- 1Department of Biological Sciences, State University of Santa Cruz, Ilhéus, Brazil
- 2Department of Phytopathology, Federal University of Lavras, Lavras, Brazil
The leaf surface combines biochemical substances and pre-existing morphological structures, as well as the presence of microorganisms. This dynamic environment constitutes a plant's initial defense, as well as the first contact of phytopathogens during invasion. Spore germination starts on the phylloplane and is a fundamental process for fungal development, and hence the establishment of disease. In this review, we address the phylloplane's innate defense mechanisms and biochemical reactions involved in the early stage of phytopathogenic fungal development. The focus is present the pre-infection molecular and biochemical processes of the interaction between Theobroma cacao and Moniliophthora perniciosa, showing how the defense mechanisms of the phylloplane can act to inhibit proteins involved at the beginning of fungal spore germination. We conclude that the phylloplane of the cocoa resistant genotype to M. perniciosa has performed chemical compounds, pre-existing morphological structures and the presence of microorganisms that participate in the pre-infection defense of the plant. Also, the inhibition of proteins involved in the germination mechanism of M. perniciosa basidiospores by chemical and structural compounds present in the cocoa phylloplane may decrease the disease index. Therefore, understanding how the phylloplane defense acts in the fungal spore germination process is essential to develop pre-infection control strategies for cacao plants against witches' broom.
Introduction
The phylloplane, defined as the leaf surface, serves as plant's initial defense against pathogens due to its structural characteristics and functional aspects (Figure 1). Structurally, the phylloplane includes the leaf cuticle, which in turn consists of a mixture of cutin and hydrophobic wax, and in some plants simple and glandular trichomes (Figures 1, 2B). This layer constitutes the first barrier against foliar pathogen contact and invasion. The cuticle contributes to the protection against mechanical damage, prevents dehydration of the phylloplane and protects the leaf tissues against UV irradiation (Kerstiens, 1997; Nawrath, 2006). Stomata act to control water and gas exchange between the plant and the environment (Vacher et al., 2016). The closing of the stomata may be involved in the innate immune system of the plant. Guard cells, which are related to the opening and closing of stomata, have the ability to detect microbe- or pathogen-associated molecular patterns (MAMPs or PAMPs) in response to infection (Burkhardt and Hunsche, 2013). Thus, the stomata actively close when exposed to PAMPs/MAMPs, which prevents the invasion of pathogenic bacteria in plant tissues (Melotto et al., 2006; Baker et al., 2010). Glandular trichomes constitutively secrete substances that act as functional factors in the plant's innate defense (Agrios, 2005; Shepherd et al., 2005; Shepherd and Wagner, 2007; Almeida et al., 2017). Trichomes assist in plant functions during water absorption, defense against insects, larvae nutrition (Werker, 2000), secretion of antipathogenic proteins (Morrissey and Osbourn, 1999; Kroumova et al., 2007; Almeida et al., 2017) and secondary metabolites (Nagel et al., 2008; Bednarek and Osbourn, 2009). In addition, trichomes develop defense mechanisms dependent on sulfur (Wienkoop et al., 2004; Amme et al., 2005) and glutathione (Gutierréz-Alcalá et al., 2000), which protect the plant against oxidative stress. The biochemical events of phylloplane defense are also influenced by microorganisms harboring in this region (Figure 2A; Lindow and Leaveu, 2002; Remus-Emsermann et al., 2014; Bringel and Couée, 2015; Santana et al., 2018). These constitutive structural and functional resistance strategies act to prevent pathogen adhesion and disease development. Secondary metabolites and proteins secreted by the phylloplane have been identified as the chemical mediators that perform the plant's innate defense (Wagner, 1991; Wagner et al., 2004; Shepherd et al., 2005; Shepherd and Wagner, 2007; Li et al., 2014; Sallets et al., 2014; Sasse et al., 2016). Specifically, the phylloplanins from Nicotiana tabacum leaf wash were the defense protein identified in the secretion of short glandular trichomes (Shepherd et al., 2005). The antifungal properties of this protein highlights its putative role in the pre-infection defense mechanisms of other plants including the cacao tree (Theobroma cacao).
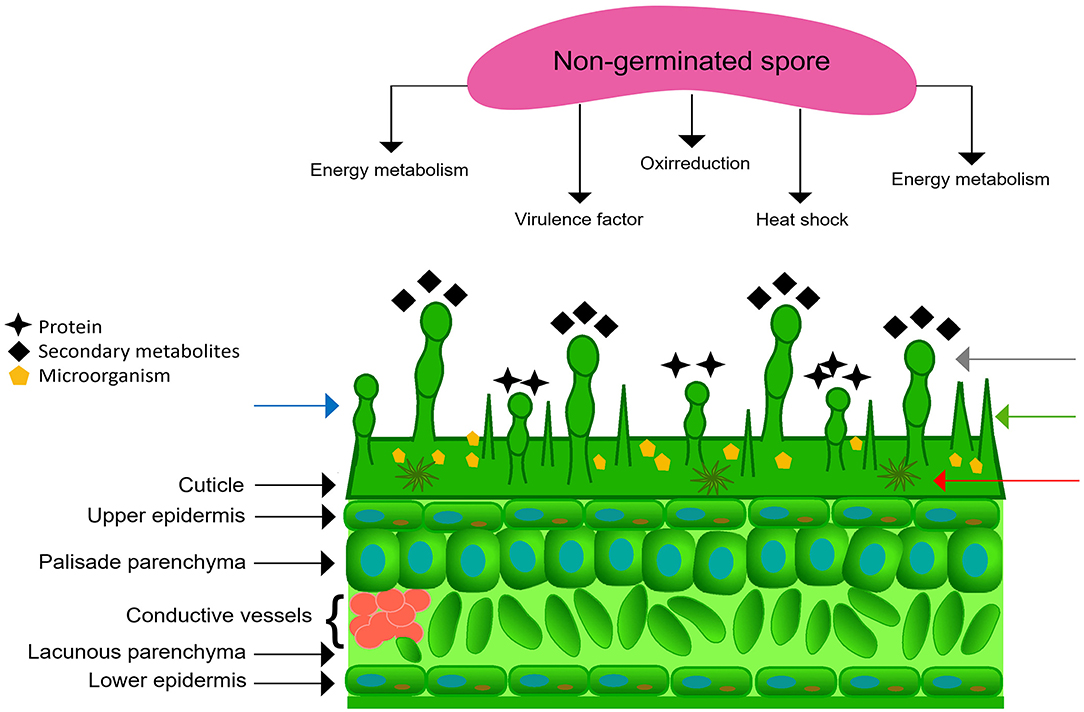
Figure 1. Pre-infection communication of pathogen and the phylloplane. Preformed proteins in a non-germinated spore and leaf cross-section. The arrows indicate simple (green), stellate (red), long glandular (gray), and short glandular (blue) trichomes.
