- 1Department of Physiology and Biophysics, Dalhousie University, Halifax, NS, Canada
- 2Escuela de Química y Farmacia, Facultad de Farmacia, Universidad de Valparaíso, Valparaíso, Chile
- 3Department of Physiology and Pharmacology, Atrium Health Wake Forest Baptist Medical Center, Winston-Salem, NC, United States
- 4Division of Neuroscience, Oregon National Primate Research Center, Beaverton, OR, United States
- 5Division of Reproductive and Developmental Sciences, Oregon National Primate Research Center, Beaverton, OR, United States
- 6Department of Behavioral Neuroscience, Oregon Health and Science University, Portland, OR, United States
Introduction: The hippocampus is especially susceptible to age-associated neuronal pathologies, and there is concern that the age-associated rise in cortisol secretion from the adrenal gland may contribute to their etiology. Furthermore, because 11β-hydroxysteroid dehydrogenase type 1 (HSD11B1) catalyzes the reduction of cortisone to the active hormone cortisol, it is plausible that an increase in the expression of this enzyme enhances the deleterious impact of cortisol in the hippocampus and contributes to the neuronal pathologies that underlie cognitive decline in the elderly.
Methods: Rhesus macaques were used as a translational animal model of human aging, to examine age-related changes in gene and protein expressions of (HSD11B1/HSD11B1) in the hippocampus, a region of the brain that plays a crucial role in learning and memory.
Results: Older animals showed significantly (p < 0.01) higher base-line cortisol levels in the circulation. In addition, they showed significantly (p < 0.05) higher hippocampal expression of HSD11B1 but not NR3C1 and NR3C2 (i.e., two receptor-encoding genes through which cortisol exerts its physiological actions). A similar age-related significant (p < 0.05) increase in the expression of the HSD11B1 was revealed at the protein level by western blot analysis.
Discussion: The data suggest that an age-related increase in the expression of hippocampal HSD11B1 is likely to raise cortisol concentrations in this cognitive brain area, and thereby contribute to the etiology of neuropathologies that ultimately lead to neuronal loss and dementia. Targeting this enzyme pharmacologically may help to reduce the negative impact of elevated cortisol concentrations within glucocorticoid-sensitive brain areas and thereby afford neuronal protection.
1 Introduction
The hippocampus (HC) is particularly vulnerable to several age-related neuro-pathologies, including Alzheimer’s disease (AD) and limbic-predominant age-related TDP-43 encephalopathy (LATE) (Stonebarger et al., 2021; Nilaver and Urbanski, 2023). Although the root causes of these pathologies are unclear, there is evidence that glucocorticoids, such as cortisol, may potentiate neurotoxicity in this brain area and thereby contribute to their etiology (Lupien et al., 1998; Hibberd et al., 2000; McEwen, 2002; Herbert et al., 2006). Possible ways in which this potentiation could be achieved include an increase in circulating cortisol levels, derived from the adrenal gland, and/or an increase in the densities of neuronal glucocorticoid receptors (GRs) and mineralocorticoid receptors (MRs). However, an additional possibility involves increased local synthesis or reduced clearance of active glucocorticoids from the brain, stemming from an age-related increase in the expression of 11 beta-hydroxysteroid dehydrogenase type 1, which is encoded by HSD11B1 (Chapman et al., 2013). This enzyme is responsible for the conversion of cortisone, a less neurotoxic steroid, to cortisol. Therefore, an increase in the expression of HSD11B1 would potentiate the negative effects of chronically elevated cortisol concentrations in brain areas where it is expressed and where cortisol is known to act (Seckl et al., 2002; Holmes et al., 2003; Seckl, 2004; Holmes and Seckl, 2006). In support of this idea are several rodent studies showing that HSD11B1 can affect cortisol levels in the hippocampus and in turn impair cognitive function (Yau et al., 2006; MacLullich et al., 2012). On the other hand, very few studies have been performed in nonhuman primates to show if similar mechanisms are likely to operate in humans and contribute to age-associated brain pathologies in the elderly.
Like humans, rhesus macaques are long-lived primates and show similar brain organization and development. Furthermore, the brains of old rhesus macaques, like elderly humans, progressively show many of the same pathological hallmarks of Alzheimer’s disease, including Aβ plaques and phosphorylated Tau protein (Uno, 1993; Paspalas et al., 2018; Stonebarger et al., 2021; Appleman et al., 2024). Importantly, however, rhesus macaques can be maintained under tightly controlled environmental conditions, and with a minimal post-mortem interval for tissue collection. Consequently, they represent a valuable translational animal model for gaining insights into mechanisms that underlie human aging (Messaoudi et al., 2011; Stonebarger et al., 2021).
In support of a causal link between hippocampal HSD11B1 and cognition, we previously showed that hippocampal expression of this gene was significantly greater in old female rhesus macaques that performed significantly worse on a delayed matching-to-sample memory task (Sorwell et al., 2017) that involves the hippocampus (Bachevalier et al., 1991; Buccafusco et al., 2002; Aizawa et al., 2009). We, therefore, hypothesized that the expression of HSD11B1 should be significantly higher in older animals, both at the mRNA and at the protein level. To test this, we used RNA-seq to compare hippocampal expression of HSD11B1 as well as cortisol-sensitive receptors NR3C1 and NR3C2, in young and old male rhesus macaques. We also used western blot analysis to examine HSD11B1 protein expression in the same animals. The data suggest that an age-related increase in the expression of HSD11B1 in vulnerable brain areas may predispose them to the neurotoxic influence of high cortisol levels.
