- 1Crop Health, Faculty of Agricultural and Environmental Sciences, University of Rostock, Rostock, Germany
- 2Grassland and Forage Sciences, Faculty of Agricultural and Environmental Sciences, University of Rostock, Rostock, Germany
Ensiling, a lactic acid fermentation process, is mainly used to preserve biomass. In addition, it has been shown to affect seed viability of some plant species. The extent to which this makes ensiling suitable as a weed control measure, however, has not yet been determined. Both the range of controllable species and the parameters of an ensiling process that safely kills seeds are still undefined. We aimed to determine the effect of varying substrate and ensiling conditions on the seed viability of 10 species selected to represent a wide range of different seed traits. Five different types of silages were made from maize or mixtures of wildflower and maize biomass and ensiled in lab-scale silos for 8 months. The pure maize silages were prepared under conditions either ideal or suboptimal for ensiling forage. Seeds of important weeds (Chenopodium album, Abutilon theophrasti) and of species from a wildflower mixture suitable for ensiling and biogas production (Cichorium intybus, Daucus carota, Echium vulgare, Malva alcea, Malva sylvestris, Melilotus albus, Melilotus officinalis) were tested. Seed viability was determined using a combination of tetrazolium and germination tests. Ensiling reduced seed viability across all 10 species significantly. Seed-killing efficacies of ensiling, however, differed widely among the species studied, largely related to whether the species could produce hard (physically dormant) seeds. Seeds from species without hardseededness were completely inactivated by ensiling, while the seed-killing efficacies for hardseeded species ranged from 5 to 60%. Variation in ensiled substrate and ensiling conditions had no consistent effect on seed survival. We concluded that ensiling has the potential to sustainably reduce seed viability of a wide range of species and therefore should be adopted as a component of integrated weed management in organic agriculture.
Introduction
In order to meet the growing demand for food in a sustainable way (El Bilali et al., 2019), it is essential that environmentally friendly approaches such as organic farming also achieve high and secure yields (Reganold and Wachter, 2016). Weeds have the potential to jeopardize these goals especially in organic cropping systems that have to manage without chemical herbicides (Stonehouse et al., 1996; Hatcher and Froud-Williams, 2017). Today, there is a broad consensus among agronomists worldwide that integrated weed management is needed to control weeds efficiently (Merfield, 2019; MacLaren et al., 2020). Tillage, crop rotation, intercropping, and increase of crop competitiveness are repeatedly referred to as tools of integrated weed management in organic farming (Barberi, 2002). Ensiling, i.e., the process of conserving biomass by promoting lactic acid fermentation, however, seems to be hardly perceived as a weed control measure in the current discussion. This is surprising, since ensiling was recognized very early as a measure for weed seed reduction, along with other agricultural fermentation processes such as bovine digestion and farmyard manure rotting (Atkeson et al., 1934; Shevkenek, 1934; Tildesley, 1937; Zahnley and Fitch, 1941). In addition, organic mixed farms in particular offer good conditions for the integration of such fermentation cascades, as they aim to close biomass and nutrient cycles (Barker, 2021). Furthermore, the contribution of agricultural fermentation processes to reducing the spread of weed seeds is also relevant in light of decades of herbicide use. Consequently, the weed-reducing potential of fermentation processes is receiving attention from various scientific communities such as forage scientists and seed biologists still (Takabayashi et al., 1975; Pleasant and Schlather, 1994; Michael et al., 2006). Particularly with regard to the germinability of weed seeds, there has been a recent resurgence of interest in the effects of ensiling (Mayer et al., 2000; Overud, 2002; Westerman et al., 2012).
With regard to weed seed load, nowadays, especially in extensively managed systems, a larger amount of seeds than in the past enters the biomass streams and thus the silos. In intensive forage production, early cutting dates hardly allow for any significant weed seed formation. One of the reasons for the higher seed load in extensive agriculture is the high proportion of leys in the forage areas designated for ensiling in organic farming systems (Döring et al., 2017). Especially during dry periods during the ley establishing phase, many seed-forming weeds emerge (Brainard et al., 2011) and lead to notable weed contamination of the forage. Another cause of the increasing weed seed load is the growing number of agri-environmental measures such as field-margin protection schemes and the integration of flowering mixtures into farming systems (Marshall, 2005) that are being adopted by organic farms (Hald and Nielsen, 2007). Furthermore, the new trend toward the carry-over of green manures as a substitute for farmyard manuring on organic farms with low livestock density (Notaris et al., 2018; Toleikiene et al., 2020) contributes to a renewed interest in the effects of ensiling and other anaerobic digestion processes on weed seed viability.
Ensiling technology is optimized to ensure that the energy content of fresh organic substrates is retained to the greatest extent possible (Müller and Bauer, 2006). This is mainly achieved by lowering the pH value through lactic acid fermentation. However, depending on the ensiled substrate and the ensiling conditions, other fermentation products are produced as well (Pahlow, 2007). It is currently completely unknown what influence the divergent, different fermentation profiles of different silage types have on seed viability. This is also due to the fact that there is no systematic research on seed survival in silages, yet. Many of the studies on ensiling seeds have dealt with only one weed species (Overud, 2002; van Eekeren et al., 2006; James et al., 2011; Trolove and Dowsett, 2015; Weller et al., 2016) or with only one type of silage (Mayer et al., 2000; Koarai et al., 2015; Piltz et al., 2017). In addition, both silage types and methods used to determine seed viability often differed. Therefore, the statement made in several studies that ensiling has the potential to reduce seed viability must always be related to the respective plant species and ensiling environment (e.g., Aper et al., 2014; Simard and Lambert-Beaudet, 2016; Piltz et al., 2017). Similarly, the interaction of species and ensiling environment in relation to seed viability parameters is poorly understood.
In this study, we aimed to determine the effect of varying substrate and ensiling conditions on seed viability. As model species we chose known weeds and species from a wild plant flowering mixture. Wildflower mixtures have gained agronomic importance in the context of enhancing the ecological value of agricultural landscapes through flowering strips (Fritch et al., 2011). In addition, their biomass is intended to be used as a source of renewable bioenergy (Cossel and von Lewandowski, 2016; Lask et al., 2020). Since they have gained importance only recently, information on the survivability of their seeds in ensiling is lacking. “Using this model species, we hypothesized that ensiling had the potential to reduce plant seed viability to an extent relevant to integrated weed management. Furthermore, we tested the hypotheses that differences in seed survival rates after ensiling could be attributed to seed species and/or certain seed characteristics. Finally, we tested the hypothesis that different types of silage affected seed viability in specific ways.”
Materials and Methods
Plant Species
Species Selection
The potential of ensiling to reduce plant seed viability was tested on 10 different dicotyledonous plant species. We did not study monocotyledonous species because other studies have already demonstrated that, with few exceptions, they quickly lose their viability during ensiling (e.g., Blackshaw and Rode, 1991; Lück, 2012; Koarai et al., 2015; Piltz et al., 2017). Our selection should include species that are important weeds, and species that have a high probability of entering silages and represent a wide range of different seed traits. In addition, half of the species should exhibit the so-called hardseededness (HS) in their seeds, i.e., physical dormancy (Baskin et al., 2000), as seeds from such species are considered particularly resistant to degradation in fermentation processes (Westerman and Gerowitt, 2013).