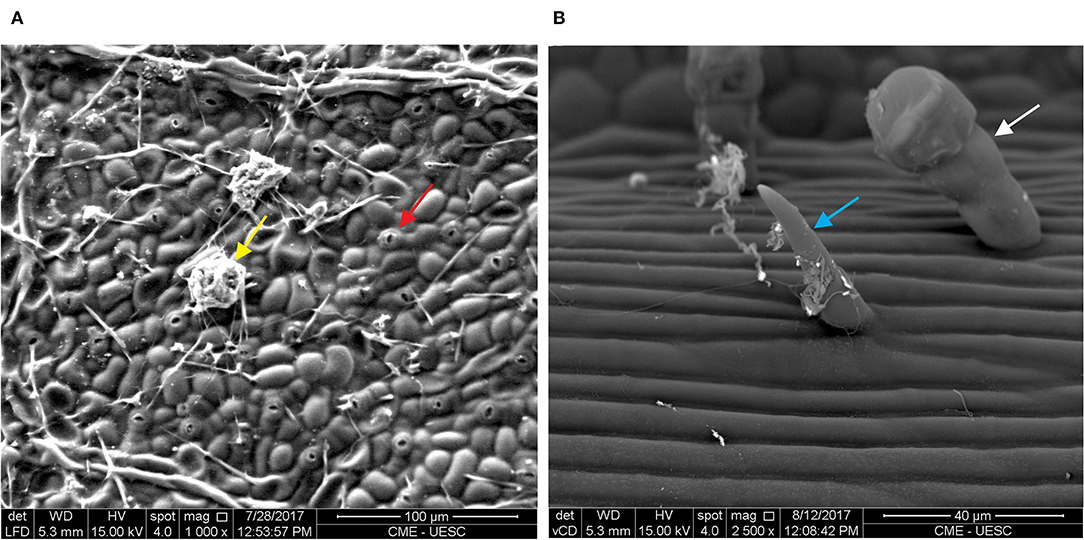
Figure 2. Abaxial surface of Theobroma cacao leaf. (A) Stomata (red arrow) and adhered microorganisms of the phylloplane (yellow arrow). (B) Glandular (white arrow) and simple trichomes (blue arrow).
The cacao tree is economically important because its beans are the raw material to produce chocolate (Judd et al., 2009). Fungal diseases cause losses of around 90% of the economic potential of this crop (Pereira et al., 1989). The fungus Moniliophthora perniciosa (previously Crinipellis perniciosa), the agent of witches' broom disease, is especially damaging in Brazil and other producing countries in South and Central America (Aime and Phillips-Mora, 2005). The interaction of the fungus with the host begins with the adhesion of its basidiospores to the phylloplane, followed by germination of the basidiospores and the formation of germ tubes (Figure 3). The onset of infection is characterized by the penetration of the germ tube into the leaf tissue through the base of trichomes (Frias et al., 1991), the stomatal pores, and by direct cuticular penetration (Sreenivasan and Dabydeen, 1989; Sena et al., 2014) and the development of primary hyphae in the apoplast (Sena et al., 2014). On the other hand, evidence exists of direct penetration of M. perniciosa basidiospores into meristematic tissues (Sena et al., 2014). The germination of fungal spores is dependent on the activity of their preformed proteins (Figure 1; Oh et al., 2010; El-Akhal et al., 2013; Mares et al., 2016, 2017), and a favorable environment on the phylloplane of the host plant (Agrios, 2005). The identification of pre-infection biochemical mechanisms involved in the T. cacao—M. perniciosa interaction may lead to a new strategy to manage this pathogen by preventing its adherence and disease development.
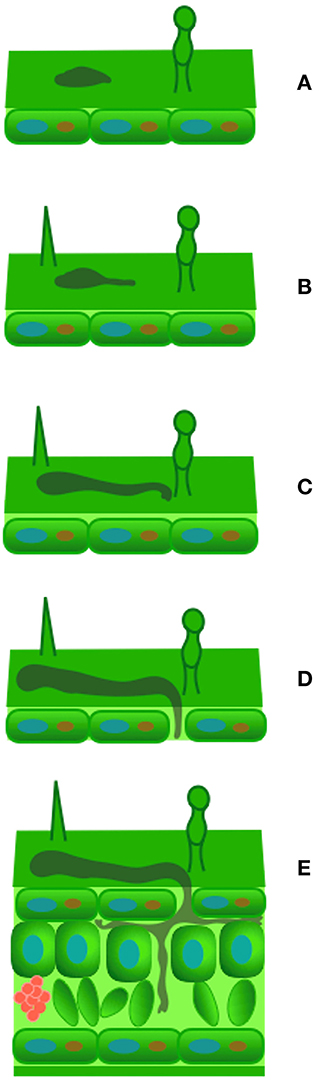
Figure 3. Theobroma cacao phylloplane and Moniliophthora perniciosa spore interaction. Adhesion (A), germination and germ tube elongation 2 h (B), after germination (hAG), club-shaped tip formation 4 hAG (C), germ tube penetration 6 hAG (D), and development of primary hyphae in the apoplast 2 days after germination (E).
In this review, we explore the state of the art of the biochemical mechanisms involved in the germination of fungal spores, the defense mechanisms of the phylloplane and the role of microorganisms residing on the phylloplane in these processes. We highlight the research associated with the pre-infection mechanisms involved in the T. cacao—M. perniciosa interaction. Finally, we suggest directions for future experimental investigations aimed at the development of technological strategies to control witches' broom in cocoa.
Cocoa Phylloplane: The First Battlefield
The management strategies available to control witches' broom disease are based on the development of resistant cocoa genotypes (Lopes et al., 2004; Silva et al., 2010; Pires et al., 2012). To achieve this, genetic improvement programs have considered factors such as improved seed yield and quality, genetic compatibility among genotypes and selection of genes of resistance to pests and pathogens (Lopes et al., 2011). However, the resistance shown by the selected clones has been overcome due to the adaptive evolution of M. perniciosa (Gramacho et al., 2016). On the other hand, several authors have suggested that the cocoa phylloplane has structural and functional characteristics useful in pre-infection defense (MacAgnan et al., 2009; Nyadanu et al., 2012; Almeida et al., 2017; Santana et al., 2018). These characteristics can be explored to develop technologies that optimize a preventive defense of the plant itself and produce varieties with a stronger and more durable resistance. A potential candidate for the biochemical mediation of this innate response is the phylloplanin protein (Shepherd et al., 2005; Shepherd and Wagner, 2007; Freire et al., 2017).
Phylloplanin is the defense protein secreted on the phylloplane identified so far. The activity of the T-phylloplanin gene promoter has been identified in short glandular trichomes of N. tabacum, which suggests that it is the secretory structure of this protein. Its anti-microbial activity was demonstrated by the inhibition of germination of Peronospora tabacina sporangia exposed to N. tabacum leaf wash. Interestingly, this anti-microbial effect was also evidenced by the exposure of the pathogen to recombinant phylloplanin produced in a heterologous system (Shepherd et al., 2005). In addition, the anti-microbial effect of leaf washing on P. tabacina is not manifested by silencing the T-phylloplanin gene in a resistant tobacco variety, making it susceptible to the pathogen (Kroumova et al., 2007). In fact, phylloplanin has an anti-microbial property, which demonstrates its biotechnological potential for the control of plant diseases (King et al., 2011). However, exogenous phylloplanin application may not be an economically viable management strategy (King et al., 2011). On the other hand, strategies to stimulate the endogenous production of phylloplanin in plants have also been proposed to explore its antifungal and anti-oomycete potential. For this, it is necessary to introduce the gene through genetic transformation of plants. Tobacco plants susceptible to P. tabacina were transformed with the T-phylloplanin gene directed toward the apoplast. This showed that the overexpression of this gene in tobacco plants conferred resistance against P. tabacina (Kroumova et al., 2013). In addition, the introduction of the synthetic phylloplanin gene in N. tabacum also provides immunity to plants susceptible to infection by P. tabacina (Sahoo et al., 2014). In the same study, the leaf wash of Datura metel and Helianthus annus also inhibited germination of the phytopathogen and reduced leaf infection (Kroumova et al., 2007). However, these results are insufficient to attribute the antifungal effect observed specifically to phylloplanin. For this purpose, investigations with antibodies to neutralize the action of phylloplanin, sequencing of the protein, as well as silencing or overexpression of the gene are still needed. The ability of phylloplanin to prevent germination of pathogen spores on the phylloplane of tobacco and sunflower (Shepherd et al., 2005; Kroumova et al., 2007) supports the possibility of investigating this function in the interaction between M. perniciosa and cacao plants. The phylloplanin inhibitory activity on the germination of spores was also observed for Pyricularia oryzae, Rhizoctonia solani, Colletotrichum coccodes, and Aspergillus fumigatus (King et al., 2011; Mattupalli et al., 2014). This shows that phylloplanin has an effect against a broad spectrum of fungi and oomycetes, which favors the idea of exploring its potential in plant breeding strategies aimed at the pre-infection control of witches' broom disease in cocoa.
