- 1Department of Microbiology, University of Manitoba, Winnipeg, MB, Canada
- 2 Department of Agricultural Chemistry, University of Jaffna, Jaffna, Sri Lanka
Modern agriculture faces several challenges due to climate change, limited resources, and land degradation. Plant-associated soil microbes harbor beneficial plant growth-promoting (PGP) traits that can be used to address some of these challenges. These microbes are often formulated as inoculants for many crops. However, inconsistent productivity can be a problem since the performance of individual inoculants/microbes vary with environmental conditions. Over the past decade, the ability to utilize Next Generation Sequencing (NGS) approaches with soil microbes has led to an explosion of information regarding plant associated microbiomes. Although this type of work has been predominantly sequence-based and often descriptive in nature, increasingly it is moving towards microbiome functionality. The synthetic microbial communities (SynCom) approach is an emerging technique that involves co-culturing multiple taxa under well-defined conditions to mimic the structure and function of a microbiome. The SynCom approach hopes to increase microbial community stability through synergistic interactions between its members. This review will focus on plant-soil-microbiome interactions and how they have the potential to improve crop production. Current approaches in the formulation of synthetic microbial communities will be discussed, and its practical application in agriculture will be considered.
Introduction
Agricultural production must increase by about 70% from its current level by 2050 to meet the demand for a growing population (ELD Initiative, 2015; Singh et al., 2020). However, current studies estimate that global food production will decrease by 12% over the next 25 years due to the degradation of agricultural lands (ELD Initiative, 2015). After the second industrial revolution, traditional agricultural practices shifted towards the use of synthetic chemical fertilizers and pesticides to improve crop production (Melillo, 2012; Dixon, 2018). The intensive use of these agrochemicals has led to the deterioration of the quality of both the soil as well as the environment (Meena V. S. et al., 2017). A possible solution to mitigate some of these problems might be the development of sustainable agriculture practices that harness crop-associated microbiomes to either increase or sustain higher yields while maintaining overall soil health and fertility (Toju et al., 2018; Singh et al., 2020).
Regardless of whether animals or plants are considered, microbial communities play vital roles in their respective ecosystems. The soil microbiome is defined as the microbial communities present in the soil and their encoded functions. Within the soil microbes can be found as both free-living or in symbiotic relationships with higher organisms (Banerjee et al., 2018), and are often considered key drivers of beneficial processes such as nutrient cycling and carbon sequestration (Fierer, 2017; Wallenstein, 2017; Qiu et al., 2019). Microorganisms that can form complex co-associations with plants obtain their carbon sources and other metabolites from the plant while performing these beneficial processes (Backer et al., 2018; Trivedi et al., 2020).
With up to 20-40% of a plant’s photosynthate becoming root exudate (Lynch and Whipps, 1990), it is not surprising that plants encourage microbial growth, and that changes in the exudation components can modify the composition of the associated microbial community (Wallenstein, 2017; Vives-Peris et al., 2020). Although many microorganisms can respond to plant exudates, it is becoming clear that plants harbor a specific subset of microorganisms, termed the core microbiome, that is consistently associated with a particular plant host across a wide range of environments (Toju et al., 2018; Walters et al., 2018). The core microbiome has been shown to provide several functional benefits to plants that include, but are not limited to, enhancing plant mineral nutrient uptake, and suppressing soil borne diseases (Lemanceau et al., 2017a; Banerjee et al., 2018; Singh et al., 2020). Additionally, it has also been observed that plants can recruit transient microbes that vary in composition and abundance to alleviate environmental stress (Berg et al., 2020).
Over the past two decades, microbes with plant growth-promoting (PGP) traits have been isolated and used as inoculants to improve crop production (Finkel et al., 2017; Banerjee et al., 2018; de Souza et al., 2020). Microbes assist plant growth either by enhancing nutrient acquisition such as nitrogen fixation, phosphorus solubilization, and siderophore production or producing plant growth promoting substances (Olanrewaju et al., 2017; Saleem et al., 2018; Chaudhary et al., 2021; Joshi et al., 2021). In addition, microbial inoculants have the potential to suppress several pathogenic organisms (Yasmin et al., 2016; Olanrewaju et al., 2017; Abbasi et al., 2021). The main drawback of microbial application is that it often fails to yield consistent results because the plant-microbe association has not been considered with respect to various biotic and abiotic stress conditions that can affect the outcome (Finkel et al., 2017; de Souza et al., 2020; Hawkins & Oresnik, 2022). For inoculums to be successful in the field, an in-depth knowledge of microbial abundance, diversity, as well as plant-microbe interactions, is essential to be able to predict overall functionality (Chodkowski and Shade, 2017).
The synthetic community (SynCom) approach is an emerging research field that incorporates a synthetic biology approach that is coupled with the knowledge that has been generated from microbial community analysis, metagenomic, and bioinformatic approaches that have become more accessible with the advent of Next Generation Sequencing technologies. Understanding the dynamic interactions within microbial ecosystems is useful to engineer microbial consortia with robust, stable, and predictable behaviours (McCarty and Ledesma-Amaro, 2019).
Briefly, SynComs are constructed by co-culturing multiple taxa under well-defined conditions to mimic the structure and function of a microbiome. The underlying principle is to reduce the complexity of the original microbial community, while still preserving some of the essential interactions between the microbes and their hosts (Vorholt et al., 2017; Kaminsky et al., 2019; de Souza et al., 2020). The goal is to facilitate an increase in community stability through synergistic interactions between its members (de Souza et al., 2020). Several studies have been reported that SynCom application enhanced plant growth under greenhouse conditions (Armanhi et al., 2021; Chai et al., 2021; Lee et al., 2021) as well as field conditions (Santhanam et al., 2015; Wang et al., 2021).
Advancements in high-throughput sequencing technologies and their associated bioinformatics tools has provided the opportunity to discover the complexities associated with plant-microbe interactions and the functionality they can provide to the plant. The aim of this review is to encapsulate factors which can play contributing roles to the outcome of an engineered plant-microbe interaction, the current state of SynCom technology, and to consider whether this type of approach has the ability to affect crop production.
Microbes at the Plant- Soil Interface
The interaction of microbes with plants occurs across their entire life cycle. These interactions can occur both above as well as below ground level. Whereas some are due to chance, many interactions are orchestrated by the plant. It can occur through vertical transfer, such as when endophytes living within a plant are transmitted via vascular connections or when bacteria become incorporated within a developing seed and may play a role in seed germination and the development of a root system to aid in initial establishment and plant survival (Mitter et al., 2016). In addition, plant roots interact with the soil and actively exude carbon containing compounds that influences all microbial growth around a root. Bacteria drawn to the plant in this manner are horizontally transferred from bulk soil to the rhizosphere.
The rhizosphere is defined as the soil under direct influence of root exudates (Moe, 2013; Reinhold-Hurek et al., 2015; Hartman and Tringe, 2019). This zone has been further subdivided into the endorhizosphere, the ectorhizosphere, and the rhizoplane (Figure 1). The endorhizosphere consists of the zone of tissue in the plant root that can be occupied by microorganisms (McNear, 2013). The endorhizoshpere is delineated by the rhizoplane, which is the surface of the root, and beyond this is the ectorhizosphere which is influenced by root exudation and rhizodeposition (Reinhold-Hurek et al., 2015).
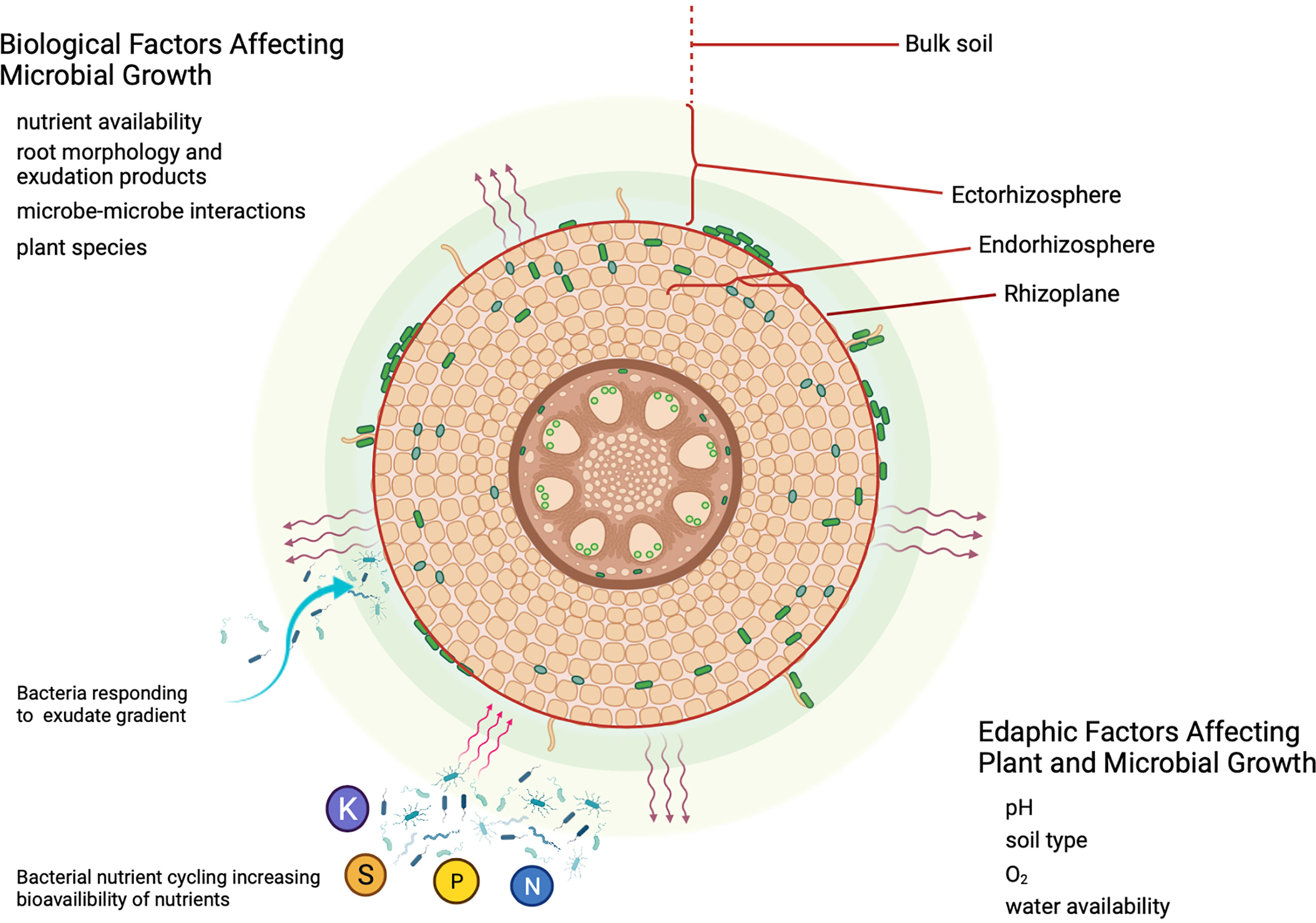
Figure 1 Root rhizosphere and factors affecting microbiome development. A diagrammatic representation of a root cross-section. Factors affecting microbial and plant growth are presented as tables (see text for details). The rhizosphere is depicted as two tones (green and pale yellow) surrounding the root representing an exudation gradient. The endorhizosphere, rhizoplane, ectorhizosphere and bulk soil are highlighted using red brackets, and lines. Endophytic bacteria and bacteria living on the rhizoplane are depicted as green rods. Plant exudation is represented as purple wavy arrows emanating from the root surface. Microbial communities in the rhizosphere are depicted as responding to exudates (single blue wavy arrow), and as bacteria involved in nutrient cycling. Major nutrients are depicted as spheres with letters (N, nitrogen; P, phosphorus; K, potassium; S, sulfur). Wavy tan arrows represent nutrients and/or bacterial factors that benefit plant growth. This figure was created with Biorender.com.
