- 1Centro de Investigaciones en Productos Naturales (CIPRONA) and Escuela de Biología, Universidad de Costa Rica, San José, Costa Rica
- 2Centro Nacional de Innovaciones Biotecnológicas (CENIBiot), Centro Nacional de Alta Tecnología, Consejo Nacional de Rectores (CeNAT-CONARE)-CONARE, San José, Costa Rica
- 3Escuela de Agronomía and Estación Experimental Fabio Baudrit, Universidad de Costa Rica, San José, Costa Rica
- 4Centro de Investigación en Contaminación Ambiental (CICA), Universidad de Costa Rica, San José, Costa Rica
- 5Department of Plant Sciences and Landscape Architecture, University of Maryland, College Park, MD, United States
The transition from conventional to organic agriculture is often challenged by the adaptation of biological control agents to environments heavily exposed to agrochemical pollutants. We studied Trichoderma species isolated from living leaf tissues of wild Rubiacaeae (coffee family) plants to determine their fungicide tolerance and potential for bioremoval. First, we assessed the in vitro tolerance to fungicides of four Trichoderma isolates (Trichoderma rifaii T1, T. aff. crassum T2, T. aff. atroviride T3, and T. aff. strigosellum T4) by placing mycelial plugs onto solid media supplemented with seven different systemic and non-systemic fungicides. After a week, most of the fungicides did not significantly inhibit the growth of the isolates, except in the case of cyproconazole, where the only isolate able to grow was T1; however, the colony morphology was affected by the presence of fungicides. Second, biological removal potential was established for selected isolates. For this experiment, the isolates T1, T2, and T4 were independently inoculated into liquid media with the fungicides azoxystrobin, chlorothalonil, cyproconazole, and trifloxystrobin. After 14 days of incubation, a removal of up to 89% was achieved for chlorothalonil, 46.4% for cyproconazole, and 33.1% for trifloxystrobin using viable biomass. In the case of azoxystrobin, the highest removal (82.2%) occurred by adsorption to fungal biomass. Ecotoxicological tests in Daphnia magna revealed that T1 has the highest removal potential, achieving significant elimination of every fungicide, while simultaneously detoxifying the aqueous matrix (except in the case of cyproconazole). Isolate T4 also exhibited an intermediate efficiency, while isolate T2 was unable to detoxify the matrix in most cases. The removal and detoxification of cyproconazole failed with all the isolates. These findings suggest that endosphere of wild plants could be an attractive guild to find new Trichoderma species with promising bioremediation capabilities. In addition, the results demonstrate that attention should be placed when combining certain types of agrochemicals with antagonistic fungi in Integrated Pest and Disease Management strategies or when transitioning to organic agriculture.
Introduction
The rapidly growing demand for food has put high pressure on the environment through the abuse of pesticides, especially fungicides (Duhamel and Vandenkoornhuyse, 2013). This affects production costs of important crops around the world and threatens the livelihoods of millions of people (Jaramillo et al., 2011; Caffarra et al., 2012; Ramirez-Villegas et al., 2012). Coffee (Coffea arabica) is currently under great stress because its productivity and quality are affected by pathogenic fungi, causing enormous economic losses. One of the most affected regions is Central America, where coffee farmers are mainly small and low-income (Davis et al., 2012; Paterson et al., 2014; Rikxoort et al., 2014; Avelino et al., 2015; Talhinhas et al., 2017; Verhage et al., 2017). Because the quality of coffee is correlated to adequate management against pathogens, safer environmental practices could help improve coffee cup quality (Feria-Morales, 2002). In addition, organic coffee demand has increased considerably because consumers are preferring food safety and agrochemical-free products (Lee et al., 2015). Therefore, sustainable and environmentally friendly disease management strategies, such as biological control, are urgently needed (Ayalew, 2014; Rice, 2018).
One of the great challenges in the transition from conventional to organic agriculture is the adaptation of introduced beneficial microorganisms (e.g., biological control and bioremediation agents) to a crop field with residual agrochemicals (Gharieb et al., 2004; Shen et al., 2019), or in areas where agrochemicals and antagonistic microorganisms are applied at the same time (Mishra et al., 2014; Nongmaithem, 2015; Palazzini et al., 2018). An alternative and rich guild of microorganisms adapted to these conditions are endophytic fungi, which inhabit the interior tissue of plants without causing apparent disease symptoms, facilitate plant adaptation to the environment, and may act as antagonists against phytopathogens; thus, they can be used as biological control and bioremediation agents (Harman, 2007; Howell, 2007; Stamatiu-Sánchez et al., 2014; Deng and Cao, 2017; Khan and Mohiddin, 2018; Holanda et al., 2019; Pietro-Souza et al., 2020).
Among common fungal endophytes of tropical plants and soil inhabitants, species of the genus Trichoderma have been used as successful biocontrollers in important crops (e.g., see review in Ghazanfar et al., 2018). In addition, some Trichoderma species can transform xenobiotic agents into non-toxic compounds, e.g., pesticides such as dichlorvos, cyanide pollutants, and even heavy metals (He et al., 2014). There are reports of Trichoderma atroviride, T. harzianum sensu lato, Trichoderma koningii, and T. viride sensu lato, capable of degrading e.g., alachlor, endosulfan, methyl-parathion, monochlorobenzene, neonicotinoids, and pentachlorophenol, among others (He et al., 2014; Vacondio et al., 2015; Cheng et al., 2017; Nunes and Malmlöf, 2018; Nykiel-Szymańska et al., 2018). Therefore, fungi that act as biocontrollers may also be capable of degrading residual pesticides in the fields or within plants, representing an important tool, not only for the transition from conventional to organic agriculture, but to complement different disease management strategies and recovery of “healthy” soil microbiomes.
Considering that: (i) tropical plants in natural forests harbor a high diversity of fungal endophytes (including Trichoderma) that generate a protective mutualism and can colonize internal plant tissues and soil (e.g., Evans et al., 2003; Chaverri and Samuels, 2013; Gazis and Chaverri, 2015; Pujade-Renaud et al., 2019); (ii) Neotropics is the Rubiaceae center of biodiversity (Manns et al., 2012); and (iii) Trichoderma possess a toolbox of enzymes and secondary metabolites which enable its species to tolerate and degrade toxic chemicals (Morales-Barrera and Cristiani-Urbina, 2008; Asemoloye et al., 2019); we hypothesize that those fungi will have the ability to tolerate and remove systemic and non-systemic fungicides, and that could be potentially applied in coffee fields. In this work, screening of Trichoderma spp. from the endosphere of wild Rubiaceae was performed to test their fungicide biodegradation potential. The specific aims of this work were to: (i) determine the ability of the isolated Trichoderma spp. to grow in the presence of fungicides in solid phase; and (ii) evaluate their capacity to remove and detoxify different fungicides in liquid medium through analytical and ecotoxicological tests. The results from this study will contribute to the design of disease management strategies that include biocontrol agents that tolerate chemical fungicides and can, at the same time, colonize soil and the plant endosphere. This is particularly critical in Latin American countries, where agricultural practices are characterized by excessive use of agrochemicals, but also an increased demand to transition into more sustainable farming, including organic.
Materials and Methods
Identification of Trichoderma Isolates
Four isolates of Trichoderma were selected for the present study. The isolates were previously obtained from living leaf tissue of Rubiaceae plants from natural forests of Costa Rica and following protocols used in Gazis and Chaverri (2010). Fungi are preserved in 20% glycerol at −80°C and at room temperature at the Natural Products Research Centre (CIPRONA), University of Costa Rica.
Isolates were grown in Petri plates with potato-dextrose-agar (PDA) (DifcoTM Laboratories, Detroit, Michigan, U.S.A.) for ca. 7 days. Then, the mycelium was harvested from the surface for DNA extraction using the kit PrepManTM Ultra (Applied Biosystems, Foster City, California, U.S.A.). Polymerase Chain Reactions (PCR) were prepared using primers ITS 4 and ITS 5 (Schoch et al., 2012) for internal transcribed spacers nuclear ribosomal DNA (ITS); EF728Mf and EF1R primers (Carbone and Kohn, 1999) for a partial region of translation elongation factor 1-alpha (TEF); and RPB25F and RPB2-7CR primers (Liu et al., 1999) for a partial region of RNA polymerase II subunit (RPB2). The 25-mL PCR reaction consisted of 12.5 mL GoTaq® Green Master Mix (Promega Corporation, Madison, Wisconsin, U.S.A.), 1 mL forward primer, 1 mL reverse primer, 1 mL of dimethyl sulfoxide (DMSO), 0.5 mL of bovine serum albumin (BSA), 6 mL of UltraPureTM distilled water, and 3 mL genomic DNA template (Herrera et al., 2013; Abreu et al., 2014). PCR products were sent to Macrogen (now Psomagen, Maryland, U.S.A.) for purification and sequencing. Sequence edition and alignment were done in Geneious version 10.2.3 (Kearse et al., 2012). To assign taxonomy, BLASTn algorithm was performed using NCBI GenBank database, comparing the queries to type specimens (Robbertse et al., 2017). The recommended barcode for Trichoderma identification is TEF (Chaverri et al., 2015). Therefore, TEF sequences with >98% identity were assigned the matching taxon names. Those with 90–97% identity were given the abbreviation “aff.” (=affinis), which means that it has affinity with but is not identical to the assigned species. Matches using ITS and RPB2 served to support those produced for TEF. Newly generated sequences have been deposited in GenBank (Supplementary Table 1).
