MarketsandMarkets (2020). Biostimulants market by active ingredient, crop type, application method, form - global forecast 2025. Available at: https://www.marketsandmarkets.com/Market-Reports/biostimulant-market-1081.html (Accessed September 7, 2020).
Marks E. A. N., Miñón J., Pascual A., Montero O., Navas L. M., Rad C. (2017). Application of a microalgal slurry to soil stimulates heterotrophic activity and promotes bacterial growth. Sci. Total. Environ. 605–606, 610–617. doi: 10.1016/j.scitotenv.2017.06.169
Marks E. A. N., Montero O., Rad C. (2019). The biostimulating effects of viable microalgal cells applied to a calcareous soil: increases in bacterial biomass, phosphorus scavenging, and precipitation of carbonates. Sci. Total. Environ. 692, 784–790. doi: 10.1016/j.scitotenv.2019.07.289
Mazhar S., Hasnain S. (2011). Screening of native plant growth promoting cyanobacteria and their impact on triticum aestivum var. uqab 2000 growth. Afr. J. Agric. Res. 6, 3988–3993. doi: 10.5897/AJAR11.560
Michalak I., Chojnacka K., Dmytryk A., Wilk R., Gramza M., Rój E. (2016). Evaluation of supercritical extracts of algae as biostimulants of plant growth in field trials. Front. Plant Sci. 7. doi: 10.3389/fpls.2016.01591
Mógor Á.F., de Oliveira Amatussi J., Mógor G., Bocchetti de Lara G. (2018a). Bioactivity of cyanobacterial biomass related to amino acids induces growth and metabolic changes on seedlings and yield gains of organic red beet. Am. J. Plant Sci. 09, 966–978. doi: 10.4236/ajps.2018.95074
Mógor Á.F., Ördög V., Lima G. P. P., Molnár Z., Mógor G. (2018b). Biostimulant properties of cyanobacterial hydrolysate related to polyamines. J. Appl. Phycol. 30, 453–460. doi: 10.1007/s10811-017-1242-z
Mordor Intelligence (2020). Europe Biostimulants market - growth, trends and forecasts, (2020-2025). Available at: https://www.mordorintelligence.com/industry-reports/europe-biostimulants-market (Accessed September 7, 2020).
Mukherjee C., Chowdhury R., Sutradhar T., Begam M., Ghosh S. M., Basak S. K., et al (2016). Parboiled rice effluent: a wastewater niche for microalgae and cyanobacteria with growth coupled to comprehensive remediation and phosphorus biofertilization. Algal Res. 19, 225–236. doi: 10.1016/j.algal.2016.09.009
Mulbry W., Westhead E. K., Pizarro C., Sikora L. (2005). Recycling of manure nutrients: use of algal biomass from dairy manure treatment as a slow release fertilizer. Bioresour. Technol. 96, 451–458. doi: 10.1016/j.biortech.2004.05.026
Mutale-joan C., Redouane B., Najib E., Yassine K., Lyamlouli K., Laila S., et al. (2020). Screening of microalgae liquid extracts for their bio stimulant properties on plant growth, nutrient uptake and metabolite profile of solanum lycopersicum l. Sci. Rep. 10, 1–12. doi: 10.1038/s41598-020-59840-4
Narain D. (2020). Transforming Indian agriculture: a policy framework t guide US-India partnership (Washington DC). Available at: http://www.fao.org/3/a- (Accessed July 6, 2021).
