- 1Department of Crop Sciences, University of Illinois, Urbana, IL, United States
- 2Departamento de Agronomía, Centro de Recursos Naturales Renovables de la Zona Semiárida (CERZOS, UNS-CONICET), Universidad Nacional del Sur, Bahía Blanca, Argentina
- 3Department of Animal Sciences, University of Illinois, Urbana, IL, United States
Much of the global nitrous oxide emissions are derived from agricultural management driving microbial N transformations. Crop rotation, no-till, and cover cropping are feasible conservation agronomic strategies used to prevent N losses to the environment, though their effect on soil microbial N cycling at the field scale remains relatively unknown. Our goal was to determine the effect of crop rotation (continuous corn [Zea mays L.], CCC; and continuous soybean [Glycine max (L.) Merr.], SSS), tillage (no-till, NT; and chisel tillage, T), and cover crops (cover crop mixture, CC; and no cover crop, NCC) on the quantification of functional genes related to the N cycle from different times throughout the growing season. The study was conducted during the growing season of the cash crops following the first season of cover crops introduced after 23 years of management. Using quantitative polymerase chain reaction (qPCR) techniques, we quantified nifH (N2 fixation), amoA (nitrification) and nirK, nirS, and nosZ (denitrification). Our results show that CCC increased nitrous oxide emissions by 44% compared to SSS and reduced soil pH by nearly 1 unit. The reduction in soil pH, coupled with an increase in fertilizer-derived ammonium, caused ammonia-oxidizing bacteria (AOB) and nirK copy numbers to increase. The SSS rotation showed opposite results. Bacterial denitrification via the nirK pathway was likely the N cycle mechanism behind nitrous oxide emissions in CCC. The cover crop mixture of cereal rye [Secale cereale L.] and hairy vetch [Vicia villosa Roth] reduced soil nitrate levels, though they did increase nitrous oxide emissions, likely due to priming and the inclusion of a legume in the cover crop mixture. Nitrous oxide emissions were affected by sampling date, crop rotation, and cover crop use, suggesting management factors that add abundantly available N alter the microbial N cycle directly or indirectly. Chisel tillage increased the abundance of all N cycle genes compared to no-till. Together, our work adds further insight into the microbial N cycle, especially nitrous oxide evolution, from three common conservation agricultural management practices, contributing to our understanding of key soil biogeochemical processes.
Introduction
Understanding the microbial basis of greenhouse gas emissions during the growing season could help us improve our current conservation agricultural practices, such as crop rotations, tillage, and even the use of cover crops. Cost-effective fertilizer inputs increased global productivity (Erisman et al., 2008), and managing the soil nitrogen (N) cycle is a major component of agricultural sustainability (Williams et al., 2016). However, N losses due to fertilization pose a significant challenge for growers and regulators. The US Midwest (Illinois, Indiana, Iowa, Michigan, Minnesota, Ohio, and Wisconsin) is one of the most intensively cultivated areas globally (Hatfield, 2012). The large levels of agriculturally derived N allow for significant greenhouse gas (GHG) emissions and nitrate leaching. Losses of N to the environment are mediated by microbial guilds involved in the N cycle (Orellana et al., 2018; Ouyang et al., 2018; Kim et al., 2021). Diazotrophs, nitrifiers, and denitrifiers are dynamic and change in their abundances and activities in close relation to the changes in the soil environment (Basu et al., 2021). Fertilization levels and strategies, the choice of crops and sequence of crops, and the decision to till or not to till a field site will all influence the soil environment and thus the dynamic of these microbial guilds (Huang et al., 2019; Behnke et al., 2020b; Ray et al., 2020).
Agricultural soil management accounts for around 80% of the total US annual nitrous oxide (N2O) emissions, including synthetic fertilizer application, tillage practices, and crop rotation systems (Venterea et al., 2011; EPA, 2016). Losses of fertilizer N of up to 50% of the applied N have been reported (Millar et al., 2010), leaving the excess available for loss. Fertilizer N stimulates nitrous oxide production by providing a substrate for microbial N transformations (Venterea et al., 2005; Norton, 2008). By quantifying microbial functional genes responsible for those N cycle transformations, researchers are able to characterize the potential of the microbial community to fix N2 (nifH, diazotrophic genes), to activate nitrification (ammonia oxidizer genes), and denitrification (nirK, nirS, nosZ, denitrifier genes) (Jetten, 2008; Levy-Booth et al., 2014). A byproduct of microbial nitrification and denitrification, is nitrous oxide, a potent greenhouse gas (Myrold, 2021). Nitrification occurs when ammonium is either added to the soil in the form of fertilizers, as N fixation by legumes, or as mineralized soil organic matter (Paustian et al., 2016; Norton and Ouyang, 2019; Linton et al., 2020). Ammonium is converted to nitrite and eventually to nitrate during this microbial process, yet small quantities can be lost as nitrous oxide (Snyder et al., 2009). Likewise, in conditions of low soil oxygen, denitrifiers use nitrate as a terminal electron acceptor, and N2O is an intermediate step in complete denitrification to N2 gas (Aulakh et al., 1991; Robertson and Groffman, 2007; Paustian et al., 2016). Therefore, under aerobic soil conditions (<60% water filled pore space), nitrous oxide emissions are attributed to nitrification; in contrast, anaerobic conditions (> 60% water filled pore space) favor denitrification as the main contributor of nitrous oxide emissions (Moreau et al., 2019; Linton et al., 2020; Prosser et al., 2020). Nitrous oxide emissions are associated with the levels of available N in the soil as well as the soil pH, yet the reported correlations in the current literature are weak or moderate at most (Behnke et al., 2018; Wang et al., 2018).
Corn [Zea mays L.] crops may generate more nitrous emissions than soybean [Glycine max (L.) Merr.] crops due to their strong dependence on N inputs, creating a pool of easily available N prone to losses (Smith et al., 2011; Behnke et al., 2018). Fertilization of corn using ammonia-based fertilizers decreases soil pH compared to unfertilized corn and soybean (Segal et al., 2017; Zuber et al., 2017; Huang et al., 2019). In a global meta-analysis, Wang et al. (2018) examined 1,104 agricultural field experiments and concluded that soil pH influenced nitrous oxide the most compared to soil texture (sand, silt, and clay %'s) bulk density, soil organic C, and total N, observing greater nitrous oxide emissions from acidic soils. Due to fertilizer inputs, decreased soil pH affects the microbial N cycle (Fierer and Jackson, 2006; Zhu et al., 2013; Huang et al., 2019). The low pH environment coupled with abundant soil N favors nitrifying bacteria (ammonia oxidizers), leading to nitrous oxide emissions (De Graaff et al., 2019; Behnke et al., 2020b, 2021).
Tillage warms and aerates the soil, conditions that favor microbial activities and likely lead to N transformations and losses (Balesdent et al., 2000). No-till systems tend to increase soil organic C and total N compared to tilled systems (West and Post, 2002; Zuber and Villamil, 2016; Congreves et al., 2017). However, no-till systems increase surface soil pH because fertilizer N accumulates on the surface and is not incorporated (Crozier et al., 1999; Zuber et al., 2017). In the same way, the ammonia oxidizers are adapted to excess N and low pH, and show increases in copy numbers from the no-till (greater) vs. tilled (lower) cropping systems from continuous and rotated corn (Behnke et al., 2020b).
Differentiation between ammonia-oxidizing archaea (AOA) and bacteria (AOB) is also critical to understanding these N conversions from various agricultural systems (Coskun et al., 2017). In an Illinois, USA study comparing a corn-soybean rotation to continuous corn, the authors found that the continuous corn rotation increased nitrous oxide emissions and lowered pH, corresponding with increased AOB and nirK copy numbers (Behnke et al., 2018, 2020b). Huang et al. (2019) observed elevated AOB copy numbers values from a high N rate compared to zero N within the continuous corn rotation also from Illinois, USA. No differences were observed between rotated corn or soybean and continuous corn from either study. In a global meta-analysis by Ouyang et al. (2018), the authors found N cycling genes were affected by crop rotation and soil pH, showing AOB copy number decreases in monocultures and increases in both AOA and AOB copy numbers in near-neutral soil pH conditions. At low soil ammonium concentrations, AOA is more prevalent than AOB (Carey et al., 2016). In the previously mentioned long-term continuous cotton study by Hu et al. (2021) comparing tillage (no-till vs. conventional tillage) and cover crops, the authors found that tillage increased the relative abundances of nifH and nirS compared to no-till due to improved soil aeration, leading to better access to substrates with tillage. The authors also found that nirS and nosZ increased in abundance with N fertilization, corresponding to high soil nitrate levels and increased nitrous oxide emissions. Without fertilization, the leguminous cover crop, hairy vetch, contained similar N cycle gene expression as the fertilized no cover treatment (Hu et al., 2021). In a Michigan, USA study, Li and Cupples (2021) observed different tillage treatments within a corn-soybean-wheat rotation and found that no-till decreased copy numbers for each denitrification gene (nirK, nirS, and nosZ) compared to conventional tillage.
In a winter wheat-corn double-crop rotation testing tillage treatments from Yangling, Shaanxi, China, Wang et al. (2021) found that no-till decreased the abundance of AOB, nirK, and nirS copy numbers compared to conventional tillage, which reported larger abundances of denitrifiers and greater nitrous oxide emissions. In a winter wheat with and without summer cover crops in Oklahoma, USA, Somenahally et al. (2018) found that cover crops increased beneficial microbial communities, though nirK was elevated during the summer season when the cover crops were actively growing. The greater nirK gene abundance indicated that cover crops could contribute to incomplete denitrification, mediated by nitrite reducing bacteria, due to the freshly added cover crop residues and warm summer temperatures. The authors also found that the winter wheat system, regardless of tillage or cover crop management, selects for fast-growing, copiotrophic communities characterized by rapid N cycling (Somenahally et al., 2018). Similarly, Linton et al. (2020) found that a diverse rotation (corn-corn-soybean-wheat+red clover crop) compared to a simple rotation (corn-corn-soybean-soybean) increased the pathways (AOB, nirK, and nosZ) for nitrous oxide emissions from a trial in Ontario, Canada.
The spring growth of cover crops is usually terminated before planting the cash crops, creating a pool of freshly decomposing residues of high quality (low C:N ratio) that also feeds the microbial N cycling (Blagodatskaya and Kuzyakov, 2008; Kuzyakov, 2010; Hu et al., 2021). While actively growing, cover crops lower nitrate levels in the soil by sequestering N in their biomass, therefore reducing the N that can reach waterways or be lost to the environment through gaseous pathways (Basche et al., 2014; Kladivko et al., 2014; Dozier et al., 2017). However, once the cover crops are terminated, the addition of organic matter provides nutrients for microbial growth and stimulates activity (Kaleeem Abbasi et al., 2015; Frasier et al., 2016; Hallama et al., 2019). Cover crops increased total soil C by 8.5% and total N by 12.8% from a cover crop meta-analysis (Mcdaniel et al., 2014). Kim et al. (2020a) found that, globally, cover cropping significantly increased microbial abundance, activity, and diversity compared to no cover. The increased microbial activity accelerates biogeochemical cycling resulting in greater availability of N for the next crop, yet it could also lead to increased N losses via leaching or emissions through a process called priming (Basche et al., 2014). Priming occurs when fresh organic matter, low in C:N ratio, is quickly broken down, which leads to rapid N mineralization (Sainju et al., 2005; Norris et al., 2013; Chen et al., 2014). In a meta-analysis conducted by Basche et al. (2014), the authors found that 60% of the studies observed an increase in nitrous oxide emissions due to cover crops, regardless of cover crop type. The potential for cover crops to reduce nitrous oxide emissions was greatest under non-leguminous species (Basche et al., 2014). Likewise, Behnke and Villamil (2019) found that in Illinois during the cover crop growing season, nitrous oxide emissions and soil nitrate values decreased as cover crop biomass increased due to N uptake by the cover crop.