Fungicide Tolerance Test in Solid Phase
The ability to grow in solid medium containing residual fungicides was assayed for four isolates. Seven fungicides commonly used in coffee plantations were tested: azoxystrobin (systemic), chlorothalonil (non-systemic), cyproconazole (systemic), propineb (systemic), tolclofos-methyl (non-systemic), trifloxystrobin (systemic), and validamycin-A (non-systemic). (Toxicity and accumulation potential for some of those fungicides are discussed in Komárek et al., 2010 and Roman et al., 2021). Trichoderma isolates were cultured onto PDA, adding the dose (volume or mass) recommended for coffee: azoxystrobin (1 g/L), chlorothalonil (5 mL/L), cyproconazole (2 mL/L), propineb (5 g/L), tolclofos-methyl (0.5 g/L), trifloxystrobin (0.5 g/L), and validamycin-A (5 mL/L). The concentrations of the active ingredients according to the commercial label are listed in Supplementary Table 2. The fungicides were added to the PDA after media sterilization to avoid their degradation. Mycelial plugs (~5 mm diam.) from 5-day-old cultures were placed 5 mm from the plate's edge. As fungal tolerance was observed with the full dosages, no additional concentrations or dilutions were done. Radial growth (in mm) was measured every 24 h for 5 days with a photoperiod of 12 h at 25°C ± 2. Light was used only in this experiment to induce sporulation; a large number of conidia are needed to produce the desired concentrations/dilutions. Consequently, for the next assay, only isolates T1, T2, and T4 were used because of their ability to produce abundant conidia for biomass production.
Fungicide Removal in Liquid Phase With Fungal Biomass
Biodegradation ability by three Trichoderma isolates (T1, T2, and T4) was evaluated against active ingredients of fungicides azoxystrobin, chlorothalonil, cyproconazole, and trifloxystrobin. An independent experiment was performed for each fungicide with three isolates separately (reaction systems, Rxn). Briefly, 1 × 106 spores/mL were inoculated into 250-mL flasks with potato-dextrose-broth (PDB) (Sigma-Aldrich, St. Louis, Missouri, U.S.A.). To obtain the desired volume of spore suspension for each flask, spore counting was performed in a Neubauer chamber in triplicate. With the average values, the number of spores per mL in the stock solution was obtained. After obtaining desired spore concentrations in the stock solution, exact amounts (in mL) were calculated to reach the final concentration of 1 × 106 spores/mL in a final volume of 50 mL. Commercial fungicides were added to the PDB media according to the recommended dose for coffee (Supplementary Table 2). The experiments were performed in triplicate, including: (i) abiotic controls (uninoculated) to evaluate degradation by abiotic factors; (ii) heat-killed controls (HKC; containing autoclaved biomass previously grown at identical conditions, but lacking the fungicide) to evaluate adsorption; and (iii) growth control (viable fungal biomass without fungicide) to determine the final dry weight. All cultures were grown for 14 days on a rotary shaker (250 rpm) (Thermo Fisher Scientific, Dubuque, Iowa, U.S.A.) at 21°C in the dark. These experiments were done in the dark to avoid degradation by photolysis.
To determine the fungicide concentration, 1.6 mL of the supernatant was collected from all the replicates at 2, 4, 7, 9, 11, and 14 days. Finally, on day 14, 20 mL samples were taken to determine biomass dry weight by filter-separating the mycelia and subsequently drying at 60°C (overnight) to reach a constant weight. After fungicide quantification (section Quantification of Fungicides), total removal was defined as percentual difference between final fungicide concentration (14 d in the Rxn treatment) and initial concentration (time “zero” in the abiotic control); this includes abiotic, adsorption, and biological removal. Final biodegradation was calculated by subtracting removal due to adsorption (HKC) to the total removal. In the case adsorption was negligible, the highest removal value (adsorption or abiotic control) was subtracted from total removal to estimate final biodegradation.
Analytical Procedures
Sample Processing
Samples were diluted in methanol as follows: 25 μL of chlorothalonil sample was mixed with 1,975 μL methanol (70%), 200 μL cyproconazole with 1,800 μL methanol (50%), 900 μL trifloxystrobin with 900 μL methanol (100%), and 100 μL azoxystrobin with 1,900 μL methanol (100%). All the samples were vortexed and filtered with a PTFE (polytetrafluoroethylene) membrane (0.45 μm). The samples were collected in HPLC vials and stored at 4°C.
Quantification of Fungicides
HPLC analysis was performed using an Agilent 1200 system equipped with a 1200 photodiode array detector (Agilent Technologies, Santa Clara, California, U.S.A.). A volume of 50 μL was injected into a Phenomenex Luna C18 (250 × 4.6 mm, 5 mm) column at a flow rate of 1 mL/min maintained at 35°C. The mobile phase consisted of 0.1% (v/v) formic acid in water (solvent A) and 0.1% (v/v) formic acid in acetonitrile (solvent B). The gradient elution program was as follows: 0–10 min, B increased linearly from 45 to 85%; 10–15 min, isocratic at 85%; 15–20 min, B increased linearly up to 100%, followed by reconditioning of the column. Quantification was performed at a wavelength of 254 nm using calibration curves of analytical standards of each fungicide.
Ecotoxicity Assay: Immobilization Test in Daphnia magna
To determine whether the fungal removal process is environmentally relevant, an ecotoxicological assay was employed to confirm detoxification, as some transformation products from pesticide degradation might present higher toxicity than the original compound (Ruíz-Hidalgo et al., 2016). An acute toxicity test was performed with the freshwater microcrustacean D. magna using untreated samples (initial work pesticide solutions in liquid culture media) and samples collected after 14 days for each treatment (abiotic and heat-killed controls, and viable fungus). The immobilization test methodology is described in Ramírez-Morales et al. (2021). Briefly, sets of 10 daphnid neonates (<24 h) were placed in 25 mL vials and exposed to 10 mL of each dilution of the sample (prepared in moderately hard reconstituted water); triplicate sets per dilution were incubated in the dark at 23 ± 1°C for 48 h. After incubation, immobility was determined and assumed as mortality. Daphnia magna was employed as a benchmark organism due to its sensitivity to xenobiotics, wide distribution, short life cycle, and ease of culturing in the laboratory, which makes it one of the most widely used bioindicators (Tkaczyk et al., 2021). Moreover, the fungicides assayed in this work exhibit high toxicity toward D. magna, thus making it an ideal bioindicator to evaluate the detoxification linked to fungicide removal.
Statistics
All the statistics and graphics were performed using R Core Team (2020). The effect of the fungicides over the different Trichoderma isolates in solid phase was analyzed using a one-way Analysis of Variance (ANOVA) for each fungicide. Biodegradation data for each fungicide was processed to generate a multiple plot of concentration vs. time and sample variability was calculated as a standard deviation (±SD). The relative sample concentration that resulted in the immobilization of 50% of daphnids (EC50) for the ecotoxicity assay was estimated using the DRC package (Ritz et al., 2015).
Results
Identification of Trichoderma Isolates
Results of BLAST searches are summarized in Supplementary Table 1. In summary, TEF sequences matched to T. rifaii (T1), T. aff. crassum (T2), T. aff. atroviride (T3), and T. aff. strigosellum (T4), with 99, 95, 90, and 96% identity, respectively. Even though ITS and RPB2 are not the recommended barcodes for Trichoderma, the BLAST results show support for the close affinities resulting from TEF identifications.
Fungicide Tolerance Test in Solid Phase
The solid phase tolerance test performed for each fungicide showed that azoxystrobin (P = 0.0001154), chlorothalonil (P = 0.0016935), cyproconazole (P = 1.558 × 10−19), and tolclofos-methyl (P = 1.72 × 10−5) produced differences among Trichoderma isolates. Meanwhile, propineb (P = 0.704234), validamycin-A (P = 0.7296269), and trifloxystrobin (P = 0.3721598) had similar effect over the fungi. The result obtained with cyproconazole (Figure 1A) was particularly noteworthy because after 5 days of evaluation it produced the highest inhibition on Trichoderma isolates T2, T3, and T4, being T1 the only one able to grow (37 mm) in the presence of this compound. Chlorothalonil followed in inhibition effect, allowing <30% of growth for all isolates (Figure 1B). Azoxystrobin, propineb, and tolclofos-methyl allowed all Trichoderma isolates to grow constantly in PDA supplemented with the fungicide (Figure 2). Finally, when exposed to validamycin A, the four isolates were able to reach a growth like that observed in the control (Figure 1B).
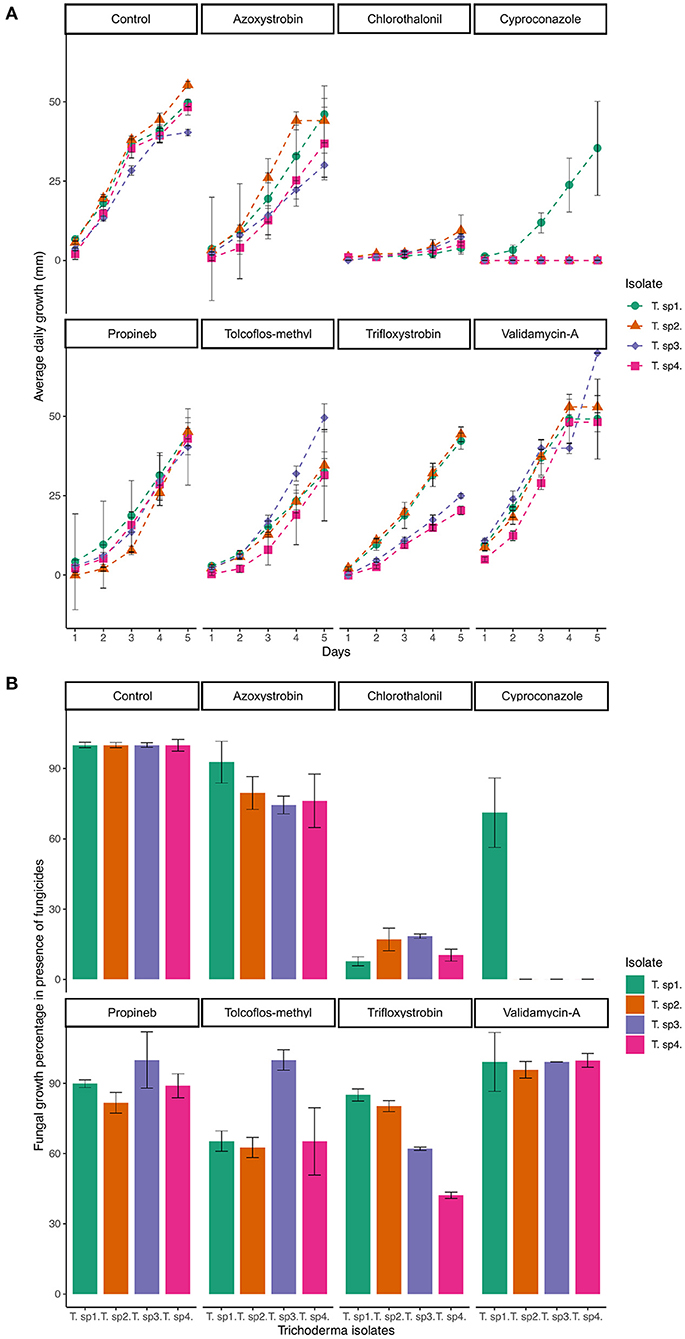
Figure 1. (A) Average daily growth of Trichoderma replicates during 5 days. Average was calculated using five replicates per fungicide. (B) Fungal growth percentages with respect to the control, in the presence of different fungicides in PDA.