In T. cacao, the leaf surface has simple and stellate trichomes along with short and long glandular trichomes (Figure 4; Nakayama et al., 1996; Almeida et al., 2017; Prihastanti and Nurchayati, 2020), which can be associated with plant defense. The amount of short glandular trichomes in the phylloplane of the resistant cocoa genotype to M. perniciosa (CCN51) infection is twice that in the susceptible cocoa genotype (Catongo) (Almeida et al., 2017). Interestingly, microscopic observation of glandular trichomes present in the cocoa phylloplane shows the storage of a reddish substance in young leaves of the resistant cocoa genotype (CCN51) (Figures 4E,F). This suggests the presence of biologically active compounds, such as secondary metabolites, phenolic compounds and pre-formed anti-microbial proteins that can act in the defense of the plant's phylloplane, preventing or postponing the entry of microorganisms, including the fungus M. perniciosa. Young cocoa leaves have short (Figure 4F) and long (Figure 4E) glandular trichomes with a reddish head, which secrete a highly oxidizing substance (Nakayama et al., 1996). In adult leaves, the long glandular trichomes have a brown head, with accumulation of phenolic compounds (Nakayama et al., 1996). T. cacao clones with resistance to M. perniciosa show the content of total soluble phenol higher than in susceptible clones (Nojosa et al., 2003). This may contribute to inhibit the germination of M. perniciosa basidiospores and could explain the resistance to witches' broom and protection against herbivory. Moreover, the phylloplane of the resistant cocoa genotype (CCN51) shows greater diversity of symbiont bacteria than phylloplane of the susceptible cocoa genotype (Catongo), harboring genera of symbiotic bacteria with antagonistic activity, such as Stenotrophomonas, Lysobacter and Paenibacillus (Santana et al., 2018). Lysobacter enzymogenes produces lytic enzymes and has a high level of resistance to antibiotics, which contributes to the biocontrol action (Folman et al., 2003; Sullivan et al., 2003). Paenibacillus peoriae shows anti-microbial activity due to secretion of proteolytic and chitinolytic enzymes (Von der Weid et al., 2003). Actinobacteria of the genus Streptomyces have been shown to produce volatile organic compounds that inhibit the germination of M. perniciosa basidiospores on cacao plants (MacAgnan et al., 2009). On the other hand, the phylloplane susceptible genotype (Catongo) has genera of bacteria Sphingomonas (Santana et al., 2018). This genus of bacteria constitutes a large part of the microbiota of the phyllosphere of plants, being considered an important microorganism for the biological control of the phylloplane (Kim et al., 1998; Innerebner et al., 2001). The presence of Sphingomonas spp. in the phylloplane of Arabidopsis thaliana reduces the growth of the pathogen P. syringae pv. tomato DC3000. This antagonistic effect of the bacteria may be due to competition with the pathogen for the use of the carbon source present on the leaf surface (Innerebner et al., 2001). In addition, Sphingomonas have of pigments that provide protection against UV radiation (Lindow and Brandl, 2003). Favorable evidence of the participation of water-soluble compounds secreted on the cocoa phylloplane as a defense mechanism against pathogens was obtained by comparing plants irrigated in the soil or by sprinkling before inoculation with M. perniciosa (Almeida et al., 2017). In fact, the progress and severity of the disease were lower in plants irrigated in the soil than in plants irrigated by sprinkling. Leaf washing by sprinkler irrigation doubled disease incidence in the susceptible genotype and tripled it in the resistant genotype. This suggests that the chemical substances secreted on the leaf surface by glandular trichomes and/or by symbiotic bacteria on the phylloplane confer resistance to M. perniciosa (Almeida et al., 2017). Also, the antifungal activity in the cocoa leaf wash is evidenced by its ability to inhibit the germination of M. perniciosa basidiospores (Almeida et al., 2017). Besides this, the high level of epicuticular wax on the surface of cocoa leaves and fruits reduces infection caused by the oomycetes Phytophthora palmivora and P. megakarya (Nyadanu et al., 2012). The epicuticular wax layer can function as a physical barrier, preventing the fungus from penetrating the intracellular space (Agrios, 2005; Nyadanu et al., 2012). Furthermore, the layer's hydrophobicity property provides less water retention, which reduces the moisture needed for spore germination and, consequently, prevents pathogen infection (Koch and Barthlott, 2006; Nyadanu et al., 2012). This suggests that the cocoa phylloplane accumulates pre-existing compounds, proteins, microorganisms and morphological structures that affect the germination of the pathogen (Nyadanu et al., 2012; Almeida et al., 2017; Santana et al., 2018).
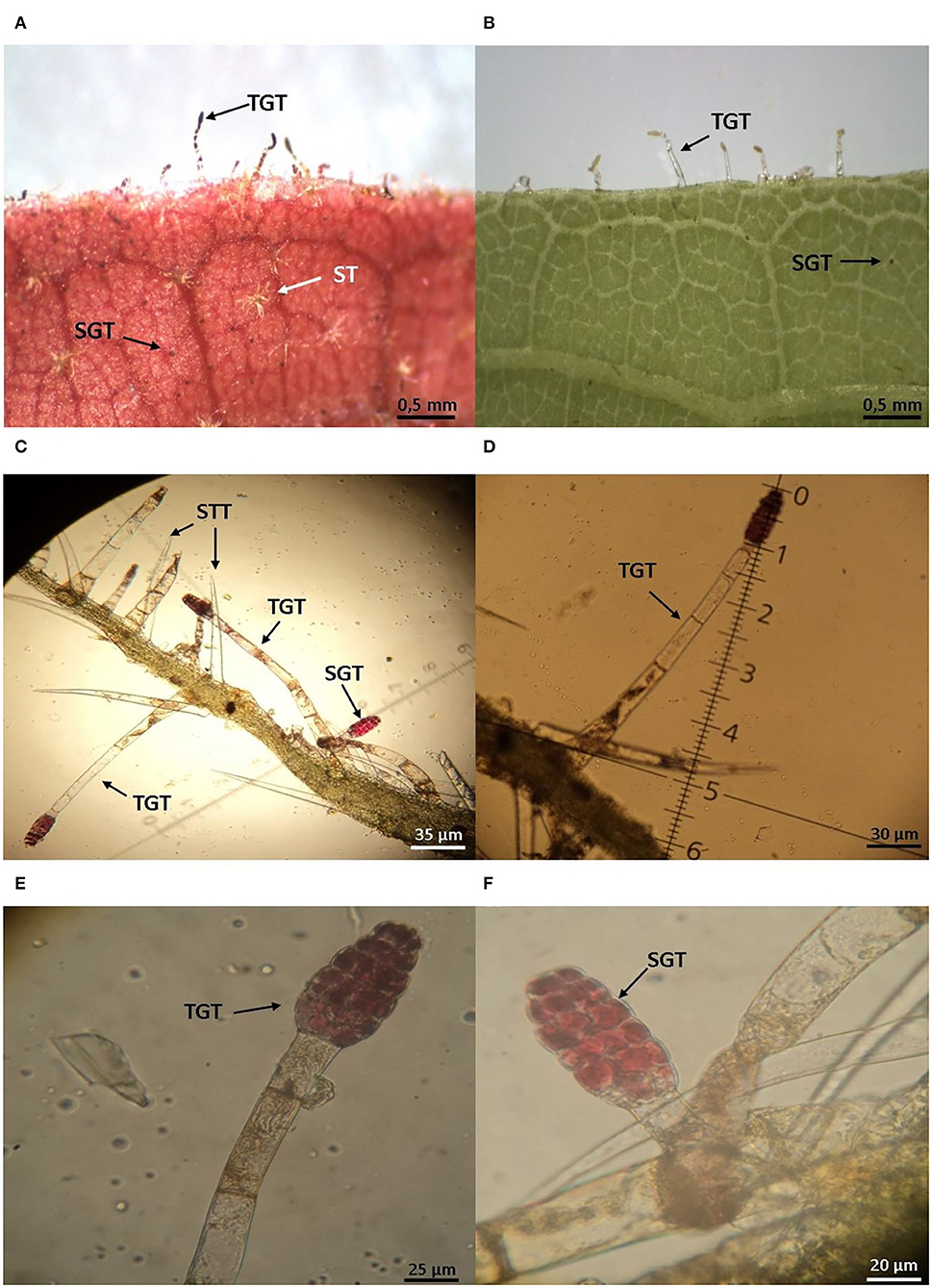
Figure 4. Morphology of trichomes on the Theobroma cacao phylloplane. (A) Distribution of simple (STT), stellate (ST), tall (long up to 5 mm) glandular (TGT), and short (measuring about 1 mm) glandular (SGT) trichomes on the abaxial leaf surface of resistant cocoa genotype to M. perniciosa (CCN51). (B) Susceptible cocoa genotype to M. perniciosa (Catongo). (C) Paradermal section of the leaf blade at the level of the central rib. (D) Magnifications of TGT. (E,F) TGT and SGT in young leaves of the resistant cocoa genotype (CCN51) containing bio-active molecules, respectively.
