- 1Department of Systems Aging Science and Medicine, Tokyo Metropolitan Institute of Gerontology, Tokyo, Japan
- 2Department of Basic Technology, Tokyo Metropolitan Institute of Medical Science, Tokyo, Japan
Alzheimer’s disease (AD) is an age-associated neurodegenerative disorder characterized by progressive impairment of memory, thinking, behavior, and dementia. Based on ample evidence showing neurotoxicity of amyloid-β (Aβ) aggregates in AD, proteolytically derived from amyloid precursor protein (APP), it has been assumed that misfolding of Aβ plays a crucial role in the AD pathogenesis. Additionally, extra copies of the APP gene caused by chromosomal duplication in patients with Down syndrome can promote AD pathogenesis, indicating the pathological involvement of the APP gene dose in AD. Furthermore, increased APP expression due to locus duplication and promoter mutation of APP has been found in familial AD. Given this background, we aimed to summarize the mechanism underlying the upregulation of APP expression levels from a cutting-edge perspective. We first reviewed the literature relevant to this issue, specifically focusing on the transcriptional regulation of APP by transcription factors that bind to the promoter/enhancer regions. APP expression is also regulated by growth factors, cytokines, and hormone, such as androgen. We further evaluated the possible involvement of post-transcriptional regulators of APP in AD pathogenesis, such as RNA splicing factors. Indeed, alternative splicing isoforms of APP are proposed to be involved in the increased production of Aβ. Moreover, non-coding RNAs, including microRNAs, post-transcriptionally regulate the APP expression. Collectively, elucidation of the novel mechanisms underlying the upregulation of APP would lead to the development of clinical diagnosis and treatment of AD.
Introduction
Alzheimer’s disease (AD) is the most common form of age-related dementia and a complex neurodegenerative disorder, phenotypically featured with progressive impairment of memory, thinking, and behavior as along with cognitive decline (Bateman et al., 2012; Mucke and Selkoe, 2012; Kirova et al., 2015; Hashimoto et al., 2018). AD is pathologically characterized by the deposition of senile plaques and neurofibrillary tangles (NTFs) in the brain (DeTure and Dickson, 2019). Senile plaques are mainly composed of soluble amyloid-β (Aβ) peptides (Panza et al., 2019), which form aberrant aggregates exhibiting neurotoxicity in the brain; therefore, the crucial role in the AD pathogenesis is assumed to be played by misfolded Aβ, namely amyloid hypothesis. Notably, NTFs is mainly comprised of a hyperphosphorylated TAU protein, which has been implicated in major neurodegenerative diseases including AD, termed Tauopathy (Panza et al., 2019). Aβ is produced through sequential proteolytic processing of a transmembrane protein, Aβ precursor protein (APP) by the β-site APP-cleaving enzyme 1 (BACE1) and γ-secretase (Panza et al., 2019), through the amyloidogenic pathway. In addition to the amyloidogenic pathway, the majority of APP undergoes non-pathogenic processing mediated by sequential cleavage of α-secretase and γ-secretase (Nguyen, 2019). In this pathway, an N-terminal secreted form of APP (sAPPα) is generated that plays numerous roles in normal physiological functions in the brain, such as neuronal proliferation, differentiation, migration, and synaptic function (Nguyen, 2019; Dar and Glazner, 2020).
To decipher the pathogenesis of AD, several studies have addressed the populations in which genetic variations are known to cause AD. In humans, the APP gene is located on chromosome 21 with 18 exons and is alternatively spliced into multiple isoforms, of which three isoforms, APP695, APP751, and APP770 are primarily generated. APP695 is predominantly expressed in neurons, whereas the remainders are expressed rather ubiquitously (Dai et al., 2018). Almost all adults with Down syndrome (DS) display neuropathological changes of AD over 40 years of age due to extra copies of APP attributed to the trisomy of chromosome 21 (Wiseman et al., 2015; Antonarakis, 2017; Lott and Head, 2019). Furthermore, a genetic variation observed in individuals with small internal duplications within chromosome 21 can result in three APP copies in a rare familial trait known as duplication of APP and can lead to an early-onset AD (Rovelet-Lecrux et al., 2006; Sleegers et al., 2006; Kasuga et al., 2009; Thonberg et al., 2011; Hooli et al., 2012; Swaminathan et al., 2012). In contrast, partial trisomy of chromosome 21 lacking an extra copy of the APP gene does not promote AD (Prasher et al., 1998; Korbel et al., 2009). The APP copy number is also mosaically amplified in the neurons of late-onset sporadic AD brains (Bushman et al., 2015). Additionally, genomic variations within APP promoter can upregulate its expression thereby increasing the risk of AD (Prasher et al., 1998; Guyant-Maréchal et al., 2007). This implies that the genetic variations involving an increase in APP mRNA levels are associated with AD pathophysiology. Moreover, increased APP expression levels have been detected in the brain, particularly in the entorhinal cortex neurons containing neurofibrillary tangles in AD patients (Cohen et al., 1988; Higgins et al., 1988; Guttula et al., 2012). Together, APP expression levels can impact the pathological processes in AD. Here, we summarized the literature relevant to this issue, specifically focusing on both transcriptional and post-transcriptional regulation of APP mRNA, and examined their roles in AD pathogenesis.
Transcriptional Regulation of APP
In this section, we summarized the annotated genomic features of the human APP promoter/enhancer and its transcriptional regulators.
APP Promoter/Enhancer Activity
The promoter of the human APP lacks TATA and CAAT boxes upstream of the transcription start site but contains a high GC region with five GGGCGC boxes (Lahiri and Robakis, 1991) (Figure 1A), which adapts to the typical characteristics of a housekeeping gene (Smale and Kadonaga, 2003). The proximal region of the promoter from –150 to –10 base pairs (bp) contains the minimum essential elements for APP promoter activity (Lahiri and Robakis, 1991; Lahiri and Nall, 1995). The region from − 600 to − 460 bp acts as a transcriptionally positive regulator; in particular, a 26 bp positioned between –489 and –462 bp acts as a strong enhancer. In contrast, the region from –450 to –150 bp works as a negative regulator. Additionally, the downstream region of the APP promoter does not match the consensus sequences for any of the downstream core promoter sequences, such as the downstream promoter element (DPE), which is generally required for efficient transcription (Vostrov et al., 2010). However, the region from + 72 to + 115 has an unknown nuclear factor-binding domain termed as DNase I protected domain (DAPB), which is required for APP promoter activity in HeLa cells.
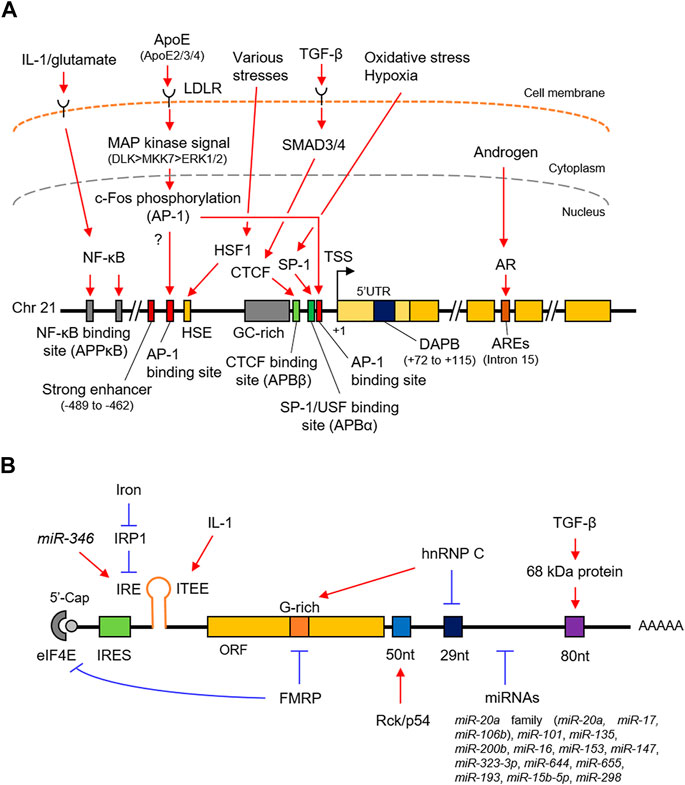
FIGURE 1. Schematic representation of the transcriptional and post-transcriptional regulation of APP. (A). The promoter structure and regulating factors for the APP transcription. (B). cis-regulatory elements and trans-acting factors for the post-transcriptional regulation. APP, amyloid-β precursor protein; SP-1, putative SP-1 binding site; AP-1, putative AP-1 binding site; HSP, heat shock element; DAPB, DNase I protected domain; ARE, androgen response element; AR, androgen receptor. IRES, internal ribosome entry site; IRE, iron responsive element; ITEE, interleukin-1 translation enhancer element; IRP1, iron response protein 1; 52 nt, 52 nt element; 29 nt, 29 nt element; 80 nt, 80 nt element.
