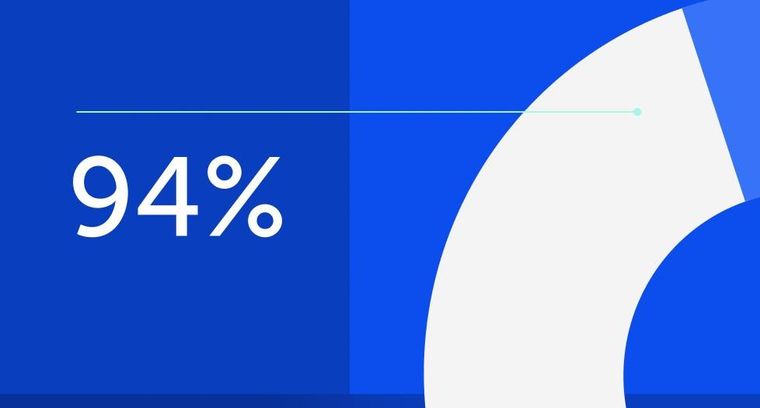
94% of researchers rate our articles as excellent or good
Learn more about the work of our research integrity team to safeguard the quality of each article we publish.
Find out more
REVIEW article
Front. Aging Neurosci., 19 June 2023
Sec. Neuroinflammation and Neuropathy
Volume 15 - 2023 | https://doi.org/10.3389/fnagi.2023.1184435
Neurodegenerative diseases (NDs) have a slow onset and are usually detected late during disease. NDs are often difficult to cure due to the presence of the blood–brain barrier (BBB), which makes it difficult to find effective treatments and drugs, causing great stress and financial burden to families and society. Currently, small extracellular vesicles (sEVs) are the most promising drug delivery systems (DDSs) for targeted delivery of molecules to specific sites in the brain as a therapeutic vehicle due to their low toxicity, low immunogenicity, high stability, high delivery efficiency, high biocompatibility and trans-BBB functionality. Here, we review the therapeutic application of sEVs in several NDs, including Alzheimer’s disease, Parkinson’s disease, and Huntington’s disease, discuss the current barriers associated with sEVs and brain-targeted DDS, and suggest future research directions.
Neurodegenerative diseases (NDs) are a group of chronic progressive diseases characterized by loss of neurons or myelin sheaths, mainly including Alzheimer’s disease (AD), Parkinson’s disease (PD), and Huntington’s disease (HD). The prevalence of NDs is closely related to age and has increased significantly with the accelerated aging of the population. The pathological and molecular mechanisms underlying these NDs are still unknown and need to be investigated. Furthermore, the blood–brain barrier (BBB) in the central nervous system (CNS), a highly selective and semi-permeable barrier, has created great challenges for treating and diagnosing NDs (Chen J. J. et al., 2019). Currently, an increasing number of diagnostic and therapeutic modalities are being used to explore this group of diseases. For example, Yu et al. (2020) found that cell-penetrating peptide (TAT) labeling of vasoactive intestinal peptide (VIP) could positively allosterically modulate the neuropeptide receptor PACAP type 1 receptor (PAC1) and enhance VIP neuroprotection in a mouse model of 1-methyl-4-phenyl-1,2,3,6-tetrahydropyridine (MPTP) in PD, which may be useful for developing therapeutic agents for ND. Moreover, Ren et al. (2013) found that implantation of mesenchymal stem cells (MSCs) with glial cell-derived neurotrophic factor (GDNF)-expressing MSCs into the striatum and substantia nigra improved motor function in PD monkeys, whereas transplantation of MSCs alone did not, suggesting that MSCs may be delivery vehicles for delivering GDNF to enhance nigrostriatal function. However, almost all large molecule drugs and >98% of small molecule drugs have failed in hybridizing the BBB, with candidates exhibiting poor biopharmaceutical and pharmacokinetic properties (Zhang et al., 2016). Drug delivery systems (DDSs) are formulations or technologies that enhance the safety and efficacy of drugs by controlling the rate, timing, and location of drug release in vivo. In clinical practice, DDS can be used to achieve precise controlled release of drugs, improve drug targeting and water solubility, regulate drug metabolism time, and promote drug bio-barrier penetration and absorption (Vader et al., 2016). Therefore, DDS are considered an effective treatment approach for these NDs, and suitable DDS that do not interfere with healthy organs and tissues are urgently needed to distribute drug molecules.