Proteins were identified in the leaf wash of a resistant cocoa genotypes to M. perniciosa (CCN51), using the 2D SDS-PAGE technique (Almeida et al., 2017). Among the plant proteins detected are β-caryophyllene synthase and terpene cyclase, involved in the biosynthesis of metabolites such as isoprenoids (terpenes); leucine-rich repeat receptor kinase, involved in the signaling pathway in defense against pathogens; E3 ubiquitin ligase PUB 14, related to plant-pathogen interaction; serine carboxypeptidase S28; C1 domain-containing protein; proteases; RNAse; and retrotransposon Ty1 (Almeida et al., 2017). However, the proteins of 37.9% of the spots detected in the 2D gel were not identified due to the inherent limitation of the technique. The cacao homolog of phylloplanin was not abundant in the phylloplane and may be part of this unidentified percentage. Interestingly, the cocoa leaf wash had an inhibitory effect on the germination of M. perniciosa spores, which suggests two possible hypotheses. First, the techniques used to detect phylloplanin in the cocoa leaf wash were not sufficiently accurate; or second, phylloplanin is not a key molecule in the innate pre-infection defense on the cocoa phylloplane. Although this defense protein has not yet been detected in 2D gels of the cocoa phylloplane, it has already been identified in the EST database of whole seedlings of the ICS1 genotype of T. cacao, a sequence that supposedly encodes cacao phylloplanin (Argout et al., 2008).
The first characterization of Tcphyll, the cocoa phylloplanin gene, was performed in silico. The ORF of 477 bp encodes a predicted protein of 158 amino acid residues with molecular weight of 16 kDa, presenting signal peptides and possible glycosylation and phosphorylation sites (Freire et al., 2017). In addition, a generated dendrogram with predicted protein sequence in comparison with others plant species' phylloplanin-like and allergenic pollen grain proteins containing the Ole e 1 domain showed that the predicted protein, despite the presence of the Ole e 1 domain, is grouped with proteins similar to phylloplanin (Freire et al., 2017). These results suggest that it is a phylloplanin candidate protein.
The expression of the cocoa phylloplanin transcript has been analyzed in different plant tissues. Expression analysis via RT-qPCR (quantitative real-time polymerase chain reaction) shows that the Tcphyll transcript accumulates in different tissues of the resistant cocoa genotype (Freire et al., 2017). In fact, phylloplanin is most often seen as a constitutively secreted phylloplane protein (Shepherd et al., 2005). However, cacao plants inoculated with M. perniciosa showed an increase in the level of their transcripts in infected meristem tissues. Specifically, early in the infection, an increase in expression occurred, followed by a decrease. Subsequently, in the transition period of the disease from the biotrophic to the necrotrophic phase (45 days after infection), a higher level of transcripts was again noted. This suggests that phylloplanin may also act in the defense response induced by biotic stresses (Freire et al., 2017). In addition, the Tcphyll promoter drives GUS expression in short glandular trichomes in transgenic tobacco plants, similar to the tobacco T-phylloplanin gene promoter (Shepherd et al., 2005). In transformed plants, the relative expression of the Tcphyll GUS-promoter also occurs in different tissues, such as meristem, young leaf, stem and root (Freire et al., 2017). Thus, we speculate that the expression of the cocoa phylloplanin gene in the subepidermal tissues of the plant and the absence of the protein in the leaf wash are related to the constant washing of the leaf surface over the years. The cacao tree is native to a region where the annual rainfall is around 2,300–3,500 mm (Fisch et al., 1998). Thus, cocoa plants submitted to this selective pressure evolved a lower expression of the phylloplanin gene on the leaf surface and the occurrence of expression in subepidermal layers (Freire et al., 2017). This may also explain the non-detection of this protein in the 2D SDS-PAGE of cocoa leaf wash (Almeida et al., 2017).
In view of these results, some routes can be explored experimentally to verify the potential of the phylloplanin as the battlefield where the cacao tree must win the war against the witches' broom pathogen. Initially, the production of recombinant phylloplanin from cocoa, through the heterologous protein expression system, is an efficient way to obtain an immunogen for the manufacture of specific antibody to be used in functional studies of the protein in the pathosystem. The polyclonal anti-phylloplanin antibody produced can be used to detect phylloplanin in different plant tissues of cocoa by western blotting. These data will provide evidence about where the highest level of phylloplanin protein accumulation in cocoa occurs. Furthermore, the recombinant phylloplanin produced can be used to perform functional bioassays against basidiospores of M. perniciosa. Another possibility is to carry out a phylogenetic analysis with plant phylloplanin sequences deposited in public databases to understand whether there is any selective pressure that promotes expression in subepidermal tissues of cocoa (Freire et al., 2017). Thus, the role of phylloplanin in the defense mechanism of cacao can be clarified.
Although it is not known whether the cocoa phylloplanin acts in the initial defense mechanism, it is known that the protection of the cocoa phylloplane involves preformed water-soluble compounds - metabolites and proteins (Almeida et al., 2017) and pre-existing morphological structures - trichomes, waxes (Nyadanu et al., 2012; Almeida et al., 2017), as well as the microorganisms harboring in this region (Santana et al., 2018; Figure 5). Thus, the resistance to the M. perniciosa fungus of the cocoa genotype CCN51 is influenced by these factors, which participate in the pre-infection defense mechanism of the plant and contribute to prevent germination of the pathogen. The cocoa phylloplane is hence the battleground where the plant's innate defense acts to prevent pathogen adhesion and disease development. Finally, we explore in the next topic the processes related to the germination of fungal spores, describing protein groups that are fundamental for the germinal development of the basidiospores of M. perniciosa.
