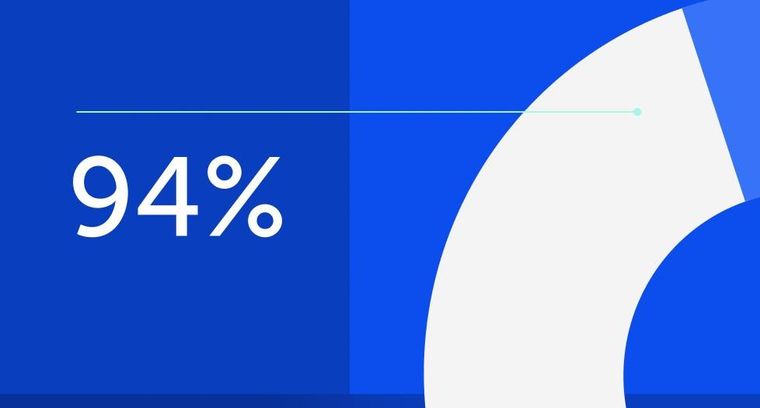
94% of researchers rate our articles as excellent or good
Learn more about the work of our research integrity team to safeguard the quality of each article we publish.
Find out more
PERSPECTIVE article
Front. Aging, 25 March 2021
Sec. Aging and Cancer
Volume 2 - 2021 | https://doi.org/10.3389/fragi.2021.655315
Reversible senescence at the cellular level emerged together with tissue specialization in Metazoans. However, this reversibility (ability to permanently rejuvenate) through recapitulation of early stages of development, was originally a part of ontogenesis, since the pressure of integrativeness was not dominant. The complication of specialization in phylogenesis narrowed this “freedom of maneuver”, gradually “truncating” remorphogenesis to local epimorphosis and further up to the complete disappearance of remorphogenesis from the ontogenesis repertoire. This evolutionary trend transformed cellular senescence into organismal aging and any recapitulation of autonomy into carcinogenesis. The crown of specialization, Homo sapiens, completed this post-unicellular stage of development, while in the genome all the potential for the next stage of development, which can be called the stage of balanced coexistence of autonomous and integrative dominants within a single whole. Here, completing the substantiation of the new section of developmental biology, we propose to call it Developmental Biogerontology.
“It is not birth, marriage, or death, but gastrulation which is truly the most important time in your life.” (Wolpert, 1991).
One of the central dogmas of unidirectional phylogenesis/ontogenesis consistently realized in evolution is a steady decrease of tissue-specific regenerative potential (epimorphosis), implementation of which requires recapitulation of early ontogenetic (embryonic) stages of development and expansion of autonomous cell potential. This is natural, because the entire second, post-unicellular stage of evolution was aimed at stabilization of multicellularity by limiting of autonomy, formation of specialized tissues and complication of integration processes. The “payment” for this achievement, which had undoubted evolutionary advantages–the conquest of new niches and improvement of all forms of life and mechanisms of adaptation, was the constant limitation of cellular autonomy in the interest of increasingly complex integrative dominants. As a result, there is a preserved but epigenetically blocked pathway for continuous and full quantitative and qualitative self-renewal of tissues, organs and functions. In other words, we have paid for reach the top of the current stage of development with inevitable involution, aging, aging associated diseases and mortality (Salnikov and Baramiya, 2020).
It is very important to understand that, in principle, there are no special genes and pathways for aging. These are the very mechanisms that ensure our functioning as a single integrated highly specialized whole. In other words, the way we exist makes us old. Yet this does not mean that we cannot change the described developmental pattern. However, any attempts to repeat the embryonic stages of development and autonomy in postnatal ontogenesis lead to carcinogenesis (disintegrating growth-DG). Consequently, the essence of the next, possible stage of Homo sapiens’ self-directed development lies in the systemic “consensus”, or dynamic balance of autonomous and integrative dominants within a single whole. As a result - the implementation of autonomous programs within the integrated whole, without disintegrating processes and with constant, complete and unlimited self-renewal of tissues, organs and functions.
The main sign of aging is insufficient quantitative and qualitative/functional recovery of cell and tissue damage/deficiency accumulated over time. Thus, aging and aging related pathologies are a direct consequence of the non-compensability of losses (NB! not consequence of the uncompensated losses) that always accompany any functioning. Currently, antagonistic pleiotropy principles are the most commonly accepted explanation for aging (Williams, 1957). Our viewpoint on the functional division of the multicellular genome does not contradict it, but also provides a new understanding of this theory. We believe that pleiotropic properties are possessed not by individual genes acquired in evolution, but by their large group, which creates multicellularity as a specialized biological system and is united in the integrative part (IntG) of the cellular genome. In other words, the phenomenon of genes pleiotropy can always be described by the transition from symbiotic relations at the beginning of development, to parasitic ones at the end. It is this transition that occurs in multicellular organisms when their organismic organization passes from the necessary symbiotic relationships to parasitic ones (Salnikov and Baramiya, 2020). The resources required for improved integrative/organismal functions are taken away from the core autonomous functions of cells, represented by the THG portion of the genome. It is necessary to separate the cellular and organismal zones of regulation. The processes occurring exclusively in the organismal regulation zone and in its interests based on the IntG part of the genome leave the cellular one to cope with the consequences of this process within the limits of the decreasing capabilities of the THG part. It is important to understand here that, ultimately, the sequential limitation of autonomy in ontogenesis with each cycle of this limitation leads to gradual underproduction/“truncation” of function/functionality, including those specialized functions, for implementation of which autonomy is limited. The advantage gained by the IntG part of the genome, plays a major role from the evolutionary point of view, where it is important how developed the main functional systems of the organism will be by the time it reaches fertility. An advantage gained at the critical moment and paid for by suppressing of autonomous and regenerative potential in the future - it is this the IntG pleiotropy. It is this steady increase of the integration “functional tax” accompanied by the simultaneous epigenetic limitation of autonomy (freedom of maneuver) both in phylogenesis and ontogenesis that is the essence and the root cause of aging inherent in the very nature of genome functioning of highly integrated multicellularity.
Since the main developmental implementation mechanism is based on the predominance of IntG, which leads to a decrease in THG functionality, aging is also an integral part of ontogenesis. The definition of functional age in postnatal ontogenesis as the ratio of the functional activity of IntG/GHG genes is also associated with this. For successful development, the IntG part of the genome must from both upregulation of its genes and epigenetic downregulation of THG. Most likely, the functional predominance of IntG during ontogenesis is mediated by repressive methylation of THG, as evidenced by some experimental data (Garagnani et al., 2012) showing the increase in epigenetic shutdown of genes that we attribute to HG, is best related to the chronological age.
We assert that ontogenetic aging proper starts with epigenetic blocking of true stemness - cell pluripotency (the onset of gastrulation) and then continues by blocking of limited stemness - multipotency (the end of gastrulation). However, this does not mean that this is where functional and, consequently, biological aging of the organism begins. Functional aging begins since exceeding the critical value of the “functional tax” on HG, which reduces the vital adaptive resource for the cells and regenerative capabilities of the organism as a whole.
Like aging, carcinogenesis is a payment for multicellularity. The cells that give rise to cancer are immortal. The HeLa line has been maintained for seven decades, without any signs of degradation. In fact, we observe them as a unicellular' culture. Thus, the conclusion is obvious - elimination of the integration burden caused by the unidirectional ontogenetic program operation makes it possible to avoid the “payment” for its implementation. However, malfunction of intercellular control and integrity for the sake of autonomy and non-aging when the stimulus for cell division is produced only by the cells itself, results in carcinogenesis with inability to return/redifferentiate, escape from external control, loss of tissue-specific functionality, blockage of division control. So what is the solution?
Evolutionary trends revealed by the analysis of comparative and developmental immunology data demonstrate an inverse correlation between the ability to regenerate damaged/lost body parts and the development of an advanced immune system (Thouveny and Tassava, 1998; Harty et al., 2003; Mescher and Neff, 2005; Godwin and Brockes, 2006). More primitively organized Metazoans that rely solely on innate immunity have a greater regenerative potential. Compared to lower vertebrates such as amphibians and teleost fishes, which are able to completely regenerate many parts of the body, mammals have limited regenerative potential. To explain this difference, it has been postulated that the loss of regenerative potential in mammals is associated with the maturation of their immune system compared to lower vertebrates Julier et al., 2017; Uygur and Lee, 2016; Godwin and Rosenthal, 2014; Eguchi et al., 2011; Aurora and Olson, 2014; Bertolotti et al., 2013; Vitulo et al., 2017a; Kishi et al., 2012; Wilgus, 2007; Porrello et al., 2011).