2 Materials and methods
2.1 Animals
Postmortem brain tissue was collected from 6 young adult (8–15 years) and 5 old (23–28 years) male rhesus macaques (Macaca mulatta) that had previously been used in an Institutional Animal Care and Use Committee approved study (Urbanski, 2017). The animals had been cared for at the Oregon National Primate Research Center (ONPRC) in accordance with the National Research Council’s Guide for the Care and Use of Laboratory Animals. They were housed indoors in a temperature-controlled environment (24°C) under a 12L:12D photoperiod (lights on from 07:00 to 19:00 h). Daily meals at ~08:00 h and ~ 15:00 h (LabDiet High Protein Monkey Chow, St. Louis, MO, United States) were supplemented with fresh fruits or vegetables; fresh drinking water was available ad libitum.
2.2 Collection of serum
Prior to necropsy, serial blood samples were collected hourly from 4 of the young and 4 of the old males, for 25 h, using a remote unobtrusive blood sampling system (Urbanski, 2011; Urbanski et al., 2014). The serum was assayed for cortisol using a chemiluminescence-based automatic clinical platform (Roche Cobas e411, Roche Diagnostics, Indianapolis, IN, United States), and the maximum and minimum values were calculated as the average of 5 consecutive peak concentrations and 5 consecutive nadir concentrations, respectively.
2.3 Collection of brain tissue
A detailed necropsy protocol previously used in our laboratory was used to systematically collect brain tissues from all the animals (Cervera-Juanes et al., 2022); other body tissues were made available to other investigators for unrelated postmortem studies. The animals were sedated with ketamine (10 mg/kg), and administered pentobarbital, followed by exsanguination, as recommended by the 2013 Edition of the American Veterinary Medical Association Guidelines for the Euthanasia of Animals. The brains were flushed with 0.9% saline and the right hemisphere was dissected to isolate the different brain regions, including the hippocampus, which was approached medially; the mid-body hippocampal subregion was subsequently used in this study. All tissues were wrapped in aluminum foil and immediately frozen in liquid nitrogen, and then archived at −80°C.
2.4 Tissue preparation for extraction
100–200 mg of hippocampal tissue was pulverized, using a tissue pulverizer (Thomas Scientific, Swedesboro, NJ, United States), and mixed. To maintain molecular integrity, while reducing degradation, the samples were maintained on dry-ice throughout the procedure. They were then stored in microcentrifuge tubes at −80°C.
2.5 RNA-seq
2.5.1 DNA/RNA isolation
Genomic DNA and RNA were extracted from the powdered hippocampal samples using the All-Prep DNA/RNA/miRNA Universal kit (Qiagen Sciences Inc., Germantown, MD, United States) following the manufacturer’s recommendations. Briefly, each brain region was pulverized and ~30 mg of tissue was used for DNA/RNA isolation. For stranded RNA-seq, cDNA libraries were prepared with the TruSeq stranded mRNA library prep Kit (cat# RS-122-2101, Illumina, San Diego, CA, United States). The resulting libraries were sequenced on a HiSeq 4000 (Genomics & Cell Characterization Core Facility, University of Oregon) using a paired-end run (2 × 150 bases). A minimum of 100 M reads was generated from each library.
Differential expression analysis: After verification of quality control of the sequences with FastQC (v. 0.1.1.2) (Andrews, 2010), sequences were aligned to the macaque Mmul_10 (INSDCA Assembly GCA_003339765.3) genome through the STAR alignment package (v. 2.7.3a) (Dobin et al., 2013). Read counts were obtained with STAR and based on the Ensembl.Mmul_10.100 genome annotation. edgeR (v. 3.28.0) (Robinson et al., 2010) from the Bioconductor package (Bioconductor, Cambridge, United Kingdom) were used for upper quartile normalization using the genes with a minimum of 0.3 CPM across samples. These thresholds translate into roughly 10 reads per minimum library size (10/minimum library size in millions) (Chen et al., 2016). Differential expression between the young and old groups was determined using edgeR’s Fisher’s exact test function, with the option of “tagwise” dispersion. The threshold for significance was unadjusted p = 0.05.
2.6 Western blot
2.6.1 Tissue protein extraction
Approximately 50 mg of powdered hippocampal tissue was homogenized in 1 mL of ice-cold RIPA buffer (Thermo Fisher Scientific, Rockford, IL, United States) using a glass Dounce homogenizer. Buffers contained HALT Protease Inhibitor Cocktail and HALT Phosphatase Inhibitor Cocktail (Thermo Fisher Scientific). After a 10 min centrifugation at 16000 g, the supernatant was immediately flash frozen and stored at −80°C. Protein concentration was determined using the Micro BCA protein Assay Kit (Thermo Fisher Scientific), following the manufacturer’s instructions.
