- 1Department of Plant and Wildlife Sciences, Brigham Young University, Provo, UT, United States
- 2Departamento de Recursos Naturales, Universidad Autónoma Agraria Antonio Narro, Saltillo Coahuila, Mexico
- 3Department of Anthropology, Brigham Young University, Provo, UT, United States
- 4Department of Animal and Rangeland Sciences, College of Agricultural Sciences, Oregon State University, Corvallis, OR, United States
- 5Instituto Nacional de Investigaciones Forestales, Agrícolas y Pecuarias (INIFAP), Campo Experimental Costa de Hermosillo, Sonora, Mexico
- 6División de Ciencias Forestales, Departamento de Estadística, Matemática y Cómputo, Universidad Autónoma Chapingo, Texcoco, Mexico
- 7Floriculture Research Division, National Institute of Horticultural and Herbal Sciences, Rural Development Administration, Wanju-gun, South Korea
Cultivation of C3 and C4 crops in semi-arid regions will be severely constrained as global temperatures rise. Consequently, alternative crops need to be sought out that adapt well to heat and drought and are productive despite limited access to water. Traits, such as crassulacean acid metabolism (CAM), enable economically important species such as those in the Agave genus adapt to drought and high temperatures. The succulence and high efficiency of agaves, which enables them to produce biomass with little water, underscores their feasibility as an alternative crop for semi-arid regions, such as the Sonoran Desert in the southwestern U.S. In this paper, we offer a review of the suitability for cultivation of agaves via dryland farming, particularly by rock mulching techniques used by pre-Columbian, Sonoran Desert farmers. This analysis dovetails with information also provided on the biological traits of Agave and its historical and present utilization. Pre-Columbian, Hohokam dryland farmers used rock mulching in the form of rock piles to cultivate agaves. Rock piles acted as a type of mulch to harvest rainfall and to retain soil moisture, which allowed the Hohokam to intensively cultivate agaves during multi-year droughts. Remains of Hohokam rock mulching for agave production can be found at archaeological sites in central Arizona, which provides evidence of the utility of dryland farming and ancient agricultural innovation to reconcile water scarcity in the region. Moreover, the use of rock piles likely bolstered Agave productivity in marginal lands. Although little is known of historic rock mulching to cultivate agaves and its biological implications on plant productivity we suggest its application as a dryland farming model could be a sustainable strategy in the U.S. Southwest.
Introduction
Increasingly limited access to water and elevating temperatures will continue to hamper productivity of conventional C3 and C4 crops in arid and semi-arid regions throughout the world in the coming decades (Porter and Semenov, 2005; De Micco and Aronne, 2012; Zandalinas et al., 2018). Contemporary agricultural challenges, limited water availability, and rising temperatures have constrained agriculture in dry regions throughout history (Ingram, 2010). Within the last 1,300 years, global warming has been associated with overexploitation of natural resources, increased urbanization, and accelerated agricultural development (Woodhouse et al., 2010). Models of changes in global temperature suggest that induced anthropogenic global warming became more frequent during the Medieval Warm Period, which occurred between 700 and 1300 CE (Galloway, 1986; Hughes and Diaz, 1994; Bradley et al., 2003; Stinchcomb et al., 2011). The Medieval Warm Period increased temperatures and reduced water levels of lakes and rivers throughout Europe, Asia, and North and South America (Van West and Dean, 2000; Chu et al., 2002; Sridhar et al., 2006; Helama et al., 2009; Woodhouse et al., 2010). In addition to the Medieval Warm Period, some have hypothesized that global temperatures rose and rainfall patterns progressively changed in arid regions during the pre-industrial period 800–1850 A.D. due to land-use changes for agriculture (e.g., conversion of forests and grasslands into cropland) (Galloway, 1986; Reick et al., 2010; Pongratz and Caldeira, 2012).
After the Industrial Revolution, the use of fossil fuels and concomitant increases in CO2 emissions accelerated climate change (Callendar, 1938; Revelle and Suess, 1957; Neftel et al., 1985; Lemonnier and Ainsworth, 2018), increasing global temperatures and the occurrence of droughts during the twentieth century (Hansen et al., 1981; Solomon et al., 2010; Smith et al., 2019). At the end of the last century and beginning of the twenty-first century, globalized industrial development and creation of large urban centers resulted in cropland expansion in arid and semi-arid regions to meet increased food-production demands (Krausmann et al., 2013; Laurance et al., 2014). Conversely, relatively warmer temperatures in the early part of the twenty-first century, high evapotranspiration rates, erratic rainfall, and increasingly severe droughts have deleterious consequences to farmland by reducing crop yields, resulting in an increase of marginal lands (i.e., farmland and wildlands with limited access to irrigation water and depleted soil nutrients) (Schlaepfer et al., 2017). These increasingly warm and dry conditions in regions with limited resources suggest future edaphic, biological, and climatic constraints for cultivation of C3 and C4 crops. Such conditions increase the need for seeking, selecting, and cultivating drought-tolerant crops, such as those found in the succulent Agave genus, which cope with drought through nocturnal CO2 fixation and CAM photosynthesis (Borland et al., 2009, 2015; Stewart, 2015).
Current challenges in dry regions to cultivate and produce food in hot and water-limited conditions bear similarity to those that native people faced long ago during severe droughts in what is now the U.S. Southwest (Ingram, 2010). Irrigation water has always been a naturally limited resource in arid regions (Troyo-Diéguez et al., 1990). For dry regions, there is a need for sustainable agricultural strategies to optimize crop yields and irrigation water (Troyo-Diéguez et al., 1990). To cope with scarce availability of water, innovative, indigenous dry-farming strategies were developed anciently to produce food during droughts (Lightfoot, 1996). These dryland farmers irrigated with rainfall runoff by optimizing rainwater catchment and rewetting the landscape using manmade stone features, such as rock terraces and rock mulch (Wilken, 1972; Lightfoot, 1994, 1996). The indelible signature left by the historic use of rock terraces and rock mulching can be seen in ancient and modern societies in dry regions throughout the world. For example, in the Negev Desert of the Middle East, the nomadic Nabateans, who settled in the region around 600-300 BCE, built and used rock terraces, check dams, and rock mulching to irrigate and catch rainfall water (Stager, 1976; Evenari et al., 1982; Lightfoot, 1994; Ashkenazi et al., 2012). At the apex of Nabatean civilization, such terraces became the main dry-farming technology to cultivate olives (Olea europaea), pomegranates (Punica granathum), and apples (Malus domestica) (Ynnilä, 2007). Similar examples can also be found in ancient civilizations throughout the deserts of Africa, Europe, and Asia (Wilken, 1972; Lightfoot, 1994; Biazin et al., 2012). In the ancient Americas, rock-farming techniques were used in a variety of cultures and time periods (Marcus, 2006; Kennett, 2012). In the Andean region, from the times of the Huarpa civilization to that of the Incan (200 BCE to 1400 CE), rock-wall terraces were heavily relied on to cultivate potatoes (Solanum tuberosum), quinoa (Chenopodium quinoa), and corn (Zea mays) (Denevan, 2003; Chapagain and Raizada, 2017). Mayans in southern Mesoamerica were very effective in cultivating corn using rock terraces (Turner, 1976; Fischbeck, 2001; Webb et al., 2004). In central and northern Mesoamerica, Aztecs cultivated marginal lands with corn and agaves in a system called milpas (Evans, 1990; Zizumbo-Villarreal et al., 2012; Trombold, 2017). Ancient Pacific Islanders used rock mulching to harvest rainwater and to cultivate perennial crops, such as taro (Colocasia esculenta), in land with limited access to water (Stevenson et al., 1999; Wozniak, 1999; Ladefoged et al., 2013). Pre-Columbian Hohokam people, which inhabited the deserts of the American Southwest, also cultivated drought-tolerant agaves in marginal lands using rock-mulching (Fish et al., 1985; Fish and Fish, 1990, 1992; Gasser and Kwiatkowski, 1991).
Among historic dry-farming examples in the U.S., Hohokam agricultural dryland systems in central and southern Arizona are key to understanding applications of dry farming for other arid regions affected by drought. The Hohokam mastered desert farming (Fish and Fish, 1992). Their dry-farming techniques were adapted and designed to produce food in extended droughts and in the harsh Sonoran Desert climate. They implemented rock-mulching to catch rainfall water and successfully cultivate agaves to feed thousands of desert dwellers during water scarcity periods (Fish and Fish, 1990). Rock mulching turned into the primary strategy to shore up food production during droughts. Agave was the main crop that allowed for unabated cultural, social, and economic development in the region (Fish, 2000). As in the prehistoric past, modern central and southern Arizona is a region constrained by the harsh Sonoran Desert climate. Here the applications of indigenous dry-farming agriculture, principally Hohokam rock mulching, opens the possibility for cultivating agaves in current and future droughts. It is our intent to portray Agave as a drought-tolerant crop, which can be cultivated through the application of rock mulching to harvest rainwater as a feasible and sustainable dry-land agriculture system for arid regions. The purpose of this paper is to summarize literature available on (1) studies on the ecophysiology of agaves under drought conditions, (2) dry farming using rock mulching to cultivate agaves, (3) ancient and modern-day uses of agaves, and (4) the potential of rock mulching and Agave cultivation in future droughts.
Biological Traits of Agaves Key for Its Cultivation in Future Droughts
Nearly 75% of the continental biological diversity of the Agave genus can be found in Mexico and 13% in U.S. deserts (Gentry, 1982; Garcia-Moya et al., 2011). The Agave genus evolved biological and morphological traits that enable species to adapt to erratic, hot, and drought-changing conditions of arid regions (Silva-Montellano and Eguiarte, 2003). Morphological traits of agaves, such as their shallow root systems, distinct rosette shape, and curved leaves to maximize rainfall interception, evolved to efficiently use small amounts of atmospheric and soil moisture in water-limited environments (Martorell and Ezcurra, 2007). Such limited water and heat conditions negatively affect the physiological performance of domesticated C3 and C4 crops (Nobel and Jordan, 1983). Crassulacean acid metabolism (CAM) photosynthesis is the main biological trait that drives productivity of these plants in hot and water-scarce conditions (Lüttge, 2004; Borland et al., 2011). Photosynthesis of agaves relies on nocturnal stomatal opening and CO2 gas exchange as a strategy to avoid high evapotranspiration rates and leaf water loss during daylight hours (Lüttge, 2004). Nocturnal CO2 fixation is the primary trait agaves use to survive dry climates and to adapt to warm temperatures (Borland et al., 2009). In addition, above and belowground morphological traits (North and Nobel, 1991), such as leaf succulence, rain-hair roots, and fibrous root architecture enable agaves to adjust physiological processes to available soil-moisture levels and heat in the different seasons of dry regions (De Micco and Aronne, 2012).
Agaves are monocarpic plants with a long life cycle to maturation (Nobel, 1977). Differences in plant maturation can be observed within and between species, regions, cultivation practices, and degree of domestication (e.g., domesticated agaves, hybrids of agaves, or wild agaves) (Zizumbo-Villarreal et al., 2013). Generally, cultivated agaves require a few years or up to a decade to mature to flower, and typically more than a decade to mature to flower in the wild (Cervantes et al., 2007; Núñez et al., 2008).
Aboveground morphological traits of agaves (e.g., shape, size of leaves, and succulence) enable these plants to survive and adapt to deserts by providing protection and storing water in the leaf parenchyma (Orians and Solbrig, 1977; Cervantes et al., 2007; Núñez et al., 2008). Additionally, the rosette arrangement of the curved Agave leaves funnel rainwater to the plant and soil during the summer monsoon season, re-wetting their rhizomes and the soil in the root zone (Gentry, 1982). Furthermore, Martorell and Ezcurra (2007) hypothesized that the rosette trait can also trap atmospheric moisture in the form of dew and fog between leaves. The thick succulent leaves of agaves function as plant water storage for periods of scarce rain and soil moisture. Even after a period of several months, when soil moisture has reached the permanent wilting point, agaves will remain physiologically functional (Nobel, 2003).
The Agave root system is composed of shallow roots (mean root length: 8 to 20 cm) and rhizomes (Arizaga and Ezcurra, 2002; Nobel, 2003; Bautista-Cruz et al., 2007). Offset growth from rhizomes and aerial bulbils act as the main asexual propagation strategy of agaves. Such offsets can extend several meters from the plant in search of soil moisture (Gibson, 1996; Nobel, 2003). Shallow roots allow rapid soil moisture absorption from the soil surface, particularly from small amounts of moisture deposited after light rain events (North and Nobel, 1991). Fibrous Agave root systems maximize soil water absorption, particularly in well-drained sandy soils with limited capacity to retain moisture (Cervantes et al., 2007). In addition, in very dry soils, dehydration of suberized peridermal cells of mature Agave roots prevents water loss and desiccation of the root vascular system (North and Nobel, 1991). Additionally, these lignified roots anchor Agave plants to the soil. When rainfall occurs, water pulses from rain rehydrate Agave roots, which promotes emergence of new root hairs, thereby increasing hydraulic conductance of Agave root systems (Palta and Nobel, 1989). Rainwater stimulates growth of ephemeral root hairs, which are vital for rapid water uptake and replenishing of water in Agave leaves (North and Nobel, 1991; Huang and Nobel, 1992).
