- YOXLO B.V., Leiden, Netherlands
Despite extensive research into extending human healthspan (HS) and compressing morbidity, the mechanisms underlying aging remain elusive. However, a better understanding of the genetic advantages responsible for the exceptional HS of healthy centenarians (HC), who live in good physical and mental health for one hundred or more years, could lead to innovative health-extending strategies. This review explores the role of NLRP3, a critical component of innate immunity that significantly impacts aging. It is activated by pathogen-associated signals and self-derived signals that increase with age, leading to low-grade inflammation implicated in age-related diseases. Furthermore, NLRP3 functions upstream in several molecular aging pathways, regulates cellular senescence, and may underlie the robust health observed in HC. By targeting NLRP3, mice exhibit a phenotype akin to that of HC, the HS of monkeys is extended, and aging symptoms are reversed in humans. Thus, targeting NLRP3 could offer a promising approach to extend HS. Additionally, a paradigm shift is proposed. Given that the HS of the broader population is 30 years shorter than that of HC, it is postulated that they suffer from a form of accelerated aging. The term ‘auto-aging’ is suggested to describe accelerated aging driven by NLRP3.
1 Introduction
The significant extension of human lifespan (LS) since the 19th century, attributed to advancements in socioeconomic conditions, healthcare, and nutrition (Oeppen and Vaupel, 2002) has propelled individuals into what is sometimes referred to as a ‘red zone.’ In this zone, the incidence of dementia, other disabilities, and frailty increases sharply (Olshansky, 2018). This situation creates a paradoxical effect where curing or preventing one disease not only extends LS but may also inadvertently allow time for the emergence of another, often more debilitating, condition. As a result, this extension of life often leads to individuals living longer but with an increased duration of disability and poor health (Spiers et al., 2021). Currently, the number of healthy life years at birth, or HS, in the Western world is 66.6 years. The duration of life spent in increasingly poor health or disability is approximately 13.5 years, cumulating in an average LS of 80.1 years (Eurostat, 2019; Scott et al., 2021). This realization that we are extending the duration of disease has catalyzed a shift in focus from simply prolonging life to extending health. Therefore, understanding the determinants of HS is crucial. The foremost question is whether these determinants are environmentally influenced or genetically predetermined.
Extensive research, including studies on identical twins, suggests that genetics accounts for approximately 25% of the variability in HS and LS (Herskind et al., 1996; Ljungquist et al., 1998). This is further exemplified by the lifespan variations observed in genetically identical C57BL mice, ranging from 13 to 28 months despite uniform genetics and environmental conditions (Marín-Aguilar et al., 2020). These differences underscore the profound impact of environmental variations and stochastic events, such as random cellular mutations and chance encounters with pathogens, on health and longevity and highlight the complex interplay between genetics and the cellular environment. Contrasting these findings, other research shows that single genetic alterations can significantly extend average LS and HS in nematodes, fruitflies, and mice by factors of 2–3, 1.5, and 1.6, respectively. Notably, these genetically modified organisms maintain a similar variability in LS as their wild-type counterparts (Brown-Borg et al., 1996; Wolkow et al., 2000; Clancy et al., 2001). Thus, while genetics account for about 25% of LS variability in genetically identical organisms, a single genetic modification can shift their LS and HS curve to the right (see Graph 1).
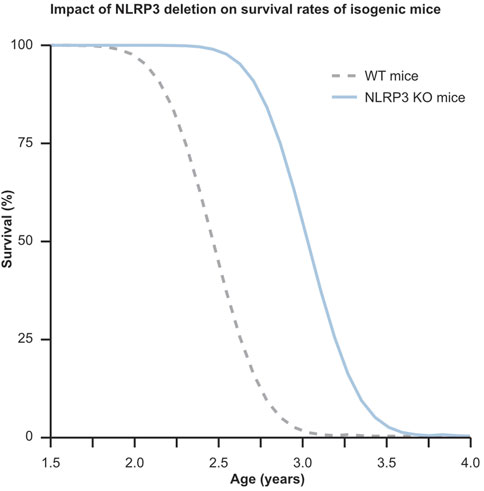
Graph 1. This graph illustrates the profound influence of a single genetic modification on extending lifespan, while also highlighting the stochastic variability in lifespan distribution among genetically identical mice. Despite genetics accounting for only about 25% of individual lifespan variation, the knockout of the NLRP3 gene in a population of mice leads to a notable 32% increase in average lifespan compared to their C57BL wild type counterparts. This underscores the significant potential of targeting aging-related genes responsible for this effect. In the graph, the dotted gray curve represents WT mice, while the solid blue curve depicts NLRP3−/− mice.
The small group of HC exemplifies the possibility for humans to maintain good physical and mental health for at least one hundred years. These remarkable individuals enjoy an extended HS that surpasses the average by over 3 decades. What is more, they typically experience a disease span (morbidity) of approximately half of the 13.5 years of morbidity observed in the general population (Evert et al., 2003; Andersen et al., 2012). The question that arises is: what makes these HC ‘escape’ the diseases that almost everybody else will get? Do they have a beneficial lifestyle and luck that places them at the end of the stochastic distribution curve, or do they possess one or more genetic advantages that protect them from environmental factors that make others sick?
While specific diets seem to increase the chance of becoming a healthy ager, the findings are not entirely consistent. On one hand, studies have found a high adherence to the Mediterranean diet, which is low in animal proteins and carbohydrates, among centenarians (Vasto S et al., 2012). On the other hand, a beneficial effect of a Western-type diet, high in animal protein, fat, and carbohydrates, has been observed among healthy agers over 85 (Gu Q et al., 2020). Additionally, a prospective study of healthy participants over age 80 showed that the chance of becoming a centenarian increased from approximately 1.2% for people adhering to a poor lifestyle to approximately 1.9% for those who strictly adhered to a healthy lifestyle (Li Y et al., 2024). Although this represents a significant effect, the overall contribution of a healthy lifestyle to the likelihood of becoming a centenarian remains relatively minimal.
This minimal effect is reflected in the finding that HC exhibit similarly unhealthy behaviors as individuals in the general population, yet still manage to reach 100 in good health (Rajpathak et al., 2011). Additionally the offspring of these HC tend to remain healthy for significantly longer than those of individuals in the general population. Furthermore, the partners of these offspring, who are likely exposed to similar lifestyle factors, do not experience the same extended HS, suggesting a genetic advantage that protects against aging and accompanying age-related diseases (Perls et al., 2002; Schoenmaker et al., 2006; Sebastiani et al., 2021). This implies that HC do not merely represent the far end of a stochastic distribution curve related to age but have a beneficial genetic trait.
Genetic aberrations that accelerate the onset of common diseases or cause orphan diseases are widely known. Conversely, genetic variants are also known to protect against certain diseases. A notable example is the APOE2 variant of the apolipoprotein E gene, which significantly reduces the risk of acquiring Alzheimer’s disease (Reiman et al., 2020). Emerging evidence suggests that genetic variants leading to altered expression levels or improved versions of specific proteins play a role in the successful aging of HC (Paolisso et al., 1998; Chondrogianni et al., 2000; Alonso-Fernández et al., 2008; Erikson et al., 2016; Tedone et al., 2019). Therefore, it is likely that HC have acquired or inherited one or more of these advantageous traits, meaning that by identifying and harnessing these genetic advantages, innovative treatments could be designed to decelerate or even reverse aging in the broader population. One potential target is the NOD-like receptor family, pyrin domain-containing 3 (NLRP3), which functions as a sensor in the innate immune system and plays a significant role in various age-related pathologies. Chapter 2 provides a detailed discussion of NLRP3’s mechanism of action and involvement in these processes, supported by extensive literature. Humans over the age of 60 exhibit NLRP3 levels that are significantly higher than those in individuals around age 25, whereas HC have been found to maintain NLRP3 expression levels comparable to those of younger individuals, and diseased centenarians exhibit NLRP3 levels that fall between those of HC and younger people (Tedone et al., 2019). Strikingly, the deletion of the Nlrp3 gene in C57BL mice leads to a phenotype akin to that of HC, characterized by delayed onset of bone loss, immunosenescence, frailty, reduced age-related diseases, and a 34% increase in mean HS and LS (Youm et al., 2012; Youm et al., 2013; Marín-Aguilar et al., 2020). These findings support the notion that the exceptional HS of HC is modulated by one or a few critical biological mechanisms, with NLRP3 standing out as a prime candidate for regulation. What is more, when using HC as a benchmark, it becomes evident that the vast majority of individuals who lack this genetic advantage experience accelerated organismal aging, resulting in prolonged morbidity and significantly reduced HS and LS. This review delves into the central role of NLRP3 in the aging process and evaluates its potential as a target to extend human HS.
2 The paradoxical role of NLRP3 in health and aging
NLRP3, also known as Cold autoinflammatory syndrome 1 protein (CIAS1), cryopyrin, NACHT, LRR, and PYD domain-containing protein 3 (NALP3), was initially discovered for its role in cryopyrin-associated periodic syndrome (CAPS) (Hoffman et al., 2001). The periodic fever episodes in CAPS patients, in which NLRP3 is overly active, are caused by the strong pyrogen and pro-inflammatory cytokine interleukin (IL)-1β (March et al., 1985). As it turns out, NLRP3 functions as a critical sensor in the innate immune system, triggering a rapid, robust, and complex response against bacterial and viral intruders and in response to tissue damage, thereby preserving health. However, emerging evidence suggests that increased priming and chronic low-grade activation of NLRP3 also play a detrimental role in aging. Therefore, it is crucial to differentiate between its beneficial short-term activation and the potentially detrimental effects of chronic activation. The following sections will thoroughly review and discuss this dichotomy and its implications for health and disease.
2.1 Short-lived activation of NLRP3 restores health
NLRP3, an innate immune system receptor, is activated through a specific two-step priming process by distinct pathogen- and damage-associated patterns (PAMPs and DAMPs) or signals of cell stress and damage. NLRP3 is part of a family of pattern recognition receptors (PRRs), which includes membrane-bound Toll-like receptors (TLRs) and cytoplasmic nucleotide-binding and oligomerization domain (NOD)-like receptors (NLRs). NLRP3 and other NLRs can recruit caspases upon activation, forming large multi-protein complexes known as inflammasomes. These complexes produce interleukin (IL)-1β and IL-18, crucial for initiating inflammatory responses and pore-forming N-terminal fragments (Nt) of gasdermin D (GSDMD). This leads to pyroptosis and the subsequent clearance of the activation signal, thereby restoring homeostasis and health (Martinon et al., 2002; Shi et al., 2015). NLRP3 plays a unique role within inflammasome-forming NLRs. Its activation leads to pyroptosis, a lytic and highly inflammatory form of programmed cell death. This process significantly contributes to the innate immune response by facilitating the rapid clearance of pathogens and damaged cells. As inflammasomes induce pyroptosis and inflammation with potentially catastrophic results, they are tightly regulated through a two-step process of priming and activation (D’souza and Heitman, 2001).
2.1.1 Priming of NLRP3
Resting cells typically exhibit low levels of NLRP3 mRNA, necessitating a priming step to enhance its expression (Bauernfeind et al., 2009). Lipopolysaccharide (LPS) from gram-negative bacteria and double-stranded RNA following viral infections are known PAMPs that upregulate NLRP3 expression. LPS binds to TLR4, while polyriboinosinic:polyribocytidylic acid (PolyI:C), a synthetic double-stranded RNA analog, induces priming via intracellular, endosomal TLR3 stimulation (Chow et al., 1999; Alexopoulou et al., 2001). Additionally, tumor necrosis factor (TNF)-α, a sterile DAMP, primes NLRP3 by binding to the TNF receptor (TNFR). (Franchi et al., 2009). Both TLR4 and TNFR signal through nuclear factor-κB (NF-κB), leading to transcription of not only NLRP3 but also pro-IL-1β, pro-IL-18, and GSDMD (Awad et al., 2017; Muhammad et al., 2019). The mature inflammasome formed after NLRP3 activation cleaves these molecules into their active forms. For a graphical representation, see Figure 1A.
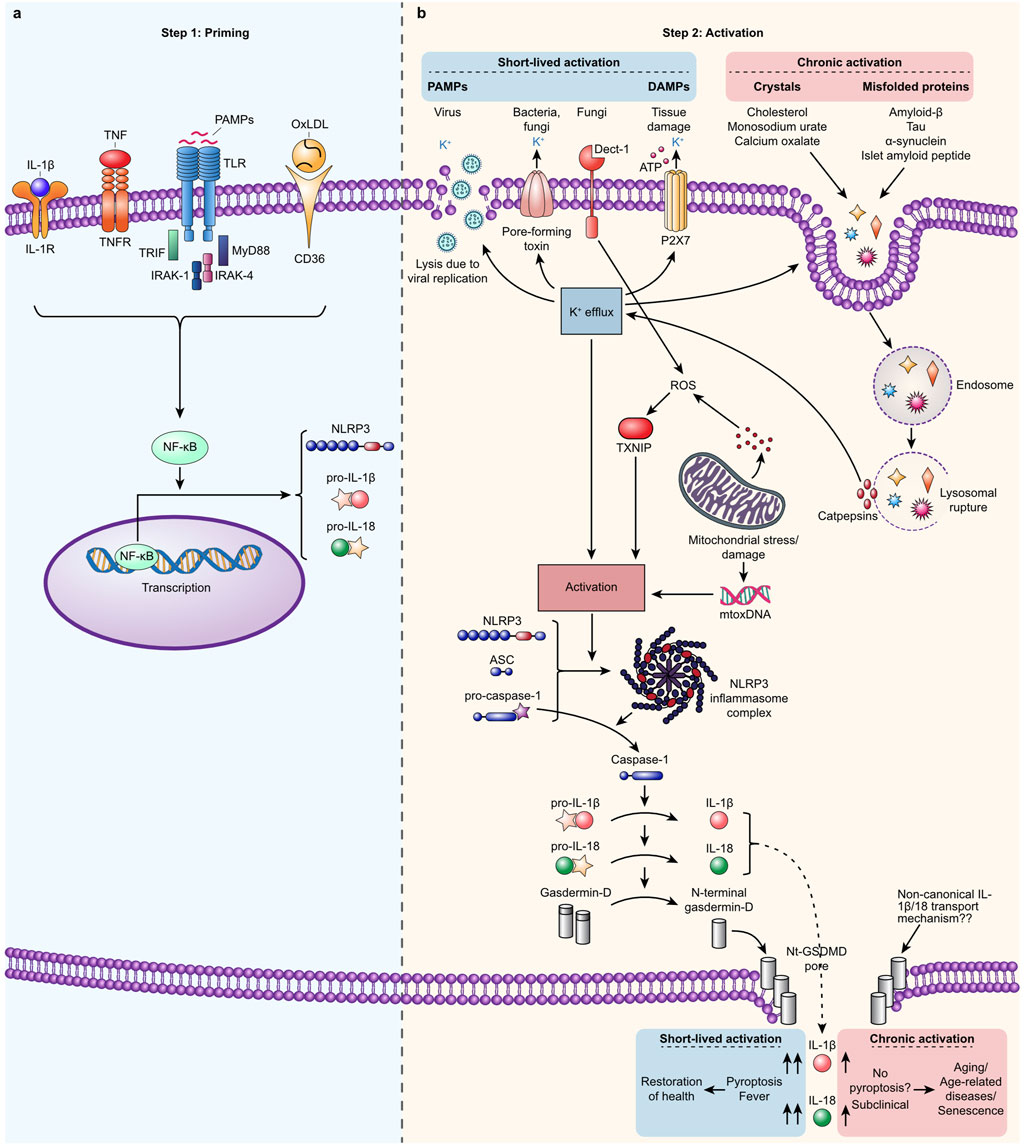
Figure 1. Canonical NLRP3 inflammasome: a two-step process involving priming and activation. (A). Step 1. Priming occurs when extracellular signals such as oxidized LDL, PAMPs like LPS, IL-1α, IL-1β, or TNFs bind to their respective receptors (CD36, TLRs, TNFR, and IL-1R), driving NF-κB-dependent expression of NLRP3, pro-IL-1β, and pro-IL-18. (B). Step 2. A broad spectrum of signals such as PAMPs derived from bacteria, viruses, and fungi and DAMPs, such as extracellular ATP, and mitochondrial stress activate NLRP3 in primed cells. Activation occurs indirectly when K+ efflux, ROS production, or disruption of the cellular membrane is induced or directly by binding mitoDNA, secreted from damaged mitochondrial. Self-derived signals such as cholesterol, monosodium urate, calcium oxalate crystals, and misfolded amyloid-β, Tau, α-synuclein, and islet amyloid peptide can indirectly activate NLRP3. This activation occurs when these substances rupture the lysosomes that contain them, leading to the release of cathepsins. Activated NLRP3 oligomerizes and forms an inflammasome complex that binds the adaptor protein ASC. This complex attracts pro-caspase-1 proteins, which cluster into what becomes the tail of the inflammasome and undergo self-cleavage to form fully active proteases. Active caspase-1 cleaves the substrates pro-IL-1β and pro-IL-18 to form IL-1β and IL- 18, respectively, and cleaves the N-terminal fragment from Gasdermin-D. Once activated, NLRP3 oligomerizes and forms an inflammasome complex that binds to the adaptor protein ASC. This complex then recruits pro-caspase-1 molecules, which cluster and undergo self-cleavage to become fully active proteases. The activated caspase-1 then processes the precursors pro-IL-1β and pro-IL-18 into their active forms, IL-1β and IL-18, respectively. Additionally, activated caspase-1 cleaves the N-terminal fragment from Gasdermin-D, allowing it to assemble into pores in the plasma membrane. These pores facilitate the secretion of active IL-1β and IL-18. Pyroptosis is induced when the concentration of these pores surpasses a certain threshold and the activity of Gasdermin-D pores is prolonged, leading to an inflammatory programmed cell death. In cases of a short and weak response, the number of GSDMD pores remains below the threshold necessary for pyroptosis, allowing cells to secrete low levels of cytokines while staying viable. Typically, DAMPs and PAMPs trigger a robust but short-lived response (lasting hours or days), resulting in pyroptosis and the high-level secretion of IL-1β and IL-18. This leads to clinical symptoms such as fever and facilitates the clearance of the activation signals, ultimately restoring health. Conversely, activation by age- and lifestyle-related signals usually triggers a weaker and more chronic response (lasting months or years), characterized by continuous low-level secretion of IL-1β and IL-18 without clinical symptoms. This prolonged low-grade inflammation contributes to aging, age-related diseases, and cellular senescence. Noncanonical and alternative activation pathways are not depicted in this description.