Stimulating Protein 1 (SP-1)
SP-1 belongs to the Sp/KLF family of transcription factors and directly binds to the DNA sequence by its own zinc finger motif to enhance gene transcription in response to oxidative stress and hypoxia (Yeh et al., 2011). Indeed, SP-1 binds to the APP promoter (Figure 1A and Table 1) and accelerates the production of APP transcripts (Pollwein, 1993; Hattori et al., 1997; Basha et al., 2005). The SP-1 binding site partially overlaps with the APP promoter binding α (APBα) domain (Figure 1A), an intact nuclear factor-binding site essential for APP transcription. Additionally, the upstream transcription factor (USF), a basic helix-loop-helix transcription factor, also binds to the APBα domain Vostrov et al. (1995) and contributes to an increased APP expression in neurons (Yang et al., 1999).
Activator Protein-1 (AP-1)
AP-1 is a heterodimer composed of proteins belonging to the c-Fos, c-Jun, activating transcription factors (ATFs), and Jun dimerization protein (JDP) families (Shaulian and Karin, 2002). AP-1 regulates gene expression in response to numerous stimuli, including cytokines, growth factors, and stress (Shaulian and Karin, 2002; Hess et al., 2004). Although two putative AP-1 recognition sites are located at the APP promoter, the distal AP-1 recognition site alone is sufficient for transcriptional activation by AP-1, such as c-Fos/-Jun heterodimer, rather than the proximal site (Trejo et al., 1994; Lahiri and Ge, 2004) (Figure 1A and Table 1).
CCCTC-Binding Factor (CTCF) and Transforming Growth Factor-β (TGF-β)
CTCF is generally a multifunctional positive or negative regulator of various target genes and plays a key role in transcriptional insulation (Quitschke et al., 2000). CTCF contains tandem 11 zinc finger motifs, of which five to seven zinc fingers are required for binding to positions –98 and –83 bp of the human APP promoter, which was formerly designated as APBβ, another intact nuclear factor-binding site essential for APP transcription (Quitschke and Goldgaber, 1992; Quitschke, 1994; Quitschke et al., 2000) (Figure 1A and Table 1). APBβ is also responsive to TGF-β, a multifunctional cytokine, in which two TGF-β signaling mediators, mothers against decapentaplegic homolog 3 (SMAD3) and SMAD4, associate with CTCF on the APBβ and promote APP transcription in cooperation with SP-1 (Burton et al., 2002; Docagne et al., 2004).
TGF-β is also implicated in the post-transcriptional regulation of APP. A TGF-β-responsive protein forms a 68 kDa RNA-protein complex and is proposed to stabilize the APP transcript by binding to the 81 nt sequence within the APP 3′UTR, increasing its translation (Amara et al., 1999; Westmark and Malter, 2012).
Heat Shock Transcription Factor 1 (HSF-1)
The transcription of APP is stimulated by various stress factors such as heat shock and treatment with ethanol and arsenite (Dewji et al., 1995). The HSF1 binds to the heat shock element (HSE) of the APP promoter (Dewji and Do, 1996) (Figure 1A and Table 1). It acts as a primary mediator of stress-responsive transcription of pro-survival genes, including heat shock proteins (Akerfelt et al., 2010). Under normal conditions, HSF1 is predominantly localized in the cytoplasm in a repressed monomeric form. Upon stress, HSF1 trimerizes and accumulates in the nucleus, where it binds to HSE.
Nuclear Factor (NF)-κB/Rel
The NF-κB transcription factor forms a dimer composed of NF-κB/Rel family subunits including NF-κB1/p50 and regulates transcription of genes involved in immune and inflammatory responses in response to stimuli such as inflammation and disease (Dresselhaus and Meffert, 2019). NF-κB1/p50-containing complex, widely expressed in neurons and glial cells in the human brain, is activated through the canonical NF-κB pathway extracellularly stimulated by cytokines and neurotransmitter in neurons, where it plays important roles for neuroprotective functions including anti-apoptosis under the neurodegenerative condition, but not well-defined in glial cells. To activate APP transcription, the NF-κB1/p50-containing complex specifically recognize APPκB sites in the distal APP promoter, where IL-1 and glutamate enhance its binding activity (Grilli et al., 1995; Grilli et al., 1996) (Figure 1A and Table 1).
Androgen
Androgens are natural steroid hormones that regulate various physiological phenomena taking place in several tissues and organs, including the brain, by binding to the androgen receptor (AR), a member of the nuclear receptor superfamily (Chang et al., 2013; Takayama and Inoue, 2013). After ligand binding, the AR homodimer translocates into the nucleus, where it binds to the androgen-responsive element (ARE), and subsequently activates gene expression often with epigenetic changes in the chromatin state (Tewari et al., 2012; Nevedomskaya et al., 2016; Nadal et al., 2017; Stelloo et al., 2019). APP is a primary androgen-regulated gene in human neuronal and prostate cancer cells (Takayama et al., 2009; Takayama et al., 2019). In neurons, AR directly binds to the ARE located within the genomic regions corresponding to the 15th intron of the APP gene (Takayama et al., 2019) (Figure 1A and Table 1). Notably, the chromatin binding level of histone H3 acetylated at lysine 9 (H3K9ac), a transcriptionally active histone mark, at the APP promoter is enhanced with overexpression of AR. Importantly, the androgen concentration declines with age in serum and brain (Rosario et al., 2011; Grimm et al., 2016; Gaignard et al., 2017) and is likely associated with AD development (Gouras et al., 2000; Wahjoepramono et al., 2008; César et al., 2016; Jayadevappa et al., 2019).
Apolipoprotein E (ApoE)
ApoE is a major component of low-density lipoprotein (LDL) and very-low-density lipoprotein (VLDL). It acts on the metabolism of fats, including the transportation of lipids, fat-soluble vitamins, and cholesterol into the lymph and blood by binding to the LDL receptor (LDLR) (Goldstein and Brown, 2015). Although it is largely synthesized in the liver, the elevated expression has also been characterized in the brain, primarily in the astrocytes (Holtzman et al., 2012; Wang and Eckel, 2014). Humans harbor three major ApoE alleles: ApoE-ε2, ApoE-ε3, and ApoE-ε4 (Ghebranious et al., 2005; Eisenberg et al., 2010). Importantly, ApoE-ε4 is a well-known genetic risk factor for atherosclerosis and AD in the brain, unlike ApoE-ε2 and ApoE-ε3 (Strittmatter et al., 1993; Farrer et al., 1997; Huang et al., 2017). In the human brain, ApoE proteins secreted from the glia stimulate APP transcription and Aβ production with different efficacy in the neurons (Huang et al., 2017; Vogrinc et al., 2021). In this pathway, secreted ApoE protein binds to the LDLR on the neuron surfaces and activates a non-canonical MAP kinase signaling pathway mediated by DLK, MKK7, and ERK1/2 (Figure 1A and Table 1). Subsequently, c-Fos, a subunit of AP-1, is phosphorylated (Shaulian and Karin, 2002), in turn enhances AP-1–dependent APP transcription (Huang et al., 2017). Notably, ApoE-ε4 is also involved in the recognition and engulfing of Aβ in the brain (Strittmatter et al., 1993; Farrer et al., 1997).