2.6.2 Protein analysis
Protein lysates (100 μg) were subjected to non-reducing SDS-PAGE on 4–12% Bis-Tris polyacrylamide gels (Thermo Fisher Scientific), electro-transferred on polyvinylidene difluoride (PVDF) membranes (Thermo Fisher Scientific), blocked for 1 h at room temperature with a solution of 5% non-fat dry milk in Tris-Buffered Saline with Tween 20 (TBST) and probed overnight at 4°C in the presence of a rabbit polyclonal antibody directed against human HSD11B1 (Abcam AB39364) diluted 1:1000 in TBST, or GAPDH (Abcam, AB8245) at 1:10,000, a housekeeping protein, as a loading control. Molecular weights were estimated by running 7 μL of Seeblue Plus2 pre-stained protein ladder (LC5925, Thermo Fisher Scientific). For protein detection, we incubated the membranes in horseradish peroxidase-conjugated anti-rabbit secondary antibodies (Thermo Fisher Scientific) diluted 1:50,000 in TBST for 1 h at room temperature and West Dura chemiluminescence (Thermo Fisher Scientific). Chemiluminescence signal was detected and digitalized using a FluorChem System (Bio-Techne, Minneapolis, MN, United States).
2.6.3 De-glycosylation of protein extracts
To test whether the different molecular weights of HSD11B1 are due to glycosylation, we performed a de-glycosylation experiment using the glycoprotein de-glycosylation kit from MilliporeSigma (Burlington, MA, United States, catalog #362280). Briefly, 200 μg protein extracts were incubated with reaction buffer and 1 μL each of N-Glycosidase F, α2-3,6,8,9-Neuraminidase, Endo-α-N-acetylgalactosaminidase, β1,4-galactosidase and 1 μL β-N-Acetylglucosaminidase and incubated for 3 h at 37°C. Protein detection was performed by western blot as described above.
2.7 Statistics
Group means were compared using unpaired Student’s t-test, with p < 0.05 being considered statistically significant. The relationship between HSD11B1 mRNA and HSD11B1 protein concentrations was analyzed by Pearson’s correlation coefficient.
3 Results
In the present study we used a male rhesus macaque model to study the effect of normative aging on cortisol pathway dynamics, and focused on two cohorts of animals: A young group composed of 6 young adult (8–15 years) and an old group with 5 old (23–28 years) male rhesus macaques. To understand circulating cortisol dynamics, we collected hourly blood samples from 4 young and 4 old males for 25 h using a remote blood sampling system. While there was no statistically significant difference in average maximum cortisol levels, there was a significant (p < 0.01) increase in the average minimum circulating cortisol levels in the old group (Table 1). These results confirm that aging in rhesus macaques is associated with a dysregulation of the circadian control of cortisol, inducing an overall chronic increase of total cortisol exposure, which ultimately may prove to be neurotoxic in some brain areas.
Next, we studied gene expression in hippocampal samples to determine if the tissue cortisol pathway is affected during aging. While the mRNA expression of 11β-hydroxysteroid dehydrogenase type 2 (HSD11B2), the enzyme that catalyzes the conversion of cortisol into cortisone, was not detectable; the expression of 11β-hydroxysteroid dehydrogenase type 1 (HSD11B1) was, and moreover, it was significantly (p < 0.05) enhanced in the old animals (Figure 1A). No age-related changes in hippocampal expression of two cortisol receptors (NR3C1 and NR3C2) was detected (Figures 1B,C), suggesting that the neurons in this brain area retain their sensitivity to cortisol, even in old age.
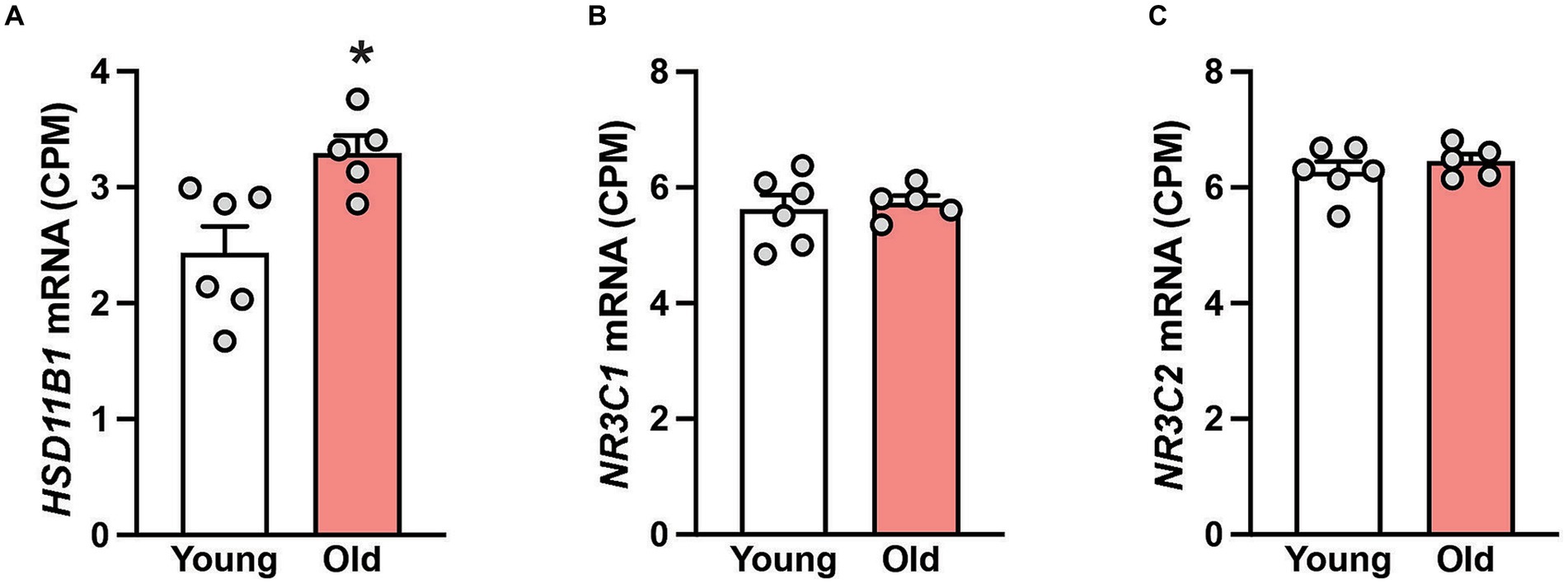
Figure 1. Gene expression in the hippocampus of 6 young and 5 old male rhesus macaques. Group means (+SEMs) are depicted for HSD11B1 (A), the glucocorticoid receptor NR3C1 (B) and the mineralocorticoid receptor NR3C2 (C). *p < 0.05 (Student’s t-test). The higher expression of HSD11B1 in the old animals implies enhanced conversion of cortisone to cortisol. The consistent expression of NR3C1 and NR3C2 across age suggests that hippocampal neurons retain their sensitivity to cortisol even in old age.
