- Department of Chemistry and Biology, Ryerson University, Toronto, ON, Canada
Hybrid offspring of crops and their wild relatives commonly possess non-adaptive phenotypes and diminished fitness. Regularly, diminished success in early-generation hybrid populations is interpreted to suggest reduced biosafety risk regarding the unintended escape of novel traits from crop populations. Yet hybrid populations have been known to evolve to recover fitness relative to wild progenitors and can do so more rapidly than wild populations, although rates of evolution (for both hybrid populations and their wild progenitors) are sensitive to environmental context. In this research, we asked whether hybrid populations evolved more rapidly than wild populations in the context of soil moisture. We estimated evolutionary rates for 40 Raphanus populations that varied in their history of hybridization and environmental context (imposed by an experimental moisture cline) in two common gardens. After five generations of growing wild and crop-wild hybrid populations across a soil-moisture gradient, hybrid populations exhibited increased seedling emergence frequencies (~6% more), earlier emergence (~1 day), later flowering (~3 days), and larger body size (15–35%)—traits correlated with fitness—relative to wild populations. Hybrid populations, however, exhibited slower evolutionary rates than wild populations. Moreover, the rate of evolution in hybrid populations was consistent across evolutionary watering environments, but varied across watering environments in wild populations. These consistent evolutionary rates exhibited in hybrid populations suggests the evolution of robust traits that perform equally across soil moisture environments—a survival strategy characterized as “jack of all trades.” Although, diverse integrated weed management practices must be applied to wild and hybrid genotypes to diversify selection on these populations, evaluating the evolutionary rates of weeds in diverse environments will support the development of multi-faceted weed control strategies and effective integrated weed management policies.
Introduction
Genes from crop and wild progenitors contribute genetic variation that may support crop-wild success in a diversity of environments (managed or unmanaged) and/or through increased competitive ability with other uncultivated populations (Warwick et al., 1986; Langevin et al., 1990). Root system structure, early flowering, and asynchronous emergence rates are examples of specific traits that wild populations can possess that better support competitive growth in multiple environments (Conner and Via, 1993; Casper and Jackson, 1997; Sahli et al., 2008). Crop plants often possess traits that are rare or absent in wild populations (e.g., salinity tolerance and herbicide resistance) which can improve crop-wild hybrid survival and reproduction in stressful environments (Gasser and Fraley, 1989; Ellstrand and Hoffman, 1990; Bagavathiannan and Van Acker, 2008). Crop-wild hybrid offspring often share morphological features with their crop progenitors and avoid eradication by farmers in the field (Ye et al., 2019); thereby enhancing their survival in agricultural fields. Furthermore, crop-derived traits such as early emergence and high seed production can contribute to crop-wild hybrid success when competing with wild populations in natural environments (Snow and Campbell, 2005; Kost et al., 2015). Thus, we predict that populations that are capable of rapidly evolving these traits may be more successful than populations that evolve these traits slowly.
Cultivated radish (Raphanus sativus L.) and wild radish (or jointed charlock, Raphanus raphanistrum L.) are annual, insect pollinated, self-incompatible, diploid species that can hybridize (Panetsos and Baker, 1967). Cultivated radish is an annual crop species that flowers late in the growing season, exhibits low rates of dormancy and rapid germination, grows large, and edible hypocotyls (i.e., roots; Snow and Campbell, 2005). In contrast, wild radish flowers early in the growing season, has a long-lived seed bank, exhibits seed dormancy, and variable germination times after soil disturbance, and develops a relatively small, inedible mature hypocotyl. Wild radish is a common weed in agricultural systems in Australia, temperate North America, Europe, and also found in disturbed and coastal sites in temperate climates (Holm et al., 1997; Ashworth et al., 2016). The success of their hybrid derivative (R. raphanistrum × R. sativus) as an aggressive weed is apparently environmentally dependent (Campbell et al., 2006, 2009a,b; Hegde et al., 2006; Campbell and Snow, 2009; Ridley and Ellstrand, 2010; Hovick et al., 2012). Moreover, hybrid radish populations tend to evolve faster than wild radish populations but this has varied with selection pressure (Campbell et al., 2009a,b). Since fitness of crop-wild hybrid radish relative to wild radish has varied with diverse moisture, temperature and ecological contexts (Campbell et al., 2006; Hovick et al., 2012), we chose to manipulate moisture conditions in field plots to explore the influence of moisture on the relative fitness of crop-wild hybrids.
The success of crop-wild hybrid populations may also depend on their environment, as certain adaptive traits unique to crop-wild hybrids may be especially advantageous in specific environments (Campbell and Snow, 2007; Arnold and Martin, 2010; Hovick et al., 2012; Hartman et al., 2013). A model example of environmentally-dependent hybrid invasive success is found in the crop-wild hybrid radish species complex. When surveyed over five decades ago, crop radish (Raphanus sativus L.) in California was found predominantly in coastal regions and wild radish (Raphanus raphanistrum L.) was found in inland regions, with the hybrid “wild” radish (R. sativus × R. raphanistrum) growing between the two areas (Panetsos and Baker, 1967). Subsequent surveys found predominantly hybrid populations had spread across all regions of California (Ellstrand and Marshall, 1985; Nason and Ellstrand, 1995). These hybrid populations were particularly successful in evolving invasive traits and genetically swamping both parental populations (Hegde et al., 2006). In contrast, experimental populations of crop-wild hybrid radish are also capable of persisting for up to a decade in Michigan or Ontario (Snow et al., 2010; Shukla et al., unpublished data) but have not spread as they did in California (Snow et al., 2001; Campbell et al., 2016b; Teitel et al., 2016b). This evidence suggests that hybrid populations successfully evolve competitive weed strategies in some environments and not others, prompting us to ask how the environment influences the rate of evolution in hybrid populations relative to wild populations.
Whether they differ among genotypes or environments, rates of evolution (ROE) vary among plant populations. A difference in ROE across natural environmental pressures such as temperature and water availability has been observed, with higher temperature and water availability resulting in an increased rate of evolution (Rohde, 1992; Wright et al., 2006; Goldie et al., 2010). A variety of other abiotic environmental factors such as CO2 concentrations (Ward et al., 2000), soil pH (Snaydon and Davies, 1972; Davies and Snaydon, 1976), and soil contaminants like zinc (Antonovics and Bradshaw, 1970) and copper (Macnair et al., 1993) also have the potential to affect a population's ROE, with stronger selection pressures increasing the rate of evolution (Bone and Farres, 2001). Artificial selection and other anthropogenic activities have also been observed to affect ROE (e.g., herbicide resistance; Powles et al., 1998; Mallory-Smith et al., 1999). These examples have demonstrated evolutionary change in response to selection over a relatively short time period (i.e., contemporary evolution), anywhere between 1 to 124 years rather than thousands of years. When we can understand how various levels of climate related phenomena can influence selection and thus rates of evolution we can explore how climate change may complement other integrated weed management techniques which alter the speed of gene flow, rate of evolution, and expression of potentially weedy traits.
The growing number of studies on adaptive evolution in agricultural weeds is contributing to the growing body of literature on weed management that includes an evolutionary perspective (Dekker, 1997; Délye et al., 2013; Vigueira et al., 2013). Crop-wild hybrid weeds present a unique set of challenges to weed management, such as their potential for rapidly evolving weedy traits, and acquiring novel crop traits (Whitney et al., 2006; Schierenbeck and Ellstrand, 2009). Integrated weed management (IWM) is a component of integrated pest management (IPM), and is the strategy of applying many diverse weed control measures to diversify selection on a population at the same time including cultural, genetic, mechanical, biological, and chemical (Rodgers, 1978; Swanton and Weise, 1991). Using an IWM approach generates a plan of action to limit the success of genetically diverse weeds in agricultural contexts. When environmental selection (such as water availability) is imposed at different intensities and with differences in the consistency of selection between years, ROE may provide a metric with which we can compare various IWM control strategies.
To investigate the rate of evolution (ROE) between wild and crop-wild hybrid populations, we measured and compared ROE of fifth generation wild (Raphanus raphanistrum) and crop-wild hybrid (R. raphanistrum × R. sativus) radish plants from different evolutionary watering environments grown together in a common garden. First, we wanted to determine if, after five generations, mean trait responses varied between radish genotypes from different evolutionary (i.e., historical, over multiple generations) watering environments. Then, considering what we know of the genetic diversity of hybrid populations, we expect a faster rate of evolution compared to wild populations (Anderson and Stebbins, 1954; Lavergne and Molofsky, 2007; Campbell et al., 2009a,b). Given this, we ask whether hybrid radish populations always evolve weedy traits faster than wild radish populations. Due to the numerous observed cases of hybrid populations being more successful in particular environments (Ellstrand and Schierenbeck, 2006; Whitney et al., 2009), we expect to see differences in rate of evolution due to the selection environment within which these plants evolved (Campbell and Snow, 2007; Hovick et al., 2012: Hartman et al., 2013). Alternatively, hybrid success across environments may resemble a generalist approach (“jack of all trades”) in which evolutionary rates are similar, suggesting the evolution of robust traits that perform equally across environments.
Materials and Methods
Seed History of Wild and Hybrid Radish Populations Used in our Experiment
Ancestral populations (i.e., F0 generation) of wild radish (Raphanus raphanistrum) were collected from greenhouse populations that were grown for several generations near Binghamton, NY, USA (Conner and Via, 1993). The crop radish (Raphanus sativus) cultivar used was Red Silk (Harris-Moran Seed Company, Modesto, CA, USA). As in (Campbell et al., 2016a), in 2010, both cultivated and wild plants (nine seedlings per genotype) were planted in 36 plots as part of a randomized block design at the Waterman Farm at Ohio State University in Columbus, Ohio USA, within a larger experiment (Sneck, 2012; Campbell et al., 2016a; Figure 1). Ancestral seedlings were planted in one of four watering treatments with one plot per treatment, per block, for a total of 10 blocks (thus we originally planted 40 populations; however four populations did not produce any F1 seeds). Plots were ~200 meters apart to minimize gene flow among plots; although likely negligible, some gene-flow may have occurred. In the F0 generation, gene-flow naturally occurred within mixed plots of wild and cultivated-crop plants and gave rise to the first generation (i.e., F1) of wild and crop-wild hybrid (R. sativus × R. raphanistrum) seeds (Teitel et al., 2016a). As previously described (e.g., Campbell et al., 2016a; Teitel et al., 2016a), we manipulated soil moisture using rain-out shelters and imposed one of four watering treatments within these plots/shelters to impose a natural selection experiment on replicated wild and crop-wild hybrid radish populations:
1. Low Rain: To create relatively dry soil conditions, water collected from low rain shelter barrels was withheld.
2. Control Unsheltered: To establish a control precipitation treatment, ambient rainwater fell on un-manipulated populations.