It is noted that NLRP3 mRNA levels are 3–5 times lower in resting compared to LPS-primed human primary peripheral blood mononuclear cells. Notably, the levels of pro-IL-1β are significantly lower in unprimed cells, exhibiting a reduction by several orders of magnitude (Awad et al., 2017). Additionally, GSDMD mRNA is upregulated by a factor of 4–5 following LPS priming (Muhammad et al., 2019). This dramatic difference underscores the profound impact of priming on inflammasome activation, highlighting the importance of regulating NLRP3, Il-1β, and GSDMD mRNA levels in controlling this specific inflammasome-mediated innate immune response. Recent research indicates that NLRP3 priming is a complex process involving not only the upregulation of expression but also transcriptional and post-translational modifications, as well as specific protein interactions, which collectively prime NLRP3 for inflammasome formation (reviewed in (Mckee and Coll, 2020)). The above underscores the crucial role of priming in regulating and activating the NLRP3 inflammasome.
2.1.2 Activation of NLRP3 and inflammasome formation
Various stimuli, including pathogens such as bacteria, viruses, and fungi, and signals indicative of cellular distress activate NLRP3. This broad spectrum of activators indicates that NLRP3 activation extends beyond simple direct receptor interactions, often involving more complex, indirect pathways reflective of cytosolic disturbances. For instance, a critical indirect pathway involves potassium efflux, a phenomenon linked to various stressors, including bacterial toxins and viral replication, significantly contributing to NLRP3 inflammasome activation. This mechanism is well-documented across numerous studies, highlighting its pivotal role in the inflammatory response to cellular stress (Mariathasan et al., 2006; Laudisi et al., 2013; Kasper et al., 2018; Da Costa et al., 2019). Additionally, extracellular adenosine triphosphate (ATP), often released by cells under stress or during cell death, can bind to the P2X7 receptor, prompting potassium efflux and subsequent NLRP3 activation. This process underscores the role of ATP as a “danger signal” in the immune response (Solle et al., 2001). Mitochondrial dysfunction, which produces reactive oxygen species (ROS), is another crucial factor in activating the NLRP3 inflammasome. The ROS generated from impaired mitochondria serve as signaling molecules that can initiate or amplify the inflammatory response by activating the NLRP3 inflammasome (Zhou et al., 2011) Moreover, mitochondrial DNA (mtDNA) has been identified as one of the few molecular patterns capable of directly binding to and activating the NLRP3 inflammasome. The release of mtDNA into the cytosol, often due to mitochondrial stress or damage, can trigger an innate immune response through NLRP3 inflammasome activation (Shimada et al., 2012). Figure 1B provides an overview of these triggers.
Upon activation, NLRP3 transforms and oligomerizes with other activated NLRP3 proteins to form large, prion-like structures. These oligomers attract the apoptosis-associated speck-like protein containing a caspase-recruitment domain (ASC) (Bryan et al., 2009). This assembly then recruits pro-caspase-1, leading to its dimerization. Consequently, caspase-1 cleaves itself to generate a fully active protease (Black et al., 1989; Martinon et al., 2002). Activated caspase-1 then processes pro-IL-1β and pro-IL-18 into their active forms and cleaves GSDMD to release Nt-GSDMD, which form pores in the plasma membrane (Mariathasan et al., 2006; Shi et al., 2015). This event allows the release of active IL-1β and IL-18 and leads to an increase in osmotic pressure inside the cells, causing them to swell and burst, resulting in pyroptosis—an inflammatory form of programmed cell death. Typically, the systemic presence of DAMPs or PAMPs can evoke robust immune responses, such as high fever from the secretion of IL-1β, potentially leading to a hyperinflammatory state that can be detrimental (Ong et al., 2017). Conversely, localized activation tends to induce a more subclinical or mild response. In both scenarios, the response abates once the activators and damaged cells are cleared. Figure 1B illustrates the cascade through which NLRP3 is activated by PAMPs and DAMPs, leading to both robust and mild immune responses.
Traditionally, NLRP3 expression and activation were thought to be confined to cells of the innate immune system, such as activated monocytes, dendritic cells, and macrophages, including specialized types like Kupffer cells and microglia (Guarda et al., 2011). However, it is now understood that NLRP3 is expressed and activated across a broad range of cell types, including epithelial cells, endothelial cells, fibroblasts, hepatocytes, adipocytes, cardiomyocytes, skeletal muscle cells, neutrophils, platelets, T-helper cells, cytotoxic T cells, hematopoietic and mesenchymal stem cells, keratinocytes, and neurons (Vandanmagsar et al., 2011; Thinwa et al., 2014; Xia et al., 2014; Boaru et al., 2015; Arbore et al., 2016; Karmakar et al., 2016; Yao et al., 2017; Boursereau et al., 2018; Chen G. et al., 2018; Sand et al., 2018; Von Herrmann et al., 2018; Cornelius et al., 2019; Ershaid et al., 2019; Ahn et al., 2020; Ratajczak et al., 2020; Javaid et al., 2023). While this ubiquity is advantageous for acute responses, it may contribute to adverse effects in chronic conditions and aging.
2.2 Chronic NLRP3 priming and activation drives aging-associated pathologies
Emerging evidence underscores the dual role of NLRP3 inflammasome priming and activation in the progression of age-related diseases. As individuals age, there is an observable increase in both the priming and activation of NLRP3, each contributing uniquely to the inflammatory milieu characteristic of aging. This section delves into the intricacies of these processes, elucidating how age-associated increases in NLRP3 priming and subsequent activation are pivotal in the pathogenesis of various age-related conditions.
2.2.1 An age-related sensitization of NLRP3 expression amplifies low-grade inflammation
Age-related declines in homeostatic mechanisms and dietary accumulations contribute to the increased presence of endogenous components, serving as priming signals for NLRP3. These signals upregulate NLRP3 expression, predominantly through TLR activation, setting the stage for heightened inflammatory responses. Oxidized low-density lipoprotein (oxLDL) immune complexes, for example, enhance the expression of inflammasome-related genes such as IL1B and NLRP3 via CD36 and TLR4 pathways, illustrating the complex interaction between lipid metabolism and inflammation (Rhoads et al., 2017). Similarly, elevated levels of saturated fatty acids (SFAs) are known to prime NLRP3 through TLR4, indicating a dietary link to inflammasome sensitization (Finucane et al., 2015). The pro-inflammatory cytokine TNF-α, whose levels increase with age, contributes to transient and sustained NLRP3 priming, further underscoring the role of chronic inflammation in aging (Franchi et al., 2009). Experimental evidence supports this age-related sensitization: old mice exhibit heightened sensitivity to NLRP3 activators compared to their younger counterparts. Isoflurane, for example, triggers NLRP3 activation leading to cognitive impairment and hippocampal inflammation in aged mice, a response absent in the young (Wang et al., 2018). Similarly, inhalation of the potent NLRP3 activator bleomycin results in lethal outcomes in old WT mice but not in young WT or old NLRP3−/− mice, highlighting the lethal impact of NLRP3 activation in the aged (Stout-Delgado et al., 2016). Furthermore, fibrillar amyloid-β (Aβ) induces IL-1β secretion in microglia isolated from aged, but not young, mice, demonstrating the age-specific activation of NLRP3 by Aβ (Wu et al., 2013). Thus, an increase in NLRP3 priming occurs during aging, which can magnify the response to activation signals and elucidate the heightened vulnerability of older mammals to NLRP3-driven inflammation.
2.2.2 Autologous and dietary sterile NLRP3 activators: Implications for age-related pathologies
Aging is associated with increased self-derived or dietary components, such as misfolded proteins and various types of crystals, that can activate the NLRP3 inflammasome (Hornung et al., 2008). This process leads to chronic, sterile inflammation, which, over years or even decades, contributes to a range of age-related diseases by perpetuating a state of low-grade inflammation (Youm et al., 2013). Figure 1B visually summarizes some of the critical components and their mechanisms of action.
2.2.2.1 Cardiovascular disease (CVD)
The role of NLRP3 in CVD is not to be underestimated. Cholesterol crystals, triggered by metabolic imbalance and high intake of certain fats, activate NLRP3 in peripheral blood mononuclear cells (PBMNCs), a process crucial in atherosclerosis development (Duewell et al., 2010). Cells derived from atherosclerotic plaques show elevated NLRP3 and IL-1β mRNA levels, releasing IL-1β when exposed to LPS and ATP or cholesterol crystals (Paramel Varghese et al., 2016). In animal models, NLRP3 deletion has proven to be a significant preventive measure against atherosclerosis and lesion development (Duewell et al., 2010; Van Der Heijden et al., 2017). NLRP3 activation is also associated with human hypertension and related vascular dysfunction (Dalekos et al., 1997; Grimsgaard et al., 1999; Wen et al., 2011). Experimental interventions silencing NLRP3 in hypertensive rats have shown reduced vascular complications and lower blood pressure, with similar results seen when NLRP3 inhibitors like MCC950 are used (Sun et al., 2017; Krishnan et al., 2019). NLRP3 also plays a role in ischemia-reperfusion injury, affecting outcomes such as infarct and stroke sizes; MCC950 administration in models of arterial occlusion has led to reduced tissue damage (Sandanger et al., 2013; Van Hout et al., 2017; Ismael et al., 2018). Colchicine, a recognized NLRP3 inhibitor traditionally used for gout, has shown the potential to reduce cardiovascular risks in clinical trials, emphasizing NLRP3’s significant role in cardiovascular disease progression and mitigation (Martinez et al., 2015; Nidorf et al., 2020).
2.2.2.2 Obesity-induced insulin resistance and type II diabetes mellitus (DMII)
Aging is intricately linked with an increase in abdominal fat, which not only leads to a heightened release of fatty acids and hormones but also results in an accumulation of adipose tissue macrophages (ATM), fundamentally altering the fat tissue’s physiology (Weisberg et al., 2003). These obesity-induced changes are critical in developing insulin resistance, characterized by a diminished ability of insulin to suppress hepatic glucose production, particularly gluconeogenesis. This inefficiency elevates blood glucose levels, increasing insulin production from pancreatic β-cells. Over time, this compensatory hyperinsulinemia may progress to DMII as β-cells fail to meet the increased insulin demand. At a molecular level, compounds like ceramide, misfolded islet amyloid polypeptides (IAPP), and palmitic acid—significantly increased in altered abdominal fat—are potent activators of the NLRP3 inflammasome, enhancing IL-1β secretion (Ebbesson et al., 2010; Masters et al., 2010; Vandanmagsar et al., 2011; Błachnio-Zabielska et al., 2012). Also, advanced glycation end products (AGEs), resulting from a non-enzymatic reaction between sugars (such as glucose) and proteins or fats, and whose formation can be accelerated by factors such as high blood sugar levels and oxidative stress, can activate NLRP3 (Kong et al., 2017). The impact of NLRP3 inflammasome activation in obesity-induced insulin resistance and DMII is profound. Chronic exposure to IL-1β impairs the ability of human pancreatic islet cells to release insulin in response to glucose, exacerbating glucotoxicity (Maedler et al., 2002). Elevated IL-1β levels are associated with a 3.3-fold increased risk of developing DMII, highlighting its significant role in the disease’s pathogenesis (Spranger et al., 2003). Research also indicates that untreated DMII patients have higher levels of NLRP3 protein in their PBMNC-derived macrophages, which secrete increased levels of IL-1β upon activation (Lee et al., 2013). In experimental models, mice on a high-fat diet develop DMII, but deletion of the NLRP3 gene protects against insulin resistance, pancreatic islet fibrosis, and β-cell death (Vandanmagsar et al., 2011; Youm et al., 2011). Additionally, administering tranilast, an NLRP3 inhibitor, prevents the elevation of fasting glucose levels in these models (Huang et al., 2018). Oral N-acetylcysteine, which acts upstream of NLRP3, ameliorated AGEs-induced pancreatic islet damage and IL-1β formation in mice, similar to the effects observed in NLRP3 knockout models (Kong et al., 2017). Interestingly, NT-0249, a brain-penetrant NLRP3 inhibitor, induced weight loss in obese mice comparable to that caused by semaglutide, an anti-diabetic drug that is also used to treat obesity (Thornton et al., 2024). These findings collectively underscore the crucial role of NLRP3 inflammasome activation in the pathogenesis of insulin resistance and DMII.
2.2.2.3 Cognitive decline and neurodegenerative diseases
Brain aging involves physiological changes such as increased region-specific astrogliosis, marked by alterations in astrocytes’ gene expression, quantity, and structure. These changes, associated with cognitive decline, are prevalent in aging humans and do not necessarily coincide with Aβ or Tau pathology (Bronzuoli et al., 2019; Mohamed et al., 2022). Research in mice indicates that hippocampal astrogliosis, critical for memory, can occur without apparent Aβ or Tau pathology and is exacerbated by a high-fat diet (HFD). Notably, NLRP3 knockout prevented age-related and diet-accelerated astrogliosis and cognitive decline, illustrating NLRP3’s significant role in these processes (Youm et al., 2013). Supporting evidence is that a novel brain-penetrant NLRP3 inhibitor also reverses astrogliosis induced by a high-fat diet (Thornton et al., 2024). Extensive reviews and studies highlight NLRP3’s crucial role in developing neurodegenerative diseases (Heneka et al., 2018). For example, postmortem analyses have revealed increased levels of IL-1β and IL-6 in the hippocampi of aged humans, supporting the inflammatory hypothesis of early Alzheimer’s disease (AD) pathogenesis (Cribbs et al., 2012). Elevated IL-1β levels in the cerebrospinal fluid and peripheral blood are linked to AD and Parkinson’s disease (PD) (Blum-Degen et al., 1995; Ng et al., 2018; Fan et al., 2020). Furthermore, NLRP3 activation by misfolded proteins, such as oligomeric Aβ and aggregated Tau in AD and α-synuclein in PD, underscores the inflammatory component of these diseases (Halle et al., 2008; Codolo et al., 2013; Stancu et al., 2019). Genetic and pharmacological inhibition of NLRP3 in various mouse models shows protection against Aβ plaque formation, Tau hyperphosphorylation, rotenone-induced PD, and related cognitive declines, highlighting NLRP3’s potential as a therapeutic target (Heneka et al., 2013; Dempsey et al., 2017; Martinez et al., 2017; Ising et al., 2019). Overall, these findings emphasize NLRP3’s central involvement in age-related astrogliosis and the progression of neurodegenerative diseases.
2.2.2.4 Inflammatory and degenerative joint diseases
Age is the primary risk factor for developing osteoarthritis (OA) and adult-onset rheumatoid arthritis (RA), with aging leading to the deposition of basic calcium phosphate crystals, such as hydroxyapatite, around joints. This deposition contributes to cartilage calcification and OA progression (Dahaghin et al., 2005; Crowson et al., 2011). These crystals activate the NLRP3 inflammasome, producing IL-1β secretion that exacerbates joint damage. Studies indicate that NLRP3 knockout mice exhibit reduced joint pathology, and NLRP3 inhibitors like MCC950 and colchicine have shown protective effects against cartilage degradation and reduced the need for joint replacements (Jin et al., 2011; Pazar et al., 2011; Ni et al., 2021; Heijman et al., 2023). While often considered a lifestyle-related disease, gout exemplifies NLRP3 activation through monosodium urate (MSU) crystal formation due to purine metabolism dysfunction (Martinon et al., 2006). These crystals trigger the NLRP3 inflammasome, increasing IL-1β release from patients’ PBMCs. Colchicine treatment effectively reduces pain during gout attacks, underscoring its role in managing the condition (Terkeltaub et al., 2010; Mylona et al., 2012). In RA, studies have shown that patients’ blood cells release more IL-1β when activated (Choulaki et al., 2015). Targeting the NLRP3 inflammasome, either through genetic manipulation such as the removal of the A20/TNFAIP3 gene, or using NLRP3 inhibitors like MCC905, significantly lessens joint inflammation and damage in mice, illustrating NLRP3’s critical role in RA (Vande Walle et al., 2014; Guo et al., 2018). Corroborating all this, treatment with an anti-IL-1β antibody in patients with atherosclerosis was associated with significant reductions in reported cases of arthritis, gout and osteoarthritis, compared to those receiving a placebo (Ridker et al., 2017b). Collectively, these findings underscore the pivotal role of NLRP3 in the pathogenesis of inflammatory and degenerative joint diseases.
2.2.2.5 Cancer
As the incidence of cancer increases with age, the majority of malignancies are recognized as age-related diseases (Nih, 2021). The NLRP3 inflammasome plays a central role in the pathogenesis of several cancers. Evidence shows that its inhibition or neutralization of IL-1β significantly impacts carcinogenesis and cancer progression. Research involving NLRP3 knockout mice has demonstrated reduced carcinoma incidence, suggesting that NLRP3 activity is linked to cancer development (Marín-Aguilar et al., 2020). For instance, NLRP3 deficiency in methylcholanthrene (MCA)-induced fibrosarcoma models delayed tumor onset and improved tumor-free survival (Chow et al., 2012). Similar protective effects are observed in breast cancer and melanoma models, where NLRP3 knockout decreased tumor growth, reduced metastasis, and enhanced survival (Van Deventer et al., 2010; Guo et al., 2016). Moreover, the potential of NLRP3 inhibitors such as MCC950 and colchicine in suppressing tumor growth has been demonstrated in experimental models of head and neck squamous cell carcinoma and gastric cancer (Chen L. et al., 2018; Zhang T. et al., 2019). The anti-inflammatory effects of IL-1β knockout have also been demonstrated in breast cancer models, where tumor regression was observed (Kaplanov et al., 2019). In clinical settings, chronic use of colchicine has been associated with decreased cancer incidence (Kuo et al., 2015; Lin et al., 2023). Notably, the clinical use of canakinumab, an anti-IL-1β agent, has shown significant promise, as it reduced the incidence of lung cancer in patients with atherosclerosis, providing a robust clinical validation of the critical role of NLRP3 and IL-1β in cancer pathophysiology (Ridker et al., 2017b).