To further understand HSD11B1 dynamics in the hippocampus of aging animals, we used western blots to examine the age-related differences in HSD11B1 expression at the protein level. Because HSD11B1 protein is highly glycosylated in various tissues (Ozols, 1995; Tomlinson et al., 2004; Chapman et al., 2013; Gathercole et al., 2013), we validated our antibody in samples treated with de-glycosidases. While the expected molecular weight (MW) of HSD11B1 is 36 kDa, both kidney and hippocampal samples show several high molecular weight bands that either disappear or are reduced after de-glycosylation (Supplementary Figure S1). Next, we used the predominant high molecular weight bands to determine HSD11B1 protein levels in the hippocampus of young and old animals (Figure 2A). Hippocampal HSD11B1 protein levels were significantly (p < 0.05) elevated in old compared to young animals (Figure 2B; Supplementary Figure S2), and showed a significant (p < 0.05) positive correlation between mRNA and protein levels (Figure 2C). In summary, the results indicate that during normative aging there is not only increased overall circulating cortisol but also an imbalance in hippocampal cortisol clearance favoring increased tissue cortisol accumulation.
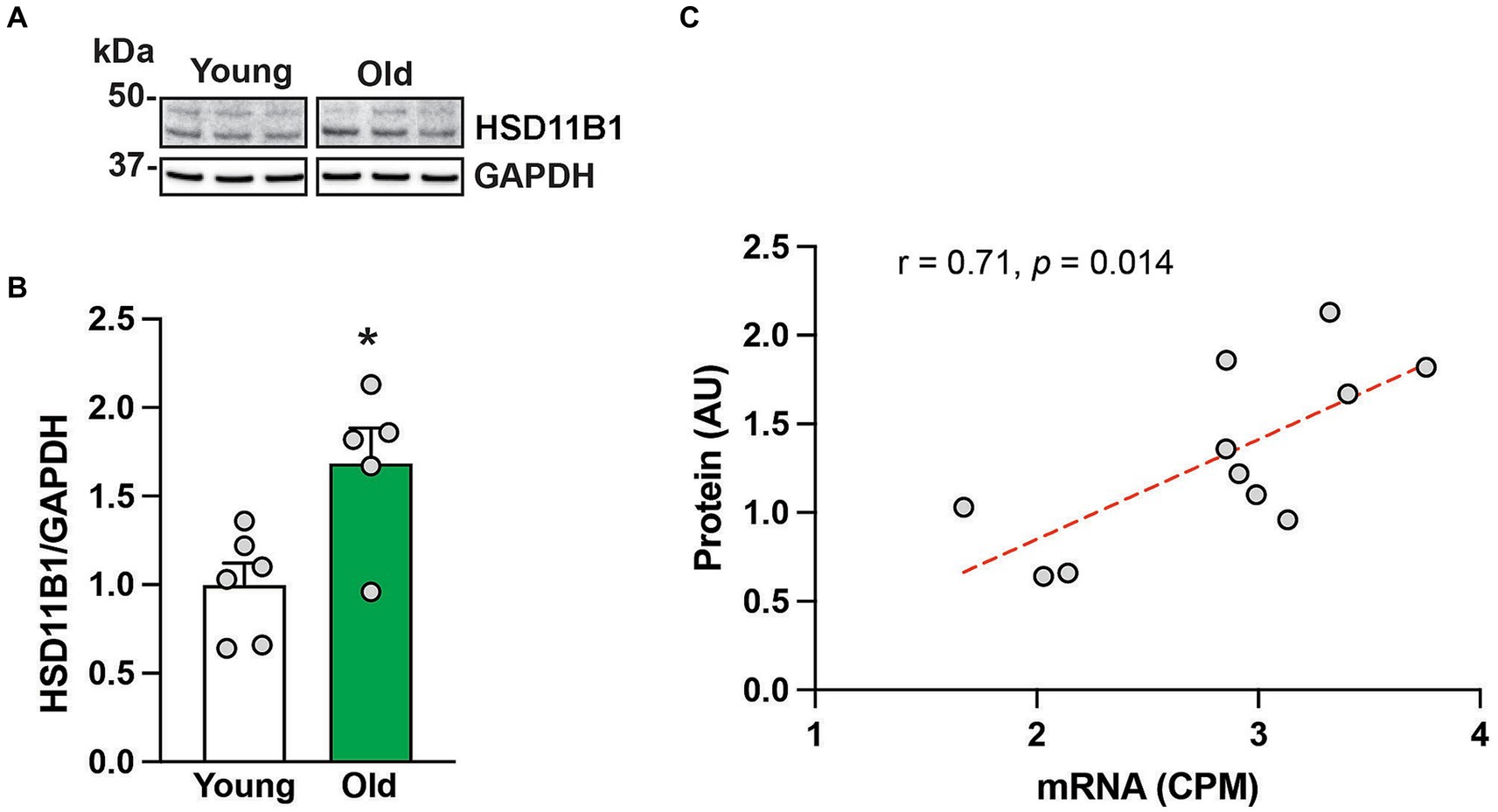
Figure 2. HSD11B protein expression in the hippocampus of 6 young and 5 old male rhesus macaques. Representative western blot images (A), and group means (+SEMs) (B). Correlation between HSD11B1 gene and HSD11B1 protein expression from the same animals (C). *p < 0.05 (Student’s t-test). The results show that HSD11B1 protein levels are higher in older animals and highly correlated with HSD11B1 mRNA levels, giving further support to the hypothesis that there is enhanced conversion of cortisone to cortisol within the aging hippocampus.
