- Davis School of Gerontology, University of Southern California, Los Angeles, CA, United States
The main genetic risk factor for Alzheimer’s disease (AD) is the apolipoprotein E ε4 allele (APOE4). AD risk associated with APOE4 disproportionately affects women. Furthermore, human and rodent studies indicate that the cognitive deficits associated with APOE4 are greater in females. One modifiable AD risk factor is obesity during middle age. Given that approximately two-thirds of US adults are overweight, it is important to understand how obesity affects AD risk, how it interacts with APOE4, and the extent to which its detrimental effects can be mitigated with therapeutics. One intervention study for women is estrogen-based hormone therapy, which can exert numerous health benefits when administered in early middle age. No experimental studies have examined the interactions among APOE4, obesity, and hormone therapy in aging females. To begin to explore these issues, we considered how obesity outcomes are affected by treatment with estradiol at the onset of middle age in female mice with human APOE3 and APOE4. Furthermore, to explore how gene dosage affects outcomes, we compared mice homozygous for APOE3 (3/3) and homozygous (4/4) or hemizygous (3/4) for APOE4. Mice were examined over a 4-month period that spans the transition into reproductive senescence, a normal age-related change that models many aspects of human perimenopause. Beginning at 5 months of age, mice were maintained on a control diet (10% fat) or high-fat diet (HFD; 60% fat). After 8 weeks, by which time obesity was present in all HFD groups, mice were implanted with an estradiol or vehicle capsule that was maintained for the final 8 weeks. Animals were assessed on a range of metabolic and neural measures. Overall, APOE4 was associated with poorer metabolic function and cognitive performance. However, an obesogenic diet induced relatively greater impairments in metabolic function and cognitive performance in APOE3/3 mice. Estradiol treatment improved metabolic and cognitive outcomes across all HFD groups, with APOE4/4 generally exhibiting the greatest benefit. APOE3/4 mice were intermediate to the homozygous genotypes on many measures but also exhibited unique profiles. Together, these findings highlight the importance of the APOE genotype as a modulator of the risks associated with obesity and the beneficial outcomes of estradiol.
1 Introduction
The ε4 allele of apolipoprotein 4 (APOE4) is the most significant genetic risk factor for late-onset Alzheimer’s disease (AD). The mechanisms by which APOE4 impacts AD risk are thought to be numerous and include regulation of β-amyloid clearance, metabolism, inflammation, glial dynamics, and vascular function (Serrano-Pozo et al., 2021; Parhizkar and Holtzman, 2022; Tzioras et al., 2019; Norwitz et al., 2021). The multifactorial nature of APOE4-associated risks for age-related cognitive decline and AD has been hypothesized to result from apoE’s actions on cell types and systems throughout the body (Martens et al., 2022). Consistent with its widespread impacts, APOE4-associated AD risk is thought to be influenced by its interaction with other risk factors, including obesity (Jones and Rebeck, 2018), female sex (Valencia-Olvera et al., 2023), and even the combined effects of obesity and sex (Moser and Pike, 2016).
Obesity at midlife is a risk factor for AD and related disorders as well as age-related cognitive decline (Whitmer et al., 2005; Besser et al., 2014). The relationship between obesity and neural vulnerability is modulated by the APOE genotype (Zade et al., 2013), which aligns with the findings that APOE4 carriers are at increased risk for metabolic syndrome and cardiovascular disease (El-Lebedy et al., 2016; Torres-Perez et al., 2016). However, the relationship is complicated by interactions that are incompletely understood (Moser and Pike, 2016). In studies of both humans (Mole et al., 2020; Osiecka et al., 2023; Shinohara et al., 2020; Zhao et al., 2023; Coad et al., 2022) and rodents (Moser and Pike, 2017; Arbones-Mainar et al., 2016; Johnson et al., 2017; Jones et al., 2021; Pandit et al., 2024; Christensen and Pike, 2019), the findings are mixed, with evidence that the deleterious CNS effects of obesity are worsened in APOE4 carriers in some studies but in APOE4 non-carriers in others.
One key factor contributing to the disparate observations is biological sex. Sex is known to interact with the APOE genotype. There is a significant female bias for many neural effects of APOE, ranging from AD risk to depression to changes in white matter volume (Valencia-Olvera et al., 2023; Delano-Wood et al., 2008; Takeuchi et al., 2021). Interestingly, females often show greater APOE-related CNS effects of obesity than males (Pandit et al., 2024; Espeland et al., 2021). The consequences of APOE on females may be particularly significant at the onset of age-associated changes in the levels of and altered responsiveness to the primary estrogen hormone, 17β-estradiol (17βE2). In women, the perimenopause transition is linked with numerous health implications including increased vulnerability to AD (Pike, 2017) and development of central adiposity and associated cardiometabolic risks (Palacios et al., 2024). Conversely, estrogen-based hormone therapies have been shown to have multi-systemic effects relevant to protection against both AD and systemic metabolic outcomes (Valencia-Olvera et al., 2023; Nerattini et al., 2023; Anagnostis and Goulis, 2019). Certainly, the use of estrogen-based therapies is not without controversy (Koire et al., 2022), but a greater understanding of its risks and benefits is needed, especially in the contexts of APOE genotype and obesity.
In the current study, we seek to gain a greater understanding of the relationships among APOE genotype, obesity, and estrogen-based treatment in females. To accomplish this, we compared systemic and neural effects of an obesogenic, high-fat diet (HFD) in the presence and absence of treatment with 17βE2 on female APOE knock-in mice at an age just prior to the onset of reproductive senescence. Importantly, our study design allowed the testing of the effects of 17βE2 after the establishment of obesity across APOE genotypes. Because the number of APOE4 alleles likely affects many metabolic and neural endpoints but has not been well investigated in human populations and not at all in rodent studies, we also considered possible differences between homo- and heterozygous APOE4 status by studying mice with APOE3/3, APOE3/4, and APOE4/4 genotypes.
2 Methods
2.1 Animals
A colony of EFAD mice, which have homozygous knock-in of human APOE and hemizygous overexpression of AD transgenes from the 5xFAD model (APOE +/+, 5xFAD+/−) (Youmans et al., 2012), were maintained at vivarium facilities at University of Southern California from breeder mice generously provided by Dr. Mary Jo LaDu (University of Illinois at Chicago). Breeding protocols for EFAD mice yield litters in which ~50% retain APOE knock-in but are non-carriers of AD transgenes (APOE +/+, 5xFAD−/−). Female EFAD non-carriers (APOE mice) with APOE 3/3, 3/4, and 4/4 genotypes were studied; APOE3/4 mice were generated by breeding APOE3/3 with APOE4/4 mice. Mice were maintained under controlled temperature, on a 12:12-h light/dark schedule (with lights on at 0600), and had ad libitum access to food and water. At 5 months of age, female APOE3/3, APOE3/4, and APOE4/4 mice were randomly assigned to either control (10% calories from fat and 7% from sugar; catalog #D12450J, Research Diets, Inc., New Brunswick, NJ, United States) or an ingredient-matched high-fat diet (HFD; 60% calories from fat and 7% from sugar; catalog #D12492i, Research Diets, Inc.) (n = 22/group). After 8 weeks of diet, animals were subcutaneously implanted between the shoulder blades with either a vehicle (cholesterol) or 17βE2-filled Silastic capsule (25% w/w 17βE2, 75% w/w cholesterol; 1.47 mm ID × 1.96 mm OD; Dow Corning, Midland, MI; n = 11/group) to administer constant release of hormone (Christensen et al., 2020). Animals were euthanized 8 weeks later, resulting in 16 total weeks of diet exposure. The experimental procedure is outlined in Figure 1. All procedures were conducted under a protocol (#20648) approved by the USC Institution for Animal Care and Use Committee and under the supervision of USC veterinarians.

Figure 1. Timeline of experiments. At Week 0 of the study, female APOE3/3, APOE3/4, and APOE4/4 knock-in mice at age 5 mo began a 16-week exposure to control or high-fat diet (HFD). At Week 8 (animal age 7 mo), animals were implanted with a Silastic capsule containing vehicle or 17β-estradiol. At Week 14 (6 weeks after capsule implant), animals were behaviorally assessed. One week later, animals were administered an oral glucose tolerance test. Finally, at Week 16 (8 weeks after capsule implant, 16 total weeks of diet, animal age 9 mo), animals were euthanized and tissues were collected for analyses.
2.2 Glucose tolerance test
A glucose tolerance test was performed at Week 15 (7 weeks after the start of hormone treatment). Animals were fasted overnight (~16 h) and orally gavaged with 2 g/kg D-glucose. Blood glucose levels were measured at 0, 15, 30, 60, and 120 min following glucose administration. Five microliters of blood were collected on a glucose test strip and assayed using a Precision Xtra Glucose Monitor (Abbott Laboratories, Abbott Park, IL, United States).
2.3 Tissue collection
At the end of the 16-week treatment period, mice were euthanized following overnight fasting, after which the brain was rapidly removed. One hemibrain was fixed for 72 h in 4% paraformaldehyde for immunohistochemistry, and the other was rapidly dissected and frozen. Blood was collected and kept on ice prior to centrifugation to collect plasma, which was stored in aliquots at −80° until assayed. The retroperitoneal and visceral (which included gonadal and uterine fat) fat pads were dissected, weighed, and frozen.
2.4 Immunohistochemistry
Fixed hemibrains were sectioned exhaustively in the horizontal plane at 40 μm using a vibratome (Leica Biosystems). The sections were stored singly in PBS with 0.03% sodium azide at 4°C until immunohistochemistry was performed. Every eighth section (from a total of ~100 per brain) was immunostained with the following primary antibodies: doublecortin (1:2,500; Santa Cruz) as a marker of new neurons, and Iba-1 (1:2,000; Wako) for microglia. In brief, the tissue sections containing the hippocampus were washed three times for 5 min in TBS, followed by a 10-min rinse with an endogenous peroxidase-blocking solution. Next, the sections were rinsed in TBS/0.1% Triton-X before being incubated for 30 min in a blocking solution consisting of TBS/2% bovine serum albumin or normal horse serum. The sections were incubated overnight at 4°C with the primary antibody diluted in a blocking solution. The sections incubated in the primary antibody were washed and then incubated with the appropriate biotinylated secondary antibody (Vector Laboratories) for 1 h and processed for diaminobenzidine visualization using a Vectastain ABC Elite kit (Vector Laboratories). The stained sections were air-dried overnight, dehydrated in a series of graded alcohols, and then coverslipped with Krystalon (EMD Millipore). One brain from the APOE3/3 HFD vehicle group was damaged during the sectioning process and could not be used for further analysis.
2.5 Quantification of immunolabeled cells
Doublecortin (DCX)-immunoreactive cells were counted in four brain sections that contained the dentate gyrus. Positive labeling was defined as cells that were darkly stained across the majority of the soma. The DCX-labeled cells were counted across the entire dentate gyrus. Cell bodies were mostly found in the subgranular zone or granule cell layer. For microglia, the activated phenotype was based on morphological analysis of Iba-1 immunoreactive cells, as previously described (Christensen and Pike, 2018; Christensen and Pike, 2017). The density of Iba-1 immunoreactive cells in the hippocampus was estimated by two-dimensional counts. In brief, an Olympus BX50 microscope equipped with a motorized stage and computer-guided CASTGrid software (Olympus) was used for unbiased sampling. In the four brain sections per animal, the area containing the subiculum and CA1-CA3 subregions of the hippocampus (excluding the dentate gyrus) was sampled at high magnification. Within each field, the cells within a counting frame (3,000 μm2) were used for analysis. Microglia were classified as either type 1 (many thin, ramified processes), type 2 (short, thick processes and a rod-shaped cell body), or type 3 (no or few short non-ramified processes or many filapodial processes) cells. Type 2 and 3 cells were considered to exhibit an activated microglia morphological phenotype.