3 The molecular interplay between NLRP3, aging signaling pathways, and cellular senescence
Central to aging are pathways such as autophagy, which is crucial for removing intracellular debris; mitochondrial homeostasis, which is essential for managing energy and ROS; and hormonal signaling, which is vital for growth and tissue health. Aging also involves DNA damage, telomere shortening, and epigenetic alterations, which undermine genomic integrity and accelerate the decline in health. These processes typically culminate in cellular senescence, marked by halted cell division, functional loss, and the secretion of inflammatory signals, all contributing to stem cell exhaustion and exacerbating aging. Notably, a myriad of aging mechanisms, including inflammaging (driven by cellular debris and misfolded proteins), metaflammation (fueled by excessive nutrient intake, dietary patterns, and gut microbiota dynamics), and garbaging (the failure to clear damaged cellular materials), appear to converge on NLRP3-mediated low-grade inflammation. This suggests a unifying inflammatory basis for aging processes, as proposed by Franceschi et al. (Franceschi et al., 2018), highlighting the profound link between metabolism, inflammation, and aging. However, the intricate relationship between NLRP3 and aging pathways is not easily deciphered. NLRP3 often acts upstream in key aging-related processes, indicating a complex and bidirectional interplay. This intricate relationship underscores NLRP3’s dual role in aging and senescence, which will be further explored in this chapter. Figure 2 provides a visual representation of how NLRP3 influences various molecular pathways involved in aging.
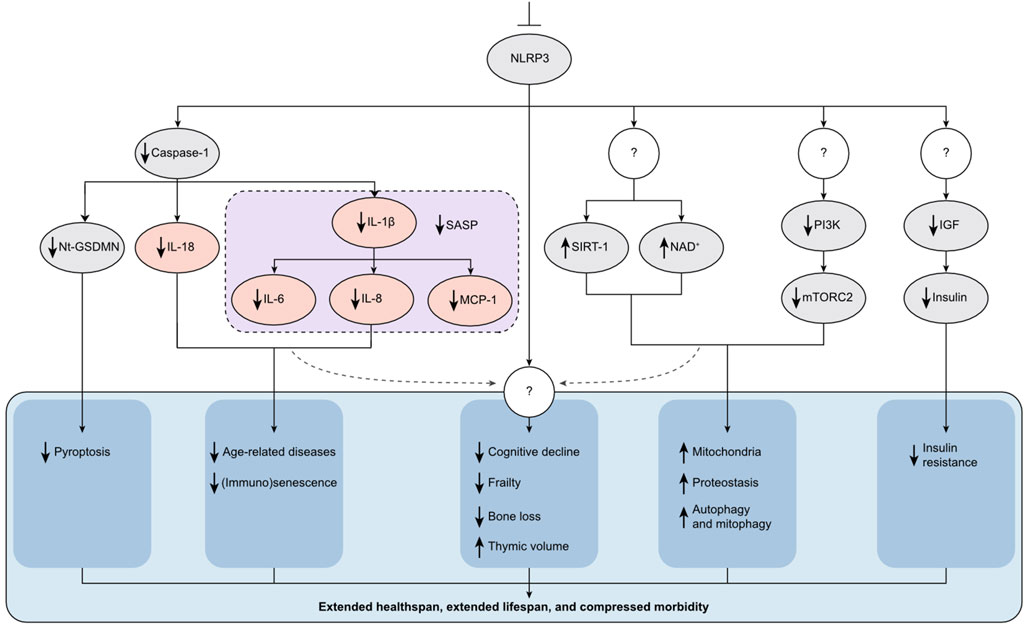
Figure 2. NLRP3’s Role in Aging and Senescence. NLRP3 operates upstream of numerous molecular pathways associated with aging and cellular senescence, presenting a viable target to extend healthspan (HS) and lifespan (LS). Inhibition of NLRP3 through genetic deletion or the use of specific small-molecule inhibitors reduces the production of inflammatory cytokines such as IL-1β, IL-18, IL-6, and TNF-α (illustrated in pink ovals). These cytokines are key contributors to the pathology of age-related diseases and are also part of the senescence-associated secretory phenotype (SASP) secreted by senescent cells. Additionally, blocking NLRP3 mitigates immunosenescence. Furthermore, inhibition of NLRP3 positively influences several metabolic pathways critical to aging. For instance, it elevates levels of SIRT-1 and NAD+, decreases mTORC2 activity, and reduces IGF and insulin levels. Consequently, symptoms of aging such as cognitive decline, frailty, bone loss, thymic involution, and insulin resistance are delayed. This comprehensive modulation of aging pathways results in extended HS and LS, and a reduction in morbidity. The figure also includes question marks to denote steps and mechanisms that remain unknown or not well understood, highlighting areas for future research.
3.1 NLRP3 interactions with key aging mechanisms
Autophagy, a critical cellular clearance mechanism, declines with age, significantly contributing to aging. The mammalian target of rapamycin (mTOR) and AMP-activated protein kinase (AMPK) are two key regulators of autophagy. For instance, mTOR inhibition by rapamycin enhances autophagy, extending HS and LS in mice (Harrison et al., 2009). Autophagy activation also reduces IL-1β production by moderating NLRP3 activation and lowering pro-IL-1β levels (Harris et al., 2011). Conversely, NLRP3 regulates autophagy; its knockout prevents the age-associated decline in autophagy typically seen in older, healthy mice (Marín-Aguilar et al., 2020; Pavillard et al., 2017). Additionally, inhibition of NLRP3 with MCC950 helps forestall the decline in autophagy associated with aging (Pavillard et al., 2017; Marin-Aguilar et al., 2020). AMPK, activated by increased AMP:ATP ratios indicative of ATP consumption, enhances autophagy and mitochondrial function. Caloric restriction (CR), known for extending LS, activates AMPK, underscoring its crucial role in longevity (Slack et al., 2012). However, inhibiting AMPK activates NLRP3 and increases IL-1β levels (Bullon et al., 2016).
Metformin, a diabetes drug under investigation for its potential to extend HS and LS, activates AMPK and inhibits the NLRP3 inflammasome (Zhou et al., 2001; Barzilai et al., 2016; Yang et al., 2019). Similarly, the HS and LS-extending effects observed with CR and metformin treatment mimic those seen in NLRP3 knockout mice, highlighting NLRP3’s pivotal role as a critical regulator in these longevity-enhancing pathways (Bullon et al., 2016; Marín-Aguilar et al., 2020). Moreover, MCC-950 treatment in obese mice increased AMPK phosphorylation levels, further illustrating the intertwined roles of AMPK activation and NLRP3 inhibition in aging (Wang et al., 2022).
Diminished mitochondrial function is a critical contributor to aging. Mitochondrial metabolic responses to diet and exercise are regulated by sirtuins (SIRT1-7), which depend on the coenzyme Nicotinamide adenine dinucleotide (NAD)+. SIRT1 is vital in maintaining cellular homeostasis in various tissues, including the liver, skeletal muscle, adipose tissue, and brain. A decline in NAD+ levels is directly linked to mitochondrial dysfunction. This condition is exacerbated by the deletion of SIRT1, leading to increased production of ROS and subsequent activation of NLRP3 (Zhou et al., 2011; Gomes et al., 2013). Moreover, a decrease in intracellular NAD+ has been associated with NLRP3 activation, whereas NAD+ supplementation has been shown to reduce IL-1β production (Misawa et al., 2013). Mitochondrial dysfunction is a well-documented driver of age-related diseases, which can be mitigated by enhancing mitochondrial function. For instance, elevating SIRT1 expression specifically in the brain has been shown to extend LS in mice (Balaban et al., 2005; Satoh et al., 2013). While whole-body overexpression of Sirt1 improved HS without affecting LS, the use of SRT1720, a selective SIRT1 activator, extended both HS and LS in mice on a standard diet (Herranz et al., 2010; Mitchell et al., 2014). Similarly, nicotinamide mononucleotide (NMN), a precursor of NAD+, boosted mitochondrial oxidative metabolism and enhanced HS in mice (Mills et al., 2016), whereas nicotinamide (NAM) and nicotinamide riboside (NR), other NAD+ precursors, showed varied effects on HS and LS (Mitchell et al., 2018; Harrison et al., 2021). Intriguingly, NLRP3 knockout in aged mice resulted in a significant increase in NAD+, SIRT1, and mitochondrial levels, indicating that NLRP3 acts upstream of processes crucial for mitochondrial function (Marín-Aguilar et al., 2020).
The insulin-like growth factor-1 (IGF-1) pathway is a critical regulator of survival, proliferation, metabolic homeostasis, HS, and LS. For example, CR induces lower levels of insulin and IGF-1, which correlate with improved HS and increased longevity (Breese et al., 1991). Notably, humans exhibiting exceptional longevity have reduced IGF-1 levels, suggesting a link between lower IGF-1 and extended LS (Milman et al., 2014).
The growth hormone (GH)/IGF-1 axis is another pivotal regulator of metabolism and aging. For instance, Ames dwarf mice, which have impaired GH production, exhibit significantly extended LS’s compared to their WT counterparts, while mice with elevated GH levels have reduced LS’s, inversely proportional to their GH plasma levels (Pendergrass et al., 1993; Brown-Borg et al., 1996). GH appears to regulate IGF-1 and NLRP3, as evidenced by GH receptor-knockout (GHRKO) mice. These mice have low serum IGF-1 levels, diminished age-related NLRP3 inflammasome activation in response to NLRP3 ligands, and a 38%–55% longer LS than WT mice (Coschigano et al., 2000; Spadaro et al., 2016). Furthermore, blocking the IGF-1 receptor in aged mice with monoclonal antibodies resulted in a female-specific increase in both HS and LS (Mao et al., 2018). Interestingly, NLRP3 has been shown to regulate IGF-1 levels, as both young and old NLRP3−/− mice exhibit significantly lower IGF-1 levels, a finding further substantiated by reduced serum IGF-1 levels in aged mice treated with the NLRP3 inhibitor MCC950 (Marin-Aguilar et al., 2020; Marín-Aguilar et al., 2020). This positions NLRP3 as an upstream regulator of the IGF-1 signaling pathway. A corroborating finding is that NLRP3 ablation improves glycemic control in aged mice on a standard diet, further highlighting the complex interplay between NLRP3, the GH/IGF-1 axis, and metabolic homeostasis in the context of aging (Youm et al., 2013).
Epigenetic alterations, including DNA methylation, histone modification, and microRNA signaling, are vital mechanisms regulating gene expression by modifying DNA accessibility and chromatin structure without altering the DNA sequence. These changes profoundly influence gene activity and cellular phenotype throughout an organism’s LS. For instance, SIRT1 deficiency leads to hypomethylation at CpG sites on the IL-1β proximal promoter, activating its transcription (Cho et al., 2015). Additionally, IL-1β triggers demethylation at specific sites on the promoters of pro-inflammatory genes IL6 and IL8, increasing their expression and secretion from human intestinal epithelial cells (Caradonna et al., 2018). Furthermore, microRNA-223 (miR-223) overexpression decreases NLRP3 expression and subsequent IL-1β secretion in primed and activated macrophages (Bauernfeind et al., 2012). These examples highlight the complex interplay of regulatory mechanisms in gene regulation.
Telomeres, consisting of tandem repeats at chromosome ends, safeguard against progressive degradation and prevent chromosomal fusion. With each somatic cell division, telomeres naturally shorten, necessitating telomerase activity to maintain their length. Inadequate maintenance leads to telomere dysfunction, which primes for NLRP3 expression and subsequent hyperactivation upon activation (Kang et al., 2018). The role of telomeres extends to aging; their attrition contributes to DNA damage, replicative senescence, and age-related diseases and is inversely correlated with LS (Samper et al., 2001; Artandi and Attardi, 2005). Counteracting this process, telomere extension mitigates DNA damage and senescence, enhances cognitive function, and promotes both HS and LS in mice (Bernardes De Jesus et al., 2012; Muñoz-Lorente et al., 2019). Interestingly, NLRP3 appears to regulate telomerase activity, as evidenced by NLRP3 knockout mice exhibiting elongated telomeres in vivo (Marín-Aguilar et al., 2020), suggesting a role for NLRP3 in the intricate balance between telomere maintenance, cellular aging, and longevity.
Thus, NLRP3 plays a central and multifaceted role in aging through its complex interactions with critical biological processes, including autophagy, mitochondrial function, the GH/IGF-1 axis, epigenetic regulation, and telomere integrity.
3.2 NLRP3 interactions with low-grade inflammation and senescence
As previously discussed, aging is associated with an increase in self-derived and dietary signals, oxidative stress from mitochondrial dysfunction, and elevated cytokines, all of which can prime or activate NLRP3. Concurrently, aging is marked by a subtle rise in inflammatory markers such as IL-6 and C-reactive protein (CRP), associated with frailty and increased mortality in the elderly (Parrinello et al., 2015; Puzianowska-Kuznicka et al., 2016). While IL-1β levels do not consistently increase with age, blocking IL-1β with antibodies reduces IL-6 and CRP levels, confirming IL-1β′s role upstream of these markers (Ridker et al., 2017a). This observation suggests that NLRP3-mediated IL-1β secretion, tightly regulated due to its potent pyrogenic effects, leads to minimal local production in response to the relatively low amount of sterile, age-related activators in healthy individuals. Consequently, systemic levels of IL-1β may stay near or below detection limits. However, its effect is amplified by the downstream production of IL-6 and CRP, which are easily detectable and thus serve as sentinels or surrogate markers for NLRP3-mediated low-grade inflammation. A meta-analysis of clinical trials with coronary artery patients revealed that colchicine intervention was associated with reducted IL-6 and hs-CRP levels (Pan et al., 2022). Additionally, a placebo-controlled trial involving the oral administration of a high dose of GlyNAC—a combination of glycine (Gly) and n-acetyl-L-cysteine (NAC), which increases intracellular glutathione and reduces oxidative stress—showed significant reductions in IL-6 and hs-CRP levels, alongside increased insulin sensitivity, gait speed, and muscle strength in healthy individuals over age 60 (Kumar et al., 2023). Others have found that intracellular glutathione acts as a negative regulator of the NLRP3 inflammasome (Zhang et al., 2021). This discovery provides a surprising link to the complex interplay of aging mechanisms through NLRP3.
Intriguingly, the characteristics of low-grade inflammation significantly overlap with those of age-related senescence. This overlap is evident when considering the senescence-associated secretory phenotype (SASP), characterized by the secretion of pro-inflammatory cytokines and chemokines such as IL-1α, IL-1β, IL-6, IL-8, and MCP-1. Senescent cells, often called ‘zombie cells,’ play a dual role in health and aging. While they can contribute significantly to tumor suppression, during aging these cells secrete compounds that disrupt normal organ and tissue functions. This activity propels age-related pathologies and contributes to a decline in overall health, potentially shortening HS (Baker et al., 2011). Both IL-1α and IL-1β are essential for inducing SASP, as inhibiting the binding of either cytokine to the IL-1 receptor (IL-1R) blocks SASP development (Lau et al., 2019). Since active caspase-1 is necessary to produce IL-1β, and its inhibition reduces SASP, coupled with the fact that senescent cells secrete IL-1β, it suggests that the NLRP3 inflammasome is a likely driver of SASP development (Acosta et al., 2013; Latz and Duewell, 2018). Further evidence of NLRP3’s role comes from studies showing its overexpression exacerbates the senescent phenotype in inherently senescent primary mesenchymal stromal cells from patients with myelodysplastic syndrome by increasing IL-1β secretion (Shi et al., 2019). Compellingly, treatment with IL-1β alone is sufficient to induce senescence in human umbilical vein endothelial cells (HUVECs), vascular smooth muscle cells (VSMCs), and mesenchymal stromal cells (Yin et al., 2017; Shang et al., 2020). Moreover, IL-1β has been identified as the sole cytokine capable of driving cellular aging by inducing senescence (Han et al., 2020). This senescence-inducing effect of IL-1β is counteracted by the NLRP3 inhibitor MCC950, which has shown efficacy in preventing senescence in chondrocytes and endothelial cells, highlighting potential therapeutic avenues to mitigate senescence (Ni et al., 2021; Romero et al., 2022). Targeted NLRP3 inhibition, whether through short hairpin RNA or MCC950 treatment, has shown promise in reducing radiation-induced and IL-1β-induced senescence in diverse cell types, including U87 human glioblastoma cells and HUVECs (Li and Liu, 2015; Romero et al., 2022). In another study, colchicine prevented ethanol-induced senescence in human endothelial cells (Zhou et al., 2023).
The role of NLRP3 in immunosenescence, which decreases immune system effectiveness and raises susceptibility to infections in the elderly, is particularly noteworthy. Aged NLRP3−/− mice show significant resistance to age-related thymic decline and support a more diverse T-cell repertoire, indicating a potential protective effect against immunosenescence (Youm et al., 2012). Given NLRP3’s established role in promoting senescence and SASP, the emerging concept that NLRP3 activation does not invariably lead to pyroptosis warrants further exploration. This notion is pivotal as senescent cells, which secrete NLRP3-mediated SASP components, can remain viable for extended periods in vitro (De Cecco et al., 2013; Kim et al., 2013; Yin et al., 2017). Such longevity would be implausible if pyroptosis were an inevitable outcome of NLRP3 activation in every senescent cell, suggesting that alternative pathways might be operational. Interestingly, the nature of the activating signals influences NLRP3 inflammasome outcomes significantly. Sterile signals generate weaker and more delayed macrophage responses than microbial signals, leading to lower and prolonged IL-1β secretion and reduced pyroptosis rates. This difference is particularly pronounced in murine cells, where a double sterile signal leads to substantially lower pyroptosis levels than a double microbial signal. However, both types of signals result in comparably low pyroptosis levels in human primary macrophages, indicating species-specific differences in NLRP3-induced pyroptosis kinetics (Bezbradica et al., 2017). Furthermore, a hyperactive cellular state has been identified where NLRP3-dependent IL-1β release can occur without pyroptosis. For instance, the removal of the Sterile alpha and HEAT Armadillo motif-containing protein (SARM) containing a Toll-IL-1R (TIR) domain drives cellular hyperactivation, allowing cultured cells to secrete IL-1β for days while remaining viable (Wolf et al., 2016; Zanoni et al., 2017; Evavold et al., 2018). An alternative pathway involves priming human monocytes with a TLR4 ligand (LPS) without an activation signal. This scenario leads to chronic, low-level NLRP3-mediated IL-1β secretion without inducing cell death, a process that can be inhibited by MCC950 (Gaidt et al., 2016). Notably, IL-1β production through this alternative activation pathway is reduced by approximately one order of magnitude compared to that observed with canonical activation pathways. Moreover, it does not trigger pyroptosis. This suggests that such alternative inflammasome pathways could significantly contribute to age-related low-grade inflammation, wherein IL-1β levels are modestly but chronically elevated. Figure 1B visually represents such non-canonical transport mechanisms for chronic, pyroptosis-free IL-1β secretion.
Thus, NLRP3 plays a pivotal role in orchestrating cellular and immunosenescence, underscoring its crucial significance in aging.