Post-Transcriptional Regulation of APP
We have reviewed the cis-regulatory elements of the APP transcript and trans-acting factors for the post-transcriptional regulation of APP.
Iron
The APP 5′untranslated region (UTR) contains an internal ribosome entry site (IRES), an iron-responsive element (IRE), and an interleukin-1 (IL-1) translation enhancer element (Figure 1B and Table 1). The IRES is a specialized RNA element that allows the recruitment of eukaryotic ribosomes to mRNA, regardless of the presence of the 5′cap (Hellen and Sarnow, 2001; López-Lastra et al., 2005); therefore, endogenous APP is translated in a cap-independent manner (Beaudoin et al., 2008). In the absence of iron, iron response protein 1 (IRP1), an iron-dependent translational repressor, is presumed to bind to the IRE of the APP transcript. This prevents the recruitment of the 40 S ribosome at the 5’cap, subsequently repressing the translation (Rogers et al., 2008; Cho et al., 2010).
Interleukin-1 (IL-1)
An IL-1 translation enhancer element (ITEE), also known as IL-1 acute box, is present close to IRE, (Rogers et al., 2002; Rogers et al., 2008; Ruberti et al., 2010; Bandyopadhyay et al., 2013) (Figure 1B and Table 1). IL-1α and IL-1β, are a group of IL-1 cytokines that play a crucial role in regulating immune and inflammatory responses to not only infections but also in all inflammatory, physiological or pathological phenomena (Dinarello, 2018; Kaneko et al., 2019). It is also known to be increased in the brains of AD patients (Cacabelos et al., 1994; Shaftel et al., 2008; Italiani et al., 2018). Using CAT assay, a 90 nt element in the APP 5′UTR, which includes the enhancer element, was found to enhance its translation without changing the steady-state mRNA level (Rogers et al., 1999; Westmark and Malter, 2012), indicating that IL-1 post-transcriptionally upregulates APP translation.
Fragile X Mental Retardation Protein (FMRP)
The protein-coding region of APP contains a G-rich motif that interacts with the FMRP (Figure 1B and Table 1) which is highly expressed in the brain. Loss of FMRP causes fragile X syndrome, largely characterized by cognitive impairment (O'Donnell and Warren, 2002). FMRP associates with the APP transcript via its own multiple RNA-binding motifs such as KH motif and RGG box to repress the translation of APP in a type 1 metabotropic glutamate receptor (mGluR)-dependent manner; thus, stimulation of mGluR elicits an increase in the APP translation (Westmark and Malter, 2007). In addition, FMRP interacts with cytoplasmic FMR1–interacting protein 1 (CYFIP1), thereby inhibiting APP translation by sequestering eukaryotic initiation factor 4E (eIF4E) (Napoli et al., 2008). Notably, loss of FMRP results in the production of excess soluble APP, which contributes to a deficiency in dendrite maturation (Pasciuto et al., 2015). Normalizing APP levels in Fmrp-knockout mice can rescue the fragile X phenotypes (Westmark et al., 2011), indicating the importance of APP homeostasis in the development of this disorder.
Heterogeneous Nuclear Ribonucleoprotein C (hnRNP C)
It has been reported that hnRNP C, a ubiquitous RNA regulatory protein, competitively binds to the same G-rich motif as FMRP (Figure 1B and Table 1). It harbors an RNA recognition motif (RRM) and is associated with pre-mRNAs to regulate RNA processing, metabolism, and transport (Piñol-Roma and Dreyfuss, 1993; Han et al., 2010). In contrast to FMRP, hnRNP C enhances APP translation by binding to the G-rich motif. Additionally, hnRNP C also binds to the repressive 29 nt element in the APP 3′UTR, thereby increasing its stability (Rajagopalan et al., 1998).
Rck/p54
Rck/p54, a member of the DEAD-box family of RNA helicases is also known as DEAD-box helicase 6 (DDX6). Rck/p54 modulates mRNA secondary structures Akao et al. (1995) by binding to the 52 nt elements downstream of the stop codon and increasing APP mRNA stability (Broytman et al., 2009) (Figure 1B and Table 1). Indeed, the helicase activity of Rck/p54 is required for APP mRNA stability.
Polypyrimidine Tract-Binding Protein-Associated Splicing Factor/Splicing Factor Proline- and Glutamine-Rich (PSF/SFPQ)
PSF, also known as SFPQ, is a ubiquitously expressed nuclear RNA-binding protein (RBP) (Knott et al., 2016). PSF/SFPQ is mainly localized at the nucleus, in particular to the membraneless condensates known as paraspeckles, in which nuclear enriched abundant transcript 1 (NEAT1), an architectural long non-coding RNA, is bound to core proteins, including PSF/SFPQ. The latter regulates various cellular mechanisms such as alternative splicing and nuclear retention of mRNAs (Nakagawa et al., 2018; Lim et al., 2020). Importantly, PSF/SFPQ plays a critical role in neural development as well as in neurodegenerative diseases, including AD (Ke et al., 2012; Lu et al., 2018; Younas et al., 2020). It directly binds to the primary APP transcripts in human neuronal cells, leading to APP mRNA stabilization (Takayama et al., 2019) (Figure 1B and Table 1).
microRNA (miRNA)
RNA silencing is a nucleotide-sequence-specific regulation of gene expression mediated by small non-coding RNAs such as miRNAs (Ghildiyal and Zamore, 2009; Kim et al., 2009; Czech and Hannon, 2011). In most cases, miRNAs interact with the 3′UTR of target mRNAs to induce their degradation by mRNA decay or inhibit their translation. In recent years, several miRNAs have been identified to participate in AD pathogenesis by regulating the expression of multiple target genes, including APP. miR-106a and miR-520c were the first miRNAs experimentally demonstrated to downregulate APP levels post-transcriptionally (Patel et al., 2008). In subsequent studies, further 15 miRNAs, as listed in Table 1, have been identified to bind directly to the 3′UTR of the human APP transcript and downregulate its expression at the post-transcriptional level. Unlike most miRNAs, miR-346 interacts with the APP 5′UTR to promote translation (Long et al., 2019) (Figure 1B). The miR-346 target site overlaps with the IRE at the APP 5′UTR, where miR-346 would displace IRP1 even at low iron levels, eliciting APP translation, suggesting that miR-346 may maintain APP homeostasis and prevent the pathogenic APP cascade in AD.
Conclusion
Here, we summarized multiple regulatory mechanisms of APP mRNA at the transcriptional and post-transcriptional levels, particularly in the human brain. An imbalance in APP levels caused by aberrancy of these mechanisms can trigger increased AD development, as mentioned above.
Perspective
Immunotherapy against ApoE improves amyloid-associated phenotypes rather than Aβ (Xiong et al., 2021), suggesting elucidation of the regulatory mechanisms of APP expression can provide effective therapeutic strategies to interrupt the development or further progression of AD. Moreover, recent advances in high-throughput sequencing technologies have facilitated the reconstruction of the entire transcriptional landscape and RNA–RBP networks in human diseases. In addition, more recently, RBPs such as transactive response DNA-binding protein 43 (TDP-43) and fused in sarcoma (FUS) related to neurodegenerative diseases have been shown to undergo liquid-liquid phase separation (LLPS). Aberrant phase transitions of these RBPs in the brain lead to the disorder (Patel et al., 2015; Wolozin and Ivanov, 2019; Boyko et al., 2020), suggesting a possible role of LLPS in AD-related RBPs in APP homeostasis.
Author Contributions
All authors listed have made a substantial, direct, and intellectual contribution to the work and approved it for publication.
Funding
This work was supported by the grants received from Japan Society for the Promotion of Science to KS (20K06596), KT (20K07350), MH, and SI (20K21667 and 21H04829). by grants from the Japan Geriatric Society Research Grant Award in Geriatrics and Gerontology (KT).
Conflict of Interest
The authors declare that the research was conducted in the absence of any commercial or financial relationships that could be construed as a potential conflict of interest.
Publisher’s Note
All claims expressed in this article are solely those of the authors and do not necessarily represent those of their affiliated organizations, or those of the publisher, the editors and the reviewers. Any product that may be evaluated in this article, or claim that may be made by its manufacturer, is not guaranteed or endorsed by the publisher.