Summarizing many other data, we conclude that the following is necessary for a successful tissue-specific epimorphic regeneration:
1. Proliferation of dedifferentiated cells with the formation of blastema, i.e. recapitulation of early ontogenetic stages of development (Gordon and Brockes, 1988; Morasso et al., 1996; Mullen et al., 1996; Carlson et al., 1998; Leask and Abraham, 2004; Vinarsky et al., 2005; Joetham et al., 2007; Lévesque et al., 2007; Rae et al., 2007; Satoh et al., 2008; Jopling et al., 2010; Campbell et al., 2011; Kubin et al., 2011; Satoh et al., 2011; Seifert et al., 2012; Godwin et al., 2013; Petrie et al., 2014; Cahill et al., 2017; Simkin et al., 2017; Vitulo et al., 2017a; Alibardi, 2020).
2. Engaging the Wnt signaling pathway and pluripotency/cellular reprogramming factors, including the so-called oncogenes (Fausto et al., 1986; Fausto, 1991; Staal et al., 2008; Maki et al., 2009; Monaghan et al., 2009; Lin et al., 2010; Neff et al., 2011; Boulter et al., 2012; Knapp et al., 2013; Looso et al., 2013; Sousounis et al., 2013; Stewart et al., 2013; Takeo et al., 2013; Godwin and Rosenthal, 2014; Hutchins et al., 2014; Raspopovic et al., 2014; Rivlin et al., 2014; Hesse et al., 2015; Kumar et al., 2015; Casey et al., 2016; Casey et al., 2017; Sarig and Tzahor, 2017; Vitulo et al., 2017b; Franco et al., 2018; Bywater et al., 2020; Shoffner et al., 2020; Xu et al., 2020; Ye et al., 2020)
3. Immunological tolerance through down-regulation (up to complete disappearance) of HLA-A, B, and -C and up-regulation of HLA-G and transformation of conventional effectors of the immune response into regulatory cells that provides the morphogenetic function of the immune system through active tolerance and stimulation of growth processes in renewing tissues (Maisel et al., 1998; Varda-Bloom et al., 2000; Veeneman et al., 2001; Krishnadasan et al., 2002; Shigematsu et al., 2002; Kubo et al., 2004; Brunetti et al., 2005; Hawrylowicz and O’Garra, 2005; Hemler, 2005; Ito et al., 2005; Taams et al., 2005; Ghiringhelli et al., 2006; Mak and Saunders, 2006; Mescher and Neff, 2006; Yamazaki et al., 2006; Yang et al., 2006; Collison et al., 2007; Joetham et al., 2007; Tiemessen et al., 2007; Staal et al., 2008; Corthay, 2009; Linfert et al., 2009; Sheng et al., 2009; Zoller, 2009; Lin et al., 2010; Perdiguero et al., 2011; Wang et al., 2010; Liu et al., 2011; Perdiguero et al., 2011; Petersen et al., 2011; Boulter et al., 2012; Rinkevich and Rinkevich, 2012; Tang et al., 2012; Burzyn et al., 2013; McHedlidze et al., 2013; Reinke et al., 2013; Takeo et al., 2013; Aurora et al., 2014; Diefenbach et al., 2014; Lavine et al., 2014; Li et al., 2014; Sadej et al., 2014; Weirather et al., 2014; Zordan et al., 2014; Artis and Spits, 2015; Castiglioni et al., 2015; Saito et al., 2015; Seubert et al., 2015; Berditchevski and Odintsova, 2016; Kudira et al., 2016; Ali et al., 2017; Hui et al., 2017; Krueger et al., 2017; Li and Hua, 2017; Mescher et al., 2017; Schiaffino et al., 2017; Simkin et al., 2017; Vannella and Wynn, 2017; Li et al., 2018; Schaper and van Spriel, 2018; Zacchigna et al., 2018; Zeng et al., 2018; Abnave and Ghigo, 2019; Li et al., 2019; Kew et al., 2020; Li et al., 2020).
4. In highly specialized mammals, including humans, they exist in two cases: during embryogenesis and carcinogenesis (syn. somatic embryogenesis). However, the outcome in each of these cases is different.
5. How the obtained experimental data is interpreted depends largely on which “paradigmatic glasses” are used to view these data. Extensive review (Wong and Whited, 2020) summarizes important parallels between wound healing, epimorphic regeneration, and solid tumors. If one gets rid of the enemy-thinking view of carcinogenesis, then the conclusion will be unambiguous. In particular, the mechanisms that are carried out in postnatal ontogenesis as carcinogenesis initially debut as a variant of epimorphosis (syn. somatic embryogenesis). Cancer does not mislead the immune system at all, but the immune system itself reacts to the recapitulation of early ontogenetic programs in accordance with its morphogenetic function. However, this cooperation with the transformation of conventional effectors of the immune system into regulatory cells, leads to an active tolerance or regulatory facilitation reaction (Voisin, 1987) in highly specialized amniotes with a developed adaptive immune system. Only after that, the processes of tissue-specific regeneration are transformed into DG. On the contrary, dedifferentiation and an increase in cell autonomy with the formation of embryonic blastema in anamniotes with poorly developed/undeveloped adaptive immune system, which are at the stage of phylogenesis, at which cell autonomy is not only acceptable, but also a necessary component of their ontogenesis, this process leads to epimorphosis but not to carcinogenesis. The totipotent/pluripotent embryo is protected from the immune surveillance system by the same transformation mechanisms of conventional immune cells into regulatory cells at the mother-fetus interface and by a multinucleated nondividing syncytium (Trowsdale and Betz, 2006; Piao et al., 2015) It is the development in immunoprivileged conditions that makes it possible to form not a tumor, but a mature fetus. Only somatic cells that recapitulate early ontogenetic stages of development and at the same time are deprived of this immunoprivilege carry out this program in the form of carcinogenesis. The standard regenerative module during regeneration works within the program of relatively rigid-specific determination, and the level of tissue-specific differentiation is determined by the principle of submitting the interests of single units to the interests of the entire organism (IG-integrating growth). The active tolerance reaction ensures stability of this particular program. In the case of reontogenesis, that is, the recapitulation of morphogenetic modules of early ontogenesis (embryonic development program) with a specific tendency to autonomy, the same interactions ensure the stability of this particular inverse vector (unlimited expanding the potential of individual units - DG) that is detrimental to the entire organism. In other words, the immune response “bluntly” supports a growth program, which is launched when an absolutely critical or relative deficiency of tissue/functions occurs; regardless of whether the regenerative (within differentiation) or embryonic (with dedifferentiation and progressive autonomy) module works currently. Regulatory immune impacts in the absence of immunoprivilege blocks the possibility of returning to the state of differentiation, and it is from this moment that what began as epimorphic regeneration is transformed into DG. It is this makes somatic embryogenesis incomplete and turns the area of potential epimorphic regeneration into “wounds that do not heal” (Dvorak, 1986). Generation a full-fledged morphogenetic field in postnatal organs by re-creation of immunoprivileged status through complete immunological tolerance to the markers of the embryonic pathway (the so-called tumor-associated antigens) has oncostatic and differentiating properties and can prevent the transformation of reontogenesis into carcinogenesis. Many reports support this concept (Whisson, 1967; McKinnell et al., 1969; Mintz and Illmensee, 1975; Pierce and Wallace, 1971; Pierce et al., 1982; Pierce et al., 1987; Webb et al., 1984; Weaver et al., 1997; Coleman et al., 1993; Li et al., 2003; Lee et al., 2005; Gootwine et al., 1982; Park et al., 2017; Shvemberger, 1987; Nancy et al., 2012; Tong et al., 2018; Negishi et al., 2018; Zhao et al., 2017; Hyde and Schust, 2016; Farjadian et al., 2018; Amiot et al., 2011; Lin and Yan, 2018; Derynck and Weinberg, 2019; Curri and Bagehawe, 1967; Claser et al., 1965; Unkelles et al., 1974; Park et al., 2017).
It might be possible to eliminate the emerging cancer by killing it through the immune system. Meanwhile, this not eliminate but exacerbate the deficiency of tissues and functions, which will again lead to spontaneous reprogramming; in other words, it will trigger the mechanisms of epimorphic regeneration, which will turn into cancer again and again in the absence of immunoprivilege. The cancer eradication paradigm plays a major role in the palliative care of patients. While we are confident that this paradigm will never eliminate the cause of cancer, it will only reduce the cancer death probability. However, when one tries to eradicate cancer, rejuvenation will inevitably end, because spontaneous reprogramming which then turns into cancer, is an attempt by any living matter to renew itself. Full-scale reprogramming (spontaneous or induced) that never turns into cancer does not have a fully-fledged biological alternative for simultaneously solving two main problems: eliminating both cancer and aging. They cannot be solved separately, because the solution to these problems is the same. Briefly, it can be summarized as follows: in trying to eliminate aging, we will always “call for” cancer. Trying to kill cancer, we will always be destined to aging.