2.6 ELISAs
Fasting levels of leptin, adiponectin, and insulin in plasma collected at euthanization were determined using mouse leptin (Millipore, Catalog # EZML-82 K), adiponectin (Millipore, Catalog #EZMADP-60 K), and insulin (Millipore, Catalog #EZRMI) ELISA kits according to the manufacturer’s instructions. Each animal and standard were tested in duplicate. For the insulin ELISA, values from eight animals were significantly above background (>0.1 ng/mL) but slightly below the lowest standard (0.2 ng/mL); these were assigned a value of 0.2 ng/mL. One animal was excluded due to an exceedingly high level (>3 standard deviations above the mean). For the leptin ELISA, two animals were excluded owing to values at or near the background level.
2.7 Lipidomics
Shotgun lipidomics of plasma was performed by the UCLA Lipidomics Core. A modified Bligh and Dyer extraction (Hsieh et al., 2020) was carried out on all plasma samples. Prior to biphasic extraction, an internal standard mixture consisting of 70 lipid standards across 17 subclasses was added to each sample (AB Sciex 5040156, Avanti 330827, Avanti 330830, Avanti 330828, Avanti 791642). Following two successive extractions, pooled organic layers were dried down in a Thermo SpeedVac SPD300DDA using ramp setting 4 at 35°C for 45 min with a total run time of 90 min. Lipid samples were resuspended in 1:1 methanol/dichloromethane with 10 mM ammonium acetate and transferred to robovials (Thermo 10800107) for analysis. The samples were analyzed on the Sciex 5500 with a DMS device (Lipidyzer Platform) with an expanded targeted acquisition list consisting of 1,450 lipid species across 17 subclasses. The differential mobility device on Lipidyzer was tuned with EquiSPLASH LIPIDOMIX (Avanti 330731). The instrument methods including settings, tuning protocol, and MRM list were as previously described (Su et al., 2021).
A total of 862 lipid species were originally detected and subjected to lipidomic bioinformatics analyses. After removing lipids not detected across all samples, 515 remained. The dataset was first normalized to the amount of plasma; then, variance stabilizing normalization was applied to the data using “limma” v.3.48.3, as recommended by previous studies (Jauhiainen et al., 2014; Li et al., 2016). Differential analysis was performed using ‘limma’ in R. Lipids with an FDR < 5% were considered statistically significant. Lipid ontology enrichment analysis was performed using the LION web-based ontology enrichment tool with all detected lipids that passed filtering used as the background (Molenaar et al., 2019).
2.8 Behavior
At Week 14, animals were behaviorally assessed using (i) spontaneous alternation behavior test in a Y-maze (SAB), (ii) novel object placement (NOP), and (iii) novel object recognition (NOR) (Figure 1). The spontaneous alternation test is dependent upon the hippocampus and other limbic structures (Lalonde, 2002) and assesses spatial memory and attention toward novelty (Hughes, 2004). Animals were allowed to acclimate to the behavior room for 30 min prior to testing. Next, animals were placed in the long arm of a Y-maze facing away from the other arms to start the test. Arm entries (at least two paws placed into an arm) were recorded for 5 min. Animals with fewer than 20 or more than 50 arm entries were excluded from the analysis. Percent spontaneous alternation was calculated as the number of correct triplicates divided by the total number of triplicate arm entries.
NOP and NOR are cognitive measures that assess learning and memory, involving the hippocampal and parahippocampal regions (Antunes and Biala, 2012). Prior to each day of testing, animals were habituated to the room for at least 30 min. Mice were habituated to the apparatus without objects for 5 min a day for 3 days prior to testing. On the test day, two identical objects were placed into the box, and the mice were allowed to explore for 5 min (sampling). After 15 min, the animals were placed again in the chamber with the same objects, but one object was moved 90 degrees in the chamber (NOP). Mice were allowed to explore for 5 min. Fifteen minutes after NOP, the mice are placed in the chamber with one familiar object and one novel object for 5 min (NOR). The time the mice spent exploring the objects was recorded, and the discrimination index was calculated as (time with novel – time with familiar)/total time with objects. Animals were excluded if they did not spend at least 8 s exploring the objects or did not spend at least some time with each object.
2.9 Statistics
All data are reported as the mean ± the standard error of the mean as well as values from individual animals where suitable. The data were analyzed using GraphPad Prism version 8. To compare the effects of experimental treatments across APOE genotypes, most data were statistically analyzed using three-way ANOVA followed by Tukey’s post-hoc tests when appropriate. Three-way repeated measure ANOVA followed by Tukey’s post-hoc tests was used to analyze data measured over time (body weight and GTT). These statistical analyses are listed in Supplementary Table S1. For comparison, the effects of experimental treatments were also assessed within individual APOE genotypes using two-way ANOVA followed by Tukey’s post-hoc tests when appropriate (Supplementary Table S2).
3 Results
3.1 17β-estradiol reduces diet-induced increases in body weight and adiposity across APOE genotypes
To investigate how vulnerability to obesity in female mice is affected by increasing APOE4 allelic dosage, APOE3/3, APOE3/4, and APOE4/4 mice were fed either a control or high-fat diet (HFD) beginning at age 5 months (Week 0), which corresponds with peak ovarian cyclicity in virgin mice (Nelson et al., 1982). To further assess estrogen regulation of this relationship, mice were implanted with a delivery capsule containing either vehicle or 17βE2 at age 7 months (Week 8) (Figure 1), the earliest timepoint of age-related irregular cycling and decreased fecundity in mice (Finch, 2014).
During Weeks 0–8, animals in all three genotypes maintained on HFD gained significantly more weight than genotype-matched mice on the control diet (Figures 2A–D; Supplementary Tables S1, S2). During Weeks 8–16 when 17βE2 or vehicle treatments were present, HFD continued to result in further increases in body weight with vehicle treatment (Figures 2A–C,E); however, this increase was only significant relative to the matched control diet group in APOE3/3 mice (Figure 2E). Treatment with 17βE2 did not significantly affect body weight with control diet but blunted HFD-induced increases in body mass (Figures 2A–C). In APOE3/3 and APOE3/4 mice, 17βE2 completely halted HFD-induced weight gain across weeks 8–16 (Figure 2E). This 17βE2 effect was stronger in APOE4/4 mice, in which there was a non-significant trend for 17βE2 to reduce body weight (p = 0.053; Figures 2C,E) such that mice-fed HFD had lowered body mass to levels very similar to the control diet (Figure 2C).
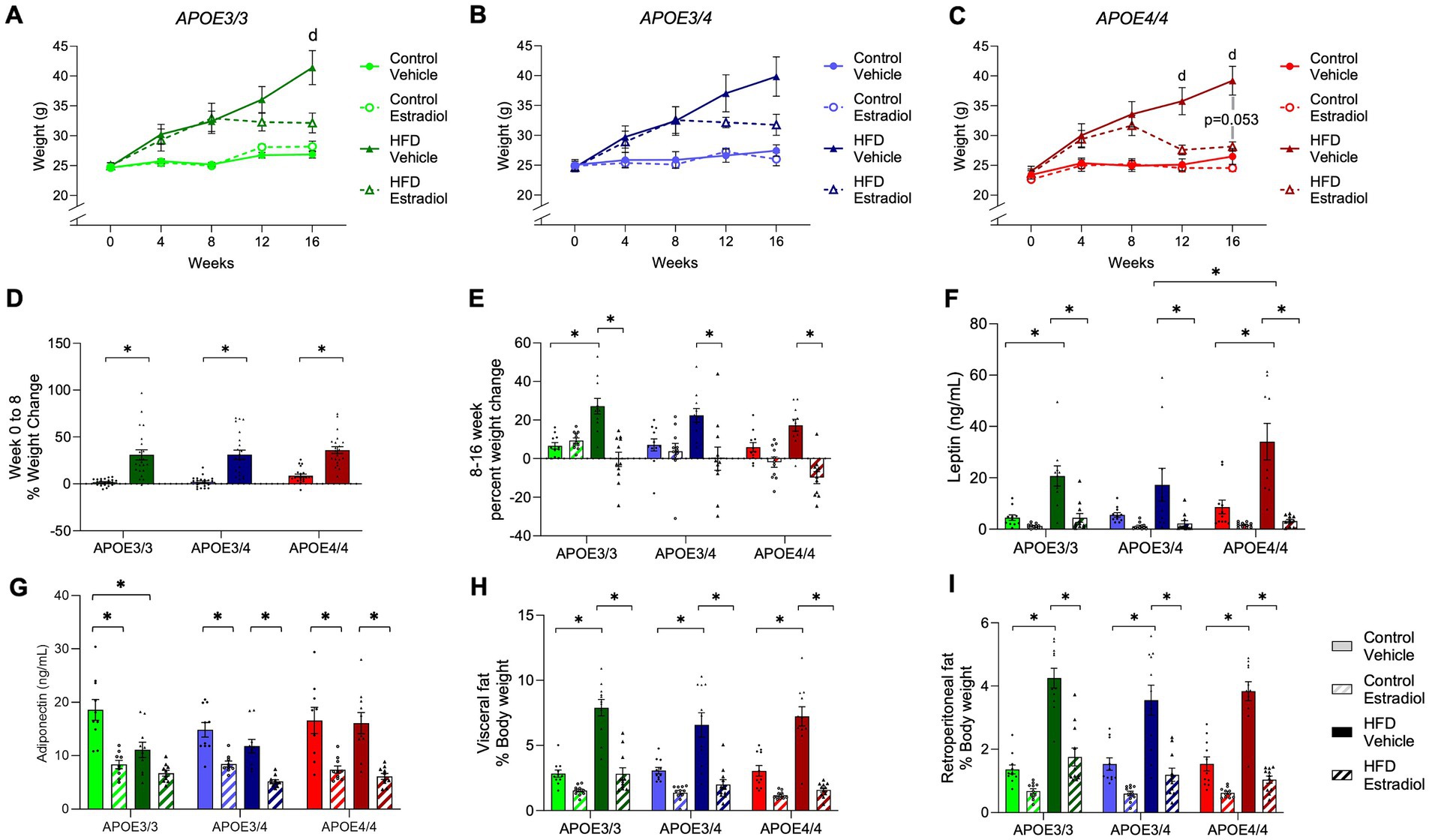
Figure 2. Effects of APOE, HFD, and estradiol on body weight. (A–C) Body weight was measured weekly. (D) The percent weight change was measured for Weeks 0–8 of the experiment, prior to capsule implant. (E) The percent weight gain was measured for Weeks 8–16 of the experiment, after capsule implant. (F) Plasma levels of leptin and (G) adiponectin were measured at the end of the experiment. (H) Visceral and (I) retroperitoneal fat masses were determined at Week 16 and are represented as the percentage of body weight. The lines and bars colored green are used for APOE3/3 mice, blue for APOE3/4 mice, and red for APOE4/4 mice. The lighter shades represent the control diet, and the darker shades represent HFD. The solid lines and bars represent vehicle capsules, and the hatched lines and bars represent 17β-estradiol capsules. All data are represented as the mean ± SEM with values shown for individual animals where appropriate; n = 11 per group. Statistical significance with p < 0.05 is denoted by asterisks. Brackets indicate significant differences between specified groups. “d” represents significant differences between control vehicle and HFD vehicle groups. In panel C, “p = 0.053” identifies the statistical trend between the HFD vehicle and HFD estradiol groups.