Wild and cultivated agaves flourish in arid environments and poor soils in marginal lands (Gentry, 1972; Cervantes et al., 2007; Núñez et al., 2008). Edaphic requirements include sandy soils with good drainage, 60% gravel content, and deep water tables (Cervantes et al., 2007). Particularly sandy loam soils with low salinity contents are optimum for healthy establishment of agaves. Agaves can be found growing in rocky soils in which temperatures can reach 70°C (Gentry, 1972; Nobel, 1994). Agaves typically perform well in soils with low nutrient content. Nitrogen levels in soils range between 31 and 35 parts per million (ppm) and low P between 2.6 and 3.0 ppm for optimal growth of agaves (Cervantes et al., 2007). Ideal growing conditions for agaves can be found in low-elevation mesic areas on hillslopes. In the Sonoran Desert of northwestern Mexico and southern Arizona, optimum elevation ranges for Agave growth have been observed between 800 and 1200 m above sea level (Gentry, 1972; Nobel and Hartsock, 1986; Núñez et al., 2008; Parker et al., 2014; Hodgson et al., 2019). However, agaves can also be found in the coastal areas of the Sonoran Desert in Mexico (Gentry, 1972; Cervantes et al., 2007; Núñez et al., 2008). Ideal precipitation levels for agaves vary from tropical to dry regions (Gentry, 1982; Nobel, 2003). In arid regions, such as Sonora, Mexico and Arizona, USA, agaves can survive rainless seasons for several years (Nobel, 2003). Some regions with wild populations of Agave receive as little as 7 mm of rain and other regions receive as much as 762 mm or more of annual precipitation (Gentry, 1972, 1982; Nobel, 1976).
Cultivation of Agaves Using Rock-Mulching by the Hohokam
Who Were the Hohokam?
The Hohokam were pre-Columbian dryland farmers that established a flourishing civilization in what is now central and southern Arizona between 450 and 1500 C.E (Fish and Fish, 2008). Hohokam agriculture was constrained by the hot, dry climate, and the wide expanse of marginal lands in the Sonoran Desert (Fish and Fish, 1990). Drought and water availability for agriculture acted as definitive factors that influenced innovation in the agricultural and cultural development of the Hohokam (Rice, 1998; Hunt et al., 2005). These two factors shaped Hohokam irrigation and dryland agriculture in the desert, leading to the use of extensive irrigation-canal networks and rock mulching to cultivate agaves during periods of drought with erratic rainfall (Woodbury, 1961; Fish and Fish, 2012). Such approaches can be compared with dryland-farming systems observed in other advanced, prehistoric indigenous societies of Mesoamerica and South America (Doolittle, 1995; Fish and Fish, 2008). The irrigation canals of the Hohokam were similar to highly engineered Andean and Aztec irrigation-canal systems in that they were a pivotal factor in their cultural development and were designed to efficiently irrigate large areas of farmland, which led to substantial food production (Armillas, 1948; Bennett, 1948; Mitchell, 1973).
Irrigated crops formed the basis of Hohokam civilization and its economy. Efficiently distributed irrigation water from rivers and canals allowed large settlements to develop along the main rivers in the Phoenix Basin (Doyel, 2007). However, recurrent droughts at the beginning of the second millennia CE triggered periods of unstable food production, which changed agricultural strategies and the crops they cultivated (Fish and Fish, 1990). The Hohokam shifted to using more dryland farming and reliance upon rainfall for crop irrigation, resulting in less canal irrigation in the region.
During this period of recurring droughts, agaves were adopted as a crop to compensate for yield deficits during water shortages and as a supplement to irrigated annual crops (Fish and Fish, 1992, 2008; Anderies et al., 2008). The Hohokam primarily used rock piles to cultivate agaves, which enhanced their productivity during drought periods in the Sonoran Desert (Figure 1; Fish et al., 1985; Fish and Fish, 2012).
Dryland Farming Using Rock-Mulching to Cultivate Agaves
In the American Southwest, as in pre-Hispanic northern Mexico, the Hohokam implemented dryland agriculture strategies to cultivate Agave to ensure food security during droughts in the Tucson Basin (Fish et al., 1985; Anderies et al., 2008). A model of pre-Hispanic Agave cultivation by Anderies et al. (2008) suggests that Agave dryland farming was likely a strategy implemented by pre-Columbian groups to cope with climates with drought that reduced corn yields in the Sonoran Desert. The Hohokam adopted upland dryland farming on hillslopes using rock piles, terraces, and check dams to cope with low precipitation in the region (Fish and Fish, 1992). These structures allowed for the efficient use of rainwater to irrigate downhill floodplain crops and riparian vegetation (Fish and Fish, 1990). Rock piles and terraces were used to harvest rainfall runoff and to cultivate agaves (Fish and Fish, 1992).
Historical remains of Hohokam rock piles and evidence of Agave cultivation and processing in roasting pits can be found at archaeological sites outside the Tucson, Arizona area in the Tortolita Mountains; the Salt Gila Basin; the community of Marana; Tonto National Forest; San Pedro Valley; and Tumamoc Hill Reserve (Figure 2; Masse, 1979; Crown, 1987; Ciolek-Torrello et al., 1997; Adams and Adams, 1998). One of the most representative Hohokam rock-pile fields, which was found in Marana and characterized by Fish and Fish (1992), consisted of at least 42,000 rock piles nested with 120,000 m2 of terraces and check dams within an area of 500 ha. They calculated that the rock pile fields could have annually produced 102,000 Agave plants with an average yield of 40.8 Mg ha−1. However, comparing average planting density (i.e., 1,000–3,000 plants ha−1) of agaves (Cervantes et al., 2007; Núñez et al., 2008) in modern plantations in the Sonoran Desert in Mexico, with the rock-pile fields found in Marana, Arizona suggests that Agave productivity in Arizona was possibly higher than previously calculated by Fish et al. (1985) (i.e., 102,000 Agave plants in 500 hectares). For example, between 1000-3000 Agave angustifolia plants ha−1 can be annually cultivated in Sonora, Mexico in grasslands with no irrigation (Cervantes et al., 2007). Similarly, McDaniel (1985) suggested a planting density of 2,000–2,500 of Agave americana plants ha−1 cultivated in grassland in southern Arizona. If the calculations of Cervantes et al. (2007) and McDaniel (1985) regarding Agave cultivation area and planting density are applied to the largest Hohokam rock-pile field in Marana, Arizona, the minimum planting density would be 1,000 plants ha−1 for 500 ha of rock-pile fields. As such, the Hohokam potentially had the capacity to cultivate nearly 500,000 agaves, which is at least five times more plants than previously estimated by Fish et al. (1985). However, commercial modern Agave cultivation differs from the cultivation strategies of the Hohokam. This example is only used to highlight the productive potential of the land to cultivate agaves in the region.
Hohokam Agaves
Agave plant remains in Hohokam rock-pile fields underscore the importance of rock mulch for modern cultivation of agaves in the region (Fish et al., 1985; Fish and Fish, 1990; Fish, 2000). Though agaves were no longer cultivated prior to the arrival of Europeans to the region, agaves still can be found growing in some rock-pile fields and archaeological sites in Arizona (Hodgson and Salywon, 2013; Hodgson et al., 2019). Minnis and Plog (1976) observed a relationship between the occurrence of wild Agave parryi plants with proximity and distribution of agaves growing at archaeological sites in the Apache-Sitgreaves National Forest, suggesting putative historic cultivation of this Agave species in central Arizona. Similarly, Parker et al. (2010) observed genetic differences between putative cultigens of A. parryi and wild A. parryi plants at archaeological sites in central Arizona in the Mogollon Rim. Recently, a taxon, which was named Agave sanpedroensis, was discovered growing only in a rock-pile field west of Tucson, which is likely a relic of Hohokam cultivation (Hodgson et al., 2019). Living plants and dried tissue of Agave at rock piles and roasting pits have been found in Hohokam rock-pile fields at archaeological sites in southern and central Arizona (Fish et al., 1985; Adams and Adams, 1998; Fish, 2000; Parker et al., 2007; Fish and Fish, 2012).
Little is known about cultivation of agaves using rock piles outside of Arizona. Minnis et al. (2006) found little evidence of rock piles around the prehistoric archaeological site of Casas Grandes in Chihuahua, Mexico. However, ethnobotanists and archaeologists that visited archaeological sites near Casas Grandes in 2018 and 2019 found agaves growing in ancient rock piles and terraces, perhaps indicating ancient cultivation similar to that found in Arizona (W. Hodgson and M. Searcy, personal communication). In 2018, putative hybrids of Agave palmeri and A. parryi were found growing in a rock terrace in the Casas Grandes region in northern Mexico (W. Hodgson, personal communication). Likewise, in 2019, Agave hybrids, which were similar in appearance to those discovered in 2018 were growing in rock piles (M. Searcy, personal communication) (Figure 3). These recent findings suggest that cultivation of agaves using rock structures was likely more widespread than originally assumed. Such discoveries offer new avenues of research in the use of rock piles for prehistoric Agave cultivation.
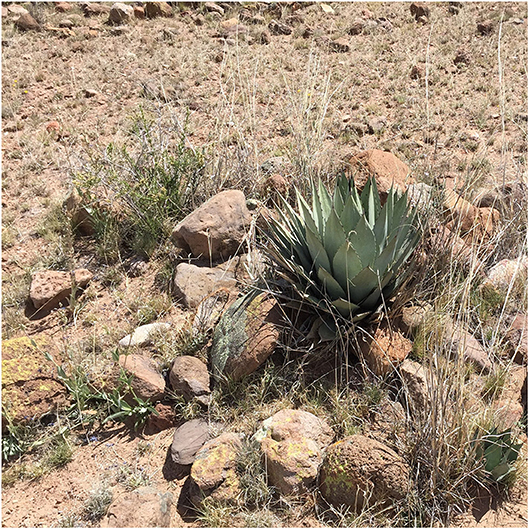
Figure 3. Agave growing in ancient rock pile at archaeological site in Casas Grandes, Chihuahua, Mexico (picture courtesy of M. Searcy, 2019).
Agave Traditional Uses Throughout History, Food, Drinks and Other Sub-Products
Uses in Ancient Times for Food and Fermented Drinks
Indigenous people in Mexico and the U.S. Southwest have used agaves as a source of carbohydrates and fiber for the past 10,000 years (Delgado-Lemus et al., 2014). Diversity in the Agave genus is largely concentrated in Mexico and the U.S. Southwest, and was cultivated mostly using dryland farming techniques, such as rock mulching using rainwater runoff (Lightfoot, 1994, 1996), widely throughout tropical and arid regions of Central and South America (Good-Avila et al., 2006). Beginning in pre-Columbian times, Agave was used for various purposes in what is now the U.S. Southwest, including beverages, ceremonial items, fiber-based products (e.g., clothing, footwear, containers, cordage, nets, etc.), food, medicine, and paint (Castetter et al., 1938). Over the span of several centuries, particularly during droughts, agaves were an important energy source that enriched the diets of indigenous people (Fish et al., 1985; Evans, 1990; Fish and Fish, 1990; Anderies et al., 2008).
The Hohokam were one of the few pre-Columbian indigenous groups in the U.S. Southwest that extensively used rock piles to cultivate agaves as a staple crop that ensured a reliable source of food, even during droughts (Fish et al., 1985; Dobyns, 1988; Fish and Fish, 1992). The Hohokam relied on the ability of these plants to concentrate sugars in stems and stalks through their long phenological cycle. Sugars in Agave are inulin-type polymers of fructose that concentrate in leaves, stems, and inflorescence stalks (Mancilla-Margalli and López, 2006; Urias-Silvas et al., 2008). However, removal of Agave stalks at the end of their life cycle induces sugar accumulation predominantly in the stem (Hodgson, 2001; Cervantes et al., 2007; Michel-Cuello et al., 2008). Inflorescence stalk emergence indicates plants have matured, and are ready to be harvested (Arizaga and Ezcurra, 2002).
Pre-Columbian indigenous people used roasting pits, also called earth ovens, to cook their food, but particularly to roast agaves (Walton, 1977; Fish et al., 1985; Cervantes et al., 2007; Perry and Flannery, 2007; Zizumbo-Villarreal et al., 2009). Roasting pits can reach temperatures between 150 and 200°C (Cervantes et al., 2007). Roasting Agave heads (or caudices) at this temperature enables thermal hydrolysis to break down carbohydrate polymers into sugar monomers, such as fructose and glucose, which are relatively easy to digest and ferment by yeast (Cervantes et al., 2007), making it possible to use agaves as a food source. Methods of cooking Agave heads using earth ovens share similarities (e.g., shape, diameter of 1.20–2.40 m, depth of 0.80–2.10 m) between indigenous groups across various regions, both in ancient and modern times (Cervantes et al., 2007; Towell and Lecón, 2010).