The important weeds examined in this study were Chenopodium album L. (common lambsquarters, Amaranthaceae) and Abutilon theophrasti Medik. (velvetleaf, Malvaceae), with seeds of A. theophrasti exhibiting HS (LaCroix and Staniforth, 1964). Both weeds are problematic in several regions of the world (e.g., Follak et al., 2014; Bajwa et al., 2019) and spread via abundant seeds that are relatively resistant to degradation in soil and fermentation processes (Toole and Brown, 1946; Bassett and Crompton, 1978; Warwick and Black, 1988; Westerman et al., 2012). In addition, C. album has been shown to have the potential to contaminate maize biomass and thus maize silage with large numbers of its seeds (Westerman and Gerowitt, 2012). The other eight species studied are highly likely to enter silages as well, because they are included in a wildflower mixture that was developed for bioenergy use and is also suitable for ensiling (Cossel and von Lewandowski, 2016; Vollrath et al., 2016; Lask et al., 2020; Müller and Hahn, 2020). This means that seeds that survive ensiling can be spread with the silage and potentially become weeds. An additional motivation to study species from this wildflower mixture was that they are already slightly influenced by breeding, which improves their germination ability, which in turn lowered the variance in our experiments. Four HS species were selected from the mixture, namely Malva alcea L. (rose mallow, Malvaceae), Malva sylvestris L. (common mallow, Malvaceae), Melilotus albus Medik. (white sweet clover, Fabaceae), and Melilotus officinalis (L.) Pall. (yellow sweet clover, Fabaceae). Regarding the non-hardseeded species, we ensured that they were from different plant families and represented as much variation in seed morphology as possible. We selected Cichorium intybus L. (blue dandelion, Asteraceae), Daucus carota L. (wild carrot, Apiaceae), Echium vulgare L. (viper‘s bugloss, Boraginaceae), and Verbascum thapsus L. (common mullein, Scrophulariaceae). Of the selected wildflower species, only M. officinalis had previously been studied in ensiling (Woodward, 1940).
Seed Acquisition and Storage
The seeds of most species were obtained from “Appels Wilde Samen” [Darmstadt, Germany (appelswilde.de)], where they were harvested in 2014. The seeds of C. album and M. sylvestris were also collected in 2014, but from plants grown on test areas of the University of Rostock. The seeds of A. theophrasti had already been harvested in 2008 from a sunflower (Helianthus annuus L.) field in Vilanova de Bellpuig, Lleida, Spain. Since then they had been stored in the dark at 7°C. After harvest and between the ensiling treatments, seeds of all species were stored in paper bags at room temperature.
Ensiling Treatment
Silage Preparation
Five types of silage were prepared, which differed in their substrate composition and/or the ensiling conditions. Substrates used were silage maize as a whole crop substrate and biomass from a wildflower mixture designed for use in bioenergy (Vollrath et al., 2016). Substrates were harvested and ensiled in two experimental series, which corresponded to two consecutive growing seasons. The silage maize was grown on the same site (Rostock, Germany, 54°04′04.1′′N 12°04′55.7′′E) in the same variety (“Ronaldinho,” breeder KWS®) in both trial years (2014 and 2015). In both years, maize was harvested at early silage ripening stage, but different weather conditions had led to varying maturation. According to the BBCH scale (Weber and Bleiholder, 1990), the developmental physiology of the maize corresponded to BBCH 82 in the first and BBCH 87 in the second trial year. The dry matter (DM) content was 28.1% in the first and 23.9% in the second year, respectively. The wildflower biomass was used for ensiling only in the second trial year, but there were two variants of it: wildflower biomass from the first year of standing and from the second year of standing, which differed due to changes in species dominance patterns. In the second standing year, the biomass was less fibrous but had a higher content of senescent material. At harvest, DM content of the wildflower biomass varied from 40.1% in the first to 42.8% in the second standing year. Further information on the species composition and the biochemical parameters of the initial substrates before ensiling is available from Müller and Hahn (2020), as the same biomass stock was used in this study.
For ensiling the maize was chopped to a length of 0.5–1.5 cm with a forage harvester, while the wild flower biomass was chopped to 2–4 cm with a commercial garden shredder. Ensiling was conducted immediately after the chopping and, if required, mixing of the substrates. We used 3 l glass jars as lab-scale silos. The jars were washed and sterilized (180°C) before the substrates were filled in layers and compressed by hand. We filled the jars to the brim so that there was no cavity under the lid. Afterwards the filled jars were closed with a rubber-lined lid that was fixed by clips. This simple but standardized method prevented air infiltration but allowed the escape of gases formed during fermentation processes. Four silage types were prepared using this optimized method: “maize 82, ideal” from 100% maize harvested at BBCH 82, “maize 87, ideal” from 100% maize harvested at BBCH 87, as well as mixtures from 67% (fresh weight) maize biomass harvested at BBCH 87 and 33% (fresh weight) wildflower biomass harvested in the first (“wildflower blend 1”) or second standing year (“wildflower blend 2”). In the first trial year, an aliquot of maize harvested at BBCH 82 was deliberately exposed to stressful ensiling conditions in order to provoke suboptimal but possibly more practical fermentation acid patterns. This silage type is hereafter referred to as “maize 82, stress.” To prepare this silage of lower quality, the substrate was stuffed less tightly, and inoculated with a soil-suspension containing Clostridia of a misfermented field silage pile. Additionally, aerobic stress was induced by lifting the lid for 24 h at the beginning, after 28 and after 42 days. This stress treatment and ensiling under ideal, optimized conditions form the two levels of the factor “ensiling condition,” namely “stress” and “ideal.”
All silage types were ensiled in at least three replicates and stored in the dark at 16°C. Substrates should be ensiled for at least 3 months to ensure that all fermentation processes have been completed and the growth of detrimental microbial populations such as yeasts has been limited (Müller and Bauer, 2006; Wyss and Pradervand, 2017).
Silage Characteristics
After ensiling and removal of the ensiled seeds (see section Ensiling of seeds), silages were sealed airtight in plastic bags and stored at −40°C before analyzing their biochemical composition, including fermentation profiles. The silage characteristics were determined as described in Müller and Hahn (2020). The biochemical composition of the silage types was visualized with non-metric Multi-Dimensional Scaling (n-MDS) based on Bray-Curtis distances using R package “vegan” (Oksanen et al., 2002). A PerManova [“adonis” function in R by Anderson (2001)] using the Bray–Curtis dissimilarity index (Bray and Curtis, 1957) and including a permutation test with 1,000 permutations was applied to test to what extent the type of silage explained the variance in the biochemical composition of the silages.
Ensiling of Seeds
The survival of seeds of all 10 species was tested in the silages “maize 82, ideal” and “maize 82, stress.” Seeds of the HS species M. alcea, M. albus, and M. officinalis were additionally tested in the silage types “maize 87, ideal,” “wildflower blend 1,” and “wildflower blend 2”. Both experimental series were accompanied by untreated controls, referred to as “untreated A” and “untreated B.” For ensiling, 100–300 seeds of one species were placed into fine-meshed polyester bags. One bag for each species was stuffed into the middle part of a silage jar together with the substrate. The number of replicates and the total number of seeds examined varied among silage types and plant species. See Supplementary Table 1 for a detailed record.
The specific ensiling duration depended on the analytical capacities and averaged 8 months, ranging from 4 to 9 months. Seeds were retrieved after 239 days from “maize 82, ideal” and after 281 days in “maize 82, stress.” From “maize 87, ideal,” “wildflower blend 1,” and “wildflower blend 2” one replicate was opened after 128 days, and the seeds from the other replicates were retrieved after 237 days.
Seed Viability Tests
After the bags containing the seeds were removed from the silage, they were rinsed with tap water. The seeds were then surface sterilized with 1% NaOCl, rinsed three times with sterilized water and placed on plates with “diaspore agar” (agar agar 13.0 g l−1, KNO3 2.0 g l−1, gibberellic acid 0.5 g l−1, ampicillin 0.1 g l−1, streptomycin 0.1 g l−1, benzimidazol 0.02 g l−1). In the following 21 days, the seeds were incubated at 20/4°C day/night temperatures with a 16 h photoperiod and germination was checked after 3, 7, 11, 16, and 21 days. A seed was considered germinated if the radical protruded at least 2 mm from the seed. The viability of all remaining non-germinated seeds was tested using 2,3,5-triphenyl tetrazolium chloride (TTC). For this purpose, the seed coats were carefully punctured with a needle or scalpel without inflicting injuries to the embryo and the punctured seeds were placed between two filter papers, soaked with 3 ml of 1.0% TTC solution and incubated in the dark at 35°C for 20–22 h.
The same procedure was used to determine the viability of untreated control seeds that had not been exposed to ensiling (minimum of 3 replicates of 300 seeds per species, Supplementary Table 1). However, the previously dry-stored control seeds were exposed to a water-saturated atmosphere for 2 days in the dark before the viability test.
Based on their response in the viability test, the seeds were classified as either dead or potentially viable. All seeds whose embryo was unstained (white) or rotten were considered “dead.” Seeds that were completely decomposed during ensiling were assigned to the “dead” category as well. All seeds that showed metabolic activity in the form of germination or staining in the tetrazolium test were considered potentially viable.