At Week 16, adipose tissues were collected and weighed. HFD increased visceral and retroperitoneal fat pad weights at similar relative levels in mice from all three genotypes (Figures 2H–I). There was a main effect of 17βE2 treatment across diets, but the decrease in fat pad weight induced by 17βE2 reached statistical significance only in the HFD-fed animals (Supplementary Table S1); 17βE2 reduced adiposity in APOE4/4 mice on the control diet when statistical analyses were performed within genotypes (Supplementary Table S2) rather than across genotypes (Supplementary Table S1). There were no statistically significant differences in adipose-lowering actions of 17βE2 across the APOE genotypes. We also measured plasma levels of leptin, a hormone secreted by adipose tissue that regulates feeding behavior and other functions (Friedman, 2019). There was a significant effect of the APOE genotype with APOE4/4 mice having the highest concentrations of leptin (Figure 2F). Treatment with 17βE2 reduced plasma leptin in both diets and all three genotypes, though the effects were significant only in the context of HFD (Figure 2F). Adiponectin is also released by adipose tissue and has been correlated with metabolic disorder and longevity (Nguyen, 2020; Atzmon et al., 2008). There was a main effect of diet on adiponectin levels, with post-hoc analyses showing a significant decrease in adiponectin levels due to HFD only in APOE3/3 mice. In contrast, APOE3/4 mice showed a non-significant decrease, and no reduction was observed in APOE4/4 mice (Figure 2G). Estradiol treatment decreased adiponectin levels even further regardless of diet or genotype (Figure 2G).
3.2 17β-estradiol improves diet-induced glucose intolerance most strongly in APOE4 carriers
To assess glucose metabolism, an oral glucose tolerance test was administered at week 15. HFD significantly impaired glucose clearance in all genotypes with evidence of modest regulation by 17βE2 (Figures 3A–C). Analysis of cumulative glucose clearance over time using area under the curve (AUC) showed a main effect of the APOE genotype. For the control diet with vehicle treatment, the lowest AUC was observed in APOE3/3, the highest was observed in APOE4/4, and intermediate levels were observed in APOE3/4 (Figure 3E). Notably, there was a significant three-way interaction among diet, APOE genotype, and 17βE2 treatment in which 17βE2 improved glucose tolerance specifically within APOE4 mice-fed HFD (Figure 3E). A similar relationship was observed when comparing the initial vs. final glucose values, which reflect the ability to return glucose levels to baseline levels. By this metric, both HFD-induced impairment and 17βE2 protection were observed only in APOE4 mice-fed HFD (Figure 3D). Fasting insulin was significantly decreased by estradiol in all genotypes (Figure 3F).
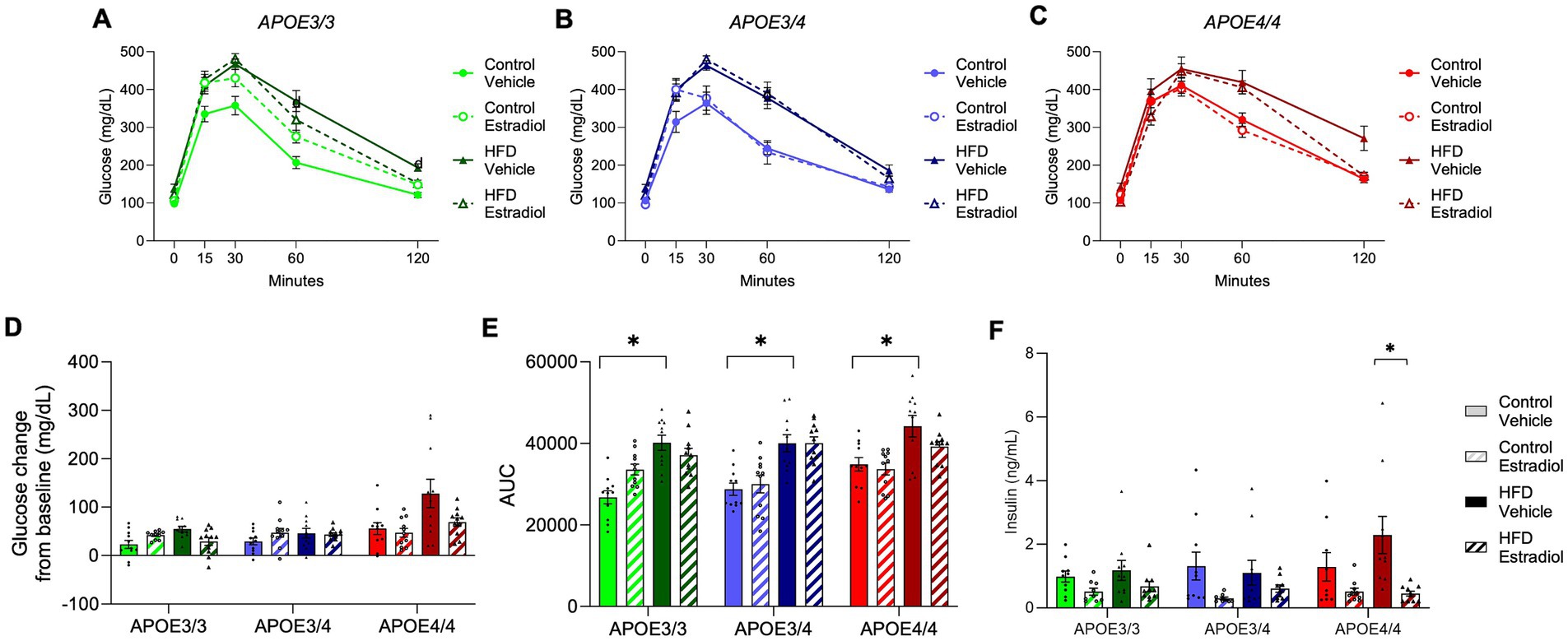
Figure 3. Effects of APOE, obesogenic diet, and estradiol on glucose clearance. (A–C) An oral glucose tolerance test was administered at Week 15. Blood glucose was measured 15, 30, 60, and 120 min after glucose administration. (D) The change in glucose concentration 2 h after glucose administration and (E) the area under the curve (AUC) were calculated from the glucose tolerance test. (F) Fasting plasma insulin was measured at the end of the experiment. The lines and bars colored green are used for APOE3/3 mice, blue for APOE3/4 mice, and red for APOE4/4 mice. Lighter shades represent the control diet, and darker shades represent HFD. The solid lines and bars represent vehicle capsules, and the hatched lines and bars represent 17β-estradiol capsules. All data are represented as the mean ± SEM with values shown for individual animals where appropriate; n = 11 per group. Statistical significance with p < 0.05 is denoted by asterisks. Brackets indicate significant differences between specified groups.
3.3 Effects of diet and 17β-estradiol on plasma lipids are strongest in APOE4 carriers
Given apoE’s key role as a regulator of the lipidome, we performed shotgun lipidomics on plasma from animals of all groups. Principal component analysis showed a clear separation of samples by diet, regardless of 17βE2 treatment or APOE genotype (Figure 4A). Examining changes in overall lipid classes, we found that multiple classes were affected by diet, 17βE2 treatment, genotype, and interactions among these factors (Supplementary Figure S1). Fatty acid (FA), ceramide (Cer), phosphatidylcholine (PC), phosphatidylinositol (PI), and phosphatidylethanolamine (PE) were significantly altered based on diet and APOE genotype, with Cer, PC, and PI also having significant interactions between diet, genotype, and 17βE2 treatment (Supplementary Figures S1A–E). In addition, there were significant main effects of APOE genotype and 17βE2 treatment on hexosyl ceramide (HexCer) levels, with 17βE2 generally increasing HexCer levels, especially when combined with HFD in the APOE3/4 mice (Supplementary Figure 1F). Abundances of triglycerides (TG) and phosphatidylglycerol (PG) showed main effects of APOE genotype, with TGs also having a significant interaction between genotype, diet, and 17βE2 treatment (Supplementary Figures 1G–H). Treatment with HFD had significant main effects on cholesterol ester (CE), diacylglycerol (DG), phosphatidic acid (PA), lyso-phosphatidylethanolamine (LPE), and lyso-phosphatidylcholine (LPC) (Supplementary Figures 1I–M). DGs were also significantly affected by 17βE2, with 17βE2 increasing DG levels. Both DG and PA had an interaction between genotype and diet, with DGs decreasing with HFD only in the APOE3/4 and APOE4/4 groups, and PA decreasing with HFD only in the APOE4/4 (Supplementary Figures 1J–K). There was an interaction between genotype and 17βE2, as well as among genotype, 17βE2, and diet, in LPE levels. Specifically, LPEs decreased only in APOE3/4 mice on HFD. Combining 17βE2 with HFD increased LPE levels in the APOE3/4 mice but decreased LPE in APOE4/4 (Supplementary Figure S1). There were no changes in plasma sphingomyelin levels across any groups (Supplementary Figures S1N).
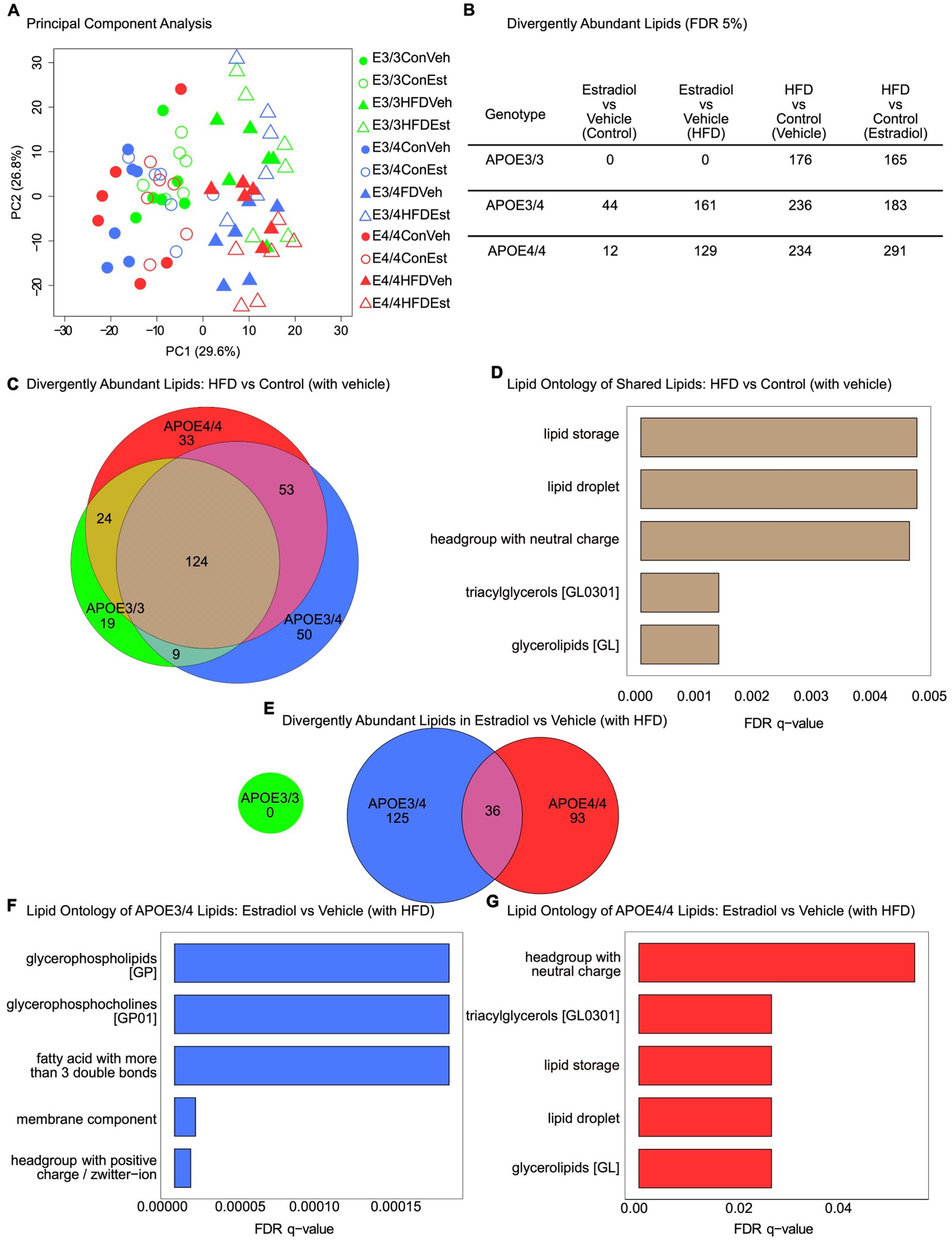
Figure 4. Effects of APOE genotype, HFD, and estradiol on plasma lipid profiles. Plasma samples collected at Week 16 were assessed by shotgun lipidomics. (A) Principal component analysis of lipidomes for plasma from all 12 groups. (B) Table of differentially abundant lipids (FDR 5%) across all relevant comparisons. (C) Venn diagram of differentially abundant lipids in the HFD vs. control (with vehicle) comparison. (D) LION lipid ontology analysis top enriched features in the shared lipids of the HFD vs. control (with vehicle) comparison. (E) Venn diagram of divergently abundant lipids in the estradiol vs. vehicle (with HFD) comparison. (F) LION lipid ontology analysis top enriched features in APOE3/4 estradiol vs. vehicle (with HFD) comparison. (G) LION lipid ontology analysis top enriched features in APOE4/4 estradiol vs. vehicle (with HFD) comparison. For all panels, green is used for APOE3/3 mice, blue for APOE3/4 mice, and red for APOE4/4 mice. For (A), circles represent the control diet, triangles represent HFD, shaded shapes represent vehicle capsules, and unshaded shapes represent 17β-estradiol capsules; n = 6 per group.
