- 1College of Land and Environment, Shenyang Agricultural University, Shenyang, China
- 2State Key Laboratory of North China Crop Improvement and Regulation, Mountain Area Research Institute, Hebei Agricultural University, Hebei, China
- 3Innovation Centre for Efficient Use of Nitrogen Fertilizer, China Nitrogen Fertilizer Industry (Xinlianxin) Technology Research Center, Henan Xinlianxin Chemical Industry Group Co., Ltd., Xinxiang, China
- 4Soil Science and Plant Nutrition, UWA School of Agriculture and Environment, The University of Western Australia, Perth, WA, Australia
- 5Institute for Adriatic Crops and Karst Reclamation, Split, Croatia
Returning straw into soil could increase soil organic carbon (SOC) and promote crop growth. However, little has been reported on the source of C for increased SOC (straw C or crop photosynthetic C). To investigate the assimilation of photosynthetic C and its distribution in soil in the maize growth season, we set up a 1-year 13C pulse-labeling experiment in a consecutive maize-straw-returning long-term trial. Four treatments were included: no straw return (control), straw mulching on the soil surface (cover), return in 0–20 cm layer (shallow), and 20–40 cm layer (deep). We found that the deep straw incorporation significantly (P < 0.05) increased maize 100-grain weight (by 7.8%), yield in the coming year (by 10.5%), and SOC (by 13.4%) compared with the control. During the growing season, the deep straw incorporation increased photosynthetic 13C assimilation in shoots by 17.4% and the partitioning of photosynthetic 13C to soil by 7.9% at early jointing, and by 11.5% at maturity. The contribution of photosynthetic C to microbial biomass C (MBC) and dissolved organic C (DOC) was highest at jointing, and at harvest amounted to 39.1 % of MBC and 28.8% of DOC. The results highlighted the importance of regulating the soil carbon dynamics via the deep straw return strategy. In conclusion, deep straw incorporation significantly increased photosynthetic efficiency and facilitated partitioning of photosynthetic C to roots and soil, thus promoting maize growth and yield.
Introduction
China produces more than 800 million tons of crop straw annually, accounting for about 30% of the world's total straw production (Bi et al., 2009), and the amount is still growing at a net rate of 12.5 million tons per year (Xia et al., 2014). The straw contains considerable amounts of nitrogen (N), phosphorus (P), potassium (K), and other nutrient resources, which are equal to 40% of the national fertilizer consumption (Xu et al., 2016; Jia et al., 2018). In addition, crop straw is an important component of the C cycle. Alterations in soil C pools influence plant growth and development, soil fertility, and nutrient cycling. However, the partitioning of photosynthetic C to roots, as well as to soil, remains poorly understood due to the complexity of the soil organic C pools.
Straw return has been an important tillage practice in China. Straw return increases the content of soil organic C (SOC), thereby improving soil quality (Cong et al., 2019). It is estimated that around 0.6–1.2 billion tons of C is sequestered into soil each year through straw return (Lal, 2009). Therefore, it is of great significance to explore the optimal use of straw for improving soil structure and quality as well as crop yield. Traditionally, straw is used mostly as soil surface mulching (cover) to increase soil moisture and crop yield (Yan et al., 2007; Qin et al., 2015; Li et al., 2020). Many early studies showed that straw surface-mulching increased soil organic carbon (SOC), crop yield and water-use efficiency (Tao et al., 2015; Yang et al., 2018; Xiao et al., 2019), but straw surface-mulching may have some negative effects on the crops in the subsequent seasons, e.g., increased incidence of pests and diseases (Dinardo-Miranda and Fracasso, 2013) and increased greenhouse gas emissions (Knoblauch et al., 2011). Thus, it is important to study better ways of returning straw to fields. Compared with surface straw-mulching, return of crushed straw in deep soil layers is more effective in improving soil physical and chemical properties (Zou et al., 2014; Li et al., 2021b). However, little has been reported on the effects of various depths of straw return on crop growth and yield due to, at least partly, a lack of appropriate measurement methods.
Soil organic C is an important C pool in the global C balance. Photosynthetic C assimilated by plants enters the soil in the form of plant residues and root secretions. Photosynthetic C would contribute to various organic C pools, including dissolved organic C (DOC) and microbial biomass C (MBC). Thus, photosynthetic C, as the hub of the C cycle in the atmosphere-plant-soil-microbe system, is closely related to the circulation of C between the soil organic C pool and the atmospheric environment (Yevdokimov et al., 2006). Microbial biomass C accounts for only 1–3% of soil organic C, and a much smaller proportion of the total soil C (Nie et al., 2012). Decomposition of SOC is closely linked to the dynamics of soil MBC as an indicator of soil activity. However, there are only few studies on the turnover and dynamics of soil as influenced by straw return (An T. T. et al., 2015), especially regarding different depths of straw addition. The dynamics of soil organic C is influenced by the interaction among plants, soil, and microorganisms, and is the main research topic in soil C sequestration. Thus, studying the effects of straw return to soil on the distribution of photosynthetic C and soil C pools is of great significance to the global C cycle and soil C sequestration. However, little has been reported on the effects of straw return on C partitioning in the soil-maize system. The C source (straw C or crop photosynthetic C) that contributes to increased SOC remains unclear.
Thus, the objectives of this study were: (1) to characterize the effects of straw return to various soil depths on maize growth and grain yield; (2) to determine changes in the photosynthetic C partitioning in maize shoots, roots, grains, SOC, DOC, and MBC; and (3) to elucidate temporal dynamics of 13C partitioning in the maize-soil system. In this study, we used in-situ 13C pulse-labeling to trace the fate of photosynthesized C in the plant-soil-microbe system and quantify the contribution of the newly fixed C to soil organic C pools. We hypothesized that deep straw return: (1) would result in increased C sequestration in soil via improved root and shoot growth; and (2) would increase soil organic C and microbial activity, thereby enhancing maize growth and grain yield.
Materials and Methods
Study Site
This study was conducted at the research station of Shenyang Agricultural University (41°31′ N-123°24′ E), Liaoning province, China, from May 12th to September 22nd, 2018. This field was uncultivated land until growing maize in 2011, and began straw returning from 2012. The soil type at the site is typical brown loamy soil. The site has a temperate semi-humid continental climate. The annual temperature ranged between 6.2 and 9.7°C, and the annual rainfall was between 584 and 692 mm. Frost-free period was around 135 days per year, and the effective accumulated temperature was 32.00°C. Maize with one harvest per year is the main cropping system in the local area, and straw mulching without irrigation is the predominant agricultural practice. Air temperature and humidity in 2018 were shown in Supplementary Figure 1. The basic physical and chemical properties of the tested soil are shown in Table 1; they were determined by the methods specified by Bao (2001).
Experimental Design
Since 2012, air-dried and chopped maize straw (average length 3 cm; C:N = 75:1) from the preceding maize plants on the same research station was returned to field at 28,000 kg/ha in the autumn (year before the experiment took place) at the soil surface (cover), 0–20 cm (shallow), or 20–40 cm (deep), with the control treatment having no straw returned. The experiment was carried out in the field micro-plots (2.4 × 1.1 m), with eight treatments (labeled and non-labeled sets of four treatments specified above) in three replicates, giving 24 plots in total, in a randomized complete block design. The labeled and non-labeled treatments were set apart by more than 10 m to avoid the interference. The straw was manually mixed with soil at 0–20 cm for the shallow treatment. In the deep-return treatment, 0–20 cm surface soil was removed, the straw was manually mixed with 20–40 cm soil, and then 0–20 cm surface soil was returned. During maize growth, no obvious pest and disease were noted, and the weeds were removed manually. No irrigation water was supplied based on the local practice.
The amount of N, P, and K fertilizers applied was based on the standard farming practice for growing maize in the area (N: 240 kg/ha, P: 33 kg/ha, and K: 72 kg/ha; as urea, superphosphate and potassium sulfate, respectively). The K and P fertilizers were applied as basal fertilizer at sowing, and N fertilizer was applied in three splits (as basal fertilizer and at jointing and tasseling) in the 3:4:3 proportion.
Maize (hybrid Jingke 968) was sown by hand planters and was thinned at the seedling stage to stand density of 57,000 plants/ha. Plant distance within rows was 30 cm, and the distance between rows was 50 cm. Border plots were included on the sides of the experimental field. Weed growth was controlled manually during the experiment.