Statistical Analysis of Viability Data
Statistical analysis was performed only for species that had not completely lost their viability due to ensiling. Various generalized linear mixed effect binomial models with a logit link function were used to estimate the proportion of dead seeds among all seeds. Ensiling (yes or no), different ensiling treatments, plant species, and duration of ensiling were considered fixed effects. The glass jar was used as random effect in each model. Additional random variables were introduced to reflect the variance structure. In all models, the respective number of seeds under study was used as a weight in the fitting process. Table 1 provides an overview of the research questions and the specified models.
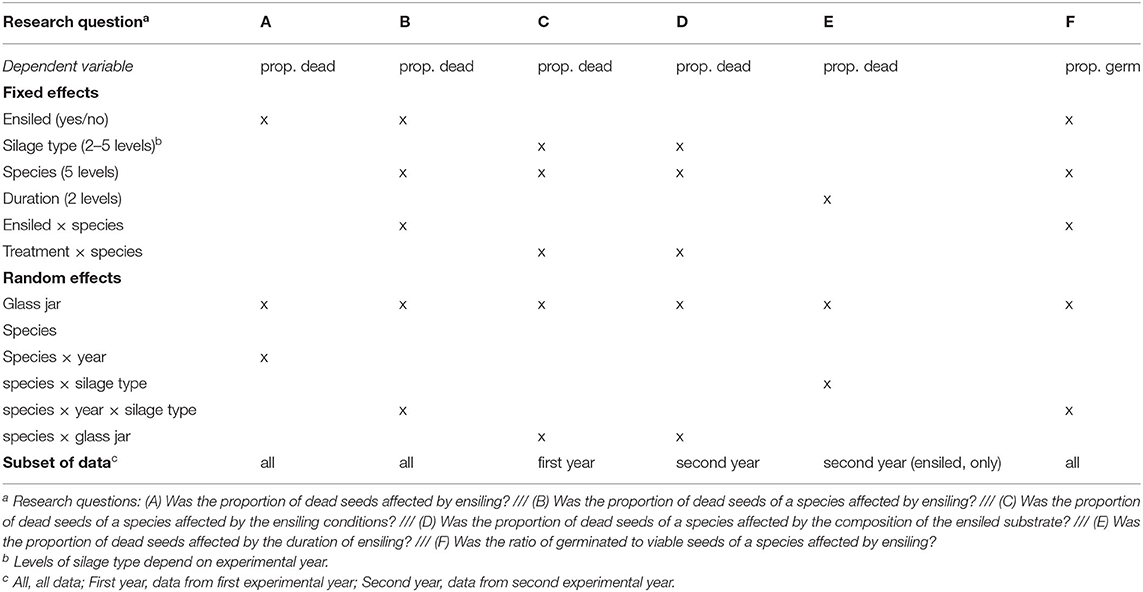
Table 1. Overview of research questions on seed persistence under different ensiling conditions and the related structure of generalized linear mixed models. The dependent variable was either the proportion of dead seeds to examined seeds (prop. dead) or the proportion of germinated seeds to viable seeds (prop. germ).
Starting from the respective null model, the deviance of the fixed effects was analyzed by sequentially adding main and interaction effects. Likelihood-ratio tests were used for pairwise comparisons of the increasingly complex models. Pairwise comparisons of the fixed effect levels were performed based on log odds ratios using Tukey's method for P-value adjustment.
If ensiling was used as a component of integrated weed management, it would affect the seed stage in the cycle of population dynamics. Against this background, the seed-killing efficacy of ensiling was calculated as follows:
All analyses were carried out using R software (R Core Team, 2020). The models were fitted using the R package “lme4” (Bates et al., 2015). The R package “emmeans” provided functions to estimate marginal means and odd ratios, and to test for significance (Lenth, 2021). All models were checked for the appropriateness of the chosen binomial distribution, for over- and under-dispersion, and for outliers with the respective test routines of the R package “DHARMa” (Hartig, 2020).
Results
Ensiling generally increased the proportion of non-viable, i.e., dead, seeds (Figure 1). However, a substantial difference existed between hardseeded (HS) and non-hardseeded (NHS) plant species. Regarding the NHS species, ensiling was 100% effective. That means that all seeds of the NHS species were dead after ensiling, while on average 75% of them were viable without ensiling. Of the HS species, an average of 36% was dead after ensiling. Since 11% had already been dead before the treatment, ensiling resulted in a highly significant seed-killing efficacy of 28% across all five HS species tested [P(Chi2) < 0.0001, Supplementary Table 2]. Among the individual HS species, the seed-killing efficacy ranged from 5 to 60%. In M. sylvestris, M. albus, and M. officinalis ensiling significantly increased the proportion of non-viable seeds compared to untreated controls (Table 2; Figure 1).
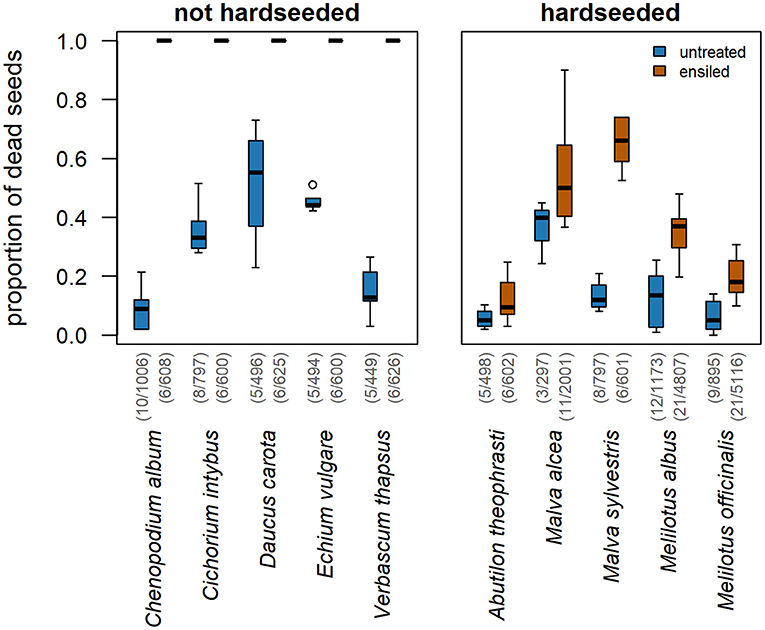
Figure 1. Ensiling increased the proportion of non-viable weed seeds from both NHS and HS species compared to untreated controls. Numbers in parentheses indicate replicates/total numbers of seeds studied.

Table 2. Effect of ensiling on the proportion of dead seeds and seed killing efficacy of ensiling in five HS species.
Across all HS species, all silage types had a highly significant seed-killing effect compared to the un-ensiled control (Figure 2, bottom). Also referring all HS species, ensiling “maize 82” under ideal conditions multiplied the proportion of dead seeds by a factor of 4.3 on average compared to un-ensiled controls, while suboptimal (stress) ensiling conditions increased the proportion of dead seeds by a factor of 4.1 (Table 3; Figure 2, left bottom). This corresponded to seed-killing efficacies of 29 and 27%, respectively. Ensiling seeds of HS species in three different substrates (“maize 87,” “wildflower blend 1,” “wildflower blend 2”) under identical, ideal conditions caused a 4.9–5.6-fold increase of the proportion of dead seeds compared to un-ensiled controls, corresponding to a seed-killing efficacy of 23–26% (Figure 2, right). The seed-killing efficacy did not differ significantly between silages with different substrate compositions (Figure 2, right bottom; Tables 3, 5). However, it should be mentioned here that across all HS species “maize 82, ideal” killed 1.5-times as many seeds as “maize 87, ideal” (Table 3). In addition, the response of the individual HS species did differ between the silage types (Figure 2, top) and even significantly with respect to the ensiling conditions (Table 4). For A. theophrasti and M. alcea the seed-killing efficacy of “maize 82, ideal” was significantly higher than that of “maize 82, stress,” while it was the opposite for M. sylvestris and M. albus. In M. officinalis, the percentage of dead seeds was the same for both ensiling conditions (Figure 2, left).