The essence of geroprotection is to dampen the involution as much as possible-to delay the onset and to slow down progression of involution and age-related pathologies. Geroprotection has several main features that fundamentally distinguish it from anti-aging:
1. Geroprotection does not change the unidirectionality (formation → growth → involution → death) of ontogenesis/developmental vector;
2. It does not affect the root cause of aging, but individual signs and mechanisms associated with aging;
3. Geroprotective effects are relatively compensatory and transient.
In short, everything that does not correct the developmental vector is called geroprotection or elimination of consequences (such as the use of stem cells, correctly called progenitor cells, since they are not carriers real stemness - toti/pluripotency; calorie restriction, fasting, mimicking fasting, heterochronic blood and therapeutic plasma exchange, young plasma, secretome-based intervention, stem cell niche updating, NAD+, Resveratrol, Rapamycin (rapalogs), Metformin, Senolytics Oxytocin, Alk5i, Curcumin, ISRIB, so-called “epigenetic drugs”).
The essence of anti-aging is to eliminate the root cause of aging and, as a result age-related diseases. This requires a change in the unidirectionality of ontogenesis, in other words, an adjustment of the development vector.
We cannot always remain non-aging at the cellular level, since such agelessness is a function of autonomy, which being constant and unlimited come into conflict with specific functions of specialized structures, which is a function of integrativity, and invariably leads to DG. Consequently, the essence of the next (third) post-multicellular stage of metazoans development lies in the systemic developmental consensus/”reconciliation” of autonomous and integration dominants.
As a result - implementation of autonomous programs within the framework of an integrated whole without “sliding down” into DG on the one hand, and into irreversible involution on the other, which is equally inevitably fraught with loss of functionality. This will lead to complete and unlimited self-renewal of tissues/organs and functions, in other words, in non-aging at the organismal level. It is important to understand that aging, as a part of the developmental program cannot be avoided by influencing the individual mechanisms through which it is implemented. Only a program can bypass the program by modifying the unidirectional vector of development. Since we cannot “reverse” ontogenesis, this process is possible only within the framework of modification of the unidirectional developmental vector through its looping and corresponding changes in the principles of genome functioning. In our opinion, the only fundamental way of implementation of this complex developmental consensus is balanced coexistence of autonomous and integrative dominants, is what we figuratively call “to remember and accept ourselves” - to remember the prenatal self in the postnatal and through restoration of the immunological memory to accepted yourself as your own. There is the only way to achieve this to protect somatic embryogenesis from any types of the immune response, including active (regulatory) tolerance. In other words, the solution is in absolute immunological tolerance to antigens associated with early stages of embryonic development, for which the adult organism does not have memory. This is the essence of the proposed solution, to which we have lead in our previous publications (Baramiya, 2000; Baramiya et al., 2020).
Aging is a process and a consequence of the processes of a steadily increasing limitation of the ability of full-fledged tissue-specific self-renewal at all levels of organization and non-compensability of losses of cells/tissues/functions (always accompanying any functioning), due to the sequential realization in the development of the central dogma of unidirectional phylogenesis/ontogenesis of metazoan - a steadfast epigenetic restriction of cellular autonomy in the interests of increasingly complex integration dominants. This turns their functional (specialized) part from a cellular symbiont into a cellular parasite, limits lifespan and leads to death due to involutive extinction of functions, failure of regulatory homeostatic mechanisms, emergence of endogenous disorders and an increased susceptibility to exogenous factors.
Involution and loss of functionality are only the consequences of aging. Aging per se is an inability to completely restore functionality, and it is inherent in the very nature of the organization (genome functioning) of highly specialized multicellularity. Until this understanding is realized and accepted, we will eliminate the consequences but not the root cause.
Living matter has an existential “urge” to conquer habitat niches by improving mechanisms and forms of adaptation, including through self-renewal. This is the only essence and “goal” of evolution. A full-fledged tissue-specific renewal is possible only through recapitulation of the early stages of ontogenesis, that is, through endogenous systemic reprogramming. Systemic natural reprogramming with zeroing of epigenetic and metabolic load and age in ontogenesis occurs twice: the first time in the prenatal period as a result of fertilization and leads to fetal formation, the second time in postnatal ontogenesis and leads to carcinogenesis. We should finally understand a clear pattern: any genes and signal pathways that inhibits senescence takes part in the potentiation of carcinogenesis; any genes, signal pathways that suppresses carcinogenesis stimulates senescence. Therefore, neither aging nor carcinogenesis can be eliminated without “reconciliation” of these processes. There are no bad genes (signaling pathways), and what we consider their insufficient or excessive functioning, or an error in turning them on and off, is in fact often quite natural developmental variants that can lead to undesirable consequences for us. To overcome them, we must simply use the other options available and not blindly suppress certain undesirable effects for us. Once again - the program of unidirectional ontogenesis can be overridden only by an alternative program, but not by purposeful suppression or stimulation of certain developmental variants, which in the absence of a three-dimensional picture of interactions and interdependencies of all signal networks is a random search with a local, but not systemic desired result and sometimes with very negative consequences. Whereas the “reconciliation” of autonomy with integrativity through the establishment of their dynamic balance will allow to get away from the functional pleiotropy of the IntG while maintaining the integrity of the symbiont with the simultaneous preservation of the functioning autonomy within the integrated whole.
There is reason to believe that Homo sapiens have not yet reached the pinnacle of evolution. Therefore, completing here our series of publications (Baramiya, 1988; Baramiya, 2000; Baramiya, 2018; Baramiya and Baranov, 2020; Baramiya et al., 2020; Salnikov and Baramiya, 2020) on the theoretical substantiation of a new section of developmental biology, we propose to call it Developmental Biogerontology.
Both authors contributed equally to the writing and preparation of the article for publication. All the authors reviewed, revised and approved the final version of the manuscript.
The authors have no relevant affiliations or financial involvement with any organization or entity with a financial interest in or financial conflict with the subject matter or materials discussed in the manuscript. This includes employment, consultancies, honoraria, stock ownership or options, expert testimony, grants or patents received or pending, or royalties. No writing assistance was utilized in the production of this manuscript.
‘This article is dedicated to my coauthor and friend, Dr. M.G. Baramia, who passed away suddenly and untimely. I am certain that his ideas and the direction in gerontology to which he dedicated his scientific life will be continued in the research of his colleagues.’ - Dr. Lev Salnikov.
LS was employed by the company SibEnzyme US LLC. MGB was employed by AntiCancer Inc.
The remaining authors declare that the research was conducted in the absence of any commercial or financial relationships that could be construed as a potential conflict of interest.
HG, housekeeping genes; AHG, autonomizing housekeeping genes; GHG, growth housekeeping genes; THG, total housekeeping genome(AHG + GHG); IntG, integrative genes; IG, integrating growth; DG, disintegrating growth.
HG housekeeping genes
AHG autonomizing housekeeping genes
GHG growth housekeeping genes
THG total housekeeping genome(AHG + +GHG)
IntG integrative genes
IG integrating growth
DG disintegrating growth
Integrating Growth a submission of potency of single cells composing an organism to the development program and functions of the whole organism
Disintegrating Growth a priority of extension potency of single cells over the development program and functions of the whole organism.