Photosynthetic C (13C) Labeling Method
In 2018, in the maize early jointing stage (on 11th July), the 13C pulse labeling was done simultaneously on all four treatments within one replicate block. The pulse labeling method (shown in Supplementary Figure 2) followed the published description (An T. et al., 2015; Zhang et al., 2020) with modifications. A sealed and transparent labeling chamber measured 2.2 m length, 0.5 m width, and 3 m height. This portable labeling chamber covered nine plants in each treatment and consisted of a transparent vinyl sheet on a steel frame. In order to provide a seal around the edges of the chamber, excess vinyl covered the contours of soil surface (Kong and Six, 2010) and was sealed with wet soil (McMahon et al., 2005). Before the start of labeling, the black plastic film mulch was used to cover the soil surface of the micro-plot to prevent the labeled CO2 from diffusing into the soil. The plastic black film was laid only during labeling and was removed immediately afterwards. To avoid any impact of plastic film cover, the non-labeled areas were also covered with black plastic film for the duration of the labeling period.
Labeling took place from 8:00 to 13:00 on a sunny day. An infrared gas analyzer was connected to the top of the labeling chamber to monitor the total CO2 concentration (Wu et al., 2009). NaOH was used to absorb CO2 in the chamber. After the CO2 concentration fell below 80 μl/L, the sodium hydroxide trap was removed and H2SO4 (50 ml, 1 mol/L) was added to the first beaker containing labeled CO3 (99 atom% 13C, Sigma-Aldrich) to obtain 13CO2 concentration of approximately 400 μl/L. When the CO2 concentration in chamber fell below 80 μl/L again, H2SO4 (50 ml, 1 mol/L) was added to the second beaker containing labeled CO3. This process was repeated five times, and each labeling chamber required 9.12 g CO3. Finally, we added sulfuric acid to the No. 6 beaker filled with non-labeled sodium carbonate (1.81 g CO3) to enhance the 13C assimilation efficiency and minimize the loss of 13CO2 (Butler et al., 2004). The entire labeling process ended, and the labeling chamber was removed, after the CO2 concentration dropped below 80 μl/L after the final adjustment.
Sample Collection and Processing
Destructive sampling of maize plants in each treatment was conducted three times. Maize plants and soil samples were taken on 13rd July (the early jointing stage; two days after labeling), 26th July (the late jointing stage; 15 days after labeling) and 27th September (the grain maturity stage; 80 days after labeling). In each straw treatment, three labeled and three non-labeled plants were randomly selected from the respective plots. Shoots were cut at the base, and then roots and soil cores were dug out as a monolith (50 cm long × 50 cm wide and 40 cm deep). The aboveground material included shoots (stems and leaves) and grains (at maturity). All the visible small roots in the soil sample were picked out. Shoots (stems and leaves) and roots were washed in deionized water, oven-dried at 70°C for 3 days and weighed. Dried root and shoot samples were ground in a mill (RetschMM200, Dusseldorf, Germany) for determining organic C.
The 0–40 cm soil was collected in each plot. The residual straw was carefully picked out (about 90% of straw was decomposed at grain harvest). The soil samples were stored in plastic bags at 4 °C and processed within 5 days. A portion of each soil sample was used for determining DOC and MBC. The remaining portion of each soil sample was air-dried, ground and passed through 0.25 mm sieve for the determination of total soil organic C. An elemental analyzer—stable isotope ratio mass spectrometer (Elementar vario PYRO-isoPrime100, Manchester, UK) was used to determine total organic C content and δ13C value in soil and plant samples.
Determination of Soil DOC and MBC Contents and δ13C Values
Microbial biomass C was determined by the chloroform-fumigation extraction method (Vance et al., 1987). Fresh soil equivalent to 10 g oven-dried soil was fumigated for 24 h and then extracted with 0.5 mol/L K2SO4. The same amount of soil was also extracted without fumigation. The non-fumigated extract was used to determine DOC. The DOC was determined by extracting the soils with deionized water (1:2.5 w/v ratio for 1 h) (Haynes, 2005). The soil extracts were measured to determine the DOC content using a Total Organic Carbon Analyzer (Multi N/C UV HS, Analytik Jena AG, Jena, Eisfeld, Germany). The MBC was calculated as the difference in DOC content between fumigated and non-fumigated soil extracts, with the conversion coefficient kEC of 0.45 (Wu et al., 1990). All K2SO4 extracts were freeze-dried (EYELA Freeze Dryer FD-1, Tokyo, Japan) to analyze 13C abundance (253Plus, Thermo Fisher, California, USA).
Calculations
(1) δ13C value and δ13C abundance (FC):
FC (%) = ((δ13C + 1000) × RPDB)/((δ13C+1000) × RPDB+1000) × 100
where RC is the 13C/12C atomic ratio of the sample, and RPDB is 0.0112372 (Lu et al., 2002a).
(2) The amount of 13C (mg) fixed in photosynthesis partitioned to maize shoots, roots, grains and soil (without considering a loss due to respiration)
where Ci is the C content (mg) of shoots, grains, roots or soil in the labeling treatment; FlC is the abundance (%) of 13C in shoots, grains, roots or soil in the labeling treatment; and FnlC is the abundance (%) of 13C in shoots, grains, roots or soil in the non-labeled treatment (Leake et al., 2006).
(3) Partitioning of 13C (%)
where 13Cfixed is the sum (mg) of 13C partitioned to shoots, grains, roots and soil in the labeling treatment, and 13Ci is the 13C content of individual plant parts or soil (Yu, 2017).
(4) Soil microbial biomass C (CMBC, mg/kg), DOC (CDOC, mg/kg), and the content of 13C (13C-CMBC, μg/kg; 13C-CDOC, μg/kg)
where CfumC and CnfumC are the DOC content (mg/kg) in the K2SO4 extracts from fumigated and non-fumigated soils, respectively, in the same treatment; FfumC, l and FnfumC, l are the 13C abundances (%) in DOC in the K2SO4 extracts from fumigated and non-fumigated soils, respectively, from the labeled treatment; FfumC, nl and FnfumC, nl are the 13C abundances (%) in DOC in the K2SO4 extracts from fumigated and non-fumigated soils, respectively, from the non-labeled treatment. kEC is the conversion coefficient, and its value is 0.45 (Wu et al., 1990).
Data Analysis
Two-way ANOVA was done on shoot biomass, root biomass, organic C in shoots, roots and soil, amount of assimilated C, and C partitioning to maize roots and soil, using sampling dates and treatments as independent variables. One-way ANOVA was conducted on parameters relative to four different treatments on each sampling date, or on 12 treatments (three sampling dates × four treatments), depending on significance of the interaction between treatments and sampling dates. Means were compared with the Tukey's honestly significant differences test at the 5% level of probability. All statistical analyses were done using the SPSS statistical software version 20.0 (IBM Corp., Armonk, NY, USA).
Results
The Effects of Straw Treatments on Plant Growth and Yield
The treatments and sampling dates significantly influenced root biomass and shoot biomass, but the interaction was non-significant (Table 2), indicating that the effects of straw return on maize plants growth increased uniformly over time. Root biomass and shoot biomass tended to have relatively high values in the shallow and deep treatments compared with those in the control and the cover treatment (Figures 1A,B). At harvest, deep straw incorporation significantly increased shoot biomass (by 16.8%, averaged across the three sampling dates) compared to the control, but the other two straw treatments did not show significantly higher shoot biomass compared to the control.
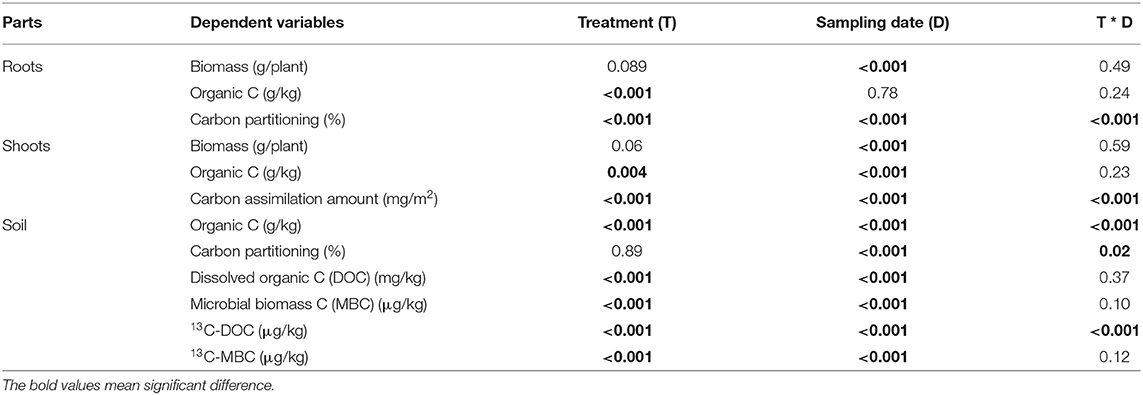
Table 2. Results of analysis of variance (P-values) for various parameters in the maize-soil system as influenced by different treatments at three sampling dates.