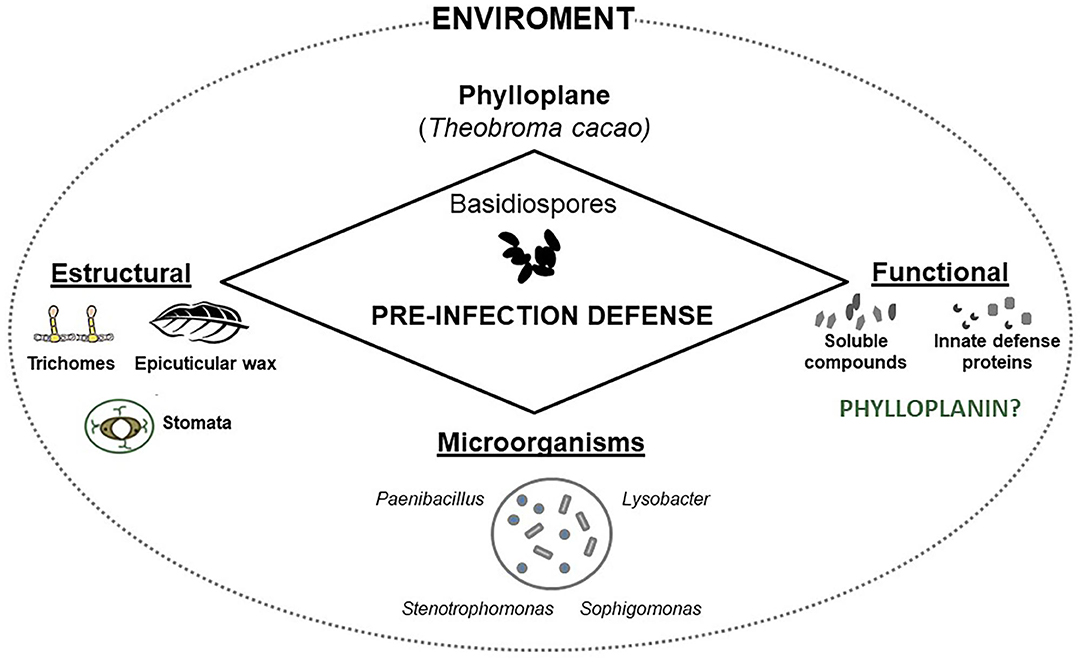
Figure 5. Pre-infection defense of the Theobroma cacao phylloplane. Schematic interaction between cocoa phylloplane (chemical and structural mediators and microorganisms) and inhibition of Moniliophthora perniciosa basidiospores during adhesion, germination, and germ tube formation signaling pathway.
Germination of Fungal Spores: Initial Stage of Disease Establishment
Spore germination is a fundamental physiological mechanism for the development of the fungal life cycle. This phenomenon is essential for the establishment of the disease, as the infecting structure of the pathogen comes into contact with the phylloplane of the host plant (Agrios, 2005). Activation of dormant spores triggers metabolic activity, inducing growth and germ tube formation (Schmit and Brody, 1976; Bonnen and Brambl, 1983; D'Enfert, 1997). In fact, fungal spore germination depends on specific protein activity at different stages of the process (Mares et al., 2016, 2017). Specifically, energy metabolism, heat shock, transcription, and synthesis proteins are crucial in early stages of fungal spore germination (Oh et al., 2010; El-Akhal et al., 2013; González-Rodríguez et al., 2015; Mares et al., 2016, 2017). Thus, a defense strategy of T. cacao against the fungus M. perniciosa can be based on the inhibition of these proteins by compounds present in the cocoa phylloplane, in order to decrease the germination of the fungus's basidiospores, and consequently the disease index. Initially, proteomic studies in non-germinated basidiospores and in different stages of germination of M. perniciosa were carried out to identify possible targets of inhibitors (Mares et al., 2016, 2017).
The first proteomic mapping performed with non-germinated basidiospores of M. perniciosa identified ribosomal proteins and proteins involved in transcription and RNA processing (eukaryotic transcription initiation factor and elongation factor EF1-Alpha). These are important for the initial phase of fungal germination, since they participate in protein biosynthesis (Mares et al., 2016). In addition, proteins involved in the stress response (oxidoreductases and ascorbate peroxidase), as well as proteins with pathogenicity functions related to the metabolic processes of lipids, carbohydrates and proteins were also identified (Mares et al., 2016, 2017). Among the proteins involved in metabolism are fumarate reductase, aspartate aminotransferase, and dihydrolipoyl dehydrogenase, which are essential for the citric acid cycle (Mares et al., 2016). Also, proteins related to the invasive development of the hypha are necessary to trigger the germination of the spore on the surface of the plant (Mares et al., 2016). On the other hand, the comparison of the protein profiles of the different germination stages of the basidiospores of M. perniciosa shows alterations in the accumulation of proteins during the development of the germ tube (Mares et al., 2017). Proteins involved in energy metabolism increase accumulation throughout germ tube development (Mares et al., 2017). Catalase A and superoxide dismutase (SOD), proteins associated with the oxidative response, are induced during primary hyphal growth (2 h after germination) and can provide detoxification of free radicals generated during the germination process (Mares et al., 2017). The presence of these proteins may be related to a tolerance of cocoa pathogens to the production of ROS by the host plant at the beginning of infection (Dias et al., 2011). Due to the high activity of catalases and peroxidases, in vitro, the fungus M. perniciosa tolerates hydrogen peroxide in concentrations above 4 mM (Pungartnik et al., 2009). Furthermore, the proteins septin and kinesin induced in basidiospores after 4 h of germination are related to the control of cell division, and consequently to the filamentation of the fungus (Mares et al., 2017). Septin may be involved in the pathogenesis of the fungus, since defects in the filament cells of the pathogens Ustilago maydis and Magnaporthe oryzae reduce the symptoms of the disease during infection (Boyce et al., 2005; Dadgas et al., 2012). Thus, the proteomic approach has mainly contributed to identify the specific proteins correlated with each phase of the germination process of M. perniciosa (Mares et al., 2016, 2017). However, only one study investigated the influence of leaf washing of resistant (CCN51) and susceptible (Catongo) cacao genotypes on basidiospore germination in M. perniciosa (Mares et al., 2020).
The germination level was lower in the fungus exposed to leaf washes of the resistant genotype. Furthermore, proteins associated with virulence, such as glycoside hydrolase and MFS multidrug transporter, were expressed exclusively in the germinated basidiospores of M. perniciosa exposed to the leaf washes of the resistant genotype. On the other hand, M. perniciosa basidiospores exposed to leaf washes of the susceptible genotype expressed immune response proteins (Mares et al., 2020). Interestingly, the germination of the basidiospores was correlated with the differential protein accumulation when exposed to the leaf washes of resistant or susceptible genotypes. Proteins specifically accumulated in M. perniciosa basidiospores germinated in leaf washes of the resistant genotype may have been induced due to the differentially secreted molecules. The identification of chemical mediators in the phylloplane that prevent the expression of virulence-related proteins seems to be a good starting point to establish an effective pre-infection defense in cacao.
The water-soluble components of the cocoa leaf wash induced the fermentative pathway in the basidiospores of M. perniciosa at the beginning of germination (Mares et al., 2020). Specifically, alcohol dehydrogenase (ADH), an enzyme that participates in the fermentation pathway, was detected exclusively in germinated basidiospores exposed to cocoa leaf washing by western blot tests (Mares et al., 2020). This seems to prepare the primary hyphae for their establishment in the intercellular space at the beginning of the parasitic phase of the pathogen (Ceita et al., 2007). Electron micrographs showed that hyphae are scarce in the parasitic phase and that they are surrounded by a thick layer of electrodense material (Sena et al., 2014; Teixeira et al., 2014), which can hinder the diffusion of oxygen into the interior of the hyphae. Thus, the accumulation of ADH can facilitate the obtainment of energy by the anaerobic route by hyphae in the initial phase of the disease. In addition, catalase, a protein involved in the oxidoreduction process, had greater expression in the basidiospores germinated in the cocoa leaf washes of the two varieties in relation to the control. This shows that the leaf surface of cocoa has defense-eliciting substances, such as reactive oxygen species (ROS). Chaperone binding immunoglobulin protein (BiP) increased expression in germinated basidiospores only in the resistant cocoa leaf wash (Mares et al., 2020), indicating the occurrence of stress at the level of the endoplasmic reticulum. Indeed, a major challenge at the basic and applied levels of research on the interaction of M. perniciosa and T. cacao is to identify the mechanism by which compounds in the phylloplane can influence the proteins involved in the germination of basidiospores of the pathogen.