Abnave, P., and Ghigo, E. (2019). Role of the immune system in regeneration and its dynamic interplay with adult stem cells. Semin. Cell Develop. Biol. 87, 160–168. doi:10.1016/j.semcdb.2018.04.002
Ali, N., Zirak, B., Rodriguez, R. S., Pauli, M. L., Truong, H. A., Lai, K., et al. (2017). Regulatory T cells in skin facilitate epithelial stem cell differentiation. Cell 169 (6), 1119–1129. doi:10.1016/j.cell.2017.05.002
Alibardi, L. (2020). Appendage regeneration in anamniotes utilizes genes active during larval-metamorphic stages that have been lost or altered in amniotes: the case for studying lizard tail regeneration. J. Morphol. 281 (11), 1358–1381. doi:10.1002/jmor.21251
Amiot, L., Ferrone, S., Grosse-Wilde, H., and Seliger, B. (2011). Biology of HLA-G in cancer: a candidate molecule for therapeutic intervention? Cell. Mol. Life Sci. 68 (3), 417–431. doi:10.1007/s00018-010-0583-4
Artis, D., and Spits, H. (2015). The biology of innate lymphoid cells. Nature 517 (7534), 293–301. doi:10.1038/nature14189
Aurora, A. B., and Olson, E. N. (2014). Immune modulation of stem cells and regeneration. Cell Stem Cell 15, 14–25. doi:10.1016/j.stem.2014.06.009
Aurora, A. B., Porrello, E. R., Tan, W., Mahmoud, A. I., Hill, J. A., Bassel-Duby, R., et al. (2014). Macrophages are required for neonatal heart regeneration. J. Clin. Invest. 124 (3), 1382–1392. doi:10.1172/JCI72181
Baramiya, M. G. (2000). Aging and carcinogenesis - insufficient metabolic cell repair as the common link. Gerontology 46 (6), 328–332. doi:10.1159/000022186
Baramiya, M. G., and Baranov, E. (2020). From cancer to rejuvenation: incomplete regeneration as the missing link (Part I: the same origin, different outcomes). Future Sci. OA 6 (3), FSO450. doi:10.2144/fsoa-2019-0119
Baramiya, M. G., Baranov, E., Saburina, I., and Salnikov, L. (2020). From cancer to rejuvenation: incomplete regeneration as the missing link (part II: rejuvenation circle). Future Sci. OA 6 (8), FSO610. doi:10.2144/fsoa-2020-008510.2144/fsoa-2019-0119
Baramiya, M. G. (2018). Cancer and anti-aging: same origin-different outcomes (paradigm shift) part.1. Reports Gerontol 65. Section Soc. Naturalists Moscow State Univ., 100–118. [in Russian, with English summary].
Baramiya, M. G. (1988). Carcinogenesis, senescence and life duration: potential of transformed cells and restrain of senescence (hypothesis). Adv. Curr. Biol. 118 (4), 421–440. [in Russian, with English summary].
Berditchevski, F., and Odintsova, E. (2016). ErbB receptors and tetraspanins: casting the net wider. Int. J. Biochem. Cell Biol. 77 (Pt A), 68–71. doi:10.1016/j.biocel.2016.05.017
Bertolotti, E., Malagoli, D., and Franchini, A. (2013). Skin wound healing in different agedXenopus laevis. J. Morphol. 274 (8), 956–964. doi:10.1002/jmor.20155
Bijlsma, M., Chou, W. C., Sun, Q., Lee, W., Rabbani, P., Loomis, C., et al. (2013). Wnt activation in nail epithelium couples nail growth to digit regeneration. Nature 499, 228–232. doi:10.1038/nature12214
Boulter, L., Govaere, O., Bird, T. G., Radulescu, S., Ramachandran, P., Pellicoro, A., et al. (2012). Macrophage-derived Wnt opposes notch signaling to specify hepatic progenitor cell fate in chronic liver disease. Nat. Med. 18 (4), 572–579. doi:10.1038/nm.2667
Brunetti, G., Colucci, S., Pignataro, P., Coricciati, M., Mori, G., Cirulli, N., et al. (2005). T cells support osteoclastogenesis in an in vitro model derived from human periodontitis patients. J. Periodontol. 76 (10), 1675–1680. doi:10.1902/jop.2005.76.10.1675
Burzyn, D., Kuswanto, W., Kolodin, D., Shadrach, J. L., Cerletti, M., Jang, Y., et al. (2013). A special population of regulatory T cells potentiates muscle repair. Cell 155 (6), 1282–1295. doi:10.1016/j.cell.2013.10.054
Bywater, M. J., Burkhart, D. L., Straube, J., Sabò, A., Pendino, V., Hudson, J. E., et al. (2020). Reactivation of Myc transcription in the mouse heart unlocks its proliferative capacity. Nat. Commun. 11 (1), 1827. doi:10.1038/s41467-020-15552-x
Cahill, T. J., Choudhury, R. P., and Riley, P. R. (2017). Heart regeneration and repair after myocardial infarction: translational opportunities for novel therapeutics. Nat. Rev. Drug Discov. 16 (10), 699–717. doi:10.1038/nrd.2017.106
Campbell, L. J., Suárez-Castillo, E. C., Ortiz-Zuazaga, H., Knapp, D., Tanaka, E. M., and Crews, C. M. (2011). Gene expression profile of the regeneration epithelium during axolotl limb regeneration. Dev. Dyn. 240 (7), 1826–1840. doi:10.1002/dvdy.22669
Carlson, M. R. J., Bryant, S. V., and Gardiner, D. M. (1998). Expression of Msx-2 during development, regeneration, and wound healing in axolotl limbs. J. Exp. Zool. 282 (6), 715–723. doi:10.1002/(sici)1097-010x(19981215)282:6<715::aid-jez7>3.0.co;2-f
Casey, S. C., Baylot, V., and Felsher, D. W. (2017). MYC: master regulator of immune privilege. Trends Immunol. 38 (4), 298–305. doi:10.1016/j.it.2017.01.002
Casey, S. C., Tong, L., Li, Y., Do, R., Walz, S., Fitzgerald, K. N., et al. (2016). MYC regulates the antitumor immune response through CD47 and PD-L1. Science 352 (6282), 227–231. doi:10.1126/science.aac9935
Castiglioni, A., Corna, G., Rigamonti, E., Basso, V., Vezzoli, M., Monno, A., et al. (2015). FOXP3+ T cells recruited to sites of sterile skeletal muscle injury regulate the fate of satellite cells and guide effective tissue regeneration. PLoS One 10, e0128094. doi:10.1371/journal.pone.0128094
Claser, E. M., Spink, P., and O'Meara, R. A. (1965). A screening test for substances inhibiting the cancer coagulative factor. Nature 208 (5014), 1008–1009. doi:10.1038/2081008a0
Coleman, W. B., Wennerberg, A. E., Smith, G. J., and Grisham, J. W. (1993). Regulation of the differentiation of diploid and some aneuploid rat liver epithelial (stemlike) cells by the hepatic microenvironment. Am. J. Pathol. 142 (5), 1373–1382.
Collison, L. W., Workman, C. J., Kuo, T. T., Boyd, K., Wang, Y., Vignali, K. M., et al. (2007). The inhibitory cytokine IL-35 contributes to regulatory T-cell function. Nature 450 (7169), 566–569. doi:10.1038/nature06306
Corthay, A. (2009). How do regulatory T cells work? Scand. J. Immunol. 70 (4), 326–336. doi:10.1111/j.1365-3083.2009.02308.x
Curri, G. A., and Bagehawe, K. D. (1967). The masking of antigene on trophoblast and cancer cells. Lancet 1 (7492), 708–710. doi:10.1016/s0140-6736(67)92183-6
Derynck, R., and Weinberg, R. A. (2019). EMT and cancer: more than meets the eye. Develop. Cell 49 (3), 313–316. doi:10.1016/j.devcel.2019.04.026
Diefenbach, A., Colonna, M., and Koyasu, S. (2014). Development, differentiation, and diversity of innate lymphoid cells. Immunity 41 (3), 354–365. doi:10.1016/j.immuni.2014.09.005
Dvorak, H. F. (1986). Tumors: wounds that do not heal. Similarities between tumor stroma generation and wound healing. N. Engl. J. Med. 315 (26), 1650–1659. doi:10.1056/NEJM198612253152606
Eguchi, G., Eguchi, Y., Nakamura, K., Yadav, M. C., Millán, J. L., and Tsonis, P. A. (2011). Regenerative capacity in newts is not altered by repeated regeneration and ageing. Nat. Commun. 2, 384. doi:10.1038/ncomms1389
Farjadian, S., Tabebordbar, M., Mokhtari, M., Safaei, A., Malekzadeh, M., and Ghaderi, A. (2018). HLA-G expression in tumor tissues and soluble HLA-G plasma levels in patients with gastrointestinal cancer. Asian Pac.J.Cancer Prev. 19 (10), 2731–2735. doi:10.22034/APJCP.2018.19.10.2731
Fausto, N., Mead, J. E., Braun, L., Thompson, N. L., Panzica, M., Goyette, M., et al. (1986). Proto-oncogene expression and growth factors during liver regeneration. Symp. Fundam. Cancer Res. 39, 69–86.