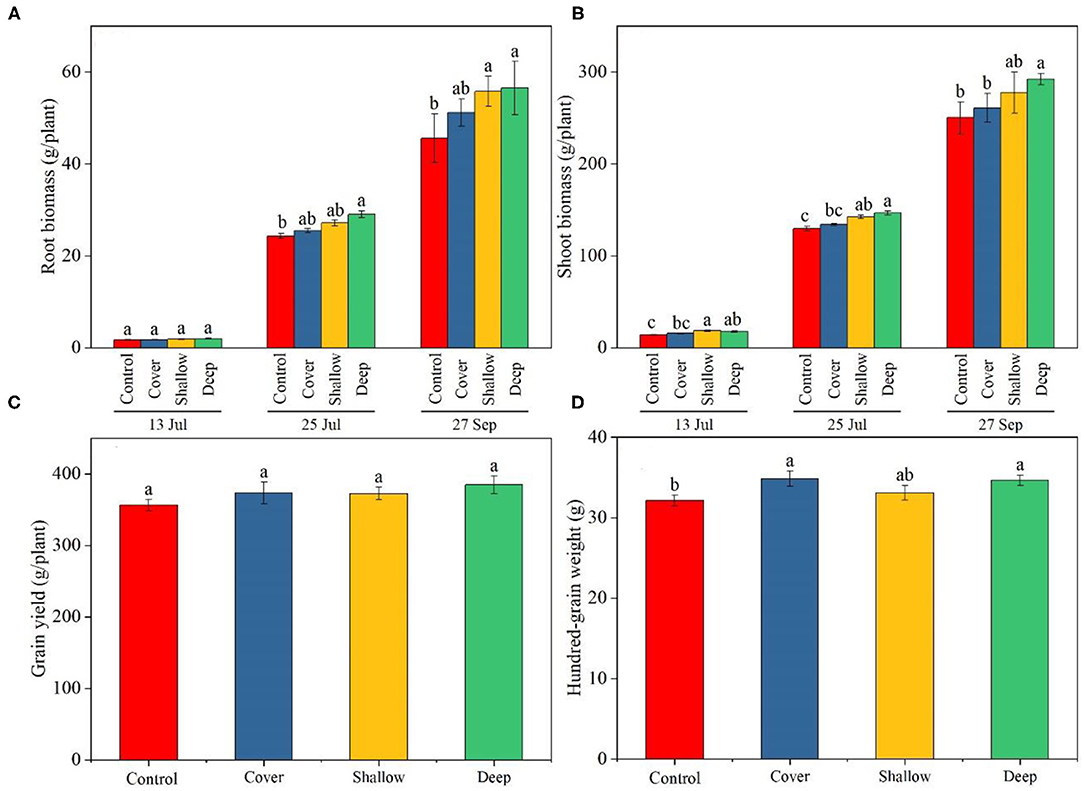
Figure 1. Biomass of maize roots (A) and shoots (B) on the three sampling dates (13 Jul: early jointing, 62 days after sowing (DAS); 25 Jul: late jointing, 74 DAS; 27 Sep: grain maturity, 138 DAS) and grain yield (C) and 100-grain weight (D) at maturity. Means ± SE (n = 3). Different letters denote significant differences among treatments on a specific sampling date (A,B) or among the treatments (C,D) (P < 0.05). Control, no straw added; Cover, straw added to the soil surface; Shallow, straw incorporated at the 0–20 cm soil depth; Deep, straw incorporated at the 20–40 cm soil depth.
At harvest, although there was no significant difference in grain yield across the treatments in 2018 (Figure 1C), the deep straw return and the cover treatments showed a significantly higher yields (by 10.5 and 8.0%, respectively) compared with the control in 2019 (Supplementary Table 1). Deep straw incorporation showed significantly higher 100-grain weight compared with the control in 2018 (Figure 1D); however, in 2019, the deep straw return treatment had similar 100-grain weight, but a longer ears compared with the control (Supplementary Table 1).
The Effects of Straw Treatments on Dynamics of Organic C in Maize Plants and Soil
Organic C in roots did not significantly differ among the three sampling dates (Table 2). Across sampling dates, average organic C concentration in roots in the shallow and deep treatments (406 and 413 g/kg, respectively) was significantly higher than that in the control and the cover treatment (391–392 g/kg) (Figure 2A).
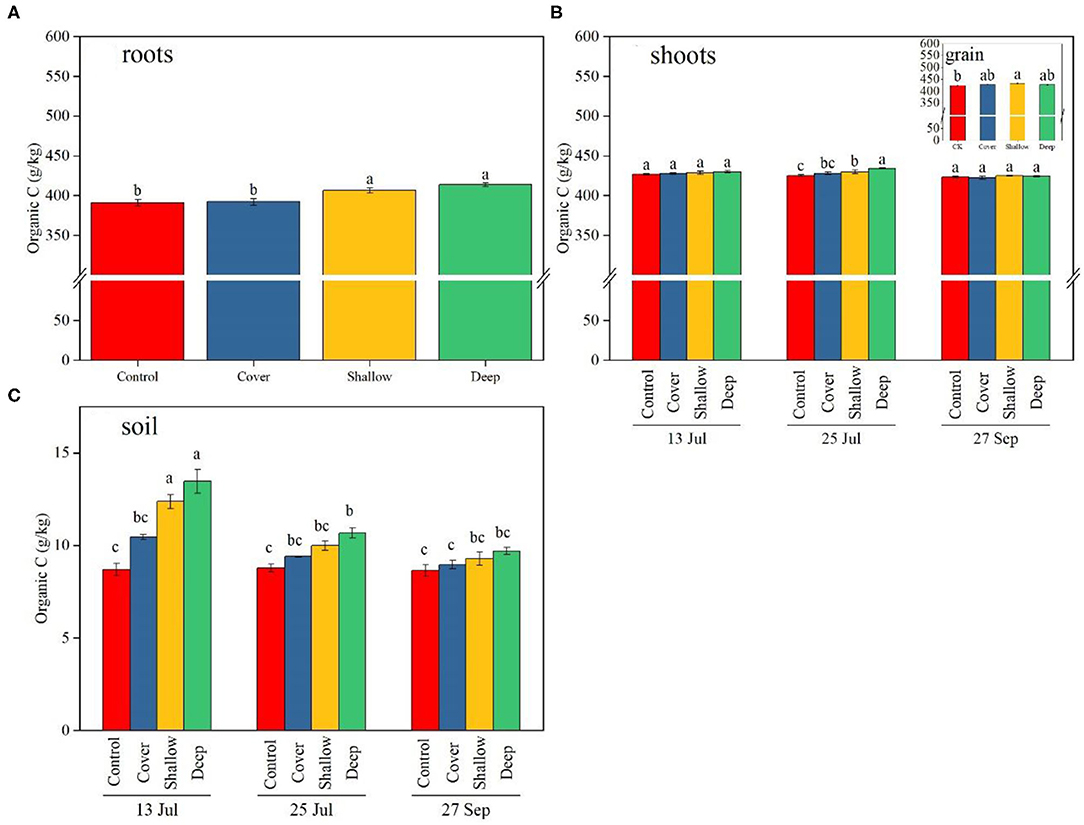
Figure 2. Organic carbon (C) content of maize roots (A), shoots (B), grain [the insert in (B)] and 0–40 cm soil layer (C) on the three sampling dates [13 Jul: early jointing, 62 days after sowing (DAS); 25 Jul: late jointing, 74 DAS; 27 Sep: grain maturity, 138 DAS]. Means ± SE (n = 3). Organic C in roots (A) was averaged across the sampling dates because the interaction (treatments × sampling dates) was not significant. Depending on significance of the interaction, different letters denote significant differences among treatments [(A), the insert in (B)], among the treatments on a given sampling date (B) or among sampling dates × treatments (C) (P < 0.05). Control, no straw added; Cover, straw added to the soil surface; Shallow, straw incorporated at the 0–20 cm soil depth; Deep, straw incorporated at the 20–40 cm soil depth.