In the past decades, many researchers have explored DDS for the treatment of NDs, which include liposomes (Qu et al., 2018a) polymeric nanoparticles (PNPs) (Zhao Y. et al., 2020), solid lipid nanoparticles (SLNs) (Dal Magro et al., 2017), nanogels (Neamtu et al., 2017), and nanoemulsions (Zhao et al., 2018). Indeed, Qu et al. (2018a) designed a brain-targeted DDS using a 29-amino acid peptide (RVG29) from rabies virus glycoprotein (RVG) as a targeting ligand and found that RVG29-lip showed significantly higher uptake efficiency in mouse brain endothelial and dopaminergic cells and penetrated the BBB in vitro with high efficiency, effectively treating PD. Moreover, Zhao Y. et al. (2020) used poly(ethylene glycol)-co-poly(ε-caprolactone) (PEG-PCL) nanoparticles to encapsulate ginkgolide B (GB) prior to slow release into the bloodstream to treat PD. Although some of these DDS have been used clinically, there are still some problems, such as high toxicity (Niu et al., 2019), high immunogenicity (Chu et al., 2020), poor stability (Frank, 1993; Chen Z. J. et al., 2020), and low delivery efficiency (Prikhozhdenko et al., 2021). Small extracellular vesicles (sEVs) are considered the key to solving these problems and represent the most promising DDS. The reader is referred to the many previous reviews about the advantages and disadvantages of these DDS (Table 1).
Extracellular vesicles (EVs) are a heterogeneous group of cell-derived membrane structures that are found in biological fluids and are involved in various physiological and pathological processes. EVs are now considered as an additional mechanism for intercellular communication, which allow cells to exchange proteins, lipids, and genetic material (van Niel et al., 2018). EVs can be classified in many ways, but depending on their size, biological properties, biogenesis, and release process, they can be broadly classified into three categories: sEVs, microvesicles, and apoptotic vesicles. sEVs are generally 50–150 nm in diameter. Membrane invaginations of vesicles can form multivesicular bodies (MVBs), some of which can fuse with the plasma membrane and release internal vesicles to form sEVs by exocytosis, whereas other MVBs are degraded by lysosomes. Microvesicles have a diameter of 50–500 nm, even up to 1 μm, and they are derived directly from the cell membrane through budding, whereas apoptotic vesicles are usually produced by apoptotic cells and are approximately 1–5 μm in diameter (van Niel et al., 2018; Han et al., 2022). Additionally, EVs are heterogeneous in terms of content, with sEVs and microvesicles known to contain mRNA, miRNA, non-coding RNAs, and cytoplasmic and membrane proteins, whereas apoptotic vesicles contain nuclear fractions and cell organelles (Abdik et al., 2019). Figure 1 shows a schematic diagram of the three vesicle release methods.
Figure 1. Extracellular vesicles secreted by cells mainly include three types: small extracellular vesicles, microvesicles, and apoptotic vesicles. Small extracellular vesicles are formed by the endosomal pathway of cells. Microvesicles are larger than small extracellular vesicles and result from the formation of outward buds in the plasma membrane. Apoptotic vesicles are bubble-like bodies produced during apoptosis.
Due to their natural origin, EVs are inherently highly biocompatible, with enhanced stability and limited immunogenicity, which offers potential advantages over traditional drug delivery vehicles such as liposomes and nanoparticles (Meng et al., 2020). Therefore, EVs are also considered as DDS (Elsharkasy et al., 2020). However, the DDS in this paper refers mainly to sEVs rather than microvesicles and apoptotic vesicles, the physicochemical properties of which make them unsuitable as DDS (De Jong and Borm, 2008). sEVs were selected as DDS for three reasons: first, sEVs carry and protect a wide range of biomolecules and can deliver them to recipient cells; second, they can cross the BBB and are distributed with some stability in the circulation, thus reaching different organs; and third, they can be designed to optimize delivery to certain tissues and have a high level of biocompatibility (Elsharkasy et al., 2020). Therefore, we focused on the application of sEVs as DDS, the biochemical properties of sEVs, and the applications of modified sEVs. sEVs can transfer proteins and genetic material in both directions between neurons and glial cells. Such cell-cell interactions contribute to the pathological progression and spread of NDs (Pinnell et al., 2021). Hence, we also discuss recent research advances in the use of sEVs for therapy of NDs, the limitations of sEVs as a DDS for the treatment of NDs, and the main directions for future research.