Fausto, N. (1991). Protooncogenes and growth factors associated with normal and abnormal liver growth. Dig. Dis Sci 36 (5), 653–658. doi:10.1007/BF01297034
Franco, L. C., Morales, F., Boffo, S., and Giordano, A. (2018). CDK9: a key player in cancer and other diseases. J. Cell. Biochem. 119 (2), 1273–1284. doi:10.1002/jcb.26293
Garagnani, P., Bacalini, M. G., Pirazzini, C., Gori, D., Giuliani, C., Mari, D., et al. (2012). Methylation ofELOVL2gene as a new epigenetic marker of age. Aging Cell 11 (6), 1132–1134. doi:10.1111/acel.12005
Ghiringhelli, F., Ménard, C., Martin, F., and Zitvogel, L. (2006). The role of regulatory T cells in the control of natural killer cells: relevance during tumor progression. Immunol. Rev. 214, 229–238. doi:10.1111/j.1600-065X.2006.00445.x
Godwin, J. W., and Brockes, J. P. (2006). Regeneration, tissue injury and the immune response. J. Anat. 209 (4), 423–432. doi:10.1111/j.1469-7580.2006.00626.x
Godwin, J. W., Pinto, A. R., and Rosenthal, N. A. (2013). Macrophages are required for adult salamander limb regeneration. Proc. Natl. Acad. Sci. USA 110, 9415–9420. doi:10.1073/pnas.1300290110
Godwin, J. W., and Rosenthal, N. (2014). Scar-free wound healing and regeneration in amphibians: immunological influences on regenerative success. Differentiation 87 (1–2), 66–75. doi:10.1016/j.diff.2014.02.002
Gootwine, E., Webb, C. G., and Sachs, L. (1982). Participation of myeloid leukaemic cells injected into embryos in haematopoietic differentiation in adult mice. Nature 299 (5878), 63–65. doi:10.1038/299063a0
Gordon, H., Brockes, J. P., and Wilson, F. (1988). Appearance and regulation of an antigen associated with limb regeneration inNotophthalmus viridescens. J. Exp. Zool. 247 (3), 232–243. doi:10.1002/jez.1402470306
Harty, M., Neff, A. W., King, M. W., and Mescher, A. L. (2003). Regeneration or scarring: an immunologic perspective. Dev. Dyn. 226 (2), 268–279. doi:10.1002/dvdy.10239
Hawrylowicz, C. M., and O'Garra, A. (2005). Potential role of interleukin-10-secreting regulatory T cells in allergy and asthma. Nat. Rev. Immunol. 5 (4), 271–283. doi:10.1038/nri1589
Hemler, M. E. (2005). Tetraspanin functions and associated microdomains. Nat. Rev. Mol. Cell Biol. 6 (10), 801–811. doi:10.1038/nrm1736
Hesse, R. G., Kouklis, G. K., Ahituv, N., and Pomerantz, J. H. (2015). The human ARF tumor suppressor senses blastema activity and suppresses epimorphic tissue regeneration. ELife 4, e07702. doi:10.7554/eLife.07702
Hui, S. P., Sheng, D. Z., Sugimoto, K., Gonzalez-Rajal, A., Nakagawa, S., Hesselson, D., et al. (2017). Zebrafish regulatory T cells mediate organ-specific regenerative programs. Develop. Cell 43 (6), 659–672. doi:10.1016/j.devcel.2017.11.010
Hutchins, E. D., Markov, G. J., Eckalbar, W. L., George, R. M., King, J. M., Tokuyama, M. A., et al. (2014). Transcriptomic analysis of tail regeneration in the lizard Anolis carolinensis reveals activation of conserved vertebrate developmental and repair mechanisms. PLoS One 9, e105004. doi:10.1371/journal.pone.0105004
Hyde, K. J., and Schust, D. J. (2016). Immunologic challenges of human reproduction: an evolving story. Fertil. Sterility 106 (3), 499–510. doi:10.1016/j.fertnstert.2016.07.1073
Ito, T., Ito, N., Saathoff, M., Stampachiacchiere, B., Bettermann, A., Bulfone-Paus, S., et al. (2005). Immunology of the human nail apparatus: the nail matrix is a site of relative immune privilege. J. Invest. Dermatol. 125 (6), 1139–1148. doi:10.1111/j.0022-202X.2005.23927.x
Jopling, C., Sleep, E., Raya, M., Martí, M., Raya, A., and Belmonte, J. C. I. (2010). Zebrafish heart regeneration occurs by cardiomyocyte dedifferentiation and proliferation. Nature 464 (7288), 606–609. doi:10.1038/nature08899
Julier, Z., Park, A. J., Briquez, P. S., and Martino, M. M. (2017). Promoting tissue regeneration by modulating the immune system. Acta Biomater. 53, 13–28. doi:10.1016/j.actbio.2017.01.056
Kew, C., Huang, W., Fischer, J., Ganesan, R., Robinson, N., Antebi, A., et al. (2020). Evolutionarily conserved regulation of immunity by the splicing factor RNP-6/PUF60. Elife 9, e57591. doi:10.7554/eLife.57591
Kishi, K., Okabe, K., Shimizu, R., and Kubota, Y. (2012). Fetal skin possesses the ability to regenerate completely: complete regeneration of skin. Keio J. Med. 61 (4), 101–108. doi:10.2302/kjm.2011-0002-ir
Knapp, D., Schulz, H., Rascon, C. A., Volkmer, M., Scholz, J., Nacu, E., et al. (2013). Comparative transcriptional profiling of the axolotl limb identifies a tripartite regeneration-specific gene program. PLoS One 8 (5), e61352. doi:10.1371/journal.pone.0061352
Krishnadasan, B., Naidu, B., Rosengart, M., Farr, A. L., Barnes, A., Verrier, E. D., et al. (2002). Decreased lung ischemia-reperfusion injury in rats after preoperative administration of cyclosporine and tacrolimus. J. Thorac. Cardiovasc. Surg. 123 (4), 756–767. doi:10.1067/mtc.2002.120351
Krueger, P. D., Narayanan, S., Surette, F. A., Brown, M. G., Sung, S.-S. J., and Hahn, Y. S. (2017). Murine liver-resident group 1 innate lymphoid cells regulate optimal priming of anti-viral CD8 + T cells. J. Leukoc. Biol. 101 (1), 329–338. doi:10.1189/jlb.3A0516-225R
Kubin, T., Pöling, J., Kostin, S., Gajawada, P., Hein, S., Rees, W., et al. (2011). Oncostatin M is a major mediator of cardiomyocyte dedifferentiation and remodeling. Cell Stem Cell 9 (5), 420–432. doi:10.1016/j.stem.2011.08.013
Kubo, T., Hatton, R. D., Oliver, J., Liu, X., Elson, C. O., and Weaver, C. T. (2004). Regulatory T cell suppression and anergy are differentially regulated by proinflammatory cytokines produced by TLR-activated dendritic cells. J. Immunol. 173 (12), 7249–7258. doi:10.4049/jimmunol.173.12.7249
Kudira, R., Malinka, T., Kohler, A., Dosch, M., de Agüero, M. G., Melin, N., et al. (2016). P2X1-regulated IL-22 secretion by innate lymphoid cells is required for efficient liver regeneration. Hepatology 63 (6), 2004–2017. doi:10.1002/hep.28492
Kumar, A., Gates, P. B., Czarkwiani, A., and Brockes, J. P. (2015). An orphan gene is necessary for preaxial digit formation during salamander limb development. Nat. Commun. 6, 8684. doi:10.1038/ncomms9684
Lavine, K. J., Epelman, S., Uchida, K., Weber, K. J., Nichols, C. G., Schilling, J. D., et al. (2014). Distinct macrophage lineages contribute to disparate patterns of cardiac recovery and remodeling in the neonatal and adult heart. Proc. Natl. Acad. Sci. USA 111 (45), 16029–16034. doi:10.1073/pnas.1406508111
Leask, A., and Abraham, D. J. (2004). TGF‐β signaling and the fibrotic response. FASEB j. 18 (7), 816–827. doi:10.1096/fj.03-1273rev
Lee, L. M. J., Seftor, E. A., Bonde, G., Cornell, R. A., and Hendrix, M. J. C. (2005). The fate of human malignant melanoma cells transplanted into zebrafish embryos: assessment of migration and cell division in the absence of tumor formation. Dev. Dyn. 233 (4), 1560–1570. doi:10.1002/dvdy.20471
Lévesque, M., Gatien, S., Finnson, K., Desmeules, S., Villiard, É., Pilote, M., et al. (2007). Transforming growth factor: β signaling is essential for limb regeneration in axolotls. PLoS One 2 (11), e1227. doi:10.1371/journal.pone.0001227
Li, J., Liang, C., Yang, K. Y., Huang, X., Han, M. Y., Li, X., et al. (2020). Specific ablation of CD4+ T-cells promotes heart regeneration in juvenile mice. Theranostics 10 (18), 8018–8035. doi:10.7150/thno.42943
Li, J., Razumilava, N., Gores, G. J., Walters, S., Mizuochi, T., Mourya, R., et al. (2014). Biliary repair and carcinogenesis are mediated by IL-33-dependent cholangiocyte proliferation. J. Clin. Invest. 124 (7), 3241–3251. doi:10.1172/JCI73742
Li, J., Tan, J., Martino, M. M., and Lui, K. O. (2018). Regulatory T-cells: potential regulator of tissue repair and regeneration. Front. Immunol. 9, 585. doi:10.3389/fimmu.2018.00585
Li, J., Yang, K. Y., Tam, R. C. Y., Chan, V. W., Lan, H. Y., Hori, S., et al. (2019). Regulatory T-cells regulate neonatal heart regeneration by potentiating cardiomyocyte proliferation in a paracrine manner. Theranostics 9 (15), 4324–4341. doi:10.7150/thno.32734
Li, L., Connelly, M. C., Wetmore, C., Curran, T., and Morgan, J. I. (2003). Mouse embryos cloned from brain tumors. Cancer Res. 63 (11), 2733–2736.