By understanding which microbial guild is activated at each stage during the growing season and how agronomic practices interact with each other in their effects on the microbiota participating in the critical steps of the microbial N cycle, we could develop targeted strategies or modify existing ones to prevent or diminish N losses to the environment. Ouyang et al. (2018) concluded that N fertilization form and duration, crop rotation, and soil pH governed microbial N cycling. While there is no way to avoid the priming effect following recent residue additions, stacking nutrient loss strategies, such as the implementation of cover crops and a reduction of fertilizer N, could reduce N losses. Likewise, acidic soil conditions due to N excesses has been shown to lead to increases in AOB copy numbers and subsequent nitrous oxide emissions, so management practices aimed at neutralizing pH and lowering N inputs could remove the competitive advantage copiotrophic microbes have in these systems (Huang et al., 2019; Behnke et al., 2020b; Kim et al., 2020b).
Crop rotation, tillage, and cover cropping modify the soil environment, yet their combined impact and interactions with the soil microbial communities are not well-understood. Though the N loss pathways are well-documented, there is limited knowledge on how soil microorganisms and their associated functional genes respond to multiple management practices at the field scale during the growing season. A few recent studies have been conducted at multiple events throughout the growing season, though all agree that timing has a significant effect on N cycle functional genes (Somenahally et al., 2018; Maul et al., 2019; Linton et al., 2020; Hu et al., 2021; Wang et al., 2021). We agree with Fierer (2017), who stated that agricultural needs have moved beyond simple bacterial to fungal ratios and descriptions of community diversity and into quantification and identification of complex patterns. Targeting and quantifying specific microbial N cycle genes is a relatively novel technique with few studies published from replicated, field-scale experiments and even fewer from long-term settings. There are no published studies investigating the combined effects of crop rotation, tillage, and cover cropping on microbial functional genes, on a temporal scale at the time of this writing.
We hypothesized that nitrous oxide emissions would increase under continuous corn rotation due to elevated levels of soil nitrate, low soil pH, and increased functional genes associated with N transformations. We also hypothesized that cover cropping would reduce nitrous oxide emissions by reducing soil nitrate and denitrification gene copy numbers. We anticipate these effects will be more pronounced at the beginning of the growing season because the systems are stressed following fertilizer application, tillage, and cover crop termination, which occurs early in the season. Likewise, soil moisture is typically greatest during the spring in Illinois. As the season progresses, the microbial community will recover back to an initial steady state, reflecting the resiliency of the systems. Therefore, the objective of this study was to use periodic gas and soil samples to compare nitrous oxide emissions and soil chemical variables with microbial functional gene quantities, specifically those related to N cycling and nitrification. This study addresses knowledge gaps as to how the genetic makeup of the nitrification and denitrification pathways relate to temporal nitrous oxide emissions. Results from this long-term study will help to identify management practices that reduce nitrous oxide emissions and understand their microbial origins.
Materials and Methods
Experimental Site Description and Management Practices
The location of the experimental site was near Monmouth, Illinois (40°55′50″N, 90°43′38″W) and took place during the growing season of 2019. The field experiment was onset in 1996, and a complete description of the site history can be found (Behnke et al., 2018, 2020b). Briefly, the soils at this location are highly fertile silty clay loam and silt loam soil series (Muscatune 43%, Sable 40%, and Osco 17%) (Web-Soil-Survey, 2021). The mean annual temperature and precipitation were 10.3°C and 871 mm, respectively (Illinois-Climate-Network, 2021). For 2019, the mean temperature was 9.9°C, and the precipitation total was 1,204 mm (Supplementary Figure S1). The experimental design was a split-split-plot arrangement of two rotation levels, two tillage levels, and two cover crop levels in a randomized complete block design with three replications (blocks). The main plots (22 m long by 12 m wide) were crop rotation treatments, which consisted of continuous corn (CCC) and continuous soybean (SSS). The first split accommodated the tillage options, either no-till (NT) or chisel tillage (T) (subplot 22 m long by 6 m wide). Cover crop options were arranged within sub-sub plots (22 m long by 3 m wide), either a cover crop mixture or no cover crop control. The cover crop mixture used in this experiment consisted of 70% cereal rye (grass), and 30% hairy vetch (legume) drilled post-harvest at a combined seeding rate of 84 kg ha−1 on October 3rd, 2018. Following biomass sampling on April 25th, 2019, cover crop growth was chemically suppressed with glyphosate [N-(phosphonomethyl)glycine] at 3.4 kg ha−1 on May 6th, 2019. Tillage occurred following cover crop suppression using a disk-ripper to 35 cm in depth, and a soil finisher was used to prepare the seedbeds. No-till plots received zero tillage. Spring N fertilizer was applied on April 26th, 2019, as injected incorporated urea ammonium nitrate (UAN) at a rate of 246 kg N ha−1; soybeans received no fertilizer. Fertilizer and pest management practices followed regional recommendations (Nafziger, 2009). Fall fertilizer P (diammonium phosphate, 18-46-0) and K (potassium chloride, 0-0-60) was last applied to these plots uniformly on November 9th, 2016 at a rate of 225 kg ha−1. In 2019, corn was planted May 26th and harvested October 7th; soybean was planted May 27th and harvested September 30th. Grain yields were harvested with a plot combine (Almaco, Nevada, IA) then adjusted to 15.5 and 13% moisture for corn and soybean, respectively.
Gas Sampling Procedures
Greenhouse gas emissions were taken five times throughout 2019 and complied with the GRACEnet chamber-based trace gas flux measurement protocol (Parkin and Venterea, 2010). The five sampling events were (1) before cover crop suppression, on April 26th, 2019; (2) the day after planting, on June 5th, 2019; (3) 1 week after fertilization, on June 13th, 2019; (4) 12 weeks after fertilization, on August 27th, 2019; and (5) 1 week before harvest, on October 10th, 2019. Prior to cover crop suppression, 24 polyvinyl chloride (PVC) white chamber bases, 0.031 m2 in size, were installed in the experimental plots before cash crop planting and fertilizer application. A pair of chamber bases were utilized in corn plots: one in-row and one between-row. Corn rows were 76 cm wide. One chamber was used in each of the soybean plots between soybean rows. Soybean rows were 38 cm wide. Measurements of GHG emissions were taken following the procedure described in Behnke et al. (2018). Briefly, chamber bases were left in place before cover crop termination, throughout the growing season, then removed before harvest. Gas samples were obtained around noon when air temperatures were near the normal for the day. Nitrous oxide emission rates were obtained by taking gas samples at 0-, 10-, 20-, and 30-min intervals. Then, gas samples were analyzed using a gas chromatograph (GC) with an electron capture detector and flame ionization detector (Shimadzu® GC 2014 with AOC-5000). Following GC analysis, soil GHG fluxes were calculated as the rate of change in gas concentration over the 30-min sampling time.
Soil Sampling, Analysis, and Procedures
Two composited soil samples were collected from each plot during gas sampling using an Eijkelkamp grass plot sampler (Eijkelkamp Soil & Water, Morrisville, North Carolina, USA) to a depth of 10 cm. Those two subsamples consisted of around ten random plugs throughout the whole plot totaling ~400 g of soil each. All samples were preserved on ice until returning to the laboratory facilities. Once at the lab, samples for soil DNA were separated into 50 mL centrifuge tubes and frozen to −20°C. The remaining field moist soil was then extracted for available N concentrations: ammonium and nitrate (NH4-N and NO3-N), using a 1:5 ratio of soil to KCl solution. The soil extracts of NH4-N and NO3-N (1M KCl) were measured colorimetrically by flow injection analysis with a Lachat Quick-Chem 8000 (Lachat Quickchem Analyzer, Lachat Instruments Loveland, CO). Gravimetric water content was determined for each soil sample and used to calculate NH4-N and NO3-N. Soil pH was determined using potentiometry (1:1 water to soil) (Mclean, 1983).
Soil DNA Extraction and Real-Time Quantitative PCR Analysis
The guidelines and protocols described in Huang et al. (2019) were followed for soil DNA extraction and qPCR determinations. Briefly, soil DNA was obtained using Power Soil DNA isolation kits (MoBio Inc., Carlsbad, California, USA), following the manufacturer's instructions. The DNA extracts were checked for purity and quantity with gel electrophoresis and a Nanodrop1000 Spectrophotometer (Thermo Fisher Scientific, Waltham, Massachusetts, USA). Soil DNA concentrations were between 10–77 ng/μl with A260/A280 values ranging 1.6–2.3. Quantification of abundance for six marker genes (AOA, AOB, nifH, nirK, nirS, and nosZ) was obtained via high-throughput qPCR at the Roy Carver Biotechnology Center for Functional Genomics lab at the University of Illinois at Urbana-Champaign, using known primers published in Huang et al. (2019) in a FluidigmBioMark HD™System. For each target gene, DNA standard curves used a dilution series (representing 102–108 copies of each target gene/μl) of fluidigm-prepared amplicons from 225 pooled soil samples as detailed in Huang et al. (2019). Standards, samples, and controls were run in six replicates on eight plates. Melting curve analyses after the final qPCR cycle permitted monitoring amplicon specificity. Under the specified qPCR conditions, the amplification efficiencies were >99% for all the primers. Target gene copy numbers were derived from standard curves of known concentrations using the comparative Ct (2–ΔΔCt) method (Livak and Schmittgen, 2001).
Statistical Analysis
The experiment aimed to test the effects of sampling time, crop rotation, tillage, and cover crops on greenhouse gas emissions, soil properties of pH and soil available N (nitrate and ammonium), and microbial functional genes. Linear mixed models were fit to each variable using the GLIMMIX procedure in SAS software version 9.4 (SAS Institute, Cary, North Carolina, USA) with a Kenward-Rogers degree of freedom adjustment (ddfm=kr) for model complexity and missing data (Gbur et al., 2012). Crop rotation, tillage, and cover crops were analyzed as fixed effects, while blocks and sampling time and their interactions with the fixed terms were considered random effects in the ANOVAs. A lognormal fit was deployed for nitrous oxide, nitrate, and ammonium due to a lack of normality of model residuals using the dist=logn option in the model statement. When appropriate, least-square means were separated using the lines option of the lsmeans statement, and the Type 1 error probability (α) was set to 0.10. Probability values associated with the analyses of variance (ANOVA) are provided in Table 1.
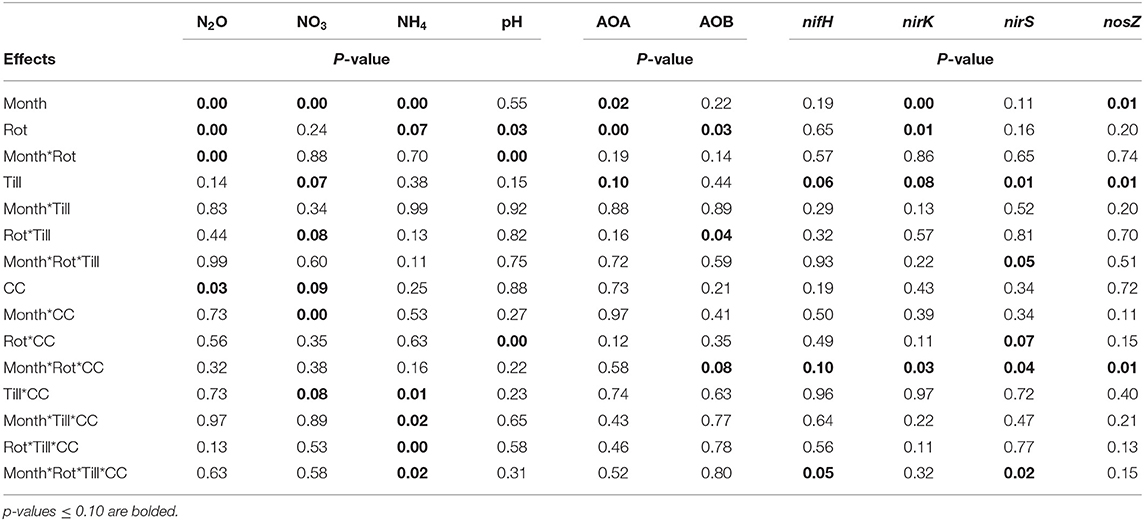
Table 1. Analysis of variance (ANOVA) results for the effects of month, crop rotation (Rot), tillage (Till), and cover crop (CC) and their interactions for nitrous oxide (N2O), available nitrogen (NO3 and NH4), pH, ammonia-oxidizing archaea (AOA), ammonia-oxidizing bacteria (AOB), nifH, nirK, nirS, and nosZ.