The production of sEVs involves dual invasion of the endosomal pathway and the plasma membrane (Kalluri and LeBleu, 2020). First, membrane proteins and extracellular soluble molecules invade the plasma membrane to form early sorting endosomes (ESEs), which then continue to mature to form late sorting endosomes (LSEs). Next, the LSE membrane invaginates to produce intracellular MVBs containing intraluminal vesicles (ILVs) (Li M. et al., 2022). MVBs have two main fates: they are fused by autophagosomes or lysosomes before being degraded and then recycled by the cell, or they are translocated to the plasma membrane, where they fuse with the plasma membrane and release ILVs via cytosolic vesicles, termed sEVs (Figure 2; Kalluri and LeBleu, 2020; Teng and Fussenegger, 2020; Gurung et al., 2021).
Figure 2. Schematic diagram of the biogenesis, secretion, and structural composition of small extracellular vesicles. The plasma membrane invaginates to form early sorting endosomes, which undergo continuous maturation to form late sorting endosomes and eventually increase multivesicular bodies (MVBs). MVBs have two main fates: they are fused by autophagosomes or lysosomes, or they fuse with the plasma membrane to release the contained intraluminal vesicles (ILVs) as small extracellular vesicles. Small extracellular vesicle structures contain proteins, lipids, and nucleic acids.
Small extracellular vesicles are composed mainly of lipids, nucleic acids, and proteins. sEVs have a lipid bilayer composed mainly of plasma membrane lipids, such as sphingomyelin (SM), desaturated phosphatidylethanolamine, phosphatidylserine (PS), desaturated phosphatidylcholine (PC), cholesterol (CHOL), GM3, and ganglioside (Subra et al., 2007; Lu et al., 2022). The lipid bilayer serves a protective and drug-carrying function, with sphingomyelin and cholesterol adding stiffness to maintain the structural stability of sEVs (Kooijmans et al., 2012). The nucleic acid component of sEVs consists mainly of DNA, mRNA, miRNA, and other non-coding RNAs that mediate intercellular communication and regulate cellular function (Men et al., 2019). sEV proteins include constitutive proteins such as tetraspanin proteins (e.g., CD9 and CD63), which can be used to identify sEVs, and cell type-specific proteins, such as histocompatibility complex (MHC) class II expressed on MHC class II cells (Thery et al., 2002). High expression of transmembrane proteins (especially CD9, CD63, and CD81) and miRNAs (e.g., miR-124, miR-132, and miR-212) confirm the targeting function of sEVs (Song et al., 2019). For example, Zhdanova et al. (2021) found labeled sEVs in the hippocampus and neocortex after intranasal administration of sEVs expressing the typical markers CD9, CD63, and CD81 in a mouse model of AD. Lee et al. (2017) injected sEVs showing high expression of miR-219 into the striatum of R6/2 transgenic HD mice, which reduced expression of the target gene RE1-silencing transcription factor, demonstrating the targeted delivery of miR-219 based on sEVs.
The isolation and purification of sEVs as a carrier for DDS is critical. Common separation methods include ultracentrifugation, density gradient centrifugation, ultrafiltration, volume exclusion chromatography (SEC), immunoaffinity capture, and polymer precipitation (Li C. et al., 2022); the advantages and disadvantages of these methods are listed in Table 2. In addition, numerous researchers have explored the combination of several different separation methods. For example, Shu et al. (2020) combined ultrafiltration and SEC in cell culture media and found that their combination produced up to 58-fold more sEVs than ultracentrifugation alone. Koh et al. (2018) also found that sEVs were more efficiently enriched by combining the use of ultracentrifugation and SEC compared to the two methods alone. Although more than two separation methods increase the yield of sEVs to some extent, they can greatly increase the experimental time, which warrants the development of more effective separation methods. In an attempt to do so, Visan et al. (2022) found that tangential flow filtration reduced the time consumption for separating sEVs before SEC and greatly improved the recovery of sEVs compared to ultracentrifugation. Furthermore, Zhao et al. (2016) developed a microfluidic device (sEVsDEP chip)-based method for the separation and detection of sEVs, enabling microsphere-mediated dielectrophoretic separation and immunoaffinity detection. This approach allows the integration of different functions into the chip, such as downstream analysis to detect the main components of sEVs (Zhao et al., 2016). Additionally, microfluidic chips have the advantage of continuous separation and automation. Although many methods have been established for isolating and purifying sEVs, the main problem faced at this stage is the lack of standardized techniques for separating sEVs that preserve their structural and functional integrity, and the need to select different separation methods for different purposes and applications or develop other approaches. With the development of scientific technology, we believe that more applicable devices will appear in the future and/or researchers will format a new guideline when sEVs have been studied in depth.