Li, N., and Hua, J. (2017). Immune cells in liver regeneration. Oncotarget 8 (2), 3628–3639. doi:10.18632/oncotarget.12275
Lin, A., and Yan, W.-H. (2018). Heterogeneity of HLA-G expression in cancers: facing the challenges. Front. Immunol. 9, 2164. doi:10.3389/fimmu.2018.02164
Lin, S.-L., Li, B., Rao, S., Yeo, E.-J., Hudson, T. E., Nowlin, B. T., et al. (2010). Macrophage Wnt7b is critical for kidney repair and regeneration. Proc. Natl. Acad. Sci. 107 (9), 4194–4199. doi:10.1073/pnas.0912228107
Linfert, D., Chowdhry, T., and Rabb, H. (2009). Lymphocytes and ischemia-reperfusion injury. Transplant. Rev. 23 (1), 1–10. doi:10.1016/j.trre.2008.08.003
Liu, Y., Wang, L., Kikuiri, T., Akiyama, K., Chen, C., Xu, X., et al. (2011). Mesenchymal stem cell-based tissue regeneration is governed by recipient T lymphocytes via IFN-γ and TNF-α. Nat. Med. 17 (12), 1594–1601. doi:10.1038/nm.2542
Looso, M., Preussner, J., Sousounis, K., Bruckskotten, M., Michel, C. S., Lignelli, E., et al. (2013). A de novo assembly of the newt transcriptome combined with proteomic validation identifies new protein families expressed during tissue regeneration. Genome Biol. 14 (2), R16. doi:10.1186/gb-2013-14-2-r16
Maisel, A., Cesario, D., Baird, S., Rehman, J., Haghighi, P., and Carter, S. (1998). Experimental autoimmune myocarditis produced by adoptive transfer of splenocytes after myocardial infarction. Circ. Res. 82 (4), 458–463. doi:10.1161/01.res.82.4.458
Mak, T., and Saunders, M. (2006). The immune response - basic and clinical principles. Amsterdam, Netherlands: Elsevier.
Maki, N., Suetsugu-Maki, R., Tarui, H., Agata, K., Del Rio-Tsonis, K., and Tsonis, P. A. (2009). Expression of stem cell pluripotency factors during regeneration in newts. Dev. Dyn. 238 (6), 1613–1616. doi:10.1002/dvdy.21959
McHedlidze, T., Waldner, M., Zopf, S., Walker, J., Rankin, A. L., Schuchmann, M., et al. (2013). Interleukin-33-dependent innate lymphoid cells mediate hepatic fibrosis. Immunity 39 (2), 357–371. doi:10.1016/j.immuni.2013.07.018
McKinnell, R. G., Deggins, B. A., and Labat, D. D. (1969). Transplantation of pluripotential nuclei from triploid frog tumors. Science 165 (3891), 394–396. doi:10.1126/science.165.3891.394
Mescher, A. L., Neff, A. W., and King, M. W. (2017). Inflammation and immunity in organ regeneration. Develop. Comp. Immunol. 66, 98–110. doi:10.1016/j.dci.2016.02.015
Mescher, A. L., and Neff, A. W. (2006). Limb regeneration in amphibians: immunological considerations. TSW Develop. Embryol. 1 (Suppl. 1), 1–11. doi:10.1100/tsw.2006.32310.1100/tswde.2006.53
Mescher, A. L., and Neff, A. W. (2005). Regenerative capacity and the developing immune system. Adv. Biochem. Eng. Biotechnol. 93, 39–66. doi:10.1007/b99966
Mintz, B., and Illmensee, K. (1975). Normal genetically mosaic mice produced from malignant teratocarcinoma cells. Proc. Natl. Acad. Sci. 72 (9), 3585–3589. doi:10.1073/pnas.72.9.3585
Monaghan, J. R., Epp, L. G., Putta, S., Page, R. B., Walker, J. A., Beachy, C. K., et al. (2009). Microarray and cDNA sequence analysis of transcription during nerve-dependent limb regeneration. BMC Biol. 7, 1. doi:10.1186/1741-7007-7-1
Morasso, M. I., Markova, N. G., and Sargent, T. D. (1996). Regulation of epidermal differentiation by a Distal-less homeodomain gene. J. Cell Biol 135 (6Pt2), 1879–1887. doi:10.1083/jcb.135.6.1879
Mullen, L. M., Bryant, S. V., Torok, M. A., Blumberg, B., and Gardiner, D. M. (1996). Nerve dependency of regeneration: the role of Distal-less and FGF signaling in amphibian limb regeneration. Development 122 (11), 3487–3497.
Nancy, P., Tagliani, E., Tay, C.-S., Asp, P., Levy, D. E., and Erlebacher, A. (2012). Chemokine gene silencing in decidual stromal cells limits T cell access to the maternal-fetal interface. Science 336 (6086), 1317–1321. doi:10.1126/science.1220030
Neff, A. W., King, M. W., and Mescher, A. L. (2011). Dedifferentiation and the role of sall4 in reprogramming and patterning during amphibian limb regeneration. Dev. Dyn. 240 (5), 979–989. doi:10.1002/dvdy.22554
Negishi, Y., Takahashi, H., Kuwabara, Y., and Takeshita, T. (2018). Innate immune cells in reproduction. J. Obstet. Gynaecol. Res. 44 (11), 2025–2036. doi:10.1111/jog.13759
Park, N. I., Guilhamon, P., Desai, K., McAdam, R. F., Langille, E., O’Connor, M., et al. (2017). ASCL1 reorganizes chromatin to direct neuronal fate and suppress tumorigenicity of glioblastoma stem cells. Cell Stem Cell 21 (2), 209–224. doi:10.1016/j.stem.2017.06.004
Perdiguero, E., Sousa-Victor, P., Ruiz-Bonilla, V., Jardí, M., Caelles, C., Serrano, A. L., et al. (2011). p38/MKP-1-regulated AKT coordinates macrophage transitions and resolution of inflammation during tissue repair. J. Cell Biol. 195 (2), 307–322. doi:10.1083/jcb.201104053
Petersen, S. H., Odintsova, E., Haigh, T. A., Rickinson, A. B., Taylor, G. S., and Berditchevski, F. (2011). The role of tetraspanin CD63 in antigen presentation via MHC class II. Eur. J. Immunol. 41 (9), 2556–2561. doi:10.1002/eji.201141438
Petrie, T. A., Strand, N. S., Tsung-Yang, C., Rabinowitz, J. S., and Moon, R. T. (2014). Macrophages modulate adult zebrafish tail fin regeneration. Development 141 (13), 2581–2591. doi:10.1242/dev.098459
Piao, H. L., Wang, S. C., Tao, Y., Fu, Q., Du, M. R., and Li, D. J. (2015). CXCL12/CXCR4 signal involved in the regulation of trophoblasts on peripheral NK cells leading to Th2 bias at the maternal-fetal interface. Eur. Rev. Med. Pharmacol. Sci. 19 (12), 2153–2161.
Pierce, G. B., Pantazis, C. G., Caldwell, J. E., and Wells, R. S. (1982). Specificity of the control of tumor formation by the blastocyst. Cancer Res. 42 (3), 1082–1087.
Pierce, G. B., and Wallace, C. (1971). Differentiation of malignant to benign cells. Cancer Res. 31 (2), 127–134.