Results
Nitrous Oxide, Soil N, and pH
Nitrous oxide emissions were affected by the sampling date (month), crop rotation, and cover crops, with one significant interaction of month*rotation (p = 0.0042), shown in detail in Figure 1. Nitrous oxide emissions were greatest in the CCC rotation following fertilization, where emissions topped out on June 5th and on June 13th and remained greater than SSS until later in the growing season or compared to before planting, where the April 26th, August 27th, and October 10th events showed no differences between CCC and SSS. Likewise, no differences in crop rotation were observed before harvest (October 10th) or planting (April 26th). The main effect of cover crop (p < 0.0001) was found to increase nitrous oxide emissions compared to the no cover control (Table 2). Soil nitrate values were affected by month, tillage, rotation*tillage, cover crop, month*cover cropping, and till*cover crop (Table 1). The month*cover crop interaction (p < 0.0001) revealed that soil nitrate values were lowest in the cover crop treatment prior to termination and remained lower than the no cover control until June 13th, when the cover crop treatment and control were not different, eventually overtaking the control and was the greatest for the entire year on August 27th (Figure 2A). Overall, the no cover crop control combined with no-till resulted in the largest nitrate values compared to the other treatment combinations (Figure 2B). Likewise, the SSS rotation combined with no-till had greater soil nitrate amounts compared to the other treatments (Figure 2C). Similar to soil nitrate, soil ammonium also contained several significant effects and interactions, including main effects of month and rotation, and interactions of tillage*cover crop, month*tillage*cover crop, rotation*tillage*cover crop, and a four-way interaction of month*rotation*tillage*cover crop. The largest ammonium values were, as expected, observed following fertilization and primarily from the CCC rotation (Figure 3) and confirmed by the significant main effect of crop rotation (p = 0.0715, Table 2). The main effect of month was also found to be a significant (p < 0.0001, Table 2) driver of the four-way interaction. The largest ammonium values in the month*rotation*tillage*cover crop interaction were observed on June 5th and June 13th and also from the CCC rotation, which did receive N fertilization prior to that sampling event (Figure 3). As the year progressed, the soil ammonium values dropped. Soil pH contained a rotation main effect, and month*rotation and rotation*cover crop interactions (Table 1). The interactions were largely driven by a strong crop rotation main effect (p = 0.0291, Table 2) with CCC decreasing pH by nearly 0.7 (Table 2). The month*rotation interaction shows the lowest pH value occurring from the CCC rotation on June 13th (Figure 4A). The other CCC sampling events were not different, but all CCC sampling events were lower than the SSS counterpart. Upon further investigation, the interaction of rotation*cover crop (p = 0.0046) showed no difference between cover crop treatments, only the main effect of rotation (Figure 4B).
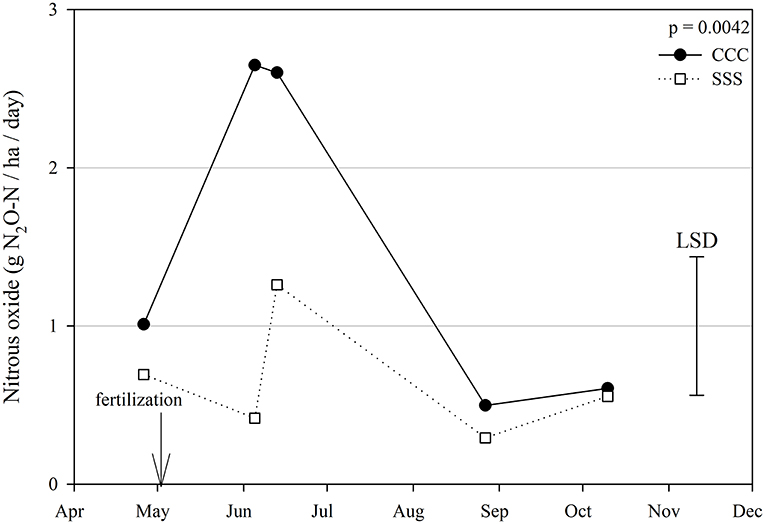
Figure 1. Mean lognormal transformed nitrous oxide emissions (g N2O-N ha−1 day −1) throughout the 2019 growing season separated by crop rotation [CCC, continuous corn (circles); SSS, continuous soybean (squares)]. Significant differences in treatment means can be interpreted by the Least Square Differences (LSD) error bar to the right of the figure. Mean values that fall beyond the distance of the LSD are significant at α = 0.10.
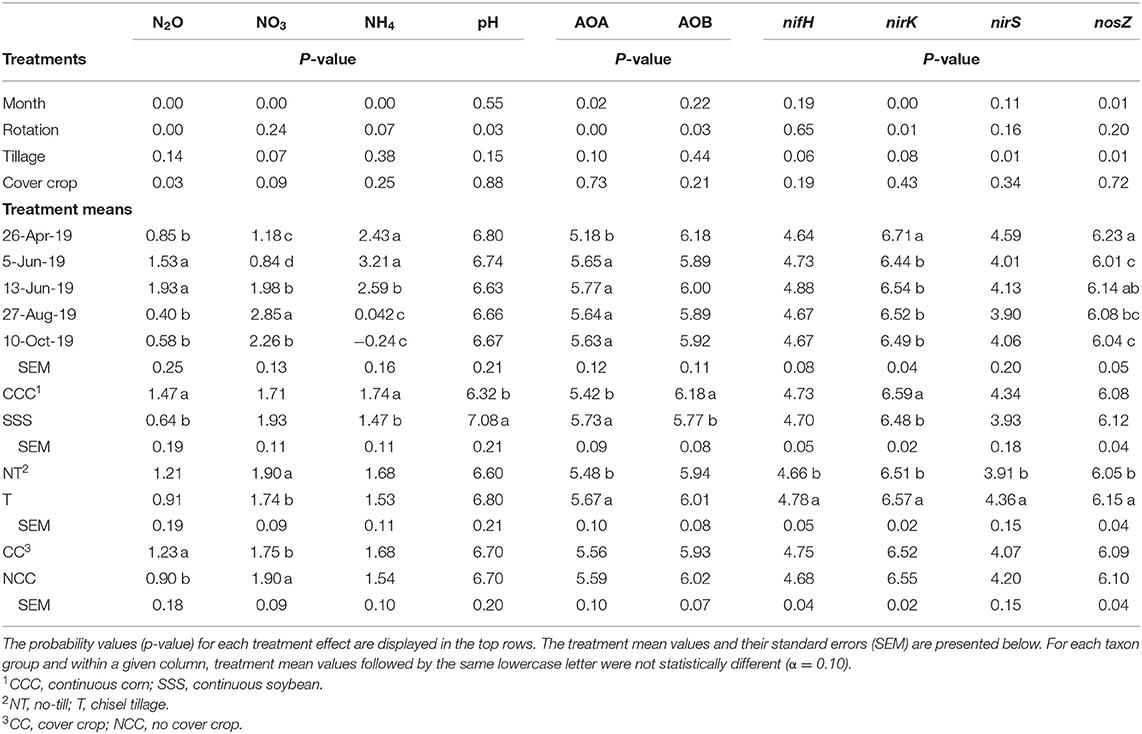
Table 2. Analysis of variance (ANOVA) results for the main effects of month, crop rotation, tillage, and cover crop on nitrous oxide (N2O, g N2O-N ha−1 day−1), available nitrogen (NO3 and NH4, mg kg−1) pH, ammonia-oxidizing archaea (AOA, copies μg DNA−1), ammonia-oxidizing bacteria (AOB, copies μg DNA−1), nifH (copies μg DNA−1), nirK (copies μg DNA−1), nirS (copies μg DNA−1), and nosZ (copies μg DNA−1).
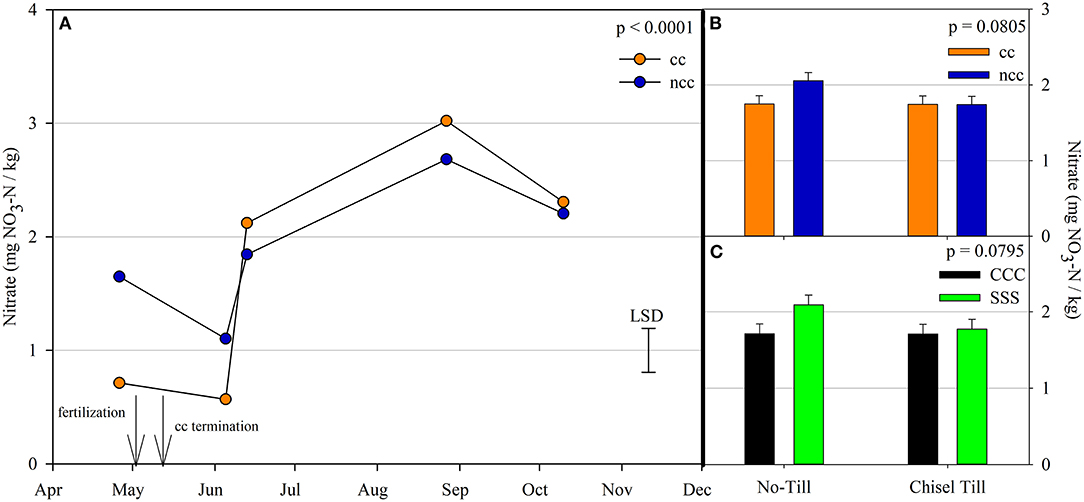
Figure 2. (A) Mean lognormal transformed soil nitrate values (mg NO3-N kg−1) throughout the 2019 growing season separated by cover crop [CC, cover crop (orange); NCC, no cover crop (blue)]. Significant differences in treatment means can be interpreted by the Least Square Differences (LSD) error bar to the right of the figure. Mean values that fall beyond the distance of the LSD are significant at α = 0.10. (B) mean soil nitrate values (mg NO3-N kg−1) separated by cover crop [CC, cover crop (orange); NCC, no cover crop (blue)] and tillage [NT, no-till; T, chisel tillage]. Error bars above each main bar represent standard errors of the mean PC scores. Differences are significant at α = 0.10. (C) mean soil nitrate values (mg NO3-N kg−1) separated by crop rotation [CCC, continuous corn (black); SSS, continuous soybean (green)] and tillage [NT, no-till; T, chisel tillage]. Error bars above each main bar represent standard errors of the mean. Differences are significant at α = 0.10.
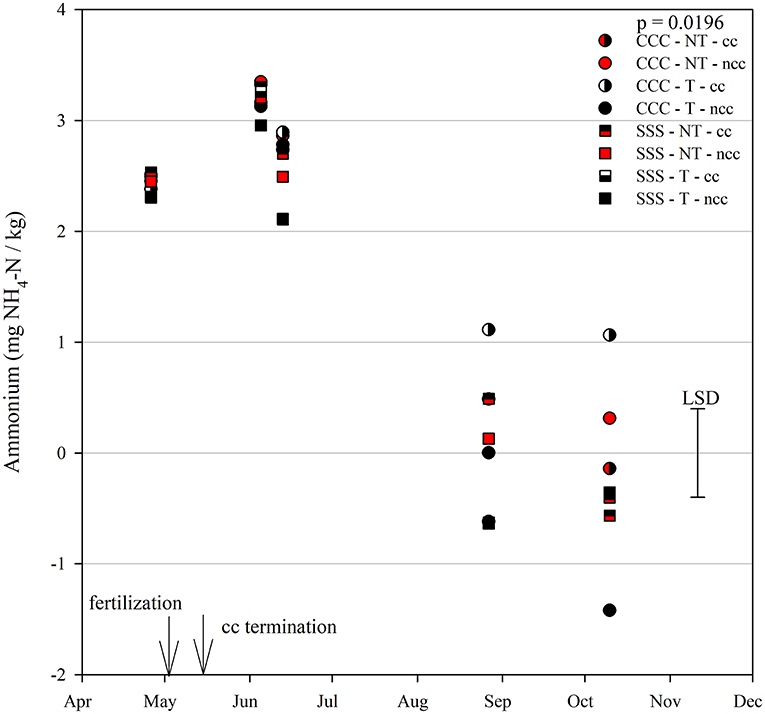
Figure 3. Mean lognormal transformed soil ammonium values (mg NH4-N kg−1) throughout the 2019 growing season separated by crop rotation [CCC, continuous corn (circles); SSS, continuous soybean (squares)], tillage [NT, no-till (red); T, chisel tillage (black)], and cover crop [CC, cover crop (partial fill); NCC, no cover crop (solid fill)]. Significant differences in treatment means can be interpreted by the Least Square Differences (LSD) error bar to the right of the figure. Mean values that fall beyond the distance of the LSD are significant at α = 0.10.