References
Akao, Y., Marukawa, O., Morikawa, H., Nakao, K., Kamei, M., Hachiya, T., et al. (1995). The Rck/p54 Candidate Proto-Oncogene Product Is a 54-kilodalton D-E-A-D Box Protein Differentially Expressed in Human and Mouse Tissues. Cancer Res. 55, 3444–3449.
Åkerfelt, M., Morimoto, R. I., and Sistonen, L. (2010). Heat Shock Factors: Integrators of Cell Stress, Development and Lifespan. Nat. Rev. Mol. Cel. Biol. 11, 545–555. doi:10.1038/nrm2938
Amara, F. M., Junaid, A., Clough, R. R., and Liang, B. (1999). TGF-β1, Regulation of Alzheimer Amyloid Precursor Protein mRNA Expression in a normal Human Astrocyte Cell Line: mRNA Stabilization. Mol. Brain Res. 71, 42–49. doi:10.1016/s0169-328x(99)00158-8
Antonarakis, S. E. (2017). Down Syndrome and the Complexity of Genome Dosage Imbalance. Nat. Rev. Genet. 18, 147–163. doi:10.1038/nrg.2016.154
Bandyopadhyay, S., Cahill, C., Balleidier, A., Huang, C., Lahiri, D. K., Huang, X., et al. (2013). Novel 5′ Untranslated Region Directed Blockers of Iron-Regulatory Protein-1 Dependent Amyloid Precursor Protein Translation: Implications for Down Syndrome and Alzheimer's Disease. PLoS One 8, e65978. doi:10.1371/journal.pone.0065978
Barbato, C., Pezzola, S., Caggiano, C., Antonelli, M., Frisone, P., Ciotti, M. T., et al. (2014). A Lentiviral Sponge for miR-101 Regulates RanBP9 Expression and Amyloid Precursor Protein Metabolism in Hippocampal Neurons. Front. Cel. Neurosci. 8, 37. doi:10.3389/fncel.2014.00037
Basha, M. R., Wei, W., Bakheet, S. A., Benitez, N., Siddiqi, H. K., Ge, Y. W., et al. (2005). The Fetal Basis of Amyloidogenesis: Exposure to Lead and Latent Overexpression of Amyloid Precursor Protein and -Amyloid in the Aging Brain. J. Neurosci. 25, 823–829. doi:10.1523/JNEUROSCI.4335-04.2005
Bateman, R. J., Xiong, C., Benzinger, T. L. S., Fagan, A. M., Goate, A., Fox, N. C., et al. (2012). Clinical and Biomarker Changes in Dominantly Inherited Alzheimer's Disease. N. Engl. J. Med. 367, 795–804. doi:10.1056/NEJMoa1202753
Beaudoin, M. E., Poirel, V.-J., and Krushel, L. A. (2008). Regulating Amyloid Precursor Protein Synthesis through an Internal Ribosomal Entry Site. Nucleic Acids Res. 36, 6835–6847. doi:10.1093/nar/gkn792
Boyko, S., Surewicz, K., and Surewicz, W. K. (2020). Regulatory Mechanisms of Tau Protein Fibrillation under the Conditions of Liquid-Liquid Phase Separation. Proc. Natl. Acad. Sci. USA 117, 31882–31890. doi:10.1073/pnas.2012460117
Broytman, O., Westmark, P. R., Gurel, Z., and Malter, J. S. (2009). Rck/p54 Interacts with APP mRNA as Part of a Multi-Protein Complex and Enhances APP mRNA and Protein Expression in Neuronal Cell Lines. Neurobiol. Aging 30, 1962–1974. doi:10.1016/j.neurobiolaging.2008.02.011
Burton, T., Liang, B., Dibrov, A., and Amara, F. (2002). Transforming Growth Factor-β-Induced Transcription of the Alzheimer β-amyloid Precursor Protein Gene Involves Interaction between the CTCF-Complex and Smads. Biochem. Biophysical Res. Commun. 295, 713–723. doi:10.1016/s0006-291x(02)00725-8
Bushman, D. M., Kaeser, G. E., Siddoway, B., Westra, J. W., Rivera, R. R., Rehen, S. K., et al. (2015). Genomic Mosaicism with Increased Amyloid Precursor Protein (APP) Gene Copy Number in Single Neurons from Sporadic Alzheimer's Disease Brains. Elife 4, e05116. doi:10.7554/eLife.05116
Cacabelos, R., Alvarez, X. A., Fernández-Novoa, L., Franco, A., Mangues, R., Pellicer, A., et al. (1994). Brain Interleukin-1 Beta in Alzheimer's Disease and Vascular Dementia. Methods Find Exp. Clin. Pharmacol. 16, 141–151.
César, K. G., Brucki, S. M. D., Takada, L. T., Nascimento, L. F. C., Gomes, C. M. S., Almeida, M. C. S., et al. (2016). Prevalence of Cognitive Impairment without Dementia and Dementia in Tremembé, Brazil. Alzheimer Dis. Assoc. Disord. 30, 264–271. doi:10.1097/WAD.0000000000000122
Chang, C., Yeh, S., Lee, S. O., and Chang, T.-m. (2013). Androgen Receptor (AR) Pathophysiological Roles in Androgen Related Diseases in Skin, Metabolism Syndrome, Bone/Muscle and Neuron/Immune Systems: Lessons Learned from Mice Lacking AR in Specific Cells. Nucl. Recept. Signal. 11, nrs.11001–e001. doi:10.1621/nrs.11001
Cho, H.-H., Cahill, C. M., Vanderburg, C. R., Scherzer, C. R., Wang, B., Huang, X., et al. (2010). Selective Translational Control of the Alzheimer Amyloid Precursor Protein Transcript by Iron Regulatory Protein-1. J. Biol. Chem. 285, 31217–31232. doi:10.1074/jbc.M110.149161
Chopra, N., Wang, R., Maloney, B., Nho, K., Beck, J. S., Pourshafie, N., et al. (2020). MicroRNA-298 Reduces Levels of Human Amyloid-β Precursor Protein (APP), β-site APP-Converting Enzyme 1 (BACE1) and Specific Tau Protein Moieties. Mol. Psychiatry. doi:10.1038/s41380-019-0610-2
Cohen, M. L., Golde, T. E., Usiak, M. F., Younkin, L. H., and Younkin, S. G. (1988). In Situ hybridization of Nucleus Basalis Neurons Shows Increased Beta-Amyloid mRNA in Alzheimer Disease. Proc. Natl. Acad. Sci. 85, 1227–1231. doi:10.1073/pnas.85.4.1227
Czech, B., and Hannon, G. J. (2011). Small RNA Sorting: Matchmaking for Argonautes. Nat. Rev. Genet. 12, 19–31. doi:10.1038/nrg2916
Dai, M.-H., Zheng, H., Zeng, L.-D., and Zhang, Y. (2018). The Genes Associated with Early-Onset Alzheimer's Disease. Oncotarget 9, 15132–15143. doi:10.18632/oncotarget.23738
Dar, N. J., and Glazner, G. W. (2020). Deciphering the Neuroprotective and Neurogenic Potential of Soluble Amyloid Precursor Protein Alpha (sAPPα). Cell. Mol. Life Sci. 77, 2315–2330. doi:10.1007/s00018-019-03404-x
Delay, C., Calon, F., Mathews, P., and Hébert, S. S. (2011). Alzheimer-specific Variants in the 3'UTR of Amyloid Precursor Protein Affect microRNA Function. Mol. Neurodegeneration 6, 70. doi:10.1186/1750-1326-6-70
DeTure, M. A., and Dickson, D. W. (2019). The Neuropathological Diagnosis of Alzheimer's Disease. Mol. Neurodegeneration 14, 32. doi:10.1186/s13024-019-0333-5
Dewji, N. N., Do, C., and Bayney, R. M. (1995). Transcriptional Activation of Alzheimer's β-amyloid Precursor Protein Gene by Stress. Mol. Brain Res. 33, 245–253. doi:10.1016/0169-328x(95)00131-b