To better understand the specific effects of experimental factors on the lipidome, we identified lipids differentially abundant by condition within each APOE genotype at a false discovery rate (FDR) < 5% (Figure 4B). Notably, 17βE2 treatment resulted in significant differences in plasma lipid species abundance in both APOE3/4 and APOE4/4 mice but not in APOE3/3 mice (Figure 4B). HFD significantly impacted the lipidome in all three APOE genotypes, regardless of 17βE2 treatment. We compared the lipids significantly changed by HFD and found 124 shared lipids changed across genotypes (Figure 4C). Enrichment analysis using LION revealed that these shared lipids are primarily involved with lipid storage and lipid droplets and contain several triacylglycerols and glycerolipids (Figure 4D, FDR < 5%). The 17βE2 treatment had no effect on the lipidome in either the control or HFD APOE3/3 groups but had an additive effect in the APOE3/4 and APOE4/4 groups. Specifically, HFD combined with 17βE2 resulted in increased changes in lipid amounts compared to HFD and vehicle treatment. We examined the overlap between these lipids changed in the APOE3/4 and APOE4/4 groups and found little commonalities (Figure 4E). The LION enrichment analysis showed that the lipids changed by 17βE2 in the presence of HFD in the APOE3/4 group are enriched for glycerophospholipids, glycerophosphocholines, membrane component, and others (Figure 4F). In the APOE4/4 group, lipids changed by 17βE2 in the presence of HFD are enriched for headgroup with neutral charge, triacylglycerols, lipid storage, and lipid droplet (Figure 4G), which is similar to the lipids changed by HFD alone (Figure 4D).
3.4 Effects of diet and 17β-estradiol on behavioral performance across APOE genotypes
At Week 14, all mice were examined behaviorally using the SAB, NOP, and NOR tasks, all of which involve aspects of hippocampal function (Lalonde, 2002; Hughes, 2004; Antunes and Biala, 2012). In SAB, only APOE3/3 mice showed significant behavioral effects of diet and 17βE2 treatment. Specifically, there was a significant diet X hormone interaction in which 17βE2 improved SAB performance with HFD exposure specifically in APOE3/3 mice, and the genotype was also associated with the largest HFD-associated impairment (Figure 5A).
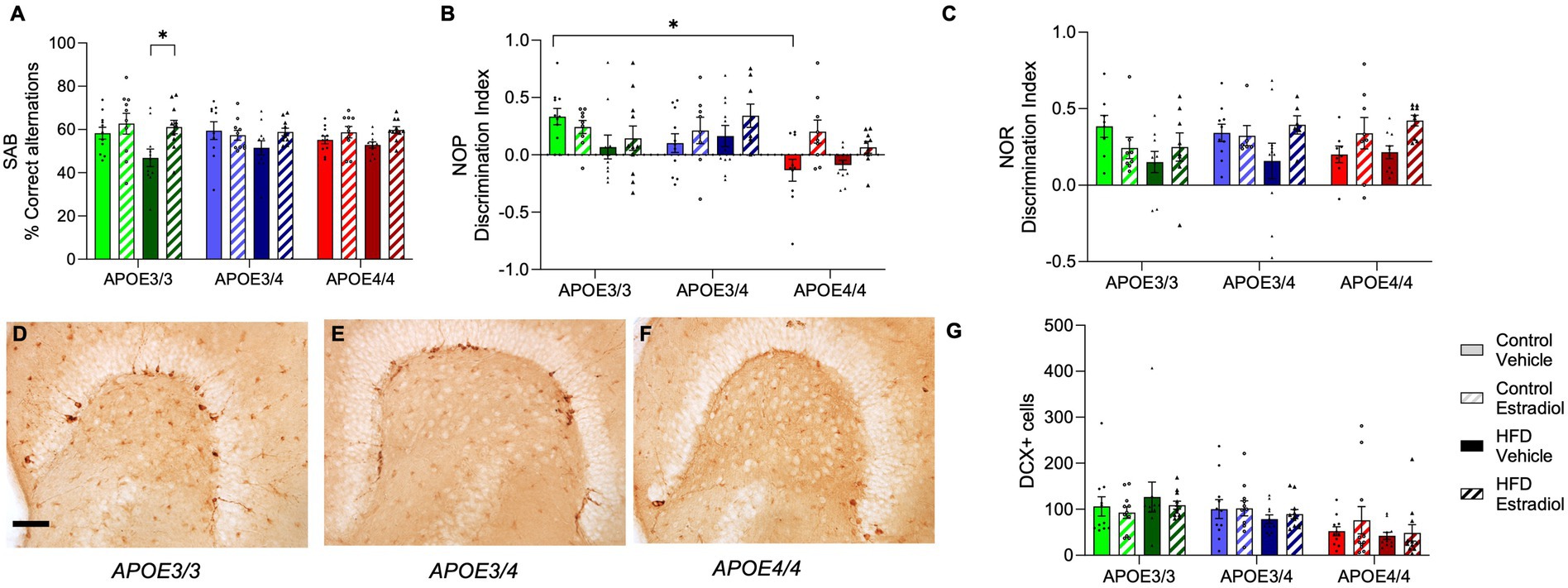
Figure 5. Effects of APOE genotype, HFD, and estradiol on behavior and neurogenesis. All behavior analyses were performed at Week 14. (A) Spontaneous alternation behavior (SAB) performance is shown as percent correct alternations. The results of (B) novel object placement (NOP) and (C) novel object recognition (NOR) are presented as the discrimination index. Representative images of doublecortin (DCX) immunostaining in the dentate gyrus from (D) APOE3/3 mice, (E) APOE3/4 mice, and (F) APOE4/4 mice. (G) Density of DCX-immunopositive cells was counted in the dentate gyrus. The scale bar is 100 μm in length. Bars colored green are used for APOE3/3 mice, blue for APOE3/4 mice, and red for APOE4/4 mice. Lighter shades represent the control diet, and darker shades represent HFD. The solid bars represent vehicle capsules, and the hatched bars represent 17β-estradiol capsules. All data are represented as the mean ± SEM with values shown for individual animals; n = 6–11 per group. Statistical significance with p < 0.05 is denoted by asterisks. Brackets indicate significant differences between specified groups.
For the NOP task, there were significant main effects of both APOE genotype and hormone treatment (Figure 5B). On the control diet, APOE4/4 mice performed significantly poorer than APOE3/3 mice with intermediate deficits observed in APOE3/4 mice. Treatment with 17βE2 significantly improved NOP performance with the strongest improvements in APOE4/4 mice and the weakest benefits in APOE3/3 mice (Figure 5B). Diet was not associated with significant main or interactive effects, but as in SAB, APOE3/3 mice trended toward showing the most HFD-associated impairment. Unlike NOP, NOR was not associated with a significant main effect of the APOE genotype. There was a significant interaction between diet and hormone treatment such that 17βE2 increased performance in the presence of HFD, an effect that trended toward stronger effects in APOE4 carriers (Figure 5C).
Because neurogenesis in the hippocampal subgranular zone is positively associated with cognitive behaviors (Aimone et al., 2014; Villeda et al., 2011) and 17βE2 (Sahab-Negah et al., 2020) but negatively impacted by APOE4 (Li et al., 2009) and HFD (Robison et al., 2020; Lindqvist et al., 2006), we determined whether differences in neurogenesis across groups may predict behavioral performances. Numbers of neural stem cells committed to neuronal differentiation were quantified by counts of DCX-positive cells in the dentate gyrus. There was a significant effect of the APOE genotype with APOE4/4 mice having fewer DCX-positive cells than APOE3/3 and APOE3/4 mice (Figures 5D–G). There were no significant main or interactive effects of diet or hormone treatment.
3.5 Microglia are differentially affected by diet and 17β-estradiol across APOE genotypes
Because microglial phenotypes are implicated in cognitive performance (McKee et al., 2023; Blank and Prinz, 2013) and are regulated by HFD (Alexaki, 2021), APOE4 genotype (Lee et al., 2023; Ferrari-Souza et al., 2023), and 17βE2 (Bruce-Keller et al., 2000; Mor et al., 1999), we next measured the numbers of total and morphologically activated microglia in the hippocampus. Interestingly, the number of microglia differed by both APOE genotype and estradiol treatment, with APOE4/4 increasing and 17βE2 treatment decreasing microglia density (Figure 6D). APOE3/4 mice showed an intermediate number of microglia and were not significantly different from APOE3/3 or APOE4/4 mice. Activation phenotype of microglia was measured by morphology as previously described (Moser and Pike, 2017; Christensen and Pike, 2019; Christensen and Pike, 2017; Ekdahl, 2012). Type 1 microglia (Figure 6A) have many long, thin ramified processes and are considered functionally homeostatic, whereas Types 2 (Figure 6B) and 3 (Figure 6C) have shorter and fewer processes, with Type 3 being associated with activated phenotypes. The microglial phenotype was significantly influenced by APOE genotype, diet, and hormones, with interactive effects of APOE and diet as well as diet and hormone (Figure 6E). Specifically, in mice maintained on the control diet, APOE genotype was significantly associated with microglial phenotypes with activation increasing in accordance with APOE4 allele dosage: APOE3/3 < APOE3/4 < APOE4/4. 17βE2 did not affect microglial activation under the control diet. HFD was associated with increased activated phenotypes, although the increase was only significantly different from the control diet in APOE3/3 mice. In HFD-fed animals, 17βE2 treatment decreased activation, with a significant effect observed in both APOE3/3 and APOE4/4 mice, although it was most robust in APOE3/3 mice (Figure 6E).