The practice of roasting agaves can be traced to its origins in pre-Columbian archaeological sites in central Arizona and northern Mexico (Fish et al., 1985). In Arizona, Agave roasting pits also can be found in rock pile-fields (Fish and Fish, 1992). These roasting pits attest to the ancient use of roasted agaves and their cultivation in rock piles in the region. In addition, several documents from the Spanish colonial period recorded historic uses of roasted agaves by natives. Early colonial Jesuits from Spain, in what is now northwestern Mexico, recorded that agaves were used for medicinal purposes and were roasted for food by the Opata people in the Sonoran Desert (Gutiérrez-Coronado et al., 2007; Flores and Araiza, 2012). Similarly, in what is now central Mexico in the mid-sixteenth century, colonial Spaniards documented medicinal uses of roasted Agave by the Aztec people in the ethnobotanical compendium Codex Florentino (Williams, 1990; Díaz et al., 1993). Uses of roasted and fermented agaves for food were also recorded in the Codex Azcatitlan and Codex Boturini (Morán, 2008).
Modern Use of Agave as a Food Source
As indicated above, out of all the organs of agaves, the stem head produces the most edible biomass (Nobel, 2003). Inflorescences, leaves, and stalks can also be used for food and to feed cattle (Bos primigenius taurus), sheep (Ovis aries), and goats (Capra aegagrus hircus) (Gentry, 1972; Pinos-Rodríguez et al., 2006, 2008, 2009; Hartung, 2016; Mellado, 2016). Gentry (1972) indicated that inflorescences of some Agave species are edible. Moreover, Fuentes-Rodriguez (1997) and Gentry (1972) suggest that the raw leaves remaining after clipping leaves from Agave stems, commonly called jimado in Spanish, can be used to feed cattle. Pinos-Rodríguez et al. (2006) found that leaves, flowering stalk, and bagasse of Agave salmiana can be used as food and to increase body weight of sheep. In popular Mexican cuisine throughout the country, Agave leaves are also used to cover goat or lamb stew while being cooked in underground roasting pits.
Alcoholic Spirits and Drinks From Agaves
Aguamiel, pulque, and mezcal constitute the main beverages produced from agaves (Stewart, 2015). In order to produce aguamiel, an emerging inflorescence is cut out of the stem head. Agave sap, which is rich in sugars, accumulates in the remaining basin. The sap juice is subsequently siphoned out of the basin and prepared as non-alcoholic drink known as aguamiel. Fermentation of aguamiel creates pulque (Rivas, 1991), a commonly consumed, mildly alcoholic beverage in rural areas of central and southern Mexico (Enríquez-Salazar et al., 2017).
It has been estimated that pulque made of Agave mapisaga, A. americana, Agave atrovirens, or Agave salmiana, was consumed in approximately 2000 B.C. (Escalante et al., 2016). Although distilled Agave spirits like Tequila or mezcals are very popular in modern times, artisanal crafting and consumption of pulque remains alive in some regions of central Mexico.
Although drinks, such as aguamiel and pulque, are still consumed in modern times, these beverages have been somewhat replaced by distilled alcoholic drinks made from distillation of fermented sap of agaves (Garay and Aurea, 2008). Distillation technologies, such as the use of copper alembic stills to distill alcohol, were adapted to produce Agave spirits in Mexico by the Spaniards in the 1500s (Gutiérrez-Coronado et al., 2007; Towell and Lecón, 2010). In combination with a wide array of distillation methods, the diversity of Agave species in the different regions and the various cooking and fermentation methods of Agave heads employed by tribes across Mexico enabled a rich diversification of Agave spirits throughout Mexico from various species (Walton, 1977).
Tequila is the most popular Agave spirit crafted in Mexico, and differs from commercial mezcal in that it is made exclusively from Agave tequilana var. Azul, which is also known as blue agave (Colunga-GarcíaMarín and Zizumbo-Villarreal, 2006; Vargas-Ponce et al., 2007). In contrast, mezcals are made from a wide diversity of agaves across Mexico. In addition, similar to some French wines, tequila has an appellation of origin (denominación de origen), which requires that blue Agave plants only be grown in certain states of Mexico that are believed to enhance the quality of tequila (Bowen, 2015). Tequila is mainly produced at an industrial scale following quality-control regulations compliant with national and international standards established by the Tequila Regulatory Council (Macías, 2001).
Despite climate change and political and economic changes throughout Mexican history, the tequila industry has experienced continual growth. For example, Walton (1977) reported 1.67 million liters of tequila were produced in 1960. Based on statistical data of total production of tequila from the Tequila Regulatory Council (2019), peak tequila production occurred in 2018 which coincided the highest production level ever reached over the past 23 years. In 1995, 104.3 million liters of tequila were produced, but increased to 309.1 million liters in 2018. Similarly, the Tequila Regulatory Council (2019) recorded that global consumption of tequila increased from 279 thousand tons in 1995 to 1,139 thousand tons in 2018. The Secretariat of Agriculture Livestock, Rural Development, Fisheries and Food (SAGARPA) in Mexico reported in 2017 that tequila, relative to mezcal, is the major product from Agave in an expansive growth phase in Mexico. Outside of Mexico, tequila is consumed mainly in the United States, Germany, Spain, France, and the United Kingdom. In addition, SAGARPA (2017) reported that the tequila industry generated approximately $27 million dollars from export revenue, which is predicted to increase to $28 million dollars by 2024 and $29 million by 2030. The mezcal industry has also experienced sustained growth from about 2.5 million liters in 1950 to about 20 million liters in 2010 (Martínez Salvador et al., 2012).
Sweeteners and Syrups
Fructose sugars extracted from blue agave have also been used as alternative sweeteners (Heyer and Crawford, 2009; Stewart, 2015). Despite disadvantages of a relatively long life cycle and the monocarpic habit of agaves compared with other annual and perennial crops used in the sugar industry, Agave sugars are used as high-quality sweeteners. This emerging product can potentially work as a companion to the tequila industry. As with Agave spirits, the sweetener industry uses Agave juices as feedstock (Heyer and Crawford, 2009; Narváez-Zapata and Sánchez-Teyer, 2010). Sugars from Agave juice, particularly fructose, are extracted through acid or enzymatic hydrolysis (Garcia-Aguirre et al., 2009; Ávila-Fernández et al., 2011; Soto et al., 2011). The fructose sugars are used as additives in commercial Agave syrups, which are considered healthier sweeteners compared with sugar cane and high-fructose corn syrup (Hooshmand et al., 2014). The proportion of fructose in Agave syrup is significantly higher compared with the proportions in cane sugar and high-fructose corn syrup. Proportions of fructose to glucose are 50/50 in cane sugar (Glasziou, 1961); 55/45 in high fructose corn syrup (O'Brien-Nabors, 2001); and as high as 95/5 in Agave syrup (Garcia-Aguirre et al., 2009).
Modern Uses of Agave Fibers
Historically, Agave sisalana, Agave fourcroydes and Agave lechuguilla fibers have been used in the Mexican textile industry. Traditional uses of Agave fiber include ropes, twine, bags, mecapales, fabrics, brushes, and brooms (Colunga-GarcíaMarín and May-Pat, 1993; Kicińska-Jakubowska et al., 2012). Sisal is a hard fiber processed from the leaves of A. sisalana. Henequen fiber from A. fourcroydes and Tampico fiber (also called Mexican fiber) from A. lechuguilla have similar tensile and flexural properties as sisal fiber (Belmares et al., 1981; Kicińska-Jakubowska et al., 2012). More recently, Agave fibers have been used to reinforce industrial products, adding flexibility and strength to polymer-based composites (Joseph et al., 1999; Silva et al., 2010; Orue et al., 2016). Fiber-reinforced polymers have application in the aerospace, marine, automotive, military, and construction industries (Yilmaz and Arifuzzaman Khan, 2019). Compounds derived from Agave fibers, can also be used in synthetic drug manufacturing (Cushman et al., 2015). Steroidal saponins, tigogenin, and hecogenin are natural compounds extracted from A. sisalana leaves, which are used in the synthesis of steroidal hormones such as corticosteroids (Cripps and Blunden, 1978; Santos and Branco, 2014). Corticosteroids drugs like dexamethasone can be synthesized from tigogenin and hecogenin (Kongkathip et al., 1997; Santos and Branco, 2014) and may have application in treating respiratory-inflammatory conditions associated with COVID-19 produced by 2 SARS-CoV-2 (Al Saleh et al., 2020; McIntosh, 2020; Zhang et al., 2020).
Potential Uses of Agaves for Bioenergy
Agaves have also been proposed as a biofuel crop due to their relatively low lignin content (Somerville et al., 2010; Davis et al., 2011). The high lignin content of C3 and C4 biofuel crops reduces the efficiency of converting sugars into bioethanol (Somerville et al., 2010). Lignin percentages in agaves range between 3 and 15% (Iñiguez-Covarrubias et al., 2001; Li et al., 2012; Delfín-Ruíz et al., 2019), and can be more efficiently processed to produce sugars than C4 crops used in the biofuel industry (Somerville et al., 2010).
Many crops used in the biofuel industry require high amounts of irrigation water, generating controversy related to their environmental footprint (Somerville, 2007; Moore et al., 2014). Agaves require much less water than C4 crops, such as corn, to produce biomass, and the superior quality of ethanol derived from Agave compared with corn makes agaves an attractive alternative (Yan et al., 2011). In addition to the low lignin content of Agave, CAM metabolism enables their growth in marginal lands and resilience to drought (Davis et al., 2011).
One challenge in the widespread use of agaves in the biofuel industry is the lack of agronomic knowledge for its cultivation, as well as the underlying ecology and climatic conditions of regions where this crop may be suitable (McDaniel, 1985). Lewis et al. (2015) suggested the U.S. Southwest, particularly Arizona, as one region for Agave cultivation for the biofuel industry, due to its suitable climate. Another constraint on Agave production involves the large amount of annual plant biomass needed to supply enough raw material to make it profitably sustainable as a bioenergy crop (Balan, 2014).
According to Escamilla-Treviño (2012), commercial cultivation of agaves for biofuel in the U.S. has been constrained mainly by the risk of low-temperature crop damage. For example, cultivation of species with a frost tolerance between −2 and −4°C, such as A. tequilana, A. fourcroydes, A. angustifolia, A. salmiana, and A. sisalana (Nobel, 2003), could be limited even in Arizona, where nocturnal low temperatures below 0°C occur throughout the winter season. Agaves could be genetically engineered to improve traits, which would allow for better adaptation from temperate to xeric environments, which would enable agaves to be widely cultivated in marginal environments in the U.S. (Yang et al., 2016). Another approach could be to use Agave species that have relatively wide cold tolerance, such as A. americana and Agave utahensis, which have been reported to adapt well to cold and hot temperatures in the region and can tolerate temperatures between −8 and −11°C (Nobel and Jordan, 1983; Escamilla-Treviño, 2012; Davis et al., 2017). Moreover, species putatively cultivated by the Hohokam in pre-Colombian times, including A. palmeri, A. murpheyi, A. parryi, and A. sanpedroensis, could potentially be used as crops in the future because they are endemic and well-adapted to hot summers, cold winters, and the dry climate of the Sonoran Desert (Adams and Adams, 1998; Parker et al., 2010; Hodgson and Salywon, 2013; Fish and Fish, 2014; Hodgson et al., 2019).
Rock Piles to Cultivate Agaves in Marginal Lands
Rock Piles as Dryland Farming System for Agaves in Marginal Lands
Agave rock-pile fields are a cultural and agricultural legacy of the ancient Hohokam tribe in the Sonoran Desert (Hodgson et al., 2019). Rock piles are specialized features associated with the ancient practice of Agave cultivation in marginal lands (Dobyns, 1988; Fish and Fish, 1990, 1992, 2012, 2014; Sandor and Homburg, 2017). Because CAM metabolism enables agaves to grow well in water-limited environments and in nutrient-poor soils (Gentry, 1982; Nobel and Valenzuela, 1987; Nobel, 1991, 2003; Garcia-Moya et al., 2011), Agave was and is a well-suited crop for marginal lands. Prehistoric groups from central and southern Arizona lived in marginal lands with limited access to irrigation water (Fish and Fish, 1992). Rainfall was the primary source of water to irrigate agaves in rock piles. Rainwater in rock-pile fields was harvested in two ways: through the interception of rainfall hitting rocks and from rain-water runoff (Crown, 1987; Fish and Fish, 1992; Lightfoot, 1994, 1996).