The bulk soil microbiome acts as a potential source of inoculants for the rhizosphere microbiome. Composition of the rhizosphere microbiome is structured differently from the soil microbiome (Crecchio et al., 2018). This differentiation is initiated by plants through root exudates that attract specific microbes to the rhizosphere to support plant growth and development (Wallenstein, 2017; Vives-Peris et al., 2020). By regulating the secretion of signaling compounds and activation of plant immune responses, the plant can influence the recruitment of a subset of microbes from the rhizosphere to attach to the rhizoplane and subsequently to move from the rhizoplane to the endorhizosphere (Hacquard et al., 2015; Hartman and Tringe, 2019). In general, it has been observed that plants modify their rhizosphere to attract organisms that have beneficial traits such as plant growth promotion, solubilization of nutrients, and inhibition of pathogen growth (Andreote et al., 2014).
When compared to bulk soil, the rhizosphere microbiome has a richer, and functionally, better-characterized microbiome. Bulgarelli et al. (2015) reported that the rhizosphere and root microbiomes of barley differentiated from the soil microbiomes as a gradient. The soil microbiomes showed higher bacterial richness and diversity compared with root samples, while the rhizosphere microbiota composition was intermediate between soil and root samples. Similarly, higher microbial richness was reported in the bulk soil surrounding the rhizosphere soil of maize (Walters et al., 2018). Additionally, it was observed that the rhizosphere microbial communities had greater network connectivity than the bulk soil in maize and wild oat (Peiffer et al., 2013; Shi et al., 2016; Walters et al., 2018). Collectively this suggests that roots can promote the development of niches with dominant taxa that favor greater interactions and more complex co-occurrence patterns over time.
Major Drivers of Microbial Diversity in Plant Ecosystem
Defining the major drivers for microbial diversity is a challenging task since plant-microbe interactions form a complex relationship. Several factors influence the composition of the microbial communities such as plant, microbe-microbe interaction, and edaphic factors. These factors influence the selection of microbes primarily through root exudates. Root exudates consist of a variety of chemicals, primary metabolites, and secondary metabolites (Rasmann and Turlings, 2016; Tsunoda and van Dam, 2017; Vives-Peris et al., 2020). Primary metabolites, such as the labile carbon of root exudates, increase the growth of fast-growing microorganisms with higher nutritional requirements enabling them to outcompete slow-growing microorganisms with lower nutritional requirements (Terrazas et al., 2016). Several studies indicate that bacteria belonging to the phylum Proteobacteria, which are known to respond to labile carbon (Peiffer et al., 2013), are enriched in the rhizosphere compared to bulk soils (Aira et al., 2010; Chauhan et al., 2011; Lundberg et al., 2012; Peiffer et al., 2013; Zhang et al., 2020). Likewise, secondary metabolites trigger varying responses in organisms. Flavonoids, for example, attract symbionts in nodule formation, stimulate mycorrhizal spore germination and hyphal branching, and influence quorum sensing in legumes (Philippot et al., 2013). By regulating the composition of root exudates, the microbial diversity in the plant ecosystem can be substantially altered.
Plant factors consist of the plant species, genotype, immune system, physiological age, nutritional status, and pathogen infection (Hawkes et al., 2007; van Overbeek and Elsas, 2008; Sharma and Verma, 2018; Zhalnina et al., 2018; Vives-Peris et al., 2020). Plant species strongly influences the structure of rhizosphere communities through differences in root morphology and exudation of different metabolites (Philippot et al., 2013). Colonization of different bacterial populations due to root exudates was observed in the rhizosphere of four plant species – wheat, maize, rape, and barrel clover (Haichar et al., 2008). Similarly, activity and dynamics of the indigenous Pseudomonas spp. In the rhizosphere were significantly influenced by host plant species (Bergsma-Vlami et al., 2005). Enrichment of antifungal microbial communities was reported in barley rhizosphere after the infection of Fusarium graminearum (Dudenhoffer et al., 2016). A high rate of nitrogen application increased the relative abundances of ammonia-oxidizing and denitrifying bacterial communities in maize rhizosphere (Zhu et al., 2016). It has also been reported that plant genotypes in A. thaliana (Micallef et al., 2009), Solanum tuberosum (Inceoglu et al., 2010), grapevine (Berlanas et al., 2019), and Zea mays (Aira et al., 2010) influence the production of root exudates thereby changing their microbial communities. Aira et al. reported that the rhizosphere microbial communities of two maize hybrids were strongly influenced by plant genotype (Aira et al., 2010). In contrast, a large-scale longitudinal study conducted in five fields with 27 maize inbred lines reported that plant age was the strongest factor shaping the rhizosphere microbial community followed by location and genotype (Walters et al., 2018). However, within a given field, plant genotype significantly influenced the richness of the microbiome (Peiffer et al., 2013). A study focused on the sugarcane microbiome under field conditions demonstrated that microbial communities were primarily influenced by the plant compartments followed by the growing region, the age and variety of the crop (Hamonts et al., 2018). The influence of plant factors on the composition of microbes is obvious under the same environmental conditions.
In addition to host-microbe associations, microbe-microbe interactions also affect the structure of microbial communities in the rhizosphere (Bulgarelli et al., 2015). There are a wide range of microbe-microbe interactions ranging from synergistic to antagonistic which could shape the composition of the plant microbiota (Hacquard et al., 2015; Terrazas et al., 2016). Soil microbes can also affect the root exudation process by consuming primary root exudates or releasing secondary compounds to stimulate specific metabolite production (Canarini et al., 2019). Specific microbial taxa on tomato rhizosphere were found to modify the chemical composition of root exudates, for example acylsucrose exudation was induced by Bacillus subtilis (Korenblum et al., 2020). Further, microbial interactions assist the host plant to mitigate several abiotic stresses through direct antagonization against pathogens or induction of systemic resistance by priming plants (Meena K. K. et al., 2017; Arif et al., 2020). Several microbes secret an enzyme, 1-aminocyclopropane-1-carboxylate (ACC) deaminase, which regulates the level of stress hormone ethylene in the plant. Strains of Arthrobacter spp., Bacillus spp., and Pseudomonas spp. Have been reported to enhance plant growth through the production of ACC deaminase (Compant et al., 2019). This indicates the bi-directional relationship between plants and their microbial communities.
Edaphic factors such as pH, soil type, indigenous microflora, oxygen, nutrient, and light availability (Hacquard et al., 2015; Kaul et al., 2018) exert considerable impact on the developmental stage and physiological status of the host plant (Hacquard et al., 2015). A recent plant phytometer study with six plant species, across diverse edaphic conditions and land use gradient, indicates that indigenous soil microflora were the direct drivers of active bacterial communities (Vieira et al., 2020). The composition of the rhizosphere microbiome was strongly dictated by soil texture, water content, and soil type instead of plant properties and root exudates (Vieira et al., 2020). Another phytometer study was conducted on clonal oak saplings (Quercus robur L., clone DF159) under different field sites with similar climatic conditions. This study revealed that the effect of environmental factors was greater than the plant effect in shaping soil microbial communities. Similar microbial compositions were observed in sites with comparable pH, soil organic carbon, and C/N ratios (Habiyaremye et al., 2020). In contrast, similar rhizosphere communities were reported in three different fields having distinct physiochemical properties (Peiffer et al., 2013). Thus, plant-soil-microbe interaction is highly complex and their effect on the composition of the microbial community is determined by the interaction between them rather than each factor alone.
Core Microbiomes and Their Application Potential
A core microbiome is comprised of microbes that are recruited by a plant regardless of the environment (Figure 2). These core microbiomes contain key microbial taxa carrying essential functional genes for the plant host (Bergsma-Vlami et al., 2005; Hacquard et al., 2015; Vandenkoornhuyse et al., 2015; Astudillo-García et al., 2017; Naylor et al., 2017; Toju et al., 2018; Berg et al., 2020; Trivedi et al., 2020). The functional redundancy of microbes i.e., the coexistence of multiple taxa performing a particular biochemical function, allows for environmental variation without comprising plant host fitness (Lemanceau et al., 2017b; Louca et al., 2018). In addition, the network of interactions between organisms provides a buffer against disturbance by recruiting different microbial combinations to fulfil specific functions (Konopka et al., 2015). A dynamic functional community can be formed by focusing on the core microbiome instead of the highly complex native microbiota for further studies (Ramirez-Villacis et al., 2020; Durán et al., 2021).