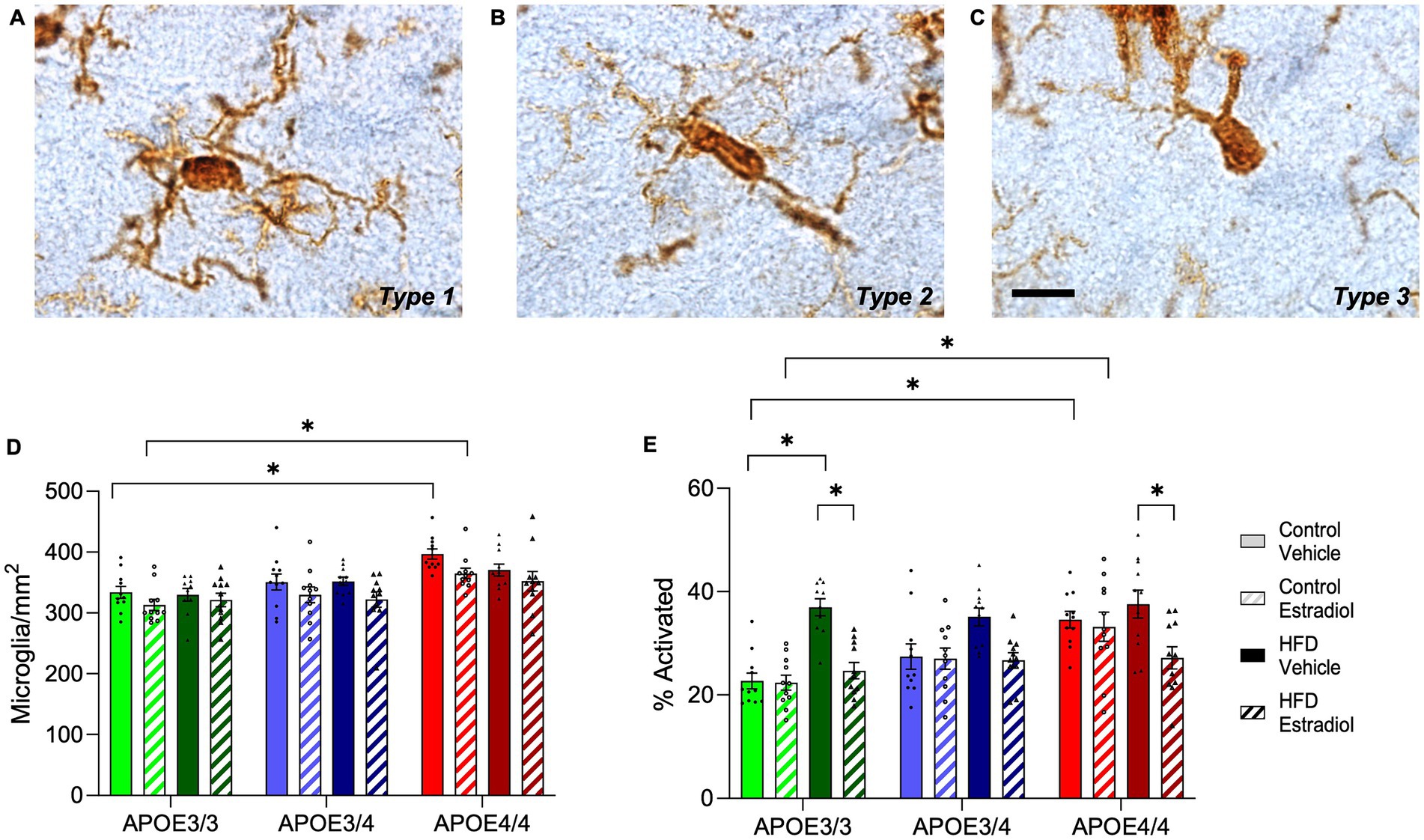
Figure 6. Effects of APOE genotype, HFD, and estradiol on microglia number and activation phenotype. Cells immunolabeled with Iba-1 were morphologically characterized as microglia with (A) type1 (homeostatic), (B) type 2 (activated), or (C) type 3 (activated) phenotypes. (D) The cell density and (E) percent activation of Iba-1 labeled microglia (relative abundance of types 2 and 3) were determined in the hippocampus. The scale bar is 10 μm in length. Bars colored green are used for APOE3/3 mice, blue for APOE3/4 mice, and red for APOE4/4 mice. Lighter shades represent control diet, and darker shades represent HFD. The solid bars represent vehicle capsules, and the hatched bars represent 17β-estradiol capsules. All data are represented as the mean ± SEM with values shown for individual animals; n = 10–11 per group. Statistical significance with p < 0.05 is denoted by asterisks. Brackets indicate significant differences between specified groups.
4 Discussion
In this study, we investigated the effects of the APOE4 genotype on systemic metabolic and CNS effects related to obesity in female mice, as well as the relative efficacy of treatment with the estrogen 17βE2. The design was planned to test the established protective effects of 17βE2 in the context of existing obesity and at an age just prior to the onset of reproductive senescence. The results suggest that for metabolic measures, 17βE2 is most effective in APOE4 homozygotes. Specifically, 17βE2 protected against HFD-induced increases in body weight, plasma leptin, and glucose intolerance most strongly in APOE4/4 mice. Conversely, 17βE2 was most effective in ameliorating the adverse neural effects of HFD in APOE3/3 mice, with significant improvements seen in both the SAB behavioral task and levels of microglial activation. However, the behavioral benefits of 17βE2 were task-dependent, with carriers of APOE4 but not APOE3/3 showing evidence of improved performance of the NOP and NOR tasks.
The impacts of APOE genotype on responses to obesity have been mixed in prior studies. In humans, evidence regarding the relationship between obesity and dementia or other neural risks is conflicting: some studies suggest these risks are greater in APOE4 carriers (Mole et al., 2020; Osiecka et al., 2023), while others indicate a more robust effect in APOE4 non-carriers (Coad et al., 2022; Shinohara et al., 2023; Zhao et al., 2023). The findings from rodent data are also contradictory, with evidence that either APOE3 (Jones et al., 2021; Pandit et al., 2024) or APOE4 (Johnson et al., 2017; Arbones-Mainar et al., 2008) is associated with greater obesity-induced impairments. Sex may be an important variable in this relationship (Moser and Pike, 2016; Mattar et al., 2022), a possibility that aligns with our prior observations in the EFAD mouse model (Moser and Pike, 2017; Christensen and Pike, 2019). In this study of female mice, obesity-induced metabolic effects were generally somewhat stronger with APOE4/4, whereas the neural effects tended to be more pronounced with APOE3/3. One limitation of our assessment of glucose metabolism is that we assessed glucose but not insulin tolerance, which would have provided a more complete understanding of metabolic responses to obesity. In terms of plasma lipids, HFD induced robust changes in lipid abundances across all groups. In agreement with the metabolic findings, lipidomics showed that APOE4 carriers had greater obesity-induced changes. One limitation is that lipidomic analyses did not assess potential changes in the brain. Collectively, these findings reinforce the position that the APOE4 genotype yields enhanced vulnerability to obesity.
Perhaps most interesting were the variable effects of APOE4 heterozygosity across different measures. As might be expected, APOE3/4 mice showed intermediate values relative to APOE3/3 and APOE4/4 mice on some measures, for example on levels of newborn neurons and microglial density and activation. However, on metabolic measures, APOE3/4 mice were generally more similar to APOE3/3 than APOE4/4 mice. For example, like APOE3/3 mice, APOE3/4 mice trended toward reduced adiponectin when challenged by HFD, whereas APOE4/4 showed no effect. In lipidomic analyses, both APOE3/4 and APOE4/4 mice showed more robust HFD-induced changes than APOE3/3, yet the pattern of alterations often differed between the APOE4 genotypes suggesting qualitative differences rather than just gene dosage effects. On the SAB task of attention and working memory, APOE3/4 mirrored APOE4/4 mice showing negligible effects of both HFD and 17βE2, whereas APOE3/3 showed an HFD-induced deficit that was rescued by 17βE2. On the NOP and NOR behavioral tests, APOE3/4 and APOE3/3 mice showed very similar performance levels under control diet conditions with APOE4/4 strongly trending toward comparatively impaired performance. Yet the significant diet X hormone interaction on NOP was driven by 17βE2-mediated improvements against HFD in APOE3/4 and APOE4/4 mice, with APOE3/3 mice showing minimal increases.
APOE4 allele number has significant effects on a range of outcomes in humans, though typically not in a linearly graded manner. For AD risk, the effects of APOE4/4 are several-fold higher than APOE3/4 (Reiman et al., 2020). Indeed, recent analyses have revealed near universal expression of AD neuropathology in APOE4 homozygotes by middle age (Fortea et al., 2024). In non-demented persons, APOE4 homozygosity significantly accelerates cognitive decline; however, the effects of APOE4 heterozygosity are comparatively modest (Gharbi-Meliani et al., 2021; Rawle et al., 2018; Caselli et al., 2011). APOE4 zygosity also impacts neuroimaging and plasma biomarkers of AD in the absence of dementia, with APOE3/4 carriers generally aligning more closely with APOE3/3 than APOE4/4 carriers (Tato-Fernandez et al., 2024). APOE4 zygosity also appears to impact the positive association between metabolic risk factors and a neuroimaging marker of neuroinflammation as APOE3/4 carriers are reported to differ from both APOE3/3 and APOE4/4 carriers (Ekblad et al., 2023). Notably, despite the significant differences with one vs. two copies of APOE4, most human studies of populations do not discriminate but rather pool homo- and heterozygous APOE4 genotypes into a combined group of APOE4 carriers.
Studies of rodents with knock-in of human APOE alleles are an excellent strategy to address existing knowledge gaps in the field. However, the issue of APOE4 homo- vs. heterozygosity is not well addressed in the preclinical literature. Few rodent studies have included APOE3/4 mice, with most comparing APOE3/3 vs. APOE4/4 mice. Among those that have considered APOE4 zygosity, there is not a consistent trend. One study of aged male and female APOE knock-in mice reported limited evidence of behavioral differences across APOE4 zygosity, in part due to high variability within groups (McLean et al., 2022). A recent targeted brain lipidome analysis in middle-aged male APOE knock-in mice reported differences across APOE3/3, APOE3/4, and APOE4/4 genotypes with APOE3/4 often positioned between the two homozygous groups (Miranda et al., 2022). In parallel to some of our observations, a cerebrocortical transcriptomic analysis found unique profiles in APOE3/4 mice (Foley et al., 2022). In the EFAD model that includes Alzheimer’s transgenes with APOE knock-in, females at age 6 months were ovariectomized and then studied with acute 17βE2 or vehicle treatments (Taxier et al., 2022). Improved memory on novel recognition tasks was observed in APOE3/3 and APOE3/4 but not APOE4/4 mice, suggesting an absence of 17βE2 neural benefits with APOE4 homozygosity. These results differ from our findings in the NOP and NOR behavioral tasks, which may reflect several factors including acute vs. chronic 17βE2 treatment and the presence vs. absence of underlying AD-related pathology. Interestingly, the findings of Taxier et al. align with our findings regarding the complex effects of APOE4 zygosity. They reported APOE3/4 outcomes varied between APOE3/3-like, intermediate, and APOE4/4-like values across different metrics of spine density (Taxier et al., 2022). Collectively, our findings add to a limited, but much needed, experimental literature aimed at understanding the differing effects of 0, 1, and 2 copies of APOE4.