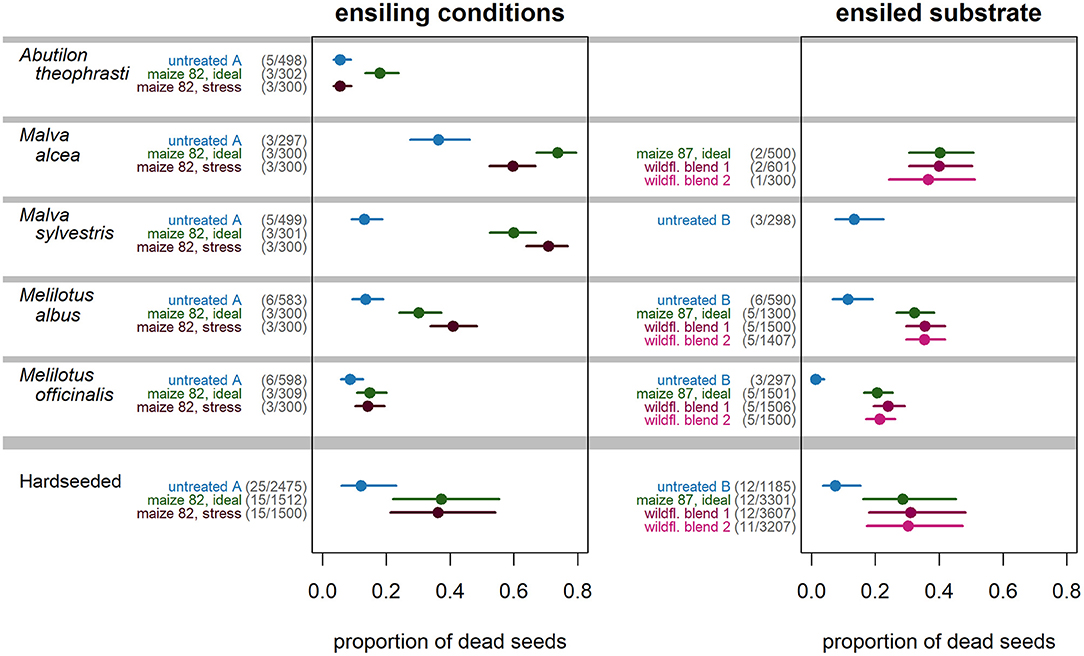
Figure 2. Effect of ensiling conditions and ensiled substrate on the proportion of non-viable seeds of HS weed species. Numbers in parentheses indicate replicates/total numbers of seeds studied.
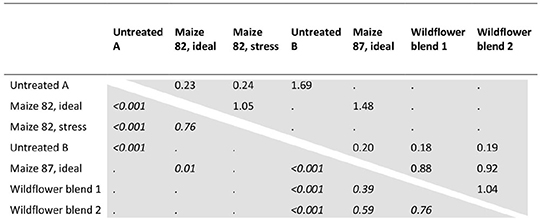
Table 3. Pairwise comparisons of the effect of different silage types and untreated controls on the proportion of dead seeds. Upper right triangle: odds ratios (column to row); lower left triangle: corresponding P (z-ratio).

Table 4. Analysis of deviance of the generalized linear mixed effects model for the proportion of dead seeds of HS species as influenced by ensiling conditions (“ideal” and “stress” in maize 82), plant species (A. theophrasti, M. alcea, M. sylvestris, M. albus, M. officinalis), and their interaction.

Table 5. Analysis of deviance of the generalized linear mixed effects model for the proportion of dead seeds of HS species as influenced by composition of ensiled substrate (“maize 87, ideal,” wildflower blends 1 and 2), plant species (M. alcea, M. albus, M. officinalis), and their interaction.
Ensiling durations differed among silage samples due to the capacities available for seed viability analyses. According to the statistical evaluation, the duration of ensiling had a significant effect on successfully killing the seeds. If the duration of ensiling was shortened from almost 8 to 4 months, the proportion of dead seeds decreased by a factor of 0.8 [P(z-ratio) = 0.0478, Supplementary Table 3]. Furthermore, significant year-to-year differences were observed in the proportion of dead seeds in the untreated control samples, being 1.7 higher in the first experimental year (Table 3).
The germination experiments revealed that the proportion of germinated to viable seeds was lower after ensiling, by a factor of 3 to a factor of 26 [P(z-ratio) < 0.002, Figure 3; Supplementary Table 4]. Only A. theophrasti was an exception to this, with the same proportion of germinating seeds regardless of ensiling. It is worth mentioning that in two of the three samples of “maize 82, ideal,” most of the germinable seeds of M. albus (about 70%) had already started to germinate in the silage itself. This means that they were already recognizable as seedlings when the silage jars were opened.
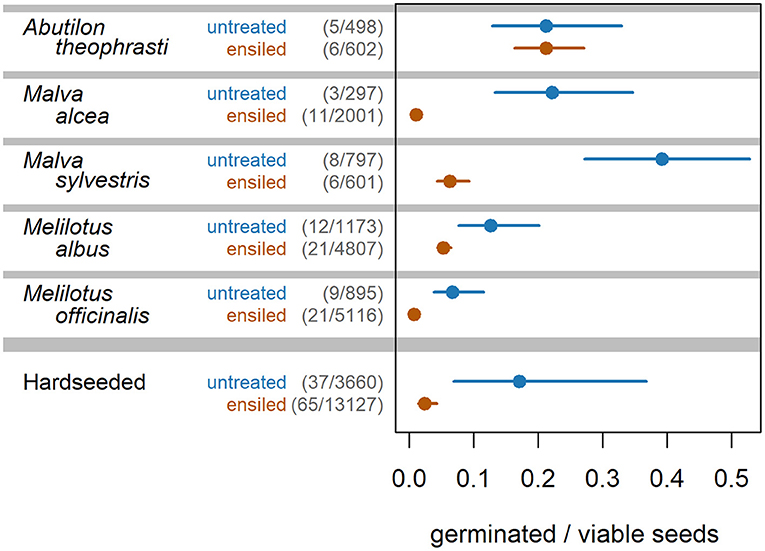
Figure 3. Proportion of germinated to viable seeds of HS species before (untreated) and after ensiling.
In terms of their biochemical characteristics, the five silage types produced differed significantly [P(F-value) = 0.0010, Table 6; Figure 4; Supplementary Table 5]. The factor “silage type” explained 95.6% of the variance in the biochemical composition of the silages (Table 6).
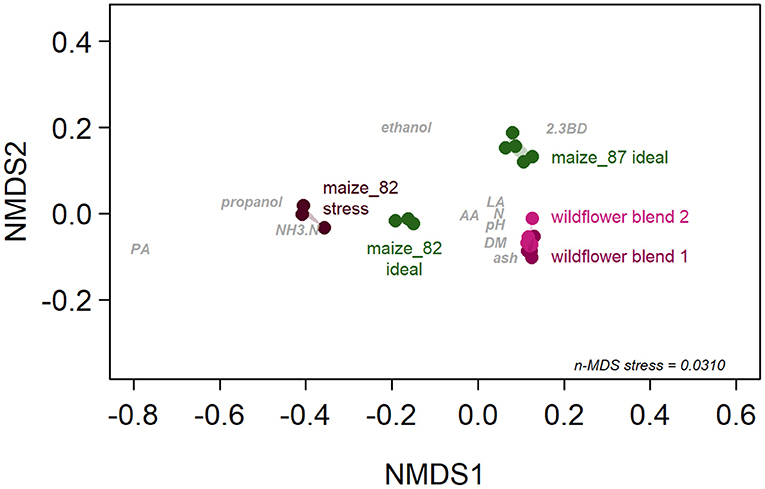
Figure 4. Differences in the biochemical composition of the five silage types displayed in non-metric Multi-Dimensional Scaling (n-MDS). Points indicate replicates and polygons mark replicates belonging to the same silage type. Nomenclature of the biochemical characteristics (gray): AA, acetic acid; DM, dry matter content; LA, lactic acid; N, total nitrogen; NH3.N, ammonium bound nitrogen; PA, propionic acid; 2.3BD, 2,3-butanediol.