Natarajan C., Prasanna R., Gupta V., Dureja P., Nain L. (2012). Characterization of the fungicidal activity of calothrix elenkinii using chemical methods and microscopy. Appl. Biochem. Microbiol. 48, 51–57. doi: 10.1134/S0003683812010115
Navarro Q. R., de Oliveira Corrêa D., Behling A., Noseda M. D., Amano É., Suzuki R. M., et al. (2021). Efficient use of biomass and extract of the microalga desmodesmus subspicatus (Scenedesmaceae) in asymbiotic seed germination and seedling development of the orchid cattleya warneri. J. Appl. Phycol. 33, 1–19. doi: 10.1007/s10811-021-02442-y
Navarro-López E., Cerón-García M., del C., López-Rodríguez M., Acién-Fernández F. G., Molina-Grima E. (2020a). Biostimulants obtained after pilot-scale high-pressure homogenization of scenedesmus sp. grown in pig manure. Algal. Res. 52, 102123. doi: 10.1016/j.algal.2020.102123
Navarro-López E., Ruíz-Nieto A., Ferreira A., Gabriel Acién F., Gouveia L. (2020b). Biostimulant potential of scenedesmus obliquus grown in brewery wastewater. Molecules 25, 1–16. doi: 10.3390/molecules25030664
Nayak M., Swain D. K., Sen R. (2019). Strategic valorization of de-oiled microalgal biomass waste as biofertilizer for sustainable and improved agriculture of rice (Oryza sativa l.)crop. Sci. Total. Environ. 682, 475–484. doi: 10.1016/j.scitotenv.2019.05.123
Nilsson M., Bhattacharya J., Rai A. N., Bergman B. (2002). Colonization of roots of rice (Oryza sativa) by symbiotic nostoc strains. New Phytol. 156, 517–525. doi: 10.1046/j.1469-8137.2002.00534.x
Nisha R., Kaushik A., Kaushik C. P. (2007). Effect of indigenous cyanobacterial application on structural stability and productivity of an organically poor semi-arid soil. Geoderma 138, 49–56. doi: 10.1016/j.geoderma.2006.10.007
Nisha R., Kiran B., Kaushik A., Kaushik C. P. (2018). Bioremediation of salt affected soils using cyanobacteria in terms of physical structure, nutrient status and microbial activity. Int. J. Environ. Sci. Technol. 15, 571–580. doi: 10.1007/s13762-017-1419-7
Osman M. E. H., El-Sheekh M. M., El-Naggar A. H., Gheda S. F. (2010). Effect of two species of cyanobacteria as biofertilizers on some metabolic activities, growth, and yield of pea plant. Biol. Fertil. Soils. 46, 861–875. doi: 10.1007/s00374-010-0491-7
Pan S., Jeevanandam J., Danquah M. K. (2019). “Benefits of algal extracts in sustainable agriculture,”. Grand Challenges in Algae Biotechnology. Grand Challenges in Biology and Biotechnology. Eds. Hallmann A., Rampelotto P. (Cham, Switzerland: Springer), 501–534.
Pereira N. S., Ferreira B. R. R., de Carvalho E. M., Damiani C. R. (2018). Application of chlorella sorokiniana (Chlorophyceae) as supplement and/or an alternative medium for the in vitro cultivation of schomburgkia crispa (Orchidaceae). J. Appl. Phycol. 30, 2347–2358. doi: 10.1007/s10811-018-1441-2
Pereira I., Ortega R., Barrientos L., Moya M., Reyes G., Kramm V. (2009). Development of a biofertilizer based on filamentous nitrogen-fixing cyanobacteria for rice crops in Chile. J. Appl. Phycol. 21, 135–144. doi: 10.1007/s10811-008-9342-4
Plaza B. M., Gómez-Serrano C., Acién-Fernández F. G., Jimenez-Becker S. (2018). Effect of microalgae hydrolysate foliar application (Arthrospira platensis and scenedesmus sp.) on petunia x hybrida growth. J. Appl. Phycol. 30, 2359–2365. doi: 10.1007/s10811-018-1427-0
Povero G., Mejia J. F., Di Tommaso D., Piaggesi A., Warrior P. (2016). A systematic approach to discover and characterize natural plant biostimulants. Front. Plant Sci. 7. doi: 10.3389/fpls.2016.00435