4 Targetting NLRP3 to extend HS
Youm et al. were the first to hypothesize that reducing NLRP3 may extend HS by delaying multiple age-related degenerative changes in the periphery and the brain. Their seminal study showed that NLRP3 ablation prevented thymic involution, brain astrogliosis, bone loss, and cognitive decline. Additionally, NLRP3−/− mice showed increased latency to fall on a rotarod and increased running time and distance on a treadmill. Overall, the deletion of NLRP3 extended HS by preventing functional decline in multiple organs (Youm et al., 2013). Subsequent studies further supported the notion that a reduction in NLRP3 could extend both HS and LS. For instance, treating 18-month-old mice with MCC950 for 12 weeks improved their health. Compared to their sham-treated littermates, autophagy, and glucose tolerance were increased, age-related hepatic dysfunction was ameliorated, and fasting blood glucose and circulating IGF-1 levels, as well as body weight, were reduced (Marin-Aguilar et al., 2020). Additional studies confirmed the HS-extending effects of NLRP3 ablation. Older mice typically appeared gray and frail with alopecic fur, whereas knockout mice remained vital with thick, dark, and shiny fur. NLRP3−/− mice also exhibited preserved cardiac integrity and reduced metabolic aging. Remarkably, male and female NLRP3−/− mice showed increases in their mean and maximum LS by 34% and 29%, respectively (Marín-Aguilar et al., 2020). Another study revealed that approximately 70% of twenty-month-old WT mice on a high-fat diet developed ulcerating dermatitis, a severe inflammatory skin disorder, in contrast to only 5% of twenty-month-old NLRP3−/− mice. Moreover, the LS of high-fat diet-fed NLRP3−/− mice was 16% higher than that of their WT counterparts on the same diet and comparable to the LS of WT mice fed a standard diet (Cañadas-Lozano et al., 2020). Further evidence from a progeria mouse model showed that MCC950 treatment to inhibit NLRP3 prevented age-related weight loss and extended mean LS by 19.2% (González-Dominguez et al., 2021). Additionally, bats, known for their extraordinary longevity, exhibit a unique bat-specific splice variant and an evolutionarily adapted LRR domain of NLRP3, thought to dampen the inflammatory response and contribute to their health and longevity by reducing chronic inflammation (Austad and Fischer, 1991; Kacprzyk et al., 2017; Ahn et al., 2019). This observation is supported by findings in long-living naked mole rats, who display a notably dampened immune response, further associating reduced inflammation with extended HS and LS (Oka et al., 2022)
In most humans, an increase in age is accompanied by an elevation in the levels of pro-inflammatory proteins or biomarkers, which are linked to age-related diseases and increased mortality (Cesari et al., 2004). Lower levels of IL-6 are observed in healthy agers—individuals over age 65 who are free from disease, have no disabilities, and maintain good cognition—and seem to predict a prolonged LS. A study of these healthy agers, with a mean age of 76.3 ± 7.9 years, demonstrated that those in the lowest quartile of IL-6 levels exhibited a sevenfold decrease in 5-year mortality rate compared to those in the highest quartile (Puzianowska-Kuznicka et al., 2016). While lower IL-6 levels are linked to extended LS in healthy agers, IL-1β, regulated by the NLRP3 inflammasome, appears to play a crucial upstream role. Research shows that blocking IL-1β reduces IL-6 levels in humans, indicating that IL-1β initiates IL-6 production (Lachmann et al., 2009; Ridker et al., 2017a).
As described in chapter 3, increased NLRP3 priming and activation are closely associated with age-related diseases. However, the role of NLRP3 extends beyond these conditions, influencing the HS preceding the onset of disease. This is supported by findings that activated monocytes from elderly individuals express three times as much NLRP3 as those from younger individuals. Notably, healthy centenarians exhibit NLRP3 levels similar to young people, in contrast to their diseased counterparts, who display levels akin to those of older individuals with health conditions (Tedone et al., 2019). Significantly, a study on 80-year-old master athletes (MA), who engage in rigorous training for approximately 14 h per week, offers a compelling real-life illustration. These MA individuals demonstrate greater muscle mass and superior cardiovascular fitness, muscle strength, and muscle volume compared to their non-athletic (NA) counterparts of the same age. Muscle biopsies from the NA group revealed elevated levels of proteins involved in NLRP3 priming and activation (NF-kB, PYCARD (ASC), and NF-kB), which were significantly lower in the MA group. This suggests that the inflammasome proteins that typically increase with aging are mitigated in physically active elderly subjects (Ubaida-Mohien et al., 2022). Interestingly, the inhibition of NLRP3 seems to play a role in the HS- and LS-extending effects of the ketogenic diet developed to treat therapy-resistant epilepsy. The underlying mechanism for its anti-aging effect is largely unknown. A ketogenic diet, which consists of more than 85% fat and involves the formation of ketone bodies such as β-hydroxybutyrate (BHB) as an alternative energy source for ATP, was shown to extend the HS and LS of mice (Roberts et al., 2017). BHB lowers IL-1β levels by blocking the NLRP3-mediated inflammasome formation, which could explain its positive effect on aging (Youm et al., 2015). In addition to its verified effect in treating epilepsy in children, an increasing body of evidence suggests that a ketogenic diet can extend HS by preventing diseases such as DMII and AD (Goday et al., 2016; Taylor et al., 2018). These observations collectively suggest that NLRP3 expression correlates with the presence of age-related diseases and significantly impacts the HS of aging populations. Despite NLRP3’s central role in aging, a clear correlation between aging and an increase in systemic IL-1β levels has not yet been established. This is likely because IL-1β levels are typically extremely low in human serum. In patients with CAPS, who overproduce IL-1β, serum levels still fall below the detection limit of 0.1 pg/mL for the assays used (Lachmann et al., 2009). In another study, serum IL-1β levels in healthy individuals were found to average 0.2 pg/mL, barely above the detection limit of another ultra-sensitive ELISA assay. This consistency across different age groups led researchers to conclude there was no significant association between age and IL-1β levels (Di Iorio et al., 2003). However, the lack of independent replications, the observation of higher serum IL-1β levels compared to those in CAPS patients, and the discontinuation of the previously used assay all warrant caution in interpreting these results, highlighting the need for more accurate IL-1β detection methods. Moreover, based on estimates that healthy individuals and CAPS patients produce approximately 6 ng and 31 ng of IL-1β per day, serum levels should be up to 500 times higher than typically detected (Lachmann et al., 2009).
One explanation for the extremely low systemic presence of IL-1β is that ELISA assays primarily measure free IL-1β. In serum, IL-1β is neutralized by soluble IL-1 receptors, such as Soluble IL-1 Receptor Types I and II (sIL-1RI and sIL-1RII), which are present at concentrations up to 1000 times higher than those of IL-1β, effectively rendering it biologically inactive. (Symons et al., 1995; Lachmann et al., 2009). Consequently, these soluble receptors act as a buffer, neutralizing the levels of IL-1β that are typically produced in healthy individuals and CAPS patients. Given that the soluble IL-1 receptors effectively neutralize IL-1β levels, resulting in indistinguishable systemic IL-1β concentrations between CAPS patients—who typically overproduce this cytokine—and healthy individuals, it is even less likely to observe differences in IL-1β levels among healthy individuals across different age groups. Therefore, different methods are required to accurately reflect local IL-1β production, such as assays that measure total IL-1β in serum. Alternatively, IL-6 can be used as a sentinel biomarker for local IL-1β production, as suggested by Hoffman et al. (Hoffman et al., 2004). Together, these studies provide compelling evidence that NLRP3 is crucial for healthy aging. However, they also highlight that serum IL-1β, as measured by current methodologies, does not serve as a reliable biomarker for its activation or lack thereof.
The quest for longevity has historically focused on identifying compounds that extend LS in model organisms such as mice. However, translating these findings to humans presents substantial challenges, primarily due to the impracticality of LS studies in humans and the growing realization that extending HS—ensuring longer periods of good health rather than merely prolonging the duration of disease—is fundamentally more important. Therefore, recent scientific efforts have pivoted towards extending HS—the period of life spent in good health—in larger mammals and humans. Given the ethical and logistical constraints of conducting LS-extending studies in healthy humans, this shift is not only pragmatic but also necessary. Current regulations typically restrict the use of experimental drugs to individuals diagnosed with a disease. This regulatory environment complicates interventions aimed at healthy individuals to prolong their HS. As a result, alternative approaches, such as using nutritional supplements, are being explored. In this context, the recently announced XPRIZE Healthspan of €101 million marks a significant incentive (XPRIZE, 2023). The prize will be awarded to the first who can demonstrably reverse specific aging markers—such as declines in muscle strength, cognition, and immune function—by at least a decade. While the precise methodologies, or endpoints, for measuring these reversals are still being refined, the strategy of incrementally reversing aging represents a highly achievable short-term goal. Focusing on reversing specific aging symptoms, rather than extending lifespan, appears to be a pragmatic and attainable objective, which could potentially accelerate developments in the field of health extension.
This section delves into the therapeutic strategies in development that target the NLRP3 inflammasome, either directly or indirectly—first some promising studies in mice that require confirmation in human trials. Rapamycin, which stimulates autophagy by inhibiting mTOR, has been shown to extend the LS of old mice in the rigorous Interventions Testing Program (ITP) (Harrison et al., 2009). Additionally, the autophagy induced by rapamycin inhibits NLRP3 activation and subsequent secretion of IL-1β in a human macrophage cell line (Ko et al., 2017). Treatment with nicotinamide riboside (NR), a variant of vitamin B3, replenishes nicotinamide adenine dinucleotide (NAD+) levels and improves mitochondrial function, thereby prolonging the LS of old mice (Zhang et al., 2016). In a mouse model for cardiac hypertrophy, NR treatment attenuated NLRP3 activation and reduced IL-1β levels (Ma et al., 2021). Nicotinic acid, another form of vitamin B3, enhances NAD+ levels and inhibits NLRP3 activation (Li et al., 2016). Spermidine, a natural polyamine, improves the LS of old mice by promoting autophagy and reducing inflammation (Eisenberg et al., 2016). In a human macrophage cell line, spermidine inhibited NLRP3 inflammasome activation and IL-1β production (Li et al., 2022).
Studies conducted in non-human primates and humans are more directly relevant to human health. For instance, taurine, a semi-essential food ingredient, exhibits significant declines in levels with aging across mice, monkeys, and humans, which is associated with age-related diseases. In aged rhesus macaques (Macaca mulatta) supplemented with taurine, notable improvements were observed: increased bone density, reduced fasting blood glucose levels, and lowered serum concentrations of liver damage markers AST and ALT. Additionally, taurine treatment decreased WBCs, monocytes, and granulocytes, which typically increase with age (Singh et al., 2023). In rodents, taurine supplementation has been shown to alleviate age-related diseases by inhibiting NLRP3 activation and reducing IL-1β production (Lee et al., 2021; Qiu et al., 2018).
Metformin, commonly used to manage DMII by reducing glucose production and enhancing insulin sensitivity, offers benefits beyond glycemic control. It is associated with the prevention of CVD, a reduced incidence of cancer, improved cognitive functions, and decreased mortality rates among patients with DMII. Additionally, metformin lowers IGF-1 levels, inhibits mTOR, reduces activity in mitochondrial complex 1 of the electron transport chain, and decreases the endogenous production of ROS. It also activates AMP-activated protein kinase (AMPK), reduces inflammation and may delay senescence (reviewed in (Mohammed et al., 2021). A large clinical trial is underway to establish whether metformin extends the HS of healthy individuals over age 65 (Barzilai et al., 2016). The broad spectrum of metformin’s effects raises the question of whether these benefits are due to multiple mechanisms or the downstream consequences of its primary action on a singular aging-related pathway. Given that metformin also acts as an NLRP3 inhibitor, it is plausible that many, if not all, of these beneficial effects are mediated through this pathway (Zhang L. et al., 2019; Xian et al., 2021).
Age-related declines in the amino acids glycine (Gly) and cysteine (Cys) and the antioxidant glutathione (GSH)—the primary building blocks of which are Gly and Cys—are observed in both mice and humans. These declines are associated with aging and various age-related diseases (Yang et al., 1995; Lyons et al., 2000; Sekhar et al., 2011). GSH, a crucial regulator of mitochondrial health, neutralizes ROS. Its levels can be replenished through the oral administration of Gly and NAC, an acetylated form of Cys known for enhanced oral bioavailability (Samiec et al., 1998; Townsend et al., 2003; Sekhar et al., 2011). In experimental models, Gly alone has been shown to modestly extend the LS of mice in ITP studies (Miller et al., 2019). Furthermore, the oral intake of the combination of Gly and NAC (GlyNAC) has demonstrated a significant 24% increase in the LS of aged mice (Kumar et al., 2022). In healthy individuals over age 70, oral NAC administration significantly improved muscular performance compared to placebo-treated, age-matched controls (Hauer et al., 2003). The administration of GlyNAC to healthy individuals over age 60 significantly improved muscle strength, insulin resistance, and systolic blood pressure. Furthermore, biomarkers of endothelial dysfunction (sVCAM-1), oxidative stress (TBARS), and inflammation (IL-6) showed significant improvements compared to placebo controls (Kumar et al., 2023). In a non-controlled pilot study, GlyNAC significantly improved cognition (Kumar et al., 2021). Traditionally, GSH is known as a ROS scavenger that indirectly modulates NLRP3 activation. However, recent findings reveal that GSH also directly regulates NLRP3 activation. ATP-induced GSH depletion in macrophages triggers NLRP3 inflammasome activation, likely by destabilizing the thioredoxin-interacting protein (TXNIP)-thioredoxin (TRX) complex, thereby freeing TXNIP to activate NLRP3. Furthermore, the exogenous administration of GSH or its oxidized form, GSSG, has been found to reduce IL-1β production and inhibit NLRP3 inflammasome activation significantly (Zhang et al., 2021). These findings not only clarify GSH’s comprehensive role in inflammatory regulation but also illuminate its potential as a promising therapeutic target for managing NLRP3-mediated conditions. Further supporting the significance of glutathione in aging, research indicates that HC exhibit higher GSH:GSSG (GSH disulfide) ratios and total GSH levels compared to individuals aged 70-79. This suggests that elevated glutathione levels may protect against oxidative stress and NLRP3 activation, contributing to these individuals’ exceptional HS and LS (Paolisso et al., 1998; Alonso-Fernández et al., 2008).
Thus, NLRP3 plays a critical role in modulating both HS and LS. Given that self-derived signals primarily drive it, the term ‘auto-aging’ is proposed to describe the accelerated aging mediated by NLRP3 in the broader population. As research progresses, the potential of targeting this inflammasome to mitigate age-related diseases and significantly enhance the quality of life during aging becomes increasingly promising.
5 Conclusion and future directions
Given that HC achieve an exceptional HS of at least one hundred years and considering the mounting evidence of their genetic advantages that protect them from age-related diseases, this review makes clear that the vast majority of the population experiences of form of accelerated aging. With the average HS of the broader population falling short by approximately 30 years, a pivotal challenge emerges: identifying these beneficial genetic traits and determining which can be targeted to enhance the HS of the general public. While the aging process remains complex, emerging research continues to illuminate the critical mechanisms involved. NLRP3, a protein primarily known for its role in innate immunity and its significant contributions to age-related diseases, is central to this phenomenon of accelerated aging. However, this does not exclude the involvement of other mechanisms that have a central role in aging. Recently, IL-11 was found to play a central role in mouse aging, with blocking IL-11 extending the LS and HS of aged mice by 24.9% (Widjaja et al., 2024). Today, the biological function of IL-11 remains poorly understood and its role has long been confusing. Recombinant human (rh) IL-11 has shown protective effects against age-related diseases in mice, whereas recombinant murine IL-11 causes age-related diseases (reviewed in Cook, 2023). Cook’s review also shows that there is no evidence that rhIL-11 harms human HS, as it remains an effective treatment for thrombocytopenia. Additionally, clinical studies with rhIL-11 antibodies in humans have not shown favorable outcomes on age-related diseases. Therefore, the role of IL-11 in human aging needs further investigation to determine if the effects observed in mice are species-specific.
This paper presents a novel perspective, amassing substantial evidence that targeting NLRP3 not only converges various aging mechanisms but also exerts regulatory control over them. This unique approach underscores a sophisticated interdependence that is yet to be fully understood. Furthermore, NLRP3 appears to regulate cellular senescence so that its activation does not lead to pyroptosis. This suggests that senescent cells could be a persistent source of IL-1β production, thereby contributing to aging. The significance of NLRP3 in aging is highlighted by studies demonstrating that its knockout endows mice with a phenotype akin to HC, extending their HS and LS (Youm et al., 2013; Marín-Aguilar et al., 2020). Additionally, treatments targeting pathways upstream of NLRP3 have been shown to extend the HS of primates and reverse various aging symptoms in humans (Kumar et al., 2023; Singh et al., 2023). Therefore, NLRP3 is not merely a participant in the aging process but potentially acts as a master regulator. Modulating NLRP3 could significantly alter the health trajectories of individuals experiencing NLRP3-mediated accelerated aging. Since this process is largely driven by autologous components, the term ‘auto-aging’ is proposed. Further research is essential to understand the role of NLRP3 in accelerated aging entirely and to develop healthspan-extending therapies targeting this key regulator. A critical question remains: Should interventions aim to completely inhibit NLRP3 activation or selectively target specific activation pathways to maximize health benefits? While there appears to be redundancy among NLRs in defending against PAMPs, broad inhibition might increase susceptibility to specific infections by weakening primary defense mechanisms (Coll et al., 2016). Additionally, better biomarkers are needed to gauge the impact of such therapies on NLRP3 activity. Assessments often focus on free IL-1β levels in plasma, which are minimal due to neutralization by sIL-Rs. Alternatives could include measuring total IL-1β or utilizing surrogate markers like IL-6 downstream of IL-1β, which may provide a more accurate reflection of NLRP3-mediated inflammatory status.
Focusing on reversing age-related symptoms, as highlighted by the XPRIZE Healthspan competition, is an effective strategy to advance this emerging field. RCTs aimed at studying HS extension can be prohibitively expensive and lengthy and may also pose ethical challenges since they involve healthy participants. Conversely, RCTs that target the reversal of common aging symptoms can bypass many of these issues. This approach could catalyze the development of new treatments designed to mitigate the detrimental effects of aging, potentially leading to rapid advancements in the exciting and emerging field of health extension.