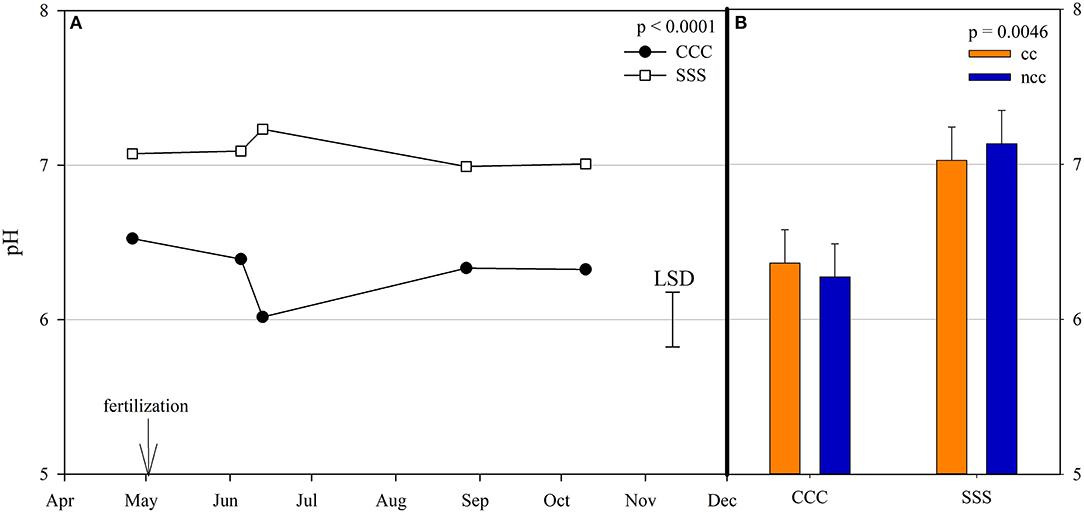
Figure 4. (A) Mean soil pH values throughout the 2019 growing season separated by crop rotation [CCC, continuous corn (circles); SSS, continuous soybean (squares)]. Significant differences in treatment means can be interpreted by the Least Square Differences (LSD) error bar to the right of the figure. Mean values that fall beyond the distance of the LSD are significant at α = 0.10. (B) mean soil pH values separated by cover crop [CC, cover crop (orange); NCC, no cover crop (blue)] and crop rotation [CCC, continuous corn; SSS, continuous soybean]. Error bars above each main bar represent standard errors of the mean PC scores. Differences are significant at α = 0.10.
Ammonia Oxidizers
Ammonia oxidizing archaea observed only main effects of month, rotation, and tillage. The lowest amount of copy numbers from AOA was observed on April 26th and was not different on any other sampling event (Table 2). The SSS rotation increased AOA copy numbers compared to CCC (Table 2). Similarly, tillage increased AOA copy numbers compared to no-till (Table 2). Ammonia oxidizing bacteria contained a strong rotation main effect, rotation*tillage, and month*rotation*cover crop interactions (Table 1). Figure 5A shows the month*rotation*cover crop interaction; all of the largest AOB copy numbers occurred in the CCC rotation, regardless of sampling event and cover crop treatment. The rotation*tillage interaction observed CCC-NT having the largest AOB copy numbers compared to both SSS-NT and SSS-T but not different from the CCC-T (Figure 5B). These interactions were driven by a highly significant main effect of rotation (p = 0.0285, Table 2), observing that CCC was greater than SSS.
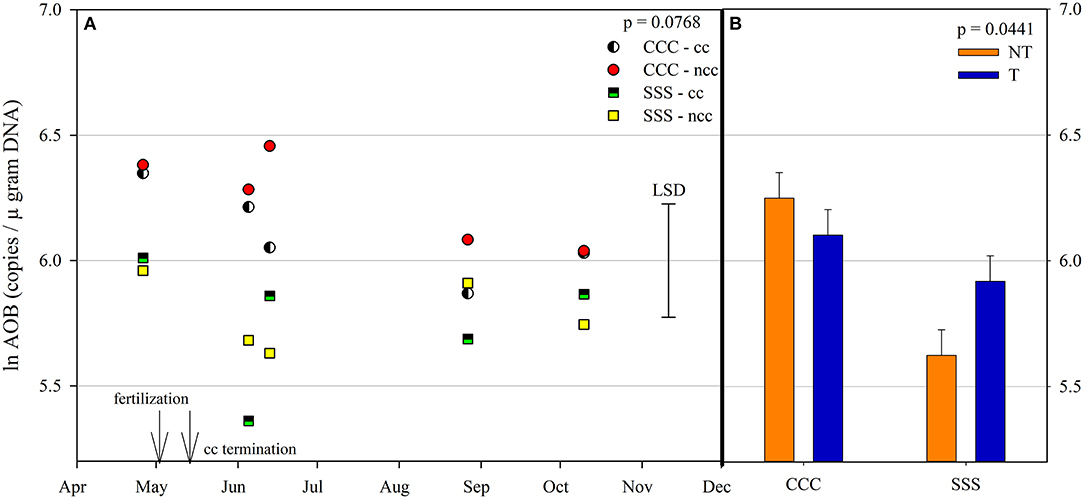
Figure 5. (A) Mean lognormal transformed ammonia-oxidizing bacteria (AOB) values (copies μgram DNA−1) throughout the 2019 growing season separated by crop rotation [CCC, continuous corn (circles); SSS, continuous soybean (squares)] and cover crop [CC, cover crop (partial fill); NCC, no cover crop (solid fill)]. Significant differences in treatment means can be interpreted by the Least Square Differences (LSD) error bar to the right of the figure. Mean values that fall beyond the distance of the LSD are significant at α = 0.10. (B) mean lognormal transformed ammonia-oxidizing bacteria (AOB) values (copies μgram DNA−1) separated by tillage [NT, no-till (orange); T, chisel tillage (blue)] and crop rotation [CCC, continuous corn; SSS, continuous soybean]. Error bars above each main bar represent standard errors of the mean PC scores. Differences are significant at α = 0.10.
N Cycle Functional Genes
The functional gene for N fixation, nifH, contained a main effect of tillage and interactions of month*rotation*cover crop and month*rotation*tillage*cover crop (Table 1). In the four-way interaction, many of the chisel till treatments contained more copy numbers compared to the no-till treatments, with little effect of sampling time (Figure 6). This trend is evident by the significant main effect of tillage (p = 0.0573), showing chisel till having greater numbers of nifH copy numbers compared to no-till (Table 2). The first denitrification functional gene, nirK, showed main effects of month, rotation, and tillage, with one interaction of month*rotation*cover crop (Table 1). The nirK copy numbers were greatest at the beginning of the season, before fertilization, and not different among rotation or cover crop treatments (Figure 7). The two June sampling events showed the greatest variability in nirK copy numbers, with the CCC rotation with no cover crops recording elevated copy numbers compared to both SSS cover crop treatments. The strong effect of rotation is validated by the detection of a significant main effect for rotation (p = 0.0052), with the CCC rotation having more copy numbers compared with the SSS rotation (Table 2). The CCC with cover crops was not different from either SSS cover crop treatment. The two later sampling events showed no differences in nirK copy numbers (Figure 7). In addition, nirK also contained a main effect of tillage with chisel tillage having greater copy numbers compared to no-till (Table 2). The second denitrification gene, nirS, contained a main effect of tillage and interactions of month*rotation*tillage, rotation*cover crop, month*rotation*cover crop, and month*rotation*tillage*cover crop (Table 1). This four-way interaction saw many no-till treatments recording fewer copy numbers than chisel till (Figure 8). This finding is confirmed by the significant main effect of tillage, where chisel till had more copy numbers compared to no-till (Table 2). The third and final denitrification gene, nosZ, included main effects of month and tillage and a month*rotation*cover crop interaction (Table 1). Similar to nirK, nosZ had elevated copy numbers at the April 26th sampling event and then decreased throughout the growing season and also observed large variability at the two June sampling events (Figure 9). The main effect of month confirms that the April sampling event did contain the largest amount of nosZ copy numbers; however, the June 13th sampling event was not different from the April 26th event (Table 2). Likewise, the final two sampling events showed no differences from each other (Figure 9). The main effect of tillage was also significant (p = 0.0069), with tillage increasing nosZ copy numbers compared to no-till (Table 2).
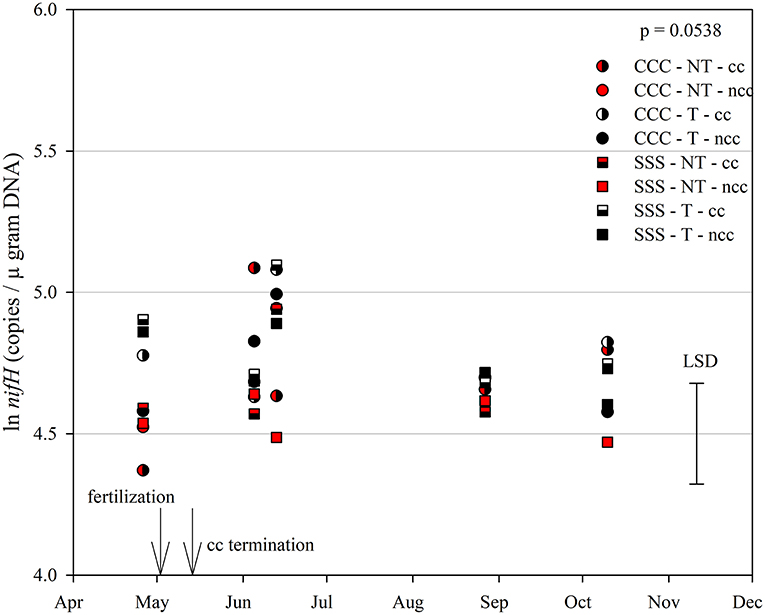
Figure 6. Mean lognormal transformed soil nifH (copies μgram DNA−1) throughout the 2019 growing season separated by crop rotation [CCC, continuous corn (circles); SSS, continuous soybean (squares)], tillage [NT, no-till (red); T, chisel tillage (black)], and cover crop [CC, cover crop (partial fill); NCC, no cover crop (solid fill)]. Significant differences in treatment means can be interpreted by the Least Square Differences (LSD) error bar to the right of the figure. Mean values that fall beyond the distance of the LSD are significant at α = 0.10.
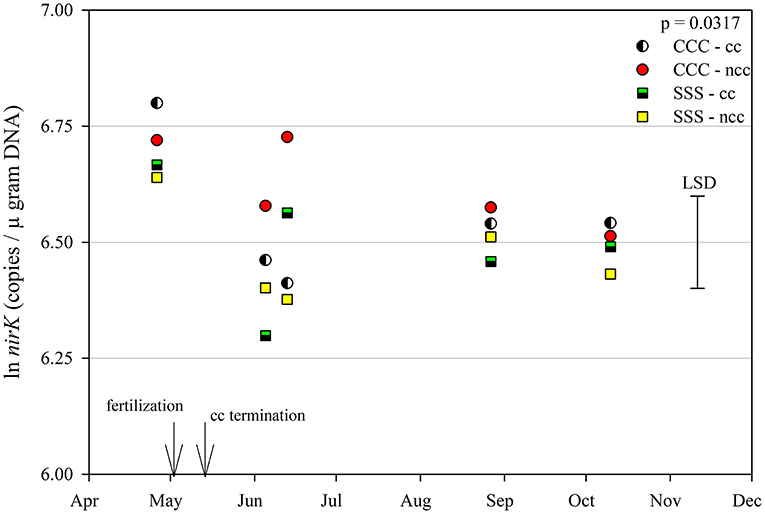
Figure 7. Mean lognormal transformed soil nirK (copies μgram DNA−1) throughout the 2019 growing season separated by crop rotation [CCC, continuous corn (circles); SSS, continuous soybean (squares)] and cover crop [CC, cover crop (partial fill); NCC, no cover crop (solid fill)]. Significant differences in treatment means can be interpreted by the Least Square Differences (LSD) error bar to the right of the figure. Mean values that fall beyond the distance of the LSD are significant at α = 0.10.