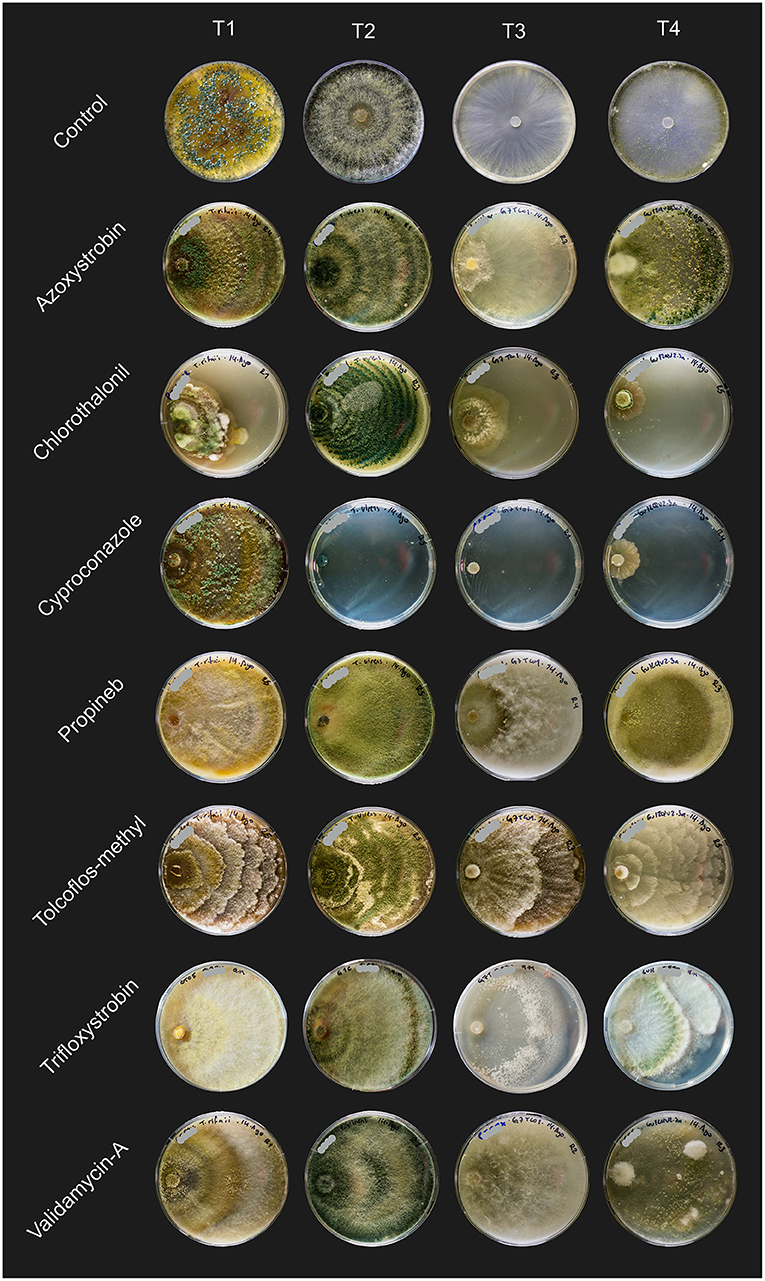
Figure 2. Morphological effects of mycelial growth after 15 days. The isolates in PDA control plates are placed in the upper side of the image. Downwards, different effects on aerial mycelium can be observed in the presence of the seven fungicides.
Even though the four isolates were able to tolerate and grow with most fungicides, some changes in the morphology patterns were observed after 15 days (Figure 2). For example, T1, which was able to grow in presence of all fungicides, produced mycelium, but not conidia in some cases. Moreover, occurrence of yellow diffusing pigment in the agar was constant in all T1 treatments. On the other hand, T2 produced concentric rings of mycelium and conidia when exposed to chlorothalonil, while T4 produced abundant green conidia in the medium containing azoxystrobin and validamycin-A, even more notably than the control. The colony surface of T3 was cottony in presence of propineb and validamycin-A, but string-like with tolclofos-methyl. Since conidia were absent from T3 even in the control, this isolate was discarded for the next assay, considering that easy and abundant sporulation for feasible propagation at a large scale is a desirable characteristic of a microorganism intended to be used as a biocontroller or in bioremediation (Hanada et al., 2010).
Fungicide Removal in Liquid Phase With Fungal Biomass
The Trichoderma isolates were able to grow in liquid phase with azoxystrobin, chlorothalonil, and trifloxystrobin. After 14 days of incubation, fungicide removals of up to 89% in chlorothalonil, 46% in cyproconazole, and 33% in trifloxystrobin were obtained. For azoxystrobin, higher removal occurred by adsorption onto fungal biomass (Figure 3).
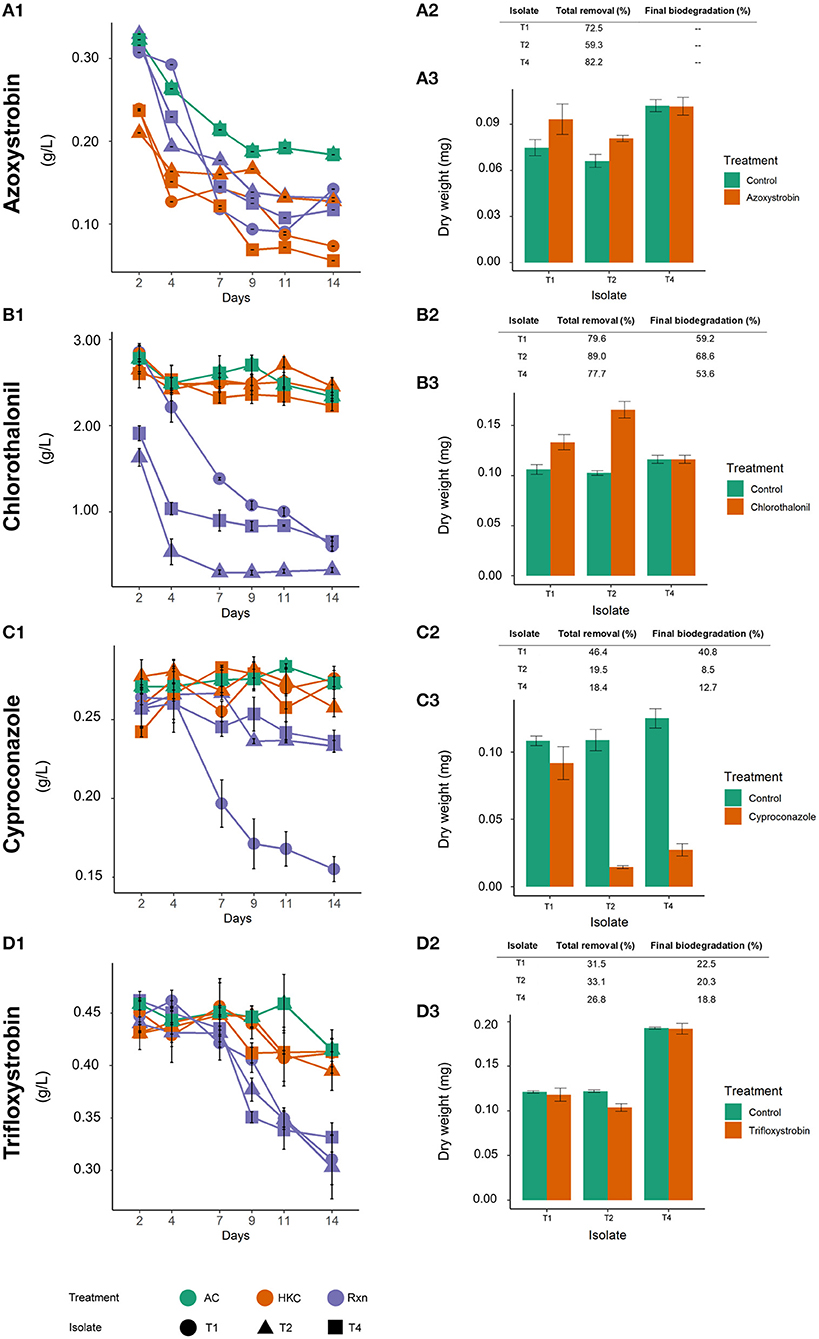
Figure 3. Fungicide removal profiles and final fungal dry weight of Trichoderma in the presence of azoxystrobin (A), chlorothalonil (B), cyproconazole (C), and trifloxystrobin (D). AC, Abiotic control; HKC, Heat-killed control; Rxn, viable fungal biomass treatment. In (A1–D1), numbers in Y axis (g/L) refer to concentration of active ingredient.
Chlorothalonil was the fungicide more efficiently removed. Removal in abiotic (20.4%) and HKC controls (18.4–24.2%) were similar; therefore, biomass adsorption was considered negligible. With a total removal of 89.0% after 14 days, T2 showed the highest biodegradation (68.6%) (Figure 3B1,B2). Similar total removal values of 79.6 and 77.7%, with an estimated biodegradation of 59.2 and 53.6% were achieved for T1 and T4, respectively. All tested Trichoderma isolates were able to grow in presence of chlorothalonil with a dry weight greater than or equal to the control (Figure 3B3).