Another interesting finding from our study concerned the effects of APOE genotype on microglia. We made the unexpected finding that, even without outside stressors, APOE4/4 was associated with a greater cell density of Iba-1 immunolabeled microglia and a higher proportion exhibiting a morphologically activated phenotype. To our knowledge, this finding has not been reported previously in males or females. APOE3/4 mice appeared to be intermediate and were not significantly different than mice with either homozygous genotype. These observations are consistent with findings from several groups indicating that, relative to APOE3/3, APOE4/4 microglia show a variety of transcriptomic and functional differences linked with poor neural outcomes (Liu et al., 2023; Lee et al., 2023; Machlovi et al., 2022; Stephen et al., 2019). Furthermore, in response to stressors ranging from demyelination (Wang et al., 2022) to systemic immune challenge (Zhu et al., 2012) and AD-related pathology (Rodriguez et al., 2014; Liu et al., 2017), the APOE genotype strongly impacts indices of microglia activation.
Importantly, we observed that treatment with 17βE2 was associated with reductions in both microglial numbers and levels of microglial activation regardless of APOE genotype. This finding is consistent with prior observations that estrogen treatments decrease inflammatory tone in the brain (Wu et al., 2016; Loiola et al., 2019). Given the noted variability in the effects of APOE genotype on numerous outcomes, it is perhaps not surprising that APOE-associated modulation on estrogen actions differs across studies. In experimental studies, there are reports that the beneficial effects of 17βE2 are alternatively better (Yun et al., 2007) or poorer (Taxier et al., 2022; Brown et al., 2008; Nathan et al., 2004) in the context of the APOE4 genotype. Furthermore, there is evidence that 17βE2 treatment increases β-amyloid deposition in APOE4 mice but improves outcomes in APOE2 and APOE3 mice (Kunzler et al., 2014). The current study finds that the relationship between 17βE2 efficacy and APOE genotype depends upon the outcome measure. For HFD-induced increases in body weight, plasma leptin, and glucose intolerance, 17βE2 was more beneficial in APOE4/4 mice. Conversely, APOE3/3 mice showed the most 17βE2-induced improvement in microglial measures and the SAB behavioral task. In lipidomic analyses, 17βE2 treatment had much greater effects in APOE4 carriers, with APOE3/4 mice showing profiles distinct from both APOE3/3 mice and APOE4/4 mice. Human studies on the efficacy of estrogen-based therapies are similarly mixed (Moser and Pike, 2016) with greater CNS benefits reported in APOE4 non-carriers (Yaffe et al., 2000; Burkhardt et al., 2004) or APOE4 carriers (Saleh et al., 2023; de Lange et al., 2020) across different studies and endpoints. Our study demonstrates that the impact of APOE4 carrier status depends upon both zygosity and the specific measure. We suggest that integrating the disparities in the human literature requires not only an acknowledgment of the complexities of the interactions between APOE genotype and 17βE2 on numerous cell types and systems throughout the body but also a consideration of APOE4 zygosity. While APOE4 is generally classified as deleterious and 17βE2 as beneficial, these are overly simplistic descriptions for factors known to exert pleiotropic actions (Patel et al., 2018; Martinez-Martinez et al., 2020).
In conclusion, this study suggests that APOE genotype, obesity, and estrogen signaling interact to affect cognitive, metabolic, and lipid outcomes. APOE4/4 carriers were more responsive to 17βE2 than APOE3/3 mice on metabolic measures after HFD, whereas APOE3/3 mice were more responsive to 17βE2 on CNS outcomes. Importantly, heterozygous APOE3/4 mice showed responses that varied between APOE3/3-like and APOE4/4-like to a unique APOE3/4 profile depending upon the outcome measure and the presence and absence of HFD and 17βE2. Given the relative rarity of APOE4 homozygosity in human populations, these results emphasize the limitation of relying on APOE4/4 mice as an experimental model. These data add to the limited but much-needed literature on understanding the effects of APOE4 allele dosage and its interactions with established modulators of systemic and CNS impairment.
Data availability statement
The raw data supporting the conclusions of this article will be made available by the authors, without undue reservation.
Ethics statement
The animal study was approved by University of Southern California Institutional Animal Care and Use Committee. The study was conducted in accordance with the local legislation and institutional requirements.
Author contributions
AC: Conceptualization, Investigation, Methodology, Supervision, Writing – review & editing, Data curation, Formal analysis, Visualization, Writing – original draft. CM: Methodology, Formal analysis, Writing – original draft, Writing – review & editing. WQ: Formal analysis, Investigation, Writing – review & editing. CP: Investigation, Writing – review & editing, Conceptualization, Funding acquisition, Methodology, Project administration, Resources, Supervision.
Funding
The author(s) declare that financial support was received for the research, authorship, and/or publication of this article. The study was supported by grants from the NIH/NIA (AG026572 and AG058068). CJM was supported by NIH/NIA grant AG084279. This content is solely the responsibility of the authors and does not necessarily represent the official views of the NIH/NIA.
Conflict of interest
The authors declare that the research was conducted in the absence of any commercial or financial relationships that could be construed as a potential conflict of interest.
The author(s) declared that they were an editorial board member of Frontiers, at the time of submission. This had no impact on the peer review process and the final decision.
Publisher’s note
All claims expressed in this article are solely those of the authors and do not necessarily represent those of their affiliated organizations, or those of the publisher, the editors and the reviewers. Any product that may be evaluated in this article, or claim that may be made by its manufacturer, is not guaranteed or endorsed by the publisher.
Supplementary material
The Supplementary material for this article can be found online at: https://www.frontiersin.org/articles/10.3389/fnagi.2024.1415072/full#supplementary-material
References
Aimone, J. B., Li, Y., Lee, S. W., Clemenson, G. D., Deng, W., and Gage, F. H. (2014). Regulation and function of adult neurogenesis: from genes to cognition. Physiol. Rev. 94, 991–1026. doi: 10.1152/physrev.00004.2014
Alexaki, V. I. (2021). The impact of obesity on microglial function: immune, metabolic and endocrine perspectives. Cells 10:1584. doi: 10.3390/cells10071584
Anagnostis, P., and Goulis, D. G. (2019). Menopause and its Cardiometabolic consequences: current perspectives. Curr. Vasc. Pharmacol. 17, 543–545. doi: 10.2174/1570161117999190228123237
Antunes, M., and Biala, G. (2012). The novel object recognition memory: neurobiology, test procedure, and its modifications. Cogn. Process. 13, 93–110. doi: 10.1007/s10339-011-0430-z
Arbones-Mainar, J. M., Johnson, L. A., Altenburg, M. K., and Maeda, N. (2008). Differential modulation of diet-induced obesity and adipocyte functionality by human apolipoprotein E3 and E4 in mice. Int. J. Obes. 32, 1595–1605. doi: 10.1038/ijo.2008.143
Arbones-Mainar, J. M., Johnson, L. A., Torres-Perez, E., Garcia, A. E., Perez-Diaz, S., Raber, J., et al. (2016). Metabolic shifts toward fatty-acid usage and increased thermogenesis are associated with impaired adipogenesis in mice expressing human APOE4. Int. J. Obes. 40, 1574–1581. doi: 10.1038/ijo.2016.93
Atzmon, G., Pollin, T. I., Crandall, J., Tanner, K., Schechter, C. B., Scherer, P. E., et al. (2008). Adiponectin levels and genotype: a potential regulator of life span in humans. J. Gerontol. A Biol. Sci. Med. Sci. 63, 447–453. doi: 10.1093/gerona/63.5.447
Besser, L. M., Gill, D. P., Monsell, S. E., Brenowitz, W., Meranus, D. H., Kukull, W., et al. (2014). Body mass index, weight change, and clinical progression in mild cognitive impairment and Alzheimer disease. Alzheimer Dis. Assoc. Disord. 28, 36–43. doi: 10.1097/WAD.0000000000000005
Blank, T., and Prinz, M. (2013). Microglia as modulators of cognition and neuropsychiatric disorders. Glia 61, 62–70. doi: 10.1002/glia.22372
Brown, C. M., Choi, E., Xu, Q., Vitek, M. P., and Colton, C. A. (2008). The APOE4 genotype alters the response of microglia and macrophages to 17beta-estradiol. Neurobiol. Aging 29, 1783–1794. doi: 10.1016/j.neurobiolaging.2007.04.018
Bruce-Keller, A. J., Keeling, J. L., Keller, J. N., Huang, F. F., Camondola, S., and Mattson, M. P. (2000). Antiinflammatory effects of estrogen on microglial activation. Endocrinology 141, 3646–3656. doi: 10.1210/endo.141.10.7693
Burkhardt, M. S., Foster, J. K., Laws, S. M., Baker, L. D., Craft, S., Gandy, S. E., et al. (2004). Oestrogen replacement therapy may improve memory functioning in the absence of APOE epsilon4. J. Alzheimers Dis. 6, 221–228. doi: 10.3233/jad-2004-6302
Caselli, R. J., Dueck, A. C., Locke, D. E. C., Hoffman-Snyder, C. R., Woodruff, B. K., Rapcsak, S. Z., et al. (2011). Longitudinal modeling of frontal cognition in APOE epsilon4 homozygotes, heterozygotes, and noncarriers. Neurology 76, 1383–1388. doi: 10.1212/WNL.0b013e3182167147
Christensen, A., Liu, J., and Pike, C. J. (2020). Aging reduces estradiol protection against neural but not metabolic effects of obesity in female 3xTg-AD mice. Front. Aging Neurosci. 12:113. doi: 10.3389/fnagi.2020.00113
Christensen, A., and Pike, C. J. (2017). Age-dependent regulation of obesity and Alzheimer-related outcomes by hormone therapy in female 3xTg-AD mice. PLoS One 12:e0178490. doi: 10.1371/journal.pone.0178490