Discussion
Seed-Killing Efficacy of Ensiling
Lab-scale ensiling for 8 months decreased the proportion of viable seeds of the hardseeded (HS) species studied and completely inactivated the non-hardseeded (NHS) ones. These results are consistent with the findings of other studies ensiling seeds on a long-term basis, i.e., for more than 3 months (Shevkenek, 1934; Woodward, 1940; Mayer et al., 2000; Overud, 2002; Lück, 2012; Westerman et al., 2012; Aper et al., 2014; Koarai et al., 2015; Simard and Lambert-Beaudet, 2016). However, regarding the HS species the seed-killing efficacies of our silages were low compared to other long-term studies (Mayer et al., 2000; Westerman et al., 2012; Koarai et al., 2015; Simard and Lambert-Beaudet, 2016). Instead, the killing efficacy in our experiments was more in the range of studies that ensiled HS seeds for a maximum of 3 months reducing the viability of HS species by about half on average (Woodward, 1940; Mayer et al., 2000; Stanton et al., 2012; Simard and Lambert-Beaudet, 2016; Piltz et al., 2017).
The seed-killing efficacies found here are nonetheless within the range of previous studies (highlighting the immense range of responses of HS species in ensiling). In fact, the viability losses of the HS species studied so far varied between 0 and 100%. In individual samples of A. pelecinus (L.) Barneby (biserrula, Fabaceae) the mean viability was even higher than in untreated controls (Stanton et al., 2012). Viability loss also varied, sometimes greatly, within HS species of which several batches were examined, e.g., A. theophrasti (Westerman et al., 2012; Simard and Lambert-Beaudet, 2016; this study), and M. officinalis (Woodward, 1940; this study). For example, our one-year-old seed batch of M. officinalis originating from Germany lost about 15% of its initial viability in our lab-scale, maize-based silages, while a batch of unknown age from the U.S. was almost completely inactivated in various silages of alfalfa (Woodward, 1940). The differences between studies could be due to possible effects of the surrounding silage substrate as well as to different initial seed qualities. Seed lot quality can vary strongly depending on factors such as seed age, plant population, and storage conditions (e.g., Hay and Probert, 2013). For example, the viability of our untreated seeds differed significantly between the two experimental years.
Regarding the differing survival rates of HS species in ensiling it might be relevant that the degree and the depth of HS differ between species and seed lots (Baskin and Baskin, 2004). In general, the coat of young seeds of HS species in the early stages of ripening is still permeable to water (Jaganathan et al., 2016 and references therein), and thus possibly also to harmful fermentation products from ensiling. Fully mature seeds of HS species, on the other hand, are likely to be protected by their hard, water-impermeable seed or fruit coat. The different killing efficacies for different aged batches of A. theophrasti from three different studies fit this theory well: After more than 3 months of ensiling, batches of freshly harvested A. theophrasti seeds were completely inactivated (Simard and Lambert-Beaudet, 2016), while a 3-year-old batch had lost only one-fifth (Westerman et al., 2012) and our 6-year-old batch had lost as few 5% of its initial viability. Whether seeds of HS species actually become more resistant to ensiling due to increasing maturity and depth of HS could be demonstrated by ensiling different batches of a HS species with known characteristics under identical conditions. Transferred into farming practice, increasing insensitivity of HS species to ensiling over time would mean that the seed-killing efficacy of ensiling would tend to be higher than that determined in our study. This is because in practice, only (young) weed seeds still on the harvested plants would enter the silo.
However, HS is not the only mechanism that can make seeds resistant to ensiling. This is evident, for example, from the fact that not all NHS species studied so far were completely inactivated by ensiling, although none survived in our study. For instance, Rumex crispus L. (curled dock, Polygonaceae) and Spergula arvensis L. (stickwort, Caryophyllaceae) had lost only a maximum of 36 and 47% of their initial viability after 3 and 8 months in ensiling, respectively (Mayer et al., 2000; Overud, 2002). The specific mechanisms by which species without HS survived ensiling are not yet clear. But seed coat thickness (Simard and Lambert-Beaudet, 2016), seed maturity (Piltz et al., 2017), and induction of dormancy (Overud, 2002) have been suggested as factors that might increase their resistance toward ensiling.
In addition to killing seeds, ensiling appeared to have weakened seed vigor because the proportion of germinated to viable seeds was lower after our ensiling. This makes it unlikely that these viable seeds will develop into seedlings. We assume the same for the seeds of M. albus that have already started to germinate in the silage, as seedlings are too unstable to survive the mechanical stresses associated with application or further processing. Other studies did not report germination in silage, but morphological changes in seeds indicating damage and, thus, limited survivability (Simard and Lambert-Beaudet, 2016; Weller et al., 2016).
Although the properties of the plant seeds themselves are important, their survival in silages is mainly influenced by the conditions of the particular ensiling process. In this regard, the influence of ensiling duration on seeds was studied and—as in our study—it was found that longer ensiling was equivalent to a higher seed-killing efficacy (Mayer et al., 2000; van Eekeren et al., 2006; Trolove and Dowsett, 2015; Simard and Lambert-Beaudet, 2016). In addition to the longer time the ensiled biomass can act on the seeds, the different biochemical phases of the ensiling process may play a role here. During these phases, various fermentation products are formed due to microbiological activity, which depends on properties of the ensiled biomass and the respective ensiling conditions until finally a relatively stable (storage) state is reached (Müller and Bauer, 2006). The biochemical characteristics of a silage thus depend on factors in which all studies on seed survival in ensiling differed to a greater or lesser extent.
Effect of Silage Type on Seed-Killing Efficacy
In agreement with other studies that have tested different silage types on more than one species (Woodward, 1940; Anonymus, 1959–1960; Simard and Lambert-Beaudet, 2016) we did not find a consistent effect on the seeds of all weed species studied. When the silage types were distinguished by substrate composition, the seed-killing effect of the types did not differ in our study or that of Woodward (1940), but it did species-specifically in Simard and Lambert-Beaudet (2016) and Anonymus (1959–1960). However, when distinguished according to ensiling conditions (ideal and stressed), the effect of our silage types differed, but again depending on the weed species. A comparison of seed-killing efficacy between silage types that differ in ensiling conditions, i.e., factors that are important to prepare a high-quality forage silage (Müller and Bauer, 2006) (high/low compaction, extent of air inflow, contamination with soil bacteria), has not been done in any other study that we are aware of. Rather, differences between the silage types were based on the variation in individual factors such as substrate composition (Anonymus, 1959–1960; Simard and Lambert-Beaudet, 2016), manipulation of DM (Overud, 2002; van Eekeren et al., 2006), addition of inoculants (Weller et al., 2016), and varying depths of incubation in the silo (Shevkenek, 1934; Tildesley, 1937; James et al., 2011; Trolove and Dowsett, 2015). Only Woodward (1940) varied three factors, namely substrate, moisture content, and addition of molasses, for his silage types.
Even though the silages studied so far have been classified into types based on certain properties, they remain largely “black boxes” and thus hardly comparable between studies because little is reported on their biochemical characteristics. Data on selected biochemical factors collected in some studies led to different assumptions about the mechanisms of action behind the seed-killing effect of ensiling. So far, moisture or DM content, changes in temperature, pressure, and pH value, CO2 content, lactic acid content and the activity of proteolytic enzymes have been discussed as possible inactivation mechanisms (Woodward, 1940; Blackshaw and Rode, 1991; van Eekeren et al., 2006; Simard and Lambert-Beaudet, 2016; Weller et al., 2016). In terms of moisture or DM, there is some evidence that silages with higher moisture and consequently lower DM content are more efficient in killing seeds (Woodward, 1940; Overud, 2002; van Eekeren et al., 2006). However, this trend is contradicted by our data: Our silage with the lowest DM content had a significantly lower seed-killing efficacy than that with the highest DM content (“maize 87, ideal”: 26.2 ± 0.31% fresh weight vs. “maize 82, ideal”: 34.4 ± 0.49% fresh weight, Supplementary Table 5). Furthermore, we can add to the discussion by noting that a significant difference in silage biochemistry does not necessarily translate into a significantly different seed-killing efficacy because, unlike other studies, we have determined the biochemical characteristics including fermentation acid patterns of our silages. The variance of the fermentation products in our silages reflects their range quite well in practice silages produced according to the current state of knowledge; although there would be a considerable amount of butyric acid in misfermented practice silages. From the fact that we found no consistent differences in seed-killing efficacy within the biochemical spectrum we studied, we conclude that neither substrate choice nor manipulation of ensiling conditions (within good practice) are effective measures to increase weed seed killing during ensiling.