Isolate T1 was able to grow in presence of cyproconazole, achieving the highest removal of 46.4% (Figure 3C1,C2). Considering the abiotic removal of 5.7%, final biodegradation was estimated at 40.8% (Figure 3C2). Lower removal was observed with T2 and T4, whose growth was severely affected by the fungicide, as the dry weight was notably lower than the respective controls (Figure 3C3). Final biodegradation was 8.5% for T2 and 12.7% for T4. Adsorption to the fungal biomass was only observed at a low extent for T2 (5.3%) (Figure 3C1,C2).
The presence of trifloxystrobin did not hinder fungal growth, as the three isolates were able to produce similar biomass amounts as the control (Figure 3D3). Total fungicide removal ranged from 26.8 to 33.1% for all tested strains. Considering abiotic losses of 8.4% and low adsorption (only in T2, 4.5%), final biodegradation was 22.5%, 20.3% and 18.0% for T1, T2, and T4, respectively (Figure 3D2).
Based on similar fungicide losses observed in abiotic and HKC (Figure 3B1,C1,D1), chlorothalonil, cyproconazole, and trifloxystrobin removal was mainly due to biodegradation, with a negligible role of adsorption. However, in the case of azoxystrobin, high removal occurred by adsorption (Figure 3A1). Biomass adsorption in the HKC ranged from 59.3 to 82.2%, while removal in Rxn treatments varied from 57.3 to 66.9% for all isolates (Figure 3A1,A2). This compound also exhibited the highest abiotic losses (43.3%), thus revealing estimated adsorption values of 29.2, 16.0, and 38.9% for T1, T2, and T4, respectively. Nonetheless, as suggested below by ecotoxicological assays, biodegradation, and not only adsorption, took place in the Rxn system. As in chlorothalonil and trifloxystrobin, azoxystrobin did not impair fungal growth in any isolate (Figure 3A3).
Ecotoxicological Evaluation of the Fungal Treatment With Immobilization Tests in D. magna
The results from the detoxification assays are shown in Table 1. Initial chlorothalonil working solution showed an EC50 value of 0.0050% (corresponding to 0.15 mg/L). After 14 d, both abiotic control and the HKC for every isolate revealed a slightly lower toxicity, similar to initial values (EC50 = 0.011–0.015%). However, toxicity clearly decreased in Rxn treatments with isolates T2 (EC50 = 0.16%), and especially for T1 (EC50 = 6.4%); detoxification was less evident for T4 (EC50 = 0.041%).
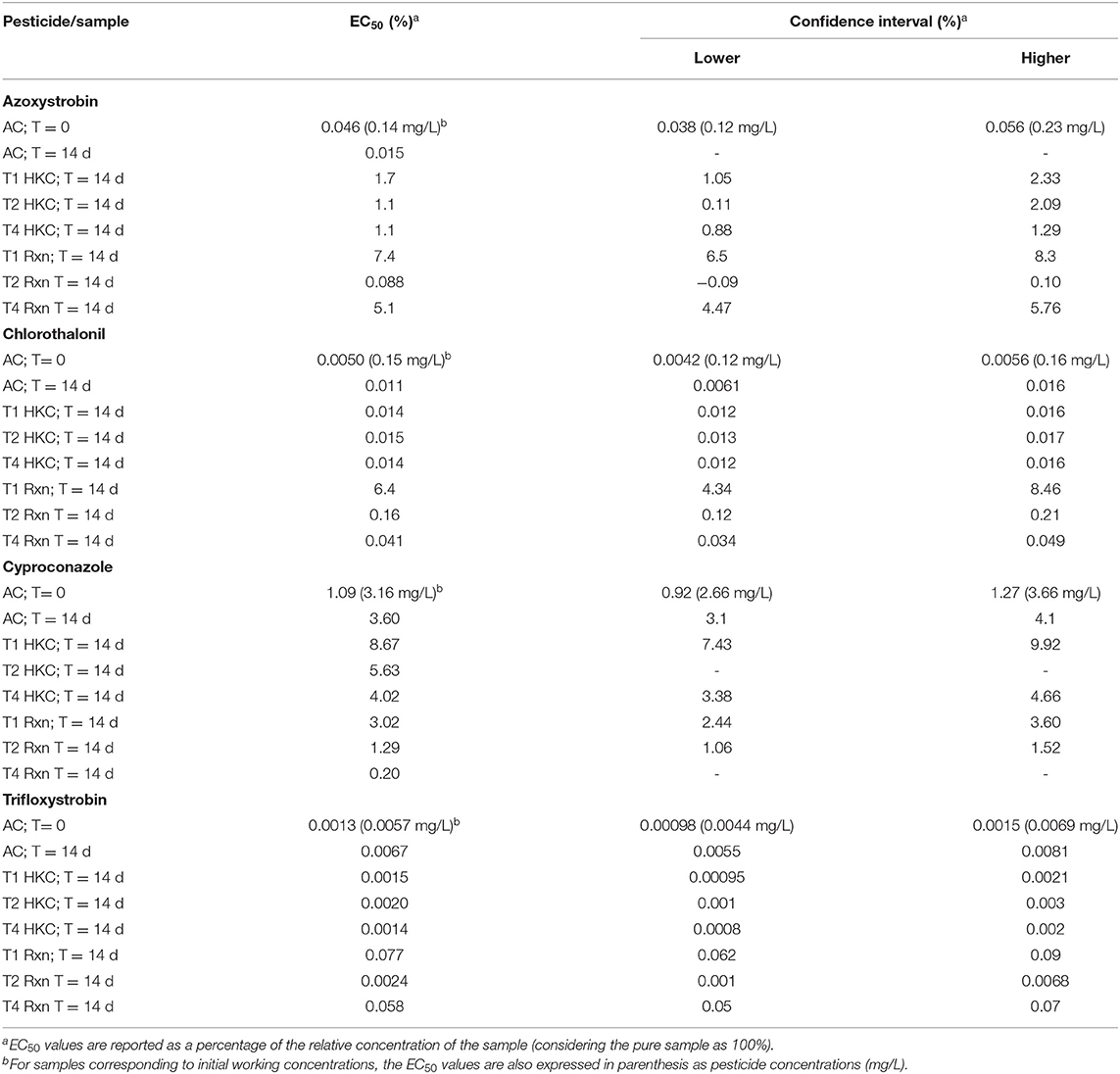
Table 1. Ecotoxicological evaluation of pesticides at initial working concentrations (abiotic control at T = 0 d) and after 14 d of treatment (AC, abiotic control; HKC, heat-killed control; Rxn, viable fungal biomass), according to immobilization tests in D. magna.
In cyproconazole, toxicity in the abiotic control decreased after 14 d (EC50 = 3.6%) with respect to the initial solution (EC50 = 1.09%). Additional detoxification was only slightly observed in HKC (increase in EC50 values from 1.1 to 2.4-fold, compared to 14-d abiotic control). However, for every isolate, toxicity was the same or higher in Rxn treatments (EC50 = 0.20–3.02%).
A 5-fold decrease was observed in trifloxystrobin abiotic control toxicity, compared to the initial solution (EC50 = 0.0067% vs. EC50 = 0.0013%). Nonetheless, HKC for every isolate showed similar toxicity to that achieved in the initial solution (EC50 = 0.0014–0.0020 %). On the contrary, Rxn treatments for T1 (EC50 = 0.077%) and T4 (EC50 = 0.058%), but not T2 (EC50 = 0.0024%), significantly decreased toxicity with respect to abiotic controls.
Finally, an increase in toxicity was determined for azoxystrobin in abiotic control after 14 d (EC50 = 0.015%), with respect to the initial solution (EC50 = 0.046%). Detoxification was achieved in HKC for all isolates, revealed as a two-order of magnitude increase in EC50 values (1.1–1.7%). Except for T2 (EC50 = 0.088%), whose toxicity was lower than the abiotic control, but slightly higher than that of the respective HKC, further detoxification was obtained in Rxn treatments T1 (EC50 = 7.4%) and T4 (EC50 = 5.1%).
Discussion
Ideally, microorganisms with biocontrol potential should tolerate agrochemicals to successfully prevail in transitional agricultural landscapes and provide benefits to plants against diseases (Sun et al., 2019). In addition, healthy soil microbiomes and ecosystems could be enhanced if biocontrol microorganisms aided in bioremoval or biodegradation of accumulated fungicides (Komárek et al., 2010; Roman et al., 2021). The results of our study show that all Trichoderma isolates tested have some level of fungicide tolerance and removal or biodegradation potential. Cyproconazole and chlorothalonil were the most toxic to Trichoderma, and propineb and validamycin-A the least. Tolerance to pesticides by Trichoderma species related to the T. harzianum complex recovered from soil have been previously reported for compounds such as pentachlorophenol (Rigot and Matsumura, 2002) or glyphosate (Levesque and Rahe, 1992). Recently, the tolerance and conidial survival in soil of several isolates of T. asperellum and T. koningiopsis to azoxystrobin and chlorothalonil, among other eleven fungicides, was demonstrated, resulting in a suggested use of T. asperellum in Integrated Pest Management strategies (Widmer, 2019). Moreover, its mycoparasitism mechanism remained even in the presence of azoxystrobin (da Silva et al., 2018).
Other mycoparasitic Trichoderma species, e.g., T. inhamatum, besides its reported use as a biocontrol agent, is also capable of transforming a highly toxic chromium into a less harmful form (Morales-Barrera and Cristiani-Urbina, 2008). Efforts to provide successful fungal biocontrol species resistant to agrochemicals has led to modification attempts of already-known biocontrol fungal species using different techniques, for example, UV-induced T. harzianum and T. atroviride mutants resistant to carbendazim and tebuconazole (Hatvani et al., 2006).