Rock piles were built on downhill slopes such that the inclined angle mitigated downward runoff and enabled rock piles to reduce erosion and increase fertility of Agave fields (Fish and Fish, 1992; Sandor and Homburg, 2011). In such rock piles, rainwater generally flows along soil slopes, and upon intercepting rock piles, it slows down, leading to increased soil moisture beneath the rock piles (Fish and Fish, 1990, 1992; Homburg and Sandor, 2011). The reduced flow of rainwater leads to less gully formation. Likewise, sediments and minerals were mixed in the rainfall runoff and deposited underneath rocks. According to Homburg and Sandor (2011), the accumulation of minerals under rock piles improved the texture of the soil-surface horizons and increased soil moisture retention capacity. The minerals, sediments, and organic matter deposited below rock piles were a source of C, N, and P, which provided a source of soil fertility for agaves cultivated by ancient indigenous groups, such as the Hohokam.
Agave Species Likely Cultivated by the Hohokam in Rock Piles
Evidence of cultivation of different Agave species in rock piles includes plant tissue, such as spines and fibers at nearby roasting pits and artifacts found in rock-pile fields, which were likely used to process and harvest agaves, such as tabular knives and scrapers (Cantley, 1991; Fish and Fish, 1992; Ciolek-Torrello et al., 1997). Among Agave species native to Arizona, A. murpheyi and A. sanpedroensis have been recognized as species that were cultivated in rock piles by the Hohokam (Adams and Adams, 1998; Parker et al., 2007; Hodgson et al., 2019). Moreover, researchers have identified Agave yavapaiensis, Agave verdensis, and Agave delamateri as pre-Columbian Agave cultigens (Parker et al., 2007; Hodgson and Salywon, 2013). Similarly, A. parryi has been associated with archaeological sites and dryland farming in Arizona (Minnis and Plog, 1976; Parker et al., 2010, 2014). Evidence of other cultivated plants used by the Hohokam include pollen grains of corn and cotton, which were found in rock piles (Crown, 1987; Fish, 1988; Bohrer, 1991). Other native species, such as Opuntia spp., Carnegia gigantea, Chenopodium spp., Amaranthus spp., Trianthema portulacastrum, Spharalcea ambigua, Boerhaavia spp., and cholla (Cylindropuntia fulgida) have been found to populate rock-pile fields in archaeological sites (Fish et al., 1986; Crown, 1987; Bohrer, 1991; Hodgson et al., 2019).
Potential Benefits of Using Rock Piles to Cultivate Agaves
Rock Pile Fields for Agaves and Their Environments
While rock piles can be found at archaeological sites throughout the southwestern U.S. and northwestern Mexico, most are located in south-central Arizona (Fish et al., 1985; Fish and Fish, 1990, 1992), providing an ideal setting for modern Agave cultivation that could incorporate aspects of prehistoric rock-pile fields. Rainfall and temperatures at rock-pile fields in Marana, Tucson, and San Pedro Valley, Arizona, whose elevations range between 600 and 900 m above sea level, suggest that Hohokam agaves were cultivated in an optimum environment that balanced temperature, rainfall, and soil moisture (Fish and Fish, 1990, 1992; Cantley, 1991; Hodgson et al., 2019). This unique balance likely maximized productivity of agaves, even in the dry and harsh conditions of the region.
Different studies using the environmental productivity index developed by Nobel for agaves (Nobel and Hartsock, 1986; Nobel and Quero, 1986; Nobel and Valenzuela, 1987; Garcia-Moya et al., 2011) found, in general, that mesic environments, such as archaeological sites with Hohokam rock piles, can lead to improved Agave productivity. The index indicates that CO2 uptake and productivity of agaves is greater at elevations between 600 and 1200 m above sea level. In addition, Woodhouse et al. (1980) found that agaves are less productive when cultivated on steep slopes. Hohokam rock-pile fields occur more frequently on softly inclined slopes, which have higher rainfall moisture interception, and less negative soil water potentials than found on relatively steeper slopes (Cantley, 1991; Fish and Fish, 1992). Such conditions possibly promoted better interception of photosynthetic radiation and rainfall, which would have led to enhanced CAM photosynthesis and biomass of agaves. Understanding Hohokam rock pile field environments can help to identify potential locations to cultivate agaves, even during severe drought events. However, more information needs to be sought out to determine the agricultural limitations and future applications of rock piles in modern times.
Soil-Water Dynamics Under Rock Piles
Hohokam rock piles functioned as a type of mulching that reduced soil evapotranspiration and used rainwater to increase soil moisture content underneath rocks (Doelle, 1978; Fish et al., 1985; Fish and Fish, 1990, 1992; Lightfoot, 1996). The positive effects of available moisture in agaves have been observed in different experiments. In an experiment with A. deserti, Jordan and Nobel (1979) observed that rainfall and soil moisture act as the most important factors influencing plant mortality in their first year of establishment. They also found that rainfall stimulated increased succulence, increased leaf growth, and helped modulate nocturnal CO2 gas exchange and water-use efficiency of first-year plants. Davis et al. (2017) found that irrigation increased efficiency of nocturnal CO2 uptake of A. americana. Similarly, Nobel et al. (1989) observed that irrigation doubled CO2 uptake of Agave lechuguilla and enhanced aerial and root biomass. Lightfoot (1996) and Sandor and Homburg (2011) hypothesized that the moisture harvested underneath rock piles from rainwater improved cultivation of crops, including Agave, in rock piles (Fish and Fish, 2014). In an experiment using agaves in rock piles conducted in different locations in central and southern Arizona, Fish and Fish (2014) found that seasonal rains replenished soil moisture below rock piles, which improved survival rates of A. murpheyi and A. americana. Nobel et al. (1992b) reported that after watering rocks with 10–30 mL of water, soil volumetric water content increased below rocks for a period ranging between 13 and 19 days, leading to increased nocturnal CO2 uptake of A. deserti.
While moisture underneath rock piles likely enhanced Agave biomass productivity, Eickmeier and Adams (1978) indicated that available water and air temperature are the two most influential factors that affect Agave carbon assimilation. Although day-night temperature is an important factor in Agave nocturnal CO2 uptake, as observed with A. angustifolia and A. americana (Holtum and Winter, 2014), soil moisture governs biomass productivity of agaves (Huang and Nobel, 1992). Nobel and Quero (1986) indicated that available soil moisture in summer and fall in the Sonoran and Chihuahuan Deserts acts as the driving factor that stimulates biomass of Agave plants by promoting emergence of new leaves, development of large aerial shoots, and enhancement of root hydraulic conductance. In addition, Nobel (1976) observed that leaf size and soil water content correlated with higher nocturnal CO2 uptake of A. deserti. However, the benefits of increasing soil moisture by using rock piles needs to be further explored through additional lab and field experiments.
Soil Temperature Underneath Rock Piles
Cool temperatures below rock piles can reduce heat stress and desiccation of roots of Agave plants (Huang and Nobel, 1992). Since daily soil temperatures in the Sonoran Desert can reach 75°C (Nobel, 2003), rock piles can reduce soil-moisture evaporation rates (Sandor and Homburg, 2011), and also work as a barrier to reduce interception of solar radiation, which leads to cooler diurnal soil temperatures relative to that found in exposed soil (Wilken, 1972). Studies made on the thermal properties of rock piles in A. deserti and A. americana illustrate the advantages of rock piles as insulation to diurnal hot temperatures. Palta and Nobel (1989) observed that low soil temperatures underneath rocks positively affected A. deserti root respiration and reduced root dryness. Nobel et al. (1992b) measured A. deserti roots underneath boulders or rock fragments and compared roots of agaves growing in exposed soils and found that low temperatures and less-negative soil water potentials underneath rocks increased root number, thickness, and length.
Kaseke et al. (2012) found that convective heat transference of rock mulch can keep soils cooler during the day and increase nocturnal soil temperatures. However, since little is known regarding patterns of diurnal and nocturnal temperatures underneath and within Hohokam rock piles, characterization of such properties is necessary in similarly arranged rock piles. In addition, experimentation is needed to characterize nocturnal convective heat transference underneath rock piles, and the level of nocturnal CO2 uptake of agaves in rock piles.
Insulative properties of rocks and their effect on temperatures below rock piles likely have a positive effect on symbiosis of microbes with agaves (Cui and Nobel, 1992). A study on the effects of soil temperatures on vesicular-arbuscular mycorrhizae infection found that soil temperatures around 25°C increased yields of Sorghum bicolor and Triticum aestivum due to high colonization of roots by mycorrhizae (Fabig et al., 1989). Little is known, however, about the effect of Hohokam rock-pile temperatures on the soil microbiome and their associated benefits. Nevertheless, the Agave rhizosphere is diverse in prokaryotic and fungal microorganisms, and is correlated with the hosting capability of agaves and their adaptions to arid climates (Coleman-Derr et al., 2016). Symbiosis of Agave roots with soil microbes enhances root hydraulic conductance and nutrient uptake, particularly solubilizing P. Cui and Nobel (1992) observed that colonization of A. deserti with mycorrhizae improved hydraulic conductance, uptake of P in roots, and P allocation in leaves. In addition, colonization of mycorrhizae positively correlated with enhanced CO2 uptake of A. deserti. Plant symbiosis with arbuscular mycorrhizae, ecto-mycorrhizae, ericoid mycorrhizae, and various bacteria contributes to increased uptake of N and P in the form of phosphates (Mensah et al., 2015). In a study where endophytic bacteria were isolated from the base of A. tequilana plants, Martínez-Rodríguez et al. (2014) identified the presence of 300 strains of bacteria with different capacities and benefits, such as N fixation, P solubilization, auxin production, and antagonism against Fusarium oxysporum.
Soil-Based Nutrients Underneath Rock Piles
Agaves in the wild are well-adapted to arid regions and generally perform adequately in rocky, nutrient-poor soils (Gentry, 1972, 1982). However, soil-based nutrients underneath rock piles (Homburg and Sandor, 2011; Sandor and Homburg, 2017) can bolster Agave primary productivity (Nobel et al., 1992a). Soil research by Homburg and Sandor (2011) suggests that the use of Hohokam rock piles enriched C, N, and available P to agaves due to organic-matter accumulation. Soil nutrient accumulation underneath Hohokam rock piles possibly occurred due to runoff, microbial decomposition of organic matter, or soil bioturbation. The available nutrients below rock piles likely enhanced the physiological response of agaves to drought and extreme temperatures, and improved growth and productivity. However, it is necessary to assess the dynamics between soil-based nutrients underneath rock piles with Agave nutrient assimilation to more fully understand the benefits afforded by rock piles to Agave productivity. In addition, agricultural parameters, such as soil pH levels, soil electric conductivity, and nutrient cycling in the soil beneath rock piles, need future research.
Nutrients in the soil under rock piles, as observed by Homburg and Sandor (2011), contributed to the productivity of cultivated agaves. Available nutrients in the soil assist in the productivity of agaves (Nobel et al., 1992a). Nobel et al. (1988) observed that fertilization with N, P, K, and B enhanced growth and nocturnal CO2 uptake of A. lechuguilla. Similarly, irrigation, in combination with fertilization with N, P, and K, increased foliar leaf area, leaf number, and concentration of sugars, particularly fructose and glucose in A. tequilana and Agave potatorum (Martínez et al., 2012; Zúñiga-Estrada et al., 2018). Valenzuela and Gonzalez (1995) found that fertilization of A. lechuguilla and A. tequilana with P and N increased leaf area. Similarly, for A. deserti, Nobel et al. (1989) observed that fertilization promoted leaf growth and high rates of CO2 uptake, which led to high biomass accumulation.
Opportunity for Researching Agaves in Rock Piles
Relict rock piles at archaeological sites represent a valuable agricultural example of how the Hohokam made marginal lands productive by cultivating agaves during severe droughts. While a number of archaeologists reported that rock piles were the main dryland-farming strategy used by the Hohokam to cultivate agaves (Masse, 1979; Fish et al., 1985; Crown, 1987; Dobyns, 1988; Fish and Fish, 1990, 1992; Cantley, 1991; Lightfoot, 1996; Sandor and Homburg, 2011), little is known about the agronomic potential and applications of rock piles in modern Agave cultivation. To bring to light possible uses of rock piles, it is necessary to sort through what has been published regarding the environmental details of rock-pile fields in order to experimentally replicate these ancient agroecosystems.
Experiments that assess cultivation, pest management, and the physiological responses of agaves using rock piles are needed, particularly to observe plant productivity, CO2 uptake, temperature requirements, and soil-plant water relations in rock piles. In addition, characterizing the hydrothermal properties of rock piles requires examining how they can preserve soil moisture and modulate soil temperatures. The microbiome and fauna of rock piles are additional factors that could potentially enhance nutrition, improve water status, and contribute to general plant health. Microbiomes in rock piles can positively impact plant health of agaves in future droughts, particularly in preventing pests and disease. Future research is needed on the environmental, social, and economic impacts of using rock piles to cultivate agave, particularly in the continually changing and fragile agroecosystems of dry regions.