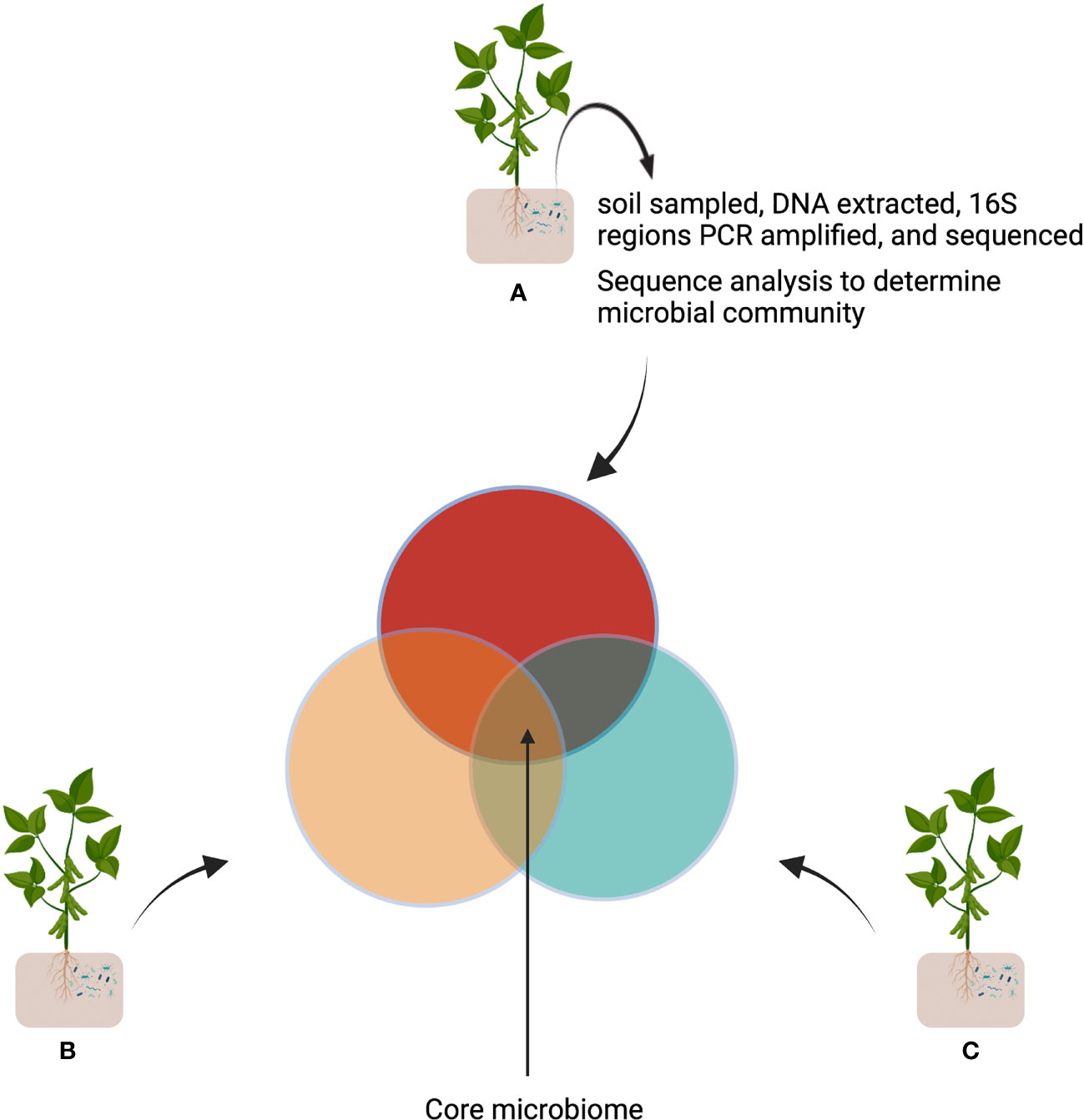
Figure 2 Determination of a core microbiome. The same plants are grown in independent locations (A-C). The soil is then sampled, sequenced, and the bacterial population (colored circles) are determined and compared (Venn diagram). The intersection represents the plants core microbiome. This figure was created with Biorender.com.
Recent advances in high throughput sequencing and bioinformatic tools have enabled the discovery of the core microbiomes of different crops. Marker gene amplicon sequencing has been widely used to study microbial association with different plant parts over a range of environmental conditions (Hacquard et al., 2015; Durán et al., 2021). Through co-occurrence network analysis of the resulting genomic data, it is possible to identify a core microbiome. It also explores the positive or negative relationship between members based on their occurrence or abundance (Rodriguez et al., 2019; Berg et al., 2020; Xue et al., 2022). Further, the positions of microbes in the network can indicate their importance within the microbial community. Highly interactive members of the core microbiome, which are called “hub” microbes, have been shown to have a strong influence in shaping the microbial communities of plant hosts (Agler et al., 2016; Muller et al., 2018; Trivedi et al., 2020).
Several studies have reported the taxonomy of core microbiomes in different crops (Table 1). Walters et al. (2018) found that seven bacterial operational taxonomic units (OTUs) were observed consistently in the maize rhizosphere at different ages and field conditions. All seven OTUs were taxonomically assigned to the phylum Proteobacteria with differences at the genus level. Likewise, the core microbiome of the citrus rhizosphere was identified through an extensive study of soil samples from twenty-three locations in eight citrus-producing countries across six continents (Xu et al., 2018). These studies show that the core microbiome can select for key members of the microbial community that can be screened in vitro for microbe-microbe interactions and putative functions (Lebeis, 2014).
Overall, there is general agreement in the literature that organisms do have strong associations with certain microbes. Many studies carry out their analysis at the genus level which does give a descriptive analysis of what organisms can be present. In some cases, more functional metatranscriptomic studies can provide more insight into which species are present as well as what genes are being expressed under a given set of conditions. Together these data are helping to develop hypotheses of how microbes might be affecting plant responses and are allowing work to be designed to ask key ecological questions regarding plant microbe interactions to be asked more directly.
Synthetic Community Approach in Sustainable Agriculture
Numerous studies have reported that beneficial microbes can be effectively used as inoculants for agricultural production since the 19th century (Bhattacharjee et al., 2008; Bhattacharyya et al., 2016; Mitter et al., 2016; Alori and Oluranti Babalola, 2018; Kaminsky et al., 2019; Qiu et al., 2019). Rhizobia-legume symbiosis and arbuscular mycorrhizal associations are examples of well-studied plant-microbe relationships that have been successfully used in agriculture (Bhattacharyya et al., 2016). Conventionally, beneficial microbes are selected based on in vitro screening for specific taxa with one or more PGP traits, such as nitrogen fixation, phosphorus solubilization, production of growth-regulating hormones, etc., with limited assessment under controlled environmental conditions (Glick, 2012; Choi et al., 2021).
Inconsistent production under field conditions is a major problem as inoculants often fail to compete with indigenous soil microbes under different climatic conditions, soil type and other environmental factors (Finkel et al., 2017; Baliyan et al., 2018; Qiu et al., 2019; de Souza et al., 2020). A successful inoculant must be able to compete with other microbes, efficiently colonize, and establish a stable association with plants throughout the growing season (Vessey, 2003; de Souza et al., 2020). Therefore, it is not surprising that current inoculants, which are formulated with pure isolates, can have problems with effectiveness. A SynCom could be a great alternative to overcome the problems associated with conventional inoculants as it can incorporate different microbial communities that can partly mimic the functional environment of those microorganisms (de Souza et al., 2019; Kaminsky et al., 2019).
The SynCom approach has become a promising technology as it integrates the concept of microbial ecology and genetics. A SynCom can be constructed using either a top-down approach or a bottom-up approach (Großkopf and Soyer, 2014). The top-down approach focuses on functional definition for a community to characterize its structure and dynamics in details. (Toju et al. 2020) applied the functional core microbiome concept to discover the best combinations of species/strains that potentially maximize functionality at the community/ecosystem level. This method produces communities with natural representation and high reproducibility while lowering the chances of missing important species. However, the effectiveness is dependent on the ability to accurately measure species diversity in a complex community. The bottom-up approach identifies common interaction patterns and processes among species. Paredes et al. (2018) used binary-association assays to design a SynCom for Arabidopsis thaliana that led to predictable plant phenotypes. Even though it facilitates establishing causality, it requires technological advances to manage high complex communities and increases the chances of missing important community members. Recently (Kehe et al. 2019) introduced a microfluidic droplet-based platform, the kChip, to automatically construct SynComs with all possible microbe combinations using a set of species. This has made the SynCom approach more efficient and viable for large scale studies but has limitations that may make it difficult to replicate for field trials
An effective SynCom can be produced by identifying functional communities through a top-down approach and then applying the bottom-up approach to study the interactions between the members of those communities. Genomic information and gene expression profiles could be used to select the microbes with beneficial functional traits or metabolic capability to design the best microbial combination for the microbial consortia (Toju et al., 2018; de Souza et al., 2020). Since multiple genes are responsible for important traits, such as colonization efficiency, and prevalence, genomic analysis for multiple markers may be key to identifying relevant microbes (de Souza et al., 2016; Cole et al., 2017; Levy et al., 2018; de Souza et al., 2019; de Souza et al., 2020). Computational tools can be used to screen for beneficial microbial candidates from existing genomic datasets, which would be less laborious than traditional methods (Finkel et al., 2017). Then, the SynCom could be constructed using a bottom-up approach by addition, elimination, or substitution at the strain level (Vorholt et al., 2017; Liu et al., 2019).