Prasanna R., Adak A., Verma S., Bidyarani N., Babu S., Pal M., et al. (2015a). Cyanobacterial inoculation in rice grown under flooded and SRI modes of cultivation elicits differential effects on plant growth and nutrient dynamics. Ecol. Eng. 84, 532–541. doi: 10.1016/j.ecoleng.2015.09.033
Prasanna R., Babu S., Bidyarani N., Kumar A., Triveni S., Monga D., et al. (2015b). Prospecting cyanobacteria-fortified composts as plant growth promoting and biocontrol agents in cotton. Exp. Agric. 51, 42–65. doi: 10.1017/S0014479714000143
Prasanna R., Babu S., Rana A., Kabi S. R., Chaudhary V., Gupta V., et al. (2013a). Evaluating the establishment and agronomic proficiency of cyanobacterial consortia as organic options in wheat-rice cropping sequence. Exp. Agric. 49, 416–434. doi: 10.1017/S001447971200107X
Prasanna R., Chaudhary V., Gupta V., Babu S., Kumar A., Singh R., et al. (2013b). Cyanobacteria mediated plant growth promotion and bioprotection against fusarium wilt in tomato. Eur. J. Plant Pathol. 136, 337–353. doi: 10.1007/s10658-013-0167-x
Prasanna R., Gupta H., Yadav V. K., Gupta K., Buddhadeo R., Gogoi R., et al. (2020). Prospecting the promise of cyanobacterial formulations developed using soil-less substrates as carriers. Environ. Technol. Innov. 18, 100652. doi: 10.1016/j.eti.2020.100652
Prasanna R., Hossain F., Babu S., Bidyarani N., Adak A., Verma S., et al. (2015c). Prospecting cyanobacterial formulations as plant-growth-promoting agents for maize hybrids. South Afr. J. Plant Soil 32, 199–207. doi: 10.1080/02571862.2015.1025444
Prasanna R., Kanchan A., Ramakrishnan B., Ranjan K., Venkatachalam S., Hossain F., et al. (2016a). Cyanobacteria-based bioinoculants influence growth and yields by modulating the microbial communities favourably in the rhizospheres of maize hybrids. Eur. J. Soil Biol. 75, 15–23. doi: 10.1016/j.ejsobi.2016.04.001
Prasanna R., Nain L., Tripathi R., Gupta V., Chaudhary V., Middha S., et al. (2008). Evaluation of fungicidal activity of extracellular filtrates of cyanobacteria - possible role of hydrolytic enzymes. J. Basic. Microbiol. 48, 186–194. doi: 10.1002/jobm.200700199
Prasanna R., Pattnaik S., Sugitha T. C. K., Nain L., Saxena A. K. (2011). Development of cyanobacterium-based biofilms and their in vitro evaluation for agriculturally useful traits. Folia Microbiol. 56, 49–58. doi: 10.1007/S12223-011-0013-5
Prasanna R., Ramakrishnan B., Ranjan K., Venkatachalam S., Kanchan A., Solanki P., et al. (2016b). Microbial inoculants with multifaceted traits suppress Rhizoctonia populations and promote plant growth in cotton. J. Phytopathol. 164, 1030–1042. doi: 10.1111/jph.12524
Prasanna R., Triveni S., Bidyarani N., Babu S., Yadav K., Adak A., et al. (2014). Evaluating the efficacy of cyanobacterial formulations and biofilmed inoculants for leguminous crops. Arch. Agron. Soil Sci. 60, 349–366. doi: 10.1080/03650340.2013.792407
Priya M., Gurung N., Mukherjee K., Bose S. (2014). “23 - Microalgae in removal of heavy metal and organic pollutants from soil,” Microbial Biodegradation and Bioremediation. ed. Das S. (London, UK: Elsevier), 519–537. doi: 10.1016/B978-0-12-800021-2.00023-6
Priya H., Prasanna R., Ramakrishnan B., Bidyarani N., Babu S., Thapa S., et al. (2015). Influence of cyanobacterial inoculation on the culturable microbiome and growth of rice. Microbiol. Res. 171, 78–89. doi: 10.1016/j.micres.2014.12.011
Puglisi I., La Bella E., Rovetto E. I., Lo Piero A. R., Baglieri A. (2020). Biostimulant effect and biochemical response in lettuce seedlings treated with a scenedesmus quadricauda extract. Plants 9, 123. doi: 10.3390/plants9010123