3. Control Sheltered: To determine the effect of a rain-out shelter (but not manipulation of moisture availability) on plant growth, ambient rainwater, collected from the shelter, was applied to the plot.
4. Double Rain: To create relatively wet soil conditions, water collected from double rain and low rain shelters was applied to double rain plots; that is, double the ambient rainfall.
The F1 and following generations of wild and crop-wild hybrid seeds were grown at the Koffler Scientific Reserve (KSR) on Jokers Hill, King City, Ontario, Canada (lat. 44°0′ N, long. 79°3′ W; elevation 285 masl) when the Campbell lab relocated from Columbus, Ohio to Toronto, Ontario, Canada. Because we were moving locations and reconstituting 40 populations from 18 wild and 18 hybrid seeds producing populations, all F1 wild seeds were randomly assigned to a population and all F1 hybrid seeds were randomly assigned to a new population at KSR. As described in Teitel et al. (2016a,b), at KSR, F1 seeds from 36 F0 populations were grown in germination trays in a hoop-house. Wild and hybrid F1 seedlings were grown to the two-leaf stage, at which point they were transplanted (~117 seeds per plot) into sheltered and non-sheltered plots, plots which were scattered across KSR and were exposed to natural conditions (e.g., weather patterns, herbivory, and pollinators) and weeded to reduce interspecific competition. The plots were exposed to one of the four soil moisture conditions for an additional three generations (Figures 1A,B). Each of the four soil moisture treatments had five replicate populations for a total of 20 wild and 20 hybrid populations.
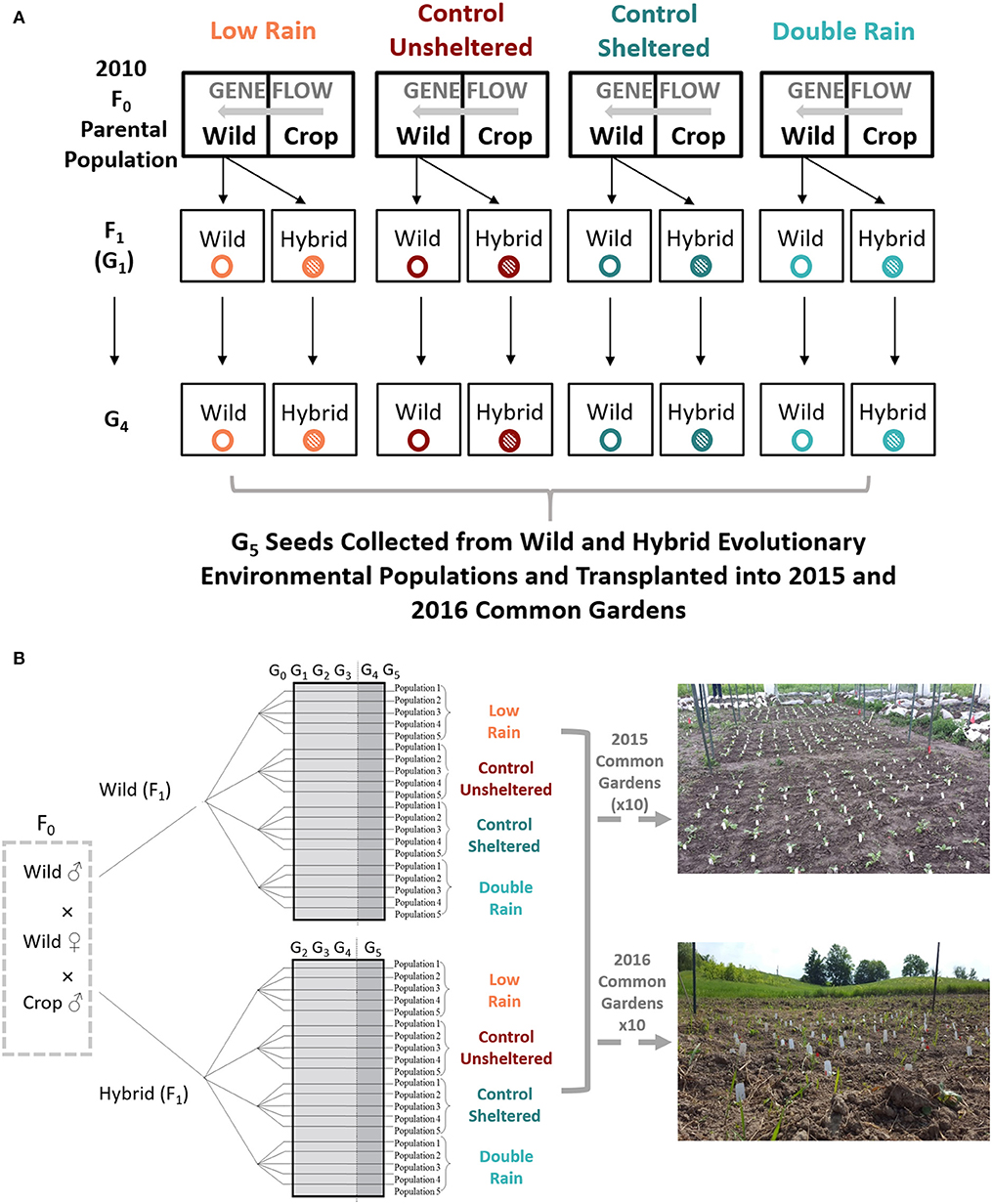
Figure 1. (A) The evolutionary history of fifth generation (G5) wild and hybrid Raphanus populations planted in 2015 and 2016 common gardens. In 2010, cultivated and wild Raphanus seeds were planted into one of four soil moisture treatments (F0: Low-Rain, Control-Unsheltered, Control-Sheltered, and Double-Rain; Waterman Farm at Ohio State University in Columbus, Ohio, USA). Gene flow naturally occurred within mixed plots of wild and cultivated plants which gave rise to first-generation (i.e., F1/G1) wild (R. raphanistrum—open colored circles) and crop-wild hybrid (R. sativus × R. raphanistrum – filled colored circles) seeds. Succeeding wild and hybrid generations (G2-G4) from 2011 to 2014 were transplanted into the same four soil moisture environments as F0 populations (Low-Rain, Control-Unsheltered, Control-Sheltered, and Double-Rain) at the Koffler Scientific Reserve (KSR), King City, Ontario, Canada. (B) Forty wild and hybrid 2nd generation (G2) to 4th generation (G4) plants (five replicate populations per watering treatment) were grown under the same watering conditions at the Koffler Scientific Reserve (KSR) in King City, Ontario, Canada. Fifth generation plants were transplanted and grown in 2015 and 2016 common gardens at KSR.
Rain-out shelters were 3.05 m by 2.44 m wooden frames with transparent sheet plastic stretched over the frame, acting as a roof that blocked rain and minimally reduced light transmission; new sheet plastic was applied each year. Using metal poles, frames were slanted and elevated to ~1.2 m above ground at their lowest corner. Frames were slanted to intercept and divert natural rainfall into a 208 L plastic collection barrel via an eavestrough attached to the lowest side of the wooden frame (Supplementary Figure 1). Shelters were placed at least 40 m apart to reduce gene flow among plots, as in the F0 generation. Since wild radish have long-lived seed banks and since annual regeneration of populations was a result of seeds that dropped to the ground and naturally germinated, fruits collected from the pedicels of senescing plants in 2015/2016 could have belonged to one of three generations (F2-F5). For simplicity, we refer to these plants as G2-G5 generation seeds. Small population size in the F1, G2, and G3 generations meant that populations may have experienced genetic drift (Teitel et al., 2016a). However, the experimental design used allowed us to detect the consequences of genetic drift—if there was substantial genetic drift, we predicted there would be significant differentiation among the five replicate lineages, within experimental treatment combinations; which we did not find (Shukla et al., unpublished data). Wild and hybrid G5 seeds were collected from G4 plants in fall 2014 and used in both 2015 and 2016 experiments.
Common Garden Set-Up
To estimate the rate at which phenotypes diverged after five generations of selection, we grew two common gardens (one in the 2015 growing season and another in 2016) of G5 wild and hybrid plants. In 2015, 10 common garden plots (3.5 m . 3.0 m), treated as blocks, were tilled and planted with a total of 120 seeds per block; from each G5 genotype by evolutionary watering environment combination, 15 seeds were randomly selected from each of the five populations of each genotype (20 wild populations and 20 hybrid populations; Figure 1B). On June 1-2, 2015, we planted seeds in the soil in a 10 × 12 grid, with 30 cm spacing between plants, arranged in a randomized, complete block design. Common garden rainfall was not manipulated in either common garden and plots were weeded to reduce competition. In 2016, we replicated the 2015 experiment at a second site at KSR. The second common garden was tilled and 10 experimental blocks (3.5 m . 3.0 m) arranged in a randomized, complete block design on May 20th and May 24th, 2016. In 2016, every genotype by evolutionary watering environment combination from 2015 along with three crop seeds (Raphanus sativus) were planted in each block. However, these plants were removed from the analysis due to lack of replication across years. Due to limited seed stock, we planted 100 seeds per block in each common garden. We harvested the plants as they senesced, when flowering was complete and at least 10 fruits were ripe. At the end of the growing season (October 15th, 2015 and 2016), all remaining plants were harvested. Natural rainfall varied over the growing season between common garden years, with a cumulative rainfall of 307.8 mm in 2015 and 206.7 mm in 2016 (nearest weather station: Buttonville, Ontario 43°51′39.000″ N, 79°22′07.000″ W; Government of Canada, 2018).
Trait Measurement
Flower color, a simply inherited trait, differs between wild and crop radish plants and is a visual marker to track crop trait introgression at one locus in hybrid populations (Snow et al., 2001; Campbell et al., 2006). Wild radish (Raphanus raphanistrum) is homozygous recessive for yellow flower petal color and crop radish (R. sativus) is homozygous dominant for white or pink flower petal color (Panetsos and Baker, 1967; Kay, 1976; Stanton et al., 1989). In hybrid populations, the white petal color exhibits Mendelian dominance over the yellow petal color, and therefore allows us to track crop allele persistence (Panetsos and Baker, 1967; Stanton et al., 1989) into advanced populations of crop-wild Raphanus hybrids. Hues of pink petal color is controlled by two additional loci (Panetsos and Baker, 1967) but variation in pink hue was not tracked in this experiment.
Radish hybrids can be heterozygous for a reciprocal translocation that can affect chromosome pairing during meiosis (Panetsos and Baker, 1967; Campbell et al., 2006). This translocation can affect pollen fertility and produce up to ~60% aborted pollen grains in F1 hybrid progeny (Snow et al., 2001; Campbell et al., 2006). After four generations of evolution, we compared hybrid pollen fertility to that of wild populations to determine the rate of evolution in hybrid pollen fertility across environments. To assess pollen viability of G5 hybrid populations relative to the pollen viability of wild radish, we collected a single, newly opened flower from each plant (n~1,000 plants/year) during August, 2015 and July–August, 2016 between the hours of 8:00 am and 12:00 pm and refrigerated at 2°C until processing. At the time of staining, two anthers were collected and wiped on microscope slides (VWR VistaVision, Radnor, PA, USA). Slides were stained with Alexander stain (Alexander, 1969) and stored in slide boxes. After staining, we measured pollen fertility by categorizing at least 100 pollen grains per plant as either the number of aborted or fertile pollen grains using a compound microscope (Nikon©, H550L, Japan).