An extensive microbial culture collection is essential to building a SynCom since it is comprised of culturable microbes (Finkel et al., 2017; Vorholt et al., 2017; de Souza et al., 2020; Choi et al., 2021). The SynCom approach is initiated from the isolation of microbial cultures from the natural ecosystem and then formulated through manipulations of the selected microbiota to perform the desired functions for the host plants (de Souza et al., 2016). Since nearly 99% of bacteria are unculturable, novel approaches are necessary to generate extensive microbial collection. One approach is to use metagenomic analysis to identify appropriate media and culture conditions (Oberhardt et al., 2015). Also, high-throughput bacterial cultivation methods, such as the limiting dilution method (Zhang et al., 2019; Zhang et al., 2021), cell sorting (Bai et al., 2015), and colony picking (Armanhi et al., 2018) provide potential solutions for capturing diverse bacterial species on a large scale (Liu et al., 2019).
The effectiveness of SynComs can be quantitatively and qualitatively assessed with plant hosts under controlled environments using different axenic systems such as agar-based (highly artificial and uniformly controlled), clay-based (mimic soil), and FlowPot (autoclaved and washed soil) systems (Bai et al., 2015; Castrillo et al., 2017; Liu et al., 2018; Paredes et al., 2018; Finkel et al., 2019; Zhang et al., 2019). Axenic systems allow for detailed investigations of its components under controlled and reproducible conditions, which facilitate the establishment of causal links between genotypes and phenotypes. Changes can also be made at the functional level by removing or adding specific functions via gene expression (Liu et al., 2019). Further, the consequences of biotic or abiotic perturbations can be monitored at all levels (Liu et al., 2018; Liu et al., 2019; Melnyk et al., 2019).
Finally, an efficient SynCom could be tested under real field conditions to offset the limitations of the traditional approach. Assessment of a SynCom on plant phenotypic traits could be done through high-throughput phenotyping technologies as they offer multiple advantages such as automated, non-destructive and dynamic monitoring of morphological and physiological traits related to growth, yield, and performance throughout their entire lifecycle (Rouphael et al., 2018). This would facilitate an effective SynCom with more compatible, efficient, and adaptable microbes (Hart et al., 2018; Choi et al., 2021).
Current Approaches in SynCom Application
SynCom approaches have been used in experimental ecology and evolution studies to understand ecological interactions as well as ecological processes (Castrillo et al., 2017; Finkel et al., 2017; Cairns et al., 2018; Levy et al., 2018; Teixeira et al., 2021). The SynCom approach started being used to test evolutionary interactions in plant-microbe studies. Then, the focus has shifted towards the improvement of plant growth and production.
Several studies have been conducted in the model plant A. thaliana as well as agricultural crops – maize, soybean, sorghum, and tomato – to understand plant-microbe interactions using SynComs under controlled environments (Table 2). Bodenhausen et al. (2014) showed that host genotype influences the phyllosphere community composition and abundance using fifty-five A. thaliana plant mutants inoculated with a SynCom. Castrillo et al. (2017) studied the effect of plant Pi stress response on the A. thaliana immune system function and microbiome assembly a SynCom composed of thirty-five members. Niu et al. (2017) constructed a simplified seven-species SynCom from microbes associated with maize root to investigate the dynamics of root colonization, interspecies interactions, and the role of each member in the community. The SynCom approach has also been used to examine the role of specialized metabolites on the colonization of bacteria in the A. thaliana rhizosphere (Voges et al., 2019). In another SynCom study, it was reported that root colonization was regulated by microbe-associated molecular patterns (MAMPs) -triggered immunity (Teixeira et al., 2021). Thus, SynComs can effectively be used to explore plant-microbe interactions, which must be considered when using microbes in large-scale agricultural applications.
Pathogens are a major threat in agriculture as they can lead to complete yield loss. Several studies have reported that SynComs can be effectively used to suppress pathogenic organisms while improving the crop performance (Santhanam et al., 2015; De Vrieze et al., 2018; Santhanam et al., 2019; Ali et al., 2021; Li et al., 2021). Li et al. (2021) constructed two SynComs by adding both high and low abundance bacteria isolated from diseased plants. Results indicated that high abundance bacteria protected host through plant growth promotion and inhibition of the pathogenic fungus, while low abundance bacteria controlled diseases by enhancing plant induced systemic resistance. It is important to note that SynComs showed a superior effect on disease suppression and growth promotion compared to the mono-inoculated plants (Ali et al., 2021; Li et al., 2021). Synergistic interactions between the members of SynComs facilitate improved plant protection as well as growth.
Regardless of other benefits, crop productivity is always a prime concern. Inoculation with a SynCom constructed from sugarcane-associated microbes increased the biomass of maize plants compared to the uninoculated controls (Armanhi et al., 2018). The same SynCom also improved drought tolerance and reduced yield loss in maize (Armanhi et al., 2021). Another SynCom, composed of desiccation-tolerant bacteria, showed increased plant growth parameters such as dry weight of shoot and root, plant height, and plant diameter when compared with either non-inoculated control or mono-inoculated treatments (Molina-Romero et al., 2017). Further, Wang et al. (2021) reported that functionally assembled SynComs improved soybean yield up to 36% under field conditions. Thus, recent studies suggest that SynCom could be effectively incorporated in agriculture to enhance crop yield.
The above studies reiterate that the SynCom approach is an effective tool for exploring plant-microbe interaction and microbe-microbe interaction. Even though most of the SynCom experiments were conducted under controlled conditions, it gives a valuable information about the interaction between each member in the community assemblage and identifying keystone members. For example, Niu et al. (2017) reported that the removal of one species from the SynCom led to drastic changes in community composition. The simplicity of this approach allows repeated experiments to ensure reproducibility which could prevent the problems in the future large-scale application. Recently, the interest in the SynCom approach has been focused on improving crop yield by extending the research in the greenhouse to field conditions which is an important milestone of the SynCom application. Despite there being a long way to go, the current application of SynCom indicates the possibilities to be incorporated into the large-scale application in the near future.
Conclusions and Future Perspectives-Are Synthetic Microbial Communities a Way Forward?
Interest in rhizosphere research has continually grown exponentially since 1994 to the present day,with the term “plant microbiome” first being used as a key word in publications in 2011 (Oresnik et al., 2016). The application of microorganisms in agriculture has emerged as a promising, sustainable approach to improve crop production as the microbiome play an essential role in several plant processes and soil fertility. Poor performance of microbial inoculants is a challenge in developing stable inoculants for agriculture. However, recent advances in high-throughput sequencing technologies create an opportunity to identify the core microbes associated with plants and facilitate the formation of effective SynComs.
Although the SynCom approach is a promising technology, several challenges must be addressed before it can be used in large-scale applications. Designing SynComs with hundreds of microbes is not practical due to a lack of industrial technologies and difficulties in handling them. This issue can be addressed by constructing SynComs with microbes that have multiple beneficial traits and synergistic interactions. Nevertheless, keeping multiple species is challenging as medium composition plays a critical role in population dynamics. Stochastic events can also cause fluctuations of population in mixed communities. Therefore, it will be necessary to monitor the population dynamics of a SynCom to ensure all members are functioning and having enough viable cell counts.
Prediction of SynCom interaction with host plant and soil microbes in natural environment is challenging due to the influence of the native microbes. Thus, maintaining the long-term stability of SynCom is another task to be attained as introduced inoculants are exposed to an environment with competitive species. The SynCom may change over time due to genomic evolution and horizontal gene transfer. In addition, some microbes show differential expression with varying environmental conditions. Sustaining the community robustness and function over a timescale is a crucial aspect. Biosensors and marker gene technologies could be incorporated to trace the interaction and behaviors of introduced SynCom.
The ability to genetically modify or to engineer both host plants as well as microbes has increased dramatically over the last five years. Whereas in the past, there were relatively few microbial genetic model systems (Miller, 1991), the ability to sequence genomes as well as tools such as CRISPR/Cas9 have allowed the genetic modification of many diverse bacteria (Shelake et al., 2019; Rubin et al., 2021). With respect to the development of a SynCom, this can lead to modifying certain community member(s) to allow desired interactions with target crops. Recently it has been shown that endophytic bacteria could be engineered to contain inducible nitrogenase activity (Ryu et al., 2020), which in principle can be combined with plants which have been modified to produce signals for targeted regulation of bacterial genes (Geddes et al., 2019).
So far, most studies have been conducted in controlled systems which are opposite to diverse natural environments. Assessing their stability and plant performance under field conditions is the ultimate target. Production of the required amount of SynComs for large-scale application is also problematic as it would require additional technologies like bioreactors. Determining the effective method of application, whether it is liquid application or seed coating, is another hurdle to be overcome. Extensive field studies with a range of climatic conditions are required to ensure the activity of the applied inoculants.
The development of an effective SynCom is a novel opportunity to improve sustainable food production. It is clear from the literature that microbes are capable of positively affecting plant health and productivity. However, the complexity of dealing with multiple microorganisms that are interacting with field crops with real world climate is challenging. It has been previously pointed out that these types of technologies would have to be transformative to growers for them to be adopted (Oresnik et al., 2016). In the short term, the SynCom approach is an opportunity to delve into the intricacies of plant-microbe interactions as well as microbial ecology. These advances are crucial to better understand how microbes can be manipulated to deliver desired traits to plants. The complex SynComs constructed are clearly important for an academic understanding but are not a pragmatic agronomic solution. The lessons that will be learned from these approaches however can provide valuable information to either produce SynComs that contain fewer microbes, or to develop SynComs that can work synergistically with the native microbial communities already present in the field. The utilization of this technology will require a long-term multidisciplinary approach that includes microbiologists, plant biologists, agronomists, as well as fermentation specialists to facilitate the delivery of a working system. Even though the application of SynCom for crop production is in its infant stage, advances in technologies are occurring at a remarkable pace and it is an approach that has the potential to deliver a solution in our quest toward sustainable agricultural.