Treatments and sampling dates, but not the interaction between them, significantly influenced organic C in shoots (Table 2). Across sampling dates, deep straw incorporation significantly increased organic C in shoots; averaged across treatments, organic C in shoots significantly decreased from jointing stage to grain maturity. Both shallow and deep straw incorporation slightly but significantly increased organic C in grain (by 2.1 and 1.2%, respectively) compared with the control at the late jointing stage (Figure 2B).
The interaction between sampling dates and treatments significantly influenced organic C in soil (Table 2). Both shallow and deep straw incorporation had the highest soil organic C (12.7 and 13.7 g/kg, respectively) on 13 July, and the control without straw had the lowest soil organic C on all three sampling dates (Figure 2C). The straw cover treatment did not show significantly higher soil organic C compared with the control on all three sampling dates (Figure 2C).
The Assimilation and Partitioning of Photosynthetic C
The effects of treatments and sampling dates, and their interaction significantly influenced the amount of assimilated C in shoots (Table 2). On 13 July, deep and shallow straw incorporation significantly increased C in shoots compared with the control on all three sampling dates (Figure 3A), but the straw cover treatment significantly increased C only in shoots compared with the control (Figure 3A).
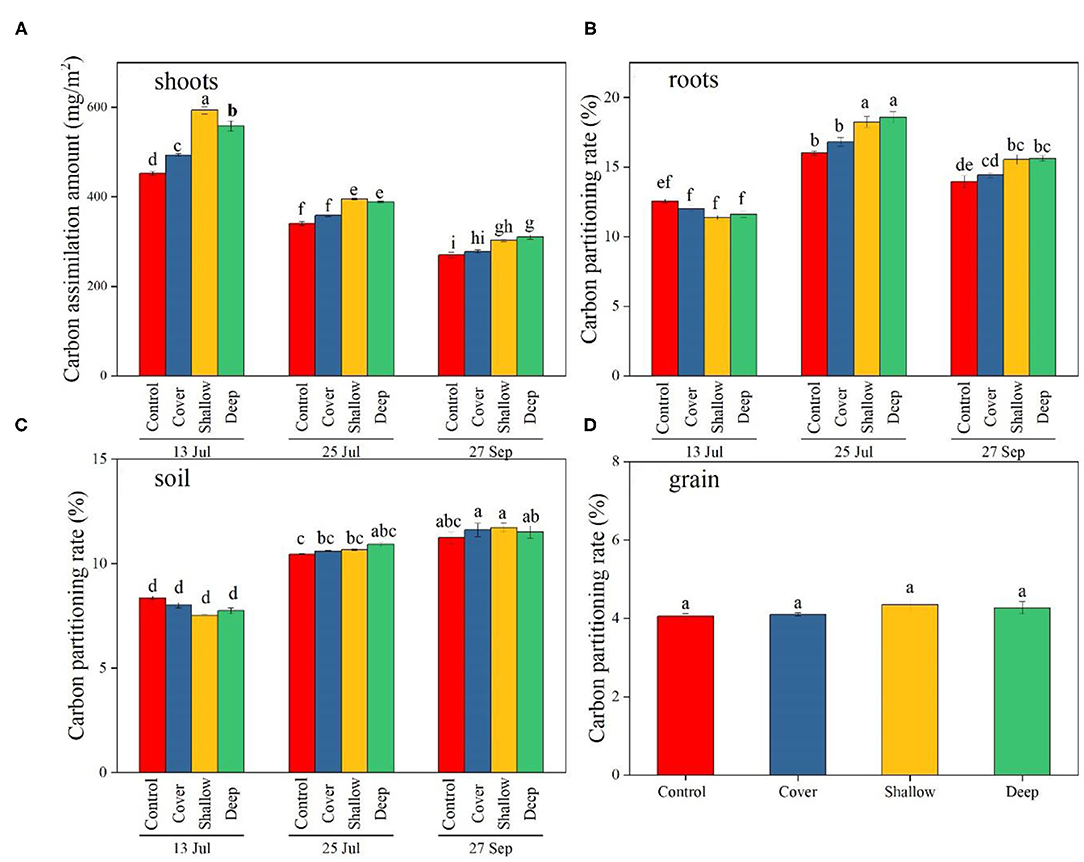
Figure 3. The amount of photosynthetic carbon (13C) accumulated in maize shoots (A) and partitioning of photosynthetic carbon (13C) to maize roots (B), 0–40 cm soil (C), and grain (D) on the three sampling dates [13 Jul: early jointing, 62 days after sowing (DAS); 25 Jul: late jointing, 74 DAS; 27 Sep: grain maturity, 138 DAS]. Carbon partitioning: 13C content of individual plant parts or soil divided by the sum of 13Cfixed (13C found in the shoots, roots, soils and grains) (expressed as %). Means ± SE (n = 3). Different letters denote significant differences among sampling dates × treatments (A–C) or among treatments (D) (P < 0.05). Control, no straw added; Cover, straw added to the soil surface; Shallow, straw incorporated at the 0–20 cm soil depth; Deep, straw incorporated at the 20–40 cm soil depth.
The interaction between treatments and sampling dates significantly altered C partitioning to roots and soil (Table 2). In roots, C partitioning rate in the treatments with shallow and deep straw incorporation was the highest (18.2 and 18.6%, respectively) on 25 July (late jointing), but the two treatments had the lowest C partitioning (11.4 and 11.6%, respectively) on 13 July (early jointing) (Figure 3B). On 27 September, the deep and the shallow straw return treatments showed a higher C partitioning rate to root compared with the control (Figure 3B).
The C partitioning to soil tended to be lower on 13 July (early jointing) than 27 September (grain harvest) (Figure 3C), but the differences among the treatments were not significant on the three sampling dates (Figure 3C). Treatments had no significant influence on C partitioning to grain (4.2% on average; Figure 3D).
Dynamics of DOC and MBC in Soils
The treatments and sampling dates significantly influenced DOC and MBC in soils, but the interaction was non-significant (Table 2). The control had lower DOC (Figure 4A) and MBC (Figure 4B) than the three straw treatments regardless of the sampling date. The shallow straw return treatment increased DOC on the first two sampling dates (Figure 4A) and increased MBC on 13 July (Figure 4B) compared with the cover and deep straw return treatments. The 13C-DOC content as well as the ratio 13C-DOC/DOC were significantly influenced by the interaction (Table 2). On 25 July (late jointing), the 13C-DOC content in the straw treatments of cover, shallow and deep was 6.9, 7.3, and 7.7 μg/kg, respectively, all of which were significantly higher than the control. However, from late jointing to grain harvest, the 13C-DOC content in soil decreased significantly to around 0.45 μg/kg, with no difference among the four treatments (Figure 4C).