Comparison With Other Pathways Reducing Seed Viability
To assess the potential of ensiling as a measure of integrated weed management, it seems useful to compare it with the better-known pathways of seed reduction in organic farming. In the field itself, seed predation in particular (e.g., Westerman et al., 2003) is similar to weed seed removal by harvesting and ensiling biomass, although animal predators additionally reduce seed depots on the soil surface. Furthermore, diverse tillage operations directly affect the pool of weed seeds by burying them (Grundy et al., 1999). The extent to which the weed seed load can be reduced by seed predation and tillage depends on management practices and environmental conditions, but most importantly on the weed species and their characteristics [e.g., Nichols et al. (2015) and references therein]. For example, Galium aparine L. (catchweed bedstraw, Rubiaceae) and Lolium rigidum Gaudin (rigid ryegrass, Poaceae) lost 49 and 62% of their seeds, respectively, to predation and 33 and 54% to 2-month burial (Baraibar et al., 2017). Comparatively low were the mean seed predation rates reported by Navntoft et al. (2009) of 17 and 10% for organically and conventionally managed fields in New Zealand, respectively. Despite this wide range of seed reduction by on-field processes, the average seed-killing potential of our silages was higher, at least for NHS species. For the HS species A. theophrasti, however, the annual predation rate of 20–80% determined by Westerman et al. (2005) was higher than the seed-killing efficacy of our silages.
Another important aspect when evaluating ensiling as a weed control measure is the further use of the silages produced, which, after all, still contain viable weed seeds in some cases. Usually the silage is fed, i.e., subjected to animal digestion, and the resulting manure is stored until it is used as fertilizer. All of these fermentation processes have the potential to inactivate seeds (e.g., Aper et al. 2014). However, seed inactivation does not necessarily increase when seeds are exposed to them sequentially (Blackshaw and Rode, 1991; Stanton et al., 2012; Piltz et al., 2017). Rather, the process step which weed seeds are exposed to first is crucial for weed control because (HS) seeds that survive the initial fermentation process often survive subsequent processes (Edwards and Younger, 2006). In terms of weed control, ensiling is likely to be more suited as a first process step than feeding, since many seed-producing weed species have evolved adaptations to survive herbivory (Blackshaw and Rode, 1991).
Ensiling and the Use of Flowering Plant Mixtures
When the biomass of wild plant flowering mixtures is used (Cossel and von Lewandowski, 2016) their seeds can be unintentionally spread and possibly become weeds. According to our study, ensiling can help reduce this risk of weed spread by killing the seeds of NHS and reducing the viability of HS wildflower species. Especially compared to direct use of biomass as in the newly emerging practice of “cut and carry” (Benke et al., 2017), in which mulched biomass is transferred from field to field as an organic nutrient source, ensiling would contribute greatly to integrated weed management. Of course, the HS species that have survived ensiling still pose a risk as potential weeds. However, this risk would presumably be lower than after direct use in a biogas plant. Westerman et al. (2012) reported that weed seed viability was generally more affected by ensiling than by anaerobic digestion. Therefore, upstream processing of wild plant biomass via ensiling would improve weed management compared to immediate biomethanization.
Conclusions
Ecological weed management aims to subject weeds to multiple, temporally variable stresses, for which Liebman and Gallandt (1997) coined the term “many little hammers.” Based on the results of this study, we conclude that ensiling is an often overlooked yet effective way to reduce weed seed loads in a sustainable manner. By reducing number and vigor of weed seeds, ensiling helps exclude weeds from fields, reducing their density and delaying their emergence before they can interfere with crop growth or reproduction. Thus, ensiling can be one of the “little hammers” in ecological weed management.
Ensiling unfolds its full seed reduction potential primarily with NHS weeds. However, it has a gap in effectiveness when it comes to reducing the viability of HS species, especially if they have reached full maturity and/or have additional dormancy mechanisms. It remains to be investigated whether this effectiveness gap can be closed by specifically varying silage properties or by extending the duration of ensiling beyond the usual storage times of forage or energy substrates.
As an on-farm integrated weed management tool ensiling targets only seeds that are part of the biomass stream intended for whole-crop storage. Also in terms of biomass streams a tendency toward specialization in organic farming can currently be observed (“conventionalization debate”). This may include a marginalization of animal husbandry in favor of well-marketable cash crops. In this context, our results suggest that the traditional and proven integration of cropping and livestock with their characteristic material flows and biomass processing steps (ensiling, manure storage) is not only beneficial for soil nutrient supply, but is also an essential element of integrated weed management in organic agriculture.
Data Availability Statement
The original contributions presented in the study are included in the article/Supplementary Material, further inquiries can be directed to the corresponding author/s.
Author Contributions
All authors contributed to the conception and the design of the study, the preparation of the silages, and writing and revision of the manuscript. JH edited the manuscript, organized the project, analyzed the viability of the seeds, and performed the multivariate statistical analyses. FdM performed the statistical analysis in coordination with JH and JM.
Funding
This study was funded by the German Federal Ministry of Food and Agriculture through the Fachagentur für Nachwachsende Rohstoffe e.V. (FNR) under grant number 22401114.
Conflict of Interest
The authors declare that the research was conducted in the absence of any commercial or financial relationships that could be construed as a potential conflict of interest.
Publisher's Note
All claims expressed in this article are solely those of the authors and do not necessarily represent those of their affiliated organizations, or those of the publisher, the editors and the reviewers. Any product that may be evaluated in this article, or claim that may be made by its manufacturer, is not guaranteed or endorsed by the publisher.
Acknowledgments
We thank Rotraut Degner, Ingolf Gliege, Diana Sicard, Markus Weinreich, Merel Hofmeijer, Thomas Kunze, Christoph Flucke, and Becke Strehlow for their assistance in preparing the silages. Likewise, we thank Maren Knipping, Rosa Minderlen, Julia Schulz, Paula R. Westerman, and Ophélie Rollin for their help in preparing and opening the silages and the subsequent analysis of the viability of the seeds. Finally, we thank Paula R. Westerman for collecting the seeds of A. theophrasti.
Supplementary Material
The Supplementary Material for this article can be found online at: https://www.frontiersin.org/articles/10.3389/fagro.2021.708851/full#supplementary-material
References
Anderson, M. J. (2001). A new method for non-parametric multivariate analysis of variance. Aust. Ecol. 26, 32–46. doi: 10.1111/j.1442-9993.2001.01070.pp.x
Anonymus (1959–1960). “Weed-free germination after storage in silage (Ukrudtsfrøs spireevne efter opbevaring i ensilage),” in Meddelelse 628-639 fra Statens Forsøgsvirksomhed i Plantekultur, ed. Statens Forsøgsvirksomhed i Plantekultur, Denmark, 715–716.
Aper, J., Cauwer, B., de, Roo, S., de, Lourenço, M., Fievez, V., Bulcke, R., et al. (2014). Seed germination and viability of herbicide resistant and susceptible Chenopodium album populations after ensiling, digestion by cattle and manure storage. Weed Res. 54, 169–177. doi: 10.1111/wre.12063
Atkeson, F. W., Hulbert, H. W., and Warren, T. R. (1934). Effect of bovine digestion and of manure storage on the viability of weed seeds. Agron. J. 26, 390–397. doi: 10.2134/agronj1934.00021962002600050006x
Bajwa, A. A., Zulfiqar, U., Sadia, S., Bhowmik, P., and Chauhan, B. S. (2019). A global perspective on the biology, impact and management of Chenopodium album and Chenopodium murale: two troublesome agricultural and environmental weeds. Environ. Sci. Pollut. Res. Int. 26, 5357–5371. doi: 10.1007/s11356-018-04104-y
Baraibar, B., Canadell, C., Torra, J., Royo-Esnal, A., and Recasens, J. (2017). Weed seed fate during summer fallow: the importance of seed predation and seed burial. Weed Sci. 65, 515–524. doi: 10.1614/WS-D-16-00031.1
Barberi, P. (2002). Weed management in organic agriculture: are we addressing the right issues? Weed Res. 42, 177–193. doi: 10.1046/j.1365-3180.2002.00277.x
Baskin, J. M., and Baskin, C. C. (2004). A classification system for seed dormancy. Seed Sci. Res. 14, 1–16. doi: 10.1079/SSR2003150
Baskin, J. M., Baskin, C. C., and Li, X. (2000). Taxonomy, anatomy and evolution of physical dormancy in seeds. Plant Spec. Biol. 15, 139–152. doi: 10.1046/j.1442-1984.2000.00034.x
Bassett, I. J., and Crompton, C. W. (1978). The Biology of Canadian Weeds. 32: Chenopodium album L. Can. J. Plant Sci. 58, 1061–1072.