Christensen, A., and Pike, C. J. (2018). TSPO ligand PK11195 improves Alzheimer-related outcomes in aged female 3xTg-AD mice. Neurosci. Lett. 683, 7–12. doi: 10.1016/j.neulet.2018.06.029
Christensen, A., and Pike, C. J. (2019). APOE genotype affects metabolic and Alzheimer-related outcomes induced by Western diet in female EFAD mice. FASEB J. 33, 4054–4066. doi: 10.1096/fj.201801756R
Coad, B. M., Ghomroudi, P. A., Sims, R., Aggleton, J. P., Vann, S. D., and Metzler-Baddeley, C. (2022). Apolipoprotein epsilon4 modifies obesity-related atrophy in the hippocampal formation of cognitively healthy adults. Neurobiol. Aging 113, 39–54. doi: 10.1016/j.neurobiolaging.2022.02.004
de Lange, A. G., Barth, C., Kaufmann, T., Maximov, I. I., van der Meer, D., Agartz, I., et al. (2020). Women’s brain aging: effects of sex-hormone exposure, pregnancies, and genetic risk for Alzheimer’s disease. Hum. Brain Mapp. 41, 5141–5150. doi: 10.1002/hbm.25180
Delano-Wood, L., Houston, W. S., Emond, J. A., Marchant, N. L., Salmon, D. P., Jeste, D. V., et al. (2008). APOE genotype predicts depression in women with Alzheimer’s disease: a retrospective study. Int. J. Geriatr. Psychiatry 23, 632–636. doi: 10.1002/gps.1953
Ekblad, L. L., Tuisku, J., Koivumaki, M., Helin, S., Rinne, J. O., and Snellman, A. (2023). Insulin resistance and body mass index are associated with TSPO PET in cognitively unimpaired elderly. J. Cereb. Blood Flow Metab. 43, 1588–1600. doi: 10.1177/0271678X231172519
Ekdahl, C. T. (2012). Microglial activation - tuning and pruning adult neurogenesis. Front. Pharmacol. 3:41. doi: 10.3389/fphar.2012.00041
El-Lebedy, D., Raslan, H. M., and Mohammed, A. M. (2016). Apolipoprotein E gene polymorphism and risk of type 2 diabetes and cardiovascular disease. Cardiovasc. Diabetol. 15, 12–11. doi: 10.1186/s12933-016-0329-1
Espeland, M. A., Yassine, H., Hayden, K. D., Hugenschmidt, C., Bennett, W. L., Chao, A., et al. (2021). Sex-related differences in cognitive trajectories in older individuals with type 2 diabetes and overweight or obesity. Alzheimers Dement. (N.Y.) 7:e12160. doi: 10.1002/trc2.12160
Ferrari-Souza, J. P., Lussier, F. Z., Leffa, D. T., Therriault, J., Tissot, C., Bellaver, B., et al. (2023). APOEepsilon4 associates with microglial activation independently of Abeta plaques and tau tangles. Sci. Adv. 9:eade1474. doi: 10.1126/sciadv.ade1474
Finch, C. E. (2014). The menopause and aging, a comparative perspective. J. Steroid Biochem. Mol. Biol. 142, 132–141. doi: 10.1016/j.jsbmb.2013.03.010
Foley, K. E., Hewes, A. A., Garceau, D. T., Kotredes, K. P., Carter, G. W., Sasner, M., et al. (2022). The APOE (epsilon3/epsilon4) genotype drives distinct gene signatures in the cortex of young mice. Front. Aging Neurosci. 14:838436. doi: 10.3389/fnagi.2022.838436
Fortea, J., Pegueroles, J., Alcolea, D., Belbin, O., Dols-Icardo, O., Vaque-Alcazar, L., et al. (2024). APOE4 homozygozity represents a distinct genetic form of Alzheimer’s disease. Nat. Med. 30, 1284–1291. doi: 10.1038/s41591-024-02931-w
Friedman, J. M. (2019). Leptin and the endocrine control of energy balance. Nat. Metab. 1, 754–764. doi: 10.1038/s42255-019-0095-y
Gharbi-Meliani, A., Dugravot, A., Sabia, S., Regy, M., Fayosse, A., Schnitzler, A., et al. (2021). The association of APOE epsilon4 with cognitive function over the adult life course and incidence of dementia: 20 years follow-up of the Whitehall II study. Alzheimers Res. Ther. 13:5. doi: 10.1186/s13195-020-00740-0
Hsieh, W., Williams, K. J., Su, B., and Bensinger, S. J. (2020). Profiling of mouse macrophage lipidome using direct infusion shotgun mass spectrometry. STAR Protoc. 2:100235. doi: 10.1016/j.xpro.2020.100235
Hughes, R. N. (2004). The value of spontaneous alternation behavior (SAB) as a test of retention in pharmacological investigations of memory. Neurosci. Biobehav. Rev. 28, 497–505. doi: 10.1016/j.neubiorev.2004.06.006
Jauhiainen, A., Madhu, B., Narita, M., Narita, M., Griffiths, J., and Tavare, S. (2014). Normalization of metabolomics data with applications to correlation maps. Bioinformatics 30, 2155–2161. doi: 10.1093/bioinformatics/btu175
Johnson, L. A., Torres, E. R. S., Impey, S., Stevens, J. F., and Raber, J. (2017). Apolipoprotein E4 and insulin resistance interact to impair cognition and Alter the epigenome and metabolome. Sci. Rep. 7:43701. doi: 10.1038/srep43701
Jones, N. S., and Rebeck, G. W. (2018). The synergistic effects of APOE genotype and obesity on Alzheimer’s disease risk. Int. J. Mol. Sci. 20:63. doi: 10.3390/ijms20010063
Jones, N. S., Watson, K. Q., and Rebeck, G. W. (2021). High-fat diet increases gliosis and immediate early gene expression in APOE3 mice, but not APOE4 mice. J. Neuroinflammation 18, 214–212. doi: 10.1186/s12974-021-02256-2
Koire, A., Joffe, H., and Buckley, R. (2022). Menopausal hormone therapy and the mind: the role of hormone replacement in the prevention and treatment of cognitive decline, dementia, and cognitive dysfunction of depression. Harv. Rev. Psychiatry 30, 215–225. doi: 10.1097/HRP.0000000000000339
Kunzler, J., Youmans, K. L., Yu, C., Ladu, M. J., and Tai, L. M. (2014). APOE modulates the effect of estrogen therapy on Abeta accumulation EFAD-Tg mice. Neurosci. Lett. 560, 131–136. doi: 10.1016/j.neulet.2013.12.032
Lalonde, R. (2002). The neurobiological basis of spontaneous alternation. Neurosci. Biobehav. Rev. 26, 91–104. doi: 10.1016/s0149-7634(01)00041-0
Lee, S., Devanney, N. A., Golden, L. R., Smith, C. T., Schwartz, J. L., Walsh, A. E., et al. (2023). APOE modulates microglial immunometabolism in response to age, amyloid pathology, and inflammatory challenge. Cell Rep. 42:112196. doi: 10.1016/j.celrep.2023.112196
Li, G., Bien-Ly, N., Andrews-Zwilling, Y., Xu, Q., Bernardo, A., Ring, K., et al. (2009). GABAergic interneuron dysfunction impairs hippocampal neurogenesis in adult apolipoprotein E4 knockin mice. Cell Stem Cell 5, 634–645. doi: 10.1016/j.stem.2009.10.015
Li, J., Ren, S., Piao, H., Wang, F., Yin, P., Xu, C., et al. (2016). Integration of lipidomics and transcriptomics unravels aberrant lipid metabolism and defines cholesteryl oleate as potential biomarker of prostate cancer. Sci. Rep. 6:20984. doi: 10.1038/srep20984
Lindqvist, A., Mohapel, P., Bouter, B., Frielingsdorf, H., Pizzo, D., Brundin, P., et al. (2006). High-fat diet impairs hippocampal neurogenesis in male rats. Eur. J. Neurol. 13, 1385–1388. doi: 10.1111/j.1468-1331.2006.01500.x
Liu, C., Wang, N., Chen, Y., Inoue, Y., Shue, F., Ren, Y., et al. (2023). Cell-autonomous effects of APOE4 in restricting microglial response in brain homeostasis and Alzheimer’s disease. Nat. Immunol. 24, 1854–1866. doi: 10.1038/s41590-023-01640-9
Liu, C., Zhao, N., Fu, Y., Wang, N., Linares, C., Tsai, C., et al. (2017). ApoE4 accelerates early seeding of amyloid pathology. Neuron 96, 1024–1032.e3. doi: 10.1016/j.neuron.2017.11.013
Loiola, R. A., Wickstead, E. S., Solito, E., and McArthur, S. (2019). Estrogen promotes pro-resolving microglial behavior and phagocytic cell clearance through the actions of Annexin A1. Front. Endocrinol. (Lausanne) 10:420. doi: 10.3389/fendo.2019.00420
Machlovi, S. I., Neuner, S. M., Hemmer, B. M., Khan, R., Liu, Y., Huang, M., et al. (2022). APOE4 confers transcriptomic and functional alterations to primary mouse microglia. Neurobiol. Dis. 164:105615. doi: 10.1016/j.nbd.2022.105615
Martens, Y. A., Zhao, N., Liu, C., Kanekiyo, T., Yang, A. J., Goate, A. M., et al. (2022). ApoE Cascade hypothesis in the pathogenesis of Alzheimer’s disease and related dementias. Neuron 110, 1304–1317. doi: 10.1016/j.neuron.2022.03.004
Martinez-Martinez, A. B., Torres-Perez, E., Devanney, N., Del Moral, R., Johnson, L. A., and Arbones-Mainar, J. M. (2020). Beyond the CNS: the many peripheral roles of APOE. Neurobiol. Dis. 138:104809. doi: 10.1016/j.nbd.2020.104809
Mattar, J. M., Majchrzak, M., Iannucci, J., Bartman, S., Robinson, J. K., and Grammas, P. (2022). Sex differences in metabolic indices and chronic Neuroinflammation in response to prolonged high-fat diet in ApoE4 Knock-in mice. Int. J. Mol. Sci. 23:3921. doi: 10.3390/ijms23073921
McKee, C. G., Hoffos, M., Vecchiarelli, H. A., and Tremblay, M. (2023). Microglia: a pharmacological target for the treatment of age-related cognitive decline and Alzheimer’s disease. Front. Pharmacol. 14:1125982. doi: 10.3389/fphar.2023.1125982
McLean, J. W., Bhattrai, A., Vitali, F., Raikes, A. C., Wiegand, J. L., and Brinton, R. D. (2022). Contributions of sex and genotype to exploratory behavior differences in an aged humanized APOE mouse model of late-onset Alzheimer’s disease. Learn. Mem. 29, 321–331. doi: 10.1101/lm.053588.122
Miranda, A. M., Ashok, A., Chan, R. B., Zhou, B., Xu, Y., McIntire, L. B., et al. (2022). Effects of APOE4 allelic dosage on lipidomic signatures in the entorhinal cortex of aged mice. Transl. Psychiatry 12, 129–126. doi: 10.1038/s41398-022-01881-6
Mole, J. P., Fasano, F., Evans, J., Sims, R., Kidd, E., Aggleton, J. P., et al. (2020). APOE-epsilon4-related differences in left thalamic microstructure in cognitively healthy adults. Sci. Rep. 10, 19787–19789. doi: 10.1038/s41598-020-75992-9
Molenaar, M. R., Jeucken, A., Wassenaar, T. A., van de Lest, C. H. A., Brouwers, J. F., and Helms, J. B. (2019). LION/web: a web-based ontology enrichment tool for lipidomic data analysis. Gigascience 8:giz061. doi: 10.1093/gigascience/giz061
Mor, G., Nilsen, J., Horvath, T., Bechmann, I., Brown, S., Garcia-Segura, L. M., et al. (1999). Estrogen and microglia: a regulatory system that affects the brain. J. Neurobiol. 40, 484–496. doi: 10.1002/(SICI)1097-4695(19990915)40:4<484::AID-NEU6>3.0.CO;2-C
Moser, V. A., and Pike, C. J. (2016). Obesity and sex interact in the regulation of Alzheimer’s disease. Neurosci. Biobehav. Rev. 67, 102–118. doi: 10.1016/j.neubiorev.2015.08.021
Moser, V. A., and Pike, C. J. (2017). Obesity accelerates Alzheimer-related pathology in APOE4 but not APOE3 mice. eNeuro 4:ENEURO.0077-17.2017. doi: 10.1523/ENEURO.0077-17.2017