Conclusions
Further experimentation and development of innovative agricultural strategies is crucial to the use of agaves as a crop under scenarios of severe drought and global warming. Throughout history, agaves have been used as commodities for food (Anderies et al., 2008; Delgado-Lemus et al., 2014), beverages (Walton, 1977; Stewart, 2015; Escalante et al., 2016), and fiber (Colunga-GarcíaMarín and May-Pat, 1993). In modern times, agaves continue to be used for such purposes, but are now also used as substrates for sweeteners (Heyer and Crawford, 2009; Stewart, 2015), biofuels (Somerville et al., 2010; Davis et al., 2011), synthetic drugs (Santos and Branco, 2014; Cushman et al., 2015) and industrial materials (Silva et al., 2010; Orue et al., 2016). We suggest dryland farming of Agave as a means to minimize the use of irrigation water and sustainably maintain crop productivity in arid regions.
Current challenges to successful cultivation of agaves, such as low rainfall and excessive heat in arid regions and marginal lands, are similar to those that prehistoric, indigenous farmers faced during droughts in the Sonoran Desert. The pre-Columbian Hohokam were skilled in the use of rock piles to cultivate agaves during droughts (Dobyns, 1988; Fish and Fish, 1992). These rock piles acted as a mulch that harvested rainwater moisture, preserved soil moisture, reduced soil evapotranspiration, and insulated soil in their immediate environs. Despite the lack of empirical data, moisture harvested during the monsoon season beneath and around rock piles likely decreased drought stress, stimulating biomass productivity of agaves.
The use of rock piles for Agave cultivation promises ecological benefits, such as minimizing soil erosion and maximizing crop productivity in marginal lands with minimal input of chemical fertilization and pesticides
Rock pile cultivation of agaves is promising, but it requires field-based research to characterize their productive potential. More research is also needed to understand how the Hohokam rock-pile system could be used to cultivate crops other than agaves. However, with even from the little that is known, rock piles provide a sustainable crop-production-technology alternative for efficient use of water in dry areas and to revive cultivation of agaves in limited-resource environments in the region.
Author Contributions
This manuscript is a collaborative effort of different individuals. The manuscript was written by HO-C and JS. The following collaborators JH-H, NH, SP, MS, RM-G, TC-M, AV-M, and PP provided valuable feedback and reviewed the final version of the manuscript. All authors contributed to the article and approved the submitted version.
Funding
Funding was provided through in-house university funds. Funding was provided through, Wildlife and Wildlans Conservations, PhD program, Department of Wildlife and Wildlife Sciences, Brigham Young University.
Conflict of Interest
The authors declare that the research was conducted in the absence of any commercial or financial relationships that could be construed as a potential conflict of interest.
Acknowledgments
We express our sincere gratitude to all the collaborators and reviewers that made this paper possible. We would like to extend a special thank you to Paul and Suzanne Fish and Wendy Hodgson for their encouragement and for sharing their knowledge with us about Hohokam Agave cultivation.
References
Adams, K. R., and Adams, R. K. (1998). How Does Our Agave Grow? Reproductive Biology of a Suspected Ancient Arizona Cultivar, Agave murpheyi Gibson. CALS Publ. Arch. Univ. Ariz. Available online at: https://repository.arizona.edu/handle/10150/554320 (accessed October 11, 2019).
Al Saleh, A. S., Sher, T., and Gertz, M. A. (2020). Multiple myeloma in the time of COVID-19. Acta Haematol. 143, 1–7. doi: 10.1159/000507690
Anderies, J. M., Nelson, B. A., and Kinzig, A. P. (2008). Analyzing the impact of agave cultivation on famine risk in arid pre-Hispanic northern Mexico. Hum. Ecol. 36, 409–422. doi: 10.1007/s10745-008-9162-9
Arizaga, S., and Ezcurra, E. (2002). Propagation mechanisms in Agave macroacantha (Agavaceae), a tropical arid-land succulent rosette. Am. J. Bot. 89, 632–641. doi: 10.3732/ajb.89.4.632
Armillas, P. (1948). A sequence of cultural development in Meso-America. Mem. Soc. Am. Archaeol. 4, 105–111. doi: 10.1017/S0081130000000423
Ashkenazi, E., Avni, Y., and Avni, G. (2012). A comprehensive characterization of ancient desert agricultural systems in the Negev Highlands of Israel. J. Arid Environ. 86, 55–64. doi: 10.1016/j.jaridenv.2012.02.020
Ávila-Fernández, Á., Galicia-Lagunas, N., Rodríguez-Alegría, M. E., Olvera, C., and López-Munguía, A. (2011). Production of functional oligosaccharides through limited acid hydrolysis of agave fructans. Food Chem. 129, 380–386. doi: 10.1016/j.foodchem.2011.04.088
Balan, V. (2014). Current challenges in commercially producing biofuels from lignocellulosic biomass. ISRN Biotechnol. 2014, 463074–463074. doi: 10.1155/2014/463074
Bautista-Cruz, A., Carrillo-González, R., Arnaud-Viñas, M. R., Robles, C., and de León-González, F. (2007). Soil fertility properties on Agave angustifolia Haw. plantations. Soil Tillage Res. 96, 342–349. doi: 10.1016/j.still.2007.08.001
Belmares, H., Barrera, A., Castillo, E., Verheugen, E., Monjaras, M., Patfoort, G. A., et al. (1981). New composite materials from natural hard fibers. Ind. Eng. Chem. Prod. Res. Dev. 20, 555–561. doi: 10.1021/i300003a026
Bennett, W. C. (1948). The Peruvian co-tradition. Mem. Soc. Am. Archaeol. 4, 1–7. doi: 10.1017/S0081130000000289
Biazin, B., Sterk, G., Temesgen, M., Abdulkedir, A., and Stroosnijder, L. (2012). Rainwater harvesting and management in rainfed agricultural systems in sub-Saharan Africa–a review. Phys. Chem. Earth Parts ABC 47, 139–151. doi: 10.1016/j.pce.2011.08.015
Bohrer, V. L. (1991). Recently recognized cultivated and encouraged plants among the Hohokam. Kiva 56, 227–235. doi: 10.1080/00231940.1991.11758169
Borland, A. M., Barrera Zambrano, V. A., Ceusters, J., and Shorrock, K. (2011). The photosynthetic plasticity of crassulacean acid metabolism: an evolutionary innovation for sustainable productivity in a changing world. New Phytol. 191, 619–633. doi: 10.1111/j.1469-8137.2011.03781.x
Borland, A. M., Griffiths, H., Hartwell, J., and Smith, J. A. C. (2009). Exploiting the potential of plants with crassulacean acid metabolism for bioenergy production on marginal lands. J. Exp. Bot. 60, 2879–2896. doi: 10.1093/jxb/erp118
Borland, A. M., Wullschleger, S. D., Weston, D. J., Hartwell, J., Tuskan, G. A., Yang, X., et al. (2015). Climate-resilient agroforestry: physiological responses to climate change and engineering of crassulacean acid metabolism (CAM) as a mitigation strategy. Plant Cell Environ. 38, 1833–1849. doi: 10.1111/pce.12479
Bowen, S. (2015). Divided Spirits: Tequila, Mezcal, and the Politics of Production. Oakland, CA: Univ of California Press. doi: 10.1525/california/9780520281042.001.0001
Bradley, R. S., Hughes, M. K., and Diaz, H. F. (2003). Climate in medieval time. Science 302, 404–405. doi: 10.1126/science.1090372
Callendar, G. S. (1938). The artificial production of carbon dioxide and its influence on temperature. Q. J. R. Meteorol. Soc. 64, 223–240. doi: 10.1002/qj.49706427503
Cantley, G. J. (1991). Archaeology of a Rockpile Field in the Santan Mountains, Arizona. MA thesis, Phoenix, AZ, Arizona State University.
Castetter, E. F., Bell, W. H., and Grove, A. R. (1938). The Early Utilization and the Distribution of Agave in the American Southwest. Albuquerque: University of New Mexico Press.
Cervantes, M., T, Armenta-Calderón, A. D., and Sánchez-Arellano, J. G. (2007). El Cultivo del Maguey Bacanora (Agave angustifolia Haw.) en la Sierra de Sonora. Hermosillo: Instituto Nacional de Investigaciones Forestales, Agrícolas y Pecuarias (INIFAP).
Chapagain, T., and Raizada, M. N. (2017). Agronomic challenges and opportunities for smallholder terrace agriculture in developing countries. Front. Plant Sci. 8:331. doi: 10.3389/fpls.2017.00331
Chu, G., Liu, J., Sun, Q., Lu, H., Gu, Z., Wang, W., et al. (2002). The ‘Medieval Warm Period’ drought recorded in Lake Huguangyan, tropical South China. Holocene 12, 511–516. doi: 10.1191/0959683602hl566ft
Ciolek-Torrello, R., Homburg, J. A., and Sandor, J. (1997). “Vanishing River Volume 2: Agricultural, Subsistence, and Environmental Studies: Part 2: Chapters 4-7,” in Vanishing River: Landscapes and Lives of the Lower Verde Valley: The Lower Verde Archaeological Project: Volume 2: Agricultural, Subsistence, and Environmental Studies. (Tucson, AZ: Statistical Research, Inc. Press), 57–147.
Coleman-Derr, D., Desgarennes, D., Fonseca-Garcia, C., Gross, S., Clingenpeel, S., Woyke, T., et al. (2016). Plant compartment and biogeography affect microbiome composition in cultivated and native Agave species. New Phytol. 209, 798–811. doi: 10.1111/nph.13697
Colunga-GarcíaMarín, P., and May-Pat, F. (1993). Agave studies in Yucatan, Mexico. I. Past and present germplasm diversity and uses. Econ. Bot. 47, 312–327. doi: 10.1007/BF02862301
Colunga-GarcíaMarín, P., and Zizumbo-Villarreal, D. (2006). “Tequila and other agave spirits from west-central Mexico: current germplasm diversity, conservation and origin,” in Plant Conservation and Biodiversity (Dordrecht: Springer), 79–93. doi: 10.1007/978-1-4020-6444-9_6
Cripps, A. L., and Blunden, G. (1978). A quantitative gas-liquid chromatographic method for the estimation of hecogenin and tigogenin in the leaves, juice and sapogenin concentrates of agave sisalana. Steroids 31, 661–669. doi: 10.1016/S0039-128X(78)80006-3
Crown, P. L. (1987). Classic period Hohokam settlement and land use in the Casa Grande Ruins area, Arizona. J. Field Archaeol. 14, 147–162. doi: 10.1179/009346987792208466
Cui, M., and Nobel, P. S. (1992). Nutrient status, water uptake and gas exchange for three desert succulents infected with mycorrhizal fungi. New Phytol. 122, 643–649. doi: 10.1111/j.1469-8137.1992.tb00092.x
Cushman, J. C., Davis, S. C., Yang, X., and Borland, A. M. (2015). Development and use of bioenergy feedstocks for semi-arid and arid lands. J. Exp. Bot. 66, 4177–4193. doi: 10.1093/jxb/erv087
Davis, S. C., Dohleman, F. G., and Long, S. P. (2011). The global potential for agave as a biofuel feedstock. GCB Bioenergy 3, 68–78. doi: 10.1111/j.1757-1707.2010.01077.x
Davis, S. C., Kuzmick, E. R., Niechayev, N., and Hunsaker, D. J. (2017). Productivity and water use efficiency of Agave americana in the first field trial as bioenergy feedstock on arid lands. GCB Bioenergy 9, 314–325. doi: 10.1111/gcbb.12324
De Micco, V., and Aronne, G. (2012). “Morpho-anatomical traits for plant adaptation to drought,” in Plant Responses to Drought Stress (Springer), 37–61. doi: 10.1007/978-3-642-32653-0
Delfín-Ruíz, M. E., Calderón-Santoyo, M., Ragazzo-Sánchez, J. A., Gómez-Rodríguez, J., López-Zamora, L., and Aguilar-Uscanga, M. G. (2019). Acid pretreatment optimization for xylose production from Agave tequilana Weber var. azul, Agave americana var. oaxacensis, Agave karwinskii, and Agave potatorum bagasses using a Box-Behnken design. Biomass Convers. Biorefinery. 1–10. doi: 10.1007/s13399-019-00497-z
Delgado-Lemus, A., Casas, A., and Téllez, O. (2014). Distribution, abundance and traditional management of Agave potatorum in the Tehuacán Valley, Mexico: bases for sustainable use of non-timber forest products. J. Ethnobiol. Ethnomed. 10:63. doi: 10.1186/1746-4269-10-63
Denevan, W. M. (2003). Cultivated Landscapes of Native Amazonia and the Andes. New York, NY: Oxford University Press.
Díaz, G., Rodgers, A., and Byland, B. E. (1993). The Codex Borgia: A Full-Color Restoration of the Ancient Mexican Manuscript. Mineola, NY: Courier Corporation.
Doelle, W. H. (1978). “Hohokam use of nonriverine resources”, in Experiments in the Archaeology of the American Southwest, ed P. Grebinger, 245–274.