4 Discussion
Many hormones show a circadian pattern in the circulation, which helps to synchronize physiological functions and behaviors with the environment. In male rhesus macaques some of these hormones, including testosterone, dehydroepiandrosterone sulfate (DHEAS) and melatonin, show a significant age-related attenuation of their levels, which may represent a reduction in circadian co-ordination within the body and contribute to age-related disorders such as perturbed sleep–wake cycles (Downs et al., 2008; Urbanski and Sorwell, 2012; Aghazadeh-Sanai et al., 2017). To what extent this attenuation of circadian hormone levels is due to decreased secretion from endocrine organs and/or an increase in their degradation is unclear. However, it is also plausible that the age-related increase in body weight and blood volume that typically occurs in male, but not female, rhesus macaques also contributes to dilution of these hormones in the circulation. It is therefore surprising that cortisol, which also shows a robust circadian pattern in the circulation (Backlund et al., 2017), does not decrease with aging in males but instead increases significantly (Downs et al., 2008; Urbanski and Sorwell, 2012; Urbanski et al., 2014), and can become neurotoxic and impair memory (Lupien et al., 1998; Hibberd et al., 2000; McEwen, 2002; Herbert et al., 2006; Holmes et al., 2010; McEwen et al., 2015).
The cortisol data from the present study are in agreement with previous reports and emphasize a significant age-related increase in the nadir or baseline serum concentrations of cortisol (Downs et al., 2008; Urbanski and Sorwell, 2012). Because cortisol plays a key role in synchronizing peripheral circadian clocks, as well as influencing physiological functions, such as metabolism, immune response and behavior, chronic elevation of baseline cortisol levels could contribute to the etiology of a wide range of age-related pathologies.
Cortisol exerts its stimulatory actions through both glucocorticoid and mineralocorticoid receptors, and so it’s possible the cortisol-induced brain pathology stems not only from increased circulating cortisol levels but also from an increase in the number of the receptors. However, our RNA-seq results do not support this possibility. Neither the gene that encodes the lower affinity glucocorticoid receptor (NR3C1) nor the gene that encodes the higher affinity mineralocorticoid receptor (NR3C2) showed an age-related change in expression in the hippocampus. On the other hand, the RNA-seq results did show a significant age-related increase in HSD11B1 expression, which was also corroborated at the protein level. This enzyme plays a key role in converting cortisone, an inactive glucocorticoid, to cortisol. Therefore, increased HSD11B1 levels during aging would contribute to enhanced availability of cortisol within the hippocampus and thereby potentiate its neurotoxic potential. Importantly, HSD11B2, which encodes an enzyme that predominantly converts cortisol to cortisone was undetectable in the hippocampus, emphasizing that HSD11B1 plays the primary role in enzymatically modulating the availability of cortisol in this cognitive brain area.
There is mounting evidence for a pathological role of glucocorticoids and HSD11B1 in obesity and metabolic syndrome. Specifically, obesity is associated with increased HSD11B1 activity in subcutaneous adipose tissue resulting in increased cortisone to cortisol conversion (Stomby et al., 2014). This enzymatic conversion of cortisone to cortisol may therefore serve as an intracellular amplifier of local glucocorticoid activity and play a key role in finely regulating glucocorticoid levels in a tissue specific manner (Staab and Maser, 2010). Such findings have prompted research aimed at inhibiting HSD11B1 as a possible pharmacological target for the prevention of adverse metabolic profiles associated with glucocorticoid excess, such as Cushing syndrome, metabolic syndrome and obesity (Pereira et al., 2012; Morgan et al., 2014). Results from the current study suggest that an age-related increase in HSD11B1 expression may fulfill a similar regulatory role within the brain, and that pharmacological targeting of this enzyme may have therapeutic potential in reducing local deleterious effects associated with excessive cortisol levels.