Our results indicate a high activity in chlorothalonil biodegradation by the selected Trichoderma isolates. In previous studies, chlorothalonil biodegradation was reported by some bacteria such as Sphingobium spp., Stenotrophomonas spp., and Ochrobactrum lupini, among others (Motonaga et al., 1996; Van Eeden et al., 2000; Shi et al., 2011; Man-yun et al., 2014; Hu et al., 2020). This action is possible due to the bacterial enzyme glutathione S-transferase (GST) that can biotransform chlorothalonil (Kim et al., 2004). In addition, this enzyme is responsible for herbicide detoxification (Scarponi et al., 1991; Neuefeind et al., 1997; Scarponi and Del Buono, 2009; Wang et al., 2010). However, in Trichoderma, the chlorothalonil biodegradation mechanism through the enzymatic action of GST has not been reported. Nonetheless, this enzyme is present in T. harzianum (Shibu et al., 2012; Bernal-Vicente et al., 2015), which is a member of the Harzianum clade; T. rifaii (T1) also belongs in that clade (Chaverri et al., 2015). Therefore, the biodegradation mechanism used by isolate T1 could be related to the expression of GST due to their close phylogenetic affinity (Chen and Zhuang, 2017). In addition, T. harzianum has been reported to achieve complete biotransformation of pentachlorophenol (Vacondio et al., 2015), a halogenated substituted monocyclic aromatic fungicide which exhibits a strong similarity to chlorothalonil at the chemical structure level (Gupta, 2017). High removal of this fungicide was observed for three isolates, including biodegradation estimated between 53.6 and 68.6%. Nonetheless, important detoxification was achieved only with isolates T1 (582-fold lower toxicity) and T2 (15-fold lower toxicity); residual toxicity observed in treatment with T4 was only 4-fold lower than the control, suggesting that transformation products of similar toxicity are released by the fungus during chlorothalonil metabolism.
The removal of cyproconazole by the fungi was not that successful. The isolate T1 was able to grow in its presence, while partially degrading it. It is known that species within the T. harzianum complex have the capacity to metabolize and tolerate tebuconazole, another triazole (Obanda and Shupe, 2009). For example, the major pathway for tebuconazole biodegradation consists in triazole ring cleavage and oxidation reactions (Obanda and Shupe, 2009; Wu et al., 2016, 2018). As cyproconazole has the same triazole ring, this pathway might be also used by T1.
Conversely, the same cyproconazole concentration resulted in toxic effects on T2 and T4. The growth of these two isolates was compromised, and consequently, their ability to remove the fungicide was low. In general, triazole fungicides are highly persistent in the environment (Bromilow et al., 1999; Murillo-Zamora et al., 2017), and cyproconazole exhibits one of the longest half-lives, making it very stable (Angioni et al., 2003). Biodegradation of azoles has been demonstrated for fungi such as Trametes versicolor and Fomitopsis palustris (Woo et al., 2010). Nonetheless, in one study, biodegradation of four triazoles with T. versicolor could not be achieved, even after fungal re-inoculation (Murillo-Zamora et al., 2017). This finding agrees with our results on low cyproconazole biodegradation efficiency observed with T2 and T4. Ecotoxicological evaluation revealed that, even though removal took place at some extent with the viable fungi, matrix detoxification did not occur. Moreover, residual toxicity was in every case higher than the control, suggesting formation of metabolites of higher toxicity than the parental compound, as it has been demonstrated in fungal degradation processes (Cruz-Morató et al., 2013; Cambronero-Heinrichs et al., 2018). Interestingly, slight but non-significant detoxification was achieved in HKC, supporting that in this case, the fungal-mediated reactions play a non-desirable role in final ecotoxicological outcome of cyproconazole transformation.
No growth inhibition was observed for strobilurins. Trichoderma resistance to trifloxystrobin has been previously reported (Kosanović et al., 2015); however, according to our results, efficiency ascribed to biodegradation was quite low for this fungicide. Despite low removal, detoxification was achieved by isolates T1 (11-fold lower toxicity than the abiotic control after 14 d) and T4 (9-fold lower), exhibiting a higher bioremediation potential than isolate T2, which produced a more toxic residue, at the same level as the HKC. For azoxystrobin, removal due to biodegradation could not be properly estimated, as that in the HKC surpassed the achieved with the viable biomass. At first sight, it could be inferred that all removal takes place by adsorption for all isolates, and that adsorption process is enhanced in thermally-inactivated biomass compared to viable fungi. This has been demonstrated for different kinds of biomass, including fungal, due to surface area modification (Bayramoglu and Arica, 2008; Legorreta-Castañeda et al., 2020). Nonetheless, when ecotoxicological analyses are considered, not only adsorption, but also biodegradation, is possibly taking place in Rxn systems using T1 and T4, in which significant detoxification was achieved (493 and 340-fold lower toxicity than abiotic control for T1 and T4, respectively). The extent of this detoxification was higher than that estimated in HKC by adsorption (and abiotic transformation), which accounted for 73–113-fold lower toxicity values than abiotic control, or for the Rxn isolate T2 (6-fold lower). The occurrence of adsorption does not imply a lack of degradation; on the contrary, degradation may be enhanced by physical proximity after adsorption has taken place, particularly when intracellular enzymes are involved in the process (Lucas et al., 2018; Tormo-Budowski et al., 2021). In general, information regarding strobilurins biodegradation is limited. So far, it is known that the major biological pathway for their degradation is methyl ester group hydrolysis in the toxophore (Clinton et al., 2011), which has been previously described in bacteria for azoxystrobin and trifloxystrobin (Clinton et al., 2011; Howell et al., 2014; Feng et al., 2020). Among fungi, two species of Aphanoascus are reported to biotransform azoxystrobin (Baćmaga et al., 2015).
Overall, the highest removal potential among isolates was observed for T1, which achieved considerable elimination of every fungicide, while simultaneously detoxifying the aqueous matrix (except in the case of cyproconazole). Isolate T4 also exhibited an intermediate efficiency, although with lower detoxification capacity than T1. Further research is needed to optimize their application in bioremediation of pesticides. On the other hand, isolate T2, despite being able to remove the fungicides at some extent, in most cases was unable to detoxify the matrix, making this fungus an unsuitable choice for biodegradation.
Lastly, three Trichoderma isolates were not confidently identified to species level, i.e., T2, T3, and T4, even though BLAST queries using ITS and RPB2 resulted in high similarity to known species. It has been shown that those two markers alone should not be used for identification (Chaverri et al., 2015; Cai and Druzhinina, 2021). We thus hypothesize that T2, T3, and T4 are new species because TEF sequences match to known species between 90 and 96% identity, below the recommended threshold for that marker (e.g., Chaverri et al., 2015; Cai and Druzhinina, 2021). On the other hand, we cannot confidently conclude that they are new species because additional evidence is needed (i.e., more isolates of the putative new species), as recommended for fungal taxonomy (e.g., see Seifert and Rossman, 2010).
Conclusions
In this study, endophytic Trichoderma isolates were able to tolerate and prevail under the presence of fungicides, generating more questions related to the mechanisms and effects of agrochemicals for the microorganisms living in agroecosystems. Assuming that these Trichoderma species can colonize soil and the endosphere of coffee plants (see Bailey et al., 2008; Pujade-Renaud et al., 2019 for examples on Theobroma and Hevea plants, respectively), and prevail in scenarios of conventional coffee production, then, their combined use with some low-toxicity fungicides (e.g., strobilurins and other compounds derived from microorganisms) could represent an alternative if transitioning to organic agriculture. Our Trichoderma isolates were not able to tolerate cyproconazole and chlorothalonil. Therefore, other compounds related to these groups of fungicides should be avoided when developing a strategy to migrate to organic or pesticide-free agriculture, as the same inhibition effect might occur on other beneficial fungi inhabiting the rhizo- or endosphere. Finally, considering its ability to remove fungicides while simultaneously decreasing their toxicity, isolate T1 seems the best candidate for fungicide bioremediation purposes. On the contrary, due to its poor removal and detoxification capacities, precautions should be taken with the isolate T2, as its use suggests a risk of transforming some fungicides into more hazardous metabolites. The combination of pesticide tolerance, removal, and ecotoxicity assays with biocontrol or bioremediation microorganisms, and interactions between agrochemicals, should be considered in the development of sustainable agriculture strategies.
Data Availability Statement
The original contributions presented in the study are included in the article/Supplementary Material, further inquiries can be directed to the corresponding author/s.
Author Contributions
PC, EE-L, and MG-M did the tolerance experiments. PA-V, EE-L, RM-A, CC-A, KV-M, MP-V, MM-R, CR-R, and JM-V conducted the bioremoval experiments. MP-V, MM-R, and CR-R conducted the ecotoxicity assays. EE-L, PA-V, and CR-R analyzed the data. EE-L, PA-V, JM-V, CR-R, and PC wrote the manuscript. PC conceived the study. JM-V and PC lead the study. All authors contributed to the article and approved the submitted version.
Funding
Funding was provided by Universidad de Costa Rica (grants 802-B8-510, 809-C1-604, and B7176), CONARE (grant FEES-809-B9-662), CENAT-CONARE Scholarships 2018 and 2019, U.S. National Science Foundation (DEB-1638976), CENIBiot (grant FP-022-2019), and MICITT (Costa Rica) (grants FI-048B-19 and FI-197B-17).
Conflict of Interest
The authors declare that the research was conducted in the absence of any commercial or financial relationships that could be construed as a potential conflict of interest.
Publisher's Note
All claims expressed in this article are solely those of the authors and do not necessarily represent those of their affiliated organizations, or those of the publisher, the editors and the reviewers. Any product that may be evaluated in this article, or claim that may be made by its manufacturer, is not guaranteed or endorsed by the publisher.
Acknowledgments
The authors thank CoopeTarrazú for providing the fungicide formulations.