In conclusion, we believe that inhibiting and/or disturbing the beginning of fungal spore germination is an alternative with potential to develop strategies for pre-infection control of cacao plants against witches' broom. The studies carried out involving the identification of the function of inhibitory compounds that participate in the defense in the cocoa phylloplane and of specific proteins in the germination process of M. perniciosa basidospores contribute to broaden the understanding of the pre-infection mechanism of the T. cacao- M. perniciosa interaction. In fact, the experimental challenges consist of developing research to understand how phylloplane defense mechanisms act to inhibit the proteins involved in the start of germination. For example, genetic engineering approaches can develop cocoa genotypes that overexpress defense proteins capable of effectively inhibiting M. perniciosa basidiospore germination. Therefore, we emphasize the importance of these studies as avenues to find new solutions to solve old problems.
Author Contributions
MZ led the writing of this review, with DA, MS, MF, JSa, PM, JSo, and CP responsible for comments and critical review of the manuscript. DA, MS, MF, JSa, and PM produced the figures. All authors contributed to the article and approved the submitted version.
Funding
This work was supported by Coordenação de Aperfeiçoamento de Pessoal de Nível Superior (CAPES) (0001) and Conselho Nacional de Desenvolvimento Científico e Tecnológico (CNPq) (303765/2019-4).
Conflict of Interest
The authors declare that the research was conducted in the absence of any commercial or financial relationships that could be construed as a potential conflict of interest.
Publisher's Note
All claims expressed in this article are solely those of the authors and do not necessarily represent those of their affiliated organizations, or those of the publisher, the editors and the reviewers. Any product that may be evaluated in this article, or claim that may be made by its manufacturer, is not guaranteed or endorsed by the publisher.
Acknowledgments
We thank João Zugaib for comments on the manuscript.
References
Aime, M. C., and Phillips-Mora, W. (2005). The causal agents of witches' broom and frosty pod rot of cacao (chocolate, Theobroma cacao) form a new lineage of Marasmiaceae. Mycologia 97, 1012–1022. doi: 10.3852/mycologia.97.5.1012
Almeida, D. S. M., Gramacho, K. P., Cardoso, T. H. S., Micheli, F., Alvim, F. C., and Pirovani, C. P. (2017). Cacao phylloplane: the first battlefield against Moniliophthora perniciosa, which causes witches' broom disease. Phytopathology 102, 864–871. doi: 10.1094/PHYTO-06-16-0226-R
Amme, S., Rutten, T., Melzer, M., Sonsmann, G., Vissers, J. P. C., Schlesier, B., et al. (2005). A proteome approach defines protective functions of tobacco leaf trichomes. Proteomics 5, 2508–2518. doi: 10.1002/pmic.200401274
Argout, X., Fouet, O., Wincker, P., Gramacho, K. P., Legavre, T., Sabau, X., et al. (2008). Towards the understanding of the cacao transcriptome: production and analysis of an exhaustive dataset of ESTs of Theobroma cacao L. Generated from various tissues and under various conditions. BMC Genomics 9:512. doi: 10.1186/1471-2164-9-512
Baker, C. M., Chitrakar, R., Obulareddy, N., Panchal, S., Williams, P., and Melotto, M. (2010). Molecular battles between plant and pathogenic bacteria in the phyllosphere. Braz. J. Med. Biol. Res. 43, 698–704. doi: 10.1590/s0100-879x2010007500060
Bednarek, P., and Osbourn, A. (2009). Plant-microbe interactions: chemical diversity in plant defence. Science 324, 746–748. doi: 10.1126/science.1171661
Bonnen, A., and Brambl, R. (1983). Germination physiology of Neurospora crassa conidia. Exp. Mycol. 7, 197–207. doi: 10.1016/0147-5975(83)90040-3
Boyce, K. J., Chang, H., D'Souza, C. A., and Kronstad, J. W. (2005). An Ustilago maydis septin is required for filamentous growth in culture and for full symptom development on maize. Eukaryo. Cell 4, 2044–2056. doi: 10.1128/EC.4.12.2044-2056.2005
Bringel, F., and Couée, I. (2015). Pivotal roles of phyllosphere microorganisms at the interface between plant functioning and atmospheric trace gas dynamics. Front. Microbiol. 6:486. doi: 10.3389/fmicb.2015.00486
Burkhardt, J., and Hunsche, M. (2013). “Breath figures” on leaf surfaces-formation and effects of microscopic leaf wetness. Front. Plant Sci. 2013:422. doi: 10.3389/fpls.2013.00422
Ceita, G. O., Macêdo, J. N. A., Santos, T. B., Alemanno, L., Gesteira, A. S., Micheli, F., et al. (2007). Involvement of calcium oxalate degradation during programmed cell death in Theobroma cacao tissues triggered by the hemibiotrophic fungus Crinipellis perniciosa. Plant Sci. 173, 106–117. doi: 10.1016/j.plantsci.2007.04.006
Dadgas, Y. F., Yoshino, K., Dagdas, G., Ryder, L. S., Bielska, E., Steinberg, G., et al. (2012). Septin-mediated plant cell invasion by the rice blast fungus Magnaporthe oryzae. Science 336, 1590–1595. doi: 10.1126/science.1222934
D'Enfert, C. (1997). Fungal spore germination: insights from the molecular genetics of Aspergillus nidulans and Neurospora crassa. Fungal Genet. Biol. 21, 163–172. doi: 10.1006/fgbi.1997.0975
Dias, C. V., Mendes, J. S., dos Santos, A. C., Pirovani, C. P., Gesteira, A. S., Micheli, F., et al. (2011). Hydrogen peroxide formation in cacao tissues infected by the hemibiotrophic fungus Moniliophthora perniciosa. Plant Physiol. Biochem. 49, 917–922. doi: 10.1016/j.plaphy.2011.05.004
El-Akhal, M. R., Colby, T., Cantoral, J. M., Harzen, A., Schmidt, J., and Fernández-Acero, F. J. (2013). Proteomic analysis of conidia germination in Colletotrichum acutatum. Arch. Microbiol. 195, 227–246. doi: 10.1007/s00203-013-0871-0
Fisch, G., Marengo, J. A., and Nobre, C. A. (1998). Uma revisão geral sobre o clima da Amazônia. Acta Amazon. 28, 101–101. doi: 10.1590/1809-43921998282126
Folman, L. B., Postma, J., and van Veen, J. A. (2003). Characterisation of Lysobacter enzymogenes (Christensen and cook 1978) strain 3.1T8, a powerful antagonist of fungal diseases of cucumber. Microbiol. Res. 158, 107–115. doi: 10.1078/0944-5013-00185
Freire, L. S., Santana, J. O., Souza, A. O., Santos, J. B., and Oliveira, I. B. (2017). TcPHYLL, a cacao phylloplanin expressed in young tissues and glandular trichomes. Physiol. Mol. Plant Pathol. 100, 126–135. doi: 10.1016/j.pmpp.2017.06.002
Frias, G. A., Purdy, L. H., and Schmidt, R. A. (1991). Infection biology of Crinipellis perniciosa on vegetative flushes cacao. Plant Dis. 75, 552–556.
González-Rodríguez, V. E., Lineiro, E., Colby, T., Harzen, A., Garrido, C., Cantoral, J. M., et al. (2015). Proteomic profiling of Botrytis cinerea conidial germination. Arch. Microbiol. 197, 117–133. doi: 10.1007/s00203-014-1029-4
Gramacho, K. P., Luz, E. D. M. N., Silva, F. S., Lopes, U. V., Pires, J. L., and Pereira, L. (2016). Pathogenic variability of Moniliophthora perniciosa in three agroecological zones of the cacao region of Bahia, Brazil. Crop Breed. Appl. Biotechnol. 16, 7–13. doi: 10.1590/1984-70332016v16n1a2
Gutierréz-Alcalá, G., Gotor, C., Meyer, A. J., Fricker, M., Veja, J. M., and Romero, L. C. (2000). Glutathione biosynthesis in Arabidopsts trichome cells. Proc. Natl. Acad. Sci. U.S.A. 97, 11108–11113. doi: 10.1073/pnas.190334497
Innerebner, G., Knief, C., and Vorholt, J. A. (2001). Protection of Arabidopsis thaliana against leaf-pathogenic Pseudomonas syringae by Sphingomonas strains in a controlled model system. Appl. Environ. Microbiol. 77, 3202–3210. doi: 10.1128/AEM.00133-11
Judd, W. S., Campbell, C. S., Kellogg, E. A., Stevens, P. F., and Donoghue, M. J. (2009). Sistemática Vegetal: Um Enfoque Filognético. Porto Alegre: Artmed
Kerstiens, G. (1997). Plant cuticles - an integrated functional approach. Plant Biol. Abstr. 47, 50–60.