Nathan, B. P., Barsukova, A. G., Shen, F., McAsey, M., and Struble, R. G. (2004). Estrogen facilitates neurite extension via apolipoprotein E in cultured adult mouse cortical neurons. Endocrinology 145, 3065–3073. doi: 10.1210/en.2003-1707
Nelson, J. F., Felicio, L. S., Randall, P. K., Sims, C., and Finch, C. E. (1982). A longitudinal study of estrous cyclicity in aging C57BL/6J mice: I. Cycle frequency, length and vaginal cytology. Biol. Reprod. 27, 327–339. doi: 10.1095/biolreprod27.2.327
Nerattini, M., Jett, S., Andy, C., Carlton, C., Zarate, C., Boneu, C., et al. (2023). Systematic review and meta-analysis of the effects of menopause hormone therapy on risk of Alzheimer’s disease and dementia. Front. Aging Neurosci. 15:1260427. doi: 10.3389/fnagi.2023.1260427
Nguyen, T. M. D. (2020). Adiponectin: role in physiology and pathophysiology. Int. J. Prev. Med. 11:136. doi: 10.4103/ijpvm.IJPVM_193_20
Norwitz, N. G., Saif, N., Ariza, I. E., and Isaacson, R. S. (2021). Precision nutrition for Alzheimer’s prevention in ApoE4 carriers. Nutrients 13:1362. doi: 10.3390/nu13041362
Osiecka, Z., Fausto, B. A., Gills, J. L., Sinha, N., Malin, S. K., and Gluck, M. A. (2023). Obesity reduces hippocampal structure and function in older African Americans with the APOE-epsilon4 Alzheimer’s disease risk allele. Front. Aging Neurosci. 15:1239727. doi: 10.3389/fnagi.2023.1239727
Palacios, S., Chedraui, P., Sanchez-Borrego, R., Coronado, P., and Nappi, R. E. (2024). Obesity and menopause. Gynecol. Endocrinol. 40:2312885. doi: 10.1080/09513590.2024.2312885
Pandit, H., Jones, N. S., and Rebeck, G. W. (2024). Obesity affects brain cortex gene expression in an APOE genotype and sex dependent manner. Int. J. Obes. 48, 841–848. doi: 10.1038/s41366-024-01481-y
Parhizkar, S., and Holtzman, D. M. (2022). APOE mediated neuroinflammation and neurodegeneration in Alzheimer’s disease. Semin. Immunol. 59:101594. doi: 10.1016/j.smim.2022.101594
Patel, S., Homaei, A., Raju, A. B., and Meher, B. R. (2018). Estrogen: the necessary evil for human health, and ways to tame it. Biomed. Pharmacother. 102, 403–411. doi: 10.1016/j.biopha.2018.03.078
Pike, C. J. (2017). Sex and the development of Alzheimer’s disease. J. Neurosci. Res. 95, 671–680. doi: 10.1002/jnr.23827
Rawle, M. J., Davis, D., Bendayan, R., Wong, A., Kuh, D., and Richards, M. (2018). Apolipoprotein-E (Apoe) epsilon4 and cognitive decline over the adult life course. Transl. Psychiatry 8:18. doi: 10.1038/s41398-017-0064-8
Reiman, E. M., Arboleda-Velasquez, J. F., Quiroz, Y. T., Huentelman, M. J., Beach, T. G., Caselli, R. J., et al. (2020). Exceptionally low likelihood of Alzheimer’s dementia in APOE2 homozygotes from a 5,000-person neuropathological study. Nat. Commun. 11, 667–668. doi: 10.1038/s41467-019-14279-8
Robison, L. S., Albert, N. M., Camargo, L. A., Anderson, B. M., Salinero, A. E., Riccio, D. A., et al. (2020). High-fat diet-induced obesity causes sex-specific deficits in adult hippocampal neurogenesis in mice. eNeuro 7:ENEURO.0391-19.2019. doi: 10.1523/ENEURO.0391-19.2019
Rodriguez, G. A., Tai, L. M., LaDu, M. J., and Rebeck, G. W. (2014). Human APOE4 increases microglia reactivity at Abeta plaques in a mouse model of Abeta deposition. J. Neuroinflammation 11:111. doi: 10.1186/1742-2094-11-111
Sahab-Negah, S., Hajali, V., Moradi, H. R., and Gorji, A. (2020). The impact of estradiol on neurogenesis and cognitive functions in Alzheimer’s disease. Cell. Mol. Neurobiol. 40, 283–299. doi: 10.1007/s10571-019-00733-0
Saleh, R. N. M., Hornberger, M., Ritchie, C. W., and Minihane, A. M. (2023). Hormone replacement therapy is associated with improved cognition and larger brain volumes in at-risk APOE4 women: results from the European prevention of Alzheimer’s disease (EPAD) cohort. Alzheimers Res. Ther. 15, 10–15. doi: 10.1186/s13195-022-01121-5
Serrano-Pozo, A., Das, S., and Hyman, B. T. (2021). APOE and Alzheimer’s disease: advances in genetics, pathophysiology, and therapeutic approaches. Lancet Neurol. 20, 68–80. doi: 10.1016/S1474-4422(20)30412-9
Shinohara, M., Gheni, G., Hitomi, J., Bu, G., and Sato, N. (2023). APOE genotypes modify the obesity paradox in dementia. J. Neurol. Neurosurg. Psychiatry 94, 670–680. doi: 10.1136/jnnp-2022-331034
Shinohara, M., Tashiro, Y., Suzuki, K., Fukumori, A., Bu, G., and Sato, N. (2020). Interaction between APOE genotype and diabetes in cognitive decline. Alzheimers Dement. (Amst.) 12:e12006. doi: 10.1002/dad2.12006
Stephen, T. L., Cacciottolo, M., Balu, D., Morgan, T. E., LaDu, M. J., Finch, C. E., et al. (2019). APOE genotype and sex affect microglial interactions with plaques in Alzheimer’s disease mice. Acta Neuropathol. Commun. 7:82. doi: 10.1186/s40478-019-0729-z
Su, B., Bettcher, L. F., Hsieh, W., Hornburg, D., Pearson, M. J., Blomberg, N., et al. (2021). A DMS shotgun Lipidomics workflow application to facilitate high-throughput, comprehensive Lipidomics. J. Am. Soc. Mass Spectrom. 32, 2655–2663. doi: 10.1021/jasms.1c00203
Takeuchi, H., Tomita, H., Browne, R., Taki, Y., Kikuchi, Y., Ono, C., et al. (2021). Sex-dependent effects of the APOE varepsilon4 allele on behavioral traits and white matter structures in young adults. Cereb. Cortex 31, 672–680. doi: 10.1093/cercor/bhaa251
Tato-Fernandez, C., Ekblad, L. L., Pietila, E., Saunavaara, V., Helin, S., Parkkola, R., et al. (2024). Cognitively healthy APOE4/4 carriers show white matter impairment associated with serum NfL and amyloid-PET. Neurobiol. Dis. 192:106439. doi: 10.1016/j.nbd.2024.106439
Taxier, L. R., Philippi, S. M., Fleischer, A. W., York, J. M., LaDu, M. J., and Frick, K. M. (2022). APOE4 homozygote females are resistant to the beneficial effects of 17beta-estradiol on memory and CA1 dendritic spine density in the EFAD mouse model of Alzheimer’s disease. Neurobiol. Aging 118, 13–24. doi: 10.1016/j.neurobiolaging.2022.06.005
Taxier, L. R., Philippi, S. M., York, J. M., LaDu, M. J., and Frick, K. M. (2022). The detrimental effects of APOE4 on risk for Alzheimer’s disease may result from altered dendritic spine density, synaptic proteins, and estrogen receptor alpha. Neurobiol. Aging 112, 74–86. doi: 10.1016/j.neurobiolaging.2021.12.006
Torres-Perez, E., Ledesma, M., Garcia-Sobreviela, M. P., Leon-Latre, M., and Arbones-Mainar, J. M. (2016). Apolipoprotein E4 association with metabolic syndrome depends on body fatness. Atherosclerosis 245, 35–42. doi: 10.1016/j.atherosclerosis.2015.11.029
Tzioras, M., Davies, C., Newman, A., Jackson, R., and Spires-Jones, T. (2019). Invited review: APOE at the interface of inflammation, neurodegeneration and pathological protein spread in Alzheimer’s disease. Neuropathol. Appl. Neurobiol. 45, 327–346. doi: 10.1111/nan.12529
Valencia-Olvera, A. C., Maldonado Weng, J., Christensen, A., LaDu, M. J., and Pike, C. J. (2023). Role of estrogen in women’s Alzheimer’s disease risk as modified by APOE. J. Neuroendocrinol. 35:e13209. doi: 10.1111/jne.13209
Villeda, S. A., Luo, J., Mosher, K. I., Zou, B., Britschgi, M., Bieri, G., et al. (2011). The ageing systemic milieu negatively regulates neurogenesis and cognitive function. Nature 477, 90–94. doi: 10.1038/nature10357
Wang, N., Wang, M., Jeevaratnam, S., Rosenberg, C., Ikezu, T. C., Shue, F., et al. (2022). Opposing effects of apoE2 and apoE4 on microglial activation and lipid metabolism in response to demyelination. Mol. Neurodegener. 17, 75–71. doi: 10.1186/s13024-022-00577-1
Whitmer, R. A., Gunderson, E. P., Barrett-Connor, E., Quesenberry, C. P. J., and Yaffe, K. (2005). Obesity in middle age and future risk of dementia: a 27 year longitudinal population based study. BMJ 330:1360. doi: 10.1136/bmj.38446.466238.E0
Wu, S., Chen, Y., Tsai, S., Wu, S., Shih, Y., Jiang-Shieh, Y., et al. (2016). Estrogen ameliorates microglial activation by inhibiting the Kir2.1 inward-rectifier K(+) channel. Sci. Rep. 6:22864. doi: 10.1038/srep22864
Yaffe, K., Haan, M., Byers, A., Tangen, C., and Kuller, L. (2000). Estrogen use, APOE, and cognitive decline: evidence of gene-environment interaction. Neurology 54, 1949–1954. doi: 10.1212/wnl.54.10.1949
Youmans, K. L., Tai, L. M., Nwabuisi-Heath, E., Jungbauer, L., Kanekiyo, T., Gan, M., et al. (2012). APOE4-specific changes in Abeta accumulation in a new transgenic mouse model of Alzheimer disease. J. Biol. Chem. 287, 41774–41786. doi: 10.1074/jbc.M112.407957
Yun, S. H., Park, K. A., Kwon, S., Woolley, C. S., Sullivan, P. M., Pasternak, J. F., et al. (2007). Estradiol enhances long term potentiation in hippocampal slices from aged apoE4-TR mice. Hippocampus 17, 1153–1157. doi: 10.1002/hipo.20357
Zade, D., Beiser, A., McGlinchey, R., Au, R., Seshadri, S., Palumbo, C., et al. (2013). Apolipoprotein epsilon 4 allele modifies waist-to-hip ratio effects on cognition and brain structure. J. Stroke Cerebrovasc. Dis. 22, 119–125. doi: 10.1016/j.jstrokecerebrovasdis.2011.06.020
Zhao, T., Zhong, T., Zhang, M., Xu, Y., Zhang, M., and Chen, L. (2023). Alzheimer’s disease: causal effect between obesity and APOE gene polymorphisms. Int. J. Mol. Sci. 24:13531. doi: 10.3390/ijms241713531
Keywords: APOE, estrogen, metabolic impairment, lipidomics, microglia, obesity
Citation: Christensen A, McGill CJ, Qian W and Pike CJ (2024) Effects of obesogenic diet and 17β-estradiol in female mice with APOE 3/3, 3/4, and 4/4 genotypes. Front. Aging Neurosci. 16:1415072. doi: 10.3389/fnagi.2024.1415072
Edited by:
G. William Rebeck, Georgetown University, United StatesReviewed by:
Liqin Zhao, University of Kansas, United StatesMoshe Levi, Georgetown University, United States
Copyright © 2024 Christensen, McGill, Qian and Pike. This is an open-access article distributed under the terms of the Creative Commons Attribution License (CC BY). The use, distribution or reproduction in other forums is permitted, provided the original author(s) and the copyright owner(s) are credited and that the original publication in this journal is cited, in accordance with accepted academic practice. No use, distribution or reproduction is permitted which does not comply with these terms.
*Correspondence: Christian J. Pike, Y2pwaWtlQHVzYy5lZHU=