Author Contributions
AS, PO, and IO conceived and wrote the manuscript. All authors contributed to the article and approved the submitted version. All authors contributed to the article and approved the submitted version.
Funding
This work was supported by a grant from the Canadian Agriculture Partnership and Manitoba Pulse and Soybean Growers (grant number 1000227246) to IO.
Conflict of Interest
The authors declare that the research was conducted in the absence of any commercial or financial relationships that could be construed as a potential conflict of interest.
Publisher’s Note
All claims expressed in this article are solely those of the authors and do not necessarily represent those of their affiliated organizations, or those of the publisher, the editors and the reviewers. Any product that may be evaluated in this article, or claim that may be made by its manufacturer, is not guaranteed or endorsed by the publisher.
References
Abbasi S., Spor A., Sadeghi A., Safaie N. (2021). Streptomyces Strains Modulate Dynamics of Soil Bacterial Communities and Their Efficacy in Disease Suppression Caused by Phytophthora Capsici. Sci. Rep. 11, 9317. doi: 10.1038/s41598-021-88495-y
Agler M. T., Ruhe J., Kroll S., Morhenn C., Kim S.-T., Weigel D., et al. (2016). Microbial Hub Taxa Link Host and Abiotic Factors to Plant Microbiome Variation. PloS Biol. 14 (1), e1002352. doi: 10.1371/journal.pbio.1002352
Aira M., Gómez-Brandón M., Lazcano C., Bååth E., Domínguez J. (2010). Plant Genotype Strongly Modifies the Structure and Growth of Maize Rhizosphere Microbial Communities. Soil Biol. Biochem. 42, 2276–2281. doi: 10.1016/j.soilbio.2010.08.029
Ali M., Ahmad Z., Ashraf M. F., Dong W. (2021). Maize Endophytic Microbial-Communities Revealed by Removing PCR and 16S rRNA Sequencing and Their Synthetic Applications to Suppress Maize Banded Leaf and Sheath Blight. Microbiol. Res. 242, 126639. doi: 10.1016/j.micres.2020.126639
Alori E. T., Oluranti Babalola O. (2018). Microbial Inoculants for Improving Crop Quality and Human Health in Africa. Front. Microbiol. 9. doi: 10.3389/fmicb.2018.02213
Andreote F. D., Gumiere T., Durrer A. (2014). Exploring Interactions of Plant Microbiomes. Sci. Agric. 71 (6), 528–539. doi: 10.1590/0103-9016-2014-0195
Arif I., Batool M., Schenk P. M. (2020). Plant Microbiome Engineering: Expected Benefits for Improved Crop Growth and Resilience. Trends Biotechnol. 38 (12), 1385–1396. doi: 10.1016/j.tibtech.2020.04.015
Armanhi J. S. L., de Souza R. S. C., Damasceno de N. B., de Araújo L. M., Imperial J., Arruda P. (2018). A Community-Based Culture Collection for Targeting Novel Plant Growth-Promoting Bacteria From the Sugarcane Microbiome. Front. Plant Sci. 8. doi: 10.3389/fpls.2017.02191
Armanhi J. S. L., de Souza R. S. C., Biazotti B. B., Yassitepe de J. E. C. T., Arruda P., et al. (2021). Modulating Drought Stress Response of Maize by a Synthetic Bacterial Community. Front. Microbiol. 12. doi: 10.3389/fmicb.2021.747541
Astudillo-García C., Bell J. J., Webster N. S., Glasl B., Jompa J., Montoya J. M., et al. (2017). Evaluating the Core Microbiota in Complex Communities: A Systematic Investigation. Environ. Microbiol. 19 (4), 1450–1462. doi: 10.1111/1462-2920.13647
Backer R., Rokem J. S., Ilangumaran G., Lamont J., Praslickova D., Ricci E., et al. (2018). Plant Growth-Promoting Rhizobacteria: Context, Mechanisms of Action, and Roadmap to Commercialization of Biostimulants for Sustainable Agriculture. Front. Plant Sci. 9. doi: 10.3389/fpls.2018.01473
Bai Y., Müller D. B., Srinivas G., Garrido-Oter R., Potthoff E., Rott M., et al. (2015). Functional Overlap of the Arabidopsis Leaf and Root Microbiota. Nature 528, 364–369. doi: 10.1038/nature16192
Baliyan N., Dheeman S., Dinesh , Maheshwari K., Dubey R. C., Vineet , et al. (2018). Rhizobacteria Isolated Under Field First Strategy Improved Chickpea Growth and Productivity. J. Environ. Sustain. 1, 461–469. doi: 10.1007/s42398-018-00042-0
Banerjee S., Schlaeppi K., Heijden M. G. A. (2018). Keystone Taxa as Drivers of Microbiome Structure and Functioning. Nat. Rev. Microbiol. 16, 567–576. doi: 10.1038/s41579-018-0024-1
Berg G., Rybakova D., Fischer D., Cernava T., Vergès M.-C. C., Charles T., et al. (2020). Microbiome Definition Re-Visited: Old Concepts and New Challenges. Microbiome 8, 103. doi: 10.1186/s40168-020-00875-0
Bergsma-Vlami M., Prins M. E., Raaijmakers J. M. (2005). Influence of Plant Species on Population Dynamics, Genotypic Diversity and Antibiotic Production in the Rhizosphere by Indigenous Pseudomonas Spp. FEMS Microbiol. Ecol. 52, 59–69. doi: 10.1016/j.femsec.2004.10.007
Berlanas C., Berbegal M., Elena G., Laidani M., Cibriain J. F., Sagües A., et al. (2019). The Fungal and Bacterial Rhizosphere Microbiome Associated With Grapevine Rootstock Genotypes in Mature and Young Vineyards. Front. Microbiol. 10. doi: 10.3389/fmicb.2019.01142
Bhattacharjee R. B., Singh A., Mukhopadhyay S. N. (2008). Use of Nitrogen-Fixing Bacteria as Biofertiliser for non-Legumes: Prospects and Challenges. Appl. Microbiol. Biotechnol. 80, 199–209. doi: 10.1007/s00253-008-1567-2
Bhattacharyya P. N., Goswami M. P., Bhattacharyya L. H. (2016). Perspective of Beneficial Microbes in Agriculture Under Changing Climatic Scenario: A Review. J. Phytol. 8, 26–41. doi: 10.19071/jp.2016.v8.3022
Bodenhausen N., Bortfeld-Miller M., Ackermann M., Vorholt J. A. (2014). A Synthetic Community Approach Reveals Plant Genotypes Affecting the Phyllosphere Microbiota. PLoS Genet. 10 (4), e1004283. doi: 10.1371/journal.pgen.1004283
Bulgarelli D., Garrido-Oter R., Münch P. C., Weiman A., Dröge J., Pan Y., et al. (2015). Structure and Function of the Bacterial Root Microbiota in Wild and Domesticated Barley. Cell Host Microbe 17, 392–403. doi: 10.1016/j.chom.2015.01.011
Cairns J., Jokela R., Hultman J., Tamminen M., Virta M., Hiltunen T., et al. (2018). Construction and Characterization of Synthetic Bacterial Community for Experimental Ecology and Evolution. Front. Genet. 6. doi: 10.3389/fgene.2018.00312
Canarini A., Kaiser C., Merchant A., Richter A., Wanek W. (2019). Root Exudation of Primary Metabolites: Mechanisms and Their Roles in Plant Responses to Environmental Stimuli. Front. Plant Sci. 10. doi: 10.3389/fpls.2019.00157
Castrillo G., Pereira P. J., Teixeira L., Herrera Paredes S., Law T. F., de Lorenzo L., et al. (2017). Root Microbiota Drive Direct Integration of Phosphate Stress and Immunity. Nature 543, 513–518. doi: 10.1038/nature21417
Chai Y. N., Ge Y., Stoerger V., Schachtman D. P. (2021). High-Resolution Phenotyping of Sorghum Genotypic and Phenotypic Responses to Low Nitrogen and Synthetic Microbial Communities. Plant Cell and Environment 44, 1611–26. doi: 10.1186/s12870-021-03298-7
Chaudhary P., Chaudhary A., Parveen H., Rani A., Kumar G., Kumar R., et al. (2021). Impact of Nanophos in Agriculture to Improve Functional Bacterial Community and Crop Productivity. BMC Plant Biol. 21, 512. doi: 10.1186/s12870-021-03298-7
Chauhan P. S., Chaudhry V., Mishra S., Nautiyal C. S. (2011). Uncultured Bacterial Diversity in Tropical Maize (Zea Mays L.).Rhizosphere. J. Basic Microbiol. 51 (1), 15–32. doi: 10.1002/jobm.201000171
Chodkowski J. L., Shade A. (2017). A Synthetic Community System for Probing Microbial Interactions Driven by Exometabolites. mSystems 2 (6), e00129–e00117. doi: 10.1128/mSystems.00129-17
Choi K., Khan R., Lee S. W. (2021). Dissection of Plant Microbiota and Plant-Microbiome Interactions. J. Microbiol. 59, 281–291. doi: 10.1007/s12275-021-0619-5
Cole B. J., Feltcher M. E., Waters R. J., Wetmore K. M., Mucyn T. S., Ryan E. M., et al. (2017). Genome-Wide Identification of Bacterial Plant Colonization Genes. PloS Biol. 15 (9), e2002860. doi: 10.1371/journal.pbio.2002860
Compant S., Samad A., Faist H., Sessitsch A. (2019). A Review on the Plant Microbiome: Ecology, Functions, and Emerging Trends in Microbial Application. J. Adv. Res. 19, 29–37. doi: 10.1016/j.jare.2019.03.004
Crecchio C., Mimmo T., Bulgarelli D., Pertot I., Pii Y., Perazzolli M., et al. (2018). “Beneficial Soil Microbiome for Sustainable Agriculture Production,” in Sustainable Agriculture Reviews (Switzerland: Springer Nature), 443–479. doi: 10.1007/978-3-319-94232-2_9
de Souza R. S. C., Armanhi J. S. L., Arruda P. (2020). From Microbiome to Traits: Designing Synthetic Microbial Communities for Improved Crop Resiliency. Front. Plant Sci. 11. doi: 10.3389/fpls.2020.01179
de Souza R. S. C. (2016). Unlocking the Bacterial and Fungal Communities Assemblages of Sugarcane Microbiome. Sci. Rep. 6, 28774. doi: 10.1038/srep28774
de Souza R. S. C., Armanhi Jaderson S. L., Damasceno de N. B., Imperial J., Arruda P. (2019). Genome Sequences of a Plant Beneficial Synthetic Bacterial Community Reveal Genetic Features for Successful Plant Colonization. Front. Microbiol. 10. doi: 10.3389/fmicb.2019.01779
de Vrieze M., Germanier F., Vuille N., Weisskopf L., et al. (2018). Combining Different Potato-Associated Pseudomonas Strains for Improved Biocontrol of Phytophthora Infestans. Front. Microbiol. 9. doi: 10.3389/fmicb.2018.02573
Dixon M. W. (2018). Chemical Fertilizer in Transformations in World Agriculture and the State System 1870 to Interwar Period. J. Agrar. Change 18 (4), 768–786. doi: 10.1111/joac.12259
Dudenhoffer J., Scheu S., Jousset A. (2016). Systemic Enrichment of Antifungal Traits in the Rhizosphere Microbiome After Pathogen Attack. J. Ecol. 104, 1566–1575. doi: 10.1111/1365-2745.12626
Durán P., Reinstädler A., Rajakrut A. L., Hashimoto M., Garrido-Oter R., Schulze-Lefert P., et al. (2021). A Fungal Powdery Mildew Pathogen Induces Extensive Local and Marginal Systemic Changes in the Arabidopsis Thaliana Microbiota. Environ. Microbiol 23, 6292–308. doi: 10.1111/1462-2920.15768
ELD Initiative (2015). Report for Policy and Decision Makers: Reaping Economic and Environmental Benefits From Sustainable Land Management (Bonn, Germany: Initiative and Deutsche Gesellschaft für Internationale Zusammenarbeit (GIZ) GmbH ).