We monitored each seed daily to record the date of seedling emergence from the soil and first flower during the experimental period (June to October 15, 2015 and June to August 26, 2016). From this, the days to seedling emergence and age at first flowering (i.e., number of days between anthesis and emergence) was calculated. Additional life-history traits (e.g., longest leaf length, stem diameter) were measured at the date of first flower.
To measure the photosynthetic performance of plants in the different watering treatments, and to evaluate whether water stress or excess water influenced photosynthesis, we measured the dark-adapted quantum efficiency of photosystem II (PSII) as the ratio of variable (Fv) to maximal (Fm) chlorophyll fluorescence (Maxwell and Johnson, 2000). Because all reaction centers were fully oxidized by shading prior to measurement, Fv/Fm represents the maximum capacity of PSII to absorb light energy. In both years, we took outdoor measurements using a portable fluorescence meter (Handy PEA fluorometer, Hansatech Instruments Ltd., King's Lynn, UK). Prior to experimental measurement, we randomly sampled 100 plants to determine a standard curve of the minimum time it took reaction centers to become fully oxidized (i.e., a dark adaptation period) where fluorescence remained consistent; this occurred after ~10 min. Then, for the experiment, we non-destructively sampled quantum efficiency of PSII on a subset of 24 plants (three plants per genotype × evolutionary watering environment combination) per block for a total of 240 plants across the whole experiment in 2015 and another 240 plants in 2016. In random order, we measured plants after a 10-min dark adaptation period. Measurements were collected on July 7, 2015 and between June 29 and July 3, 2016, between 8:00 am and noon.
Evolutionary Divergence Rate Metrics
Calculations of evolutionary rates are based upon the average phenotypic difference between two populations (usually described as Δ), relative to the time since isolation (i.e., Δt) and are commonly measured in darwins or, more recently, haldanes (Haldane, 1949; Gingerich, 1983, 1993). Because we compared independently evolving populations, we calculated synchronic rates of evolutionary divergence in haldanes (Hendry and Kinnison, 1999; Bone and Farres, 2001), using the following equation:
where the mean trait values of control-sheltered wild and control-sheltered hybrid populations were represented by and trait values of low rain, control-unsheltered, or double rain wild and hybrid populations were represented by in Equation 1. By calculating haldanes, mean trait evolution was standardized by incorporating pooled trait variances (sp) and measuring evolutionary change through time (t2-t1 = 5 generations, or F0 – F5) (Haldane, 1949; Gingerich, 1993, 2001). We calculated the natural log of trait values to reduce heteroscedasticity in the dataset since standard deviations are typically expected to increase with the mean, particularly for morphological traits (Wright, 1968; Hendry and Kinnison, 1999). Although haldanes are more commonly used to measure contemporary evolutionary rates, we have calculated evolutionary rates in darwins (d), as well, presented in Supplementary Table 1.
Statistical Analysis
To determine if seedling emergence and flowering frequency differed between genotypes (wild vs. hybrid) from different evolutionary watering environments (Low Rain, Control Unsheltered, Control Sheltered, and Double Rain), we ran a generalized linear mixed-model ANOVA fitted to a binomial distribution; 0 indicating no emergence/flowering and 1 indicating emergence/flowering. In our model, genotype and evolutionary watering environment were fixed effects and year a random effect; block as a random nested effect within year was not significant and, therefore, omitted from the model to increase statistical power.
To determine if four phenotypic traits (days to emergence, days to first flower, longest leaf length, and stem diameter) differed between genotypes (wild vs. hybrid) from different evolutionary watering environments (Low Rain, Control Unsheltered, Control Sheltered, and Double Rain), we ran a linear nested mixed-model ANOVA. Considering our traits of interest are highly correlated (Supplementary Table 2), we performed a principal components analysis (PCA) which allows correlated variables to be condensed into two or fewer composite proxy variables (Abdi and Williams, 2010; Mohammed et al., 2016). In the PCA, we fitted our data to a correlation matrix, extracted the first two principal components, and ran these in our mixed-model ANOVA (loadings discussed in Results). In our ANOVA model, genotype and evolutionary watering environment were fixed effects and block nested within year as a random effect. Finally, to determine whether white flower color, pollen fertility, and chlorophyll fluorescence differed between genotypes (wild vs. hybrid) from different evolutionary watering environments, we ran a linear nested mixed-model ANOVA, with genotype and evolutionary water environment as fixed effects and block nested within year as a random effect. Due to varying sample sizes associated with white flower color, pollen fertility, and chlorophyll fluorescence, these traits were unable to be incorporated into our PCA.
To determine if rates of evolutionary divergence of four phenotypic traits (days to emergence, days to first flower, longest leaf length, and stem diameter) differed between genotypes (wild vs. hybrid) from different evolutionary watering environments (Low Rain, Control Unsheltered, and Double Rain), we performed a mixed-model, repeated-measures ANOVA. Similar to our mean trait analysis, evolutionary divergence rates of our traits were highly correlated (Supplementary Table 2) so, we ran a principal components analysis (PCA) fitted to a correlation matrix and extracted two principal components and ran these in our mixed-model ANOVA (loadings discussed in Results). Genotype, evolutionary watering environment, and their interaction were fixed between-subjects effects, and year was a random within-subjects effect. Finally, we ran a repeated-measures ANOVA for the frequency of white petalled plants with evolutionary watering environment as the main between-subjects effect and year as the random within-subjects effect. Due to smaller datasets for the traits chlorophyll fluorescence and pollen fertility (i.e., sample size within each experimental level within a treatment), we did not statistically compare these traits among genotypes or evolutionary watering environment.
Prior to running any PCA or mixed model ANOVA, response variables were transformed, if needed, to meet assumptions of normality. Furthermore, due to the non-orthogonality of these data, type III ANOVA results applied a Kenward-Roger's adjustment for computing the denominator degrees of freedom (Luke, 2017). Analyses were performed in R-Studio (Version 1.3.959; packages stats and lme4).
Results
Trait Variation Between Crop-Wild Hybrid and Wild Populations From Varying Evolutionary Watering Environments
Hybrid seedlings, irrespective of the watering environment they evolved in, had significantly higher (~6.0%) emergence frequency than wild seedlings. However, of the plants that emerged, survival to flowering (i.e., flowering frequency) did not differ between wild and hybrid populations (Table 1). Irrespective of genotype, seedling emergence frequency and flowering frequency did not significantly vary between plants from different evolutionary watering environment histories (Table 1). Finally, considering the genotype by evolutionary watering environment interaction, hybrid plants from double rain environments emerged significantly more frequently (29% more, Table 1), but did not differ significantly in their survival to flowering, than wild plants from the same environment (Table 1). However, seedling emergence frequency and flowering frequency of hybrid and wild populations from low rain, control unsheltered, and control sheltered environments did not significantly differ (Table 1).
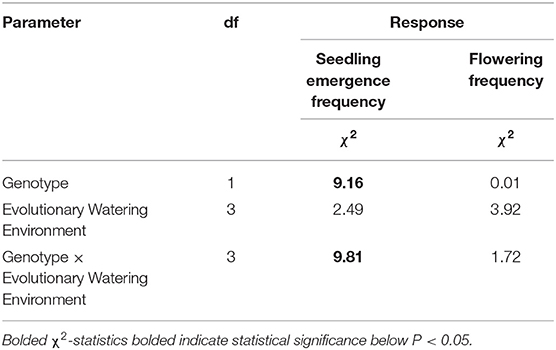
Table 1. Mixed-model ANOVA for seedling emergence (n=1966) and flowering frequency (n=1413) fitted to a binomial distribution.
Hybrid populations exhibited lower pollen fertility (~9%, Table 2) and higher chlorophyll fluorescence (i.e., better quantum use efficiency of PSII; Table 2) than wild populations, however, both traits did not differ between plants from different evolutionary watering environments and had no significant genotype by evolutionary watering environment interactions (Table 2; mean values presented in Supplementary Table 3). Finally, considering only hybrid populations, the frequency of white flowered plants did not significantly differ between populations from different evolutionary watering environments (Table 2; mean values presented in Supplementary Table 3).
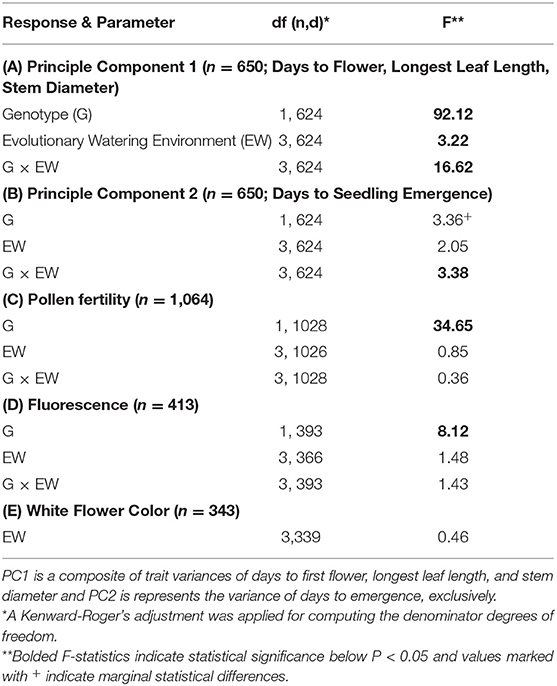
Table 2. Mixed-model ANOVA of mean phenotypic trait values (A) principle component 1, (B) principle component 2, (C) pollen fertility, and (D) chlorophyll fluorescence (proxy of water-use-efficiency).
Running our PCA, we found that two principal components were sufficient to cumulatively explain 83.5% of the trait variance in our four traits. The variance of principal component 1 (PC1) loaded heavily onto days to first flower (49.3%), leaf length (59.8%), and stem diameter (62.0%). Principal component 2 (PC2) loaded almost exclusively onto days to emergence (99.1 %). We, therefore, ran our ANOVA on our two proxy composite variables (PC1 and PC2). For traits that loaded onto PC1 (days to first flower, longest leaf length, and stem diameter), there was a significant genotype, evolutionary watering environment, and genotype by evolutionary watering environment effect. For the trait that loaded onto PC2 (days to emergence) there was a marginally significant genotype effect and a significant genotype by evolutionary watering environment interaction but no evolutionary watering environment effect (Table 2). Below, we present trends associated with PC2 (i.e., emergence time) followed by PC1 (flowering time, leaf length, and stem diameter) based on their model significances.