Doolittle, W. E. (1995). Indigenous development of Mesoamerican irrigation. Geogr. Rev. 85:301. doi: 10.2307/215275
Doyel, D. E. (2007). “Irrigation, production, and power in Phoenix basin Hohokam society,” in Hohokam Millenn (Santa Fe: School of Advanced Research Press), 82–89.
Eickmeier, W. G., and Adams, M. S. (1978). Gas exchange in Agave lecheguilla Torr. (Agavaceae) and its ecological implications. Southwest. Nat. 22, 473–485. doi: 10.2307/3670254
Enríquez-Salazar, M. I., Veana, F., Aguilar, C. N., Iliana, M., López, M. G., Rutiaga-Quinones, O. M., et al. (2017). Microbial diversity and biochemical profile of aguamiel collected from Agave salmiana and A. atrovirens during different seasons of year. Food Sci. Biotechnol. 26, 1003–1011. doi: 10.1007/s10068-017-0141-z
Escalante, A., López, D. S., Velázquez, J. G., Giles-Gómez, M., Bolívar, F., and López-Munguía, A. (2016). Pulque, a traditional mexican alcoholic fermented beverage: historical, microbiological, and technical aspects. Front. Microbiol. 7:1026. doi: 10.3389/fmicb.2016.01026
Escamilla-Treviño, L. L. (2012). Potential of plants from the genus agave as bioenergy crops. BioEnergy Res. 5, 1–9. doi: 10.1007/s12155-011-9159-x
Evans, S. T. (1990). The productivity of maguey terrace agriculture in central Mexico during the Aztec period. Lat. Am. Antiq. 1, 117–132. doi: 10.2307/971983
Evenari, M., Shanan, L., Tadmor, N., and Shkolnik, A. (1982). The Negev: The Challenge of a Desert. Cambridge: Harvard University Press. doi: 10.4159/harvard.9780674419254
Fabig, B., Moawad, A. M., and Achtnich, W. (1989). Effect of VA Mycorrhiza on dry weight and phosphorus content in shoots of cereal crops fertilized with rock phosphates at different soil pH and temperature levels. Z. Für Pflanzenernähr. Bodenkd. 152, 255–259. doi: 10.1002/jpln.19891520218
Fischbeck, S. L. (2001). Agricultural Terrace Productivity in the Maya Lowlands of Belize. Ph.D., dissertation, University of Wisconsin.
Fish, P. R., Fish, S. K., Long, A., and Miksicek, C. (1986). Early corn remains from Tumamoc Hill, southern Arizona. Am. Antiq. 51, 563–572. doi: 10.2307/281752
Fish, S., and Fish, P. (1990). “An archaeological assessment of ecosystem in the Tucson Basin of southern Arizona”, in The Ecosystem Approach in Anthropology: From Concept to Practice, ed E. F. Moran, 159–160.
Fish, S. K. (1988). “Environment and subsistence: The pollen evidence,” in Recent Research on Tucson Basin Prehistory Proceedings of the Second Tucson Basin Conference, Institute for American Research Anthropological Papers (Tucson AZ: Institute for American Research), 31–38.
Fish, S. K. (2000). “Hohokam impacts on Sonoran Desert environment”, in Imperfect Balance: Landscape Transformations in the Precolumbian Americas, ed D. L. Lentz, 251–280. doi: 10.7312/lent11156-013
Fish, S. K., and Fish, P. R. (1992). Prehistoric landscapes of the Sonoran desert Hohokam. Popul. Environ. 13, 269–283. doi: 10.1007/BF01271027
Fish, S. K., and Fish, P. R. (2008). The Hohokam Millennium. Santa Fe: School for Advanced Research Press.
Fish, S. K., and Fish, P. R. (2012). “Hohokam Society and Water Management,” in The Oxford Handbook of North American Archaeology (New York).
Fish, S. K., and Fish, P. R. (2014). “Agave (Agave spp.): a crop lost and found in the US-Mexico borderlands,” in New Lives for Ancient and Extinct Crops, ed P. E. Minnis (Tucson, AZ: University of Arizona Press), 102–138.
Fish, S. K., Fish, P. R., Miksicek, C., and Madsen, J. (1985). Prehistoric Cultivation in Southern Arizona. Tucson, AZ: Desert plants USA.
Flores, N. B., and Araiza, P. L. S. (2012). El mezcal en Sonora, México, más que una bebida espirituosa. Etnobotánica de Agave angustifolia Haw. Estud. Soc. Rev. Aliment. Contemp. Desarro. Reg. 2, 173–197.
Fuentes-Rodriguez, J. (1997). A comparison of the nutritional value of opuntia and agave plants for ruminants. J. Prof. Assoc. Cactus Dev. 2, 20–22.
Galloway, P. R. (1986). Long-term fluctuations in climate and population in the preindustrial era. Popul. Dev. Rev. 12, 1–24. doi: 10.2307/1973349
Garay, T., and Aurea, M. (2008). “El Recreo de los Amigos.” Mexico City's Pulquerias During the Liberal Republic (1856-1911). Available online at: https://repository.arizona.edu/handle/10150/194973 (accessed November 17, 2019).
Garcia-Aguirre, M., Saenz-Alvaro, V. A., Rodriguez-Soto, M. A., Vicente-Magueyal, F. J., Botello-Alvarez, E., Jimenez-Islas, H., et al. (2009). Strategy for biotechnological process design applied to the enzymatic hydrolysis of agave fructo-oligosaccharides to obtain fructose-rich syrups. J. Agric. Food Chem. 57, 10205–10210. doi: 10.1021/jf902855q
Garcia-Moya, E., Romero-Manzanares, A., and Nobel, P. S. (2011). Highlights for agave productivity. Gcb Bioenergy 3, 4–14. doi: 10.1111/j.1757-1707.2010.01078.x
Gasser, R. E., and Kwiatkowski, S. M. (1991). Regional signatures of Hohokam plant use. Kiva 56, 207–226. doi: 10.1080/00231940.1991.11758168
Gentry, H. S. (1972). The Agave Family in Sonora. Washington, DC: US Agricultural Research Service, US Department of Agriculture.
Gibson, P. T. (1996). Correcting for inbreeding in parent-offspring regression estimates of heritability with non-additive and genotype x environment effects present. Crop Sci. 36, 594–600. doi: 10.2135/cropsci1996.0011183X003600030012x
Glasziou, K. T. (1961). Accumulation & transformation of sugars in stalks of sugar cane. Origin of glucose & fructose in the inner space 1. Plant Physiol. 36, 175–179. doi: 10.1104/pp.36.2.175
Good-Avila, S. V., Souza, V., Gaut, B. S., and Eguiarte, L. E. (2006). Timing and rate of speciation in Agave (Agavaceae). Proc. Natl. Acad. Sci. U.S.A. 103, 9124–9129. doi: 10.1073/pnas.0603312103
Gutiérrez-Coronado, M. L., Acedo-Félix, E., and Valenzuela-Quintanar, A. I. (2007). Industria del bacanora y su proceso de elaboración. CYTA-J. Food 5, 394–404. doi: 10.1080/11358120709487718
Hansen, J., Johnson, D., Lacis, A., Lebedeff, S., Lee, P., Rind, D., et al. (1981). Climate impact of increasing atmospheric carbon dioxide. Science 213, 957–966. doi: 10.1126/science.213.4511.957
Hartung, T. (2016). Cattail Moonshine & Milkweed Medicine: The Curious Stories of 43 Amazing North American Native Plants. North Adams, MA: Storey Publishing.
Helama, S., Timonen, M., Holopainen, J., Ogurtsov, M. G., Mielikäinen, K., Eronen, M., et al. (2009). Summer temperature variations in Lapland during the Medieval Warm Period and the little Ice Age relative to natural instability of thermohaline circulation on multi-decadal and multi-centennial scales. J. Quat. Sci. Publ. Quat. Res. Assoc. 24, 450–456. doi: 10.1002/jqs.1291
Heyer, J. A., and Crawford, J. R. (2009). Use of Natural Agave Extract as a Natural Sweetener Replacing Other Added Sweeteners in Food Products and Medicines. U.S. Patent Application 11/999, 719.
Hodgson, W. C. (2001). Taxonomic novelties in American Agave (Agavaceae). Novon 11, 410–416. doi: 10.2307/3393152
Hodgson, W. C., and Salywon, A. M. (2013). Two new Agave species (Agavaceae) from central Arizona and their putative pre-Columbian domesticated origins. Brittonia 65, 5–15. doi: 10.1007/s12228-012-9255-z
Hodgson, W. C., Salywon, A. M., and Doelle, W. H. (2019). Hohokam lost crop found: A new Agave (Agavaceae) species only known from large-scale pre-Columbian agricultural fields in southern Arizona. Syst. Bot. 43, 734–740. doi: 10.1600/036364418X697445
Holtum, J. A. M., and Winter, K. (2014). Limited photosynthetic plasticity in the leaf-succulent CAM plant Agave angustifolia grown at different temperatures. Funct. Plant Biol. 41, 843–849. doi: 10.1071/FP13284
Homburg, J. A., and Sandor, J. A. (2011). Anthropogenic effects on soil quality of ancient agricultural systems of the American Southwest. Catena 85, 144–154. doi: 10.1016/j.catena.2010.08.005
Hooshmand, S., Holloway, B., Nemoseck, T., Cole, S., Petrisko, Y., Hong, M. Y., et al. (2014). Effects of agave nectar versus sucrose on weight gain, adiposity, blood glucose, insulin, and lipid responses in mice. J. Med. Food 17, 1017–1021. doi: 10.1089/jmf.2013.0162
Huang, B., and Nobel, P. S. (1992). Hydraulic conductivity and anatomy for lateral roots of Agave deserti during root growth and drought-induced abscission. J. Exp. Bot. 43, 1441–1449. doi: 10.1093/jxb/43.11.1441
Hughes, M. K., and Diaz, H. F. (1994). Was there a Medieval Warm Period, and if so, where and when? Clim. Change 26, 109–142. doi: 10.1007/978-94-011-1186-7_1
Hunt, R. C., Guillet, D., Abbott, D. R., Bayman, J., Fish, P., Fish, S., et al. (2005). Plausible ethnographic analogies for the social organization of Hohokam canal irrigation. Am. Antiq. 70, 433–456. doi: 10.2307/40035308
Ingram, S. E. (2010). Human Vulnerability to Climatic Dry Periods in the Prehistoric US Southwest. PhD dissertation, Arizona State University.
Iñiguez-Covarrubias, G., Diaz-Teres, R., Sanjuan-Dueñas, R., Anzaldo-Hernández, J., and Rowell, R. M. (2001). Utilization of by-products from the tequila industry. Part 2: potential value of Agave tequilana Weber azul leaves. Bioresour. Technol. 77, 101–108. doi: 10.1016/S0960-8524(00)00167-X
Jordan, P. W., and Nobel, P. S. (1979). Infrequent establishment of seedlings of Agave deserti (Agavaceae) in the northwestern Sonoran Desert. Am. J. Bot. 66, 1079–1084. doi: 10.1002/j.1537-2197.1979.tb06325.x
Joseph, P. V., Joseph, K., and Thomas, S. (1999). Effect of processing variables on the mechanical properties of sisal-fiber-reinforced polypropylene composites. Compos. Sci. Technol. 59, 1625–1640. doi: 10.1016/S0266-3538(99)00024-X
Kaseke, K. F., Mills, A. J., Henschel, J., Seely, M. K., Esler, K., and Brown, R. (2012). The effects of desert pavements (gravel mulch) on soil micro-hydrology. Pure Appl. Geophys. 169, 873–880. doi: 10.1007/s00024-011-0367-2
Kennett, D. J. (2012). Archaic-Period Foragers and Farmers in Mesoamerica. The Oxford Handbook of Mesoamerican Archaeology. New York, NY: Oxford University Press. 141–150. doi: 10.1093/oxfordhb/9780195390933.013.0010
Kicińska-Jakubowska, A., Bogacz, E., and Zimniewska, M. (2012). Review of natural fibers. Part I—vegetable fibers. J. Nat. Fibers 9, 150–167. doi: 10.1080/15440478.2012.703370
Kongkathip, N., Kongkathip, B., Noimai, N., and Suvipa San-oon. (1997). Synthesis of Antiinflammatory Steroids Intermediates From Steroids Extracted From Industrial Waste of Agave Sisalana. Warasan Witthayasat MoKo. Available online at: https://agris.fao.org/agris-search/search.do?recordID=TH2001002855 (accessed June 18, 2020).