Kim, H., Nishiyama, M., Kunito, T., Senoo, K., Kawahara, K., Murakami, K., et al. (1998). High population of Sphingomonas species on plant surface. J. Appl. Microbiol. 85, 731–736. doi: 10.1111/j.1365-2672.1998.00586.x
King, B., Williams, D. W., and Wagner, G. J. (2011). Phylloplanins reduce the severity of gray leaf spot and brown patch diseases on turfgrasses. Crop Sci. 51, 2829–2839. doi: 10.2135/cropsci2010.12.0727
Koch, K., and Barthlott, W. (2006). Plant epicuticular waxes: chemistry, form, self-assembly and function. Nat. Prod. Commun. 1, 1067–1072. doi: 10.1177/1934578X0600101123
Kroumova, A. B., Shepherd, R. W., and Wagner, G. J. (2007). Impacts of T-phylloplanin gene knockdown and of Helianthus and Datura Phylloplanins on Peronospora tabacina spore germination and disease potential. Plant Physiol. 144, 1843–1851. doi: 10.1104/pp.107.097584
Kroumova, A. B. M., Sahoo, D. K., Raha, S., Goodin, M., Maiti, I. B., and Wagner, G. J. (2013). Expression of an apoplast-directed, T-phylloplanin-GFP fusion gene confers resistance against Peronospora tabacina disease in a susceptible tobacco. Plant Cell Rep. 32, 1771–1782. doi: 10.1007/s00299-013-1490-6
Li, C.-H., Liu, Y., Hua, J., Luo, S.-H., and Li, S.-H. (2014). Peltate glandular trichomes of Colquhounia seguinii harbor new defensive clerodane diterpenoids. J. Integr. Plant Biol. 56, 928–940. doi: 10.1111/jipb.12242
Lindow, S. E., and Brandl, M. T. (2003). Microbiology of the phyllosphere. Appl. Environ. Microbiol. 69, 1875–83. doi: 10.1128/AEM.69.4.1875-1883.2003
Lindow, S. E., and Leaveu, J. H. J. (2002). Phyllosphere microbiology. Environ. Biotechnol. 13, 238–243. doi: 10.1016/s0958-1669(02)00313-0
Lopes, U. V., Monteiro, W. R., Pires, J. L., Clement, D., Yamada, M. M., and Gramacho, K. P. (2011). Cacao breeding in Bahia, Brazil - strategies and results. Crop Breed. Appl. Biotechnol. 11, 73–81. doi: 10.1590/S1984-70332011000500011
Lopes, U. V., Monteiro, W. R., Pires, J. L., Rocha, J. B., and Pinto, L. R. M. (2004). On farm selection for witches' broom resistance in bahia, brazil- a historical retrospective. Agrotópica 16, 61–66.
MacAgnan, D., Romeiro, R. S., and Pomella, A. W. V. (2009). Inibição da germinação de basidiósporos de Crinipellis perniciosa por compostos voláteis produzidos por cinco actinomicetos residentes de filoplano de cacaoeiro. Summa Phytopathol. 35, 140–142. doi: 10.1590/S0100-54052009000200011
Mares, J. H., Gramacho, K. P., Santana, J. O., Souza, A. O., Alvim, F. C., and Pirovani, C. P. (2020). Hydrosoluble phylloplane components of Theobroma cacao modulate the metabolism of Moniliophthora perniciosa spores during germination. Fungal Biol. 124, 73–81. doi: 10.1016/j.funbio.2019.11.008
Mares, J. H., Gramacho, K. P., Santos, E. C., Santiago, A. S., Santana, J. O., Souza, A. O., et al. (2017). Proteomic analysis during of spore germination of Moniliophthora perniciosa, the causal agent of witches' broom disease in cacao. BMC Microbiol. 17:176. doi: 10.1186/s12866-017-1085-4
Mares, J. H., Gramacho, K. P., Santos, E. C., Santiago, A. S., Silva, E. M. A., Alvim, F. C., et al. (2016). Protein profile and protein interaction network of Moniliophthora perniciosa basidiospores. BMC Microbiol. 16:120. doi: 10.1186/s12866-016-0753-0
Mattupalli, C., Spraker, J. E., Berthier, E., Charkowski, A. O., Keller, N. P., and Shepherd, R. W. (2014). A microfluidic assay for identifying differential responses of plant and human fungal pathogens to tobacco phylloplanins. Plant Health Prog. 15, 130–134. doi: 10.1094/PHP-RS-14-0009
Melotto, M., Underwood, W., Koczan, J., Nomura, K., and Yang He, S. (2006). Plant stomata fuction in innate immunity against bacterial invasion. Cell Press 126, 969–980. doi: 10.1016/j.cell.2006.06.054
Morrissey, J. P., and Osbourn, A. E. (1999). Fungal resistance to plant antibiotics as a mechanism of pathogenesis. Microbiol. Mol. Biol. Rev. 63, 708–724. doi: 10.1128/MMBR.63.3.708-724.1999
Nagel, J., Culley, L. K., Lu, Y., Liu, E., Matthews, P. D., Stevens, J. F., et al. (2008). EST analysis of hop glandular trichomes identifi es an O-methyltransferase that catalyzes the biosynthesis of xanthohumol. Plant Cell 20, 186–200. doi: 10.1105/tpc.107.055178
Nakayama, L. H. I., Soares, M. K. M., and Appezzato-da-Gloria, B. (1996). Contribuição ao estudo anatômico da folha e do caule do cacaoeiro (Theobroma cacao L.). Sci. Agric. 53, 73–73. doi: 10.1590/S0103-90161996000100010
Nawrath, C. (2006). Unraveling the complex network of cuticular structure and function. Curr. Opin. Plant Biol. 9, 281–287. doi: 10.1016/j.pbi.2006.03.001
Nojosa, G. B. A., Resende, M. L. V., Aguilar, M. A. G., Bezerra, K. M. T., and Anhert, D. E. (2003). Componentes fenólicos e enzimas oxidativas em clones de Theobroma cacao resistentes e suscetíveis a Crinipellis perniciosa. Fitopatol. Bras. 28, 148–154. doi: 10.1590/S0100-41582003000200005
Nyadanu, D., Akromah, R., Adomako, B., Kwoseh, C., Dzahini-Ob, H., Lowor, S. T., et al. (2012). Host plant resistance to Phytophthora pod rot in cacao (Theobroma cacao L.): the role of epicuticular wax on pod leaf surfaces. Int. J. Bot. 8, 13–21. doi: 10.3923/ijb.2012.13.21
Oh, T. Y., Ahn, C., Kim, J. G., Ro, H., Lee, C., and Kim, J. W. (2010). Proteomic analysis of early phase of conidia germination in Aspergillus nidulans. Fung. Genet. Biol. 47, 246–253.doi: 10.1016/j.fgb.2009.11.002
Pereira, J. L., Ram, A., Figueredo, J. M., and Almeida, L. C. C. (1989). Primeira ocorrência de vassoura-de-bruxa na principal região produtora de cacao do Brasil. Agrotrópica 1, 79–81.