Fierer N. (2017). Embracing the Unknown: Disentangling the Complexities of the Soil Microbiome. Nat. Rev. Microbiol. 15, 579–590. doi: 10.1038/nrmicro.2017.87
Figueiredo dos Santos L., Fernandes Souta J., de PaulaSoares C., Oliveira daRocha L., Luiza CarvalhoSantos M., Grativol C., et al. (2021). Insights Into the Structure and Role of Seed-Borne Bacteriome During Maize Germination. FEMS Microbiol Ecol. 97. doi: 10.1093/femsec/fiab024
Finkel O. M., Castrillo G., Paredes S. H., Salas González I., Dangl J. L. (2017). Understanding and Exploiting Plant Beneficial Microbes. Curr. Opin. Plant Biol. 38, 155–163. doi: 10.1016/J.PBI.2017.04.018
Finkel O. M., Salas-Gonzá lez I., Castrillo G., Spaepen S., Law T. F., Teixeira P. J. P.L., et al. (2019). The Effects of Soil Phosphorus Content on Plant Microbiota are Driven by the Plant Phosphate Starvation Response. PloS Biol. 17 (11), e3000534. doi: 10.1371/journal.pbio.3000534
Geddes B. A., Paramasivan P., Joffrin A., Thompson A. L., Christensen K., Jorrin B., et al. (2019). Engineering Transkingdom Signalling in Plants to Control Gene Expression in Rhizosphere Bacteria. Nat. Commun. 10, 3430. doi: 10.1038/s41467-019-10882-x
Glick B. R. (2012). Plant Growth-Promoting Bacteria: Mechanisms and Applications. Scientifica 2012, 963401. doi: 10.6064/2012/963401
Großkopf T., Soyer O. S. (2014). Synthetic Microbial Communities. Curr. Opin. Microbiol. 18, 72–77. doi: 10.1016/j.mib.2014.02.002
Habiyaremye J., de D., Goldmann K., Reitz T., Herrmann S., Buscot F. (2020). Tree Root Zone Microbiome: Exploring the Magnitude of Environmental Conditions and Host Tree Impact. Front. Microbiol. 11. doi: 10.3389/fmicb.2020.00749
Hacquard S., Garrido-Oter R., González A., Spaepen S., Ackermann G., Lebeis S., et al. (2015). Microbiota and Host Nutrition Across Plant and Animal Kingdoms. Cell Host Microbe 17, 603–616. doi: 10.1016/j.chom.2015.04.009
Haichar F. E. Z., Marol C., Berge O., Rangel-Castro J. I., Prosser J. I., Balesdent J., et al. (2008). Plant Host Habitat and Root Exudates Shape Soil Bacterial Community Structure. ISME J. 2, 1221–1230. doi: 10.1038/ismej.2008.80
Hamonts K., Trivedi P., Garg A., Janitz C., Grinyer J., Holford P., et al. (2018). Field Study Reveals Core Plant Microbiota and Relative Importance of Their Drivers. Environ. Microbiol. 20 (1), 124–140. doi: 10.1111/1462-2920.14031
Hart M. M., Antunes P. M., Chaudhary V. B., Abbott L. K. (2018). Fungal Inoculants in the Field: Is the Reward Greater Than the Risk? Funct. Ecol. 32, 126–135. doi: 10.1111/1365-2435.12976
Hartman K., Tringe S. G. (2019). Interactions Between Plants and Soil Shaping the Root Microbiome Under Abiotic Stress. Biochem. J. 476, 2705–2724. doi: 10.1042/BCJ20180615
Hawkes C., Deangelis K. M., Firestone M. K. (2007). “Root Interactions With Soil Microbial Communities and Processes,” in The Rhizosphere: An Ecological Perspective (New York: Elsevier), 1–29.
Hawkins J. P., Oresnik I. J. (2022). The Rhizobium-Legume Symbiosis: Co-Opting Successful Stress Management. Front. Plant Sci. 12. doi: 10.3389/fpls.2021.796045
Inceoglu O., Salles J. F., van Overbeek L., Dirk Van Elsas J., et al. (2010). Effects of Plant Genotype and Growth Stage on the Betaproteobacterial Communities Associated With Different Potato Cultivars in Two Fields. Appl. Environ. Microbiol. 76 (11), 3675–3684. doi: 10.1128/AEM.00040-10
Joshi G., Kumar V., Brahmachari S. K. (2021). Screening and Identification of Novel Halotolerant Bacterial Strains and Assessment for Insoluble Phosphate Solubilization and IAA Production. Bull. Natl. Res. Cent. 45, 83. doi: 10.1186/s42269-021-00545-7
Kaminsky L. M., Trexler R., Malik R. J., Hockett K. L., Bell T. H. (2019). The Inherent Conflicts in Developing Soil Microbial Inoculants. Trends Biotechnol. 37 (2), 140–151. doi: 10.1016/j.tibtech.2018.11.011
Kaul S., Gupta S., Sharma T., Dhar M. K. (2018). “Unfolding the Role of Rhizomicrobiome Toward Sustainable Agriculture,” in Root Biology (Cham: Springer), 341–365. doi: 10.1007/978-3-319-75910-4_14
Kehe J., Kulesa A., Ortiz A., Ackerman C. M., Gowtham Thakku S., Sellers D, et al. (2019). Massively Parallel Screening of Synthetic Microbial Communities. Acad Sci USA 116, 12804–09. doi: 10.1073/pnas.1900102116
Konopka A., Lindemann S., Fredrickson J. (2015). Dynamics in Microbial Communities: Unraveling Mechanisms to Identify Principles. ISME J. 9, 1488–1495. doi: 10.1038/ismej.2014.251
Korenblum E., Dong Y., Szymanski J., Panda S., Jozwiak A., Massalha H., et al. (2020). Rhizosphere Microbiome Mediates Systemic Root Metabolite Exudation by Root-to-Root Signaling. Proc. Natl. Acad. Sci. U.S.A. 117 (7), 3874–3883. doi: 10.1073/pnas.1912130117
Lebeis S. L. (2014). The Potential for Give and Take in Plant-Microbiome Relationships. Front. Plant Sci. 5. doi: 10.3389/fpls.2014.00287
Lebeis S. L., Paredes S. H., Lundberg D. S., Breakfield N., Gehring J., McDonald M., et al. (1979). Salicylic Acid Modulates Colonization of the Root Microbiome by Specific Bacterial Taxa. Science 349, 860–3.