Supplementary Material
The Supplementary Material for this article can be found online at: https://www.frontiersin.org/articles/10.3389/fagro.2021.772170/full#supplementary-material
References
Abreu, L. M., Moreira, G. M., Ferreira, D., Rodrigues-Filho, E., and Pfenning, L. H. (2014). Diversity of Clonostachys species assessed by molecular phylogenetics and MALDI-TOF mass spectrometry. Fungal Biol. 118, 1004–1012. doi: 10.1016/j.funbio.2014.10.001
Angioni, A., Aguilera Del Real, A., Russo, M., Melis, M., Cabitza, F., and Cabras, P. (2003). Triazole fungicide degradation in peaches in the field and in model systems. Food Addit. Contam. 20, 368–374. doi: 10.1080/0265203021000060904
Asemoloye, M. D., Jonathan, S. G., and Ahmad, R. (2019). Degradation of 2, 2-Dichlorovinyl dimethyl phosphate (dichlorvos) through the rhizosphere interaction between Panicum maximum Jacq and some selected fungi. Chemosphere 221, 403–411. doi: 10.1016/j.chemosphere.2019.01.058
Avelino, J., Cristancho, M., Georgiou, S., Imbach, P., Aguilar, L., Bornemann, G., et al. (2015). The coffee rust crises in Colombia and Central America (2008–2013): impacts, plausible causes and proposed solutions. Food Secur. 7, 303–321. doi: 10.1007/s12571-015-0446-9
Ayalew, T (2014). Characterization of organic coffee production, certification and marketing systems: Ethiopia as a main indicator: A Review Asian J. Agric. Res. 8, 170–180. doi: 10.3923/ajar.2014.170.180
Baćmaga, M., Kucharski, J., and Wyszkowska, J. (2015). Microbial and enzymatic activity of soil contaminated with azoxystrobin. Environ. Monit. Assess. 187:4827. doi: 10.1007/s10661-015-4827-5
Bailey, B. A., Bae, H., Strem, M. D., Crozier, J., Thomas, S. E., Samuels, G. J., et al. (2008). Antibiosis, mycoparasitism, and colonization success for endophytic Trichoderma isolates with biological control potential in Theobroma cacao. Biol. Control 46, 24–35. doi: 10.1016/j.biocontrol.2008.01.003
Bayramoglu, G., and Arica, M. Y. (2008). Removal of heavy mercury(II), cadmium(II) and zinc(II) metal ions by live and heat inactivated Lentinus edodes pellets. Chem. Eng. J. 143, 133–140. doi: 10.1016/j.cej.2008.01.002
Bernal-Vicente, A., Pascual, J. A., Tittarelli, F., Hernández, J. A., and Diaz-Vivancos, P. (2015). Trichoderma harzianum T-78 supplementation of compost stimulates the antioxidant defence system in melon plants. J. Sci. Food Agric. 95, 2208–2214. doi: 10.1002/jsfa.6936
Bromilow, R. H., Evans, A. A., and Nicholls, P. H. (1999). Factors affecting degradation rates of five triazole fungicides in two soil types: 2 field studies. Pestic. Sci. 55, 1135–1142. doi: 10.1002/(SICI)1096-9063(199912)55:12<1135::AID-PS73>3.0.CO;2-1
Caffarra, A., Rinaldi, M., Eccel, E., Rossi, V., and Pertot, I. (2012). Modelling the impact of climate change on the interaction between grapevine and its pests and pathogens: European grapevine moth and powdery mildew. Agric. Ecosyst. Environ. 148, 89–101. doi: 10.1016/j.agee.2011.11.017
Cai, F., and Druzhinina, I. S. (2021). In honor of John Bissett: authoritative guidelines on molecular identification of Trichoderma. Fungal Diversity 107, 1–69. doi: 10.1007/s13225-020-00464-4
Cambronero-Heinrichs, J. C., Masís-Mora, M., Quirós-Fournier, J. P., Lizano-Fallas, V., Mata-Araya, I., and Rodríguez-Rodríguez, C. E. (2018). Removal of herbicides in a biopurification system is not negatively affected by oxytetracycline or fungally pretreated oxytetracycline. Chemosphere 198, 198–203. doi: 10.1016/j.chemosphere.2018.01.122
Carbone, I., and Kohn, L. M. (1999). A method for designing primer sets for speciation studies in filamentous Ascomycetes. Mycologia 91, 553–556
Chaverri, P., Branco-Rocha, F., Jaklitsch, W., Gazis, R., Degenkolb, T., and Samuels, G. J. (2015). Systematics of the Trichoderma harzianum species complex and the re-identification of commercial biocontrol strains. Mycologia 107, 558–590. doi: 10.3852/14-147
Chaverri, P., and Samuels, G. J. (2013). Evolution of habitat preference and nutrition mode in a cosmopolitan fungal genus with evidence of interkingdom host jumps and major shifts in ecology. Evolution 67, 2823–2837. doi: 10.1111/evo.12169
Chen, K., and Zhuang, W. (2017). Discovery from a large-scaled survey of Trichoderma in soil of China. Sci. Rep. 2017, 1–37. doi: 10.1038/s41598-017-07807-3
Cheng, Z., Li, C., Kennes, C., Ye, J., Chen, D., Zhang, S., et al. (2017). Improved biodegradation potential of chlorobenzene by a mixed fungal-bacterial consortium. Int. Biodeterior. Biodegrad. 123, 276–285. doi: 10.1016/j.ibiod.2017.07.008
Clinton, B., Warden, A. C., Haboury, S., Easton, C. J., Kotsonis, S., Taylor, M. C., et al. (2011). Bacterial degradation of strobilurin fungicides: a role for a promiscuous methyl esterase activity of the subtilisin proteases? Biocatal. Biotransformation 29, 119–129. doi: 10.3109/10242422.2011.578740
Cruz-Morató, C., Jelić, A., Perez, S., Petrović, M., Barceló, D., Marco-Urrea, E., et al. (2013). Continuous treatment of clofibric acid by Trametes versicolor in a fluidized bed bioreactor: Identification of transformation products and toxicity assessment. Biochem. Eng. J. 75, 79–85. doi: 10.1016/j.bej.2013.03.020
da Silva, M. A. F., de Moura, K. E., de Moura, K. E., Salomão, D., and Patrício, F. R. A. (2018). Compatibility of Trichoderma isolates with pesticides used in lettuce crop. Summa Phytopathol. 44, 137–142. doi: 10.1590/0100-5405/176873
Davis, A. P., Gole, T. W., Baena, S., and Moat, J. (2012). The impact of climate change on indigenous arabica coffee (Coffea arabica): Predicting future trends and identifying priorities. PLoS ONE 7:e047981. doi: 10.1371/journal.pone.0047981
Deng, Z., and Cao, L. (2017). Fungal endophytes and their interactions with plants in phytoremediation: A review. Chemosphere 168, 1100–1106. doi: 10.1016/j.chemosphere.2016.10.097
Duhamel, M., and Vandenkoornhuyse, P. (2013). Sustainable agriculture: Possible trajectories from mutualistic symbiosis and plant neodomestication. Trends Plant Sci. 18, 597–600. doi: 10.1016/j.tplants.2013.08.010
Evans, H. C., Holmes, K. A., and Thomas, S. E. (2003). Endophytes and mycoparasites associated with an indigenous forest tree, Theobroma gileri, in Ecuador and a preliminary assessment of their potential as biocontrol agents of cocoa diseases. Mycol. Progress 2, 149–160. doi: 10.1007/s11557-006-0053-4
Feng, Y., Zhang, W., Pang, S., Lin, Z., Zhang, Y., Huang, Y., et al. (2020). Kinetics and new mechanism of azoxystrobin biodegradation by an Ochrobactrum anthropi strain SH14. Microorganisms 8:625. doi: 10.3390/microorganisms8050625
Feria-Morales, A. M (2002). Examining the case of green coffee to illustrate the limitations of grading systems/expert tasters in sensory evaluation for quality control. Food Qual. Prefer. 13, 355–367. doi: 10.1016/S0950-3293(02)00028-9
Gazis, R., and Chaverri, P. (2010). Diversity of fungal endophytes in leaves and stems of wild rubber trees (Hevea brasiliensis) in Peru. Fungal Ecol. 3, 240–254. doi: 10.1016/j.funeco.2009.12.001
Gazis, R., and Chaverri, P. (2015). Wild trees in the Amazon basin harbor a great diversity of beneficial endosymbiotic fungi: Is this evidence of protective mutualism? Fungal Ecol. 17, 18–29. doi: 10.1016/j.funeco.2015.04.001
Gharieb, M. M., Ali, M. I., and El-Shoura, A. A. (2004). Transformation of copper oxychloride fungicide into copper oxalate by tolerant fungi and the effect of nitrogen source on tolerance. Biodegradation 15, 49–57. doi: 10.1023/B:BIOD.0000009962.48723.df
Ghazanfar, M. U., Raza, M., Raza, W., and Qamar, M. I. (2018). Trichoderma as potential biocontrol agent, its exploitation in agriculture: a review. Plant Prot. 2, 109–135. Available online at: https://esciencepress.net/journals/index.php/PP/article/view/3142/1571
Gupta, P. K (2017). “Chapter 37 - Herbicides and Fungicides,” in Reproductive and Developmental Toxicology, ed. R. C. Gupta (New York, NY: Academic Press), 657–679. doi: 10.1016/B978-0-12-804239-7.00037-8
Hanada, R. E., Pomella, A. W. V., Costa, H. S., Bezerra, J. L., Loguercio, L. L., and Pereira, J. O. (2010). Endophytic fungal diversity in Theobroma cacao (cacao) and T. grandiflorum (cupuaçu) trees and their potential for growth promotion and biocontrol of black-pod disease. Fungal Biol. 114, 901–910. doi: 10.1016/j.funbio.2010.08.006
Harman, G. E (2007). Overview of mechanisms and uses of Trichoderma spp Phytopathology 96, 190–194. doi: 10.1094/phyto-96-0190
Hatvani, L., Manczinger, L., Kredics, L., Szekeres, A., Antal, Z., and Vágvölgyi, C. (2006). Production of Trichoderma strains with pesticide-polyresistance by mutagenesis and protoplast fusion. Int. J. Gen. Mol. Microbiol. 89, 387–393. doi: 10.1007/s10482-005-9042-x
He, X., Wubie, A. J., Diao, Q., Li, W., Xue, F., Guo, Z., et al. (2014). Biodegradation of neonicotinoid insecticide, imidacloprid by restriction enzyme mediated integration (REMI) generated Trichoderma mutants. Chemosphere 112, 526–530. doi: 10.1016/j.chemosphere.2014.01.023
Herrera, C., Rossman, A. Y., Samuels, G. J., Lechat, C., and Chaverri, P. (2013). Revision of the genus Corallomycetella with Corallonectria gen. nov. for C. jatrophae (Nectriaceae, Hypocreales). Mycosytema 32, 518–544.