Pires, J. L., de Melo, G. P., Yamada, M. M., and Gramacho, K. P. (2012). Association among sources of resistance to witches' broom disease for the increment of the level and durability of the character. Agratópica 24, 27–30. doi: 10.21757/0103-3816.2012v24n1p27-30
Prihastanti, E., and Nurchayati, Y. (2020). Differences in leaf area, trichome density, and xylem structure between the two types of Theobroma cacao l. cultivation: with or without shade plants. Int. J. Plant Biol. 11, 28–31. doi: 10.4081/pb.2020.8790
Pungartnik, C., Melo, S. C. O., Basso, T. S., Macena, W. G., Cascardo, J. C. M., and Brendel, M. (2009). Reactive oxygen species and autophagy play a role in survival and differentiation of the phytopathogen Moniliophthora perniciosa. Fung. Genet. Biol. 46, 461–472. doi: 10.1016/j.fgb.2009.03.007
Remus-Emsermann, M. N. P., Lücker, S., Müller, D. B., Potthoff, E., Daims, H., and Vorholt, J. A. (2014). Spatial distribution analyses of natural phyllosphere colonizing bacteria on Arabidopsis thaliana revealed by fluorescence in situ hybridization. Environ. Microbiol. 16, 2329–2340. doi: 10.1111/1462-2920.12482
Sahoo, D. K., Raha, S., Hall, J. T., and Maiti, I. B. (2014). Overexpression of the synthetic chimeric native-T-phylloplanin-GFP genes optimized for monocot and dicot plants renders enhanced resistance to blue mold disease in tobacco (N. tabacum L). Sci. World J. 2014:601314. doi: 10.1155/2014/601314
Sallets, A., Beyaert, M., Boutry, M., and Champagne, A. (2014). Comparative proteomics of short and all glandular trichomes of Nicotiana tabacum reveals differential metabolic activities. J. Proteome Res. 13, 3386–3396. doi: 10.1021/pr5002548
Santana, J. O., Gramacho, K. P., Ferreira, K. T. S. E., Rezende, R. P., Mangabeira, P. A. O., Dias, R., et al. (2018). Witches' broom resistant genotype CCN51 shows greater diversity of symbiont bacteria in its phylloplane than susceptible genotype catongo. BMC Microbiol. 18:194. doi: 10.1186/s12866-018-1339-9
Sasse, J., Schlegel, M., Borghi, L., Ullrich, F., Lee, M., Liu, G.-W., et al. (2016). Petunia hybrida PDR2 is involved in herbivore defence by controlling steroidal contents in trichomes. Plant Cell Environ. 36, 2725–2739. doi: 10.1111/pce.12828
Schmit, J. C., and Brody, S. (1976). Biochemical genetics of Neurospora crassa conidial germination. Bacteriol. Rev. 40, 1–41. doi: 10.1128/br.40.1.1-41.1976
Sena, K., Alemanno, L., and Gramacho, K. P. (2014). The infection process of Moniliophthora perniciosa in cacao. Plant Pathol. 63, 1272–1281. doi: 10.1111/ppa.12224
Shepherd, R. W., Bass, W. T., Houtz, R. L., and Wagner, G. J. (2005). Phylloplanins of tobacco are defensive proteins deployed on serial surfaces by short glandular trichomes. Plant Cell 17, 1851–1861. doi: 10.1105/tpc.105.031559
Shepherd, R. W., and Wagner, G. J. (2007). Phyloplane proteins: emerging defences at the aerial frontline? Trends Plant Sci. 12, 1360–1385. doi: 10.1016/j.tplants.2006.12.003
Silva, S. D. V. M., Luz, E. D. M. N., Pires, J. L., Yamada, M. M., and Filho, L. P. S. (2010). Parent selection for cacao resistance to witches'-broom. Pesquisa Agropec. Bras. 45, 680–685. doi: 10.1590/S0100-204X2010000700007
Sreenivasan, T. N., and Dabydeen, S. (1989). Modes of penetration of young cacao leaves by Crinipellis perniciosa. Plant Dis. 73, 478–481.
Sullivan, R. F., Holtman, M. A., Zylstra, G. J., White, J. F., and Kobayashi, D. Y. (2003). Taxonomic positioning of two biological control agents for plant diseases as Lysobacter enzymogenes based on phylogenetic analysis of 16S rDNA, fatty acid composition and phenotypic characteristics. J. Appl. Microbiol. 94, 1079–1086. doi: 10.1046/j.1365-2672.2003.01932.x
Teixeira, J. P. L., Thomazella, D. P. T., Reis, O., do Padro, P. F. V., do Rio, M. C. S., Fiorin, G. L., et al. (2014). High-resolution transcript profiling of the atypical biotrophic interaction between Theobroma cacao and the Fungal Pathogen Moniliophthora perniciosa. Plant Cell 26, 4245–4269. doi: 10.1105/tpc.114.130807
Vacher, C., Hampe, A., Porté, A. J., Sauer, U., Compant, S., and Morris, C. E. (2016). The phyllosphere: microbial jungle at the plant–climate interface. Annu. Rev. Ecol. Evol. Syst. 47, 1–24. doi: 10.1146/annurev-ecolsys-121415-032238
Von der Weid, I., Alviano, D. S., Santos, A. L., Soares, R. M., Alviano, C. S., and Seldin, L. (2003). Antimicrobial activity of Paenibacillus peoriae strain NRRL BD-62 against a broad spectrum of phytopathogenic bacteria and fungi. Appl. Microbiol. 95, 1143–1151. doi: 10.1046/j.13652672.2003.02097.x
Wagner, G. J. (1991). Secreting glandular trichomes: more than just hairs. Plant Physiol. 96, 675–679. doi: 10.1104/pp.96.3.675
Wagner, G. J., Wang, E., and Shepherd, R. W. (2004). New approaches for studying and exploiting an old protuberance, the plant trichome. Ann. Bot. 93, 3–11. doi: 10.1093/aob/mch011
Werker, E. (2000). Trichome diversity and development. Adv. Bot. Res. 31, 1–35. doi: 10.1016/S0065-2296(00)31005-9
Wienkoop, S., Zoeller, D., Ebert, B., Simon-Rosin, U., Fisahn, J., Glinski, M., et al. (2004). Cell-specific protein profiling in Arabidopsis thaliana trichomes: identification of trichome-located proteins involved in sulfur metabolism and detoxification. Phytochemistry 65,1641–1649. doi: 10.1016/j.phytochem.2004.03.026
Keywords: leaf surface, Theobroma cacao, Moniliophthora perniciosa, innate defense, spore germination
Citation: Zugaib M, Almeida DSM, de Santana MR, Macêdo Ferreira M, Santana JO, Mangabeira PAO, Souza JTd and Pirovani CP (2022) Pre-infection Mechanisms on the Phylloplane: The First Biochemical Battlefield Between the Cacao Tree and Witches' Broom Pathogen. Front. Agron. 4:871908. doi: 10.3389/fagro.2022.871908
Received: 08 February 2022; Accepted: 20 April 2022;
Published: 27 May 2022.
Edited by:
Giovanni Bubici, National Research Council (CNR), ItalyReviewed by:
Antonio Figueira, University of São Paulo, BrazilGiovanni Luigi Bruno, University of Bari Aldo Moro, Italy
Copyright © 2022 Zugaib, Almeida, de Santana, Macêdo Ferreira, Santana, Mangabeira, Souza and Pirovani. This is an open-access article distributed under the terms of the Creative Commons Attribution License (CC BY). The use, distribution or reproduction in other forums is permitted, provided the original author(s) and the copyright owner(s) are credited and that the original publication in this journal is cited, in accordance with accepted academic practice. No use, distribution or reproduction is permitted which does not comply with these terms.
*Correspondence: Carlos Priminho Pirovani, cGlyb3ZhbmkmI3gwMDA0MDt1ZXNjLmJy