Rachidi F., Benhima R., Kasmi Y., Sbabou L., El Arroussi H. (2021). Evaluation of microalgae polysaccharides as biostimulants of tomato plant defense using metabolomics and biochemical approaches. Sci. Rep. 11, 930. doi: 10.1038/s41598-020-78820-2
Ramírez-López C., Esparza-García F. J., Ferrera-Cerrato R., Alarcón A., Cañizares-Villanueva R. O. (2019). Short-term effects of a photosynthetic microbial consortium and nitrogen fertilization on soil chemical properties, growth, and yield of wheat under greenhouse conditions. J. Appl. Phycol. 31, 3617–3624. doi: 10.1007/s10811-019-01861-2
Rana A., Joshi M., Prasanna R., Shivay Y. S., Nain L. (2012). Biofortification of wheat through inoculation of plant growth promoting rhizobacteria and cyanobacteria. Eur. J. Soil Biol. 50, 118–126. doi: 10.1016/J.EJSOBI.2012.01.005
Ranglová K., Lakatos G. E., Câmara Manoel J. A., Grivalský T., Suárez Estrella F., Acién Fernández F. G., et al. (2021). Growth, biostimulant and biopesticide activity of the MACC-1 Chlorella strain cultivated outdoors in inorganic medium and wastewater. Algal. Res. 53, 102136. doi: 10.1016/j.algal.2020.102136
Rashad S., El-Hassanin A. S., Mostafa S. S.M., El-Chaghaby G. A. (2019). Cyanobacteria cultivation using olive milling wastewater for bio-fertilization of celery plan. Glob. J. Environ. Sci. Manag. 5, 167–174. doi: 10.22034/gjesm.2019.02.03
Regulation (EU) 2019/1009 (2019). REGULATION (EU) 2019/1009 OF THE EUROPEAN PARLIAMENT AND OF THE COUNCIL of 5 June 2019 laying down rules on the making available on the market of EU fertilising products and amending regulations (EC) no 1069/2009 and (EC) no 1107/2009 and repealing regula.
Renuka N., Guldhe A., Prasanna R., Singh P., Bux F. (2018). Microalgae as multi-functional options in modern agriculture: current trends, prospects and challenges. Biotechnol. Adv. 36, 1255–1273. doi: 10.1016/j.biotechadv.2018.04.004
Renuka N., Prasanna R., Sood A., Ahluwalia A. S., Bansal R., Babu S., et al. (2016). Exploring the efficacy of wastewater-grown microalgal biomass as a biofertilizer for wheat. Environ. Sci. pollut. Res. 23, 6608–6620. doi: 10.1007/s11356-015-5884-6
Righini H., Francioso O., Di Foggia M., Prodi A., Quintana A. M., Roberti R. (2021). Tomato seed biopriming with water extracts from anabaena minutissima, ecklonia maxima and jania adhaerens as a new agro-ecological option against rhizoctonia solani. Sci. Hortic. (Amsterdam). 281, 109921. doi: 10.1016/J.SCIENTA.2021.109921
Ronga D., Biazzi E., Parati K., Carminati D., Carminati E., Tava A. (2019). Microalgal biostimulants and biofertilisers in crop productions. Agronomy 9, 192. doi: 10.3390/agronomy9040192
Saadaoui I., Sedky R., Rasheed R., Bounnit T., Almahmoud A., Elshekh A., et al. (2019). Assessment of the algae-based biofertilizer influence on date palm (Phoenix dactylifera l.) cultivation. J. Appl. Phycol. 31, 457–463. doi: 10.1007/s10811-018-1539-6
Saadatnia H., Riahi H. (2009). Cyanobacteria from paddy fields in Iran as a biofertilizer in rice plants. Plant. Soil Environ. 55, 207–212. doi: 10.17221/384-pse
Saber A. A., Hamed S. M., Abdel-Rahim E. F. M., Cantonati M. (2018). Insecticidal prospects of algal and cyanobacterial extracts against the cotton leafworm spodoptera littoralis. Vie. Milieu. 68, 199–212.
Sahu D., Priyadarshani I., Rath B. (2012). Cyanobacteria -as potential biofertilizer. CIBTech. J. Microbiol. Jul.-Sept. Oct.-Dec 1, 2319–386720. Available at: http://www.cibtech.org/cjm.htm. (Accessed May 29, 2023).