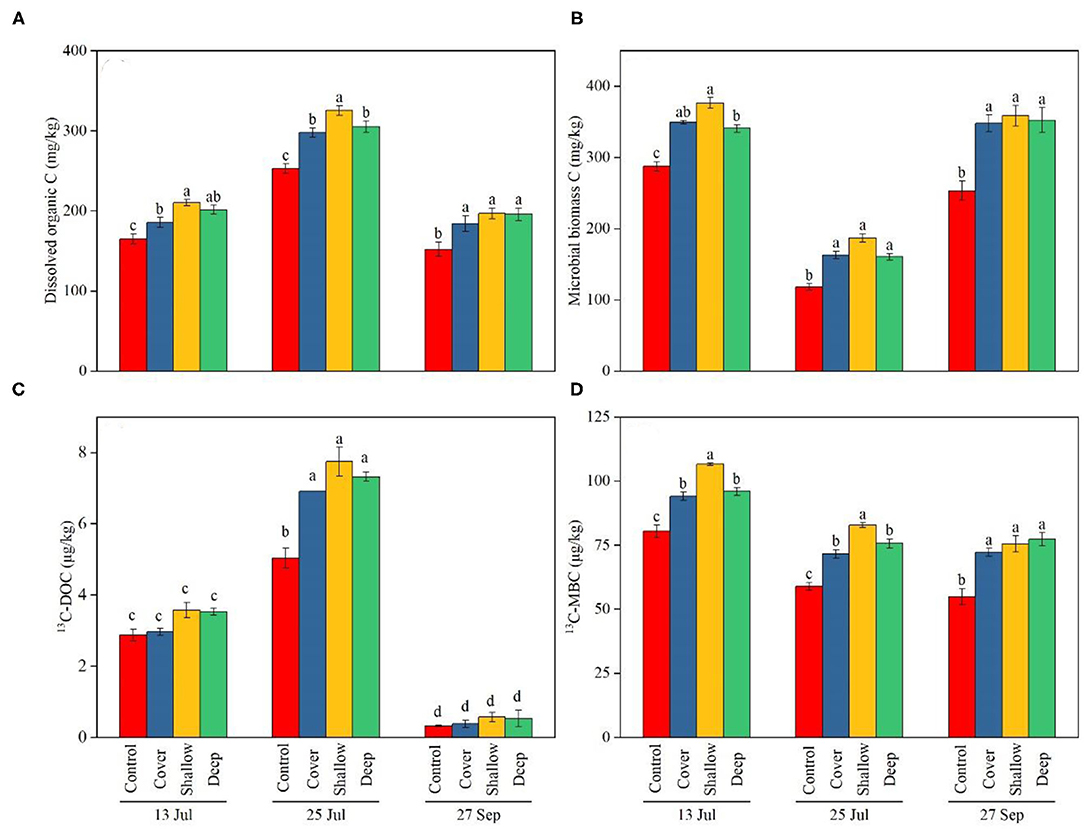
Figure 4. Dissolved organic carbon (DOC) (A), microbial biomass carbon (MBC) (B), 13C in DOC (C), and 13C in MBC (D) in 0–40 cm soil layer on the three sampling dates [13 Jul: early jointing, 62 days after sowing (DAS); 25 Jul: late jointing, 74 DAS; 27 Sep: grain maturity, 138 DAS]. Means ± SE (n = 3). Depending on significance of the interaction, different letters denote significant differences among treatments on a specific sampling date (A,B,D) or among sampling dates × treatments (C) (P < 0.05). Control, no straw added; Cover, straw added to the soil surface; Shallow, straw incorporated at the 0–20 cm soil depth; Deep, straw incorporated at the 20–40 cm soil depth.
Both main effects significantly influenced the MBC as well as 13C-MBC contents, but the interaction was non-significant (Table 2). At all three sampling dates, the control had significantly lower MBC than the other treatments. Regarding the temporal dynamics, 13C-MBC was relatively high in early jointing (about 36.8 μg/kg on average) and at grain harvest (about 29.1 μg/kg on average), but dropped at late jointing (25 July) (about 26.3 μg/kg on average) (Figure 4D). The 13C-MBC content (Figure 4D) followed exactly the same trends as MBC (Figure 4B).
Discussion
Effects of Straw Incorporation on Maize Growth
We showed that straw incorporation in deep soil tended to increase root and shoot biomass compared with the control (Figures 1A,B), which could be associated with a higher 100-grain weight (Figure 1D). This may be the nutrients released from straw filled SOC and accelerated microbial activities in the deep layer (Zou et al., 2016; Ma et al., 2019; Chen S. Y. et al., 2020). Our results are in agreement with other studies, whereby maize plants in the treatments with straw tended to have a bigger root or shoot biomass or even higher grain yield compared with plants grown without straw added (Chen Y. Y. et al., 2020; Han Y. L. et al., 2020; Xian et al., 2020).
Higher photosynthetic C allocation to the root at late jointing in the treatment with incorporated straw than the straw cover treatment (Figure 3B) indicated that straw incorporation into soils was beneficial to the growth of maize roots (Figure 1A). The bigger biomass of roots after deep straw incorporation would enhance uptake of water and nutrients from the deep soil (Huang et al., 2013).
Straw mulching on soil surface or shallow incorporation requires the optimal amount of straw because excessive straw or uneven distribution may directly reduce the germination of seeds, and cause adverse phenomena such as chlorotic seedlings and reduced growth (Zou et al., 2016). Decomposition of maize straw in northern China generally takes about 2 years (Han Y. et al., 2020). The straw trapped at shallow soil depth or on the soil surface can disturb sowing in spring, and the release of organic acids during straw decomposition may not be conducive to root growth (Ma et al., 2016). Thus, to avoid these problems, we tested straw incorporation into deeper soil. Deep straw incorporation could improve not only the capacity of soil to store water and conserve fertilizers (Zou et al., 2014), but also increase soil carbon content, improve soil fertility (Wang et al., 2015), promote soil microbial growth, and improve soil biological functions (Zhao et al., 2015).
Effects of the Depth of Straw Incorporation Into Soils on Soil Organic C and Assimilation and Partitioning of Photosynthetic C in the Maize-Soil System
Straw incorporation at 20–40 cm soil depth increased 13C assimilation in shoots compared with the control across the whole maize growth period (Figure 3). This might have been because straw incorporation at 20–40 cm soil depth increased root and shoot biomass (Figure 1), and also increased microbial biomass (MBC; Figure 4B), thus enhancing crop and microbial respiration (Baptist et al., 2015). Rhizosphere deposition at the early stage of crop growth can be influenced by tillage methods (Munoz-Romero et al., 2013), soil fertility (Sun et al., 2019) and other factors, e.g., plant species (Baptist et al., 2015) and nutrient availability (Merckx et al., 1987). In our study, the photosynthetic 13C products were distributed mainly in the maize parts (Figure 3), whereas a relatively small proportion entering soil during maize growth (Figure 3C). These findings were in agreement with the early studies showing photosynthetic C had a fast conversion rate in plants: the photosynthetic C content in shoots reached a peak 6 hours after labeling, and photosynthetic C partitioned to roots was detected 4 hours after labeling (Ostle et al., 2000; Johnson et al., 2002).
In the present study, straw incorporation at 20-40 cm soil depth was associated with the relatively high soil organic C content compared with the other treatments (Figure 2C). This finding could be a consequence of enhanced above- and below-ground plant productivity in the treatment with deep straw incorporation (Figure 1). Plants in the deep straw treatment invested relatively more assimilates into root growth than plants in the control (Figure 3A), resulting in the higher root biomass (Figure 1A) and root length in the deep treatment at harvest (data not shown). This can be explained by straw incorporation into deep soil promoting the formation of dominant aggregates and increasing organic C accumulation in them (Zhu et al., 2015). Although there was no significant difference between the deep and shallow straw return treatments in terms of soil organic C, shoot and root biomass, and C partitioning to roots (Figures 1–3), Li et al. (2021b) found that deep straw incorporation (40 cm) altered soil bacteria abundance and improved soil fertility compared with other ways of returning maize straw. Li et al. (2021a) reported that the more straw was incorporated at 40 cm soil depth, the greater the total organic C was. In the study presented here, straw incorporation at 20-40 cm soil depth increased maize yield (Supplementary Table 1), and could represent a more effective use of maize straw to underpin sustainable agriculture.
Effects of the Depth of Straw Incorporation Into Soils on Dynamics of Photosynthetic C Allocation in the Maize-Soil System
In this study, we found that the proportions of photosynthetic C allocated were: 69-80% to shoots, 12–17% to roots, and about 7.9–12% to soil (Figure 3). The observed values were similar to results reported by Tian et al. (2013) for a rice system. 13C partitioning to soil was significantly influenced by the depth of straw incorporation. The larger relative exudation of organic compounds from roots is often associated with enhanced microbial growth and enzymatic activities connected with nutrient mining from soil organic matter, which then facilitates plant nutrient uptake (Kaštovská et al., 2018).
The amount of soil photosynthetic C partitioning in each treatment was greater at early jointing than grain maturity, which might have been due to the maize roots growing vigorously at jointing, leading to a large amount of root exudates entering the soil. However, approaching grain maturity, maize root system gradually lost its activity, resulting in less root exudation (Barber, 1995).
The distribution of 13C to roots was proportional to the development of root system over time. Root growth may also lead to a temporal increase in soil 13C allocation (Figure 3C) due to an increase in root exudation. The observed differences in temporal rhizodeposition dynamics indicated treatment-related differences in the quantity of the released organic compounds. The partitioning rate of photosynthetic 13C to soil was not significantly different among treatments (Table 2; Figure 3C), indicating that straw addition regardless of soil depth did not change partitioning of photosynthetic C to soil in a short term.