Dewji, N. N., and Do, C. (1996). Heat Shock Factor-1 Mediates the Transcriptional Activation of Alzheimer's β-amyloid Precursor Protein Gene in Response to Stress. Mol. Brain Res. 35, 325–328. doi:10.1016/0169-328x(95)00214-d
Dinarello, C. A. (2018). Overview of the IL-1 Family in Innate Inflammation and Acquired Immunity. Immunol. Rev. 281, 8–27. doi:10.1111/imr.12621
Docagne, F., Gabriel, C., Lebeurrier, N., Lesné, S., Hommet, Y., Plawinski, L., et al. (2004). Sp1 and Smad Transcription Factors Co-operate to Mediate TGF-β-dependent Activation of Amyloid-β Precursor Protein Gene Transcription. Biochem. J. 383, 393–399. doi:10.1042/BJ20040682
Dresselhaus, E. C., and Meffert, M. K. (2019). Cellular Specificity of NF-Κb Function in the Nervous System. Front. Immunol. 10, 1043. doi:10.3389/fimmu.2019.01043
Eisenberg, D. T. A., Kuzawa, C. W., and Hayes, M. G. (2010). Worldwide Allele Frequencies of the Human Apolipoprotein E Gene: Climate, Local Adaptations, and Evolutionary History. Am. J. Phys. Anthropol. 143, 100–111. doi:10.1002/ajpa.21298
Farrer, L. A., Cupples, L. A., Haines, J. L., Hyman, B., Kukull, W. A., Mayeux, R., et al. (1997). Effects of Age, Sex, and Ethnicity on the Association between Apolipoprotein E Genotype and Alzheimer Disease. A Meta-Analysis. APOE and Alzheimer Disease Meta Analysis Consortium. JAMA 278, 1349–1356. doi:10.1001/jama.278.16.1349
Gaignard, P., Liere, P., Thérond, P., Schumacher, M., Slama, A., and Guennoun, R. (2017). Role of Sex Hormones on Brain Mitochondrial Function, with Special Reference to Aging and Neurodegenerative Diseases. Front. Aging Neurosci. 9, 406. doi:10.3389/fnagi.2017.00406
Galimberti, D., Villa, C., Fenoglio, C., Serpente, M., Ghezzi, L., Cioffi, S. M. G., et al. (2014). Circulating miRNAs as Potential Biomarkers in Alzheimer's Disease. Jad 42, 1261–1267. doi:10.3233/JAD-140756
Ghebranious, N., Ivacic, L., Mallum, J., and Dokken, C. (2005). Detection of ApoE E2, E3 and E4 Alleles Using MALDI-TOF Mass Spectrometry and the Homogeneous Mass-Extend Technology. Nucleic Acids Res. 33, e149. doi:10.1093/nar/gni155
Ghildiyal, M., and Zamore, P. D. (2009). Small Silencing RNAs: an Expanding Universe. Nat. Rev. Genet. 10, 94–108. doi:10.1038/nrg2504
Goldstein, J. L., and Brown, M. S. (2015). A century of Cholesterol and Coronaries: from Plaques to Genes to Statins. Cell 161, 161–172. doi:10.1016/j.cell.2015.01.036
Gouras, G. K., Xu, H., Gross, R. S., Greenfield, J. P., Hai, B., Wang, R., et al. (2000). Testosterone Reduces Neuronal Secretion of Alzheimer's Beta -amyloid Peptides. Proc. Natl. Acad. Sci. 97, 1202–1205. doi:10.1073/pnas.97.3.1202
Grilli, M., Goffi, F., Memo, M., and Spano, P. (1996). Interleukin-1β and Glutamate Activate the NF-κB/Rel Binding Site from the Regulatory Region of the Amyloid Precursor Protein Gene in Primary Neuronal Cultures. J. Biol. Chem. 271, 15002–15007. doi:10.1074/jbc.271.25.15002
Grilli, M., Ribola, M., Alberici, A., Valerio, A., Memo, M., and Spano, P. (1995). Identification and Characterization of a κB/Rel Binding Site in the Regulatory Region of the Amyloid Precursor Protein Gene. J. Biol. Chem. 270, 26774–26777. doi:10.1074/jbc.270.45.26774
Grimm, A., Mensah-Nyagan, A. G., and Eckert, A. (2016). Alzheimer, Mitochondria and Gender. Neurosci. Biobehavioral Rev. 67, 89–101. doi:10.1016/j.neubiorev.2016.04.012
Guttula, S. V., Allam, A., and Gumpeny, R. S. (2012). Analyzing Microarray Data of Alzheimer's Using Cluster Analysis to Identify the Biomarker Genes. Int. J. Alzheimers Dis. 2012, 649456. doi:10.1155/2012/649456
Guyant-Maréchal, L., Rovelet-Lecrux, A., Goumidi, L., Cousin, E., Hannequin, D., Raux, G., et al. (2007). Variations in the APP Gene Promoter Region and Risk of Alzheimer Disease. Neurology 68, 684–687. doi:10.1212/01.wnl.0000255938.33739.46
Han, S. P., Tang, Y. H., and Smith, R. (2010). Functional Diversity of the hnRNPs: Past, Present and Perspectives. Biochem. J. 430, 379–392. doi:10.1042/BJ20100396
Hashimoto, M., Ho, G., Sugama, S., Takamatsu, Y., Shimizu, Y., Takenouchi, T., et al. (2018). Evolvability of Amyloidogenic Proteins in Human Brain. J. Alzheimers Dis. 62, 73–83. doi:10.3233/JAD-170894
Hattori, M., Tsukahara, F., Furuhata, Y., Tanahashi, H., Hirose, M., Saito, M., et al. (1997). A Novel Method for Making Nested Deletions and its Application for Sequencing of a 300 Kb Region of Human APP Locus. Nucleic Acids Res. 25, 1802–1808. doi:10.1093/nar/25.9.1802
Hébert, S. S., Horré, K., Nicolaï, L., Bergmans, B., Papadopoulou, A. S., Delacourte, A., et al. (2009). MicroRNA Regulation of Alzheimer's Amyloid Precursor Protein Expression. Neurobiol. Dis. 33, 422–428. doi:10.1016/j.nbd.2008.11.009
Hellen, C. U. T., and Sarnow, P. (2001). Internal Ribosome Entry Sites in Eukaryotic mRNA Molecules. Genes Dev. 15, 1593–1612. doi:10.1101/gad.891101
Hess, J., Angel, P., and Schorpp-Kistner, M. (2004). AP-1 Subunits: Quarrel and harmony Among Siblings. J. Cel. Sci. 117, 5965–5973. doi:10.1242/jcs.01589
Higgins, G. A., Lewis, D. A., Bahmanyar, S., Goldgaber, D., Gajdusek, D. C., Young, W. G., et al. (1988). Differential Regulation of Amyloid-Beta-Protein mRNA Expression within Hippocampal Neuronal Subpopulations in Alzheimer Disease. Proc. Natl. Acad. Sci. 85, 1297–1301. doi:10.1073/pnas.85.4.1297
Holtzman, D. M., Herz, J., and Bu, G. (2012). Apolipoprotein E and Apolipoprotein E Receptors: normal Biology and Roles in Alzheimer Disease. Cold Spring Harbor Perspect. Med. 2, a006312. doi:10.1101/cshperspect.a006312
Hooli, B. V., Mohapatra, G., Mattheisen, M., Parrado, A. R., Roehr, J. T., Shen, Y., et al. (2012). Role of Common and Rare APP DNA Sequence Variants in Alzheimer Disease. Neurology 78, 1250–1257. doi:10.1212/WNL.0b013e3182515972
Huang, Y.-W. A., Zhou, B., Wernig, M., and Südhof, T. C. (2017). ApoE2, ApoE3, and ApoE4 Differentially Stimulate APP Transcription and Aβ Secretion. Cell 168, 427–441.e21. doi:10.1016/j.cell.2016.12.044
Italiani, P., Puxeddu, I., Napoletano, S., Scala, E., Melillo, D., Manocchio, S., et al. (2018). Circulating Levels of IL-1 Family Cytokines and Receptors in Alzheimer's Disease: New Markers of Disease Progression?. J. Neuroinflammation 15, 342. doi:10.1186/s12974-018-1376-1