Salvi L., Niccolai A., Cataldo E., Sbraci S., Paoli F., Storchi P., et al. (2020). Effects of arthrospira platensis extract on physiology and berry traits in vitis vinifera. Plants 9, 1805. doi: 10.3390/plants9121805
Schreiber C., Schiedung H., Harrison L., Briese C., Ackermann B., Kant J., et al. (2018). Evaluating potential of green alga chlorella vulgaris to accumulate phosphorus and to fertilize nutrient-poor soil substrates for crop plants. J. Appl. Phycol. 30, 2827–2836. doi: 10.1007/s10811-018-1390-9
Shaaban M. M. (2001a). Green microalgae water extract as foliar feeding to wheat plants. Pakistan J. Biol. Sci. 4, 628–632. doi: 10.3923/pjbs.2001.628.632
Shaaban M. M. (2001b). Nutritional status and growth of maize plants as affected by green microalgae as soil additives. J. Biol. Sci. 1, 475–479. doi: 10.3923/jbs.2001.475.479
Shamim A., Mahfooz S., Hussain A., Farooqui A. (2020). Ability of Al-acclimatized immobilized nostoc muscorum to combat abiotic stress and its potential as a biofertilizer. J. Pure. Appl. Microbiol. 14, 1377–1386. doi: 10.22207/JPAM.14.2.35
Shariatmadari Z., Riahi H., Seyed Hashtroudi M., Ghassempour A., Aghashariatmadary Z. (2013). Plant growth promoting cyanobacteria and their distribution in terrestrial habitats of Iran. Soil Sci. Plant Nutr. 59, 535–547. doi: 10.1080/00380768.2013.782253
Sharma R., Khokhar M. K., Jat R. L., Khandelwal S. K. (2012). Role of algae and cyanobacteria in sustainable agriculture system. Wudpecker. J. Agric. Res. 1, 381–388.
Sholkamy E. N., El-Komy H. M., Ali H. M. (2015). Enhancement of soybean (Glycine max l.) growth by bio-fertilizers of Nostoc muscorum and Nostoc rivulare. Pakistan J. Bot. 47, 1199–1204. Available at: http://www.pakbs.org/pjbot/PDFs/47(3)/50.pdf. (Accessed May 29, 2023).
Silambarasan S., Logeswari P., Sivaramakrishnan R., Incharoensakdi A., Cornejo P., Kamaraj B., et al. (2021). Removal of nutrients from domestic wastewater by microalgae coupled to lipid augmentation for biodiesel production and influence of deoiled algal biomass as biofertilizer for solanum lycopersicum cultivation. Chemosphere 268, 129323. doi: 10.1016/j.chemosphere.2020.129323
Silva D.S.O.e, Rocha R. H. C., da Nóbrega J. S., Dias G. A., de Lima J. F., Guedes W. A. (2017). Post-harvest quality of lettuce cv. Elba in relation to spirulina platensis foliar applications. Científica 45, 162–168. doi: 10.15361/1984-5529.2017v45n2p162-168
Simranjit K., Kanchan A., Prasanna R., Ranjan K., Ramakrishnan B., Singh A. K., et al. (2019). Microbial inoculants as plant growth stimulating and soil nutrient availability enhancing options for cucumber under protected cultivation. World J. Microbiol. Biotechnol. 35, 51. doi: 10.1007/s11274-019-2623-z
Singh J. S., Kumar A., Rai A. N., Singh D. P. (2016). Cyanobacteria: a precious bio-resource in agriculture, ecosystem, and environmental sustainability. Front. Microbiol. 7. doi: 10.3389/fmicb.2016.00529
Smith C., Hill A. K., Torrente-Murciano L. (2020). Current and future role of haber–Bosch ammonia in a carbon-free energy landscape. Energy Environ. Sci. 13, 331–344. doi: 10.1039/C9EE02873K