Lastly, will health extension be achieved through pharmaceutical treatments, or could it be managed with supplements? Pharmaceuticals are traditionally targeted at treating diseases and cannot be prescribed to healthy individuals. There are proposals to classify healthy people of a certain age as ‘de facto’ diseased to circumvent this issue. However, even if regulatory bodies accept this classification, the question remains: at what age should this apply? A conservative approach would likely set this age at 70 or older. For optimal effectiveness, healthspan-extending interventions might need to start earlier, which means that pharmaceutical treatments would be delayed until individuals are already experiencing a decline, potentially reducing their efficacy and benefits. Moreover, the costs of such treatments and the likelihood that insurers may not cover a universally applicable intervention are significant considerations.
Supplements, on the other hand, hold promise in addressing several of these challenges. They could be a viable solution, albeit with their own restrictions. Dietary supplements can be marketed to improve specific functions or to reduce disease risk factors, such as improving muscle strength or lowering cholesterol, which are considered health claims. However, making claims about reducing risks associated with aging will likely be challenging, as aging is not (yet) classified as a disease. Additionally, it is still being determined whether claims combining multiple benefits (e.g., improving muscle strength and cognition) can be substantiated and approved. For a supplement to carry a specific health claim, it must undergo development with the same rigor as a pharmaceutical, including obtaining pre-market approval. Moreover, if one or more bioactive ingredients have not been used previously in food, they must be developed and approved as novel foods to ensure they are non-toxic. However, these processes can be completed much faster and at significantly lower cost, resulting in more affordable product pricing. This affordability might help overcome the barrier of supplements being unlikely to be reimbursed.
Overall, the convergence of scientific breakthroughs and innovations is not just a trend, but a transformative force that is steadily bringing the prospect of exceptional health significantly closer. This exciting progress is not just a dream, but a reality that we are inching closer to. The ability to reverse aging symptoms in humans is seen as a crucial stepping stone toward the audacious goal of extending HS for everyone.
Author contributions
SV: Writing–original draft, Writing–review and editing.
Funding
The author(s) declare that no financial support was received for the research, authorship, and/or publication of this article.
Conflict of interest
SV is the founder and owner of YOXLO BV, a research-stage biotechnology company developing health extenders.
Publisher’s note
All claims expressed in this article are solely those of the authors and do not necessarily represent those of their affiliated organizations, or those of the publisher, the editors and the reviewers. Any product that may be evaluated in this article, or claim that may be made by its manufacturer, is not guaranteed or endorsed by the publisher.
References
Acosta, J. C., Banito, A., Wuestefeld, T., Georgilis, A., Janich, P., Morton, J. P., et al. (2013). A complex secretory program orchestrated by the inflammasome controls paracrine senescence. Nat. Cell. Biol. 15, 978–990. doi:10.1038/ncb2784
Ahn, J. S., Seo, Y., Oh, S. J., Yang, J. W., Shin, Y. Y., Lee, B. C., et al. (2020). The activation of NLRP3 inflammasome potentiates the immunomodulatory abilities of mesenchymal stem cells in a murine colitis model. BMB Rep. 53, 329–334. doi:10.5483/BMBRep.2020.53.6.065
Ahn, M., Anderson, D. E., Zhang, Q., Tan, C. W., Lim, B. L., Luko, K., et al. (2019). Dampened NLRP3-mediated inflammation in bats and implications for a special viral reservoir host. Nat. Microbiol. 4, 789–799. doi:10.1038/s41564-019-0371-3
Alexopoulou, L., Holt, A. C., Medzhitov, R., and Flavell, R. A. (2001). Recognition of double-stranded RNA and activation of NF-kappaB by Toll-like receptor 3. Nature 413, 732–738. doi:10.1038/35099560
Alonso-Fernández, P., Puerto, M., Maté, I., Ribera, J. M., and De La Fuente, M. (2008). Neutrophils of centenarians show function levels similar to those of young adults. J. Am. Geriatr. Soc. 56, 2244–2251. doi:10.1111/j.1532-5415.2008.02018.x
Andersen, S. L., Sebastiani, P., Dworkis, D. A., Feldman, L., and Perls, T. T. (2012). Health span approximates life span among many supercentenarians: compression of morbidity at the approximate limit of life span. J. Gerontol. A Biol. Sci. Med. Sci. 67, 395–405. doi:10.1093/gerona/glr223
Arbore, G., West, E. E., Spolski, R., Robertson, A. a. B., Klos, A., Rheinheimer, C., et al. (2016). T helper 1 immunity requires complement-driven NLRP3 inflammasome activity in CD4⁺ T cells. Science 352, aad1210. doi:10.1126/science.aad1210
Artandi, S. E., and Attardi, L. D. (2005). Pathways connecting telomeres and p53 in senescence, apoptosis, and cancer. Biochem. Biophys. Res. Commun. 331, 881–890. doi:10.1016/j.bbrc.2005.03.211
Austad, S. N., and Fischer, K. E. (1991). Mammalian aging, metabolism, and ecology: evidence from the bats and marsupials. J. Gerontol. 46, B47–B53. doi:10.1093/geronj/46.2.b47
Awad, F., Assrawi, E., Jumeau, C., Georgin-Lavialle, S., Cobret, L., Duquesnoy, P., et al. (2017). Impact of human monocyte and macrophage polarization on NLR expression and NLRP3 inflammasome activation. PLoS One 12, e0175336. doi:10.1371/journal.pone.0175336
Baker, D. J., Wijshake, T., Tchkonia, T., Lebrasseur, N. K., Childs, B. G., Van De Sluis, B., et al. (2011). Clearance of p16Ink4a-positive senescent cells delays ageing-associated disorders. Nature 479, 232–236. doi:10.1038/nature10600
Balaban, R. S., Nemoto, S., and Finkel, T. (2005). Mitochondria, oxidants, and aging. Cell. 120, 483–495. doi:10.1016/j.cell.2005.02.001
Barzilai, N., Crandall, J. P., Kritchevsky, S. B., and Espeland, M. A. (2016). Metformin as a tool to target aging. Cell. Metab. 23, 1060–1065. doi:10.1016/j.cmet.2016.05.011
Bauernfeind, F., Rieger, A., Schildberg, F. A., Knolle, P. A., Schmid-Burgk, J. L., and Hornung, V. (2012). NLRP3 inflammasome activity is negatively controlled by miR-223. J. Immunol. 189, 4175–4181. doi:10.4049/jimmunol.1201516
Bauernfeind, F. G., Horvath, G., Stutz, A., Alnemri, E. S., Macdonald, K., Speert, D., et al. (2009). Cutting edge: NF-kappaB activating pattern recognition and cytokine receptors license NLRP3 inflammasome activation by regulating NLRP3 expression. J. Immunol. 183, 787–791. doi:10.4049/jimmunol.0901363
Bernardes De Jesus, B., Vera, E., Schneeberger, K., Tejera, A. M., Ayuso, E., Bosch, F., et al. (2012). Telomerase gene therapy in adult and old mice delays aging and increases longevity without increasing cancer. EMBO Mol. Med. 4, 691–704. doi:10.1002/emmm.201200245
Bezbradica, J. S., Coll, R. C., and Schroder, K. (2017). Sterile signals generate weaker and delayed macrophage NLRP3 inflammasome responses relative to microbial signals. Cell. Mol. Immunol. 14, 118–126. doi:10.1038/cmi.2016.11
Błachnio-Zabielska, A. U., Pułka, M., Baranowski, M., Nikołajuk, A., Zabielski, P., Górska, M., et al. (2012). Ceramide metabolism is affected by obesity and diabetes in human adipose tissue. J. Cell. Physiol. 227, 550–557. doi:10.1002/jcp.22745
Black, R. A., Kronheim, S. R., Merriam, J. E., March, C. J., and Hopp, T. P. (1989). A pre-aspartate-specific protease from human leukocytes that cleaves pro-interleukin-1 β. J. Biol. Chem. 264, 5323–5326. doi:10.1016/s0021-9258(18)83546-3
Blum-Degen, D., Müller, T., Kuhn, W., Gerlach, M., Przuntek, H., and Riederer, P. (1995). Interleukin-1 beta and interleukin-6 are elevated in the cerebrospinal fluid of Alzheimer's and de novo Parkinson's disease patients. Neurosci. Lett. 202, 17–20. doi:10.1016/0304-3940(95)12192-7
Boaru, S. G., Borkham-Kamphorst, E., Van De Leur, E., Lehnen, E., Liedtke, C., and Weiskirchen, R. (2015). NLRP3 inflammasome expression is driven by NF-κB in cultured hepatocytes. Biochem. Biophys. Res. Commun. 458, 700–706. doi:10.1016/j.bbrc.2015.02.029
Boursereau, R., Abou-Samra, M., Lecompte, S., Noel, L., and Brichard, S. M. (2018). Downregulation of the NLRP3 inflammasome by adiponectin rescues Duchenne muscular dystrophy. BMC Biol. 16, 33. doi:10.1186/s12915-018-0501-z
Breese, C. R., Ingram, R. L., and Sonntag, W. E. (1991). Influence of age and long-term dietary restriction on plasma insulin-like growth factor-1 (IGF-1), IGF-1 gene expression, and IGF-1 binding proteins. J. Gerontol. 46, B180–B187. doi:10.1093/geronj/46.5.b180
Bronzuoli, M. R., Facchinetti, R., Valenza, M., Cassano, T., Steardo, L., and Scuderi, C. (2019). Astrocyte function is affected by aging and not alzheimer's disease: a preliminary investigation in hippocampi of 3xTg-AD mice. Front. Pharmacol. 10, 644. doi:10.3389/fphar.2019.00644
Brown-Borg, H. M., Borg, K. E., Meliska, C. J., and Bartke, A. (1996). Dwarf mice and the ageing process. Nature 384, 33. doi:10.1038/384033a0
Bryan, N. B., Dorfleutner, A., Rojanasakul, Y., and Stehlik, C. (2009). Activation of inflammasomes requires intracellular redistribution of the apoptotic speck-like protein containing a caspase recruitment domain. J. Immunol. 182, 3173–3182. doi:10.4049/jimmunol.0802367
Bullon, P., Alcocer-Gomez, E., Carrion, A. M., Marin-Aguilar, F., Garrido-Maraver, J., Roman-Malo, L., et al. (2016). AMPK phosphorylation modulates pain by activation of NLRP3 inflammasome. Antioxid. Redox Signal 24, 157–170. doi:10.1089/ars.2014.6120
Cañadas-Lozano, D., Marín-Aguilar, F., Castejón-Vega, B., Ryffel, B., Navarro-Pando, J. M., Ruiz-Cabello, J., et al. (2020). Blockade of the NLRP3 inflammasome improves metabolic health and lifespan in obese mice. Geroscience 42, 715–725. doi:10.1007/s11357-019-00151-6
Caradonna, F., Cruciata, I., Schifano, I., La Rosa, C., Naselli, F., Chiarelli, R., et al. (2018). Methylation of cytokines gene promoters in IL-1β-treated human intestinal epithelial cells. Inflamm. Res. 67, 327–337. doi:10.1007/s00011-017-1124-5
Cesari, M., Penninx, B. W., Pahor, M., Lauretani, F., Corsi, A. M., Rhys Williams, G., et al. (2004). Inflammatory markers and physical performance in older persons: the InCHIANTI study. J. Gerontol. A Biol. Sci. Med. Sci. 59, 242–248. doi:10.1093/gerona/59.3.m242
Chen, G., Chelu, M. G., Dobrev, D., and Li, N. (2018a). Cardiomyocyte inflammasome signaling in cardiomyopathies and atrial fibrillation: mechanisms and potential therapeutic implications. Front. Physiol. 9, 1115. doi:10.3389/fphys.2018.01115
Chen, L., Huang, C. F., Li, Y. C., Deng, W. W., Mao, L., Wu, L., et al. (2018b). Blockage of the NLRP3 inflammasome by MCC950 improves anti-tumor immune responses in head and neck squamous cell carcinoma. Cell. Mol. Life Sci. 75, 2045–2058. doi:10.1007/s00018-017-2720-9
Cho, S. H., Chen, J. A., Sayed, F., Ward, M. E., Gao, F., Nguyen, T. A., et al. (2015). SIRT1 deficiency in microglia contributes to cognitive decline in aging and neurodegeneration via epigenetic regulation of IL-1β. J. Neurosci. 35, 807–818. doi:10.1523/JNEUROSCI.2939-14.2015
Chondrogianni, N., Petropoulos, I., Franceschi, C., Friguet, B., and Gonos, E. S. (2000). Fibroblast cultures from healthy centenarians have an active proteasome. Exp. Gerontol. 35, 721–728. doi:10.1016/s0531-5565(00)00137-6
Choulaki, C., Papadaki, G., Repa, A., Kampouraki, E., Kambas, K., Ritis, K., et al. (2015). Enhanced activity of NLRP3 inflammasome in peripheral blood cells of patients with active rheumatoid arthritis. Arthritis Res. Ther. 17, 257. doi:10.1186/s13075-015-0775-2
Chow, J. C., Young, D. W., Golenbock, D. T., Christ, W. J., and Gusovsky, F. (1999). Toll-like receptor-4 mediates lipopolysaccharide-induced signal transduction. J. Biol. Chem. 274, 10689–10692. doi:10.1074/jbc.274.16.10689
Chow, M. T., Sceneay, J., Paget, C., Wong, C. S., Duret, H., Tschopp, J., et al. (2012). NLRP3 suppresses NK cell-mediated responses to carcinogen-induced tumors and metastases. Cancer Res. 72, 5721–5732. doi:10.1158/0008-5472.CAN-12-0509
Clancy, D. J., Gems, D., Harshman, L. G., Oldham, S., Stocker, H., Hafen, E., et al. (2001). Extension of life-span by loss of CHICO, a Drosophila insulin receptor substrate protein. Science 292, 104–106. doi:10.1126/science.1057991
Codolo, G., Plotegher, N., Pozzobon, T., Brucale, M., Tessari, I., Bubacco, L., et al. (2013). Triggering of inflammasome by aggregated α-synuclein, an inflammatory response in synucleinopathies. PLoS One 8, e55375. doi:10.1371/journal.pone.0055375
Coll, R. C., O'neill, L., and Schroder, K. (2016). Questions and controversies in innate immune research: what is the physiological role of NLRP3? Cell. Death Discov. 2, 16019. doi:10.1038/cddiscovery.2016.19
Cook, S. A. (2023). Understanding interleukin 11 as a disease gene and therapeutic target. Biochem. J. 23, 1987–2008. doi:10.1042/BCJ20220160
Cornelius, D. C., Baik, C. H., Travis, O. K., White, D. L., Young, C. M., Austin Pierce, W., et al. (2019). NLRP3 inflammasome activation in platelets in response to sepsis. Physiol. Rep. 7, e14073. doi:10.14814/phy2.14073
Coschigano, K. T., Clemmons, D., Bellush, L. L., and Kopchick, J. J. (2000). Assessment of growth parameters and life span of GHR/BP gene-disrupted mice. Endocrinology 141, 2608–2613. doi:10.1210/endo.141.7.7586
Cribbs, D. H., Berchtold, N. C., Perreau, V., Coleman, P. D., Rogers, J., Tenner, A. J., et al. (2012). Extensive innate immune gene activation accompanies brain aging, increasing vulnerability to cognitive decline and neurodegeneration: a microarray study. J. Neuroinflammation 9, 179. doi:10.1186/1742-2094-9-179
Crowson, C. S., Matteson, E. L., Myasoedova, E., Michet, C. J., Ernste, F. C., Warrington, K. J., et al. (2011). The lifetime risk of adult-onset rheumatoid arthritis and other inflammatory autoimmune rheumatic diseases. Arthritis Rheum. 63, 633–639. doi:10.1002/art.30155
Da Costa, L. S., Outlioua, A., Anginot, A., Akarid, K., and Arnoult, D. (2019). RNA viruses promote activation of the NLRP3 inflammasome through cytopathogenic effect-induced potassium efflux. Cell. Death Dis. 10, 346. doi:10.1038/s41419-019-1579-0
Dahaghin, S., Bierma-Zeinstra, S. M., Ginai, A. Z., Pols, H. A., Hazes, J. M., and Koes, B. W. (2005). Prevalence and pattern of radiographic hand osteoarthritis and association with pain and disability (the Rotterdam study). Ann. Rheum. Dis. 64, 682–687. doi:10.1136/ard.2004.023564
Dalekos, G. N., Elisaf, M., Bairaktari, E., Tsolas, O., and Siamopoulos, K. C. (1997). Increased serum levels of interleukin-1beta in the systemic circulation of patients with essential hypertension: additional risk factor for atherogenesis in hypertensive patients? J. Lab. Clin. Med. 129, 300–308. doi:10.1016/s0022-2143(97)90178-5
De Cecco, M., Criscione, S. W., Peckham, E. J., Hillenmeyer, S., Hamm, E. A., Manivannan, J., et al. (2013). Genomes of replicatively senescent cells undergo global epigenetic changes leading to gene silencing and activation of transposable elements. Aging Cell. 12, 247–256. doi:10.1111/acel.12047
Dempsey, C., Rubio Araiz, A., Bryson, K. J., Finucane, O., Larkin, C., Mills, E. L., et al. (2017). Inhibiting the NLRP3 inflammasome with MCC950 promotes non-phlogistic clearance of amyloid-β and cognitive function in APP/PS1 mice. Brain Behav. Immun. 61, 306–316. doi:10.1016/j.bbi.2016.12.014
Di Iorio, A., Ferrucci, L., Sparvieri, E., Cherubini, A., Volpato, S., Corsi, A., et al. (2003). Serum IL-1beta levels in health and disease: a population-based study. 'The InCHIANTI study. Cytokine 22, 198–205. doi:10.1016/s1043-4666(03)00152-2
D'souza, C. A., and Heitman, J. (2001). Dismantling the cryptococcus coat. Trends Microbiol. 9, 112–113. doi:10.1016/s0966-842x(00)01945-4
Duewell, P., Kono, H., Rayner, K. J., Sirois, C. M., Vladimer, G., Bauernfeind, F. G., et al. (2010). NLRP3 inflammasomes are required for atherogenesis and activated by cholesterol crystals. Nature 464, 1357–1361. doi:10.1038/nature08938
Ebbesson, S. O., Tejero, M. E., López-Alvarenga, J. C., Harris, W. S., Ebbesson, L. O., Devereux, R. B., et al. (2010). Individual saturated fatty acids are associated with different components of insulin resistance and glucose metabolism: the GOCADAN study. Int. J. Circumpolar Health 69, 344–351. doi:10.3402/ijch.v69i4.17669
Eisenberg, T., Abdellatif, M., Schroeder, S., Primessnig, U., Stekovic, S., Pendl, T., et al. (2016). Cardioprotection and lifespan extension by the natural polyamine spermidine. Nat. Med. 22, 1428–1438. doi:10.1038/nm.4222
Erikson, G. A., Bodian, D. L., Rueda, M., Molparia, B., Scott, E. R., Scott-Van Zeeland, A. A., et al. (2016). Whole-genome sequencing of a healthy aging cohort. Cell. 165, 1002–1011. doi:10.1016/j.cell.2016.03.022
Ershaid, N., Sharon, Y., Doron, H., Raz, Y., Shani, O., Cohen, N., et al. (2019). NLRP3 inflammasome in fibroblasts links tissue damage with inflammation in breast cancer progression and metastasis. Nat. Commun. 10, 4375. doi:10.1038/s41467-019-12370-8
Eurostat (2019). Health in the European Union – facts and figures. Available at: https://ec.europa.eu/eurostat/statistics-explained/index.php?title=Healthy_life_years_statistics (Accessed June 20, 2024).