Lee S. M., Kong H. G., Song G. C., Ryu C. M. (2021). Disruption of Firmicutes and Actinobacteria Abundance in Tomato Rhizosphere Causes the Incidence of Bacterial Wilt Disease. ISME J 15, 330–47. doi: 10.1038/s41396-020-00785-x
Lemanceau P., Blouin M., Muller D., Moënne-Loccoz Y. (2017b). Let the Core Microbiota be Functional. Trends Plant Sci. 22 (7), 583–595. doi: 10.1016/j.tplants.2017.04.008
Lemanceau P., Barret M., Mazurier S., Mondy S., Pivato B., Fort T., et al. (2017a). “Plant Communication With Associated Microbiota in the Spermosphere, Rhizosphere and Phyllosphere,” in Adv Bot Res (Cambridge: Academic Press Inc.), 101–133. doi: 10.1016/bs.abr.2016.10.007
Levy A., Salas Gonzalez I., Mittelviefhaus M., Clingenpeel S., Herrera Paredes S., Miao J., et al. (2018). Genomic Features of Bacterial Adaptation to Plants. Nat. Genet. 50, 138–150. doi: 10.1038/s41588-017-0012-9
Li Z., Bai X., Jiao S., Li Y., Li P., Yang Y., et al. (2021). A Simplified Synthetic Community Rescues Astragalus Mongholicus From Root Rot Disease by Activating Plant-Induced Systemic Resistance. Microbiome 9 (217), 217–20. doi: 10.1186/s40168-021-01169-9
Liu Z., Beskrovnaya P., Melnyk R. A., Hossain S. S., Khorasani S., O’Sullivan L. R., et al. (2018). A Genome-Wide Screen Identifies Genes in Rhizosphere-Associated Pseudomonas Required to Evade Plant Defenses. mBio 9 (6), e00433–e00418. doi: 10.1128/mBio
Liu Y.-X., Qin Y., Bai Y. (2019). Reductionist Synthetic Community Approaches in Root Microbiome Research. Curr. Opin. Microbiol. 49, 97–102. doi: 10.1016/j.mib.2019.10.010
Louca S., Polz M. F., Mazel F., Albright M. B.N., Huber J. A., O’Connor M. I., et al. (2018). Function and Functional Redundancy in Microbial Systems. Nat. Ecol. Evol. 2, 936–943. doi: 10.1038/s41559-018-0519-1
Lundberg D. S., Lebeis S. L., Paredes H., Yourstone S., Gehring J., Malfatti S., et al. (2012). Defining the Core Arabidopsis thaliana Root Microbiome. Nature 488, 86–95. doi: 10.1038/nature11237
Lynch J. M., Whipps J. M. (1990). Substrate Flow in the Rhizosphere. Plant Soil 129, 1–10. doi: 10.1007/BF00011685
McCarty N. S., Ledesma-Amaro R. (2019). Synthetic Biology Tools to Engineer Microbial Communities for Biotechnology. Trends Biotechnol. 37 (2), 181–197. doi: 10.1016/j.tibtech.2018.11.002
McNear D. H. (2013). The Rhizosphere-Roots, Soil and Everything in Between. Nat. Educ. Knowl. 4 (3), 1–15.
Meena K. K., Sorty A. M., Bitla U. M., Choudhary K., Gupta P., Pareek A., et al. (2017a). Abiotic Stress Responses and Microbe-Mediated Mitigation in Plants: The Omics Strategies. Front. Plant Sci. 8. doi: 10.3389/fpls.2017.00172
Meena V. S., Meena S. K., Verma J. P., Kumar A., Aeron A., Mishra P. K., et al. (2017b). Plant Beneficial Rhizospheric Microorganism (PBRM) Strategies to Improve Nutrients Use Efficiency: A Review. Ecol. Eng. 107, 8–32. doi: 10.1016/j.ecoleng.2017.06.058
Melillo E. D. (2012). The First Green Revolution: Debt Peonage and the Making of the Nitrogen Fertilizer Trade 1840–1930. Am. Hist. Rev. 117, 1028–1060. doi: 10.1093/ahr/117.4.1028
Melnyk R. A., Hossain S. S., Haney C. H. (2019). Convergent Gain and Loss of Genomic Islands Drive Lifestyle Changes in Plant-Associated Pseudomonas. ISME J. 13, 1575–1588. doi: 10.1038/s41396-019-0372-5
Micallef S. A., Channer S., Shiaris M. P., Colón-Carmona A. (2009). Plant Age and Genotype Impact the Progression of Bacterial Community Succession in the Arabidopsis Rhizosphere. Plant Signal. Behav. 4 (8), 777–780. doi: 10.1093/jxb/erp053
Mitter B., Pfaffenbichler N., Sessitsch A. (2016). Plant-Microbe Partnerships in 2020. Microb. Biotechnol. 9 (5), 635–640. doi: 10.1111/1751-7915.12382
Moe L. A. (2013). Amino Acids in the Rhizosphere: From Plants to Microbes. Am. J. Bot. 100 (9), 1692–1705. doi: 10.3732/AJB.1300033
Molina-Romero D., Baez A., Quintero-Herná ndez V., Castañeda-Lucio M., Fuentes-Ramírez L. E., Bustillos-Cristales del M. R., et al. (2017). Compatible Bacterial Mixture, Tolerant to Desiccation, Improves Maize Plant Growth. PLoS One 12 (11), e0187913. doi: 10.1371/journal.pone.0187913
Muller E. E. L., Faust K., Widder S., Herold M., Martínez Arbas S., Wilmes P. (2018). Using Metabolic Networks to Resolve Ecological Properties of Microbiomes. Curr. Opin. Syst. Biol. 8, 73–80. doi: 10.1016/j.coisb.2017.12.004
Naylor D., Degraaf S., Purdom E., Coleman-Derr D. (2017). Drought and Host Selection Influence Bacterial Community Dynamics in the Grass Root Microbiome. ISME J. 11, 2691–2704. doi: 10.1038/ismej.2017.118
Niu B., Paulson J. N., Zheng X., Kolter R. (2017). Simplified and Representative Bacterial Community of Maize Roots. Proc. Natl. Acad. Sci. U.S.A. 114, E2450–E2459. doi: 10.1073/pnas.1616148114
Oberhardt M. A., Zarecki R., Gronow S., Lang E., Klenk H.-P., Gophna U., et al. (2015). Harnessing the Landscape of Microbial Culture Media to Predict New Organism-Media Pairings. Nat. Commun. 6, 8493. doi: 10.1038/ncomms9493
Olanrewaju O. S., Glick B. R., Babalola O. O. (2017). Mechanisms of Action of Plant Growth Promoting Bacteria. World J. Microbiol. Biotechnol. 33, 197. doi: 10.1007/s11274-017-2364-9
Oresnik I. J., Mascarehnas L., Yost C. K. (2016). Does it Take a Community to Raise a Plant? A Summary or the Canadian Crop Microbiome Workshop. Can. J. Microbiol. 62 (11), 1–3. doi: 10.1139/cjm-2016-0108
Paredes S. H., Gao T., Law T. F., Finkel O. M., Mucyn T., Teixeira P. J.P.L., et al. (2018). Design of Synthetic Bacterial Communities for Predictable Plant Phenotypes. PloS Biol. 16 (2), e2003962. doi: 10.1371/journal.pbio.2003962
Peiffer J. A., Spor A., Koren O., Jin Z., Green Tringe S., Dangl J. L., et al. (2013). Diversity and Heritability of the Maize Rhizosphere Microbiome Under Field Conditions. Proc. Natl. Acad. Sci. U.S.A. 110 (16), 6548–6553. doi: 10.1073/pnas.1302837110
Pfeiffer S., Mitter B., Oswald A., Schloter-Hai B., Schloter M., Declerck S., et al. (2017). Rhizosphere Microbiomes of Potato Cultivated in the High Andes Show Stable and Dynamic Core Microbiomes With Different Responses to Plant Development. FEMS Microbiol. Ecol. 93, 1–12. doi: 10.1093/femsec/fiw242
Philippot L., Raaijmakers J. M., Lemanceau P., vanderPutten W. H. (2013). Going Back to the Roots: The Microbial Ecology of the Rhizosphere. Nat. Rev. Microbiol. 11, 789–799. doi: 10.1038/nrmicro3109
Pérez-Jaramillo J. E., de Hollander M., Ramírez C. A., Mendes R., Raaijmakers J. M., Carrión V. J. (2019). Deciphering Rhizosphere Microbiome Assembly of Wild and Modern Common Bean (Phaseolus Vulgaris) in Native and Agricultural Soils From Colombia. Microbiome 7, 114. doi: 10.1186/s40168-019-0727-1
Qiu Z., Egidi E., Liu H., Kaur S., Singh B. K. (2019). New Frontiers in Agriculture Productivity: Optimised Microbial Inoculants and in Situ Microbiome Engineering. Biotechnol. Adv. 37, 107371. doi: 10.1016/J.BIOTECHADV.2019.03.010
Ramirez-Villacis D. X., Finkel O. M., Salas-González I., Fitzpatrick C. R., Dangl J. L., Jones C. D., et al. (2020). Root Microbiome Modulates Plant Growth Promotion Induced by Low Doses of Glyphosate. mSphere 5 (4), e0048420. doi: 10.1128/mSphere
Rasmann S., Turlings T. C. J. (2016). Root Signals That Mediate Mutualistic Interactions in the Rhizosphere. Curr. Opin. Plant Biol. 32, 62–68. doi: 10.1016/j.pbi.2016.06.017
Reinhold-Hurek B., Bünger W., Burbano C. S., Sabale M., Hurek T. (2015). Roots Shaping Their Microbiome: Global Hotspots for Microbial Activity. Annu. Rev. Phytopathol. 53, 403–424. doi: 10.1146/annurev-phyto-082712-102342
Rodriguez P. A., Rothballer M., Chowdhury S. P., Nussbaumer T., Gutjahr C., Falter-Braun P. (2019). Systems Biology of Plant-Microbiome Interactions. Mol. Plant 12 (6), 804–821. doi: 10.1016/j.molp.2019.05.006
Rouphael Y., Spíchal L., Panzarová K., Casa R., Colla G. (2018). High-Throughput Plant Phenotyping for Developing Novel Biostimulants: From Lab to Field or From Field to Lab? Front. Plant Sci. 9. doi: 10.3389/fpls.2018.01197
Rubin B. E., Spencer D., Brady F. C., Alexander C.-C., Yue C., louAdair L. B., et al. (2021). Species-And Site-Specific Genome Editing in Complex Bacterial Communities. Nat. Microbiol. 7, 34–47. doi: 10.1038/s41564-021-01014-7
Ryu M. H., Zhang J., Toth T., Khokhani D., Geddes B. A., Mus F., et al. (2020). Control of Nitrogen Fixation in Bacteria That Associate With Cereals. Nat. Microbiol. 5, 314–330. doi: 10.1038/s41564-019-0631-2