In summary, the results from the present study are the first ones to demonstrate an age-related increase in hippocampal expression of HSD11B1 in a translational nonhuman primate species. Importantly, because of the many physiological, neuronal and endocrine similarities between rhesus macaques and humans (Messaoudi et al., 2011; Sorwell et al., 2012; Stonebarger et al., 2021), the results support the idea that an age-related increase in HSD11B1 expression in the hippocampus, together with elevated circulating cortisol levels, predispose this vulnerable brain area to neurotoxicity, cognitive decline and ultimately to development of dementia in the elderly. The studies also lay a foundation for pharmacological interventions that target HSD11B1, as a possible therapeutic for these age-related disorders (Sandeep et al., 2004; MacLullich et al., 2012; Sooy et al., 2015).
Data availability statement
The original contributions presented in the study are publicly available. This data can be found at: https://www.ncbi.nlm.nih.gov/geo/, GSE249907.
Ethics statement
The animal study was approved by ONPRC Institutional Animal Care and Use Committee. The study was conducted in accordance with the local legislation and institutional requirements.
Author contributions
AL: Methodology, Validation, Writing – original draft. SL: Methodology, Writing – review & editing. RC-J: Methodology, Writing – review & editing. M-LA: Writing – review & editing. SGK: Resources, Writing – review & editing. HFU: Conceptualization, Funding acquisition, Writing – original draft, Writing – review & editing.
Funding
The author(s) declare that financial support was received for the research, authorship, and/or publication of this article. This research was supported by National Institutes of Health Grants: P30 AG066518, P51 OD011092, and RF1 AG062220.
Conflict of interest
The authors declare that the research was conducted in the absence of any commercial or financial relationships that could be construed as a potential conflict of interest.
The author(s) declared that they were an editorial board member of Frontiers, at the time of submission. This had no impact on the peer review process and the final decision.
Publisher’s note
All claims expressed in this article are solely those of the authors and do not necessarily represent those of their affiliated organizations, or those of the publisher, the editors and the reviewers. Any product that may be evaluated in this article, or claim that may be made by its manufacturer, is not guaranteed or endorsed by the publisher.
Supplementary material
The Supplementary material for this article can be found online at: https://www.frontiersin.org/articles/10.3389/fnagi.2024.1328543/full#supplementary-material
References
Aghazadeh-Sanai, N., Downs, J. L., Mattison, J. A., Ingram, D. K., Kohama, S. G., and Urbanski, H. F. (2017). Effect of caloric restriction on plasma melatonin levels in aged rhesus macaques. Neurobiol. Aging 55, 213–216. doi: 10.1016/j.neurobiolaging.2017.03.032
Aizawa, K., Ageyama, N., Yokoyama, C., and Hisatsune, T. (2009). Age-dependent alteration in hippocampal neurogenesis correlates with learning performance of macaque monkeys. Exp. Anim. 58, 403–407. doi: 10.1538/expanim.58.403
Andrews, S. (2010). ASTQC. A quality control tool for high throughput sequence data. Available at: https://www.bibsonomy.org/bibtex/2b6052877491828ab53d3449be9b293b3/ozborn
Appleman, M. L., Nilaver, B. I., Weiss, A., Kohama, S. G., and Urbanski, H. F. (2024). Effect of hormone replacement therapy on amyloid beta (Aβ) plaque density in the rhesus macaque amygdala. Front. Aging Neurosci, 15, 1326747. doi: 10.3389/fnagi.2023.1326747
Bachevalier, J., Landis, L. S., Walker, L. C., Brickson, M., Mishkin, M., Price, D. L., et al. (1991). Aged monkeys exhibit behavioral deficits indicative of widespread cerebral dysfunction. Neurobiol. Aging 12, 99–111. doi: 10.1016/0197-4580(91)90048-o
Backlund, P. S., Urbanski, H. F., Doll, M., Hein, D. W., Bozinoski, M., Mason, C. E., et al. (2017). Daily rhythm in plasma N-acetyltryptamine. J. Biol. Rhythm. 32, 195–211. doi: 10.1177/0748730417700458
Buccafusco, J. J., Terry, A. V. Jr., and Murdoch, P. B. (2002). A computer-assisted cognitive test battery for aged monkeys. J. Mol. Neurosci. 19, 179–185. doi: 10.1007/s12031-002-0030-6
Cervera-Juanes, R., Darakjian, P., Ball, M., Kohama, S. G., and Urbanski, H. F. (2022). Effects of estradiol supplementation on the brain transcriptome of old rhesus macaques maintained on an obesogenic diet. Geroscience 44, 229–252. doi: 10.1007/s11357-021-00453-8