Soppelsa S., Kelderer M., Casera C., Bassi M., Robatscher P., Matteazzi A., et al. (2019). Foliar applications of biostimulants promote growth, yield and fruit quality of strawberry plants grown under nutrient limitation. Agronomy 9, 483. doi: 10.3390/agronomy9090483
Srivastava S., Srivastava S., Bist V., Awasthi S., Chauhan R., Chaudhry V., et al. (2018). Chlorella vulgaris and pseudomonas putida interaction modulates phosphate trafficking for reduced arsenic uptake in rice (Oryza sativa l.). J. Hazard. Mater. 351, 177–187. doi: 10.1016/j.jhazmat.2018.02.039
Stirk W. A., Bálint P., Vambe M., Lovász C., Molnár Z., van Staden J., et al. (2020). Effect of cell disruption methods on the extraction of bioactive metabolites from microalgal biomass. J. Biotechnol. 307, 35–43. doi: 10.1016/j.jbiotec.2019.10.012
Stirk W. A., Ördög V., Van Staden J., Jäger K. (2002). Cytokinin- and auxin-like activity in cyanophyta and microalgae. J. Appl. Phycol. 14, 215–221. doi: 10.1023/A:1019928425569
Supraja K. V., Behera B., Balasubramanian P. (2020). Efficacy of microalgal extracts as biostimulants through seed treatment and foliar spray for tomato cultivation. Ind. Crops Prod. 151, 112453. doi: 10.1016/j.indcrop.2020.112453
Suresh A., Soundararajan S., Elavarasi S., Lewis Oscar F., Thajuddin N. (2019). Evaluation and characterization of the plant growth promoting potentials of two heterocystous cyanobacteria for improving food grains growth. Biocatal. Agric. Biotechnol. 17, 647–652. doi: 10.1016/j.bcab.2019.01.002
Suresh Kumar K., Dahms H. U., Won E. J., Lee J. S., Shin K. H. (2015). Microalgae – a promising tool for heavy metal remediation. Ecotoxicol. Environ. Saf. 113, 329–352. doi: 10.1016/J.ECOENV.2014.12.019
Swarnalakshmi K., Prasanna R., Kumar A., Pattnaik S., Chakravarty K., Shivay Y. S., et al. (2013). Evaluating the influence of novel cyanobacterial biofilmed biofertilizers on soil fertility and plant nutrition in wheat. Eur. J. Soil Biol. 55, 107–116. doi: 10.1016/j.ejsobi.2012.12.008
Tejada-Ruiz S., Gonzalez-Lopez C., Rojas E., Jiménez-Becker S. (2020). Effect of the foliar application of microalgae hydrolysate (Arthrospira platensis) and silicon on the growth of pelargonium hortorum L.H. bailey under salinity conditions. Agronomy 10, 1713. doi: 10.3390/agronomy10111713
Tripathi R. D., Dwivedi S., Shukla M. K., Mishra S., Srivastava S., Singh R., et al. (2008). Role of blue green algae biofertilizer in ameliorating the nitrogen demand and fly-ash stress to the growth and yield of rice (Oryza sativa l.) plants. Chemosphere 70, 1919–1929. doi: 10.1016/j.chemosphere.2007.07.038
Triveni S., Prasanna R., Kumar A., Bidyarani N., Singh R., Saxena A. K. (2015). Evaluating the promise of trichoderma and anabaena based biofilms as multifunctional agents in macrophomina phaseolina-infected cotton crop. Biocontrol Sci. Technol. 25, 656–670. doi: 10.1080/09583157.2015.1006171
USGS (2021). Map of worldwide croplands. In: Glob. food secur. anal. data 30 meters proj. Available at: https://www.croplands.org/app/map?lat=0.17578&lng=0&zoom=2 (Accessed July 6, 2021).