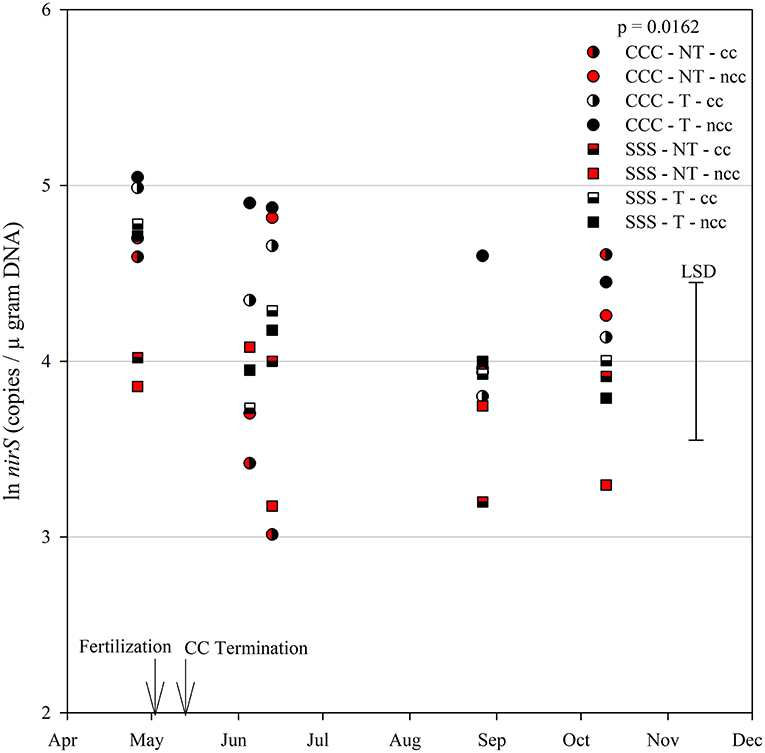
Figure 8. Mean lognormal transformed soil nirS (copies μgram DNA−1) throughout the 2019 growing season separated by crop rotation [CCC, continuous corn (circles); SSS, continuous soybean (squares)], tillage [NT, no-till (red); T, chisel tillage (black)], and cover crop [CC, cover crop (partial fill); NCC, no cover crop (solid fill)]. Significant differences in treatment means can be interpreted by the Least Square Differences (LSD) error bar to the right of the figure. Mean values that fall beyond the distance of the LSD are significant at α = 0.10.
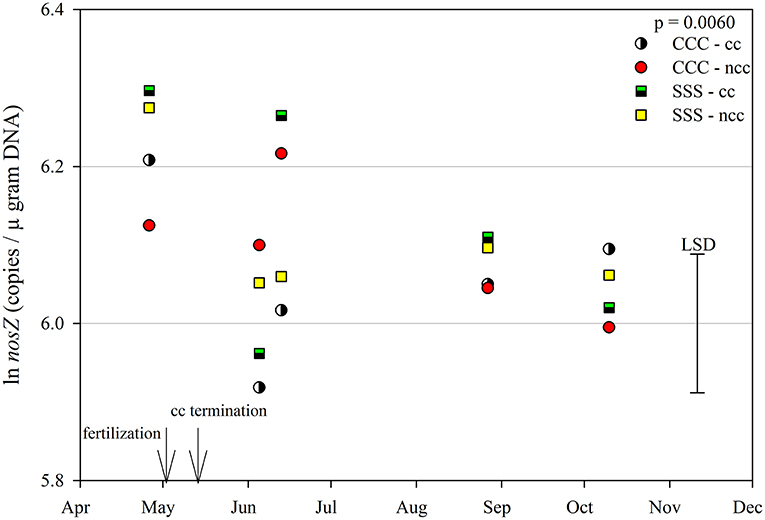
Figure 9. Mean lognormal transformed soil nosZ (copies μgram DNA−1) throughout the 2019 growing season separated by crop rotation [CCC, continuous corn (circles); SSS, continuous soybean (squares)] and cover crop [CC, cover crop (partial fill); NCC, no cover crop (solid fill)]. Significant differences in treatment means can be interpreted by the Least Square Differences (LSD) error bar to the right of the figure. Mean values that fall beyond the distance of the LSD are significant at α = 0.10.
Corn yields were affected by the addition of cover crops, but not tillage or their interaction (Table 3). Compared to no cover, a corn yield reduction of 2.4 Mg/ha was observed in the cover crop treatment. Soybean yields were affected by both main effects, tillage and cover crops, and their interaction (p = 0.0781). The chisel till with no cover crops had the greatest yields at 3.99 Mg/ha, then no difference between the no-till, no cover crop treatment and the cover crop, tillage pair (3.75 and 3.55 Mg/ha, respectively) and, lastly, the no-till, cover crop treatment combination at 3.04 Mg/ha (Table 3). Cover crop biomass was not affected by the treatments in this study and averaged 2.69 Mg/ha over the entire experiment (Table 3).
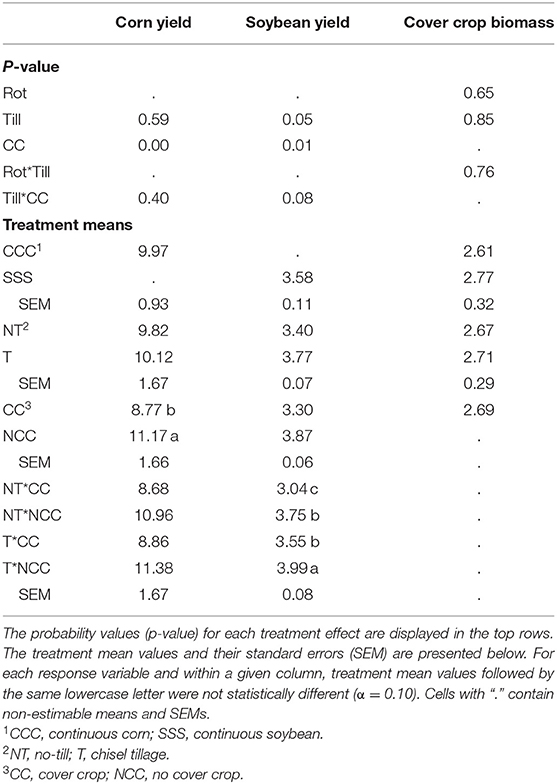
Table 3. Analysis of variance (ANOVA) results for the main effects and interactions of crop rotation (Rot), tillage (Till), and cover crop (CC) on corn and soybean yields (Mg ha−1) and cover crop biomass (Biomass, Mg ha−1).
Discussion
Temporal Trends in Microbial N Cycling
Following more than 20 years of consistent crop rotation and tillage management, cover crops were included in the rotation to study their effects on soil microbial N dynamics, nitrous oxide evolution, and other soil properties. There are no published studies from the field-scale investigating the effects of crop rotation, tillage, and cover cropping simultaneously on soil microbial genes at multiple points throughout the growing season. Overall, sampling event date had a significant main effect or was part of an interaction for all of the variables tested, meaning that each variable changed throughout the growing season. Tracking those changes is particularly interesting for the microbial functional genes as little is known about how the microbial N cycle changes at different parts of the growing season. At corn fertilization, an influx of inorganic N alters the soil environment and pH drops, which temporarily increases bacterial nitrification (this will be discussed in detail in subsection Soil pH Dictates Microbial Nitrification) and nitrous oxide emissions. As the season progresses and soil ammonium values drop, pH returns to pre fertilization levels, and bacterial nitrification decreases. The microbial N cycle in the SSS rotation behaved differently due to the neutral soil conditions and, unlike the CCC, did not experience a large peak of activity and nitrous oxide emissions. Our results show AOA having the lowest values prior to fertilization then increasing throughout the growing season (AOA will be discussed in detail in subsection Bacterial Denitrification via nirK Linked to Nitrous Oxide Emissions). Other studies have shown that fertilized row crops experience increases in microbial N cycle activity near fertilization followed by a leveling out as the growing season progresses due to an influx of inorganic N; a peak in nitrous oxide emissions following fertilization substantiate that finding (Somenahally et al., 2018; Linton et al., 2020; Hu et al., 2021; Wang et al., 2021).
Crop Rotation, Tillage, and Cover Cropping Influence the Soil Environment
Similar to a previous 4-year study at these same plots, nitrous oxide emissions from the CCC rotation were greater than the SSS due to N fertilization (Behnke et al., 2018). Results from other studies also show corn nitrous oxide emissions were greater than soybean due to fertilizer inputs (Omonode et al., 2011; Smith et al., 2011). In a global meta-analysis studying nitrous oxide emissions, Shcherbak et al. (2014) found a near-linear relationship between N rate and nitrous oxide emissions. Those authors also found that acidic soil conditions are related to greater nitrous oxide emissions. Wang et al. (2018) and Wang et al. (2021) found that low soil pH resulted in greater nitrous oxide emissions. Therefore, soil pH has been identified as an influential variable associated with microbial community composition (Behnke et al., 2021), microbial N cycle functional genes (Ouyang et al., 2018; Huang et al., 2019; Sun et al., 2019; Behnke et al., 2020b). Furthermore, soil pH was found to be correlated with numerous response variables in this study as well. In a low pH environment, as characterized by the CCC rotation, nitrous oxide emissions increase (p = 0.0365), so too does nirK copy numbers (p = 0.0603) and AOB copy numbers (p = 0.0079), whereas AOA copy numbers decrease (p = 0.0001).
Soil ammonium values were driven by strong main effects of time of sampling (month) and crop rotation (Table 2). The CCC rotation would be expected to have elevated soil ammonium values immediately following the spring UAN fertilizer application, then level off as crop uptake and microbial N transformations occur. The actively growing cover crops reduced soil nitrate through plant uptake prior to termination and slightly after but returned to no differences later in the season (Figure 2). In a Midwestern rye cover crop experiment over 10 years, (Parkin et al., 2016) found no differences between the no-cover control and the rye cover crop; however, the rye cover crop did reduce nitrate leaching by 54%. Following 5 years of cover cropping from a corn-soybean rotation, Behnke and Villamil (2019) observed that when spring cover crop biomass had favorable winter growing conditions, nitrous oxide emissions were reduced due to a significant reduction in soil nitrate values. However, post-termination GHG samples were not taken in that study.
In agreement with other studies, our results showed that actively growing cover crops decreased soil nitrate values through biomass immobilization (Acuña and Villamil, 2014; Lacey and Armstrong, 2015; Behnke and Villamil, 2019; Behnke et al., 2020a). Once the cover crops are terminated, however, the fresh residues provide the substrate needed for microbial growth and activity (Kaleeem Abbasi et al., 2015; Frasier et al., 2016; Hallama et al., 2019). Following termination, the increased microbial activity explains why cover crops increased nitrous oxide emissions in our study (Table 2). Cover crops were also found to increase nitrous oxide emissions more than half of the time, as observed in the meta-analysis by Basche et al. (2014). In a cover crop and tillage study in Illinois, USA, Kim et al. (2020b) observed that the decomposition of legume-grass cover crop species results in greater soil nitrate levels compared to grass-only covers. The authors found that the legume-grass cover crop rotations favored copiotrophic decomposers due to the higher inorganic N environment. The surplus N triggers positive priming, a situation where organic matter and residues are quickly decomposed (Norris et al., 2013; Chen et al., 2014). The low C:N ratio of the decomposing residues leads to N mineralization (Sainju et al., 2005). The stimulation of N cycling due to leguminous cover crops was also observed in a long-term cotton trial in Tennessee, USA; the authors found that hairy vetch increased N cycling genes compared to winter wheat, with effects lasting through the cotton-growing season (Hu et al., 2021). Cover cropping with cereal rye in Illinois, USA was found to have greater laboratory potential denitrification, though field scale nitrous oxide measurements had the opposite result (Foltz et al., 2021). Those authors found that rye cover crops decreased field nitrate levels and increased soil C, creating conditions that favor increases in laboratory assays (Foltz et al., 2021).