Jayadevappa, R., Chhatre, S., Malkowicz, S. B., Parikh, R. B., Guzzo, T., and Wein, A. J. (2019). Association between Androgen Deprivation Therapy Use and Diagnosis of Dementia in Men with Prostate Cancer. JAMA Netw. Open 2, e196562. doi:10.1001/jamanetworkopen.2019.6562
Kaneko, N., Kurata, M., Yamamoto, T., Morikawa, S., and Masumoto, J. (2019). The Role of Interleukin-1 in General Pathology. Inflamm. Regener 39, 12. doi:10.1186/s41232-019-0101-5
Kasuga, K., Shimohata, T., Nishimura, A., Shiga, A., Mizuguchi, T., Tokunaga, J., et al. (2009). Identification of Independent APP Locus Duplication in Japanese Patients with Early-Onset Alzheimer Disease. J. Neurol. Neurosurg. Psychiatry 80, 1050–1052. doi:10.1136/jnnp.2008.161703
Ke, Y., Dramiga, J., Schütz, U., Kril, J. J., Ittner, L. M., Schröder, H., et al. (2012). Tau-mediated Nuclear Depletion and Cytoplasmic Accumulation of SFPQ in Alzheimer's and Pick's Disease. PLoS One 7, e35678. doi:10.1371/journal.pone.0035678
Kim, V. N., Han, J., and Siomi, M. C. (2009). Biogenesis of Small RNAs in Animals. Nat. Rev. Mol. Cel. Biol. 10, 126–139. doi:10.1038/nrm2632
Kirova, A.-M., Bays, R. B., and Lagalwar, S. (2015). Working Memory and Executive Function Decline across normal Aging, Mild Cognitive Impairment, and Alzheimer's Disease. Biomed. Res. Int. 2015, 1–9. doi:10.1155/2015/748212
Knott, G. J., Bond, C. S., and Fox, A. H. (2016). The DBHS Proteins SFPQ, NONO and PSPC1: a Multipurpose Molecular Scaffold. Nucleic Acids Res. 44, 3989–4004. doi:10.1093/nar/gkw271
Korbel, J. O., Tirosh-Wagner, T., Urban, A. E., Chen, X.-N., Kasowski, M., Dai, L., et al. (2009). The Genetic Architecture of Down Syndrome Phenotypes Revealed by High-Resolution Analysis of Human Segmental Trisomies. Proc. Natl. Acad. Sci. 106, 12031–12036. doi:10.1073/pnas.0813248106
Lahiri, D. K., and Ge, Y.-W. (2004). Role of the APP Promoter in Alzheimer's Disease: Cell Type-specific Expression of the β-Amyloid Precursor Protein. Ann. N. Y. Acad. Sci. 1030, 310–316. doi:10.1196/annals.1329.039
Lahiri, D. K., and Nall, C. (1995). Promoter Activity of the Gene Encoding the Beta-Amyloid Precursor Protein Is Up-Regulated by Growth Factors, Phorbol Ester, Retinoic Acid and Interleukin-1. Mol. Brain Res. 32, 233–240. doi:10.1016/0169-328x(95)00078-7
Lahiri, D. K., and Robakis, N. K. (1991). The Promoter Activity of the Gene Encoding Alzheimer β-amyloid Precursor Protein (APP) Is Regulated by Two Blocks of Upstream Sequences. Mol. Brain Res. 9, 253–257. doi:10.1016/0169-328x(91)90009-m
Liang, C., Zhu, H., Xu, Y., Huang, L., Ma, C., Deng, W., et al. (2012). MicroRNA-153 Negatively Regulates the Expression of Amyloid Precursor Protein and Amyloid Precursor-like Protein 2. Brain Res. 1455, 103–113. doi:10.1016/j.brainres.2011.10.051
Lim, Y. W., James, D., Huang, J., and Lee, M. (2020). The Emerging Role of the RNA-Binding Protein SFPQ in Neuronal Function and Neurodegeneration. Int. J. Mol. Sci. 21, 7151. doi:10.3390/ijms21197151
Liu, C.-G., Song, J., Zhang, Y.-Q., and Wang, P.-C. (2014b). MicroRNA-193b Is a Regulator of Amyloid Precursor Protein in the Blood and Cerebrospinal Fluid Derived Exosomal microRNA-193b Is a Biomarker of Alzheimer's Disease. Mol. Med. Rep. 10, 2395–2400. doi:10.3892/mmr.2014.2484
Liu, C.-g., Wang, J.-l., Li, L., Xue, L.-x., Zhang, Y.-q., and Wang, P.-c. (2014a). MicroRNA-135a and -200b, Potential Biomarkers for Alzheimer׳s Disease, Regulate β Secretase and Amyloid Precursor Protein. Brain Res. 1583, 55–64. doi:10.1016/j.brainres.2014.04.026
Liu, H. Y., Fu, X., Li, Y. F., Li, X. L., Ma, Z. Y., Zhang, Y., et al. (2019). miR-15b-5p Targeting Amyloid Precursor Protein Is Involved in the Anti-amyloid Eflect of Curcumin in swAPP695-Hek293 Cells. Neural Regen. Res. 14, 1603–1609. doi:10.4103/1673-5374.255979
Long, J. M., and Lahiri, D. K. (2011). MicroRNA-101 Downregulates Alzheimer's Amyloid-β Precursor Protein Levels in Human Cell Cultures and Is Differentially Expressed. Biochem. Biophysical Res. Commun. 404, 889–895. doi:10.1016/j.bbrc.2010.12.053
Long, J. M., Maloney, B., Rogers, J. T., and Lahiri, D. K. (2019). Novel Upregulation of Amyloid-β Precursor Protein (APP) by microRNA-346 via Targeting of APP mRNA 5′-untranslated Region: Implications in Alzheimer's Disease. Mol. Psychiatry 24, 345–363. doi:10.1038/s41380-018-0266-3
Long, J. M., Ray, B., and Lahiri, D. K. (2012). MicroRNA-153 Physiologically Inhibits Expression of Amyloid-β Precursor Protein in Cultured Human Fetal Brain Cells and Is Dysregulated in a Subset of Alzheimer Disease Patients. J. Biol. Chem. 287, 31298–31310. doi:10.1074/jbc.M112.366336
López-lastra, M., Rivas, A., and Barría, M. I. (2005). Protein Synthesis in Eukaryotes: the Growing Biological Relevance of Cap-independent Translation Initiation. Biol. Res. 38, 121–146. doi:10.4067/s0716-97602005000200003
Lott, I. T., and Head, E. (2019). Dementia in Down Syndrome: Unique Insights for Alzheimer Disease Research. Nat. Rev. Neurol. 15, 135–147. doi:10.1038/s41582-018-0132-6
Lu, J., Shu, R., and Zhu, Y. (2018). Dysregulation and Dislocation of SFPQ Disturbed DNA Organization in Alzheimer's Disease and Frontotemporal Dementia. J. Alzheimers Dis. 61, 1311–1321. doi:10.3233/JAD-170659
Mucke, L., and Selkoe, D. J. (2012). Neurotoxicity of Amyloid -Protein: Synaptic and Network Dysfunction. Cold Spring Harbor Perspect. Med. 2, a006338. doi:10.1101/cshperspect.a006338
Nadal, M., Prekovic, S., Gallastegui, N., Helsen, C., Abella, M., Zielinska, K., et al. (2017). Structure of the Homodimeric Androgen Receptor Ligand-Binding Domain. Nat. Commun. 8, 14388. doi:10.1038/ncomms14388
Nakagawa, S., Yamazaki, T., and Hirose, T. (2018). Molecular Dissection of Nuclear Paraspeckles: towards Understanding the Emerging World of the RNP Milieu. Open Biol. 8, 180150. doi:10.1098/rsob.180150
Napoli, I., Mercaldo, V., Boyl, P. P., Eleuteri, B., Zalfa, F., De Rubeis, S., et al. (2008). The Fragile X Syndrome Protein Represses Activity-dependent Translation through CYFIP1, a New 4E-BP. Cell 134, 1042–1054. doi:10.1016/j.cell.2008.07.031