Effects of the Depth of Straw Incorporation Into Soils and Maize Growth Stage on Soil DOC and MBC
In our study, rate of photosynthetic C partitioning to DOC and MBC was influenced by growth stages of maize (Figure 4). The contents of DOC and 13C-DOC increased from early to late jointing and decreased at grain maturity (Figures 4A,C). It was probably due to relatively strong root exudation at jointing, with a decline toward maturity. Similarly, soil MBC in each treatment decreased from early to late jointing and increased at the maturity stage, which might have been associated with decomposition of dead roots (Figures 4B,D). This is consistent with the previous study showing that the proportions of 14C in DOC and MBC varied with rice progressing from jointing to grain filling (Lu et al., 2002b).
Microbial biomass C was the main component of active soil organic C because microorganisms could preferentially utilize dissolved C in the rhizosphere (Grantina-Ievina et al., 2014). In our study, three treatments with straw addition (especially deep and shallow incorporation treatments) increased the partitioning of photosynthetic C in MBC (Figure 4D). This might have been due to straw addition promoting the growth of maize roots, improving exudation into the rhizosphere, and thus enhancing microorganism growth. Straw addition could also increase soil microbial activity via microbial decomposition of straw. Compared with the cover treatment, the higher content of soil organic C was found in the deep and shallow incorporation treatments (Figure 2). In addition, straw incorporated in the deeper layer lowered soil bulk density and improved soil aeration (Zou et al., 2014), both of which would accelerate decomposition of straw.
Different natural conditions in various soil layers would be associated with varied composition and abundance of microbial populations, leading to differential straw degradation rates (Frey et al., 1999; Coppens et al., 2006). Soil moisture and nutrient availability interact in influencing plant C acquisition and partitioning in the plant-microbe-soil systems (Atere et al., 2017). In our study, we indeed found significant differences in soil water content (Supplementary Figure 3). In addition, temperature has an important effect on soil organic C and MBC (Ghosh et al., 2020; Yanni et al., 2020); however, in our study there was no significant difference in soil temperature among the four treatments (Supplementary Figure 4).
Straw incorporation at the 20–40 cm soil depth had the positive effects not only on maize plants at harvest such as a significant grain yield increase (Supplementary Table 1), significantly longer ears and higher 100-grain weight (Supplementary Table 1; Figure 1D), but also on soil such as higher SOC compared with the surface and 0-20 cm depth straw addition (Figures 2C, 5). However, based on the current research, the mechanisms underlying an increase in soil organic C can be predicted only to some extent, and the contribution of different factors cannot be determined qualitatively and/or quantitatively, like C emission and energy-consumption. There is still a scope for research on the soil mechanisms at the microscopic scale regarding the effect of straw incorporation at various soil depths.
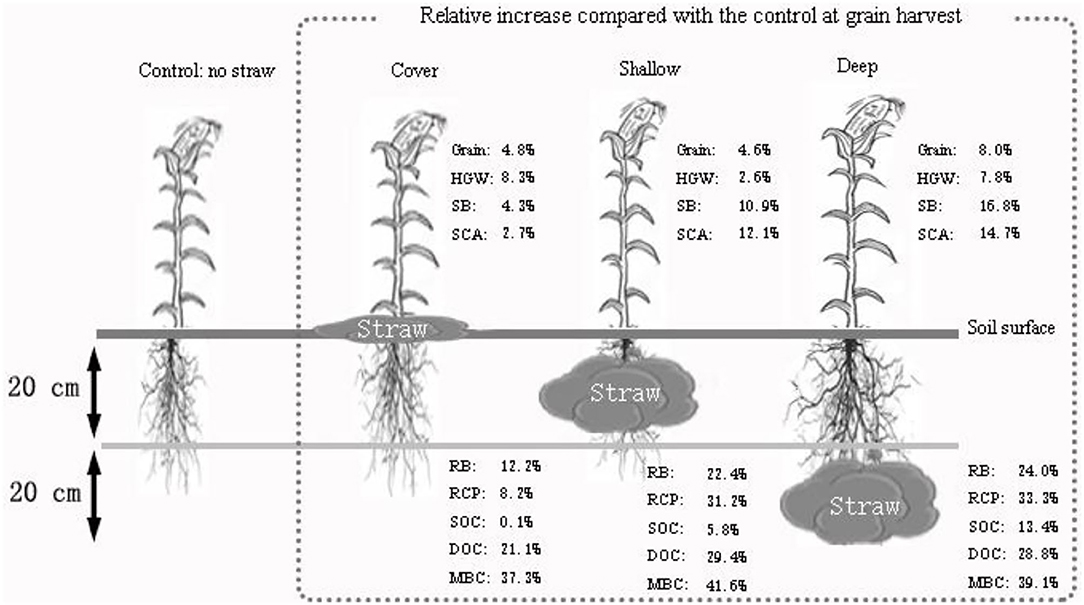
Figure 5. Relative increase in various parameters at maize harvest in the three treatments with straw addition compared with the treatment without straw. Control, no straw added; Cover, straw added to the soil surface; Shallow, straw incorporated at the 0–20 cm soil depth; Deep, straw incorporated at the 20–40 cm soil depth. HGW, 100-grain weight; SB, shoot biomass; RB, root biomass; SCA, shoot carbon assimilation; RCP, root carbon partitioning; SOC, soil organic carbon; DOC, dissolved organic carbon; MBC, microbial biomass carbon.
Conclusions
The results have supported our hypothesis that deep straw incorporation can promote net photosynthetic C assimilation and maize growth via increased soil organic C and an increase in microbial activity (MBC), thus increasing grain yield. Hence, deep straw incorporation could be recommended in the maize production in Northeast China.
Data Availability Statement
The original contributions presented in the study are included in the article/Supplementary Material, further inquiries can be directed to the corresponding author/s.
Author Contributions
HZ designed the experiment and acquired funding for the study. X-XW and JL analyzed the data and drafted the manuscript. JL and DW performed the experiments, collected the samples and the data. TA, WQ, and ZR revised the manuscript and improved English. All authors discussed the results, commented on the manuscript, and agree with the submission of this version.
Funding
This study was financially supported by Liao Ning Revitalization Talents Program (XLYC1905010) and Key R&D Program of Liao Ning Province (2019JH2/10200004). X-XW is supported by State Key Laboratory of North China Crop Improvement and Regulation.
Conflict of Interest
WQ was employed by company Henan Xinlianxin Chemical Industry Group Co., Ltd.
The remaining authors declare that the research was conducted in the absence of any commercial or financial relationships that could be construed as a potential conflict of interest.
Publisher's Note
All claims expressed in this article are solely those of the authors and do not necessarily represent those of their affiliated organizations, or those of the publisher, the editors and the reviewers. Any product that may be evaluated in this article, or claim that may be made by its manufacturer, is not guaranteed or endorsed by the publisher.
Supplementary Material
The Supplementary Material for this article can be found online at: https://www.frontiersin.org/articles/10.3389/fagro.2022.805320/full#supplementary-material
References
An, T., Schaeffer, S., Li, S., Fu, S., Pei, J., Li, H., et al. (2015). Carbon fluxes from plants to soil and dynamics of microbial immobilization under plastic film mulching and fertilizer application using 13C pulse-labeling. Soil Biol. Biochem. 80, 53–61. doi: 10.1016/j.soilbio.2014.09.024
An, T. T., Schaeffer, S., Zhuang, J., Radosevich, M., Li, S. Y., Li, H., et al. (2015). Dynamics and distribution of 13C-labeled straw carbon by microorganisms as affected by soil fertility levels in the black soil region of northeast China. Biol. Fertil. Soils 51, 605–613. doi: 10.1007/s00374-015-1006-3
Atere, C. T., Ge, T., Zhu, Z., Tong, C., Jones, D. L., Shibistova, O., et al. (2017). Rice rhizodeposition and carbon stabilisation in paddy soil are regulated via drying-rewetting cycles and nitrogen fertilisation. Biol. Fertil. Soils 53, 407–417. doi: 10.1007/s00374-017-1190-4
Baptist, F., Aranjuelo, I., Legay, N., Lopez-Sangil, L., Molero, G., Rovira, P., et al. (2015). Rhizodeposition of organic carbon by plants with contrasting traits for resource acquisition: responses to different fertility regimes. Plant Soil 394, 391–406. doi: 10.1007/s11104-015-2531-4
Barber, S. A. (1995). Soil Nutrient Bioavailability: A Mechanistic Approach. New York, NY: John Wiley and Sons.