Hybrid plants tended to emerge slightly earlier (~1 day), flower later (~3 days), grow longer leaves (~15%), and wider stems (~35%) than wild plants. Irrespective of genotype, plants from double rain evolutionary environments flowered later (~2 days), grow longer leaves (~6%), and wider stems (~8%) than plants from control sheltered evolutionary environments, with no differences between emergence times. Finally, days to emergence (PC2) did not differ between hybrid and wild plants from low rain, control unsheltered, control sheltered, and double rain environments (i.e., no genotype by evolutionary watering interaction; Table 3). However, hybrid plants from low rain, control unsheltered, and double rain environments took longer to flower, had longer leaves, and wider stem diameters then wild plants from the same environments (i.e., differed with respect to PC1; trait mean table presented in Table 3), with no difference between genotypes from control sheltered environments.
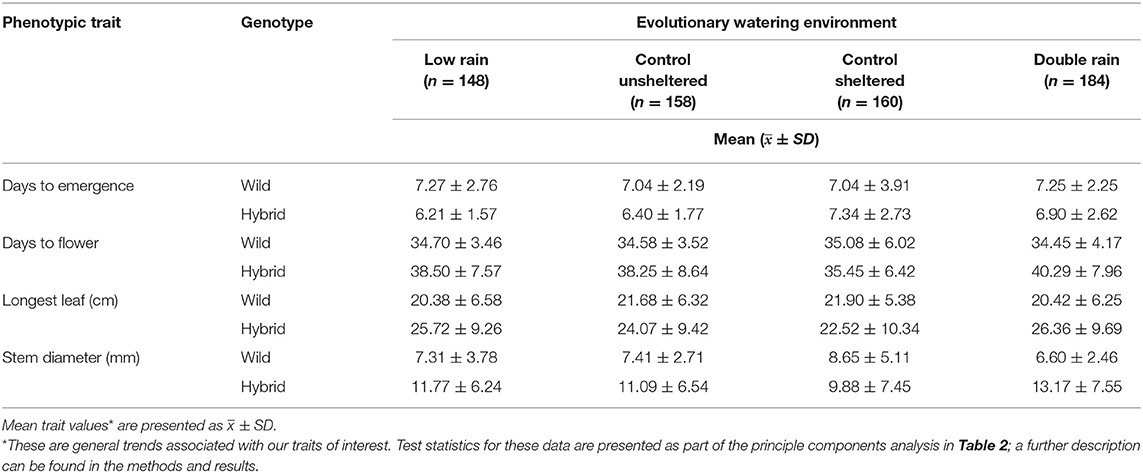
Table 3. Mean phenotype values* of four traits across evolutionary watering environments for wild and hybrid radish plants.
Evolutionary Divergence Rates of Crop-Wild Hybrid and Wild Populations From Extreme Watering Environments
Considering hybrid populations only, the watering environment in which hybrid population evolved did not significantly affect divergence rates of white-flower colored plants. In fact, the rate at which the proportion of white-flowered plants evolved in environments of low rain, control unsheltered, and double rain did not deviate from white-flowered plants of control sheltered environments (Table 4).
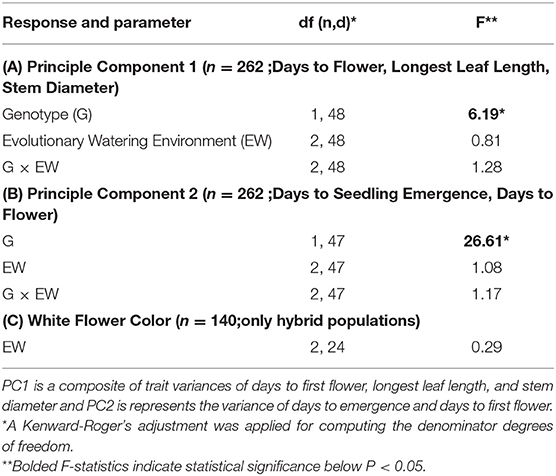
Table 4. Mixed model ANOVA of mean evolutionary divergence rates of (A) principle component 1 (PC1), (B) principle component 2 (PC2), and (C) white flower color.
We found two principal components were sufficient to cumulatively explain 86.6% of the variation among divergence rates of our four traits. Principal component 1 (PC1) loaded, relatively evenly, onto the rate of evolutionary divergence of days to first flower (55.9%), leaf length (61.0%), and stem diameter (54.6%). Principle component 2 (PC2) loaded heavily onto rates of divergence of days to emergence (82.9 %) and days to first flower (50.2%). We, therefore, ran our ANOVA on our two proxy composite variables (PC1 and PC2). For the divergence rates of traits that loaded on to PC1 (days to first flower, longest leaf length, and stem diameter) and PC2 (days to emergence, days to first flowering) there was a significant genotype effect but no evolutionary watering environment or genotype by evolutionary watering environment interaction (Table 4; Figures 2, 3). Below, we present trends of divergence rates associated with PC2 and then PC1.
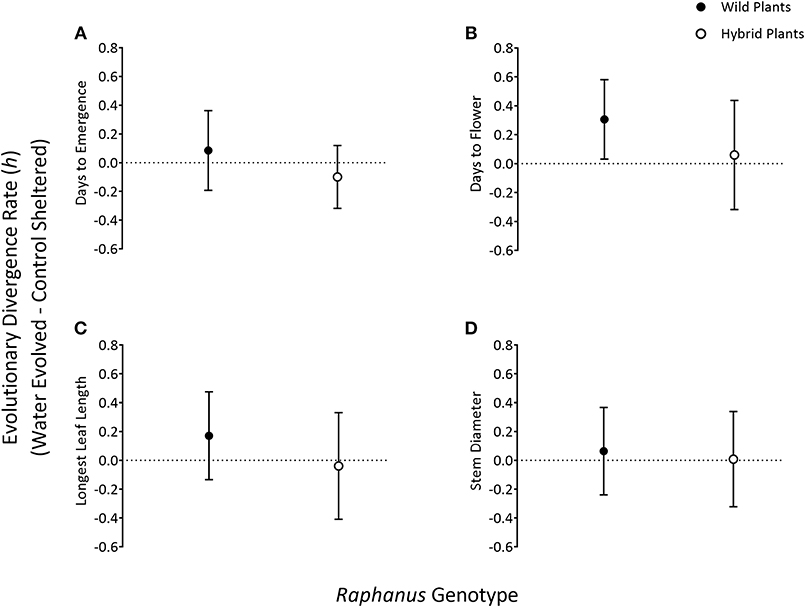
Figure 2. Comparing evolutionary rate estimates [presented in haldanes (h) ± SD] of four traits—days to seedling emergence (A), days to flowering (B), longest leaf length (C), and stem diameter (D)—between G5 radish weed genotypes (wild—closed circles, hybrid—open circles). Among wild populations, values represent the change between wild, water-evolved populations and wild, control-sheltered (CS) populations. Similarly, among hybrid populations, values represent the change between hybrid, water-evolved, and control-sheltered populations.
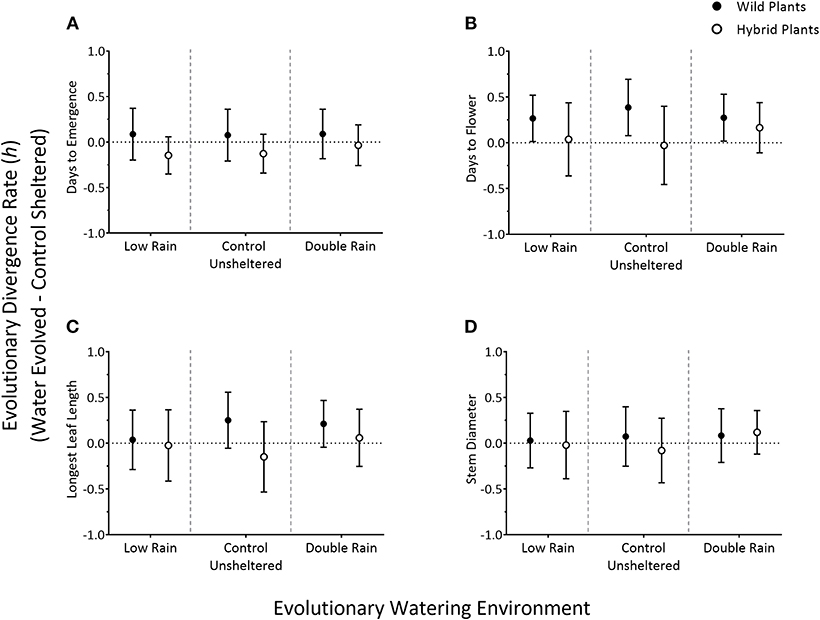
Figure 3. Comparing evolutionary rate estimates [in haldanes (h) ± SD] of four traits—days to seedling emergence (A), days to flowering (B), longest leaf length (C), and stem diameter (D)—of G5 wild and hybrid radish plants (wild—closed circles, hybrid—open circles) from one of three environmental conditions (low-rain, control-unsheltered, double rain; separated by a gray dashed line) grown in common garden conditions. Values represent change between water-evolved plants and control sheltered plants for each respective treatment (i.e., change between Low Rain-evolved and Control Sheltered, Control Unsheltered-evolved and Control Sheltered, and Double Rain-evolved and Control Sheltered).
After five generations of evolution, hybrid and wild populations evolved similar days to seedling emergence rates but in different in directions (PC2). Specifically, hybrid populations from extreme watering environments evolved earlier days to emergence relative to hybrid control sheltered populations (Figure 2A) whereas, wild populations from extreme watering environments evolved longer days to emergence relative to wild control sheltered populations (Figure 2A). The speed of evolution of days to first flower (PC1 & PC2) also differed, where hybrid populations from extreme watering environments evolved more slowly than wild populations from extreme watering environments relative to their control sheltered phenotypes, respectively (Figure 2B). Leaf morphology (PC1) evolved more slowly in hybrid populations and in different directions compared to wild populations. Specifically, hybrid populations from extreme watering environments evolved shorter leaves relative to hybrid control sheltered populations whereas, wild populations from extreme watering environments evolved longer leaves relative to wild control sheltered populations (Figure 2C). Lastly, after five generations, stem diameter morphology (PC1) evolved more slowly in hybrid populations compared to wild populations. Specifically, stem diameter phenotypes in hybrid population from extreme watering environments did not evolve away from hybrid control shelter phenotypes (Figure 2D); but wild populations from extreme watering environments evolved larger stem diameters relative to wild control sheltered population (Figure 2D).