Krausmann, F., Erb, K.-H., Gingrich, S., Haberl, H., Bondeau, A., Gaube, V., et al. (2013). Global human appropriation of net primary production doubled in the 20th century. Proc. Natl. Acad. Sci. U.S.A. 110, 10324–10329. doi: 10.1073/pnas.1211349110
Ladefoged, T. N., Flaws, A., and Stevenson, C. M. (2013). The distribution of rock gardens on Rapa Nui (Easter Island) as determined from satellite imagery. J. Archaeol. Sci. 40, 1203–1212. doi: 10.1016/j.jas.2012.09.006
Laurance, W. F., Sayer, J., and Cassman, K. G. (2014). Agricultural expansion and its impacts on tropical nature. Trends Ecol. Evol. 29, 107–116. doi: 10.1016/j.tree.2013.12.001
Lemonnier, P., and Ainsworth, E. A. (2018). “Crop Responses to Rising Atmospheric [CO2] and Global Climate Change,” in Food Security and Climate Change (Chichester: John Wiley & Sons, Ltd), 51–69. doi: 10.1002/9781119180661.ch3
Lewis, S. M., Gross, S., Visel, A., Kelly, M., and Morrow, W. (2015). Fuzzy gis-based multi-criteria evaluation for us agave production as a bioenergy feedstock. Gcb Bioenergy 7, 84–99. doi: 10.1111/gcbb.12116
Li, H., B. Foston, M., Kumar, R., Samuel, R., Gao, X., Hu, F., et al. (2012). Chemical composition and characterization of cellulose for Agave as a fast-growing, drought-tolerant biofuels feedstock. RSC Adv. 2, 4951–4958. doi: 10.1039/c2ra20557b
Lightfoot, D. R. (1994). Morphology and ecology of lithic-mulch agriculture. Geogr. Rev. 172–185. doi: 10.2307/215329
Lightfoot, D. R. (1996). The nature, history, and distribution of lithic mulch agriculture: an ancient technique of dryland agriculture. Agric. Hist. Rev. 44, 206–222.
Lüttge, U. (2004). Ecophysiology of crassulacean acid metabolism (CAM). Ann. Bot. 93, 629–652. doi: 10.1093/aob/mch087
Macías, M. A. (2001). El cluster en la industria del tequila en Jalisco, México1. Agroalimentaria 6, 55–72.
Mancilla-Margalli, N. A., and López, M. G. (2006). Water-soluble carbohydrates and fructan structure patterns from Agave and Dasylirion species. J. Agric. Food Chem. 54, 7832–7839. doi: 10.1021/jf060354v
Marcus, J. (2006). The roles of ritual and technology in Mesoamerican water management. Agric. Strateg. 221–254. doi: 10.2307/j.ctvdjrr1w.14
Martínez Salvador, M., Mata-González, R., Morales Nieto, C., and Valdez-Cepeda, R. (2012). Agave salmiana plant communities in central mexico as affected by commercial use. Environ. Manage. 49, 55–63. doi: 10.1007/s00267-011-9759-4
Martínez, R., S., Trinidad, S. A., Robles, C., Galvis, S., et al. (2012). Crecimiento y sólidos solubles de Agave potatorum Zucc. inducidos por riego y fertilización. Rev. Fitotec. Mex. 35, 61–68.
Martínez-Rodríguez, J., del, C., Mora-Amutio, M. D. la, Plascencia-Correa, L. A., Audelo-Regalado, E., Guardado, F. R., Hernández-Sánchez, E., et al. (2014). Cultivable endophytic bacteria from leaf bases of Agave tequilana and their role as plant growth promoters. Braz. J. Microbiol. 45, 1333–1339. doi: 10.1590/S1517-83822014000400025
Martorell, C., and Ezcurra, E. (2007). The narrow-leaf syndrome: a functional and evolutionary approach to the form of fog-harvesting rosette plants. Oecologia 151, 561–573. doi: 10.1007/s00442-006-0614-x
Masse, W. B. (1979). An intensive survey of prehistoric dry farming systems near Tumamoc Hill in Tucson, Arizona. Kiva 45, 141–186. doi: 10.1080/00231940.1979.11757933
McIntosh, J. J. (2020). Corticosteroid guidance for pregnancy during COVID-19 pandemic. Am. J. Perinatol. 37, 809–812. doi: 10.1055/s-0040-1709684
Mellado, M. (2016). Dietary selection by goats and the implications for range management in the Chihuahuan Desert: a review. Rangel. J. 38, 331–341. doi: 10.1071/RJ16002
Mensah, J. A., Koch, A. M., Antunes, P. M., Kiers, E. T., Hart, M., and Bücking, H. (2015). High functional diversity within species of arbuscular mycorrhizal fungi is associated with differences in phosphate and nitrogen uptake and fungal phosphate. Metabolism. 25, 533-546. doi: 10.1007/s00572-015-0631-x
Michel-Cuello, C., Juárez-Flores, B. I., Aguirre-Rivera, J. R., and Pinos-Rodríguez, J. M. (2008). Quantitative characterization of nonstructural carbohydrates of mezcal Agave (Agave salmiana Otto ex Salm-Dick). J. Agric. Food Chem. 56, 5753–5757. doi: 10.1021/jf800158p
Minnis, P. E., and Plog, S. E. (1976). A study of the site specific distribution of Agave parryi in east central Arizona. Kiva 41, 299–308. doi: 10.1080/00231940.1976.11757854
Minnis, P. E., Whalen, M. E., and Howell, R. E. (2006). Fields of power: upland farming in the prehispanic casas grandes polity, Chihuahua, Mexico. Am. Antiq. 71, 707–722. doi: 10.2307/40035885
Mitchell, W. P. (1973). The hydraulic hypothesis: a reappraisal. Curr. Anthropol. 14, 532–534. doi: 10.1086/201379
Moore, K. J., Karlen, D. L., and Lamkey, K. R. (2014). “Future prospects for corn as a biofuel crop”, in Compendium of Bioenergy Plants: Corn, Chapter 13, eds S. L. Goldman and C. Kole, 5, 331–352.
Morán, E. (2008). Constructing identity: the role of food in mexica migration and creation accounts. Lat. Am. 52, 15–27 doi: 10.1111/j.1557-203X.2008.00003.x
Narváez-Zapata, J. A., and Sánchez-Teyer, L. F. (2010). Agaves as a raw material: recent technologies and applications. Recent Pat. Biotechnol. 3, 185–191. doi: 10.2174/187220809789389144
Neftel, A., Moor, E., Oeschger, H., and Stauffer, B. (1985). Evidence from polar ice cores for the increase in atmospheric CO2 in the past two centuries. Nature 315:45. doi: 10.1038/315045a0
Nobel, P. S. (1976). Water relations and photosynthesis of a desert CAM plant, Agave deserti. Plant Physiol. 58, 576–582. doi: 10.1104/pp.58.4.576
Nobel, P. S. (1977). Water relations of flowering of Agave deserti. Bot. Gaz. 138, 1–6. doi: 10.1086/336888
Nobel, P. S. (1991). Achievable productivities of certain CAM plants: basis for high values compared with C3 and C4 plants. New Phytol. 119, 183–205. doi: 10.1111/j.1469-8137.1991.tb01022.x
Nobel, P. S. (2003). Environmental Biology of Agaves and Cacti. Cambridge, NY: Cambridge University Press.
Nobel, P. S., García-Moya, E., and Quero, E. (1992a). High annual productivity of certain agaves and cacti under cultivation. Plant Cell Environ. 15, 329–335. doi: 10.1111/j.1365-3040.1992.tb00981.x
Nobel, P. S., and Hartsock, T. L. (1986). Temperature, water, and PAR influences on predicted and measured productivity of Agave deserti at various elevations. Oecologia 68, 181–185. doi: 10.1007/BF00384785
Nobel, P. S., and Jordan, P. W. (1983). Transpiration stream of desert species: resistances and capacitances for a C3, a C4, and a CAM plant. J. Exp. Bot. 34, 1379–1391. doi: 10.1093/jxb/34.10.1379
Nobel, P. S., Miller, P. M., and Graham, E. A. (1992b). Influence of rocks on soil temperature, soil water potential, and rooting patterns for desert succulents. Oecologia 92, 90–96. doi: 10.1007/BF00317267
Nobel, P. S., and Quero, E. (1986). Environmental productivity indices for a Chihuahuan desert CAM plant, Agave lechuguilla. Ecology 67, 1–11. doi: 10.2307/1938497
Nobel, P. S., Quero, E., and Linares, H. (1988). Differential growth responses of agaves to nitrogen, phosphorus, potassium, and boron applications. J. Plant Nutr. 11, 1683–1700. doi: 10.1080/01904168809363925
Nobel, P. S., Quero, E., and Linares, H. (1989). Root versus shoot biomass: responses to water, nitrogen, and phosphorus applications for Agave lechuguilla. Bot. Gaz. 150, 411–416. doi: 10.1086/337787
Nobel, P. S., and Valenzuela, A. G. (1987). Environmental responses and productivity of the CAM plant, Agave tequilana. Agric. For. Meteorol. 39, 319–334. doi: 10.1016/0168-1923(87)90024-4
North, G. B., and Nobel, P. S. (1991). Changes in hydraulic conductivity and anatomy caused by drying and rewetting roots of Agave deserti (Agavaceae). Am. J. Bot. 78, 906–915. doi: 10.1002/j.1537-2197.1991.tb14494.x
Núñez, N., L, Salazar-Solano, V., and Acedo-Felix, E. (2008). El Bacanora: Cultivo, Regulación y Mercados. Hermosillo: Centro de Investigación en Alimentos y Desarrollo (CIAD).
O'Brien-Nabors, L. (2001). Alternative Sweeteners, Third Edition, Revised and Expanded. Boca Raton, FL: CRC Press.
Orians, G. H., and Solbrig, O. T. (1977). A cost-income model of leaves and roots with special reference to arid and semiarid areas. Am. Nat. 111, 677–690. doi: 10.1086/283199
Orue, A., Jauregi, A., Unsuain, U., Labidi, J., Eceiza, A., and Arbelaiz, A. (2016). The effect of alkaline and silane treatments on mechanical properties and breakage of sisal fibers and poly(lactic acid)/sisal fiber composites. Compos. Part Appl. Sci. Manuf. 84, 186–195. doi: 10.1016/j.compositesa.2016.01.021
Palta, J. A., and Nobel, P. S. (1989). Influence of soil O2 and CO2 on root respiration for Agave deserti. Physiol. Plant. 76, 187–192. doi: 10.1111/j.1399-3054.1989.tb05630.x
Parker, K. C., Hamrick, J. L., Hodgson, W. C., Trapnell, D. W., Parker, A. J., and Kuzoff, R. K. (2007). Genetic consequences of pre-Columbian cultivation for Agave murpheyi and A. delamateri (Agavaceae). Am. J. Bot. 94, 1479–1490. doi: 10.3732/ajb.94.9.1479
Parker, K. C., Trapnell, D. W., Hamrick, J. L., and Hodgson, W. C. (2014). Genetic and morphological contrasts between wild and anthropogenic populations of Agave parryi var. huachucensis in south-eastern Arizona. Ann. Bot. 113, 939–952. doi: 10.1093/aob/mcu016
Parker, K. C., Trapnell, D. W., Hamrick, J. L., Hodgson, W. C., and Parker, A. J. (2010). Inferring ancient agave cultivation practices from contemporary genetic patterns. Mol. Ecol. 19, 1622–1637. doi: 10.1111/j.1365-294X.2010.04593.x
Perry, L., and Flannery, K. V. (2007). Precolumbian use of chili peppers in the valley of Oaxaca, Mexico. Proc. Natl. Acad. Sci. U.S.A. 104, 11905–11909. doi: 10.1073/pnas.0704936104
Pinos-Rodríguez, J. M., Aguirre-Rivera, J. R., García-López, J. C., Rivera-Miranda, M. T., González-Muñoz, S., López-Aguirre, S., et al. (2006). Use of “Maguey” (Agave salmiana Otto ex. Salm-Dick) as forage for ewes. J. Appl. Anim. Res. 30, 101–107. doi: 10.1080/09712119.2006.9706596
Pinos-Rodríguez, J. M., Zamudio, M., and González, S. S. (2008). The effect of plant age on the chemical composition of fresh and ensiled Agave salmiana leaves. South Afr. J. Anim. Sci. 38, 43–50. doi: 10.4314/sajas.v38i1.4108
Pinos-Rodríguez, J. M., Zamudio, M., González, S. S., Mendoza, G. D., Bárcena, R., Ortega, M. E., et al. (2009). Effects of maturity and ensiling of Agave salmiana on nutritional quality for lambs. Anim. Feed Sci. Technol. 152, 298–306. doi: 10.1016/j.anifeedsci.2009.05.002
Pongratz, J., and Caldeira, K. (2012). Attribution of atmospheric CO2 and temperature increases to regions: importance of preindustrial land use change. Environ. Res. Lett. 7:034001. doi: 10.1088/1748-9326/7/3/034001
Porter, J. R., and Semenov, M. A. (2005). Crop responses to climatic variation. Philos. Trans. R. Soc. B Biol. Sci. 360, 2021–2035. doi: 10.1098/rstb.2005.1752
Reick, C., Raddatz, T., Pongratz, J., and Claussen, M. (2010). Contribution of anthropogenic land cover change emissions to pre-industrial atmospheric CO2. Tellus B Chem. Phys. Meteorol. 62, 329–336. doi: 10.1111/j.1600-0889.2010.00479.x
Revelle, R., and Suess, H. E. (1957). Carbon dioxide exchange between atmosphere and ocean and the question of an increase of atmospheric CO2 during the past decades. Tellus 9, 18–27. doi: 10.3402/tellusa.v9i1.9075
Rice, G. (1998). War and water: an ecological perspective on Hohokam irrigation. Kiva 63, 263–301. doi: 10.1080/00231940.1998.11758357
Rivas, H. G. (1991). Cocina Prehispánica Mexicana: la Comida de los Antiguos Mexicanos. Mexico, D.F: Panorama Editorial.