Bates, D., Mächler, M., Bolker, B., and Walker, S. (2015). Fitting linear mixed-effects models using lme4. J. Stat. Soft. 67, 1–48. doi: 10.18637/jss.v067.i01
Benke, A. P., Rieps, A.-M., Wollmann, I., Petrova, I., Zikeli, S., and Möller, K. (2017). Fertilizer value and nitrogen transfer efficiencies with clover-grass ley biomass based fertilizers. Nutr. Cycl. Agroecosyst. 107, 395–411. doi: 10.1007/s10705-017-9844-z
Blackshaw, R. E., and Rode, L. M. (1991). Effect of ensiling and rumen digestion by cattle on weed seed viability. Weed Sci. 39, 104–108.
Brainard, D. C., Bellinder, R. R., and Kumar, V. (2011). Grass–legume mixtures and soil fertility affect cover crop performance and weed seed production. Weed Technol. 25, 473–479. doi: 10.1614/WT-D-10-00134.1
Bray, J. R., and Curtis, J. T. (1957). An ordination of the upland forest communities of Southern Wisconsin. Ecol. Monogr. 27, 325–349. doi: 10.2307/1942268
Cossel, M., and von Lewandowski, I. (2016). Perennial wild plant mixtures for biomass production: impact of species composition dynamics on yield performance over a five-year cultivation period in southwest Germany. Eur. J. Agron. 79, 74–89. doi: 10.1016/j.eja.2016.05.006
Döring, T. F., Storkey, J., Baddeley, J. A., Collins, R. P., Crowley, O., Howlett, S. A., et al. (2017). Weeds in organic fertility-building leys: aspects of species richness and weed management. Org. Farming 3, 51–65. doi: 10.12924/of2017.03010051
Edwards, A. R., and Younger, A. (2006). The dispersal of traditionally managed hay meadow plants via farmyard manure application. Seed Sci. Res. 16, 137–147. doi: 10.1079/SSR2006244
El Bilali, H., Callenius, C., Strassner, C., and Probst, L. (2019). Food and nutrition security and sustainability transitions in food systems. Food Energy Secur. 8:e00154. doi: 10.1002/fes3.154
Follak, S., Aldrian, U., and Schwarz, M. (2014). Spread dynamics of Abutilon theophrasti in Central Europe. Plant Prot. Sci. 50, 157–163. doi: 10.17221/55/2013-PPS
Fritch, R. A., Sheridan, H., Finn, J. A., Kirwan, L., and hUallacháin, D. Ó. (2011). Methods of enhancing botanical diversity within field margins of intensively managed grassland: a 7-year field experiment. J. Appl. Ecol. 48, 551–560. doi: 10.1111/j.1365-2664.2010.01951.x
Grundy, A. C., Mead, A., and Burston, S. (1999). Modelling the effect of cultivation on seed movement with application to the prediction of weed seedling emergence. J. Appl. Ecol. 36, 663–678. doi: 10.1046/j.1365-2664.1999.00438.x
Hald, A. B., and Nielsen, A. L. (2007). Field margin management for nature within rotational grass fields at organic dairy farms. Aspects Appl. Biol. 81, 267–270.
Hartig, F. (2020). DHARMa: Residual Diagnostics for Hierarchical (Multi-Level/Mixed) Regression Models: R Package Version 0.3.3.0.
Hatcher, P., and Froud-Williams, R. J. (eds.) (2017). Weed Research: Expanding Horizons. Hoboken, NJ: John Wiley and Sons Ltd.
Hay, F. R., and Probert, R. J. (2013). Advances in seed conservation of wild plant species: a review of recent research. Conserv. Physiol. 1:cot030. doi: 10.1093/conphys/cot030
Jaganathan, G. K., Yule, K., and Liu, B. (2016). On the evolutionary and ecological value of breaking physical dormancy by endozoochory. Perspect. Plant Ecol. Evol. System. 22, 11–22. doi: 10.1016/j.ppees.2016.07.001
James, T. K., Rahman, A., McGill, C. R., and Trivedi, P. D. (2011). Biology and survival of broom corn millet (Panicum miliaceum) seed. NZPP 64, 142–148. doi: 10.30843/nzpp.2011.64.6013
Koarai, A., Hattori, I., Suzuki, T., Sumiyoshi, T., Ohdan, H., Sato, K., et al. (2015). Seed viability of paddy weeds ensiled by forage rice. J. Weed Sci. Technol. 60, 93–100. doi: 10.3719/weed.60.93
LaCroix, L. J., and Staniforth, D. W. (1964). Seed dormancy in velvetleaf. Weeds 12, 171–174. doi: 10.2307/4040721
Lask, J., Martínez Guajardo, A., Weik, J., Cossel, M., von, Lewandowski, I., and Wagner, M. (2020). Comparative environmental and economic life cycle assessment of biogas production from perennial wild plant mixtures and maize (Zea mays L.) in southwest Germany. GCB Bioenergy 12, 571–585. doi: 10.1111/gcbb.12715
Lenth, R. V. (2021). emmeans: Estimated Marginal Means, aka Least-Squares Means: R Package Version 1.5.5–1.
Liebman, M., and Gallandt, E. R. (1997). “Many little hammers: ecological management of crop-weed interactions,” in Ecology in Agriculture, ed L. E. Jackson (San Diego, CA: Academic Press) 291–343.
Lück, C. (2012). Überlebensfähigkeit von Gräsersamen in der Biogasprozesskette. Master Thesis, Universität Rostock, Phytomedizin, Rostock.
MacLaren, C., Storkey, J., Menegat, A., Metcalfe, H., and Dehnen-Schmutz, K. (2020). An ecological future for weed science to sustain crop production and the environment. A review. Agron. Sustain. Dev. 40:24. doi: 10.1007/s13593-020-00631-6
Marshall, E. J. (2005). Field Margins in Northern Europe: Integrating Agricultural, Environmental and Biodiversity Functions. Lacombe, AB: Canadian Weed Science Society.
Mayer, F., Albrecht, H., and Pfadenhauer, J. (2000). The influence of digestion and storage in silage and organic manure on the germinative ability of six weed species (Papaver argemone, P. dubium, Legousia speculum-veneris, Centaurea cyanus, Spergula arvensis, Trifolium arvense). Z. Pflanzenkr. Pflanzens. 47–54.
Merfield, C. N. (2019). “Integrated weed management in organic farming,” in Organic farming: Global perspectives and methods, eds S. Chandran, M. R. Unni, and S. Thomas (Duxford; Cambridge, MA; Kidlington: WP Whead Publishing an Imprint of Elsevier) 117–180.
Michael, P. J., Steadman, K. J., Plummer, J. A., and Vercoe, P. (2006). Sheep rumen digestion and transmission of weedy Malva parviflora seeds. Aust. J. Exp. Agric. 46, 1251–1256. doi: 10.1071/EA05285
Müller, J., and Bauer, R. (2006). “Futterkonservierung,” in Pflanzliche Erzeugung: Grundlagen des Acker- und Pflanzenbaus und der guten fachlichen Praxis, eds M. Munzert and J. Frahm (München: BLV Buchverlag) 865–933.