Evavold, C. L., Ruan, J., Tan, Y., Xia, S., Wu, H., and Kagan, J. C. (2018). The pore-forming protein gasdermin D regulates interleukin-1 secretion from living macrophages. Immunity 48, 35–44. doi:10.1016/j.immuni.2017.11.013
Evert, J., Lawler, E., Bogan, H., and Perls, T. (2003). Morbidity profiles of centenarians: survivors, delayers, and escapers. J. Gerontol. A Biol. Sci. Med. Sci. 58, 232–237. doi:10.1093/gerona/58.3.m232
Fan, Z., Pan, Y. T., Zhang, Z. Y., Yang, H., Yu, S. Y., Zheng, Y., et al. (2020). Systemic activation of NLRP3 inflammasome and plasma α-synuclein levels are correlated with motor severity and progression in Parkinson's disease. J. Neuroinflammation 17, 11. doi:10.1186/s12974-019-1670-6
Finucane, O. M., Lyons, C. L., Murphy, A. M., Reynolds, C. M., Klinger, R., Healy, N. P., et al. (2015). Monounsaturated fatty acid-enriched high-fat diets impede adipose NLRP3 inflammasome-mediated IL-1β secretion and insulin resistance despite obesity. Diabetes 64, 2116–2128. doi:10.2337/db14-1098
Franceschi, C., Garagnani, P., Parini, P., Giuliani, C., and Santoro, A. (2018). Inflammaging: a new immune-metabolic viewpoint for age-related diseases. Nat. Rev. Endocrinol. 14, 576–590. doi:10.1038/s41574-018-0059-4
Franchi, L., Eigenbrod, T., and Núñez, G. (2009). Cutting edge: TNF-alpha mediates sensitization to ATP and silica via the NLRP3 inflammasome in the absence of microbial stimulation. J. Immunol. 183, 792–796. doi:10.4049/jimmunol.0900173
Gaidt, M. M., Ebert, T. S., Chauhan, D., Schmidt, T., Schmid-Burgk, J. L., Rapino, F., et al. (2016). Human monocytes engage an alternative inflammasome pathway. Immunity 44, 833–846. doi:10.1016/j.immuni.2016.01.012
Goday, A., Bellido, D., Sajoux, I., Crujeiras, A. B., Burguera, B., García-Luna, P. P., et al. (2016). Short-term safety, tolerability and efficacy of a very low-calorie-ketogenic diet interventional weight loss program versus hypocaloric diet in patients with type 2 diabetes mellitus. Nutr. Diabetes 6, e230. doi:10.1038/nutd.2016.36
Gomes, A. P., Price, N. L., Ling, A. J., Moslehi, J. J., Montgomery, M. K., Rajman, L., et al. (2013). Declining NAD(+) induces a pseudohypoxic state disrupting nuclear-mitochondrial communication during aging. Cell. 155, 1624–1638. doi:10.1016/j.cell.2013.11.037
González-Dominguez, A., Montañez, R., Castejón-Vega, B., Nuñez-Vasco, J., Lendines-Cordero, D., Wang, C., et al. (2021). Inhibition of the NLRP3 inflammasome improves lifespan in animal murine model of Hutchinson-Gilford Progeria. EMBO Mol. Med. 13, e14012. doi:10.15252/emmm.202114012
Grimsgaard, S., Bonaa, K. H., Jacobsen, B. K., and Bjerve, K. S. (1999). Plasma saturated and linoleic fatty acids are independently associated with blood pressure. Hypertension 34, 478–483. doi:10.1161/01.hyp.34.3.478
Gu, Q., Sable, C. M., Brooks-Wilson, A., and Murphy, R. A. (2020). Dietary patterns in the healthy oldest old in the healthy aging study and the Canadian longitudinal study of aging: a cohort study. BMC Geriatr. 1, 106. doi:10.1186/s12877-020-01507-w
Guarda, G., Zenger, M., Yazdi, A. S., Schroder, K., Ferrero, I., Menu, P., et al. (2011). Differential expression of NLRP3 among hematopoietic cells. J. Immunol. 186, 2529–2534. doi:10.4049/jimmunol.1002720
Guo, B., Fu, S., Zhang, J., Liu, B., and Li, Z. (2016). Targeting inflammasome/IL-1 pathways for cancer immunotherapy. Sci. Rep. 6, 36107. doi:10.1038/srep36107
Guo, C., Fu, R., Wang, S., Huang, Y., Li, X., Zhou, M., et al. (2018). NLRP3 inflammasome activation contributes to the pathogenesis of rheumatoid arthritis. Clin. Exp. Immunol. 194, 231–243. doi:10.1111/cei.13167
Halle, A., Hornung, V., Petzold, G. C., Stewart, C. R., Monks, B. G., Reinheckel, T., et al. (2008). The NALP3 inflammasome is involved in the innate immune response to amyloid-beta. Nat. Immunol. 9, 857–865. doi:10.1038/ni.1636
Han, L., Zhang, Y., Zhang, M., Guo, L., Wang, J., Zeng, F., et al. (2020). Interleukin-1β-Induced senescence promotes osteoblastic transition of vascular smooth muscle cells. Kidney Blood Press Res. 45, 314–330. doi:10.1159/000504298
Harris, J., Hartman, M., Roche, C., Zeng, S. G., O'shea, A., Sharp, F. A., et al. (2011). Autophagy controls IL-1beta secretion by targeting pro-IL-1beta for degradation. J. Biol. Chem. 286, 9587–9597. doi:10.1074/jbc.M110.202911
Harrison, D. E., Strong, R., Reifsnyder, P., Kumar, N., Fernandez, E., Flurkey, K., et al. (2021). 17-a-estradiol late in life extends lifespan in aging UM-HET3 male mice; nicotinamide riboside and three other drugs do not affect lifespan in either sex. Aging Cell. 20, e13328. doi:10.1111/acel.13328
Harrison, D. E., Strong, R., Sharp, Z. D., Nelson, J. F., Astle, C. M., Flurkey, K., et al. (2009). Rapamycin fed late in life extends lifespan in genetically heterogeneous mice. Nature 460, 392–395. doi:10.1038/nature08221
Hauer, K., Hildebrandt, W., Sehl, Y., Edler, L., Oster, P., and Dröge, W. (2003). Improvement in muscular performance and decrease in tumor necrosis factor level in old age after antioxidant treatment. J. Mol. Med. Berl. 81, 118–125. doi:10.1007/s00109-002-0406-7
Heijman, M. W. J., Fiolet, A. T. L., Mosterd, A., Tijssen, J. G. P., Van Den Bemt, B. J. F., Schut, A., et al. (2023). Association of low-dose colchicine with incidence of knee and hip replacements: exploratory analyses from a randomized, controlled, double-blind trial. Ann. Intern Med. 176, 737–742. doi:10.7326/M23-0289
Heneka, M. T., Kummer, M. P., Stutz, A., Delekate, A., Schwartz, S., Vieira-Saecker, A., et al. (2013). NLRP3 is activated in Alzheimer's disease and contributes to pathology in APP/PS1 mice. Nature 493, 674–678. doi:10.1038/nature11729
Heneka, M. T., Mcmanus, R. M., and Latz, E. (2018). Inflammasome signalling in brain function and neurodegenerative disease. Nat. Rev. Neurosci. 19, 610–621. doi:10.1038/s41583-018-0055-7
Herranz, D., Muñoz-Martin, M., Cañamero, M., Mulero, F., Martinez-Pastor, B., Fernandez-Capetillo, O., et al. (2010). Sirt1 improves healthy ageing and protects from metabolic syndrome-associated cancer. Nat. Commun. 1, 3. doi:10.1038/ncomms1001
Herskind, A. M., Mcgue, M., Holm, N. V., Sorensen, T. I., Harvald, B., and Vaupel, J. W. (1996). The heritability of human longevity: a population-based study of 2872 Danish twin pairs born 1870-1900. Hum. Genet. 97, 319–323. doi:10.1007/BF02185763
Hoffman, H. M., Mueller, J. L., Broide, D. H., Wanderer, A. A., and Kolodner, R. D. (2001). Mutation of a new gene encoding a putative pyrin-like protein causes familial cold autoinflammatory syndrome and Muckle-Wells syndrome. Nat. Genet. 29, 301–305. doi:10.1038/ng756
Hoffman, H. M., Rosengren, S., Boyle, D. L., Cho, J. Y., Nayar, J., Mueller, J. L., et al. (2004). Prevention of cold-associated acute inflammation in familial cold autoinflammatory syndrome by interleukin-1 receptor antagonist. Lancet 364, 1779–1785. doi:10.1016/S0140-6736(04)17401-1
Hornung, V., Bauernfeind, F., Halle, A., Samstad, E. O., Kono, H., Rock, K. L., et al. (2008). Silica crystals and aluminum salts activate the NALP3 inflammasome through phagosomal destabilization. Nat. Immunol. 9, 847–856. doi:10.1038/ni.1631
Huang, Y., Jiang, H., Chen, Y., Wang, X., Yang, Y., Tao, J., et al. (2018). Tranilast directly targets NLRP3 to treat inflammasome-driven diseases. EMBO Mol. Med. 10, e8689. doi:10.15252/emmm.201708689
Ising, C., Venegas, C., Zhang, S., Scheiblich, H., Schmidt, S. V., Vieira-Saecker, A., et al. (2019). NLRP3 inflammasome activation drives tau pathology. Nature 575, 669–673. doi:10.1038/s41586-019-1769-z
Ismael, S., Zhao, L., Nasoohi, S., and Ishrat, T. (2018). Inhibition of the NLRP3-inflammasome as a potential approach for neuroprotection after stroke. Sci. Rep. 8, 5971. doi:10.1038/s41598-018-24350-x
Javaid, H. M. A., Ko, E., Joo, E. J., Kwon, S. H., Park, J. H., Shin, S., et al. (2023). TNFα-induced NLRP3 inflammasome mediates adipocyte dysfunction and activates macrophages through adipocyte-derived lipocalin 2. Metabolism 142, 155527. doi:10.1016/j.metabol.2023.155527
Jin, C., Frayssinet, P., Pelker, R., Cwirka, D., Hu, B., Vignery, A., et al. (2011). NLRP3 inflammasome plays a critical role in the pathogenesis of hydroxyapatite-associated arthropathy. Proc. Natl. Acad. Sci. U. S. A. 108, 14867–14872. doi:10.1073/pnas.1111101108
Kacprzyk, J., Hughes, G. M., Palsson-Mcdermott, E. M., Quinn, S. R., Puechmaille, S. J., O'neill, L. a. J., et al. (2017). A potent anti-inflammatory response in bat macrophages may Be linked to extended longevity and viral tolerance. Acta Chiropterologica 19, 219–228. doi:10.3161/15081109acc2017.19.2.001
Kang, Y., Zhang, H., Zhao, Y., Wang, Y., Wang, W., He, Y., et al. (2018). Telomere dysfunction disturbs macrophage mitochondrial metabolism and the NLRP3 inflammasome through the PGC-1α/TNFAIP3 Axis. Cell. Rep. 22, 3493–3506. doi:10.1016/j.celrep.2018.02.071
Kaplanov, I., Carmi, Y., Kornetsky, R., Shemesh, A., Shurin, G. V., Shurin, M. R., et al. (2019). Blocking IL-1β reverses the immunosuppression in mouse breast cancer and synergizes with anti-PD-1 for tumor abrogation. Proc. Natl. Acad. Sci. U. S. A. 116, 1361–1369. doi:10.1073/pnas.1812266115
Karmakar, M., Katsnelson, M. A., Dubyak, G. R., and Pearlman, E. (2016). Neutrophil P2X7 receptors mediate NLRP3 inflammasome-dependent IL-1β secretion in response to ATP. Nat. Commun. 7, 10555. doi:10.1038/ncomms10555
Kasper, L., König, A., Koenig, P. A., Gresnigt, M. S., Westman, J., Drummond, R. A., et al. (2018). The fungal peptide toxin Candidalysin activates the NLRP3 inflammasome and causes cytolysis in mononuclear phagocytes. Nat. Commun. 9, 4260. doi:10.1038/s41467-018-06607-1
Kim, Y. M., Byun, H. O., Jee, B. A., Cho, H., Seo, Y. H., Kim, Y. S., et al. (2013). Implications of time-series gene expression profiles of replicative senescence. Aging Cell. 12, 622–634. doi:10.1111/acel.12087
Ko, J. H., Yoon, S. O., Lee, H. J., and Oh, J. Y. (2017). Rapamycin regulates macrophage activation by inhibiting NLRP3 inflammasome-p38 MAPK-NFκB pathways in autophagy- and p62-dependent manners. Oncotarget 8, 40817–40831. doi:10.18632/oncotarget.17256
Kong, X., Lu, A. L., Yao, X. M., Hua, Q., Li, X. Y., Qin, L., et al. (2017). Activation of NLRP3 inflammasome by advanced glycation end products promotes pancreatic islet damage. Oxid. Med. Cell. Longev. 2017, 9692546. doi:10.1155/2017/9692546
Krishnan, S. M., Ling, Y. H., Huuskes, B. M., Ferens, D. M., Saini, N., Chan, C. T., et al. (2019). Pharmacological inhibition of the NLRP3 inflammasome reduces blood pressure, renal damage, and dysfunction in salt-sensitive hypertension. Cardiovasc Res. 115, 776–787. doi:10.1093/cvr/cvy252
Kumar, P., Liu, C., Hsu, J. W., Chacko, S., Minard, C., Jahoor, F., et al. (2021). Glycine and N-acetylcysteine (GlyNAC) supplementation in older adults improves glutathione deficiency, oxidative stress, mitochondrial dysfunction, inflammation, insulin resistance, endothelial dysfunction, genotoxicity, muscle strength, and cognition: results of a pilot clinical trial. Clin. Transl. Med. 11, e372. doi:10.1002/ctm2.372
Kumar, P., Liu, C., Suliburk, J., Hsu, J. W., Muthupillai, R., Jahoor, F., et al. (2023). Supplementing Glycine and N-acetylcysteine (GlyNAC) in older adults improves glutathione deficiency, oxidative stress, mitochondrial dysfunction, inflammation, physical function, and aging hallmarks: a randomized clinical trial. J. Gerontol. A Biol. Sci. Med. Sci. 78, 75–89. doi:10.1093/gerona/glac135
Kumar, P., Osahon, O. W., and Sekhar, R. V. (2022). Mechanistic role of Jak3 in obesity-associated cognitive impairments. Nutrients 14, 3715. doi:10.3390/nu14183715
Kuo, M. C., Chang, S. J., and Hsieh, M. C. (2015). Colchicine significantly reduces incident cancer in gout male patients: a 12-year cohort study. Med. Baltim. 94, e1570. doi:10.1097/MD.0000000000001570
Lachmann, H. J., Lowe, P., Felix, S. D., Rordorf, C., Leslie, K., Madhoo, S., et al. (2009). In vivo regulation of interleukin 1beta in patients with cryopyrin-associated periodic syndromes. J. Exp. Med. 206, 1029–1036. doi:10.1084/jem.20082481
Latz, E., and Duewell, P. (2018). NLRP3 inflammasome activation in inflammaging. Semin. Immunol. 40, 61–73. doi:10.1016/j.smim.2018.09.001
Lau, L., Porciuncula, A., Yu, A., Iwakura, Y., and David, G. (2019). Uncoupling the senescence-associated secretory phenotype from cell cycle exit via interleukin-1 inactivation unveils its protumorigenic role. Mol. Cell. Biol. 39 (12), e00586–18. doi:10.1128/MCB.00586-18
Laudisi, F., Spreafico, R., Evrard, M., Hughes, T. R., Mandriani, B., Kandasamy, M., et al. (2013). Cutting edge: the NLRP3 inflammasome links complement-mediated inflammation and IL-1β release. J. Immunol. 191, 1006–1010. doi:10.4049/jimmunol.1300489
Lee, C. C., Chen, W. T., Chen, S. Y., and Lee, T. M. (2021). Taurine alleviates sympathetic innervation by inhibiting NLRP3 inflammasome in postinfarcted rats. J. Cardiovasc Pharmacol. 6, 745–755. doi:10.1097/FJC.0000000000001005
Lee, H. M., Kim, J. J., Kim, H. J., Shong, M., Ku, B. J., and Jo, E. K. (2013). Upregulated NLRP3 inflammasome activation in patients with type 2 diabetes. Diabetes 62, 194–204. doi:10.2337/db12-0420
Li, L., and Liu, Y. (2015). Aging-related gene signature regulated by Nlrp3 predicts glioma progression. Am. J. Cancer Res. 5, 442–449.