Uysal O., Uysal F. O., Ekinci K. (2015). Evaluation of microalgae as microbial fertilizer. Eur. J. Sustain. Dev. 4, 77–82. doi: 10.14207/ejsd.2015.v4n2p77
Viegas C., Gouveia L., Gonçalves M. (2021a). Aquaculture wastewater treatment through microalgal. biomass potential applications on animal feed, agriculture, and energy. J. Environ. Manage. 286, 112187. doi: 10.1016/J.JENVMAN.2021.112187
Viegas C., Gouveia L., Gonçalves M. (2021b). Bioremediation of cattle manure using microalgae after pre-treatment with biomass ash. Bioresour. Technol. Rep. 14, 100681. doi: 10.1016/J.BITEB.2021.100681
Viegas C., Gouveia L., Gonçalves M. (2021c). Evaluation of microalgae as bioremediation agent for poultry effluent and biostimulant for germination. Environ. Technol. Innov. 24, 102048. doi: 10.1016/J.ETI.2021.102048
Win T. T., Barone G. D., Secundo F., Fu P. (2018). Algal biofertilizers and plant growth stimulants for sustainable agriculture. Ind. Biotechnol. 14, 203–211. doi: 10.1089/ind.2018.0010
Wuang S. C., Khin M. C., Chua P. Q. D., Luo Y. D. (2016). Use of spirulina biomass produced from treatment of aquaculture wastewater as agricultural fertilizers. Algal. Res. 15, 59–64. doi: 10.1016/j.algal.2016.02.009
Xu Y., Rossi F., Colica G., Deng S., De Philippis R., Chen L. (2013). Use of cyanobacterial polysaccharides to promote shrub performances in desert soils: a potential approach for the restoration of desertified areas. Biol. Fertil. Soils. 49, 143–152. doi: 10.1007/s00374-012-0707-0
Yadavalli R., Heggers G. R. V. N. (2013). Two stage treatment of dairy effluent using immobilized chlorella pyrenoidosa. J. Environ. Heal. Sci. Eng. 11, 1–6. doi: 10.1186/2052-336x-11-36
Zaki S. S., Belal E. E. E., Rady M. M. (2019). Cyanobacteria and glutathione applications improve productivity, nutrient contents, and antioxidant systems of salt-stressed soybean plant. Int. Lett. Nat. Sci. 76, 72–85. doi: 10.18052/www.scipress.com/ilns.76.72
Zarezadeh S., Moheimani N. R., Jenkins S. N., Hülsen T., Riahi H., Mickan B. S. (2019). Microalgae and phototrophic purple bacteria for nutrient recovery from agri-industrial effluents: influences on plant growth, rhizosphere bacteria, and putative carbon- and nitrogen-cycling genes. Front. Plant Sci. 10. doi: 10.3389/fpls.2019.01193
Zayadan B. K., Matorin D. N., Baimakhanova G. B., Bolathan K., Oraz G. D., Sadanov A. K. (2014). Promising microbial consortia for producing biofertilizers for rice fields. Microbiol. (Russian. Fed. 83, 391–397. doi: 10.1134/S0026261714040171
Zhang J., Song X., Wei H., Zhou W., Peng C., Li D. (2021). Effect of substituting nitrogen fertilizer with nitrogen-fixing cyanobacteria on yield in a double-rice cropping system in southern China. J. Appl. Phycol. 33, 2221–2232. doi: 10.1007/s10811-021-02455-7
Zilio M., Pigoli A., Rizzi B., Herrera A., Tambone F., Geromel G., et al. (2022). Using highly stabilized digestate and digestate-derived ammonium sulphate to replace synthetic fertilizers: the effects on soil, environment, and crop production. Sci. Total. Environ. 815, 152919. doi: 10.1016/J.SCITOTENV.2022.152919
Keywords: wastewater, microalgae, cyanobacteria, biostimulants, biofertilizers, biopesticides, biocontrol
Citation: Ferreira A, Bastos CRV, Marques-dos-Santos C, Acién-Fernandez FG and Gouveia L (2023) Algaeculture for agriculture: from past to future. Front. Agron. 5:1064041. doi: 10.3389/fagro.2023.1064041
Received: 07 October 2022; Accepted: 25 May 2023;
Published: 09 June 2023.
Edited by:
Rachid Bouharroud, National Institute of Agronomic Research, MoroccoReviewed by:
Mohammed Antar, McGill University, Macdonald Campus, CanadaCopyright © 2023 Ferreira, Bastos, Marques-dos-Santos, Acién-Fernandez and Gouveia. This is an open-access article distributed under the terms of the Creative Commons Attribution License (CC BY). The use, distribution or reproduction in other forums is permitted, provided the original author(s) and the copyright owner(s) are credited and that the original publication in this journal is cited, in accordance with accepted academic practice. No use, distribution or reproduction is permitted which does not comply with these terms.
*Correspondence: Alice Ferreira, YWxpY2UuZmVycmVpcmFAbG5lZy5wdA==
†These authors have contributed equally to this work
Disclaimer: All claims expressed in this article are solely those of the authors and do not necessarily represent those of their affiliated organizations, or those of the publisher, the editors and the reviewers. Any product that may be evaluated in this article or claim that may be made by its manufacturer is not guaranteed or endorsed by the publisher.
Research integrity at Frontiers
Learn more about the work of our research integrity team to safeguard the quality of each article we publish.