Müller, J., and Hahn, J. (2020). Ensilability of biomass from effloresced flower strips as co-substrate in bioenergy production. Front. Bioeng. Biotechnol. 8:14. doi: 10.3389/fbioe.2020.00014
Navntoft, S., Wratten, S. D., Kristensen, K., and Esbjerg, P. (2009). Weed seed predation in organic and conventional fields. Biol. Control 49, 11–16. doi: 10.1016/j.biocontrol.2008.12.003
Nichols, V., Verhulst, N., Cox, R., and Govaerts, B. (2015). Weed dynamics and conservation agriculture principles: a review. Field Crop. Res. 183, 56–68. doi: 10.1016/j.fcr.2015.07.012
Notaris, C., de, Sørensen, P., Møller, H. B., Wahid, R., and Eriksen, J. (2018). Nitrogen fertilizer replacement value of digestates from three green manures. Nutr. Cycl. Agroecosyst. 112, 355–368. doi: 10.1007/s10705-018-9951-5
Oksanen, J., F. G., Blanchet, M., Friendly, R., Kindt, P., Legendre, D., et al. (2002). Effects of Ensiling on Seed Germinability and Viability in Rumex crispus L. Master Thesis, Swedish University of Agricultural Sciences, Department of Ecology and Crop Production Science, Uppsala.
Overud, S. (2002). Effects of ensiling on seed germinability and viability in Rumex crispus L. Master thesis. Uppsala: Swedish University of Agricultural Sciences, Department of Ecology and Crop Production Science.
Piltz, J. W., Stanton, R. A., and Wu, H. (2017). Effect of ensiling and in sacco digestion on the viability of seeds of selected weed species. Weed Res. 57, 382–389. doi: 10.1111/wre.12269
Pleasant, J. M., and Schlather, K. J. (1994). Incidence of weed seed in cow (Bos sp.) manure and its importance as a weed source for cropland. Weed Technol. 8, 304–310.
Reganold, J. P., and Wachter, J. M. (2016). Organic agriculture in the twenty-first century. Nat. Plants 2:15221. doi: 10.1038/nplants.2015.221
Shevkenek, W. (1934). Viability of Weed Seeds in Manure and Silage. Master thesis, University of Saskatchewan, Saskatchewan, Canada.
Simard, M.-J., and Lambert-Beaudet, C. (2016). Weed seed survival in experimental mini-silos of corn and alfalfa. Can. J. Plant Sci. 96, 448–454. doi: 10.1139/cjps-2015-0261
Stanton, R. A., Piltz, J. W., Rodham, C., and Wu, H. (2012). “Silage for managing weed seeds,” in Proceedings of the 18th Australasian Weeds Conference 2012: Developing Solutions to Evolving Weed Problems, ed. V. Eldershaw (Melbourne, VIC), 219–221.
Stonehouse, D. P., Weise, S. F., Sheardown, T., Gill, R. S., and Swanton, C. J. (1996). A case study approach to comparing weed management strategies under alternative farming systems in Ontario. Can J Agric Econ 44, 81–99. doi: 10.1111/j.1744-7976.1996.tb00144.x
Takabayashi, M., Abe, H., and Kubota, T. (1975). Studies on the dissemination of weed seeds by livestocks. J. Weed Sci. Technol. 1975, 36–41. doi: 10.3719/weed.1975.36
Tildesley, W. T. (1937). A study of some ingredients found in ensilage juice and its effect on the vitality of certain weed seeds. Sci. Agric. (Ottawa) 17, 492–501. doi: 10.4141/sa-1937-0036
Toleikiene, M., Arlauskiene, A., Šarunaite, L., Šidlauskaite, G., and KadŽiuliene, Ž. (2020). The effect of plant-based organic fertilisers on the yield and nitrogen utilization of spring cereals in the organic cropping system. Zemdir. Agric. 107, 17–24. doi: 10.13080/z-a.2020.107.003
Toole, E. H., and Brown, E. (1946). Final results of the duvel buried seed experiment. J. Agric. Res. 72, 201–210.
Trolove, M. R., and Dowsett, C. A. (2015). Yellow bristle grass seed killed in maize silage. N. Z. Plant Protect. 68:442. doi: 10.30843/nzpp.2015.68.5847
van Eekeren, N., Fehér, L., Smeding, F., Prins, U., and Jansonius, P. J. (2006). “Controlling broad-leaved dock (Rumex obtusifolius) in grass clover mixtures,” in Sustainable Grassland Productivity: Proceedings of 21st General Meeting of the European Grassland Federation, eds. J. Lloveras, A. Gonzáles-Rodríguez, O. Vázquez-Yánez, J. Pineiro, O. Santamaría, L. Olea, et al. (Madrid, Spain), 396–398.
Vollrath, B., Werner, A., Kretzer, D., Marzini, K., Illies, I., and Klemisch, M. (2016). Energetische Verwertung von kräuterreichen Ansaaten in der Agrarlandschaft - eine ökologische und wirtschaftliche Alternative bei der Biogasproduktion (Phase II). Veitshöchheim: Bayerische Landesanstalt für Weinbau und Gartenbau.
Warwick, S. I., and Black, L. D. (1988). The Biology of Canadian Weeds. 90: Abutilon theophrasti. Can. J. Plant Sci. 68, 1069–1085.
Weber, E., and Bleiholder, H. (1990). Explanations of the BBCH decimal codes for the growth stages of maize, rape, faba beans, sunflowers and peas - with illustrations. Gesun. Pflanz. 42, 308–321.
Weller, S. L., Florentine, S. K., Sillitoe, J. F., Grech, C. J., and McLaren, D. A. (2016). An investigation of the effects of stage of ensilage on Nassella neesiana seeds, for reducing seed viability and injury to livestock. Sci. Rep. 6, 1–7. doi: 10.1038/srep22345
Westerman, P. R., and Gerowitt, B. (2012). The probability of maize biomass contamination with weed seeds. J. Plant Dis. Protect. 119, 68–73. doi: 10.1007/BF03356422
Westerman, P. R., and Gerowitt, B. (2013). Weed seed survival during anaerobic digestion in biogas plants. Bot. Rev. 79, 281–316. doi: 10.1007/s12229-013-9118-7
Westerman, P. R., Hildebrandt, F., and Gerowitt, B. (2012). Weed seed survival following ensiling and mesophilic anaerobic digestion in batch reactors. Weed Res. 52, 286–295. doi: 10.1111/j.1365-3180.2012.00918.x
Westerman, P. R., Liebman, M., Menalled, F. D., Heggenstaller, A. H., Hartzler, R. G., and Dixon, P. M. (2005). Are many little hammers effective? Velvetleaf (Abutilon theophrasti) population dynamics in two- and four-year crop rotation systems. Weed Sci. 53, 382–392. doi: 10.1614/WS-04-130R
Westerman, P. R., Wes, J. S., Kropff, M. J., and van der Werf, W. (2003). Annual losses of weed seeds due to predation in organic cereal fields. J. Appl. Ecol. 40, 824–836. doi: 10.1046/j.1365-2664.2003.00850.x
Woodward, T. E. (1940). The Viability Of Seeds As Affected By The Siloing Process. J. Dairy Sci. 23, 267–271. doi: 10.3168/jds.S0022-0302(40)95520-5
Wyss, U., and Pradervand, N. (2017). Einfluss der Silierdauer auf die Qualität einer Maissilage. Agrarforsch. Schw. 8, 348–355. https://www.agrarforschungschweiz.ch/2017/09/einfluss-der-silierdauer-auf-die-qualitaet-einer-maissilage/
Keywords: silage substrate, ensiling condition, integrated weed management, seed survival, hard seeds
Citation: Hahn J, de Mol F and Müller J (2021) Ensiling Reduces Seed Viability: Implications for Weed Management. Front. Agron. 3:708851. doi: 10.3389/fagro.2021.708851
Received: 12 May 2021; Accepted: 12 July 2021;
Published: 05 August 2021.
Edited by:
Francesco Vidotto, University of Turin, ItalyReviewed by:
Ali Ahsan Bajwa, New South Wales Department of Primary Industries, AustraliaMichaela Kolarova, Czech University of Life Sciences Prague, Czechia
Copyright © 2021 Hahn, de Mol and Müller. This is an open-access article distributed under the terms of the Creative Commons Attribution License (CC BY). The use, distribution or reproduction in other forums is permitted, provided the original author(s) and the copyright owner(s) are credited and that the original publication in this journal is cited, in accordance with accepted academic practice. No use, distribution or reproduction is permitted which does not comply with these terms.
*Correspondence: Juliane Hahn, to.juliane.hahn@web.de