Tillage Impacts N Fixation
The abundance of nifH copy numbers was elevated under chisel tillage compared to no-till (Table 2) and drove the four-way interaction (Figure 6). In this study, soil samples were taken from the top 10 cm, so surface-applied N or crop residues (as is the case no-till) accumulate and cause ammonium levels to increase, leading to a decrease in fixation from no-till. In a continuous cotton experiment with tillage and cover crop treatments in Tennessee, USA, Hu et al. (2021) also found that overall tillage increased nifH compared to no-till. Ouyang et al. (2018) found that nifH is sensitive to soil N concentrations, and N fertilization may reduce the potential for biological N fixation. Furthermore, N fixation requires energy investments are as N becomes more abundant, fixation decreases (Gelfand and Robertson, 2015). From a trial in Iowa, soybean biomass production was the best predictor of total biological N fixation (Córdova et al., 2019). Soybean yields did improve with tillage by nearly 0.4 Mg/ha (Table 3). Similarly, soybean yields improved with tillage in a 4-year study from this site (Behnke et al., 2018).
Soil pH Dictates Microbial Nitrification
Prior to the addition of cover crops to these plots, Behnke et al. (2021) observed that bacteria, fungi, and archaea all responded to pH, creating niches for each indicator species at varying pH levels. Bacterial indicator species were grouped into acidophile and organic matter dependent vs. neutrophile and N adverse. Likewise, archaea indicator microbes were organized by pH requirements. Crop rotation and tillage practices that influence soil pH and soil organic matter were the drivers for the differences in indicator microbes (Behnke et al., 2021). A strong rotation effect drove soil pH values in our current study, with nearly one unit decrease in the CCC rotation compared to the SSS (Table 2). The month*rotation interaction for pH shows divergence on June 13th for both rotations, the CCC rotation decreased, and the SSS rotation increased slightly (Figure 4). This dip in soil pH can also be seen by the rise in AOB copy numbers in the CCC pairs that same sampling event (Figure 5). The soil pH drop (June 5th and 13th) followed the influx of fertilizer ammonium likely caused the spike in AOB copy numbers; then, as soil ammonium values decrease, so too does AOB. In this study, AOB and soil pH were negatively correlated (p = −0.0079). Other long-term studies have reported increased AOB abundance at lower soil pH (Segal et al., 2017; Huang et al., 2019; Sun et al., 2019; Behnke et al., 2020b). The increased levels of AOB in these low soil pH systems could also be due to an increase in ammonia from the UAN fertilizer application. Several studies also found that, in agricultural soils, AOB is more responsive to N fertilization (Ouyang et al., 2016, 2017; Ying et al., 2017).
Copy numbers of AOA were elevated in the unfertilized SSS rotation (Table 2), with lower soil ammonium concentrations and greater pH values (Table 2). Soil ammonia is less available at a low pH, which would normally favor the AOA community (Hatzenpichler, 2012; Zhalnina et al., 2012; Lehtovirta-Morley, 2018). However, since AOA prefers low ammonia environments, the addition of fertilizer N to a system reduces the AOA numbers, and AOB has a competitive advantage (Carey et al., 2016; Ouyang et al., 2016; Ying et al., 2017; Kim et al., 2021). This trend is observed as soil ammonium values decrease throughout the growing season (Figure 3); AOB copy numbers do as well, though they are always greater in the CCC rotation than the unfertilized SSS rotation. Chisel tillage also increased AOA copy numbers, likely due to the mixing of fertilizer N, which would cause a dilution effect since AOA prefers low ammonium environments. Other studies have observed a decrease in soil pH under no-till due to an accumulation of surface-applied fertilizer N (Crozier et al., 1999; Zuber et al., 2017; Behnke et al., 2020b). In a long-term tillage study in Nebraska, Segal et al. (2017) found that tillage did not affect AOA abundance. Similarly, in another long-term CCC study, the effect of tillage was less pronounced in the summer for AOA abundance due to a lack of competitive advantage compared to AOB (Liu et al., 2020).
Bacterial Denitrification via nirK Linked to Nitrous Oxide Emissions
Our results indicate that nitrous oxide emissions were produced by bacterial denitrification, specifically using the nirK pathway. The functional gene nirK was positively correlated with AOB (p < 0.0001) and inversely correlated with AOA (p = 0.0165), suggesting that the nirK function was bacterial. In fact, all of the microbial N cycle genes (nifH, nirK, nirS, and nosZ were strongly correlated (p < 0.0001) to AOB, indicating that the bacteria were very active in these soils, so any emissions would be influenced by bacteria. Behnke et al. (2020b) also observed AOB having a positive relationship with nirK, nirS, and nosZ. The soils with elevated nitrous oxide emissions were affected by the CCC rotation, which contained 56% more nitrous oxide compared to the SSS rotation (Table 2). As discussed earlier, the CCC rotation also contained elevated levels of AOB due to lower pH conditions and elevated levels of soil ammonium. The strong effect of crop rotation (Table 2) influenced nirK copy numbers, showing increases in the CCC rotation. Behnke et al. (2020b) also observed that nirK copy numbers were elevated in CCC and speculated that nirK was the culprit for nitrous oxide emissions. Linton et al. (2020) found that the nirK community was stimulated following input of fertilizer N in corn-soybean systems. In instances of low soil oxygen, some AOB, known to harbor nirK, could also contribute to nitrous oxide emissions (Coskun et al., 2017; Tosi et al., 2020). Other studies have shown that nirK was involved in nitrous oxide production in corn (Wang et al., 2021), cotton (Hu et al., 2021), wheat (Somenahally et al., 2018), and functionally (Graf et al., 2014). On the other hand, some studies do not show correlations between nitrous oxide emissions and N cycle genes, though they were from more diverse cropping systems (Maul et al., 2019) and biochar (Pereira et al., 2015).
Chisel tillage increased all microbial N cycle genes (nifH, nirK, nirS, and nosZ) compared to no-till (Table 2). In a study from the Kellogg Biological Station in Michigan, each denitrification gene was also reduced in the no-till treatment compared to conventional tillage (Li and Cupples, 2021). Chisel tillage is used to help break down residues, which stimulates microbial activity through aeration and warmer soil conditions (Balesdent et al., 2000; Martens, 2000; Hu et al., 2021). In the meta-analysis by Zuber and Villamil (2016), the authors found that metabolic quotient, a proxy for general microbial activity, was elevated with tillage compared to no-till. Other studies have observed elevated levels of nifH and nirS (Hu et al., 2021); nirK and nirS (Tatti et al., 2015; Wang et al., 2019); nirS, nirK, and nosZ in tilled systems compared to no-till. In a long-term tillage study on wheat in China, Wang et al. (2021) found that conservation tillage decreased the abundance of genes involved in nitrous oxide production, nirK and nirS, but not nosZ. The authors found that nirK and nirS were likely to cause nitrous oxide production in conventionally tilled systems.
Conclusions
The results presented here offer a unique look into the seasonal dynamics that soil microbes experience throughout a typical growing season. Nitrous oxide emissions and ammonia oxidizing bacteria copy numbers were greater in the CCC rotation fundamentally due to acidic soil conditions, confirming our hypothesis. Of the four microbial N cycle genes, only nirK was affected by crop rotation, with elevated copy numbers in the CCC rotation, as well. Soil pH was negatively correlated with nirK, ammonia oxidizing bacteria, and nitrous oxide emissions. Our findings indicate that bacterial nirK denitrification is likely responsible for the elevated nitrous oxide emissions in our study. Cover crops were found to increase nitrous oxide emissions, which was contrary to our hypothesis; however, cover crops did reduce soil nitrate levels, which we did predict. Cover cropping was involved as part of interactions with microbial N cycle genes, though they were found not to be a significant driver of those interactions upon further investigation. We agree with the conclusion in Hu et al. (2021), microbial-driven nitrous oxide emissions are situational and determined by localities, such as soil properties and the environment. In systems where corn follows corn, greater N fertilizer amounts are needed to sustain high yields. These systems are characterized by large nitrous oxide emissions and excess soil N, leading to an acidic environment that favors bacterial nitrification and denitrification via nirK. Understanding the microbial dynamics behind typical agronomic practices that influence nitrous oxide emissions is critical in identifying mitigation strategies. Our work highlights the complex and temporal effect agricultural management has on key microbial functions related to the N cycle, adding to our understanding of soil biology and biogeochemical processes from an agricultural setting.
Data Availability Statement
The raw data supporting the conclusions of this article will be made available by the authors, without undue reservation.
Author Contributions
GB and MV: conceptualization, data curation, writing—original draft preparation, supervision, project administration, and funding acquisition. GB, NK, CR, MZ, and SR-Z: methodology. GB, NK, and MV: formal analysis. MV, SR-Z, and CR: resources. NK, CR, SR-Z, and MZ: writing—review and editing. All authors have read and agreed to the published version of the manuscript.
Funding
This research was primarily funded by Award No. 2019-67012-29525 from the United States Department of Agriculture, USDA-NIFA AFRI Post-doctoral Fellowships, and partially funded by a HATCH Grant (no. ILLU-802- 978) and by Award No. AG 2018-67019-27807 both from the United States Department of Agriculture, USDA-NIFA.
Conflict of Interest
The authors declare that the research was conducted in the absence of any commercial or financial relationships that could be construed as a potential conflict of interest.
Publisher's Note
All claims expressed in this article are solely those of the authors and do not necessarily represent those of their affiliated organizations, or those of the publisher, the editors and the reviewers. Any product that may be evaluated in this article, or claim that may be made by its manufacturer, is not guaranteed or endorsed by the publisher.
Acknowledgments
We acknowledge Dr. Alvaro Hernandez and Dr. Mark Band from the Roy Carver Biotechnology Center for Functional Genomics lab at the University of Illinois at Urbana-Champaign for their assistance in creating the amplicon libraries. We are thankful to Dr. Greg Steckel and Mr. Marty Johnson for their contribution in managing the experimental plots.
Supplementary Material
The Supplementary Material for this article can be found online at: https://www.frontiersin.org/articles/10.3389/fagro.2022.833338/full#supplementary-material
Supplementary Figure S1. Top panel: Monthly precipitation (mm) totals for 2019 (red) and the historical average (1989-2019, black). Error bars for the historical average represent standard errors of the 30-year mean. Bottom panel: Monthly temperature averages (°C) for 2019 (red) and the historical average (1989-2019, black). Error bars above each main bar represent standard errors of the mean.