Discussion
After five generations of selection on wild and crop-wild hybrid populations across a soil-moisture gradient, and contrary to our expectations, wild populations were more phenotypically diverse and evolved selective traits faster than their hybrid relatives, even though white flower color (a crop derived trait) in hybrid populations remained at relatively high frequencies across watering environments. Further, the proportion of white flower color plants, after five generations, did not diverge away from control phenotypes in response to extreme watering conditions. This suggests that crop traits in our populations have introgressed and persisted across soil moisture environments (Strauss et al., 2004; Irwin and Strauss, 2005). Hybridization and evolutionary watering pressures, separately, promote increased emergence frequency, early emergence, later flowering, and larger morphology relative to wild populations. Furthermore, hybridization but not watering environment slowed rates of evolution across evolutionary watering environments. In contrast, wild populations across evolutionary watering environments grew to a smaller size, were quicker to flower and evolved relatively faster than crop-wild hybrid populations. Given the patterns in ROE we measured, we predict selection varied most in the control unsheltered treatment between years and plots whereas selection may have been more consistent in the sheltered plots (although still varying between years). Thus, based on the rate of evolution in wild versus hybrid populations measured here, wild populations may be more aggressive weeds than hybrid populations in Ontario if weediness is measured by rate of evolution (Bone and Farres, 2001; Whitney et al., 2006). We discuss the potentially adaptive weed strategies both wild and hybrid populations may be demonstrating and the implications of these strategies on weed management.
Hybridization and the Introduction of Canalized Traits as a “General Phenotype” Weed Strategy
Crop-wild hybrid populations emerged more frequently and earlier, flowered later, and were larger than wild populations. Among crop-wild hybrid populations, evolutionary rates across common gardens irrespective of their evolutionary watering selection history (Table 4; Figures 2, 3). In contrast, wild populations exhibited faster evolutionary rate responses across evolutionary watering histories. This insensitivity of hybrid populations to diverse watering environments may be driven by crop trait inheritance patterns. Traits in crop populations are sometimes selected to have reduced environmental sensitivity (but see Sadras et al., 2009) and produce a specific phenotype in response to a particular environment or a consistent response in variable environments (Nicotra et al., 2010). Traits that display this environmental insensitivity are, more generally, referred to as canalized traits (Weinig, 2000; Valladares et al., 2007; Matesanz et al., 2010). To achieve these standards, breeders limit the phenotypic response by breeding for allelic homozygosity (either through dominant or recessive alleles), depending on the trait (e.g., seed size and flowering time) (Nicotra et al., 2010; Snow et al., 2010; Flint-Garcia, 2013); these traits, therefore, may share the same set of alleles that respond similarly across environments (i.e., genetically identical and correlated; Via and Lande, 1985). Introgression of these canalized crop traits into crop-wild hybrid populations, may explain the lack of phenotypic plasticity and minimal differences in evolutionary rate estimates in our hybrid populations across environments.
Canalized trait responses, and therefore slow evolutionary rates, do not necessarily put crop-wild hybrids weeds at a disadvantage. Previous work conducted on related radish populations have found that hybrid radish populations have performed equally to, and at times better than, their wild radish counterparts across common gardens exposed to a variety of soil-moisture conditions (Shukla et al., unpublished data). Performing equally well, or slightly better, is characteristic of a generalist phenotype or “jack-of-all trades” adaptive strategy, where phenotypes display little plasticity and perform similarly across environments (Baker, 1965; Richards et al., 2006). This strategy has been seen before in other species (e.g., Solanum hispidum Pers., Ageratum houstonianum Mill., Chloris virgata Sw., and more, Hastwell and Panetta, 2005; Corispermum macrocarpum L. and Salsola collina Pall., Huang et al., 2009; Pichancourt and van Klinken, 2012; Parkinsonia aculeata L.). For example, fitness of a Centura species has similar expression across water flooding regimes (flooded vs. normal) (Richards et al., 2006). Similarly, two European lineages of Taraxacum officinale L. displayed a generalist strategy in response to water availability and shade (Oplaat and Verhoeven, 2015). Overall, our data suggest that hybridization and the introgression of crop traits, rather than extreme watering environments, may be a stronger factor influencing rates at which hybrid phenotypes evolve. The robust, and consistent, evolutionary rates displayed across extreme watering environments (i.e., lack of divergence from hybrid control phenotypes) suggest our crop-wild hybrid weeds are broadly tolerant to extreme watering regimes.
Water Availability Is Not a Strong Enough Disturbance to Drive Evolutionary Rates in Crop-Wild Hybrids
After five generations of growth under diverse and relatively extreme watering environments for Ontario, Canada, soil moisture alone does not appear to be a strong enough factor to drive rapid evolutionary divergence among crop-wild hybrid radish weeds. In response to water availability, hybrid weed populations may need longer than five generations for traits to evolve before they reach their “adaptive optimum” (Bock et al., 2015)—if they haven't already (Ord and Hundt, 2020). Alternatively, different abiotic and/or biotic factors (e.g., latitudinal clines, temperature, competition, herbivory, etc.) may elicit stronger and more rapid evolutionary responses and force hybrid weeds to “act,” or to use their standing genetic variation (Richards et al., 2006; Whitney and Gabler, 2008; Bock et al., 2015). For example, crop-wild hybrid sunflowers have rapidly (7–10 generations) evolved several morphological, phenological, and eco-physiological traits that increase survival (fitness-related trait) in response to varying herbicide, pesticide, and competition treatments (Mercer et al., 2007; Baack et al., 2008; Dlugosch and Parker, 2008; Presotto et al., 2016). Similarly, transgenic crops have contributed Bt, herbivory-resistance genes and glyphosate, herbicide-resistance genes from sunflower (Helianthus species) and kochia (Kochia species) plants, respectively, to sexually compatible, wild populations of related species. The resulting hybrid populations demonstrate strong selection over a few generations for these traits (Snow, 2002; Beckie et al., 2013). These selection pressures may drive rapid adaptation of weeds since pesticide/herbicide resistance and polyculture farming practices are important aspects in agroecology.
The relative success of crop-wild hybrid populations have varied across North America—from highly successful in California and Texas (Hegde et al., 2006; Hovick et al., 2012) to moderately successful in Michigan, USA and Ontario, Canada (Campbell et al., 2006; Campbell and Snow, 2007; Teitel et al., 2016a). Along with differences in soil moisture, one of the most apparent differences among these studies is temperature and length of growing season. Any one of these factors in conjunction with soil moisture could overcome canalized trait responses and increase the speed of contemporary evolution in hybrid radish weed populations. Furthermore, outside of an agronomic context, hybrid radish is a significant weed in natural and agricultural areas in California and Australia, as well as in its natural range in Eurasia (Ridley and Ellstrand, 2010). Our research suggests that soil moisture may impose variable strengths and directions of selection depending on where populations grow (cultivated vs. uncultivated areas) and may be reflected as diverse evolutionary rates; we encourage future research to compare rates of evolution in uncultivated areas. Finally, we recognize that the absence of phenotypic trait divergence in response to hybridization, water availability, and their interaction may be confounded with factors like abiotic and biotic pressures in their growth environment, phenotypic plasticity, and epigenetic effects of the maternal environment (Richards et al., 2006; Wolf and Wade, 2009; Germain et al., 2013; Campbell et al., 2015). With that in mind, future studies should consider taking the offspring of late generation plants (via a resurrection common garden approach; see Franks et al., 2018)—ensuring wild-wild and hybrid-hybrid mating—to measure evolutionary rates void of confounding environmental and epigenetic effects prior to dismissing the effect of soil moisture on evolutionary rates.
Water Availability as a Low-Disturbance Abiotic Factor Driving Evolutionary Rates in Wild Radish Populations
We found that wild radish populations were quicker to flower but had shorter leaves and thinner stem diameters than hybrid populations from the same evolutionary soil moisture environments. However, wild populations rapidly evolved later flowering phenologies and larger morphologies in response to extreme moisture environments (Figure 2) relative to wild control populations and did so faster than hybrid populations. Many weedy or wild relatives have evolved varying adaptive strategies to extremely low or high water availability over short time periods. For example, wild Mediterranean shrub Fumana thymifolia L. decreased seedling emergence during periods of drought (Jump et al., 2008) and wild weed Lupinus luteus L. delayed flowering in high rainfall environments (Berger et al., 2008; Berger and Ludwig, 2014). Delayed flowering and investment in growth can be an adaptive evolutionary approach to ensure survival in response to extreme—potentially unfavorable—environments (Grime, 1977; Berger and Ludwig, 2014). Though they may not flower as early, or for as long, investing in growth and defense against competitors increases opportunity for survival (Grime, 1977; Arendt, 1997).
Although our wild radish weed populations responded to watering environment, phenotypic changes and evolutionary rates were moderate compared to documented changes in response to other abiotic pressures. Wild or weedy plant species have displayed faster and stronger evolutionary rates in response to other abiotic environments (Bone and Farres, 2001; Berger and Ludwig, 2014), including but not limited to salinity (Kiang, 1982), herbicides (Powles et al., 1998; Mallory-Smith et al., 1999), and elevated soil pH (Snaydon and Davies, 1972; Davies and Snaydon, 1976). In each of these cases, the environment prompted the expression of novel phenotypes due to the traits' inherent plasticity and over a short period have been fixed due to their fitness-enhancing advantage (i.e., measured as reproductive success). Compared to our crop-wild hybrid populations, after five generations, our wild populations exhibited a more dramatic evolutionary response to watering environment but have been documented as having significant seed production more than or equally fecund to crop-wild hybrid populations (Shukla et al., unpublished data). Although wild radish have traditionally been known to grow and evolve relatively quickly in high disturbance environments (Snow and Campbell, 2005), our results suggest that wild radish populations can also evolve just as quickly in response to low-disturbance environments like soil moisture.
Implications for Weed Management and Future Directions
Weed management strategies within extreme watering environments will differ between wild and hybrid radishes. Hybrid seedlings may emerge more frequently, but these populations do not appear to evolve in response to soil moisture. Since hybrid populations responded to diverse watering environments in Ontario in similar ways (“generalist phenotype” strategy), weed management may be relatively consistent across moisture environments. Tilling repeatedly early in the season and delaying crop planting will support attempts to eradicate weedy crop-wild hybrid radish from agricultural environments under any moisture regime. Notably, hybrid radish appears to be easily controlled in agricultural environments worldwide but it is a significant weed in natural areas in California (Ridley and Ellstrand, 2010); suggesting that the environmental conditions that impose selection can vary depending on where populations are growing and may be reflected as different evolutionary rates. Therefore, eradication strategies of hybrid weeds in areas with different land-use histories may be more difficult to control and require new IWM strategies or strategies similar to those used for wild radish (see below); this, however, would need to be further investigated before any strategies can be implemented.