Bi, Y., Gao, C., Wang, Y., and Li, B. (2009). Estimation of straw resources in China. Trans. Chin. Soc. Agric. Eng. 25, 211–217 (in Chinese with English abstract).
Butler, J. L., Bottomley, P. J., Griffith, S. M., and Myrold, D. D. (2004). Distribution and turnover of recently fixed photosynthate in ryegrass rhizospheres. Soil Biol. Biochem. 36, 371–382. doi: 10.1016/j.soilbio.2003.10.011
Chen, S. Y., Zhang, X. Y., Shao, L. W., Sun, H. Y., Niu, J. F., and Liu, X. W. (2020). Effects of straw and manure management on soil and crop performance in North China Plain. Catena 187, 104359. doi: 10.1016/j.catena.2019.104359
Chen, Y. Y., Fan, P. S., Li, L., Tian, H., Ashraf, U., Mo, Z. W., et al. (2020). Straw incorporation coupled with deep placement of nitrogen fertilizer improved grain yield and nitrogen use efficiency in direct-seeded rice. J. Soil Sci. Plant Nutr. 20, 2338–2347. doi: 10.1007/s42729-020-00301-2
Cong, P., Li, Y., Gao, Z., Wang, J., Zhang, L., and Pang, H. (2019). High dosage of pelletized straw returning rapidly improving soil organic carbon content and wheat-maize yield. Trans. Chin. Soc. Agric. Eng. 35, 148–156 (in Chinese with English abstract). doi: 10.11975/j.issn.1002-6819.2019.01.018
Coppens, F., Garnier, P., De Gryze, S., Merckx, R., and Recous, S. (2006). Soil moisture, carbon and nitrogen dynamics following incorporation and surface application of labelled crop residues in soil columns. Eur. J. Soil Sci. 57, 894–905. doi: 10.1111/j.1365-2389.2006.00783.x
Dinardo-Miranda, L. L., and Fracasso, J. V. (2013). Sugarcane straw and the populations of pests and nematodes. Sci. Agric. 70, 369–374. doi: 10.1590/S0103-90162013000500012
Frey, S. D., Elliott, E. T., and Paustian, K. (1999). Bacterial and fungal abundance and biomass in conventional and no-tillage agroecosystems along two climatic gradients. Soil Biol. Biochem. 31, 573–585. doi: 10.1016/S0038-0717(98)00161-8
Ghosh, A., Das, A., Das, D., Ray, P., Bhattacharyya, R., Biswas, D. R., et al. (2020). Contrasting land use systems and soil organic matter quality and temperature sensitivity in North Eastern India. Soil Till. Res. 199, 104573. doi: 10.1016/j.still.2020.104573
Grantina-Ievina, L., Karlsons, A., Andersone-Ozola, U., and Ievinsh, G. (2014). Effect of freshwater sapropel on plants in respect to its growth-affecting activity and cultivable microorganism content. Zemdir. Agric. 101, 355–366. doi: 10.13080/z-a.2014.101.045
Han, Y., Yao, S. H., Jiang, H., Ge, X. L., Zhang, Y. L., Mao, J. D., et al. (2020). Effects of mixing maize straw with soil and placement depths on decomposition rates and products at two cold sites in the mollisol region of China. Soil Till. Res. 197, 104519. doi: 10.1016/j.still.2019.104519
Han, Y. L., Ma, W., Zhou, B. Y., Yang, X. L., Salah, A., Li, C. F., et al. (2020). Effects of straw-return method for the maize-rice rotation system on soil properties and crop yields. Agronomy 10, 461. doi: 10.3390/agronomy10040461
Haynes, R. (2005). Labile organic matter fractions as centralcomponents of the quality of agricultural soils: an overview. Adv. Agron. 5, 221–268. doi: 10.1016/S0065-2113(04)85005-3
Huang, Y., Bi, S. Y., Zou, H. T., and Dou, S. (2013). Effect of straw deep returning on corn root system and yield. J. Maize Sci. 21, 109–112 (in Chinese with English abstract).
Jia, W., Qin, W., Zhang, Q., Wang, X., Ma, Y., and Chen, Q. (2018). Evaluation of crop residues and manure production and their geographical distribution in China. J. Clean. Prod. 188, 954–965. doi: 10.1016/j.jclepro.2018.03.300
Johnson, D., Leake, J. R., Ostle, N., Ineson, P., and Read, D. J. (2002). In situ 13CO2 pulse-labelling of upland grassland demonstrates a rapid pathway of carbon flux from arbuscular mycorrhizal mycelia to the soil. New Phytol. 153, 327–334. doi: 10.1046/j.0028-646X.2001.00316.x
Kaštovská, E., Straková, P., Edwards, K., Urbanová, Z., Bárta, J., Mastný, J., et al. (2018). Cotton-grass and blueberry have opposite effect on peat characteristics and nutrient transformation in peatland. Ecosystems 21, 443–458. doi: 10.1007/s10021-017-0159-3
Knoblauch, C., Maarifat, A. A., Pfeiffer, E. M., and Haefele, S. M. (2011). Degradability of black carbon and its impact on trace gas fluxes and carbon turnover in paddy soils. Soil Biol. Biochem. 43, 1768–1778. doi: 10.1016/j.soilbio.2010.07.012
Kong, A. Y. Y., and Six, J. (2010). Tracing root vs. residue carbon into soils from conventional and alternative cropping systems. Soil Sci. Soc. Am. J. 74, 1201–1210. doi: 10.2136/sssaj2009.0346
Lal, R. (2009). Soil quality impacts of residue removal for bioethanol production. Soil Till. Res. 102, 233–241. doi: 10.1016/j.still.2008.07.003
Leake, J., Ostle, N., Rangel-Castro, J., and Johnson, D. (2006). Carbon fluxes from plants through soil organisms determined by field 13CO2 pulse-labelling in an upland grassland. Appl. Soil Ecol. 33, 152–175. doi: 10.1016/j.apsoil.2006.03.001
Li, F., Han, X., Ma, X. L., Wang, Y. J., Song, T. Y., and Wang, Y. Y. (2020). Straw mulch controls runoff and nitrogen and phosphorus loss from slope farmland in black soil rigion of Northeast China. J. Soil Water Conserv. 34, 37–42 (in Chinese with English abstract).
Li, J., Ye, X., An, J., Jin, X., Fan, Q., Zou, H., et al. (2021a). The more straw we deep-bury, the more soil TOC will be accumulated: when soil bacteria abundance keeps growing. J. Soils Sediments. 22, 162–171. doi: 10.1007/s11368-021-03068-w
Li, J., Ye, X., Zhang, Y., Chen, J., Yu, N., and Zou, H. (2021b). Maize straw deep-burying promotes soil bacteria community abundance and improves soil fertility. J. Soil Sci. Plant Nutr. 21, 1397–1407. doi: 10.1007/s42729-021-00448-6
Lu, Y., Watanabe, A., and Kimura, M. (2002a). Contribution of plant-derived carbon to soil microbial biomass dynamics in a paddy rice microcosm. Biol. Fertil. Soils 36, 136–142. doi: 10.1007/s00374-002-0504-2
Lu, Y., Watanabe, A., and Kimura, M. (2002b). Input and distribution of photosynthesized carbon in a flooded rice soil. Glob. Biogeochem. Cycles 16, 32–31. doi: 10.1029/2002GB001864
Ma, L. J., Kong, F. X., Wang, Z., Luo, Y., Lv, X. B., Zhou, Z. G., et al. (2019). Growth and yield of cotton as affected by different straw returning modes with an equivalent carbon input. Field Crops Res. 243, 107616. doi: 10.1016/j.fcr.2019.107616
Ma, Z., Marsolais, F., Bernards, M. A., Sumarah, M. W., Bykova, N. V., and Igamberdiev, A. U. (2016). Glyoxylate cycle and metabolism of organic acids in the scutellum of barley seeds during germination. Plant Sci. 248, 37–44. doi: 10.1016/j.plantsci.2016.04.007
McMahon, S. K., Williams, M. A., Bottomley, P. J., and Myrold, D. D. (2005). Dynamics of microbial communities during decomposition of carbon-13 labeled ryegrass fractions in soil. Soil Sci. Soc. Am. J. 69, 1238–1247. doi: 10.2136/sssaj2004.0289
Merckx, R., Dijkstra, A., Den Hartog, A., and Van Veen, J. A. (1987). Production of root-derived material and associated microbial growth in soil at different nutrient levels. Biol. Fertil. Soils 5, 126–132. doi: 10.1007/BF00257647
Munoz-Romero, V., Lopez-Bellido, R. J., Redondo, R., and Lopez-Bellido, L. (2013). Nitrogen rhizodeposition by wheat under different tillage systems in a rainfed Vertisol. Field Crops Res. 144, 148–153. doi: 10.1016/j.fcr.2013.01.005
Nie, S. A., Zhou, P., Ge, T. D., Tong, C. L., Xiao, H. A., Wu, J. S., et al. (2012). Quantifying rice (Oryza sativa L.) photo-assimilated carbon input into soil organic carbon pools following continuous 14C labeling. Environ. Sci. (in Chinese) 33, 1346–1351.