References
Acuña, J. C. M., and Villamil, M. B. (2014). Short-term effects of cover crops and compaction on soil properties and soybean production in Illinois. Agron. J. 106, 860–870. doi: 10.2134/agronj13.0370
Aulakh, M., Walters, D., Doran, J., Francis, D., and Mosier, A. (1991). Crop residue type and placement effects on denitrification and mineralization. Soil Sci. Soc. Am. J. 55, 1020–1025. doi: 10.2136/sssaj1991.03615995005500040022x
Balesdent, J., Chenu, C., and Balabane, M. (2000). Relationship of soil organic matter dynamics to physical protection and tillage. Soil Tillage Res. 53, 215–230. doi: 10.1016/S0167-1987(99)00107-5
Basche, A. D., Miguez, F. E., Kaspar, T. C., and Castellano, M. J. (2014). Do cover crops increase or decrease nitrous oxide emissions? A meta-analysis. J. Soil Water Conserv. 69, 471–482. doi: 10.2489/jswc.69.6.471
Basu, S., Kumar, G., Chhabra, S., and Prasad, R. (2021). “Chapter 13 - Role of soil microbes in biogeochemical cycle for enhancing soil fertility,” in New and Future Developments in Microbial Biotechnology and Bioengineering, eds J. P. Verma, C. A. Macdonald, V. K. Gupta, and A. R. Podile (Amsterdam: Elsevier), 149–157. doi: 10.1016/B978-0-444-64325-4.00013-4
Behnke, G. D., Kim, N., and Villamil, M. B. (2020a). Agronomic assessment of cover cropping and tillage practices across environments. Agron. J. 112, 3913–3928. doi: 10.1002/agj2.20337
Behnke, G. D., Kim, N., Zabaloy, M. C., Riggins, C. W., Rodriguez-Zas, S., and Villamil, M. B. (2021). Soil microbial indicators within rotations and tillage systems. Microorganisms 9, 1244. doi: 10.3390/microorganisms9061244
Behnke, G. D., and Villamil, M. B. (2019). Cover crop rotations affect greenhouse gas emissions and crop production in Illinois, USA. Field Crops Res. 241, 107580. doi: 10.1016/j.fcr.2019.107580
Behnke, G. D., Zabaloy, M. C., Riggins, C., Rodriguez-Zas, S., Huang, L., and Villamil, M. (2020b). Acidification in corn monocultures favor fungi, ammonia oxidizing bacteria, and nirK-denitrifier groups. Sci. Total Environ. 720, 137514. doi: 10.1016/j.scitotenv.2020.137514
Behnke, G. D., Zuber, S. M., Pittelkow, C. M., Nafziger, E. D., and Villamil, M. B. (2018). Long-term crop rotation and tillage effects on soil greenhouse gas emissions and crop production in Illinois, USA. Agric. Ecosyst. Environ. 261, 62–70. doi: 10.1016/j.agee.2018.03.007
Blagodatskaya, E., and Kuzyakov, Y. (2008). Mechanisms of real and apparent priming effects and their dependence on soil microbial biomass and community structure: critical review. Biol. Fertil. Soils 45, 115–131. doi: 10.1007/s00374-008-0334-y
Carey, C. J., Dove, N. C., Beman, J. M., Hart, S. C., and Aronson, E. L. (2016). Meta-analysis reveals ammonia-oxidizing bacteria respond more strongly to nitrogen addition than ammonia-oxidizing archaea. Soil Biol. Biochem. 99, 158–166. doi: 10.1016/j.soilbio.2016.05.014
Chen, R., Senbayram, M., Blagodatsky, S., Myachina, O., Dittert, K., Lin, X., et al. (2014). Soil C and N availability determine the priming effect: microbial N mining and stoichiometric decomposition theories. Glob. Chang. Biol. 20, 2356–2367. doi: 10.1111/gcb.12475
Congreves, K., Hooker, D., Hayes, A., Verhallen, E., and Van Eerd, L. (2017). Interaction of long-term nitrogen fertilizer application, crop rotation, and tillage system on soil carbon and nitrogen dynamics. Plant Soil 410, 113–127. doi: 10.1007/s11104-016-2986-y
Córdova, S. C., Castellano, M. J., Dietzel, R., Licht, M. A., Togliatti, K., Martinez-Feria, R., et al. (2019). Soybean nitrogen fixation dynamics in Iowa, USA. Field Crops Res. 236, 165–176. doi: 10.1016/j.fcr.2019.03.018
Coskun, D., Britto, D. T., Shi, W., and Kronzucker, H. J. (2017). Nitrogen transformations in modern agriculture and the role of biological nitrification inhibition. Nature Plants 3, 1–10. doi: 10.1038/nplants.2017.74
Crozier, C. R., Naderman, G. C., Tucker, M. R., and Sugg, R. E. (1999). Nutrient and pH stratification with conventional and no-till management. Commun. Soil Sci. Plant Anal. 30, 65–74. doi: 10.1080/00103629909370184
De Graaff, M.-A., Hornslein, N., Throop, H. L., Kardol, P., and Van Diepen, L. T. A. (2019). “Chapter one - effects of agricultural intensification on soil biodiversity and implications for ecosystem functioning: a meta-analysis,” in Advances in Agronomy, ed D. L. Sparks (Amsterdam: Academic Press), 1–44. doi: 10.1016/bs.agron.2019.01.001
Dozier, I. A., Behnke, G. D., Davis, A. S., Nafziger, E. D., and Villamil, M. B. (2017). Tillage and cover cropping effects on soil properties and crop production in Illinois. Agron. J. 1261–1270. doi: 10.2134/agronj2016.10.0613
EPA (2016). Inventory of US Greenhouse Gas Emissions and Sinks: 1990-2014. Washington, DC: EPA 430-R-16-002.
Erisman, J. W., Sutton, M. A., Galloway, J., Klimont, Z., and Winiwarter, W. (2008). How a century of ammonia synthesis changed the world. Nat. Geosci. 1, 636–639. doi: 10.1038/ngeo325
Fierer, N. (2017). Embracing the unknown: disentangling the complexities of the soil microbiome. Nat. Rev. Microbiol. 15, 579. doi: 10.1038/nrmicro.2017.87
Fierer, N., and Jackson, R. B. (2006). The diversity and biogeography of soil bacterial communities. Proc. Nat. Acad. Sci. U.S.A. 103, 626–631. doi: 10.1073/pnas.0507535103
Foltz, M. E., Kent, A. D., Koloutsou-Vakakis, S., and Zilles, J. L. (2021). Influence of rye cover cropping on denitrification potential and year-round field N2O emissions. Sci. Total Environ. 765, 144295. doi: 10.1016/j.scitotenv.2020.144295
Frasier, I., Noellemeyer, E., Figuerola, E., Erijman, L., Permingeat, H., and Quiroga, A. (2016). High quality residues from cover crops favor changes in microbial community and enhance C and N sequestration. Glob. Ecol. Conserv. 6, 242–256. doi: 10.1016/j.gecco.2016.03.009
Gbur, E., Stroup, W., Mccarter, K., Durham, S., Young, L., Christman, M., et al. (2012). Analysis of Generalized Linear Mixed Models in the Agricultural and Natural Resource Sciences. American Society of Agronomy. Madison, WI: Soil Science Society of America
Gelfand, I., and Robertson, G. P. (2015). A reassessment of the contribution of soybean biological nitrogen fixation to reactive N in the environment. Biogeochemistry 123, 175–184. doi: 10.1007/s10533-014-0061-4
Graf, D. R., Jones, C. M., and Hallin, S. (2014). Intergenomic comparisons highlight modularity of the denitrification pathway and underpin the importance of community structure for N2O emissions. PLoS ONE 9, e114118. doi: 10.1371/journal.pone.0114118
Hallama, M., Pekrun, C., Lambers, H., and Kandeler, E. (2019). Hidden miners-the roles of cover crops and soil microorganisms in phosphorus cycling through agroecosystems. Plant Soil 434, 7–45. doi: 10.1007/s11104-018-3810-7
Hatfield, J. (2012). “Agriculture in the midwest,” in U.S. National Climate Assessment Midwest Technical Input Report (Ames, IA: Great Lakes Integrated Sciences and Assessments (GLISA) Center). Available online at: http://glisa.msu.edu/docs/NCA/MTIT_Agriculture.pdf (accessed November 11, 2021).
Hatzenpichler, R. (2012). Diversity, physiology, and niche differentiation of ammonia-oxidizing archaea. Appl. Environ. Microbiol. 78, 7501–7510. doi: 10.1128/AEM.01960-12
Hu, J., Jin, V. L., Konkel, J. Y. M., Schaeffer, S. M., Schneider, L. G., Debruyn, J. M., et al. (2021). Soil health management enhances microbial nitrogen cycling capacity and activity. mSphere 6, e01237–e01220. doi: 10.1128/mSphere.01237-20
Huang, L., Riggins, C. W., Rodriguez-Zas, S., Zabaloy, M. C., and Villamil, M. B. (2019). Long-term N fertilization imbalances potential N acquisition and transformations by soil microbes. Sci. Total Environ. 691, 562–571. doi: 10.1016/j.scitotenv.2019.07.154
Illinois-Climate-Network (2021). Water and Atmospheric Resources Monitoring Program. Champaign, IL: Illinois State Water Survey.
Jetten, M. S. M. (2008). The microbial nitrogen cycle. Environ. Microbiol. 10, 2903–2909. doi: 10.1111/j.1462-2920.2008.01786.x
Kaleeem Abbasi, M., Mahmood Tahir, M., Sabir, N., and Khurshid, M. (2015). Impact of the addition of different plant residues on nitrogen mineralization-immobilization turnover and carbon content of a soil incubated under laboratory conditions. Solid Earth 6, 197–205. doi: 10.5194/se-6-197-2015
Kim, N., Riggins, C., Rodriguez-Zas, S., Zabaloy, M. C., and Villamil, M. B. (2021). Long-term residue removal under tillage decreases amoA-nitrifiers and stimulates nirS-denitrifier groups in the soil. Appl. Soil Ecol. 157, 103730. doi: 10.1016/j.apsoil.2020.103730
Kim, N., Zabaloy, M. C., Guan, K., and Villamil, M. B. (2020a). Do cover crops benefit soil microbiome? A meta-analysis of current research. Soil Biol. Biochem. 142, 107701. doi: 10.1016/j.soilbio.2019.107701
Kim, N., Zabaloy, M. C., Riggins, C. W., Rodríguez-Zas, S., and Villamil, M. B. (2020b). Microbial shifts following five years of cover cropping and tillage practices in fertile agroecosystems. Microorganisms 8, 1773. doi: 10.3390/microorganisms8111773
Kladivko, E. J., Kaspar, T. C., Jaynes, D. B., Malone, R. W., Singer, J., Morin, X. K., et al. (2014). Cover crops in the upper midwestern United States: potential adoption and reduction of nitrate leaching in the Mississippi River Basin. J. Soil Water Conserv. 69, 279–291. doi: 10.2489/jswc.69.4.279
Kuzyakov, Y. (2010). Priming effects: interactions between living and dead organic matter. Soil Biol. Biochem. 42, 1363–1371. doi: 10.1016/j.soilbio.2010.04.003
Lacey, C., and Armstrong, S. (2015). The efficacy of winter cover crops to stabilize soil inorganic nitrogen after fall-applied anhydrous ammonia. J. Environ. Qual. 44, 442–448. doi: 10.2134/jeq2013.12.0529
Lehtovirta-Morley, L. E. (2018). Ammonia oxidation: ecology, physiology, biochemistry and why they must all come together. FEMS Microbiol. Lett. 365, 1–9. doi: 10.1093/femsle/fny058
Levy-Booth, D. J., Prescott, C. E., and Grayston, S. J. (2014). Microbial functional genes involved in nitrogen fixation, nitrification and denitrification in forest ecosystems. Soil Biol. Biochem. 75, 11–25. doi: 10.1016/j.soilbio.2014.03.021
Li, Z., and Cupples, A. M. (2021). Diversity of nitrogen cycling genes at a Midwest long-term ecological research site with different management practices. Appl. Microbiol. Biotechnol. 105, 4309–4327. doi: 10.1007/s00253-021-11303-0
Linton, N. F., Ferrari Machado, P. V., Deen, B., Wagner-Riddle, C., and Dunfield, K. E. (2020). Long-term diverse rotation alters nitrogen cycling bacterial groups and nitrous oxide emissions after nitrogen fertilization. Soil Biol. Biochem. 149, 107917. doi: 10.1016/j.soilbio.2020.107917
Liu, S., Coyne, M., Grove, J., and Flythe, M. (2020). Tillage, not fertilization, dominantly influences ammonia-oxidizing archaea diversity in long-term, continuous maize. Appl. Soil Ecol. 147, 103384. doi: 10.1016/j.apsoil.2019.103384
Livak, K. J., and Schmittgen, T. D. (2001). Analysis of relative gene expression data using real-time quantitative PCR and the 2–ΔΔCt method. Methods 25, 402–408. doi: 10.1006/meth.2001.1262
Martens, D. A. (2000). Management and crop residue influence soil aggregate stability. J. Environ. Qual. 29, 723–727. doi: 10.2134/jeq2000.00472425002900030006x
Maul, J. E., Cavigelli, M. A., Vinyard, B., and Buyer, J. S. (2019). Cropping system history and crop rotation phase drive the abundance of soil denitrification genes nirK, nirS and nosZ in conventional and organic grain agroecosystems. Agric. Ecosyst. Environ. 273, 95–106. doi: 10.1016/j.agee.2018.11.022
Mcdaniel, M. D., Tiemann, L. K., and Grandy, A. S. (2014). Does agricultural crop diversity enhance soil microbial biomass and organic matter dynamics? A meta-analysis. Ecol. Appl. 24, 560–570. doi: 10.1890/13-0616.1
Mclean, E. (1983). “Soil pH and lime requirement,” in Methods of Soil Analysis: Part 2 Chemical and Microbiological Properties, ed A. L. Page (Madison: Soil Science Society of America), 199–224.