Evolutionary knowledge could be incorporated into managing plant population dynamics (and thus potentially weed management strategies) in a number of ways. For instance, reducing population size of a weed through altered cultural practices such as tilling or herbicides applications lowers the chance of population persistence via random genetic drift, assuming standing genetic variance is low (Goodman, 1987; Lande, 1993; Gomulkiewicz and Holt, 1995). However, if genetic variation is high (as in hybrid populations), crop-wild hybrid weed populations may still be able to respond to selection, overcome drift and reduce the degree to which hybrid populations are maladapted through rapid evolution (Husband and Campbell, 2004). This is where understanding rate of evolution can be a critical tool in weed management; if a weed population's ROE is slow, even moderate rates of genetic diversity will likely not be enough to support persistence, as population size may remain at a critically low level for too long (Gomulkiewicz and Holt, 1995). By understanding rate of evolution metrics and relative fitness of crop-wild hybrids, weed culling events (i.e., herbicide application or tilling) can be timed when the population is in a phase that is vulnerable to rapid demographic decline. Rapidly evolving populations will be slower to reach this vulnerable phase than slowly evolving populations, all else being equal; thus, it is important to know if weedy, crop-wild hybrid populations evolve at different rates from weedy progenitors. Knowing how these weed control methods act as selective pressures in promoting adaptive evolution is also vital in the execution of a successful IWM strategy. For example, extensive herbicide use can increase the rate of evolution of herbicide resistance. Therefore, mitigation techniques should diversify the herbicides used and reduce the number of application events to control weeds (Swanton and Weise, 1991; Bond and Grundy, 2001; Beckie, 2006). Although weed management strategies tend to vary between cultivars, understanding the effects of genetic diversity along with environmental context on the rate of adaptive evolution of weeds will be a key tool in an IWM plan. Here, we contribute to this work by starting to measure the rate of evolution in weed populations with elevated genetic diversity and diverse forms of selection imposed.
Wild radish weed mitigation strategies will differ from hybrid strategies, considering their ability to evolve relatively quickly in response to different watering environments. Wild radishes appear to pose a greater challenge in south-western Ontario agricultural environments because they are more likely to evade eradication based on traditional tilling schedules and herbicide applications (Monjardino et al., 2003; Warwick and Francis, 2005). For example, in Australia where wild radish is an aggressive weed, frequently rotating herbicide type and application time or altering crop harvest times (early vs. late) are strategies implemented to control wild radish weeds. Varying application strategies aim to prevent rapid evolution of herbicide resistance and/or phenology matching (between weed and crop), respectively, to ensure more successful weed removal and management (Ashworth et al., 2016). Knowing the rate at which new weeds are evolving, however, will help us implement a multi-faceted timed weed control strategy (i.e., varying tilling times and planting sites, varying water schedules, varying herbicide/pesticide treatments, etc.) that slows wild weed evolution. Furthermore, for existing weeds that have already employed a multi-faceted IWM strategy, periodically evaluating evolutionary rates of their weedy traits can support the efficacy of current IWM strategies and assess whether changes need to be made.
Research addressing the influence of the environment on the spread of crop-wild hybrid weeds is an important, preventative step in managing the risk of the evolution of crop-wild hybrid weeds. Our work evaluates the effect of watering on the rate of evolution of crop-wild hybrid weeds and, although our results suggest watering does not drive weedy trait evolution in crop-wild hybrids, it raises new questions on what facilitates the rate of evolution and success of hybrid radish documented in other environments (Campbell et al., 2006; Hegde et al., 2006; Campbell and Snow, 2007; Hovick et al., 2012; Teitel et al., 2016a). Although, research evaluating environmental variation on invasive potential can take time to properly test and evaluate, thorough research can be critical in creating and implementing effective IWM and policy. For example, to create IWM applicable across environments, assessing both wild and crop-wild hybrid genotypes in response to a range of abiotic and biotic selection forces and across a range of managed and unmanaged environments will be imperative. Alternatively, assessing multiple traits (particularly in connection to genetically modified crops) or non-canalized crop traits, if any, in these environments can make the difference in predicting and controlling invasive plant outbreaks and crop destruction. Finally, our work demonstrates only one of the many trajectories of crop-wild hybrid weed populations. Our research, more importantly, enacts the precautionary principle in environmental decision making, where precautionary measures should be taken even if results are not fully established or significant (Kriebel et al., 2001; Agriculture Agro-Food Canada, 2016).
Data Availability Statement
The datasets presented in this study can be found in online repositories. The names of the repository/repositories and accession number(s) can be found at: figshare https://doi.org/10.6084/m9.figshare.12893927.v1.
Author Contributions
LC designed the experiment. KS ran the data analysis with support from AL. KS and SS wrote the manuscript with support from LC and AL. KS, SS, and JB helped with experimental setup, data collection, and data analysis. All authors contributed to the article and approved the submitted version.
Funding
The authors gratefully acknowledge the funding support from the Natural Sciences and Engineering Research Council of Canada (NSERC) Discovery Grants program (No. 402305-2011 and 2019-05780 to LC), Ontario Graduate Scholarship (OGS), Queen Elizabeth II Graduate Scholarship (QEII), and the Faculty of Science Ryerson University (for a research fellowship to KS).
Conflict of Interest
The authors declare that the research was conducted in the absence of any commercial or financial relationships that could be construed as a potential conflict of interest.
Acknowledgments
We appreciate insights of A. Snow, K. Mercer, and A. Weis in the development of this study and thankful to H. Maherali, M.T. Arts, and S. Melles for helpful edits to the MS. Many students, too numerous to name, assisted in population maintenance and data collection between 2010 and 2016. The staff of the University of Toronto's Koffler Scientific Research provided logistical support.
Supplementary Material
The Supplementary Material for this article can be found online at: https://www.frontiersin.org/articles/10.3389/fagro.2020.600346/full#supplementary-material
References
Abdi, H., and Williams, L. J. (2010). Principal component analysis. WIREs Comp. Stat. 2, 433–459. doi: 10.1002/wics.101
Agriculture and Agro-Food Canada (2016). Reduced-Risk Strategy for Integrated Weed Management in Field Vegetables.
Alexander, M. (1969). Differential staining of aborted and nonaborted pollen. Stain Technol. 44, 117–122. doi: 10.3109/10520296909063335
Anderson, E., and Stebbins, G. L. Jr. (1954). Hybridization as an evolutionary stimulus. Evolution 8, 378–388. doi: 10.1111/j.1558-5646.1954.tb01504.x
Antonovics, G., and Bradshaw, A. (1970). Evolution in closely adjacent plant populations VIII. Clinal patterns at a mine boundary. Heredity 25:349. doi: 10.1038/hdy.1970.36
Arendt, J. D. (1997). Adaptive intrinsic growth rates: an integration across taxa. Q. Rev. Biol. 72, 149–177. doi: 10.1086/419764
Arnold, M. L., and Martin, N. H. (2010). Hybrid fitness across time and habitats. Trends Ecol. Evol. 25, 530–536. doi: 10.1016/j.tree.2010.06.005
Ashworth, M. B., Walsh, M. J., Flower, K. C., Vila-Aiub, M. M., and Powles, S. B. (2016). Directional selection for flowering time leads to adaptive evolution in Raphanus raphanistrum (Wild radish). Evol. Appl. 9, 619–629. doi: 10.1111/eva.12350
Baack, E. J., Sapir, Y., Chapman, M. A., Burke, J. M., and Rieseberg, L. H. (2008). Selection on domestication traits and quantitative trait loci in crop–wild sunflower hybrids. Mol. Ecol. 17, 666–677. doi: 10.1111/j.1365-294X.2007.03596.x
Bagavathiannan, M. V., and Van Acker, R. C. (2008). Crop ferality: implications for novel trait confinement. Agric. Ecosyst. Environ. 127, 1–6. doi: 10.1016/j.agee.2008.03.009
Baker, H. G. (1965). Characteristics and Modes of Origin of Weeds. New York, NY: Academic Press Inc., 147–172.
Beckie, H. J. (2006). Herbicide-resistant weeds: management tactics and practices. Weed Technol. 20, 793–814. Available online at: https://www.jstor.org/stable/4495755?seq=1
Beckie, H. J., Blackshaw, R. E., Low, R., Hall, L. M., Sauder, C. A., Martin, S., et al. (2013). Glyphosate-and acetolactate synthase inhibitor–resistant kochia (Kochia scoparia) in western Canada. Weed Sci. 61, 310–318. doi: 10.1614/WS-D-12-00140.1
Berger, J. D., Adhikari, K. N., Wilkinson, D., Buirchell, B. J., and Sweetingham, M. W. (2008). Ecogeography of the Old World lupins. 1. Ecotypic variation in yellow lupin (Lupinus luteus L.). Aust. J. Agric. Res. 59, 691–701. doi: 10.1071/AR07384
Berger, J. D., and Ludwig, C. (2014). Contrasting adaptive strategies to terminal drought-stress gradients in Mediterranean legumes: phenology, productivity, and water relations in wild and domesticated Lupinus luteus L. J. Exp. Bot. 65, 6219–6229. doi: 10.1093/jxb/eru006
Bock, D. G., Caseys, C., Cousens, R. D., Hahn, M. A., Heredia, S. M., Hübner, S., et al. (2015). What we still don't know about invasion genetics. Mol. Eco. 24, 2277–2297. doi: 10.1111/mec.13032
Bond, W., and Grundy, A. C. (2001). Non-chemical weed management in organic farming systems. Weed Res. 41, 383–405. doi: 10.1046/j.1365-3180.2001.00246.x
Bone, E., and Farres, A. (2001). Trends and rates of microevolution in plants. Genetica 112, 165–182. doi: 10.1023/A:1013378014069