Nevedomskaya, E., Stelloo, S., van der Poel, H. G., de Jong, J., Wessels, L. F. A., Bergman, A. M., et al. (2016). Androgen Receptor DNA Binding and Chromatin Accessibility Profiling in Prostate Cancer. Genomics Data 7, 124–126. doi:10.1016/j.gdata.2015.12.020
Nguyen, K. V. (2019). β-Amyloid Precursor Protein (APP) and the Human Diseases. AIMS Neurosci. 6, 273–281. doi:10.3934/Neuroscience.2019.4.273
O'Donnell, W. T., and Warren, S. T. (2002). A Decade of Molecular Studies of Fragile X Syndrome. Annu. Rev. Neurosci. 25, 315–338. doi:10.1146/annurev.neuro.25.112701.142909
Panza, F., Lozupone, M., Logroscino, G., and Imbimbo, B. P. (2019). A Critical Appraisal of Amyloid-β-Targeting Therapies for Alzheimer Disease. Nat. Rev. Neurol. 15, 73–88. doi:10.1038/s41582-018-0116-6
Pasciuto, E., Ahmed, T., Wahle, T., Gardoni, F., D’Andrea, L., Pacini, L., et al. (2015). Dysregulated ADAM10-Mediated Processing of APP during a Critical Time Window Leads to Synaptic Deficits in Fragile X Syndrome. Neuron 87, 382–398. doi:10.1016/j.neuron.2015.06.032
Patel, A., Lee, H. O., Jawerth, L., Maharana, S., Jahnel, M., Hein, M. Y., et al. (2015). A Liquid-To-Solid Phase Transition of the ALS Protein FUS Accelerated by Disease Mutation. Cell 162, 1066–1077. doi:10.1016/j.cell.2015.07.047
Patel, N., Hoang, D., Miller, N., Ansaloni, S., Huang, Q., Rogers, J. T., et al. (2008). MicroRNAs Can Regulate Human APP Levels. Mol. Neurodegeneration 3, 10. doi:10.1186/1750-1326-3-10
Piñol-Roma, S., and Dreyfuss, G. (1993). hnRNP proteins:Localization and Transport between the Nucleus and the Cytoplasm. Trends Cel Biol. 3, 151–155. doi:10.1016/0962-8924(93)90135-n
Pollwein, P. (1993). Overlapping Binding Sites of Two Different Transcription Factors in the Promoter of the Human Gene for the Alzheimer Amyloid Precursor Protein. Biochem. Biophys. Res. Commun. 190, 637–647. doi:10.1006/bbrc.1993.1096
Prasher, V. P., Farrer, M. J., Kessling, A. M., Fisher, E. M. C., West, R. J., Barber, P. C., et al. (1998). Molecular Mapping of Alzheimer-type Dementia in Down's Syndrome. Ann. Neurol. 43, 380–383. doi:10.1002/ana.410430316
Quitschke, W. W., and Goldgaber, D. (1992). The Amyloid Beta-Protein Precursor Promoter. A Region Essential for Transcriptional Activity Contains a Nuclear Factor Binding Domain. J. Biol. Chem. 267, 17362–17368. doi:10.1016/s0021-9258(18)41934-5
Quitschke, W. W., Taheny, M. J., Fochtmann, L. J., and Vostrov, A. A. (2000). Differential Effect of Zinc finger Deletions on the Binding of CTCF to the Promoter of the Amyloid Precursor Protein Gene. Nucleic Acids Res. 28, 3370–3378. doi:10.1093/nar/28.17.3370
Quitschke, W. W. (1994). Two Nuclear Factor Binding Domains Activate Expression from the Human Amyloid Beta-Protein Precursor Promoter. J. Biol. Chem. 269, 21229–21233. doi:10.1016/s0021-9258(17)31952-x
Rajagopalan, L. E., Westmark, C. J., Jarzembowski, J. A., and Malter, J. S. (1998). hnRNP C Increases Amyloid Precursor Protein (APP) Production by Stabilizing APP mRNA. Nucleic Acids Res. 26, 3418–3423. doi:10.1093/nar/26.14.3418
Rogers, J. T., Bush, A. I., Cho, H.-H., Smith, D. H., Thomson, A. M., Friedlich, A. L., et al. (2008). Iron and the Translation of the Amyloid Precursor Protein (APP) and Ferritin mRNAs: Riboregulation against Neural Oxidative Damage in Alzheimer's Disease. Biochem. Soc. Trans. 36, 1282–1287. doi:10.1042/BST0361282
Rogers, J. T., Leiter, L. M., McPhee, J., Cahill, C. M., Zhan, S.-S., Potter, H., et al. (1999). Translation of the Alzheimer Amyloid Precursor Protein mRNA Is Up-Regulated by Interleukin-1 through 5′-Untranslated Region Sequences. J. Biol. Chem. 274, 6421–6431. doi:10.1074/jbc.274.10.6421
Rogers, J. T., Randall, J. D., Cahill, C. M., Eder, P. S., Huang, X., Gunshin, H., et al. (2002). An Iron-Responsive Element Type II in the 5′-Untranslated Region of the Alzheimer's Amyloid Precursor Protein Transcript. J. Biol. Chem. 277, 45518–45528. doi:10.1074/jbc.M207435200
Rosario, E. R., Chang, L., Head, E. H., Stanczyk, F. Z., and Pike, C. J. (2011). Brain Levels of Sex Steroid Hormones in Men and Women during normal Aging and in Alzheimer's Disease. Neurobiol. Aging 32, 604–613. doi:10.1016/j.neurobiolaging.2009.04.008
Rovelet-Lecrux, A., Hannequin, D., Raux, G., Meur, N. L., Laquerrière, A., Vital, A., et al. (2006). APP Locus Duplication Causes Autosomal Dominant Early-Onset Alzheimer Disease with Cerebral Amyloid Angiopathy. Nat. Genet. 38, 24–26. doi:10.1038/ng1718
Ruberti, F., Barbato, C., and Cogoni, C. (2010). Post-transcriptional Regulation of Amyloid Precursor Protein by microRNAs and RNA Binding Proteins. Commun. Integr. Biol. 3, 499–503. doi:10.4161/cib.3.6.13172
Shaftel, S. S., Griffin, W. S. T., and O'Banion, M. K. (2008). The Role of Interleukin-1 in Neuroinflammation and Alzheimer Disease: an Evolving Perspective. J. Neuroinflammation 5, 7. doi:10.1186/1742-2094-5-7
Shaulian, E., and Karin, M. (2002). AP-1 as a Regulator of Cell Life and Death. Nat. Cel Biol. 4, E131–E136. doi:10.1038/ncb0502-e131
Sleegers, K., Brouwers, N., Gijselinck, I., Theuns, J., Goossens, D., Wauters, J., et al. (2006). APP Duplication Is Sufficient to Cause Early Onset Alzheimer's Dementia with Cerebral Amyloid Angiopathy. Brain 129, 2977–2983. doi:10.1093/brain/awl203
Smale, S. T., and Kadonaga, J. T. (2003). The RNA Polymerase II Core Promoter. Annu. Rev. Biochem. 72, 449–479. doi:10.1146/annurev.biochem.72.121801.161520
Stelloo, S., Bergman, A. M., and Zwart, W. (2019). Androgen Receptor Enhancer Usage and the Chromatin Regulatory Landscape in Human Prostate Cancers. Endocr. Relat. Cancer 26, R267–R285. doi:10.1530/ERC-19-0032
Strittmatter, W. J., Weisgraber, K. H., Huang, D. Y., Dong, L. M., Salvesen, G. S., Pericak-Vance, M., et al. (1993). Binding of Human Apolipoprotein E to Synthetic Amyloid Beta Peptide: Isoform-specific Effects and Implications for Late-Onset Alzheimer Disease. Proc. Natl. Acad. Sci. 90, 8098–8102. doi:10.1073/pnas.90.17.8098
Swaminathan, S., Huentelman, M. J., Corneveaux, J. J., Myers, A. J., Faber, K. M., Foroud, T., et al. (2012). Analysis of Copy Number Variation in Alzheimer's Disease in a Cohort of Clinically Characterized and Neuropathologically Verified Individuals. PLoS One 7, e50640. doi:10.1371/journal.pone.0050640