Millar, N., Robertson, G. P., Grace, P. R., Gehl, R. J., and Hoben, J. P. (2010). Nitrogen fertilizer management for nitrous oxide (N2O) mitigation in intensive corn (Maize) production: an emissions reduction protocol for US Midwest agriculture. Mitig. Adapt. Strategies Glob Change 15, 185–204. doi: 10.1007/s11027-010-9212-7
Moreau, D., Bardgett, R. D., Finlay, R. D., Jones, D. L., and Philippot, L. (2019). A plant perspective on nitrogen cycling in the rhizosphere. Funct. Ecol. 33, 540–552. doi: 10.1111/1365-2435.13303
Myrold, D. D. (2021). “15 - Transformations of nitrogen,” in Principles and Applications of Soil Microbiology (Third Edition), eds T. J. Gentry, J. J. Fuhrmann, and D. A. Zuberer (Amsterdam: Elsevier), 385–421. doi: 10.1016/B978-0-12-820202-9.00015-0
Norris, M. D., Avis, P. G., Reich, P. B., and Hobbie, S. E. (2013). Positive feedbacks between decomposition and soil nitrogen availability along fertility gradients. Plant Soil 367, 347–361. doi: 10.1007/s11104-012-1449-3
Norton, J., and Ouyang, Y. (2019). Controls and adaptive management of nitrification in agricultural soils. Front. Microbiol. 10, 1931. doi: 10.3389/fmicb.2019.01931
Norton, J. M. (2008). Nitrification in agricultural soils. Agron. J. 49, 173. doi: 10.2134/agronmonogr49.c6
Omonode, R. A., Smith, D. R., Gál, A., and Vyn, T. J. (2011). Soil nitrous oxide emissions in corn following three decades of tillage and rotation treatments. Soil Sci. Soc. Am. J. 75, 152–163. doi: 10.2136/sssaj2009.0147
Orellana, L. H., Chee-Sanford, J. C., Sanford, R. A., Löffler, F. E., Konstantinidis, K. T., and Elliot, M. A. (2018). Year-round shotgun metagenomes reveal stable microbial communities in agricultural soils and novel ammonia oxidizers responding to fertilization. Appl. Environ. Microbiol. 84, e01646–e01617. doi: 10.1128/AEM.01646-17
Ouyang, Y., Evans, S. E., Friesen, M. L., and Tiemann, L. K. (2018). Effect of nitrogen fertilization on the abundance of nitrogen cycling genes in agricultural soils: a meta-analysis of field studies. Soil Biol. Biochem. 127, 71–78. doi: 10.1016/j.soilbio.2018.08.024
Ouyang, Y., Norton, J. M., and Stark, J. M. (2017). Ammonium availability and temperature control contributions of ammonia oxidizing bacteria and archaea to nitrification in an agricultural soil. Soil Biol. Biochem. 113, 161–172. doi: 10.1016/j.soilbio.2017.06.010
Ouyang, Y., Norton, J. M., Stark, J. M., Reeve, J. R., and Habteselassie, M. Y. (2016). Ammonia-oxidizing bacteria are more responsive than archaea to nitrogen source in an agricultural soil. Soil Biol. Biochem. 96, 4–15. doi: 10.1016/j.soilbio.2016.01.012
Parkin, T. B., Kaspar, T. C., Jaynes, D. B., and Moorman, T. B. (2016). Rye cover crop effects on direct and indirect nitrous oxide emissions. Soil Sci. Soc. Am. J. 80, 1551–1559. doi: 10.2136/sssaj2016.04.0120
Parkin, T. B., and Venterea, R. T. (2010). “USDA-ARS GRACEnet project protocols, chapter 3. chamber-based trace gas flux measurements,” in Sampling Protocols (Beltsville, MD), 1–39.
Paustian, K., Lehmann, J., Ogle, S., Reay, D., Robertson, G. P., and Smith, P. (2016). Climate-smart soils. Nature 532, 49–57. doi: 10.1038/nature17174
Pereira, E. I. P., Suddick, E. C., Mansour, I., Mukome, F. N. D., Parikh, S. J., Scow, K., et al. (2015). Biochar alters nitrogen transformations but has minimal effects on nitrous oxide emissions in an organically managed lettuce mesocosm. Biol. Fertil. Soils 51, 573–582. doi: 10.1007/s00374-015-1004-5
Prosser, J. I., Hink, L., Gubry-Rangin, C., and Nicol, G. W. (2020). Nitrous oxide production by ammonia oxidizers: physiological diversity, niche differentiation and potential mitigation strategies. Glob. Chang. Biol. 26, 103–118. doi: 10.1111/gcb.14877
Ray, P., Lakshmanan, V., Labb,é, J. L., and Craven, K. D. (2020). Microbe to microbiome: a paradigm shift in the application of microorganisms for sustainable agriculture. Front. Microbiol. 11, 622926. doi: 10.3389/fmicb.2020.622926
Robertson, G. P., and Groffman, P .M. (2007). “Nitrogen transformations,” in Soil Microbiology, Ecology and Biochemistry, 3rd Edn, ed. E. A. Paul. (San Diego, CA: Academic Press), 341–364.
Sainju, U. M., Whitehead, W. F., and Singh, B. P. (2005). Biculture legume-cereal cover crops for enhanced biomass yield and carbon and nitrogen. Agron. J. 97, 1403–1412. doi: 10.2134/agronj2004.0274
Segal, L. M., Miller, D. N., Mcghee, R. P., Loecke, T. D., Cook, K. L., Shapiro, C. A., et al. (2017). Bacterial and archaeal ammonia oxidizers respond differently to long-term tillage and fertilizer management at a continuous maize site. Soil Tillage Res. 168, 110–117. doi: 10.1016/j.still.2016.12.014
Shcherbak, I., Millar, N., and Robertson, G. P. (2014). Global metaanalysis of the nonlinear response of soil nitrous oxide (N < sub>2 < /sub>O) emissions to fertilizer nitrogen. Proc. Nat. Acad. Sci. U.S.A. 111, 9199–9204. doi: 10.1073/pnas.1322434111
Smith, D. R., Hernandez-Ramirez, G., Armstrong, S. D., Bucholtz, D. L., and Stott, D. E. (2011). Fertilizer and tillage management impacts on non-carbon-dioxide greenhouse gas emissions. Soil Sci. Soc. Am. J. 75, 1070–1082. doi: 10.2136/sssaj2009.0354
Snyder, C., Bruulsema, T., Jensen, T., and Fixen, P. (2009). Review of greenhouse gas emissions from crop production systems and fertilizer management effects. Agric. Ecosyst. Environ. 133, 247–266. doi: 10.1016/j.agee.2009.04.021
Somenahally, A., Dupont, J. I., Brady, J., Mclawrence, J., Northup, B., and Gowda, P. (2018). Microbial communities in soil profile are more responsive to legacy effects of wheat-cover crop rotations than tillage systems. Soil Biol. Biochem. 123, 126–135. doi: 10.1016/j.soilbio.2018.04.025
Sun, R., Myrold, D. D., Wang, D., Guo, X., and Chu, H. (2019). AOA and AOB communities respond differently to changes of soil pH under long-term fertilization. Soil Ecol. Lett. 1, 126–135. doi: 10.1007/s42832-019-0016-8
Tatti, E., Goyer, C., Burton, D. L., Wertz, S., Zebarth, B. J., Chantigny, M., et al. (2015). Tillage management and seasonal effects on denitrifier community abundance, gene expression and structure over winter. Microb. Ecol. 70, 795–808. doi: 10.1007/s00248-015-0591-x
Tosi, M., Brown, S., Machado, P. V. F., Wagner-Riddle, C., and Dunfield, K. (2020). Short-term response of soil N-cycling genes and transcripts to fertilization with nitrification and urease inhibitors, and relationship with field-scale N2O emissions. Soil Biol. Biochem. 142, 107703. doi: 10.1016/j.soilbio.2019.107703
Venterea, R. T., Bijesh, M., and Dolan, M. S. (2011). Fertilizer source and tillage effects on yield-scaled nitrous oxide emissions in a corn cropping system. J. Environ. Qual. 40, 1521–1531. doi: 10.2134/jeq2011.0039
Venterea, R. T., Burger, M., and Spokas, K. A. (2005). Nitrogen oxide and methane emissions under varying tillage and fertilizer management. J. Environ. Qual. 34, 1467–1477. doi: 10.2134/jeq2005.0018
Wang, W., Hou, Y., Pan, W., Vinay, N., Mo, F., Liao, Y., et al. (2021). Continuous application of conservation tillage affects in situ N2O emissions and nitrogen cycling gene abundances following nitrogen fertilization. Soil Biol. Biochem. 157, 108239. doi: 10.1016/j.soilbio.2021.108239
Wang, W., Yang, M., Shen, P., Zhang, R., Qin, X., Han, J., et al. (2019). Conservation tillage reduces nitrous oxide emissions by regulating functional genes for ammonia oxidation and denitrification in a winter wheat ecosystem. Soil Tillage Res. 194, 104347. doi: 10.1016/j.still.2019.104347
Wang, Y., Guo, J., Vogt, R. D., Mulder, J., Wang, J., and Zhang, X. (2018). Soil pH as the chief modifier for regional nitrous oxide emissions: new evidence and implications for global estimates and mitigation. Glob. Chang. Biol. 24, e617–e626. doi: 10.1111/gcb.13966
Web-Soil-Survey (2021). Available online at: https://websoilsurvey.sc.egov.usda.gov/App/HomePage.htm (accessed October 14, 2019)
West, T. O., and Post, W. M. (2002). Soil organic carbon sequestration rates by tillage and crop rotation: a global data analysis. Soil Sci. Soc. Am. J. 66, 1930–1946. doi: 10.2136/sssaj2002.1930
Williams, A., Davis, A. S., Ewing, P. M., Grandy, A. S., Kane, D. A., Koide, R. T., et al. (2016). Precision control of soil nitrogen cycling via soil functional zone management. Agric. Ecosyst. Environ. 231, 291–295. doi: 10.1016/j.agee.2016.07.010
Ying, J., Li, X., Wang, N., Lan, Z., He, J., and Bai, Y. (2017). Contrasting effects of nitrogen forms and soil pH on ammonia oxidizing microorganisms and their responses to long-term nitrogen fertilization in a typical steppe ecosystem. Soil Biol. Biochem. 107, 10–18. doi: 10.1016/j.soilbio.2016.12.023
Zhalnina, K., Dörr De Quadros, P., Ao Camargo, F., and Triplett, E. W. (2012). Drivers of archaeal ammonia-oxidizing communities in soil. Front. Microbiol. 3, 210. doi: 10.3389/fmicb.2012.00210
Zhu, X., Burger, M., Doane, T. A., and Horwath, W. R. (2013). Ammonia oxidation pathways and nitrifier denitrification are significant sources of N2O and NO under low oxygen availability. Proc. Nat. Acad. Sci. U.S.A. 110, 6328–6333. doi: 10.1073/pnas.1219993110
Zuber, S. M., Behnke, G. D., Nafziger, E. D., and Villamil, M. B. (2017). Multivariate assessment of soil quality indicators for crop rotation and tillage in Illinois. Soil Tillage Res. 174, 147–155. doi: 10.1016/j.still.2017.07.007
Keywords: nitrous oxide, denitrification, no-till, corn, soybean, ammonia oxidizing archaea, ammonia oxidizing bacteria, nirK
Citation: Behnke GD, Kim N, Riggins CW, Zabaloy MC, Rodriguez-Zas SL and Villamil MB (2022) A Longitudinal Study of the Microbial Basis of Nitrous Oxide Emissions Within a Long-Term Agricultural Experiment. Front. Agron. 4:833338. doi: 10.3389/fagro.2022.833338
Received: 11 December 2021; Accepted: 25 February 2022;
Published: 22 March 2022.
Edited by:
Lydiah Gatere, Independent Researcher, Nairobi, KenyaReviewed by:
Jinyang Wang, Nanjing Agricultural University, ChinaEduardo Mariano, University of São Paulo, Brazil
Copyright © 2022 Behnke, Kim, Riggins, Zabaloy, Rodriguez-Zas and Villamil. This is an open-access article distributed under the terms of the Creative Commons Attribution License (CC BY). The use, distribution or reproduction in other forums is permitted, provided the original author(s) and the copyright owner(s) are credited and that the original publication in this journal is cited, in accordance with accepted academic practice. No use, distribution or reproduction is permitted which does not comply with these terms.
*Correspondence: Gevan D. Behnke, Z2JlaG5rZTImI3gwMDA0MDtpbGxpbm9pcy5lZHU=; María B. Villamil, dmlsbGFtaWwmI3gwMDA0MDtpbGxpbm9pcy5lZHU=