Campbell, L. G., Parker, R. J., Blakelock, G., Pirimova, N., and Mercer, K. L. (2015). Maternal environment influences propagule pressure of an invasive plant, Raphanus raphanistrum (Brassicaceae). Int. J. Plant Sci. 176, 393–403. doi: 10.1086/680683
Campbell, L. G., Shukla, K., Sneck, M. E., Chaplin, C., and Mercer, K. L. (2016a). The effect of altered soil moisture on hybridization rate in a crop-wild system (Raphanus spp.). PLoS ONE. 11:166802. doi: 10.1371/journal.pone.0166802
Campbell, L. G., and Snow, A. A. (2007). Competition alters life history and increases the relative fecundity of crop-wild radish hybrids (Raphanus spp.). New Phytol. 173, 648–660. doi: 10.1111/j.1469-8137.2006.01941.x
Campbell, L. G., and Snow, A. A. (2009). Can feral weeds evolve from cultivated radish (Raphanus sativus, Brassicaceae)? Am. J. Bot. 96, 498–506. doi: 10.3732/ajb.0800054
Campbell, L. G., Snow, A. A., and Ridley, C. E. (2006). Weed evolution after crop gene introgression: Greater survival and fecundity of hybrids in a new environment. Ecol. Lett. 9, 1198–1209. doi: 10.1111/j.1461-0248.2006.00974.x
Campbell, L. G., Snow, A. A., and Sweeney, P. M. (2009b). When divergent life histories hybridize: Insights into adaptive life-history traits in an annual weed. New Phytol. 184, 806–818. doi: 10.1111/j.1469-8137.2009.03036.x
Campbell, L. G., Snow, A. A., Sweeney, P. M., and Ketner, J. M. (2009a). Rapid evolution in crop-weed hybrids under artificial selection for divergent life histories. Evol. App. 2, 172–186. doi: 10.1111/j.1752-4571.2008.00051.x
Campbell, L. G., Teitel, Z., and Miriti, M. N. (2016b). Contemporary evolution and the dynamics of invasion in crop-wild hybrids with heritable variation for two weedy life-histories. Evol. Appl. 9, 697–708. doi: 10.1111/eva.12366
Casper, B. B., and Jackson, R. B. (1997). Plant competition underground. Annu. Rev. Ecol. Syst. 28, 517–544. doi: 10.1146/annurev.ecolsys.28.1.545
Conner, J., and Via, S. (1993). Patterns of phenotypic and genetic correlations among morphological and life-history traits in wild radish, Raphanus raphanistrum. Evolution. 47, 704–711. doi: 10.2307/2410086
Davies, M. S., and Snaydon, R. W. (1976). Rapid population differentiation in a mosaic environment III. Measures of selection pressures. Heredity 36, 59–66. doi: 10.1038/hdy.1976.6
Dekker, J. (1997). Weed diversity and weed management. Weed Sci. 45, 357–363. doi: 10.1017/S0043174500092985
Délye, C., Menchari, Y., Michel, S., Cadet, É., and Le Corre, V. (2013). A new insight into arable weed adaptive evolution: mutations endowing herbicide resistance also affect germination dynamics and seedling emergence. Ann. Bot. 111, 681–691. doi: 10.1093/aob/mct018
Dlugosch, K. M., and Parker, I. M. (2008). Invading populations of an ornamental shrub show rapid life history evolution despite genetic bottlenecks. Ecol. Lett. 11, 701–709. doi: 10.1111/j.1461-0248.2008.01181.x
Ellstrand, N. C., and Hoffman, C. A. (1990). Hybridization as an avenue of escape for engineered genes. Bioscience 40, 438–442. doi: 10.2307/1311390
Ellstrand, N. C., and Marshall, D. L. (1985). The impact of domestication on distribution of allozyme variation within and among cultivars of radish, Raphanus sativus L. Theor. Appl. Genet. 69, 393–398. doi: 10.1007/BF00570908
Ellstrand, N. C., and Schierenbeck, K. A. (2006). Hybridization as a stimulus for the evolution of invasiveness in plants? Euphytica 97, 7043–7050. doi: 10.1073/pnas.97.13.7043
Flint-Garcia, S. A. (2013). Genetics and consequences of crop domestication. J Agr. Food Chem. 61, 8267–8276. doi: 10.1021/jf305511d
Franks, S. J., Hamann, E., and Weis, A. E. (2018). Using the resurrection approach to understand contemporary evolution in changing environments. Evol. Appl. 11, 17–28. doi: 10.1111/eva.12528
Gasser, C. S., and Fraley, R. T. (1989). Genetically engineering plants for crop improvement. Science 244, 1293–1299. doi: 10.1126/science.244.4910.1293
Germain, R. M., Caruso, C. M., and Maherali, H. (2013). Mechanisms and consequences of water stress–induced parental effects in an invasive annual grass. Int. J. Plant Sci. 174, 886–895. doi: 10.1086/670691
Gingerich, P. D. (1983). Rates of evolution: effects of time and temporal scaling. Science 222, 159–161. doi: 10.1126/science.222.4620.159
Gingerich, P. D. (1993). Quantification and comparison of evolutionary rates. Am. J. Sci. 293, 453–478. doi: 10.2475/ajs.293.A.453
Gingerich, P. D. (2001). Rates of evolution on the time scale of the evolutionary process. Genetica 8, 127–144. doi: 10.1023/A:1013311015886
Goldie, X., Gillman, L., Crisp, M., and Wright, S. (2010). Evolutionary speed limited by water in arid Australia. in Proc. Roy. Soc. B. 277, 2645–2653. doi: 10.1098/rspb.2010.0439
Gomulkiewicz, R., and Holt, R. D. (1995). When does evolution by natural selection prevent extinction? Evolution 49, 201–207. doi: 10.1111/j.1558-5646.1995.tb05971.x
Goodman, D. (1987). “The demography of chance extinction” in Viable Populations for Conservation, ed. M. E. Soulé (Cambridge: Cambridge University Press), 11–34. doi: 10.1017/CBO9780511623400.003
Government of Canada (2018). Historical Climate Data - Buttonville A in E. a. N. Resources, ed. Government of Canada.
Grime, J. P. (1977). Evidence for the existence of three primary strategies in plants and its relevance to ecological and evolutionary theory. Am. Nat. 111, 1169–1194. doi: 10.1086/283244
Haldane, J. B. S. (1949). Suggestions as to quantitative measurement of rates of evolution. Evolution 51–56. doi: 10.1111/j.1558-5646.1949.tb00004.x
Hartman, Y., Uwimana, B., Hooftman, D. A. P., Schranz, M. E., van de Wiel, C. C. M., Smulders, M. J. M., et al. (2013). Genomic and environmental selection patterns in two distinct lettuce crop-wild hybrid crosses. Evol. Appl. 6, 569–584. doi: 10.1111/eva.12043
Hastwell, G. T., and Panetta, F. D. (2005). Can differential responses to nutrients explain the success of environmental weeds? J. Veg. Sci. 16, 77–84. doi: 10.1111/j.1654-1103.2005.tb02340.x
Hegde, S. G., Nason, J. D., Clegg, J. M., and Ellstrand, N. C. (2006). The evolution of California's wild radish has resulted in the extinction of its progenitors. Evolution 60, 1187–1197. doi: 10.1554/05-634.1
Hendry, A. P., and Kinnison, M. T. (1999). The pace of modern life: measuring rates of micro-evolution. Evolution 53, 1637–1653. doi: 10.1111/j.1558-5646.1999.tb04550.x
Holm, L., Del, Y., Holm, E., and Herberger, T. (1997). World Weeds: Natural Histories and Distribution. New York, NY: John Wiley & Sons.
Hovick, S. M., Campbell, L. G., Snow, A. A., and Whitney, K. D. (2012). Hybridization alters early life-history traits and increases plant colonization success in a novel region. Am. Nat. 179, 192–203. doi: 10.1086/663684
Huang, Y., Zhao, X., Zhang, H., Huang, G., Luo, Y., and Japhet, W. (2009). A comparison of phenotypic plasticity between two species occupying different positions in a successional sequence. Ecol. Res. 24:1335. doi: 10.1007/s11284-009-0615-4
Husband, B. C., and Campbell, L. G. (2004). Population responses to novel environments: implications for ex situ plant conservation. in Ex situ plant conservation: supporting species survival in the wild, eds. E. O. Guerrant, Jr, K. Havens, and M. Maunder (Washington DC: Island Press), p. 231–266.
Irwin, Rebecca, E., and Strauss, Sharon, Y. (2005). Flower color microevolution in wild radish: evolutionary response to pollinator-mediated election. Am. Nat. 165, 225–237. doi: 10.1086/426714
Jump, A. S., Peñuelas, J., Rico, L., Ramallo, E., Estiarte, M., Martínez-Izquierdo, J. A., et al. (2008). Simulated climate change provokes rapid genetic change in the Mediterranean shrub Fumana thymifolia. Glob. Chang. Biol. 13, 1605–1615. doi: 10.1111/j.1365-2486.2007.01521.x
Kay, Q. (1976). Preferential pollination of yellow-flowered morphs of Raphanus raphanistrum by Pieris and Eristalis spp. Nature 261:230. doi: 10.1038/261230a0
Kiang, Y. T. (1982). Local differentiations of Anthoxanthum odoratum L. population on roadsides. Am. Midl. Nat. 107, 340–350. doi: 10.2307/2425384
Kost, M. A., Alexander, H. M., Jason Emry, D., and Mercer, K. L. (2015). Life history traits and phenotypic selection among sunflower crop-wild hybrids and their wild counterpart: implications for crop allele introgression. Evol. Appl. 8, 560–572. doi: 10.1111/eva.12261
Kriebel, D., Tickner, J., Epstein, P., Lemons, J., Levins, R., Loechler, E. L., et al. (2001). The precautionary principle in environmental science. Environ. Health Perspect. 109, 871–876. doi: 10.1289/ehp.01109871
Lande, R. (1993). Risks of population extinction from demographic and environmental stochasticity and random catastrophes. Am. Nat. 142, 911–927. doi: 10.1086/285580
Langevin, S. A., Clay, K., and Grace, J. B. (1990). The incidence and effects of hybridization between cultivated rice and its related weed red rice (Oryza sativa L.). Evolution 4, 1000–1008. doi: 10.1111/j.1558-5646.1990.tb03820.x
Lavergne, S., and Molofsky, J. (2007). Increased genetic variation and evolutionary potential drive the success of an invasive grass. Proc. Natl. Acad. Sci. U. S. A. 104, 3883–3888. doi: 10.1073/pnas.0607324104
Luke, S. G. (2017). Evaluating significance in linear mixed-effects models in R. Behav. Res. Methods. 49, 1494–1502. doi: 10.3758/s13428-016-0809-y
Macnair, M. R., Smith, S. E., and Cumbes, Q. J. (1993). Heritability and distribution of variation in degree of copper tolerance in Mimulus guttatus at Copperopolis, California. Heredity 71, 445–455. doi: 10.1038/hdy.1993.162
Mallory-Smith, C., Hendrickson, P., and Mueller-Warrant, G. (1999). Cross-resistance of primisulfuron-resistant Bromus tectorum L. (downy brome) to sulfosulfuron. Weed Sci. 47, 256–257. doi: 10.1017/S0043174500091736
Matesanz, S., Gianoli, E., and Valladares, F. (2010). Global change and the evolution of phenotypic plasticity in plants. Ann. NY Acad. Sci. 1206, 35–55. doi: 10.1111/j.1749-6632.2010.05704.x
Maxwell, K., and Johnson, G. N. (2000). Chlorophyll fluorescence—a practical guide. J. Exp. Bot. 51:659–668. doi: 10.1093/jexbot/51.345.659
Mercer, K. L., Andow, D. A., Wyse, D. L., and Shaw, R. G. (2007). Stress and domestication traits increase the relative fitness of crop–wild hybrids in sunflower. Ecol. Let. 10, 383–393. doi: 10.1111/j.1461-0248.2007.01029.x
Mohammed, S. B., Khalid, A., Osman, S. E. F., and Helali, R. G. M. (2016). Usage of Principal Component Analysis (PCA) in AI Applications. Khartoum, Sudan. IJERT. 5.