Saleem R. A., Brunetti C., Khalid A., della Rocca G., Raio A., Emiliani G., et al. (2018). Drought Response of Mucuna Pruriens (L.) DC. Inoculated With ACC Deaminase and IAA Producing Rhizobacteria. PLoS One 13, e0191218. doi: 10.1371/journal.pone.0191218
Santhanam R., Luu V. T., Weinhold A., Goldberg J., Oh Y., Baldwin I. T., et al. (2015). Native Root-Associated Bacteria Rescue a Plant From a Sudden-Wilt Disease That Emerged During Continuous Cropping. Proc. Natl. Acad. Sci. USA 112, E5013–E5020. doi: 10.1073/pnas.1505765112
Santhanam R., Menezes R. C., Grabe V., Li D., Baldwin I. T., Groten K., et al. (2019). A Suite of Complementary Biocontrol Traits Allows a Native Consortium of Root-Associated Bacteria to Protect Their Host Plant From a Fungal Sudden-Wilt Disease. Mol. Ecol. 28 (5), 1154–1169. doi: 10.1111/MEC.15012
Schlatter D. C., Yin C., Hulbert S., Paulitz T. C. (2020). Core Rhizosphere Microbiomes of Dryland Wheat Are Influenced by Location and Land Use History. doi: 10.1128/AEM
Sharma A., Verma R. K. (2018). “Root–microbe Interactions: Understanding and Exploitation of Microbiome,” in Root Biology (Cham: Springer), 323–339. doi: 10.1007/978-3-319-75910-4_13
Shelake R. M., Pramanik D., Kim J.-Y. (2019). Exploration of Plant-Microbe Interactions for Sustainable Agriculture in CRISPR Era. Microorganisms 7, 269. doi: 10.3390/microorganisms7080269
Shi S., Nuccio E. E., Shi Z. J., He Z., Zhou J., Firestone M. K. (2016). The Interconnected Rhizosphere: High Network Complexity Dominates Rhizosphere Assemblages. Ecol. Lett. 19, 926–936. doi: 10.1111/ele.12630
Singh B. K., Trivedi P., Egidi E., Macdonald C. A., Delgado-Baquerizo M. (2020). Crop Microbiome and Sustainable Agriculture. Nat. Rev. Microbiol. 18 (11), 601–602. doi: 10.1038/s41579-020-00446-y
Teixeira P. J. P. L., Colaianni N. R., Law T. F., Conway J. M., Gilbert S., Li H., et al. (2021). Specific Modulation of the Root Immune System by a Community of Commensal Bacteria. Proc. Natl. Acad. Sci. U. S. A. 118 (16), e2100678118. doi: 10.1073/pnas.2100678118
Terrazas R. A., Giles C., Paterson E., Robertson-Albertyn S., Cesco S., Mimmo T., et al. (2016). Plant Microbiota Interactions as a Driver of the Mineral Turnover in the Rhizosphere. Adv. Appl. Microbiol. 95, 1–67. doi: 10.1016/bs.aambs.2016.03.001
Toju H., Peay K. G., Yamamichi M., Narisawa K., Hiruma K., Naito K., et al. (2018). Core Microbiomes for Sustainable Agroecosystems. Nat. Plants 4, 247–257. doi: 10.1038/s41477-018-0139-4
Toju H., Abe M. S., Ishii C., Hori Y., Fujita H., Fukuda S. (2020). Scoring Species for Synthetic Community Design: Network Analyses of Functional Core Microbiomes. Front Microbiol 11, 1361. doi: 10.3389/fmicb.2020.01361
Trivedi P., Leach J. E., Tringe S. G., Sa T., Singh B. K. (2020). Plant-Microbiome Interactions: From Community Assembly to Plant Health. Nat. Rev. Microbiol. 18, 607–621. doi: 10.1038/s41579-020-0412-1
Tsunoda T., van Dam N. M. (2017). Root Chemical Traits and Their Roles in Belowground Biotic Interactions. Pedobiologia 65, 58–67. doi: 10.1016/J.PEDOBI.2017.05.007
Vandenkoornhuyse P., Quaiser A., Duhamel M., le Van A., Dufresne A. (2015). The Importance of the Microbiome of the Plant Holobiont. New Phytol. 206, 196–1206. doi: 10.1111/nph.13312
van Overbeek L., van Elsas J. D. (2008). Effects of Plant Genotype and Growth Stage on the Structure of Bacterial Communities Associated With Potato (Solanum Tuberosum L.). FEMS Microbiol. Ecol. 64, 283–296. doi: 10.1111/j.1574-6941.2008.00469.x
Vessey J. K. (2003). Plant Growth Promoting Rhizobacteria as Biofertilizers. Plant Soil 255, 571–586. doi: 10.1023/A:1026037216893
Vieira S., Sikorski J., Dietz S., Katharina HerzSchrumpf M., Bruelheide H. (2020). Drivers of the Composition of Active Rhizosphere Bacterial Communities in Temperate Grasslands. ISME J. 14, 463–475. doi: 10.1038/s41396-019-0543-4
Vives-Peris V., de Ollas C., Gómez-Cadenas A., Pérez-Clemente R. M. (2020). Root Exudates: From Plant to Rhizosphere and Beyond. Plant Cell Rep. 39, 3–17. doi: 10.1007/s00299-019-02447-5
Voges M.J.E.E.E., Bai Y., Schulze-Lefert P., Sattely E. S. (2019). Plant-Derived Coumarins Shape the Composition of an Arabidopsis Synthetic Root Microbiome. Proc. Natl. Acad. Sci. U. S. A. 116 (25), 12558–12565. doi: 10.1073/pnas.1820691116
Vorholt J. A., Vogel C., Carlström C. I., Müller D. B. (2017). Establishing Causality: Opportunities of Synthetic Communities for Plant Microbiome Research. Cell Host Microbe 22 (2), 142–155. doi: 10.1016/j.chom.2017.07.004
Wagner M. R., Tang C., Salvato F., Clouse K. M., Bartlett A, Vintila S., et al. (2021). Microbe-Dependent Heterosis in Maize. Proc Natl Acad Sci USA 118, e2021965118. doi: 10.1073/pnas.2021965118
Wallenstein M. D. (2017). Managing and Manipulating the Rhizosphere Microbiome for Plant Health: A Systems Approach. Rhizosphere 3, 230–232. doi: 10.1016/j.rhisph.2017.04.004
Walters W. A., Jin Z., Youngblut N., Wallace J. G., Sutter J., Zhang W., et al. (2018). Large-Scale Replicated Field Study of Maize Rhizosphere Identifies Heritable Microbes. Proc. Natl. Acad. Sci. U.S.A. 115 (28), 7368–7373. doi: 10.1073/pnas.1800918115
Wang C., Li Y., Li M., Zhang K., Ma W., Zheng L., et al. (2021). Functional Assembly of Root-Associated Microbial Consortia Improves Nutrient Efficiency and Yield in Soybean. J. Integr. Plant Biol. 63 (6), 1021–1035. doi: 10.1111/jipb.13073
Xue P., Minasny B., McBratney A. B. (2022). Land-Use Affects Soil Microbial Co-Occurrence Networks and Their Putative Functions. Appl. Soil Ecol. 169, 104184. doi: 10.1016/j.apsoil.2021.104184
Xu J., Zhang Y., Zhang P., Trivedi P., Riera N., Wang Y., et al. (2018). The Structure and Function of the Global Citrus Rhizosphere Microbiome. Nat. Commun. 9 (1). doi: 10.1038/s41467-018-07343-2
Yasmin S., Zaka A., Imran A., Awais Zahid M., Yousaf S., Rasul G., et al. (2016). Plant Growth Promotion and Suppression of Bacterial Leaf Blight in Rice by Inoculated Bacteria. PLoS One 11, e0160688. doi: 10.1371/journal.pone.0160688
Zarraonaindia I., Owens S. M., Weisenhorn P., West K., Hampton-Marcell J., Lax S., et al. (2015). The Soil Microbiome Influences Grapevine-Associated Microbiota. mBio 6, e02527–14. doi: 10.1038/s41564-018-0129-3
Zhalnina K., Louie K. B., Hao Z., Mansoori N., Nunes DaRocha U., Shi S., et al. (2018). Dynamic Root Exudate Chemistry and Microbial Substrate Preferences Drive Patterns in Rhizosphere Microbial Community Assembly. Nat. Microbiol. 3, 470–480. doi: 10.1038/s41564-018-0129-3
Zhang J., Liu Y.-X., Zhang N., Hu B., Jin T. (2019). NRT1.1B is Associated With Root Microbiota Composition and Nitrogen Use in Field-Grown Rice. Nat. Biotechnol. 37, 676–684. doi: 10.1038/s41587-019-0104-4
Zhang X., Zhao C., Yu S., Jiang Z., Liu S., Wu Y., et al. (2020). Rhizosphere Microbial Community Structure is Selected by Habitat But Not Plant Species in Two Tropical Seagrass Beds. Front. Microbiol. 11. doi: 10.3389/fmicb.2020.00161
Zhang J., Liu Y.-X., Guo X., Qin Y., Garrido-Oter R., Schulze-Lefert P., et al. (2021). High-Throughput Cultivation and Identification of Bacteria From the Plant Root Microbiota. Nat. Protoc. 16, 988–1012. doi: 10.1038/s41596-020-00444-7
Keywords: plant-associated microbes, rhizosphere, plant growth-promoting traits, synthetic communities (SynCom), plant microbe interaction
Citation: Shayanthan A, Ordoñez PAC and Oresnik IJ (2022) The Role of Synthetic Microbial Communities (SynCom) in Sustainable Agriculture. Front. Agron. 4:896307. doi: 10.3389/fagro.2022.896307
Received: 14 March 2022; Accepted: 27 May 2022;
Published: 30 June 2022.
Edited by:
Rahul Mahadev Shelake, Gyeongsang National University, South KoreaReviewed by:
Hassan Etesami, University of Tehran, IranSopan Ganpatrao Wagh, Global Change Research Institute, Czech Academy of Sciences, Czechia
Copyright © 2022 Shayanthan, Ordoñez and Oresnik. This is an open-access article distributed under the terms of the Creative Commons Attribution License (CC BY). The use, distribution or reproduction in other forums is permitted, provided the original author(s) and the copyright owner(s) are credited and that the original publication in this journal is cited, in accordance with accepted academic practice. No use, distribution or reproduction is permitted which does not comply with these terms.
*Correspondence: Ivan John Oresnik, Ivan.Oresnik@umanitoba.ca