Li, X., Zhou, X., Liu, X., Li, X., Jiang, X., Shi, B., et al. (2022). Spermidine protects against acute kidney injury by modulating macrophage NLRP3 inflammasome activation and mitochondrial respiration in an eIF5A hypusination-related pathway. Mol. Med. 28, 103. doi:10.1186/s10020-022-00533-1
Li, Y., Wang, K., Jigeer, G., Jensen, G., Tucker, K. L., Lv, Y., et al. (2024). Healthy lifestyle and the likelihood of becoming a centenarian. JAMA Netw. Open 7 (6), e2417931. doi:10.1001/jamanetworkopen.2024.17931
Li, Y., Yang, G., Yang, X., Wang, W., Zhang, J., He, Y., et al. (2016). Nicotinic acid inhibits NLRP3 inflammasome activation via SIRT1 in vascular endothelial cells. Int. Immunopharmacol. 40, 211–218. doi:10.1016/j.intimp.2016.09.003
Lin, J. J., Lin, C. L., Chen, C. C., Lin, Y. H., Cho, D. Y., Chen, X., et al. (2023). Unlocking colchicine's untapped potential: a paradigm shift in hepatocellular carcinoma prevention. Cancers (Basel) 15, 5031. doi:10.3390/cancers15205031
Ljungquist, B., Berg, S., Lanke, J., Mcclearn, G. E., and Pedersen, N. L. (1998). The effect of genetic factors for longevity: a comparison of identical and fraternal twins in the Swedish Twin Registry. J. Gerontol. A Biol. Sci. Med. Sci. 53, M441–M446. doi:10.1093/gerona/53a.6.m441
Lyons, J., Rauh-Pfeiffer, A., Yu, Y. M., Lu, X. M., Zurakowski, D., Tompkins, R. G., et al. (2000). Blood glutathione synthesis rates in healthy adults receiving a sulfur amino acid-free diet. Proc. Natl. Acad. Sci. U. S. A. 97, 5071–5076. doi:10.1073/pnas.090083297
Ma, S., Feng, J., Lin, X., Liu, J., Tang, Y., Nie, S., et al. (2021). Nicotinamide riboside alleviates cardiac dysfunction and remodeling in pressure overload cardiac hypertrophy. Oxid. Med. Cell. Longev. 2021, 5546867. doi:10.1155/2021/5546867
Maedler, K., Sergeev, P., Ris, F., Oberholzer, J., Joller-Jemelka, H. I., Spinas, G. A., et al. (2002). Glucose-induced beta cell production of IL-1beta contributes to glucotoxicity in human pancreatic islets. J. Clin. Investig. 110, 851–860. doi:10.1172/JCI15318
Mao, K., Quipildor, G. F., Tabrizian, T., Novaj, A., Guan, F., Walters, R. O., et al. (2018). Late-life targeting of the IGF-1 receptor improves healthspan and lifespan in female mice. Nat. Commun. 9, 2394. doi:10.1038/s41467-018-04805-5
March, C. J., Mosley, B., Larsen, A., Cerretti, D. P., Braedt, G., Price, V., et al. (1985). Cloning, sequence and expression of two distinct human interleukin-1 complementary DNAs. Nature 315, 641–647. doi:10.1038/315641a0
Mariathasan, S., Weiss, D. S., Newton, K., Mcbride, J., O'rourke, K., Roose-Girma, M., et al. (2006). Cryopyrin activates the inflammasome in response to toxins and ATP. Nature 440, 228–232. doi:10.1038/nature04515
Marin-Aguilar, F., Castejon-Vega, B., Alcocer-Gomez, E., Lendines-Cordero, D., Cooper, M. A., De La Cruz, P., et al. (2020). NLRP3 inflammasome inhibition by MCC950 in aged mice improves health via enhanced autophagy and PPARα activity. J. Gerontol. A Biol. Sci. Med. Sci. 75, 1457–1464. doi:10.1093/gerona/glz239
Marín-Aguilar, F., Lechuga-Vieco, A. V., Alcocer-Gómez, E., Castejón-Vega, B., Lucas, J., Garrido, C., et al. (2020). NLRP3 inflammasome suppression improves longevity and prevents cardiac aging in male mice. Aging Cell. 19, e13050. doi:10.1111/acel.13050
Martinez, E. M., Young, A. L., Patankar, Y. R., Berwin, B. L., Wang, L., Von Herrmann, K. M., et al. (2017). Editor's highlight: Nlrp3 is required for inflammatory changes and nigral cell loss resulting from chronic intragastric rotenone exposure in mice. Toxicol. Sci. 159, 64–75. doi:10.1093/toxsci/kfx117
Martinez, G. J., Robertson, S., Barraclough, J., Xia, Q., Mallat, Z., Bursill, C., et al. (2015). Colchicine acutely suppresses local cardiac production of inflammatory cytokines in patients with an acute coronary syndrome. J. Am. Heart Assoc. 4, e002128. doi:10.1161/JAHA.115.002128
Martinon, F., Burns, K., and Tschopp, J. (2002). The inflammasome: a molecular platform triggering activation of inflammatory caspases and processing of proIL-beta. Mol. Cell. 10, 417–426. doi:10.1016/s1097-2765(02)00599-3
Martinon, F., Pétrilli, V., Mayor, A., Tardivel, A., and Tschopp, J. (2006). Gout-associated uric acid crystals activate the NALP3 inflammasome. Nature 440, 237–241. doi:10.1038/nature04516
Masters, S. L., Dunne, A., Subramanian, S. L., Hull, R. L., Tannahill, G. M., Sharp, F. A., et al. (2010). Activation of the NLRP3 inflammasome by islet amyloid polypeptide provides a mechanism for enhanced IL-1β in type 2 diabetes. Nat. Immunol. 11, 897–904. doi:10.1038/ni.1935
Mckee, C. M., and Coll, R. C. (2020). NLRP3 inflammasome priming: a riddle wrapped in a mystery inside an enigma. J. Leukoc. Biol. 108, 937–952. doi:10.1002/JLB.3MR0720-513R
Miller, R. A., Harrison, D. E., Astle, C. M., Bogue, M. A., Brind, J., Fernandez, E., et al. (2019). Glycine supplementation extends lifespan of male and female mice. Aging Cell. 18, e12953. doi:10.1111/acel.12953
Mills, K. F., Yoshida, S., Stein, L. R., Grozio, A., Kubota, S., Sasaki, Y., et al. (2016). Long-term administration of nicotinamide mononucleotide mitigates age-associated physiological decline in mice. Cell. Metab. 24, 795–806. doi:10.1016/j.cmet.2016.09.013
Milman, S., Atzmon, G., Huffman, D. M., Wan, J., Crandall, J. P., Cohen, P., et al. (2014). Low insulin-like growth factor-1 level predicts survival in humans with exceptional longevity. Aging Cell. 13, 769–771. doi:10.1111/acel.12213
Misawa, T., Takahama, M., Kozaki, T., Lee, H., Zou, J., Saitoh, T., et al. (2013). Microtubule-driven spatial arrangement of mitochondria promotes activation of the NLRP3 inflammasome. Nat. Immunol. 14, 454–460. doi:10.1038/ni.2550
Mitchell, S. J., Bernier, M., Aon, M. A., Cortassa, S., Kim, E. Y., Fang, E. F., et al. (2018). Nicotinamide improves aspects of healthspan, but not lifespan, in mice. Cell. Metab. 27, 667–676. doi:10.1016/j.cmet.2018.02.001
Mitchell, S. J., Martin-Montalvo, A., Mercken, E. M., Palacios, H. H., Ward, T. M., Abulwerdi, G., et al. (2014). The SIRT1 activator SRT1720 extends lifespan and improves health of mice fed a standard diet. Cell. Rep. 6, 836–843. doi:10.1016/j.celrep.2014.01.031
Mohamed, M. A., Zeng, Z., Gennaro, M., Lao-Kaim, N. P., Myers, J. F. M., Calsolaro, V., et al. (2022). Astrogliosis in aging and Parkinson's disease dementia: a new clinical study with (11)C-BU99008 PET. Brain Commun. 4, fcac199. doi:10.1093/braincomms/fcac199
Mohammed, I., Hollenberg, M. D., Ding, H., and Triggle, C. R. (2021). A critical review of the evidence that metformin is a putative anti-aging drug that enhances healthspan and extends lifespan. Front. Endocrinol. (Lausanne) 12, 718942. doi:10.3389/fendo.2021.718942
Muhammad, J. S., Jayakumar, M. N., Elemam, N. M., Venkatachalam, T., Raju, T. K., Hamoudi, R. A., et al. (2019). Gasdermin D hypermethylation inhibits pyroptosis and LPS-induced IL-1β release from NK92 cells. Immunotargets Ther. 8, 29–41. doi:10.2147/ITT.S219867
Muñoz-Lorente, M. A., Cano-Martin, A. C., and Blasco, M. A. (2019). Mice with hyper-long telomeres show less metabolic aging and longer lifespans. Nat. Commun. 10, 4723. doi:10.1038/s41467-019-12664-x
Mylona, E. E., Mouktaroudi, M., Crisan, T. O., Makri, S., Pistiki, A., Georgitsi, M., et al. (2012). Enhanced interleukin-1β production of PBMCs from patients with gout after stimulation with Toll-like receptor-2 ligands and urate crystals. Arthritis Res. Ther. 14, R158. doi:10.1186/ar3898
Ng, A., Tam, W. W., Zhang, M. W., Ho, C. S., Husain, S. F., Mcintyre, R. S., et al. (2018). IL-1β, IL-6, TNF- α and CRP in elderly patients with depression or alzheimer's disease: systematic review and meta-analysis. Sci. Rep. 8, 12050. doi:10.1038/s41598-018-30487-6
Ni, B., Pei, W., Qu, Y., Zhang, R., Chu, X., Wang, Y., et al. (2021). MCC950, the NLRP3 inhibitor, protects against cartilage degradation in a mouse model of osteoarthritis. Oxid. Med. Cell. Longev. 2021, 4139048. doi:10.1155/2021/4139048
Nidorf, S. M., Fiolet, A. T. L., Mosterd, A., Eikelboom, J. W., Schut, A., Opstal, T. S. J., et al. (2020). Colchicine in patients with chronic coronary disease. N. Engl. J. Med. 383, 1838–1847. doi:10.1056/NEJMoa2021372
Nih (2021). Age and cancer risk. Available at: https://www.cancer.gov/about-cancer/causes-prevention/risk/age (Accessed.
Oeppen, J., and Vaupel, J. W. (2002). Demography. Broken limits to life expectancy. Science 296, 1029–1031. doi:10.1126/science.1069675
Oka, K., Fujioka, S., Kawamura, Y., Komohara, Y., Chujo, T., Sekiguchi, K., et al. (2022). Resistance to chemical carcinogenesis induction via a dampened inflammatory response in naked mole-rats. Commun. Biol. 5, 287. doi:10.1038/s42003-022-03241-y
Olshansky, S. J. (2018). From lifespan to healthspan. JAMA 320, 1323–1324. doi:10.1001/jama.2018.12621
Ong, J. D., Mansell, A., and Tate, M. D. (2017). Hero turned villain: NLRP3 inflammasome-induced inflammation during influenza A virus infection. J. Leukoc. Biol. 101, 863–874. doi:10.1189/jlb.4MR0616-288R
Pan, Z., Cheng, J., Yang, W., Chen, L., and Wang, J. (2022). Effect of colchicine on inflammatory markers in patients with coronary artery disease: a meta-analysis of clinical trials. Eur. J. Pharmacol. 927, 175068. doi:10.1016/j.ejphar.2022.175068
Paolisso, G., Tagliamonte, M. R., Rizzo, M. R., Manzella, D., Gambardella, A., and Varricchio, M. (1998). Oxidative stress and advancing age: results in healthy centenarians. J. Am. Geriatr. Soc. 46, 833–838. doi:10.1111/j.1532-5415.1998.tb02716.x
Paramel Varghese, G., Folkersen, L., Strawbridge, R. J., Halvorsen, B., Yndestad, A., Ranheim, T., et al. (2016). NLRP3 inflammasome expression and activation in human atherosclerosis. J. Am. Heart Assoc. 5, e003031. doi:10.1161/JAHA.115.003031
Parrinello, C. M., Lutsey, P. L., Ballantyne, C. M., Folsom, A. R., Pankow, J. S., and Selvin, E. (2015). Six-year change in high-sensitivity C-reactive protein and risk of diabetes, cardiovascular disease, and mortality. Am. Heart J. 170, 380–389. doi:10.1016/j.ahj.2015.04.017
Pavillard, L. E., Cañadas-Lozano, D., Alcocer-Gómez, E., Marín-Aguilar, F., Pereira, S., Robertson, A. a. B., et al. (2017). NLRP3-inflammasome inhibition prevents high fat and high sugar diets-induced heart damage through autophagy induction. Oncotarget 8, 99740–99756. doi:10.18632/oncotarget.20763
Pazar, B., Ea, H. K., Narayan, S., Kolly, L., Bagnoud, N., Chobaz, V., et al. (2011). Basic calcium phosphate crystals induce monocyte/macrophage IL-1β secretion through the NLRP3 inflammasome in vitro. J. Immunol. 186, 2495–2502. doi:10.4049/jimmunol.1001284
Pendergrass, W. R., Li, Y., Jiang, D., and Wolf, N. S. (1993). Decrease in cellular replicative potential in "giant" mice transfected with the bovine growth hormone gene correlates to shortened life span. J. Cell. Physiol. 156, 96–103. doi:10.1002/jcp.1041560114
Perls, T. T., Wilmoth, J., Levenson, R., Drinkwater, M., Cohen, M., Bogan, H., et al. (2002). Life-long sustained mortality advantage of siblings of centenarians. Proc. Natl. Acad. Sci. U. S. A. 99, 8442–8447. doi:10.1073/pnas.122587599
Puzianowska-Kuznicka, M., Owczarz, M., Wieczorowska-Tobis, K., Nadrowski, P., Chudek, J., Slusarczyk, P., et al. (2016). Interleukin-6 and C-reactive protein, successful aging, and mortality: the PolSenior study. Immun. Ageing 13, 21. doi:10.1186/s12979-016-0076-x
Qiu, T., Pei, P., Yao, X., Jiang, L., Wei, S., Wang, Z., et al. (2018). Taurine attenuates arsenic-induced pyroptosis and nonalcoholic steatohepatitis by inhibiting the autophagic-inflammasomal pathway. Cell. Death Dis. 10, 946. doi:10.1038/s41419-018-1004-0
Rajpathak, S. N., Liu, Y., Ben-David, O., Reddy, S., Atzmon, G., Crandall, J., et al. (2011). Lifestyle factors of people with exceptional longevity. J. Am. Geriatr. Soc. 59, 1509–1512. doi:10.1111/j.1532-5415.2011.03498.x
Ratajczak, M. Z., Bujko, K., Cymer, M., Thapa, A., Adamiak, M., Ratajczak, J., et al. (2020). The Nlrp3 inflammasome as a "rising star" in studies of normal and malignant hematopoiesis. Leukemia 34, 1512–1523. doi:10.1038/s41375-020-0827-8
Reiman, E. M., Arboleda-Velasquez, J. F., Quiroz, Y. T., Huentelman, M. J., Beach, T. G., Caselli, R. J., et al. (2020). Exceptionally low likelihood of Alzheimer's dementia in APOE2 homozygotes from a 5,000-person neuropathological study. Nat. Commun. 11, 667. doi:10.1038/s41467-019-14279-8
Rhoads, J. P., Lukens, J. R., Wilhelm, A. J., Moore, J. L., Mendez-Fernandez, Y., Kanneganti, T. D., et al. (2017). Oxidized low-density lipoprotein immune complex priming of the Nlrp3 inflammasome involves TLR and FcγR cooperation and is dependent on CARD9. J. Immunol. 198, 2105–2114. doi:10.4049/jimmunol.1601563
Ridker, P. M., Everett, B. M., Thuren, T., Macfadyen, J. G., Chang, W. H., Ballantyne, C., et al. (2017a). Antiinflammatory therapy with canakinumab for atherosclerotic disease. N. Engl. J. Med. 377, 1119–1131. doi:10.1056/nejmoa1707914
Ridker, P. M., Macfadyen, J. G., Thuren, T., Everett, B. M., Libby, P., Glynn, R. J., et al. (2017b). Effect of interleukin-1β inhibition with canakinumab on incident lung cancer in patients with atherosclerosis: exploratory results from a randomised, double-blind, placebo-controlled trial. Lancet 390, 1833–1842. doi:10.1016/S0140-6736(17)32247-X
Roberts, M. N., Wallace, M. A., Tomilov, A. A., Zhou, Z., Marcotte, G. R., Tran, D., et al. (2017). A ketogenic diet extends longevity and healthspan in adult mice. Cell. Metab. 26, 539–546. doi:10.1016/j.cmet.2017.08.005
Romero, A., Dongil, P., Valencia, I., Vallejo, S., Hipólito-Luengo Á, S., Díaz-Araya, G., et al. (2022). Pharmacological blockade of NLRP3 inflammasome/IL-1β-positive loop mitigates endothelial cell senescence and dysfunction. Aging Dis. 13, 284–297. doi:10.14336/AD.2021.0617
Samiec, P. S., Drews-Botsch, C., Flagg, E. W., Kurtz, J. C., Sternberg, P., Reed, R. L., et al. (1998). Glutathione in human plasma: decline in association with aging, age-related macular degeneration, and diabetes. Free Radic. Biol. Med. 24, 699–704. doi:10.1016/s0891-5849(97)00286-4
Samper, E., Flores, J. M., and Blasco, M. A. (2001). Restoration of telomerase activity rescues chromosomal instability and premature aging in Terc-/- mice with short telomeres. EMBO Rep. 2, 800–807. doi:10.1093/embo-reports/kve174
Sand, J., Haertel, E., Biedermann, T., Contassot, E., Reichmann, E., French, L. E., et al. (2018). Expression of inflammasome proteins and inflammasome activation occurs in human, but not in murine keratinocytes. Cell. Death Dis. 9, 24. doi:10.1038/s41419-017-0009-4
Sandanger, O., Ranheim, T., Vinge, L. E., Bliksoen, M., Alfsnes, K., Finsen, A. V., et al. (2013). The NLRP3 inflammasome is up-regulated in cardiac fibroblasts and mediates myocardial ischaemia-reperfusion injury. Cardiovasc Res. 99, 164–174. doi:10.1093/cvr/cvt091
Satoh, A., Brace, C. S., Rensing, N., Cliften, P., Wozniak, D. F., Herzog, E. D., et al. (2013). Sirt1 extends life span and delays aging in mice through the regulation of Nk2 homeobox 1 in the DMH and LH. Cell. Metab. 18, 416–430. doi:10.1016/j.cmet.2013.07.013
Schoenmaker, M., de Craen, A. J., de Meijer, P. H., Beekman, M., Blauw, G. J., Slagboom, P. E., et al. (2006). Evidence of genetic enrichment for exceptional survival using a family approach: the Leiden Longevity Study. Eur. J. Hum. Genet. 14, 79–84. doi:10.1038/sj.ejhg.5201508