Pierce, G. B., Arechaga, J., Jones, A., Lewellyn, A., and Wells, R. S. (1987). The fate of embryonal-carcinoma cells in mouse blastocysts. Differentiation 33 (3), 247–253. doi:10.1111/j.1432-0436.1987.tb01564.x
Porrello, E. R., Mahmoud, A. I., Simpson, E., Hill, J. A., Richardson, J. A., Olson, E. N. E. N., et al. (2011). Transient regenerative potential of the neonatal mouse heart. Science 331 (6020), 1078–1080. doi:10.1126/science.1200708
Rae, F., Woods, K., Sasmono, T., Campanale, N., Taylor, D., Ovchinnikov, D. A., et al. (2007). Characterisation and trophic functions of murine embryonic macrophages based upon the use of a Csf1r-EGFP transgene reporter. Develop. Biol. 308 (1), 232–246. doi:10.1016/j.ydbio.2007.05.027
Raspopovic, J., Marcon, L., Russo, L., and Sharpe, J. (2014). Digit patterning is controlled by a Bmp-Sox9-Wnt Turing network modulated by morphogen gradients. Science 345 (6196), 566–570. doi:10.1126/science.1252960
Reinke, S., Geissler, S., Taylor, W. R., Schmidt-Bleek, K., Juelke, K., Schwachmeyer, V., et al. (2013). Terminally differentiated CD8(+) T cells negatively affect bone regeneration in humans. Sci. Transl Med. 5 (177). doi:10.1126/scitranslmed.300475410.1126/scitranslmed.3004754
Rinkevich, B., and Rinkevich, Y. (2012). The “stars and stripes” metaphor for animal regeneration-elucidating two fundamental strategies along a continuum. Cells 2 (1), 1–18. doi:10.3390/cells2010001
Rivlin, N., Katz, S., Doody, M., Sheffer, M., Horesh, S., Molchadsky, A., et al. (2014). Rescue of embryonic stem cells from cellular transformation by proteomic stabilization of mutant p53 and conversion into WT conformation. Proc. Natl. Acad. Sci. 111 (19), 7006–7011. doi:10.1073/pnas.1320428111
Sadej, R., Grudowska, A., Turczyk, L., Kordek, R., and Romanska, H. M. (2014). CD151 in cancer progression and metastasis: a complex scenario. Lab. Invest. 94 (1), 41–51. doi:10.1038/labinvest.2013.136
Saito, M., Ohyama, M., and Amagai, M. (2015). Exploring the biology of the nail: an intriguing but less-investigated skin appendage. J. Dermatol. Sci. 79 (3), 187–193. doi:10.1016/j.jdermsci.2015.04.011
Salnikov, L., and Baramiya, M. G. (2020). The Ratio of the genome two functional parts activity as the prime cause of aging. Front. Aging 1. doi:10.3389/fragi.2020.608076
Sarig, R., and Tzahor, E. (2017). The cancer paradigms of mammalian regeneration: can mammals regenerate as amphibians? Carcinogenesis 38 (4), 359–366. doi:10.1093/carcin/bgw103
Satoh, A., Graham, G. M. C., Bryant, S. V., and Gardiner, D. M. (2008). Neurotrophic regulation of epidermal dedifferentiation during wound healing and limb regeneration in the axolotl (Ambystoma mexicanum). Develop. Biol. 319 (2), 321–335. doi:10.1016/j.ydbio.2008.04.030
Satoh, A., Makanae, A., Hirata, A., and Satou, Y. (2011). Blastema induction in aneurogenic state and Prrx-1 regulation by MMPs and FGFs in Ambystoma mexicanum limb regeneration. Develop. Biol. 355 (2), 263–274. doi:10.1016/j.ydbio.2011.04.017
Schaper, F., and van Spriel, A. B. (2018). Antitumor immunity is controlled by tetraspanin proteins. Front. Immunol. 9, 1185. doi:10.3389/fimmu.2018.01185
Schiaffino, S., Pereira, M. G., Ciciliot, S., and Rovere-Querini, P. (2017). Regulatory T cells and skeletal muscle regeneration. FEBS J. 284, 517–524. doi:10.1111/febs.13827
Seifert, A. W., Monaghan, J. R., Voss, S. R., and Maden, M. (2012). Skin regeneration in adult axolotls: a blueprint for scar-free healing in vertebrates. PLoS ONE 7 (4), e32875. doi:10.1371/journal.pone.0032875
Seubert, B., Cui, H., Simonavicius, N., Honert, K., Schäfer, S., Reuning, U., et al. (2015). Tetraspanin CD63 acts as a pro-metastatic factorviaβ-catenin stabilization. Int. J. Cancer 136 (10), 2304–2315. doi:10.1002/ijc.29296
Sheng, K.-C., van Spriel, A. B., Gartlan, K. H., Sofi, M., Apostolopoulos, V., Ashman, L., et al. (2009). Tetraspanins CD37 and CD151 differentially regulate Ag presentation and T-cell co-stimulation by DC. Eur. J. Immunol. 39 (1), 50–55. doi:10.1002/eji.200838798
Shigematsu, T., Wolf, R. E., and Granger, D. N. (2002). T-lymphocytes modulate the microvascular and inflammatory responses to intestinal ischemia-reperfusion. Microcirculation 9 (2), 99–109. doi:10.1080/mic.9.2.99.109
Shoffner, A., Cigliola, V., Lee, N., Ou, J., and Poss, K. D. (2020). Tp53 suppression promotes cardiomyocyte proliferation during zebrafish heart regeneration. Cell Rep. 32, 108089. doi:10.1016/j.celrep.2020.108089
Simkin, J., Gawriluk, T. R., Gensel, J. C., and Seifert, A. W. (2017). Macrophages are necessary for epimorphic regeneration in African spiny mice. Elife 6, e24623. doi:10.7554/eLife.24623
Sousounis, K., Michel, C. S., Bruckskotten, M., Maki, N., Borchardt, T., Braun, T., et al. (2013). A microarray analysis of gene expression patterns during early phases of newt lens regeneration. Mol. Vis. 19, 135–145.
Staal, F. J. T., Luis, T. C., and Tiemessen, M. M. (2008). WNT signalling in the immune system: WNT is spreading its wings. Nat. Rev. Immunol. 8 (8), 581–593. doi:10.1038/nri2360
Stewart, R., Rascón, C. A., Tian, S., Nie, J., Barry, C., Chu, L.-F., et al. (2013). Comparative RNA-seq analysis in the unsequenced axolotl: the oncogene burst highlights early gene expression in the blastema. PLos Comput. Biol. 9, e1002936. doi:10.1371/journal.pcbi.1002936
Taams, L. S., van Amelsfort, J. M. R., Tiemessen, M. M., Jacobs, K. M. G., de Jong, E. C., Akbar, A. N., et al. (2005). Modulation of monocyte/macrophage function by human CD4+CD25+ regulatory T cells. Hum. Immunol. 66 (3), 222–230. doi:10.1016/j.humimm.2004.12.006
Takigawa, A., Takada, K., Taube, C., Miyahara, N., Matsubara, S., Koya, T., et al. (2007). Naturally occurring lung CD4+CD25+ T cell regulation of airway allergic responses depends on IL-10 induction of TGF-β. J. Immunol. 178 (3), 1433–1442. doi:10.4049/jimmunol.178.8.540010.4049/jimmunol.178.3.1433
Tang, T. T., Yuan, J., Zhu, Z. F., Zhang, W. C., Xiao, H., Xia, N., et al. (2012). Regulatory T cells ameliorate cardiac remodeling after myocardial infarction. Basic Res. Cardiol. 107 (1), 232. doi:10.1007/s00395-011-0232-6
Thouveny, Y., and Tassava, R. (1998). “Regeneration through phylogenesis,” in Cellular and molecular basis of regeneration: from invertebrates to humans. Editors P. Ferretti, and J. Geraudie (Chichester, United Kingdom: John Wiley & Sons), 9–43.
Tiemessen, M. M., Jagger, A. L., Evans, H. G., van Herwijnen, M. J. C., John, S., and Taams, L. S. (2007). CD4+CD25+Foxp3+ regulatory T cells induce alternative activation of human monocytes/macrophages. Proc. Natl. Acad. Sci. 104 (49), 19446–19451. doi:10.1073/pnas.0706832104
Tong, M., Abrahams, V. M., and Chamley, L. W. (2018). Immunological effects of placental extracellular vesicles. Immunol. Cell Biol Available at: https://onlinelibrary.wiley.com/doi/full/10.1111/imcb.12049 (Accessed March 31, 2018).