Holanda, F. H., Birolli, W. G., Morais, E. S., Sena, I. S., Ferreira, A. M., et al. (2019). Study of biodegradation of chloramphenicol by endophytic fungi isolated from Bertholletia excelsa (Brazil nuts). Biocatal. Agric. Biotechnol. 20:101200. doi: 10.1016/j.bcab.2019.101200
Howell, C. C., Semple, K. T., and Bending, G. D. (2014). Isolation and characterisation of azoxystrobin degrading bacteria from soil. Chemosphere 95, 370–378. doi: 10.1016/j.chemosphere.2013.09.048
Howell, C. R (2007). Mechanisms employed by Trichoderma species in the biological control of plant diseases: the history and evolution of current concepts. Plant Dis. 87, 4–10. doi: 10.1094/pdis.2003.87.1.4
Hu, S., Cheng, X., Liu, G., Lu, Y., Qiao, W., Chen, K., et al. (2020). International biodeterioration & biodegradation degradation of chlorothalonil via thiolation and nitrile hydration by marine strains isolated from the surface seawater of the Northwestern Pacific. Int. Biodeterior. Biodegradation 154:105049. doi: 10.1016/j.ibiod.2020.105049
Jaramillo, J., Muchugu, E., Vega, F. E., Davis, A., Borgemeister, C., and Chabi-Olaye, A. (2011). Some like it hot: The influence and implications of climate change on coffee berry borer (Hypothenemus hampei) and coffee production in East Africa. PLoS ONE 6:e024528. doi: 10.1371/journal.pone.0024528
Kearse, M., Moir, R., Wilson, A., Stones-Havas, S., Cheung, M., Sturrock, S., et al. (2012). Geneious Basic: an integrated and extendable desktop software platform for the organization and analysis of sequence data. Bioinformatics 28, 1647–1649. doi: 10.1093/bioinformatics/bts199
Khan, M. R., and Mohiddin, F. A. (2018). “Chapter 13 - Trichoderma: Its Multifarious Utility in Crop Improvement,” in Crop Improvement Through Microbial Biotechnology, eds. R. Prasad, S. S. Gill, and N. Tuteja (Elsevier), 263–291. doi: 10.1016/B978-0-444-63987-5.00013-X
Kim, Y. M., Park, K., Joo, G. J., Jeong, E. M., Kim, J. E., and Rhee, I. K. (2004). Glutathione-dependent biotransformation of the fungicide clorothalonil. J. Agric. Food Chem. 52, 4192–4196. doi: 10.1021/jf040047u
Komárek, M. E., Cadková Chrastný, V., Bordas, F., and Bollinger, J. C. (2010). Contamination of vineyard soils with fungicides: A review of environmental and toxicological aspects, Environ. Int. 36, 138–151. doi: 10.1016/j.envint.2009.10.005
Kosanović, D., Potočnik, I., Vukojević, J., Stajić, M., Rekanović, E., Stepanović, M., et al. (2015). Fungicide sensitivity of Trichoderma spp. from Agaricus bisporus farms in Serbia. J. Environ. Sci. Heal. - Part B Pestic. Food Contam. Agric. Wastes 50, 607–613. doi: 10.1080/03601234.2015.1028849
Lee, K. H., Bonn, M. A., and Cho, M. (2015). Consumer motives for purchasing organic coffee: The moderating effects of ethical concern and price sensitivity. Int. J. Contemp. Hosp. Manag. 27, 1157–1180. doi: 10.1108/IJCHM-02-2014-0060
Legorreta-Castañeda, A. J., Lucho-Constantino, C. A., Beltrán-Hernández, R. I., Coronel-Olivares, C., and Vázquez-Rodríguez, G. A. (2020). Biosorption of water pollutants by fungal pellets. Water 12:1155. doi: 10.3390/W12041155
Levesque, C. A., and Rahe, J. E. (1992). Herbicide interactions with fungal root pathogens, with special reference to glyphosate. Annu. Rev. Phytopathol. 30, 579–602.
Liu, Y. J., Whelen, S., and Hall, B. D. (1999). Phylogenetic relationships among Ascomycetes: evidence from an RNA polymerase II subunit. Mol. Biol. Evol. 1999, 1799–1808.
Lucas, D., Castellet-Rovira, F., Villagrasa, M., Badia-Fabregat, M., Barceló, D., Vicent, T., et al. (2018). The role of sorption processes in the removal of pharmaceuticals by fungal treatment of wastewater. Sci. Total Environ. 610–611, 1147–1153. doi: 10.1016/j.scitotenv.2017.08.118
Manns, U., Wikström, N., Taylor, C.M., and Bremer, B. (2012). Historical biogeography of the predominantly neotropical subfamily cinchonoideae (Rubiaceae): Into or Out of America? Int. J. Plant Sci. 173, 261–286. doi: 10.1086/663971
Man-yun, Z., Ying, T., Ye, Z. H. U., Jun, W., Yong-ming, L. U. O., Christie, P., et al. (2014). Isolation and characterization of chlorothalonil-degrading bacterial strain H4 and its potential for remediation of contaminated soil. Pedosph. An Int. J. 24, 799–807. doi: 10.1016/S1002-0160(14)60067-9
Mishra, G., Kumar, N., Giri, K., Pandey, S., and Kumar, R. (2014). Effect of fungicides and bioagents on number of microorganisms in soil and yield of soybean (Glycine max). Nusant. Biosci. 6, 45–48. doi: 10.13057/nusbiosci/n060108
Morales-Barrera, L., and Cristiani-Urbina, E. (2008). Hexavalent chromium removal by a Trichoderma inhamatum fungal strain isolated from tannery effluent. Water. Air. Soil Pollut. 187, 327–336. doi: 10.1007/s11270-007-9520-z
Motonaga, K., Takagi, K., and Matumoto, S. (1996). Biodegradation of chlorothalonil in soil after suppression of degradation. Biol. Fertil. Soils 23, 340–345. doi: 10.1007/BF00335964
Murillo-Zamora, S., Castro-Gutiérrez, V., Masís-Mora, M., Lizano-Fallas, V., and Rodríguez-Rodríguez, C. E. (2017). Elimination of fungicides in biopurification systems: Effect of fungal bioaugmentation on removal performance and microbial community structure. Chemosphere 186, 625–634. doi: 10.1016/j.chemosphere.2017.07.162
Neuefeind, T., Reinemer, P., and Bieseler, B. (1997). Plant glutathione S-transferases and herbicide detoxification. Biol. Chem. 378, 199–205.
Nongmaithem, N (2015). Compatibility of pesticides with Trichoderma spp. and their antagonistic potential against some pathogenic soil borne pathogens. Indian J. Agric. Res. 49, 193–196. doi: 10.5958/0976-058X.2015.00030.X
Nunes, S., and Malmlöf, K. (2018). Enzymatic decontamination of antimicrobials, phenols, heavy metals, pesticides, polycyclic aromatic hydrocarbons, dyes, and animal waste. Enzym. Hum. Anim. Nutr. Princ. Perspect. 331–359. doi: 10.1016/B978-0-12-805419-2.00017-4
Nykiel-Szymańska, J., Bernat, P., and Słaba, M. (2018). Potential of Trichoderma koningii to eliminate alachlor in the presence of copper ions. Ecotoxicol. Environ. Saf. 162, 1–9. doi: 10.1016/j.ecoenv.2018.06.060
Obanda, D. N., and Shupe, T. F. (2009). Biotransformation of tebuconazole by microorganisms: Evidence of a common mechanism. Wood Fiber Sci. 41, 157–167.
Palazzini, J. M., Torres, A. M., and Chulze, S. N. (2018). Tolerance of triazole-based fungicides by biocontrol agents used to control Fusarium head blight in wheat in Argentina. Lett. Appl. Microbiol. 66, 434–438. doi: 10.1111/lam.12869
Paterson, R. R. M., Lima, N., and Taniwaki, M. H. (2014). Coffee, mycotoxins and climate change. Food Res. Int. 61, 1–15. doi: 10.1016/j.foodres.2014.03.037
Pietro-Souza, W., de Campos Pereira, F., Mello, I. S., Stachack, F. F. F., Terezo, A. J., Cunha, C. N., et al. (2020). Mercury resistance and bioremediation mediated by endophytic fungi. Chemosphere 240:124874. doi: 10.1016/j.chemosphere.2019.124874
Pujade-Renaud, V., Déon, M., Gazis, R., Ribeiro, S., Dessailly, F., Granet, F., et al. (2019). Endophytes from wild rubber trees as antagonists of the pathogen Corynespora cassiicola. Phytopathology 109, 1888–1899. doi: 10.1094/PHYTO-03-19-0093-R
R Core Team (2020). R: A language and environment for statistical computing. R Foundation for Statistical Computing, Vienna, Austria. Available online at: https://www.R-project.org/
Ramírez-Morales, D., Masís-Mora, M., Beita-Sandí, W., Montiel-Mora, J. R., Fernández-Fernández, E., Méndez-Rivera, M., et al. (2021). Pharmaceuticals in farms and surrounding surface water bodies: hazard and ecotoxicity in a swine production area in Costa Rica. Chemosphere 272:129574. doi: 10.1016/j.chemosphere.2021.129574
Ramirez-Villegas, J., Salazar, M., Jarvis, A., and Navarro-Racines, C. E. (2012). A way forward on adaptation to climate change in Colombian agriculture: Perspectives towards 2050. Clim. Change 115, 611–628. doi: 10.1007/s10584-012-0500-y
Rice, R. A (2018). Coffee in the crosshairs of climate change: agroforestry as abatis. Agroecol. Sustain. Food Syst. 42, 1058–1076. doi: 10.1080/21683565.2018.1476428
Rigot, J., and Matsumura, F. (2002). Assessment of the rhizosphere competency and pentachlorophenol-metabolizing activity of a pesticide-degrading strain of Trichoderma harziamum introduced into the root zone of corn seedlings. J. Environ. Sci. Heal. B 37, 201–210.