Chapman, K., Holmes, M., and Seckl, J. (2013). 11β-hydroxysteroid dehydrogenases: intracellular gate-keepers of tissue glucocorticoid action. Physiol. Rev. 93, 1139–1206. doi: 10.1152/physrev.00020.2012
Chen, Y., Lun, A. T., and Smyth, G. K. (2016). From reads to genes to pathways: differential expression analysis of RNA-Seq experiments using Rsubread and the edgeR quasi-likelihood pipeline. F1000Res. 5:1438. doi: 10.12688/f1000research.8987.2
Dobin, A., Davis, C. A., Schlesinger, F., Drenkow, J., Zaleski, C., Jha, S., et al. (2013). STAR: ultrafast universal RNA-seq aligner. Bioinformatics 29, 15–21. doi: 10.1093/bioinformatics/bts635
Downs, J. L., Mattison, J. A., Ingram, D. K., and Urbanski, H. F. (2008). Effect of age and caloric restriction on circadian adrenal steroid rhythms in rhesus macaques. Neurobiol. Aging 29, 1412–1422. doi: 10.1016/j.neurobiolaging.2007.03.011
Gathercole, L. L., Lavery, G. G., Morgan, S. A., Cooper, M. S., Sinclair, A. J., Tomlinson, J. W., et al. (2013). 11β-hydroxysteroid dehydrogenase 1: translational and therapeutic aspects. Endocr. Rev. 34, 525–555. doi: 10.1210/er.2012-1050
Herbert, J., Goodyer, I. M., Grossman, A. B., Hastings, M. H., de Kloet, E. R., Lightman, S. L., et al. (2006). Do corticosteroids damage the brain? J. Neuroendocrinol. 18, 393–411. doi: 10.1111/j.1365-2826.2006.01429.x
Hibberd, C., Yau, J. L., and Seckl, J. R. (2000). Glucocorticoids and the ageing hippocampus. J. Anat. 197, 553–562. doi: 10.1046/j.1469-7580.2000.19740553.x
Holmes, M. C., Carter, R. N., Noble, J., Chitnis, S., Dutia, A., Paterson, J. M., et al. (2010). 11β-hydroxysteroid dehydrogenase type 1 expression is increased in the aged mouse hippocampus and parietal cortex and causes memory impairments. J. Neurosci. 30, 6916–6920. doi: 10.1523/JNEUROSCI.0731-10.2010
Holmes, M. C., and Seckl, J. R. (2006). The role of 11β-hydroxysteroid dehydrogenases in the brain. Mol. Cell. Endocrinol. 248, 9–14. doi: 10.1016/j.mce.2005.12.002
Holmes, M. C., Yau, J. L., Kotelevtsev, Y., Mullins, J. J., and Seckl, J. R. (2003). 11β-hydroxysteroid dehydrogenases in the brain: two enzymes two roles. Ann. N. Y. Acad. Sci. 1007, 357–366. doi: 10.1196/annals.1286.035
Lupien, S. J., de Leon, M., de Santi, S., Convit, A., Tarshish, C., Nair, N. P., et al. (1998). Cortisol levels during human aging predict hippocampal atrophy and memory deficits. Nat. Neurosci. 1, 69–73. doi: 10.1038/271
MacLullich, A. M., Ferguson, K. J., Reid, L. M., Deary, I. J., Starr, J. M., Wardlaw, J. M., et al. (2012). 11β-hydroxysteroid dehydrogenase type 1, brain atrophy and cognitive decline. Neurobiol. Aging 33, 207.e1–207.e8. doi: 10.1016/j.neurobiolaging.2010.09.010
McEwen, B. S. (2002). Sex, stress and the hippocampus: allostasis, allostatic load and the aging process. Neurobiol. Aging 23, 921–939. doi: 10.1016/s0197-4580(02)00027-1
McEwen, B. S., Gray, J. D., and Nasca, C. (2015). 60 years of neuroendocrinology: redefining neuroendocrinology: stress, sex and cognitive and emotional regulation. J. Endocrinol. 226, T67–T83. doi: 10.1530/JOE-15-0121
Messaoudi, I., Urbanski, H. F., and Kohama, S. G. (2011). “Integrative models of aging in the nonhuman primate” in Monkeys: biology, behavior and disorders. eds. R. M. Williams and R. M. Williams (New York: Nova Science Publishers), 1–54.
Morgan, S. A., McCabe, E. L., Gathercole, L. L., Hassan-Smith, Z. K., Larner, D. P., Bujalska, I. J., et al. (2014). 11β-HSD1 is the major regulator of the tissue-specific effects of circulating glucocorticoid excess. Proc. Natl. Acad. Sci. U.S.A. 111, E2482–E2491. doi: 10.1073/pnas.1323681111
Nilaver, B. I., and Urbanski, H. F. (2023). Mechanisms underlying TDP-43 pathology and neurodegeneration: an updated mini-review. Front. Aging Neurosci. 15:1142617. doi: 10.3389/fnagi.2023.1142617
Ozols, J. (1995). Lumenal orientation and post-translational modifications of the liver microsomal 11 beta-hydroxysteroid dehydrogenase. J. Biol. Chem. 270, 2305–2312. doi: 10.1074/jbc.270.5.2305
Paspalas, C. D., Carlyle, B. C., Leslie, S., Preuss, T. M., Crimins, J. L., Huttner, A. J., et al. (2018). The aged rhesus macaque manifests Braak stage III/IV Alzheimer’s-like pathology. Alzheimers Dement. 14, 680–691. doi: 10.1016/j.jalz.2017.11.005
Pereira, C. D., Azevedo, I., Monteiro, R., and Martins, M. J. (2012). 11β-hydroxysteroid dehydrogenase type 1: relevance of its modulation in the pathophysiology of obesity, the metabolic syndrome and type 2 diabetes mellitus. Diabetes Obes. Metab. 14, 869–881. doi: 10.1111/j.1463-1326.2012.01582.x