Takayama, K.-i., and Inoue, S. (2013). Transcriptional Network of Androgen Receptor in Prostate Cancer Progression. Int. J. Urol. 20, 756–768. doi:10.1111/iju.12146
Takayama, K.-i., Tsutsumi, S., Suzuki, T., Horie-Inoue, K., Ikeda, K., Kaneshiro, K., et al. (2009). Amyloid Precursor Protein Is a Primary Androgen Target Gene that Promotes Prostate Cancer Growth. Cancer Res. 69, 137–142. doi:10.1158/0008-5472.CAN-08-3633
Takayama, K. I., Fujiwara, K., and Inoue, S. (2019). Amyloid Precursor Protein, an Androgen‐regulated Gene, Is Targeted by RNA‐binding Protein PSF/SFPQ in Neuronal Cells. Genes Cells 24, 719–730. doi:10.1111/gtc.12721
Tewari, A. K., Yardimci, G., Shibata, Y., Sheffield, N. C., Song, L., Taylor, B. S., et al. (2012). Chromatin Accessibility Reveals Insights into Androgen Receptor Activation and Transcriptional Specificity. Genome Biol. 13, R88. doi:10.1186/gb-2012-13-10-r88
Thonberg, H., Fallström, M., Björkström, J., Schoumans, J., Nennesmo, I., and Graff, C. (2011). Mutation Screening of Patients with Alzheimer Disease Identifies APP Locus Duplication in a Swedish Patient. BMC Res. Notes 4, 476. doi:10.1186/1756-0500-4-476
Trejo, J., Massamiri, T., Deng, T., Dewji, N. N., Bayney, R. M., and Brown, J. H. (1994). A Direct Role for Protein Kinase C and the Transcription Factor Jun/AP-1 in the Regulation of the Alzheimer's Beta-Amyloid Precursor Protein Gene. J. Biol. Chem. 269, 21682–21690. doi:10.1016/s0021-9258(17)31860-4
Vilardo, E., Barbato, C., Ciotti, M., Cogoni, C., and Ruberti, F. (2010). MicroRNA-101 Regulates Amyloid Precursor Protein Expression in Hippocampal Neurons. J. Biol. Chem. 285, 18344–18351. doi:10.1074/jbc.M110.112664
Vogrinc, D., Goričar, K., and Dolžan, V. (2021). Genetic Variability in Molecular Pathways Implicated in Alzheimer's Disease: A Comprehensive Review. Front. Aging Neurosci. 13, 646901. doi:10.3389/fnagi.2021.646901
Vostrov, A. A., Quitschke, W. W., Vidal, F., Schwarzman, A. L., and Goldaber, D. (1995). USF Binds to the APBα Sequence in the Promoter of the Amyloid β-protein Precursor Gene. Nucl. Acids Res. 23, 2734–2741. doi:10.1093/nar/23.14.2734
Vostrov, A. A., Taheny, M. J., Izkhakov, N., and Quitschke, W. W. (2010). A Nuclear Factor-Binding Domain in the 5'-untranslated Region of the Amyloid Precursor Protein Promoter: Implications for the Regulation of Gene Expression. BMC Res. Notes 3, 4. doi:10.1186/1756-0500-3-4
Wahjoepramono, E. J., Wijaya, L. K., Taddei, K., Martins, G., Howard, M., de Ruyck, K., et al. (2008). Distinct Effects of Testosterone on Plasma and Cerebrospinal Fluid Amyloid-β Levels. J. Alzheimers Dis. 15, 129–137. doi:10.3233/jad-2008-15111
Wang, H., and Eckel, R. H. (2014). What Are Lipoproteins Doing in the Brain?. Trends Endocrinol. Metab. 25, 8–14. doi:10.1016/j.tem.2013.10.003
Westmark, C. J., and Malter, J. S. (2007). FMRP Mediates mGluR5-dependent Translation of Amyloid Precursor Protein. Plos Biol. 5, e52. doi:10.1371/journal.pbio.0050052
Westmark, C. J., and Malter, J. S. (2012). The Regulation of AβPP Expression by RNA-Binding Proteins. Ageing Res. Rev. 11, 450–459. doi:10.1016/j.arr.2012.03.005
Westmark, C. J., Westmark, P. R., O'Riordan, K. J., Ray, B. C., Hervey, C. M., Salamat, M. S., et al. (2011). Reversal of Fragile X Phenotypes by Manipulation of AβPP/Aβ Levels in Fmr1KO Mice. PLoS One 6, e26549. doi:10.1371/journal.pone.0026549
Wiseman, F. K., Al-Janabi, T., Hardy, J., Karmiloff-Smith, A., Nizetic, D., Tybulewicz, V. L. J., et al. (2015). A Genetic Cause of Alzheimer Disease: Mechanistic Insights from Down Syndrome. Nat. Rev. Neurosci. 16, 564–574. doi:10.1038/nrn3983
Wolozin, B., and Ivanov, P. (2019). Stress Granules and Neurodegeneration. Nat. Rev. Neurosci. 20, 649–666. doi:10.1038/s41583-019-0222-5
Xiong, M., Jiang, H., Serrano, J. R., Gonzales, E. R., Wang, C., Gratuze, M., et al. (2021). APOE Immunotherapy Reduces Cerebral Amyloid Angiopathy and Amyloid Plaques while Improving Cerebrovascular Function. Sci. Transl. Med. 13, eabd7522. doi:10.1126/scitranslmed.abd7522
Yang, Y., Quitschke, W., Vostrov, A. A., and Brewer, G. J. (1999). CTCF Is Essential for Up-Regulating Expression from the Amyloid Precursor Protein Promoter during Differentiation of Primary Hippocampal Neurons. J. Neurochem. 73, 2286–2298. doi:10.1046/j.1471-4159.1999.0732286.x
Yeh, S. H., Yang, W. B., Gean, P. W., Hsu, C. Y., Tseng, J. T., Su, T. P., et al. (2011). Translational and Transcriptional Control of Sp1 against Ischaemia through a Hydrogen Peroxide-Activated Internal Ribosomal Entry Site Pathway. Nucleic Acids Res. 39, 5412–5423. doi:10.1093/nar/gkr161
Younas, N., Zafar, S., Shafiq, M., Noor, A., Siegert, A., Arora, A. S., et al. (2020). SFPQ and Tau: Critical Factors Contributing to Rapid Progression of Alzheimer's Disease. Acta Neuropathol. 140, 317–339. doi:10.1007/s00401-020-02178-y
Keywords: amyloid precursor protein, Alzheimer’s disease, dementia, transcription, post-transcription, RNA-binding protein, alternative splicing, microRNA
Citation: Sato K, Takayama K-i, Hashimoto M and Inoue S (2021) Transcriptional and Post-Transcriptional Regulations of Amyloid-β Precursor Protein (APP) mRNA. Front. Aging 2:721579. doi: 10.3389/fragi.2021.721579
Received: 07 June 2021; Accepted: 28 July 2021;
Published: 11 August 2021.
Edited by:
Andrea Cristina Paula-Lima, University of Chile, ChileReviewed by:
Adriano Sebollela, University of Sao Paulo, BrazilJaime Diaz-Zuniga, University of Chile, Chile
Copyright © 2021 Sato, Takayama, Hashimoto and Inoue. This is an open-access article distributed under the terms of the Creative Commons Attribution License (CC BY). The use, distribution or reproduction in other forums is permitted, provided the original author(s) and the copyright owner(s) are credited and that the original publication in this journal is cited, in accordance with accepted academic practice. No use, distribution or reproduction is permitted which does not comply with these terms.
*Correspondence: Satoshi Inoue, c2lub3VlQHRtaWcub3IuanA=