SAGARPA (2017). Planeación Agrícola Nacional 2017-2030, Agave tequilero y mezcalero Mexicano. Available online at: https://www.gob.mx/cms/uploads/attachment/file/257066/Potencial-Agave_Tequilero_y_Mezcalero.pdf (accessed September 11, 2019).
Sandor, J. A., and Homburg, J. A. (2011). “Soil and landscape responses to American Indian agriculture in the Southwest,” in Movement, Connectivity, and Landscape Change in the Ancient Southwest: the 20th Anniversary Southwest Symposium. (Boulder, CO: University Press of Colorado), 141–59.
Sandor, J. A., and Homburg, J. A. (2017). Anthropogenic soil change in ancient and traditional agricultural fields in arid to semiarid regions of the Americas. J. Ethnobiol. 37, 196–218. doi: 10.2993/0278-0771-37.2.196
Santos, J. D. G., and Branco, A. (2014). GC-MS characterisation of sapogenins from sisal waste and a method to isolate pure hecogenin. BioResources 9, 1325–1333. doi: 10.15376/biores.9.1.1325-1333
Schlaepfer, D. R., Bradford, J. B., Lauenroth, W. K., Munson, S. M., Tietjen, B., Hall, S. A., et al. (2017). Climate change reduces extent of temperate drylands and intensifies drought in deep soils. Nat. Commun. 8:14196. doi: 10.1038/ncomms14196
Silva, F. de A., Filho, R. D. T., Filho, J. de A. M., and Fairbairn, E. de M. R. (2010). Physical and mechanical properties of durable sisal fiber?cement composites. Constr. Build. Mater. 24, 777–785. doi: 10.1016/j.conbuildmat.2009.10.030
Silva-Montellano, A., and Eguiarte, L. E. (2003). Geographic patterns in the reproductive ecology of Agave lechuguilla (Agavaceae) in the Chihuahuan desert. I. Floral characteristics, visitors, and fecundity. Am. J. Bot. 90, 377–387. doi: 10.3732/ajb.90.3.377
Smith, M. N., Stark, S. C., Taylor, T. C., Ferreira, M. L., de Oliveira, E., Restrepo-Coupe, N., et al. (2019). Seasonal and drought-related changes in leaf area profiles depend on height and light environment in an Amazon forest. New Phytol. 222, 1284–1297. doi: 10.1111/nph.15726
Solomon, S., Rosenlof, K. H., Portmann, R. W., Daniel, J. S., Davis, S. M., Sanford, T. J., et al. (2010). Contributions of stratospheric water vapor to decadal changes in the rate of global warming. Science 327, 1219–1223. doi: 10.1126/science.1182488
Somerville, C., Youngs, H., Taylor, C., Davis, S. C., and Long, S. P. (2010). Feedstocks for lignocellulosic biofuels. Science 329, 790–792. doi: 10.1126/science.1189268
Soto, J. L. M., González, J. V., Nicanor, A. B., and Ramírez, E. G. R. (2011). Enzymatic production of high fructose syrup from Agave tequilana fructans and its physicochemical characterization. Afr. J. Biotechnol. 10, 19137–19143. doi: 10.5897/AJB11.2704
Sridhar, V., Loope, D. B., Swinehart, J. B., Mason, J. A., Oglesby, R. J., and Rowe, C. M. (2006). Large wind shift on the Great Plains during the Medieval Warm Period. Science 313, 345–347. doi: 10.1126/science.1128941
Stager, L. E. (1976). Farming in the Judean desert during the Iron Age. Bull. Am. Sch. Orient. Res. 221, 145–158. doi: 10.2307/1356097
Stevenson, C. M., Wozniak, J., and Haoa, S. (1999). Prehistoric agricultural production on Easter Island (Rapa Nui), Chile. Antiquity 73, 801–812. doi: 10.1017/S0003598X00065546
Stewart, J. R. (2015). Agave as a model CAM crop system for a warming and drying world. Front. Plant Sci. 6:684. doi: 10.3389/fpls.2015.00684
Stinchcomb, G. E., Messner, T. C., Driese, S. G., Nordt, L. C., and Stewart, R. M. (2011). Pre-colonial (AD 1100–1600) sedimentation related to prehistoric maize agriculture and climate change in eastern North America. Geology 39, 363–366. doi: 10.1130/G31596.1
Tequila Regulatory Council (2019). Estadísticas de Producción Total: Tequila y Tequila 100%, Consumo de agave para Tequila y Tequila 100% de agave. Available online at: https://www.crt.org.mx (accessed November 15, 2019).
Towell, J. L., and Lecón, A. A. (2010). Caminos y Mercados de México. Mexico D.F: Universidad Nacional Autónoma de México/Instituto Nacional de Antropología e Historia.
Trombold, C. D. (2017). Agricultural Intensification on the epiClassic northern Mesoamerican frontier: the La Quemada Terraces. J. Anthropol. Archaeol. 48, 309–319. doi: 10.1016/j.jaa.2017.09.005
Troyo-Diéguez, E., de Lachica-Bonilla, F., and Fernàndez-Zayas, J. L. (1990). A simple aridity equation for agricultural purposes in marginal zones. J. Arid Environ. 19, 353–362. doi: 10.1016/S0140-1963(18)30799-7
Turner, B. L. (1976). Population density in the classic Maya lowlands: new evidence for old approaches. Geogr. Rev. 66, 73–82. doi: 10.2307/213316
Urias-Silvas, J. E., Cani, P. D., Delmée, E., Neyrinck, A., López, M. G., and Delzenne, N. M. (2008). Physiological effects of dietary fructans extracted from Agave tequilana Gto. and Dasylirion spp. Br. J. Nutr. 99, 254–261. doi: 10.1017/S0007114507795338
Valenzuela, Z., A. G., and Gonzalez, E., D. R. (1995). Fertilization of (Agave tequilana Weber) of Tequila. Jalisco: TERRA Mex.
Van West, C. R., and Dean, J. S. (2000). Environmental characteristics of the AD 900–1300 period in the Central Mesa Verde region. Kiva 66, 19–44. doi: 10.1080/00231940.2000.11758420
Vargas-Ponce, O., Zizumbo-Villarreal, D., and Marin, P. C.-G. (2007). In situ diversity and maintanenace of traditional Agave landraces used in spirits production in West-Central Mexico. Econ. Bot. 61:362. doi: 10.1663/0013-0001(2007)61[362:ISDAMO]2.0.CO;2
Walton, M. K. (1977). The evolution and localization of mezcal and tequila in Mexico. Rev. Geográfica 85, 113–132.
Webb, E. A., Schwarcz, H. P., and Healy, P. F. (2004). Detection of ancient maize in lowland Maya soils using stable carbon isotopes: evidence from Caracol, Belize. J. Archaeol. Sci. 31, 1039–1052. doi: 10.1016/j.jas.2004.01.001
Wilken, G. C. (1972). Microclimate management by traditional farmers. Geogr. Rev. 62, 544–560. doi: 10.2307/213267
Williams, D. E. (1990). A review of sources for the study of Náhuatl plant classification. Adv. Econ. Bot. 8, 249–270.
Woodbury, R. B. (1961). A reappraisal of Hohokam irrigation. Am. Anthropol. 63, 550–560. doi: 10.1525/aa.1961.63.3.02a00070
Woodhouse, C. A., Meko, D. M., MacDonald, G. M., Stahle, D. W., and Cook, E. R. (2010). A 1,200-year perspective of 21st century drought in southwestern North America. Proc. Natl. Acad. Sci. U.S.A. 107, 21283–21288. doi: 10.1073/pnas.0911197107
Woodhouse, R. M., Williams, J. G., and Nobel, P. S. (1980). Leaf orientation, radiation interception, and nocturnal acidity Increases by the CAM plant Agave Deserti (Agavaceae). Am. J. Bot. 67, 1179–1185. doi: 10.1002/j.1537-2197.1980.tb07751.x
Wozniak, J. A. (1999). Prehistoric horticultural practices on Easter Island: lithic mulched gardens and field systems. Rapa Nui J. 13, 95–99.
Yan, X., Tan, D. K., Inderwildi, O. R., Smith, J. A. C., and King, D. A. (2011). Life cycle energy and greenhouse gas analysis for agave-derived bioethanol. Energy Environ. Sci. 4, 3110–3121. doi: 10.1039/c1ee01107c
Yang, X., Cushman, J. C., Borland, A. M., Edwards, E. J., Wullschleger, S. D., Tuskan, G. A., et al. (2016). A roadmap for research on crassulacean acid metabolism (CAM) to enhance sustainable food and bioenergy production in a hotter, drier world. New Phytol. 207, 491–504.
Yilmaz, N. D., and Arifuzzaman Khan, G. M. (2019). “2 - Flexural behavior of textile-reinforced polymer composites,” in Mechanical and Physical Testing of Biocomposites, Fibre-Reinforced Composites and Hybrid Composites Woodhead Publishing Series in Composites Science and Engineering. eds. M. Jawaid, M. Thariq, and N. Saba (Woodhead Publishing), 13–42. doi: 10.1016/B978-0-08-102292-4.00002-3
Ynnilä, H. (2007). To Petra via Jabal Haroun: Nabataean-Roman road remains in the Finnish Jabal Haroun project survey area. MA thesis. University of Helsinki. Available online at: https://helda.helsinki.fi/handle/10138/19544 (accessed August 30, 2020).
Zandalinas, S. I., Mittler, R., Balfagón, D., Arbona, V., and Gómez-Cadenas, A. (2018). Plant adaptations to the combination of drought and high temperatures. Physiol. Plant. 162, 2–12. doi: 10.1111/ppl.12540
Zhang, X., Song, K., Tong, F., Fei, M., Guo, H., Lu, Z., et al. (2020). First case of COVID-19 in a patient with multiple myeloma successfully treated with tocilizumab. Blood Adv. 4, 1307–1310. doi: 10.1182/bloodadvances.2020001907
Zizumbo-Villarreal, D., Flores-Silva, A., and Marín, P. C.-G. (2012). The archaic diet in Mesoamerica: incentive for milpa development and species domestication. Econ. Bot. 66, 328–343. doi: 10.1007/s12231-012-9212-5
Zizumbo-Villarreal, D., González-Zozaya, F., Olay-Barrientos, A., Platas-Ruíz, R., Cuevas-Sagardí, M., Almendros-López, L., et al. (2009). Archaeological evidence of the cultural importance of Agave spp. in pre-Hispanic Colima, Mexico. Econ. Bot. 63, 288–302. doi: 10.1007/s12231-009-9092-5
Zizumbo-Villarreal, D., Vargas-Ponce, O., Rosales-Adame, J. J., and Colunga-García Marín, P. (2013). Sustainability of the traditional management of Agave genetic resources in the elaboration of mezcal and tequila spirits in western Mexico. Genet. Resour. Crop Evol. 60, 33–47. doi: 10.1007/s10722-012-9812-z
Keywords: crassulacean, succulent, dryland farming, rock mulching, rock piles
Citation: Ortiz-Cano H, Hernandez-Herrera JA, Hansen NC, Petersen SL, Searcy MT, Mata-Gonzalez R, Cervantes-Mendívil T, Villanueva-Morales A, Park PM and Stewart JR (2020) Pre-Columbian Rock Mulching as a Strategy for Modern Agave Cultivation in Arid Marginal Lands. Front. Agron. 2:10. doi: 10.3389/fagro.2020.00010
Received: 14 March 2020; Accepted: 10 August 2020;
Published: 24 September 2020.
Edited by:
Karolina Heyduk, University of Hawaii, United StatesReviewed by:
Daniel Sacristán Moraga, University of the Balearic Islands, SpainJessé Rodrigo Fink, Instituto Federal do Paraná Câmpus Palmas, Brazil
Copyright © 2020 Ortiz-Cano, Hernandez-Herrera, Hansen, Petersen, Searcy, Mata-Gonzalez, Cervantes-Mendívil, Villanueva-Morales, Park and Stewart. This is an open-access article distributed under the terms of the Creative Commons Attribution License (CC BY). The use, distribution or reproduction in other forums is permitted, provided the original author(s) and the copyright owner(s) are credited and that the original publication in this journal is cited, in accordance with accepted academic practice. No use, distribution or reproduction is permitted which does not comply with these terms.
*Correspondence: Hector Ortiz-Cano, aGVjdG9yb2MmI3gwMDA0MDtnbWFpbC5jb20=