Trowsdale, J., and Betz, A. G. (2006). Mother’s little helpers: mechanisms of maternal-fetal tolerance. Nat. Immunol. 7 (3), 241–246. doi:10.1038/ni1317
Unkelles, J., Cordon, S., and Retch, E. (1974). Secretion of plasminogen activator by stimulated macrophages. J. Exp. Med. 139 (4), 834–850. doi:10.1084/jem.139.4.834
Uygur, A., and Lee, R. T. (2016). Mechanisms of cardiac regeneration. Develop. Cell 36 (4), 362–374. doi:10.1016/j.devcel.2016.01.018
Vannella, K. M., and Wynn, T. A. (2017). Mechanisms of organ injury and repair by macrophages. Annu. Rev. Physiol. 79, 593–617. doi:10.1146/annurev-physiol-022516-034356
Varda-Bloom, N., Leor, J., Ohad, D. G., Hasin, Y., Amar, M., Fixler, R., et al. (2000). Cytotoxic T lymphocytes are activated following myocardial infarction and can recognize and kill healthy myocytes in vitro. J. Mol. Cell Cardiol. 32 (12), 2141–2149. doi:10.1006/jmcc.2000.1261
Veeneman, J. M., de Jong, P. E., Huisman, R. M., and Reijngoud, D.-J. (2001). Why is muscle protein synthesis, but not whole body protein synthesis, reduced in CRF patients?. Am. J. Physiology-Endocrinology Metab. 280 (1), E197. doi:10.1152/ajpendo.2001.280.1.E197
Vinarsky, V., Atkinson, D. L., Stevenson, T. J., Keating, M. T., and Odelberg, S. J. (2005). Normal newt limb regeneration requires matrix metalloproteinase function. Develop. Biol. 279 (1), 86–98. doi:10.1016/j.ydbio.2004.12.003
Vitulo, N., Dalla Valle, L., Valle, G., Alibardi, L., and Alibardi, L. (2017a). Downregulation of lizard immuno-genes in the regenerating tail and myogenes in the scarring limb suggests that tail regeneration occurs in an immuno-privileged organ. Protoplasma 254 (6), 2127–2141. doi:10.1007/s00709-017-1107-y
Vitulo, N., Dalla Valle, L., Skobo, T., Valle, G., and Alibardi, L. (2017b). Transcriptome analysis of the regenerating tail vs. the scarring limb in lizard reveals pathways leading to successful vs. unsuccessful organ regeneration in amniotes. Dev. Dyn. 246 (2), 116–134. doi:10.1002/dvdy.24474
Voisin, G. A. (1987). Regulatory facilitation reaction and active tolerance: a non-euclidian view of the immune reaction authenticated by immunology of reproduction. Immunol. Lett. 16 (3–4), 283–289. doi:10.1016/0165-2478(87)90159-3
Wang, G., Miyahara, Y., Guo, Z., Khattar, M., Stepkowski, S. M., and Chen, W. (2010). “Default” generation of neonatal regulatory T cells. J.I. 185 (1), 71–78. doi:10.4049/jimmunol.0903806
Weaver, V. M., Petersen, O. W., Wang, F., Larabell, C. A., Briand, P., Damsky, C., et al. (1997). Reversion of the malignant phenotype of human breast cells in three-dimensional culture and in vivo by integrin blocking antibodies. J. Cell. Biol. 137 (1), 231–245. doi:10.1083/jcb.137.1.231
Webb, C. G., Gootwine, E., and Sachs, L. (1984). Developmental potential of myeloid leukemia cells injected into midgestation embryos. Develop. Biol. 101 (1), 221–224. doi:10.1016/0012-1606(84)90132-5
Weirather, J., Hofmann, U. D. W., Beyersdorf, N., Ramos, G. C., Vogel, B., Frey, A., et al. (2014). Foxp3 + CD4 + T cells improve healing after myocardial infarction by modulating monocyte/macrophage differentiation. Circ. Res. 115 (1), 55–67. doi:10.1161/CIRCRESAHA.115.303895
Whisson, M. (1967). “The interaction of tumors and embryonic tissue in vitro,” in Cell differentiation. Editors A. V. S. de Reuck, and J. Knight (London, United Kingdom: Churchill), 219–230.
Wilgus, T. A. (2007). Regenerative healing in fetal skin: a review of the literature. Ostomy Wound Manage 53 (6), 16–23.
Williams, G. C. (1957). Pleiotropy, natural selection, and the evolution of senescence. Evolution 11 (4), 398–411. doi:10.2307/2406060
Wong, A. Y., and Whited, J. L. (2020). Parallels between wound healing, epimorphic regeneration and solid tumors. Development 147. doi:10.1242/dev.181636
Xu, Y., Zhang, Y., García-Cañaveras, J. C., Guo, L., Kan, M., Yu, S., et al. (2020). Chaperone-mediated autophagy regulates the pluripotency of embryonic stem cells. Science 369 (6502), 397–403. doi:10.1126/science.abb4467
Yamazaki, S., Inaba, K., Tarbell, K. V., and Steinman, R. M. (2006). Dendritic cells expand antigen-specific Foxp3+CD25+CD4+ regulatory T cells including suppressors of alloreactivity. Immunol. Rev. 212, 314–329. doi:10.1111/j.0105-2896.2006.00422.x
Yang, Z., Day, Y.-J., Toufektsian, M.-C., Xu, Y., Ramos, S. I., Marshall, M. A., et al. (2006). Myocardial infarct-sparing effect of adenosine A 2A receptor activation is due to its action on CD4 + T lymphocytes. Circulation 114 (19), 2056–2064. doi:10.1161/CIRCULATIONAHA.106.649244
Ye, S., Zhao, T., Zhang, W., Tang, Z., Gao, C., Ma, Z., et al. (2020). p53 isoform Δ113p53 promotes zebrafish heart regeneration by maintaining redox homeostasis. Cell Death Dis 11 (7), 568. doi:10.1038/s41419-020-02781-7
Zacchigna, S., Martinelli, V., Moimas, S., Colliva, A., Anzini, M., Nordio, A., et al. (2018). Paracrine effect of regulatory T cells promotes cardiomyocyte proliferation during pregnancy and after myocardial infarction. Nat. Commun. 9 (1), 2432. doi:10.1038/s41467-018-04908-z
Zeng, A., Li, H., Guo, L., Gao, X., McKinney, S., Wang, Y., et al. (2018). Prospectively isolated tetraspanin+ neoblasts are adult pluripotent stem cells underlying planaria regeneration. Cell 173 (7), 1593–1608. doi:10.1016/j.cell.2018.05.006
Zhao, H.-X., Jiang, F., Zhu, Y.-J., Wang, L., Li, K., Li, Y., et al. (2017). Enhanced immunological tolerance by HLA-G1 from neural progenitor cells (NPCs) derived from human embryonic stem cells (hESCs). Cell Physiol. Biochem. 44 (4), 1435–1444. doi:10.1159/000485539
Zöller, M. (2009). Tetraspanins: push and pull in suppressing and promoting metastasis. Nat. Rev. Cancer 9 (1), 40–55. doi:10.1038/nrc2543
Zordan, P., Rigamonti, E., Freudenberg, K., Conti, V., Azzoni, E., Rovere-Querini, P., et al. (2014). Macrophages commit postnatal endothelium-derived progenitors to angiogenesis and restrict endothelial to mesenchymal transition during muscle regeneration. Cell Death Dis 5 (1), e1031. doi:10.1038/cddis.2013.558
Keywords: aging, senescence, multicellularity, carcinogenesis, reontogenesis, immunological tolerance, epigenetic
Citation: Salnikov L and Baramiya MG (2021) From Autonomy to Integration, From Integration to Dynamically Balanced Integrated Co-existence: Non-aging as the Third Stage of Development. Front. Aging 2:655315. doi: 10.3389/fragi.2021.655315
Received: 18 January 2021; Accepted: 02 February 2021;
Published: 25 March 2021.
Edited by:
Sergei Vatolin, Case Western Reserve University, United StatesReviewed by:
Saveli Goldberg, Massachusetts General Hospital Cancer Center, United StatesCopyright © 2021 Salnikov and Baramiya. This is an open-access article distributed under the terms of the Creative Commons Attribution License (CC BY). The use, distribution or reproduction in other forums is permitted, provided the original author(s) and the copyright owner(s) are credited and that the original publication in this journal is cited, in accordance with accepted academic practice. No use, distribution or reproduction is permitted which does not comply with these terms.
*Correspondence: Lev Salnikov, bGVvc2Fsbmlrb3ZAZ21haWwuY29t
†deceased
Disclaimer: All claims expressed in this article are solely those of the authors and do not necessarily represent those of their affiliated organizations, or those of the publisher, the editors and the reviewers. Any product that may be evaluated in this article or claim that may be made by its manufacturer is not guaranteed or endorsed by the publisher.
Research integrity at Frontiers
Learn more about the work of our research integrity team to safeguard the quality of each article we publish.