Scott, A. J., Ellison, M., and Sinclair, D. A. (2021). The economic value of targeting aging. Nat. Aging 1, 616–623. doi:10.1038/s43587-021-00080-0
Sebastiani, P., Federico, A., Morris, M., Gurinovich, A., Tanaka, T., Chandler, K. B., et al. (2021). Protein signatures of centenarians and their offspring suggest centenarians age slower than other humans. Aging Cell. 2, e13290. doi:10.1111/acel.13290
Sekhar, R. V., Patel, S. G., Guthikonda, A. P., Reid, M., Balasubramanyam, A., Taffet, G. E., et al. (2011). Deficient synthesis of glutathione underlies oxidative stress in aging and can be corrected by dietary cysteine and glycine supplementation. Am. J. Clin. Nutr. 94, 847–853. doi:10.3945/ajcn.110.003483
Shang, D., Hong, Y., Xie, W., Tu, Z., and Xu, J. (2020). Interleukin-1β drives cellular senescence of rat astrocytes induced by oligomerized amyloid β peptide and oxidative stress. Front. Neurol. 11, 929. doi:10.3389/fneur.2020.00929
Shi, J., Zhao, Y., Wang, K., Shi, X., Wang, Y., Huang, H., et al. (2015). Cleavage of GSDMD by inflammatory caspases determines pyroptotic cell death. Nature 526, 660–665. doi:10.1038/nature15514
Shi, L., Zhao, Y., Fei, C., Guo, J., Jia, Y., Wu, D., et al. (2019). Cellular senescence induced by S100A9 in mesenchymal stromal cells through NLRP3 inflammasome activation. Aging (Albany NY) 11, 9626–9642. doi:10.18632/aging.102409
Shimada, K., Crother, T. R., Karlin, J., Dagvadorj, J., Chiba, N., Chen, S., et al. (2012). Oxidized mitochondrial DNA activates the NLRP3 inflammasome during apoptosis. Immunity 36, 401–414. doi:10.1016/j.immuni.2012.01.009
Singh, P., Gollapalli, K., Mangiola, S., Schranner, D., Yusuf, M. A., Chamoli, M., et al. (2023). Taurine deficiency as a driver of aging. Science 380, eabn9257. doi:10.1126/science.abn9257
Slack, C., Foley, A., and Partridge, L. (2012). Activation of AMPK by the putative dietary restriction mimetic metformin is insufficient to extend lifespan in Drosophila. PLoS One 7, e47699. doi:10.1371/journal.pone.0047699
Solle, M., Labasi, J., Perregaux, D. G., Stam, E., Petrushova, N., Koller, B. H., et al. (2001). Altered cytokine production in mice lacking P2X(7) receptors. J. Biol. Chem. 276, 125–132. doi:10.1074/jbc.M006781200
Spadaro, O., Goldberg, E. L., Camell, C. D., Youm, Y. H., Kopchick, J. J., Nguyen, K. Y., et al. (2016). Growth hormone receptor deficiency protects against age-related NLRP3 inflammasome activation and immune senescence. Cell. Rep. 14, 1571–1580. doi:10.1016/j.celrep.2016.01.044
Spiers, G. F., Kunonga, T. P., Beyer, F., Craig, D., Hanratty, B., and Jagger, C. (2021). Trends in health expectancies: a systematic review of international evidence. BMJ Open 11, e045567. doi:10.1136/bmjopen-2020-045567
Spranger, J., Kroke, A., Möhlig, M., Hoffmann, K., Bergmann, M. M., Ristow, M., et al. (2003). Inflammatory cytokines and the risk to develop type 2 diabetes: results of the prospective population-based European Prospective Investigation into Cancer and Nutrition (EPIC)-Potsdam Study. Diabetes 52, 812–817. doi:10.2337/diabetes.52.3.812
Stancu, I. C., Cremers, N., Vanrusselt, H., Couturier, J., Vanoosthuyse, A., Kessels, S., et al. (2019). Aggregated Tau activates NLRP3-ASC inflammasome exacerbating exogenously seeded and non-exogenously seeded Tau pathology in vivo. Acta Neuropathol. 137, 599–617. doi:10.1007/s00401-018-01957-y
Stout-Delgado, H. W., Cho, S. J., Chu, S. G., Mitzel, D. N., Villalba, J., El-Chemaly, S., et al. (2016). Age-dependent susceptibility to pulmonary fibrosis is associated with NLRP3 inflammasome activation. Am. J. Respir. Cell. Mol. Biol. 55, 252–263. doi:10.1165/rcmb.2015-0222OC
Sun, H. J., Ren, X. S., Xiong, X. Q., Chen, Y. Z., Zhao, M. X., Wang, J. J., et al. (2017). NLRP3 inflammasome activation contributes to VSMC phenotypic transformation and proliferation in hypertension. Cell. Death Dis. 8, e3074. doi:10.1038/cddis.2017.470
Symons, J. A., Young, P. R., and Duff, G. W. (1995). Soluble type II interleukin 1 (IL-1) receptor binds and blocks processing of IL-1 beta precursor and loses affinity for IL-1 receptor antagonist. Proc. Natl. Acad. Sci. U. S. A. 92, 1714–1718. doi:10.1073/pnas.92.5.1714
Taylor, M. K., Sullivan, D. K., Mahnken, J. D., Burns, J. M., and Swerdlow, R. H. (2018). Feasibility and efficacy data from a ketogenic diet intervention in Alzheimer's disease. Alzheimers Dement. (N Y) 4, 28–36. doi:10.1016/j.trci.2017.11.002
Tedone, E., Huang, E., O'hara, R., Batten, K., Ludlow, A. T., Lai, T. P., et al. (2019). Telomere length and telomerase activity in T cells are biomarkers of high-performing centenarians. Aging Cell. 18, e12859. doi:10.1111/acel.12859
Terkeltaub, R. A., Furst, D. E., Bennett, K., Kook, K. A., Crockett, R. S., and Davis, M. W. (2010). High versus low dosing of oral colchicine for early acute gout flare: twenty-four-hour outcome of the first multicenter, randomized, double-blind, placebo-controlled, parallel-group, dose-comparison colchicine study. Arthritis Rheum. 62, 1060–1068. doi:10.1002/art.27327
Thinwa, J., Segovia, J. A., Bose, S., and Dube, P. H. (2014). Integrin-mediated first signal for inflammasome activation in intestinal epithelial cells. J. Immunol. 193, 1373–1382. doi:10.4049/jimmunol.1400145
Thornton, P., Reader, V., Digby, Z., Smolak, P., Lindsay, N., Harrison, D., et al. (2024). Reversal of high fat diet-induced obesity, systemic inflammation, and astrogliosis by the NLRP3 inflammasome inhibitors NT-0249 and NT-0796. J. Pharmacol. Exp. Ther. 388, 813–826. doi:10.1124/jpet.123.002013
Townsend, D. M., Tew, K. D., and Tapiero, H. (2003). The importance of glutathione in human disease. Biomed. Pharmacother. 57, 145–155. doi:10.1016/s0753-3322(03)00043-x
Ubaida-Mohien, C., Spendiff, S., Lyashkov, A., Moaddel, R., Macmillan, N. J., Filion, M. E., et al. (2022). Unbiased proteomics, histochemistry, and mitochondrial DNA copy number reveal better mitochondrial health in muscle of high-functioning octogenarians. Elife 11, e74335. doi:10.7554/eLife.74335
Vandanmagsar, B., Youm, Y. H., Ravussin, A., Galgani, J. E., Stadler, K., Mynatt, R. L., et al. (2011). The NLRP3 inflammasome instigates obesity-induced inflammation and insulin resistance. Nat. Med. 17, 179–188. doi:10.1038/nm.2279
Van Der Heijden, T., Kritikou, E., Venema, W., Van Duijn, J., Van Santbrink, P. J., Slütter, B., et al. (2017). NLRP3 inflammasome inhibition by MCC950 reduces atherosclerotic lesion development in apolipoprotein E-deficient mice-brief report. Arterioscler. Thromb. Vasc. Biol. 37, 1457–1461. doi:10.1161/ATVBAHA.117.309575
Van Deventer, H. W., Burgents, J. E., Wu, Q. P., Woodford, R. M., Brickey, W. J., Allen, I. C., et al. (2010). The inflammasome component NLRP3 impairs antitumor vaccine by enhancing the accumulation of tumor-associated myeloid-derived suppressor cells. Cancer Res. 70, 10161–10169. doi:10.1158/0008-5472.CAN-10-1921
Vande Walle, L., Van Opdenbosch, N., Jacques, P., Fossoul, A., Verheugen, E., Vogel, P., et al. (2014). Negative regulation of the NLRP3 inflammasome by A20 protects against arthritis. Nature 512, 69–73. doi:10.1038/nature13322
Van Hout, G. P., Bosch, L., Ellenbroek, G. H., De Haan, J. J., Van Solinge, W. W., Cooper, M. A., et al. (2017). The selective NLRP3-inflammasome inhibitor MCC950 reduces infarct size and preserves cardiac function in a pig model of myocardial infarction. Eur. Heart J. 38, 828–836. doi:10.1093/eurheartj/ehw247
Vasto, S., Rizzo, C., and Caruso, C. (2012). Centenarians and diet: what they eat in the Western part of Sicily. Immun. Ageing 23, 10. doi:10.1186/1742-4933-9-10
Von Herrmann, K. M., Salas, L. A., Martinez, E. M., Young, A. L., Howard, J. M., Feldman, M. S., et al. (2018). NLRP3 expression in mesencephalic neurons and characterization of a rare NLRP3 polymorphism associated with decreased risk of Parkinson's disease. NPJ Park. Dis. 4, 24. doi:10.1038/s41531-018-0061-5
Wang, M., Zhao, M., Yu, J., Xu, Y., Zhang, J., Liu, J., et al. (2022). MCC950, a selective NLRP3 inhibitor, attenuates adverse cardiac remodeling following heart failure through improving the cardiometabolic dysfunction in obese mice. Front. Cardiovasc Med. 9, 727474. doi:10.3389/fcvm.2022.727474
Wang, Z., Meng, S., Cao, L., Chen, Y., Zuo, Z., and Peng, S. (2018). Critical role of NLRP3-caspase-1 pathway in age-dependent isoflurane-induced microglial inflammatory response and cognitive impairment. J. Neuroinflammation 15, 109. doi:10.1186/s12974-018-1137-1
Weisberg, S. P., Mccann, D., Desai, M., Rosenbaum, M., Leibel, R. L., and Ferrante, A. W. (2003). Obesity is associated with macrophage accumulation in adipose tissue. J. Clin. Investig. 112, 1796–1808. doi:10.1172/JCI19246
Wen, H., Gris, D., Lei, Y., Jha, S., Zhang, L., Huang, M. T., et al. (2011). Fatty acid-induced NLRP3-ASC inflammasome activation interferes with insulin signaling. Nat. Immunol. 12, 408–415. doi:10.1038/ni.2022
Widjaja, A. A., Lim, W. W., Viswanathan, S., Chothani, S., Corden, B., Dasan, C. M., et al. (2024). Inhibition of IL-11 signalling extends mammalian healthspan and lifespan. Nature 8023, 157–165. doi:10.1038/s41586-024-07701-9
Wolf, A. J., Reyes, C. N., Liang, W., Becker, C., Shimada, K., Wheeler, M. L., et al. (2016). Hexokinase is an innate immune receptor for the detection of bacterial peptidoglycan. Cell. 166, 624–636. doi:10.1016/j.cell.2016.05.076
Wolkow, C. A., Kimura, K. D., Lee, M. S., and Ruvkun, G. (2000). Regulation of C. elegans life-span by insulinlike signaling in the nervous system. Science 290, 147–150. doi:10.1126/science.290.5489.147
Wu, Z., Sun, L., Hashioka, S., Yu, S., Schwab, C., Okada, R., et al. (2013). Differential pathways for interleukin-1β production activated by chromogranin A and amyloid β in microglia. Neurobiol. Aging 34, 2715–2725. doi:10.1016/j.neurobiolaging.2013.05.018
Xia, M., Boini, K. M., Abais, J. M., Xu, M., Zhang, Y., and Li, P. L. (2014). Endothelial NLRP3 inflammasome activation and enhanced neointima formation in mice by adipokine visfatin. Am. J. Pathol. 184, 1617–1628. doi:10.1016/j.ajpath.2014.01.032
Xian, H., Liu, Y., Rundberg Nilsson, A., Gatchalian, R., Crother, T. R., Tourtellotte, W. G., et al. (2021). Metformin inhibition of mitochondrial ATP and DNA synthesis abrogates NLRP3 inflammasome activation and pulmonary inflammation. Immunity 54, 1463–1477.e11. doi:10.1016/j.immuni.2021.05.004
XPRIZE (2023). XPRIZE healthspan competition. Available at: https://www.xprize.org/prizes/healthspan (Accessed June 20, 204).
Yang, C. S., Chou, S. T., Liu, L., Tsai, P. J., and Kuo, J. S. (1995). Effect of ageing on human plasma glutathione concentrations as determined by high-performance liquid chromatography with fluorimetric detection. J. Chromatogr. B Biomed. Appl. 674, 23–30. doi:10.1016/0378-4347(95)00287-8
Yang, F., Qin, Y., Wang, Y., Meng, S., Xian, H., Che, H., et al. (2019). Metformin inhibits the NLRP3 inflammasome via AMPK/mTOR-dependent effects in diabetic cardiomyopathy. Int. J. Biol. Sci. 15, 1010–1019. doi:10.7150/ijbs.29680
Yao, Y., Chen, S., Cao, M., Fan, X., Yang, T., Huang, Y., et al. (2017). Antigen-specific CD8(+) T cell feedback activates NLRP3 inflammasome in antigen-presenting cells through perforin. Nat. Commun. 8, 15402. doi:10.1038/ncomms15402
Yin, Y., Zhou, Z., Liu, W., Chang, Q., Sun, G., and Dai, Y. (2017). Vascular endothelial cells senescence is associated with NOD-like receptor family pyrin domain-containing 3 (NLRP3) inflammasome activation via reactive oxygen species (ROS)/thioredoxin-interacting protein (TXNIP) pathway. Int. J. Biochem. Cell. Biol. 84, 22–34. doi:10.1016/j.biocel.2017.01.001
Youm, Y. H., Adijiang, A., Vandanmagsar, B., Burk, D., Ravussin, A., and Dixit, V. D. (2011). Elimination of the NLRP3-ASC inflammasome protects against chronic obesity-induced pancreatic damage. Endocrinology 152, 4039–4045. doi:10.1210/en.2011-1326
Youm, Y. H., Grant, R. W., Mccabe, L. R., Albarado, D. C., Nguyen, K. Y., Ravussin, A., et al. (2013). Canonical Nlrp3 inflammasome links systemic low-grade inflammation to functional decline in aging. Cell. Metab. 18, 519–532. doi:10.1016/j.cmet.2013.09.010
Youm, Y. H., Kanneganti, T. D., Vandanmagsar, B., Zhu, X., Ravussin, A., Adijiang, A., et al. (2012). The Nlrp3 inflammasome promotes age-related thymic demise and immunosenescence. Cell. Rep. 1, 56–68. doi:10.1016/j.celrep.2011.11.005
Youm, Y. H., Nguyen, K. Y., Grant, R. W., Goldberg, E. L., Bodogai, M., Kim, D., et al. (2015). The ketone metabolite β-hydroxybutyrate blocks NLRP3 inflammasome-mediated inflammatory disease. Nat. Med. 21, 263–269. doi:10.1038/nm.3804
Zanoni, I., Tan, Y., Di Gioia, M., Springstead, J. R., and Kagan, J. C. (2017). By capturing inflammatory lipids released from dying cells, the receptor CD14 induces inflammasome-dependent phagocyte hyperactivation. Immunity 47, 697–709. doi:10.1016/j.immuni.2017.09.010
Zhang, H., Ryu, D., Wu, Y., Gariani, K., Wang, X., Luan, P., et al. (2016). NAD⁺ repletion improves mitochondrial and stem cell function and enhances life span in mice. Science 352, 1436–1443. doi:10.1126/science.aaf2693
Zhang, L., Lu, L., Zhong, X., Yue, Y., Hong, Y., Li, Y., et al. (2019a). Metformin reduced NLRP3 inflammasome activity in Ox-LDL stimulated macrophages through adenosine monophosphate activated protein kinase and protein phosphatase 2A. Eur. J. Pharmacol. 852, 99–106. doi:10.1016/j.ejphar.2019.03.006
Zhang, T., Chen, W., Jiang, X., Liu, L., Wei, K., Du, H., et al. (2019b). Anticancer effects and underlying mechanism of Colchicine on human gastric cancer cell lines in vitro and in vivo. Biosci. Rep. 39. doi:10.1042/BSR20181802
Zhang, T., Tsutsuki, H., Islam, W., Ono, K., Takeda, K., Akaike, T., et al. (2021). ATP exposure stimulates glutathione efflux as a necessary switch for NLRP3 inflammasome activation. Redox Biol. 41, 101930. doi:10.1016/j.redox.2021.101930
Zhou, G., Myers, R., Li, Y., Chen, Y., Shen, X., Fenyk-Melody, J., et al. (2001). Role of AMP-activated protein kinase in mechanism of metformin action. J. Clin. Investig. 108, 1167–1174. doi:10.1172/JCI13505
Zhou, H., Khan, D., Gerdes, N., Hagenbeck, C., Rana, M., Cornelius, J. F., et al. (2023). Colchicine protects against ethanol-induced senescence and senescence-associated secretory phenotype in endothelial cells. Antioxidants (Basel) 12, 960. doi:10.3390/antiox12040960
Keywords: NLRP3, aging, senescence, healthy centenarians, health extension, accelerated aging, auto-aging
Citation: Verlinden SF (2024) The genetic advantage of healthy centenarians: unraveling the central role of NLRP3 in exceptional healthspan. Front. Aging 5:1452453. doi: 10.3389/fragi.2024.1452453
Received: 20 June 2024; Accepted: 19 August 2024;
Published: 05 September 2024.
Edited by:
Andréa Cristina Paula-Lima, University of Chile, ChileReviewed by:
Ilias Gkikas, Foundation for Research and Technology Hellas (FORTH), GreeceCarol D. SanMartín, University of Chile, Chile
Copyright © 2024 Verlinden. This is an open-access article distributed under the terms of the Creative Commons Attribution License (CC BY). The use, distribution or reproduction in other forums is permitted, provided the original author(s) and the copyright owner(s) are credited and that the original publication in this journal is cited, in accordance with accepted academic practice. No use, distribution or reproduction is permitted which does not comply with these terms.
*Correspondence: Stef F. Verlinden, c3RlZkB5b3hsby5jb20=