Monjardino, M., Pannell, D. J., and Powles, S. B. (2003). Multispecies resistance and integrated management: a bioeconomic model for integrated management of rigid ryegrass (Lolium rigidum) and wild radish (Raphanus raphanistrum). Weed Sci. 51, 798–809. doi: 10.1614/P2002-118
Nason, J. D., and Ellstrand, N. C. (1995). Lifetime estimates of biparental inbreeding depression in the self-incompatible annual plant Raphanus sativus. Evolution 49, 307–316. doi: 10.1111/j.1558-5646.1995.tb02243.x
Nicotra, A. B., Atkin, O. K., Bonser, S. P., Davidson, A. M., Finnegan, E., Mathesius, U., et al. (2010). Plant phenotypic plasticity in a changing climate. Trends Plant Sci. 15, 684–692. doi: 10.1016/j.tplants.2010.09.008
Oplaat, C., and Verhoeven, K. J. F. (2015). Range expansion in asexual dandelions: selection for general-purpose genotypes? J. Ecol. 103, 261–268. doi: 10.1111/1365-2745.12347
Ord, T. J., and Hundt, P. J. (2020). Crossing extreme habitat boundaries: jack-of-all-trades facilitates invasion but is eroded by adaptation to a master-of-one. Func. Ecol. 34, 1404–1415. doi: 10.1111/1365-2435.13600
Panetsos, C. A., and Baker, H. G. (1967). The origin of variation in “wild” Raphanus sativus (Cruciferae) in California. Genetica 38, 243–274. doi: 10.1007/BF01507462
Pichancourt, J.-B., and van Klinken, R. D. (2012). Phenotypic plasticity influences the size, shape and dynamics of the geographic distribution of an invasive plant. PLoS ONE 7:e32323. doi: 10.1371/journal.pone.0032323
Powles, S. B., Lorraine-Colwill, D. F., Dellow, J. J., and Preston, C. (1998). Evolved resistance to glyphosate in rigid ryegrass (Lolium rigidum) in Australia. Weed Sci. 46, 604–607. doi: 10.1017/S0043174500091165
Presotto, A., Pandolfo, C., Poverene, M., and Cantamutto, M. (2016). Can achene selection in sunflower crop–wild hybrids by pre-dispersal seed predators hasten the return to phenotypically wild sunflowers? Euphytica 208, 453–462. doi: 10.1007/s10681-015-1579-9
Richards, C. L., Bossdorf, O., Muth, N. Z., Gurevitch, J., and Pigliucci, M. (2006). Jack of all trades, master of some? On the role of phenotypic plasticity in plant invasions. Ecol. Lett. 9, 981–993. doi: 10.1111/j.1461-0248.2006.00950.x
Ridley, C. E., and Ellstrand, N. C. (2010). Rapid evolution of morphology and adaptive life history in the invasive California wild radish (Raphanus sativus) and the implications for management. Evol. App. 3, 64–76. doi: 10.1111/j.1752-4571.2009.00099.x
Rodgers, E. G. (1978). “Weeds and their control,” in Fundamentals of Plant-Pest Control, ed. D.A. Roberts (San Francisco, CA: W. H. Freeman and Co.), 164–168.
Rohde, K. (1992). Latitudinal gradients in species diversity: The search for the primary cause. Oikos. 65, 514–527. doi: 10.2307/3545569
Sadras, V., Reynolds, M., De la Vega, A., Petrie, P., and Robinson, R. (2009). Phenotypic plasticity of yield and phenology in wheat, sunflower and grapevine. Field Crop Res. 110, 242–250. doi: 10.1016/j.fcr.2008.09.004
Sahli, H. F., Conner, J. K., Shaw, F. H., Howe, S., and Lale, A. (2008). Adaptive differentiation of quantitative traits in the globally distributed weed, wild radish (Raphanus raphanistrum). Genetics 180, 945–955. doi: 10.1534/genetics.107.085084
Schierenbeck, K. A., and Ellstrand, N. C. (2009). Hybridization and the evolution of invasiveness in plants and other organisms. Biol. Invasions. 11:1093. doi: 10.1007/s10530-008-9388-x
Snaydon, R. W., and Davies, M. S. (1972). Rapid population differentiation in a mosaic environment. II. Morphological variation in Anthoxanthum odoratum. Evolution 26, 390–405. doi: 10.1111/j.1558-5646.1972.tb01944.x
Sneck, M. (2012). Evolutionary Responses to Global Change: An Experimental Test of the Effect of Altered Precipitation on Hybridization Rates in Sunflower (Helianthus). (Master Thesis). Rice University, Houston, TX, United States.
Snow, A. A. (2002). Transgenic crops—why gene flow matters. Nat. Biotechnol. 20, 542–542. doi: 10.1038/nbt0602-542
Snow, A. A., and Campbell, L. G. (2005). “Can feral radishes become weeds?” in Crop Ferality and Volunteerism, ed. J. B. Gressel (Florida, FL: CRC Press), 193-208.
Snow, A. A., Culley, T. M., Campbell, L. G., Sweeney, P. M., Hegde, S. G., and Ellstrand, N. C. (2010). Long-term persistence of crop alleles in weedy populations of wild radish (Raphanus raphanistrum). New Phytol. 186, 537–548. doi: 10.1111/j.1469-8137.2009.03172.x
Snow, A. A., Uthus, K. L., and Culley, T. M. (2001). Fitness of hybrids between weedy and cultivated radish: Implications for weed evolution. Ecol. Appl. 11, 999–1007. doi: 10.1890/1051-0761(2001)0110934:FOHBWA2.0.CO;2
Stanton, M. L., Snow, A. A., Handel, S. N., and Bereczky, J. (1989). The impact of a flower-color polymorphism on mating patterns in experimental populations of wild radish (Raphanus raphanistrum L.). Evolution 43, 335–346. doi: 10.1111/j.1558-5646.1989.tb04231.x
Strauss, S. Y., Irwin, R. E., and Lambrix, V. M. (2004). Optimal defence theory and flower petal colour predict variation in the secondary chemistry of wild radish. J. Ecol. 92, 132–141. doi: 10.1111/j.1365-2745.2004.00843.x
Swanton, C. J., and Weise, S. F. (1991). Integrated weed management: the rationale and approach. Weed Technol. 5, 657–663. doi: 10.1017/S0890037X00027512
Teitel, Z., Klimowski, A., and Campbell, L. G. (2016a). Assessing the effects of hybridization and precipitation on invasive weed demography using strength of selection on vital rates. BMC Evol. Bio. 16:266. doi: 10.1186/s12862-016-0833-7
Teitel, Z., Laursen, A. E., and Campbell, L. G. (2016b). Germination rates of weedy radish populations (Raphanus spp.) altered by crop-wild hybridisation, not human-mediated changes to soil moisture. Weed Res. 56, 149–158. doi: 10.1111/wre.12194
Valladares, F., Gianoli, E., and Gómez, J. M. (2007). Ecological limits to plant phenotypic plasticity. New Phytol. 176, 749–763. doi: 10.1111/j.1469-8137.2007.02275.x
Via, S., and Lande, R. (1985). Genotype-environment interaction and the evolution of phenotypic plasticity. Evolution 39, 505–522. doi: 10.1111/j.1558-5646.1985.tb00391.x
Vigueira, C. C., Olsen, K. M., and Caicedo, A. L. (2013). The red queen in the corn: agricultural weeds as models of rapid adaptive evolution. Heredity 110, 303–311. doi: 10.1038/hdy.2012.104
Ward, J. K., Antonovics, J., Thomas, R. B., and Strain, B. R. (2000). Is atmospheric CO2 a selective agent on model C3 annuals? Oecologia 123, 330–341. doi: 10.1007/s004420051019
Warwick, S. I., and Francis, A. (2005). The biology of Canadian weeds. 132. Raphanus raphanistrum L. Can. J. Plant Sci. 85, 709–733. doi: 10.4141/P04-120
Warwick, S. I., Phillips, D., and Andrews, C. (1986). Rhizome depth: the critical factor in winter survival of Sorghum halepense (L.) Pers. (Johnson grass). Weed Res. 26, 381–388. doi: 10.1111/j.1365-3180.1986.tb00721.x
Weinig, C. (2000). Plasticity versus canalization: population differences in the timing of shade-avoidance responses. Evolution 54, 441–451. doi: 10.1111/j.0014-3820.2000.tb00047.x
Whitney, K. D., Ahern, J. R., and Campbell, L. G. (2009). Hybridization-prone plant families do not generate more invasive species. Biol. Invasions. 11, 1205–1215. doi: 10.1007/s10530-008-9390-3
Whitney, K. D., and Gabler, C. A. (2008). Rapid evolution in introduced species, “invasive traits” and recipient communities: challenges for predicting invasive potential. Divers Distrib. 14, 569–580. doi: 10.1111/j.1472-4642.2008.00473.x
Whitney, K. D., Randell, R. A., and Rieseberg, L. H. (2006). Adaptive introgression of herbivore resistance traits in the weedv sunflower Helianthus annuus. Am. Nat. 167, 794–807 doi: 10.1086/504606
Wolf, J. B., and Wade, M. J. (2009). What are maternal effects (and what are they not)? Phil. T. Roy. Soc. B 364, 1107–1115. doi: 10.1098/rstb.2008.0238
Wright, S. (1968). Evolution and the Genetics of Populations. Vol. 1. Genetic and Biométrie Foundations. London and Chicago: University of Chicago Press.
Wright, S., Keeling, J., and Gillman, L. (2006). The road from Santa Rosalia: a faster tempo of evolution in tropical climates. Proc. Natl. Acad. Sci. U. S. A. 103, 7718–7722. doi: 10.1073/pnas.0510383103
Keywords: evolutionary divergence rates, haldanes, crop-wild hybrids, integrated weed management, soil moisture
Citation: Shukla K, Sbrizzi S, Laursen AE, Benavides J and Campbell LG (2020) Hybridization Slows Rate of Evolution in Crop-Wild Compared to Wild Populations of Weedy Raphanus Across a Moisture Gradient. Front. Agron. 2:600346. doi: 10.3389/fagro.2020.600346
Received: 29 August 2020; Accepted: 09 November 2020;
Published: 03 December 2020.
Edited by:
Karla Leigh Gage, Southern Illinois University Carbondale, United StatesReviewed by:
Carolina Zamorano-Montañez, University of Caldas, ColombiaHui Xia, Shanghai Agrobiological Gene Center, China
Alejandro Presotto, National University of the South, Argentina
Copyright © 2020 Shukla, Sbrizzi, Laursen, Benavides and Campbell. This is an open-access article distributed under the terms of the Creative Commons Attribution License (CC BY). The use, distribution or reproduction in other forums is permitted, provided the original author(s) and the copyright owner(s) are credited and that the original publication in this journal is cited, in accordance with accepted academic practice. No use, distribution or reproduction is permitted which does not comply with these terms.
*Correspondence: Kruti Shukla, a3J1dGkuc2h1a2xhQHJ5ZXJzb24uY2E=