Rikxoort, H., Schroth, G., Läderach, P., and Rodríguez-Sánchez, B. (2014). Carbon footprints and carbon stocks reveal climate-friendly coffee production. Agron. Sustain. Dev. Springer-Verlag/EDP Sci. 34, 887–897. doi: 10.1007/s13593-014-0223-8
Ritz, C., Baty, F., Streibig, J. C., and Gerhard, D. (2015). Dose-response analysis using R. PLoS ONE 10:e146021. doi: 10.1371/journal.pone.0146021
Robbertse, B., Strope, P., Chaverri, P., Gazis, R., and Schoch, C. L. (2017). Taxonomic accuracy for fungi in public sequence databases: applying ‘One name one species' in well-defined genera with Trichoderma/Hypocrea as a test case. Database 2017:bax072. doi: 10.1093/database/bax072
Roman, D. L., Voiculescu, D. I., Filip, M., Ostafe, V., and Isvoran, A. (2021). Effects of triazole fungicides on soil microbiota and on the activities of enzymes found in soil: a review. Agriculture 11:893. doi: 10.3390/agriculture11090893
Ruíz-Hidalgo, K., Masís-Mora, M., Barbieri, E., Carazo-Rojas, E., and Rodríguez-Rodríguez, C. E. (2016). Ecotoxicological analysis during the removal of carbofuran in fungal bioaugmented matrices. Chemosphere 144, 864–871. doi: 10.1016/j.chemosphere.2015.09.056
Scarponi, L., and Del Buono, D. (2009). Festuca arundinacea, glutathione S-transferase and herbicide safeners: A preliminary case study to reduce herbicidal pollution. J. Environ. Sci. Heal. - Part B Pestic. Food Contam. Agric. Wastes 44, 805–809. doi: 10.1080/03601230903238400
Scarponi, L., Perucci, P., and Martinetti, L. (1991). Conjugation of 2-chloroacetanilide herbicides with glutathione: role of molecular structures and of glutathione s-transferase enzymes. J. Agric. Food Chem. 39, 2010–2013. doi: 10.1021/jf00011a027
Schoch, C. L., Seifert, K. A., Huhndorf, S., Robert, V, Spouge, J. L., Levesque, A., et al. (2012). Nuclear ribosomal internal transcribed spacer (ITS) region as a universal DNA barcode marker for Fungi. Proc. Natl. Acad. Sci. U.S.A. 109, 6241–6246. doi: 10.1073/pnas.1117018109
Seifert, K. A., and Rossman, A. Y. (2010). How to describe a new fungal species. IMA Fungus 1, 109–116. doi: 10.5598/imafungus.2010.01.02.02
Shen, F. T., Yen, J. H., Liao, C., Chen, W. C., and Chao, Y. T. (2019). Screening of rice endophytic biofertilizers with fungicide tolerance and plant growth-promoting characteristics. Sustain. 11:1133. doi: 10.3390/su11041133
Shi, X. Z., Guo, R. J., Takagi, K., Miao, Z. Q., and Li, S. D. (2011). Chlorothalonil degradation by Ochrobactrum lupini strain TP-D1 and identification of its metabolites. World J. Microbiol. Biotechnol. 27, 1755–1764. doi: 10.1007/s11274-010-0631-0
Shibu, M. A., Hong-Shin, L., Hsueh-Hui, Y., and Kou-Cheng, P. (2012). Trichoderma harzianum ETS 323-mediated resistance in Brassica oleracea var. capitata to Rhizoctonia solani involves the novel expression of a glutathione S-transferase and a deoxycytidine deaminase. J. Agric. Food Chem. 60, 10723–10732. doi: 10.1021/jf3025634
Stamatiu-Sánchez, K., Alarcón, A., Ferrera-Cerrato, R., Nava-Díaz, C., Sánchez-Escudero, J., Cruz-Sánchez, J. S., et al. (2014). Tolerancia de hongos filamentosos a endosulfán, clorpirifós y clorotalonil en condiciones in vitro. Rev. Int. Contam. Ambient. 31, 23–37. Available online at: http://www.scielo.org.mx/pdf/rica/v31n1/v31n1a2.pdf
Sun, J., Zhang, T., Li, Y., Wang, X., and Chen, J. (2019). Functional characterization of the ABC transporter TaPdr2 in the tolerance of biocontrol the fungus Trichoderma atroviride T23 to dichlorvos stress. Biol. Control 129, 102–108. doi: 10.1016/j.biocontrol.2018.10.004
Talhinhas, P., Batista, D., Diniz, I., Vieira, A., Silva, D. N., Loureiro, A., et al. (2017). The coffee leaf rust pathogen Hemileia vastatrix: one and a half centuries around the tropics. Mol. Plant Pathol. 18, 1039–1051. doi: 10.1111/mpp.12512
Tkaczyk, A., Bownik, A., Dudka, J., Kowal, K., and Slaska, B. (2021). Daphnia magna model in the toxicity assessment of pharmaceuticals: A review. Sci. Total Environ. 763:143038. doi: 10.1016/j.scitotenv.2020.143038
Tormo-Budowski, R., Cambronero-Heinrichs, J. C., Durán, J. E., Masís-Mora, M., Ramírez-Morales, D., Quirós-Fournier, J. P., et al. (2021). Removal of pharmaceuticals and ecotoxicological changes in wastewater using Trametes versicolor: A comparison of fungal stirred tank and trickle-bed bioreactors. Chem. Eng. J. 410:128210. doi: 10.1016/j.cej.2020.128210
Vacondio, B., Birolli, W. G., Ferreira, I. M., Seleghim, M. H. R., Gonçalves, S., Vasconcellos, S. P., et al. (2015). Biodegradation of pentachlorophenol by marine-derived fungus Trichoderma harzianum CBMAI 1677 isolated from ascidian Didemnun ligulum. Biocatal. Agric. Biotechnol. 4, 266–275. doi: 10.1016/j.bcab.2015.03.005
Van Eeden, M., Potgieter, H. C., and Van Der Walt, A. M. (2000). Microbial degration of chlorothalonil in agricultural soil: A laboratory investigation. Environ. Toxicol. 15, 533–539. doi: 10.1002/1522-7278(2000)15:5<533::AID-TOX25>3.0.CO;2-D
Verhage, F. Y. F., Anten, N. P. R., and Sentelhas, P. C. (2017). Carbon dioxide fertilization offsets negative impacts of climate change on Arabica coffee yield in Brazil. Clim. Change 144, 671–685. doi: 10.1007/s10584-017-2068-z
Wang, J., Jiang, Y., Chen, S., Xia, X., Shi, K., Zhou, Y., et al. (2010). The different responses of glutathione-dependent detoxification pathway to fungicide chlorothalonil and carbendazim in tomato leaves. Chemosphere 79, 958–965. doi: 10.1016/j.chemosphere.2010.02.020
Widmer, T. L (2019). Compatibility of Trichoderma asperellum isolates to selected soil fungicides. Crop Prot. 120, 91–96. doi: 10.1016/j.cropro.2019.02.017
Woo, C., Daniels, B., Stirling, R., and Morris, P. (2010). Tebuconazole and propiconazole tolerance and possible degradation by Basidiomycetes: A wood-based bioassay. Int. Biodeterior. Biodegrad. 64, 403–408. doi: 10.1016/j.ibiod.2010.01.009
Wu, H., Shen, J., Jiang, X., Liu, X., Sun, X., Li, J., et al. (2018). Bioaugmentation strategy for the treatment of fungicide wastewater by two triazole-degrading strains. Chem. Eng. J. 349, 17–24. doi: 10.1016/j.cej.2018.05.066
Keywords: biodegradation, bioremediation, ecotoxicity, fungal endophytes, organic agriculture, Trichoderma
Citation: Escudero-Leyva E, Alfaro-Vargas P, Muñoz-Arrieta R, Charpentier-Alfaro C, Granados-Montero MdM, Valverde-Madrigal KS, Pérez-Villanueva M, Méndez-Rivera M, Rodríguez-Rodríguez CE, Chaverri P and Mora-Villalobos JA (2022) Tolerance and Biological Removal of Fungicides by Trichoderma Species Isolated From the Endosphere of Wild Rubiaceae Plants. Front. Agron. 3:772170. doi: 10.3389/fagro.2021.772170
Received: 09 September 2021; Accepted: 30 December 2021;
Published: 03 February 2022.
Edited by:
Mauricio Schoebitz, University of Concepcion, ChileReviewed by:
Ernesto A. Moya-Elizondo, University of Concepcion, ChileAngela Machuca, Universidad de Concepcion, Chile
Natalia Paulucci, National University of Río Cuarto, Argentina
Copyright © 2022 Escudero-Leyva, Alfaro-Vargas, Muñoz-Arrieta, Charpentier-Alfaro, Granados-Montero, Valverde-Madrigal, Pérez-Villanueva, Méndez-Rivera, Rodríguez-Rodríguez, Chaverri and Mora-Villalobos. This is an open-access article distributed under the terms of the Creative Commons Attribution License (CC BY). The use, distribution or reproduction in other forums is permitted, provided the original author(s) and the copyright owner(s) are credited and that the original publication in this journal is cited, in accordance with accepted academic practice. No use, distribution or reproduction is permitted which does not comply with these terms.
*Correspondence: Priscila Chaverri, cGNoYXZlcnJAdW1kLmVkdQ==
†These authors have contributed equally to this work