Ostle, N., Ineson, P., Benham, D., and Sleep, D. (2000). Carbon assimilation and turnover in grassland vegetation using an in situ 13CO2 pulse labelling system. Rapid Commun. Mass Spectrom. 14, 1345–1350. doi: 10.1002/1097-0231(20000815)14:15andlt;1345::AID-RCM22andgt;3.0.CO;2-B
Qin, W., Hu, C., and Oenema, O. (2015). Soil mulching significantly enhances yields and water and nitrogen use efficiencies of maize and wheat: a meta-analysis. Sci. Rep. 5, 16210. doi: 10.1038/srep16210
Sun, Z. A., Wu, S. X., Zhang, Y. W., Meng, F. Q., Zhu, B., and Chen, Q. (2019). Effects of nitrogen fertilization on pot-grown wheat photosynthate partitioning within intensively farmed soil determined by 13C pulse-labeling. J. Plant Nutr. Soil Sci. 182, 896–907. doi: 10.1002/jpln.201800603
Tao, Z., Li, C., Li, J., Ding, Z., Xu, J., Sun, X., et al. (2015). Tillage and straw mulching impacts on grain yield and water use efficiency of spring maize in Northern Huang–Huai–Hai Valley. Crop J. 3, 445–450. doi: 10.1016/j.cj.2015.08.001
Tian, J., Pausch, J., Fan, M., Li, X., Tang, Q., and Kuzyakov, Y. (2013). Allocation and dynamics of assimilated carbon in rice-soil system depending on water management. Plant Soil 363, 273–285. doi: 10.1007/s11104-012-1327-z
Vance, E. D., Brookes, P. C., and Jenkinson, D. S. (1987). An extraction method for measuring soil microbial biomass C. Soil Biol. Biochem. 19, 703–707. doi: 10.1016/0038-0717(87)90052-6
Wang, S. N., Zou, H. T., Zhang, Y. L., Yu, N., Zhang, Y. L., Fan, Q. F., et al. (2015). Effect of straw deep returning on the soil water features and soil organic carbon components. J. Soil Water Conserv. 1, 154–158 (in Chinese with English abstract).
Wu, J., Joergensen, R. G., Pommerening, B., Chaussod, R., and Brookes, P. C. (1990). Measurement of soil microbial biomass C by fumigation-extraction—an automated procedure. Soil Biol. Biochem. 22, 1167–1169. doi: 10.1016/0038-0717(90)90046-3
Wu, W. X., Liu, W., Lu, H. H., Chen, Y. X., Devare, M., and Thies, J. (2009). Use of 13C labeling to assess carbon partitioning in transgenic and nontransgenic (parental) rice and their rhizosphere soil microbial communities. FEMS Microbiol. Ecol. 67, 93–102. doi: 10.1111/j.1574-6941.2008.00599.x
Xia, L., Wang, S., and Yan, X. (2014). Effects of long-term straw incorporation on the net global warming potential and the net economic benefit in a rice–wheat cropping system in China. Agric. Ecosyst. Environ. 197, 118–127. doi: 10.1016/j.agee.2014.08.001
Xian, Y. R., Chen, Y., Chen, C., He, R. R., Chen, X. W., Chen, Y., et al. (2020). Does extending recycling chain of using rice straw contribute to improving yield and reducing GHGs emissions in paddy field? An integrated analysis based on field research and system assessment. J. Clean. Prod. 264, 121508. doi: 10.1016/j.jclepro.2020.121508
Xiao, L., Zhao, R., and Kuhn, N. J. (2019). Straw mulching is more important than no tillage in yield improvement on the Chinese Loess Plateau. Soil Till. Res. 194, 104314. doi: 10.1016/j.still.2019.104314
Xu, J., Hu, N., and Zhu, L. (2016). Effect of amount of annual straw returning on soil nutrients and yield in winter wheat field. J. Tritic. Crops 36, 215–222 (in Chinese with English abstract).
Yan, D. Z., Wang, D. J., and Yang, L. Z. (2007). Long-term effect of chemical fertilizer, straw, and manure on labile organic matter fractions in a paddy soil. Biol. Fertil. Soils 44, 93–101. doi: 10.1007/s00374-007-0183-0
Yang, Y., Yu, K., and Feng, H. (2018). Effects of straw mulching and plastic film mulching on improving soil organic carbon and nitrogen fractions, crop yield and water use efficiency in the Loess Plateau, China. Agric. Water Manage. 201, 133–143. doi: 10.1016/j.agwat.2018.01.021
Yanni, S. F., Helgason, B. L., Janzen, H. H., Ellert, B. H., and Gregorich, E. G. (2020). Warming effects on carbon dynamics and microbial communities in soils of diverse texture. Soil Biol. Biochem. 140, 107631. doi: 10.1016/j.soilbio.2019.107631
Yevdokimov, I., Ruser, R., Buegger, F., Marx, M., and Munch, J. (2006). Microbial immobilisation of 13C rhizodeposits in rhizosphere and root-free soil under continuous 13C labelling of oats. Soil Biol. Biochem. 38, 1202–1211. doi: 10.1016/j.soilbio.2005.10.004
Yu, P. (2017). Distribution of Photosynthetic Carbon and Fertilizer Nitrogen in Rice-Soil System Relative to Rice Growth Stages. Master Degree, Shenyang Agricultural University.
Zhang, Y., Hou, W., Chi, M., Sun, Y., An, J., Yu, N., et al. (2020). Simulating the effects of soil temperature and soil moisture on CO2 and CH4 emissions in rice straw-enriched paddy soil. Catena 194, 104677. doi: 10.1016/j.catena.2020.104677
Zhao, Y. L., Guo, H. B., Xue, Z. W., Mu, X. Y., and Li, C. H. (2015). Effects of tillage and straw returning on microorganism quantity, enzyme activities in soils and grain yield. Chin. J. Appl. Ecol. 26, 1785–1792 (in Chinese with English abstract).
Zhu, S., Dou, S., and Chen, L. Z. (2015). Effect of deep application of straw on composition of humic acid in soil aggregates. Acta Pedolog. Sin. 52, 747–758 (in Chinese with English abstract).
Keywords: straw return, 13C pulse-labeling, net photosynthetic rate, photosynthetic C partitioning, soil depth
Citation: Wang X-X, Li J, Wang D, An T, Qin W, Zou H and Rengel Z (2022) Straw Incorporation Effects on Net Photosynthetic Carbon Assimilation and Maize Growth. Front. Agron. 4:805320. doi: 10.3389/fagro.2022.805320
Received: 11 November 2021; Accepted: 25 January 2022;
Published: 02 March 2022.
Edited by:
Subhash Babu, Indian Agricultural Research Institute (ICAR), IndiaReviewed by:
Vivek Yadav, Northwest A&F University, ChinaRam Swaroop Bana, Indian Agricultural Research Institute (ICAR), India
Copyright © 2022 Wang, Li, Wang, An, Qin, Zou and Rengel. This is an open-access article distributed under the terms of the Creative Commons Attribution License (CC BY). The use, distribution or reproduction in other forums is permitted, provided the original author(s) and the copyright owner(s) are credited and that the original publication in this journal is cited, in accordance with accepted academic practice. No use, distribution or reproduction is permitted which does not comply with these terms.
*Correspondence: Hongtao Zou, em91aG9uZ3RhbzIwMDFAMTYzLmNvbQ==
†These authors have contributed equally to this work