Robinson, M. D., McCarthy, D. J., and Smyth, G. K. (2010). edgeR: a Bioconductor package for differential expression analysis of digital gene expression data. Bioinformatics 26, 139–140. doi: 10.1093/bioinformatics/btp616
Sandeep, T. C., Yau, J. L. W., MacLullich, A. M. J., Noble, J., Deary, I. J., Walker, B. R., et al. (2004). 11Beta-hydroxysteroid dehydrogenase inhibition improves cognitive function in healthy elderly men and type 2 diabetics. Proc. Natl. Acad. Sci. U.S.A. 101, 6734–6739. doi: 10.1073/pnas.0306996101
Seckl, J. R. (2004). 11β-hydroxysteroid dehydrogenases: changing glucocorticoid action. Curr. Opin. Pharmacol. 4, 597–602. doi: 10.1016/j.coph.2004.09.001
Seckl, J. R., Yau, J., and Holmes, M. (2002). 11β-hydroxysteroid dehydrogenases: a novel control of glucocorticoid action in the brain. Endocr. Res. 28, 701–707. doi: 10.1081/erc-120016990
Sooy, K., Noble, J., McBride, A., Binnie, M., Yau, J. L., Seckl, J. R., et al. (2015). Cognitive and disease-modifying effects of 11β-hydroxysteroid dehydrogenase type 1 inhibition in male Tg2576 mice, a model of Alzheimer’s disease. Endocrinology 156, 4592–4603. doi: 10.1210/en.2015-1395
Sorwell, K. G., Kohama, S. G., and Urbanski, H. F. (2012). Perimenopausal regulation of steroidogenesis in the nonhuman primate. Neurobiol. Aging 33, 1487.e1–1487.e13. doi: 10.1016/j.neurobiolaging.2011.05.004
Sorwell, K. G., Renner, L., Weiss, A. R., Neuringer, M., Kohama, S. G., and Urbanski, H. F. (2017). Cognition in aged rhesus macaques: effect of DHEA and correlation with steroidogenic gene expression. Genes Brain Behav. 16, 361–368. doi: 10.1111/gbb.12351
Staab, C. A., and Maser, E. (2010). 11beta-hydroxysteroid dehydrogenase type 1 is an important regulator at the interface of obesity and inflammation. J. Steroid Biochem. Mol. Biol. 119, 56–72. doi: 10.1016/j.jsbmb.2009.12.013
Stomby, A., Andrew, R., Walker, B. R., and Olsson, T. (2014). Tissue-specific dysregulation of cortisol regeneration by 11βHSD1 in obesity: has it promised too much? Diabetologia 57, 1100–1110. doi: 10.1007/s00125-014-3228-6
Stonebarger, G. A., Bimonte-Nelson, H. A., and Urbanski, H. F. (2021). The rhesus macaque as a translational model for neurodegeneration and Alzheimer’s disease. Front. Aging Neurosci. 13:734173. doi: 10.3389/fnagi.2021.734173
Tomlinson, J. W., Walker, E. A., Bujalska, I. J., Draper, N., Lavery, G. G., Cooper, M. S., et al. (2004). 11β-hydroxysteroid dehydrogenase type 1: a tissue-specific regulator of glucocorticoid response. Endocr. Rev. 25, 831–866. doi: 10.1210/er.2003-0031
Uno, H. (1993). The incidence of senile plaques and multiple infarction in aged macaque brain. Neurobiol. Aging 14, 673–674. doi: 10.1016/0197-4580(93)90067-l
Urbanski, H. F. (2011). “Circadian variation in the physiology and behavior of humans and nonhuman primates” in Animal models of behavioral analysis. ed. J. Raber (New York, NY: Humana Press Inc.), 217–235.
Urbanski, H. F. (2017). Effect of androgen supplementation on 24-hour activity-rest patterns of aged male rhesus macaques. Neurobiol. Aging 54, 100–102. doi: 10.1016/j.neurobiolaging.2017.02.020
Urbanski, H. F., and Sorwell, G. K. (2012). Age-related changes in neuroendocrine rhythmic function in the rhesus macaque. Age 34, 1111–1121. doi: 10.1007/s11357-011-9352-z
Urbanski, H. F., Sorwell, K. G., Garyfallou, V. T., Garten, J., Weiss, A., Renner, L., et al. (2014). Androgen supplementation during aging: development of a physiologically appropriate protocol. Rejuvenation Res. 17, 150–153. doi: 10.1089/rej.2013.1518
Keywords: cortisol, cortisone, HSD11B1, NR3C1, NR3C2, RNA-Seq
Citation: Lomniczi A, Luna SL, Cervera-Juanes R, Appleman M-L, Kohama SG and Urbanski HF (2024) Age-related increase in the expression of 11β-hydroxysteroid dehydrogenase type 1 in the hippocampus of male rhesus macaques. Front. Aging Neurosci. 16:1328543. doi: 10.3389/fnagi.2024.1328543
Edited by:
Puliyur MohanKumar, University of Georgia, United StatesReviewed by:
Roberto Cosimo Melcangi, University of Milan, ItalyAjeet Kumar, Washington University in St. Louis, United States
Copyright © 2024 Lomniczi, Luna, Cervera-Juanes, Appleman, Kohama and Urbanski. This is an open-access article distributed under the terms of the Creative Commons Attribution License (CC BY). The use, distribution or reproduction in other forums is permitted, provided the original author(s) and the copyright owner(s) are credited and that the original publication in this journal is cited, in accordance with accepted academic practice. No use, distribution or reproduction is permitted which does not comply with these terms.
*Correspondence: Henryk F. Urbanski, dXJiYW5za2lAb2hzdS5lZHU=