The characterization of sEVs is essential for understanding the characteristics, structure, and function of sEVs as they affect drug loading and drug delivery (Kimiz-Gebologlu and Oncel, 2022). Numerous methods are available to verify the size, concentration, and purity of sEVs. The Minimal Information for Study of Extracellular Vesicles (MISEV) 2018 guidelines recommend the following steps for characterization: evaluation of sEV markers using two techniques (e.g., electron microscopy/imaging and nanoparticle tracking analysis/dynamic light scattering) and the identification of contaminants in sEV preparations by western blotting or flow cytometry, and the quantification of sEV preparation (e.g., protein concentration to particle concentration ratio) (Thery et al., 2018).
The MISEV2018 guidelines recommended that the characterization of sEVs by protein require at least three positive protein markers, including at least one transmembrane/lipid binding protein and at least one negative protein marker (Thery et al., 2018). Although protein blotting is a simple and widespread method for the analysis of sEVs, it has limited specificity, lacks multiplicity, offers only a small amount of information for a large number of sEV proteins, and does not exclude contaminants from other vesicles (Doyle and Wang, 2019; Zhang et al., 2019). To analyze the concentration and size of sEVs, one can choose between nanoparticle tracking analysis (NTA) and tunable resistive pulse sensing (van der Pol et al., 2014; Kurtjak et al., 2022). However, these methods cannot distinguish among EVs, protein aggregates, and lipoproteins, so not all small particles are quantified. Recently, a microstructured fiber-assisted nanoparticle tracking analysis (FaNTA) has been developed, which allows for the separation of all types of nano-objects less than 20 nm in diameter by recording the ultra-long trajectories of rapidly diffusing polydisperse nanoparticles, greatly improving the criteria for accurate characterization of monodisperse and polydisperse nanoparticle samples (Nissen et al., 2022). Dynamic light scattering (DLS) can be used to determine the size distribution of EVs, but cannot distinguish between EVs, protein lipoproteins, or other particulate matter (Haney et al., 2015). Electron microscopy enables visualization of sEVs to accurately assess sEV morphology and quality, as well as any other co-isolated contaminants such as proteins, DNA, lipoprotein, or viruses (Noble et al., 2020). Flow cytometry can also be used to analyze sEVs but it requires a separate suspension of particles to detect sEVs, which can lead to inaccurate data when the concentration of sEVs is too high or when sEVs are clustered together (Szatanek et al., 2017; Doyle and Wang, 2019). Nanoflow cytometry techniques have also been developed, which analyze individual sEVs with improved resolution and allow for multi-parameter measurements (Padda et al., 2019). Nanoflow cytometry shows great promise in the analysis of sEVs, but is still in the developmental stage and appropriate instrumentation, antibodies, and controls must be used to ensure accurate sEV detection (Morales-Kastresana et al., 2019).
Additionally, there are several ways to characterize sEVs. Smith et al. (2015) used Raman spectroscopy, a technique based on the illumination of a sample by a laser, to study the chemical composition of individual sEVs. Moreover, Zhao et al. (2016) developed a microfluidic epifluorescence search chip that is easy to handle and detects three sEV tumor markers (CA-125, EpCAM, and CD24). This design allows the detection of sEVs at a limit of 750 particles/μl, which is 1,000 times more sensitive than conventional methods such as protein blotting (Zhao et al., 2016).
Although the standard indications for the storage of biological samples or sEVs are no longer provided in the MISEV2018 guidelines (Thery et al., 2018), improper storage methods can affect the concentration, physical properties, and function of sEVs (Wu et al., 2021; Gelibter et al., 2022). Therefore, it is both important and necessary to explore more appropriate storage conditions. For example, at present, sEVs are commonly stored in phosphate buffer (PBS) at −80°C without freeze-drying or spray-drying (Wu et al., 2021). Gelibter et al. (2022) conducted three sets of experiments: in the first set of experiments, they proved that under −80°C storage conditions, the concentration and purity of sEVs decreased continuously with increasing time, the particle size increased, and the Zeta potential changed; in the second set of experiments, they found that either a fast or slow freeze–thaw cycling mode resulted in a gradual increase in particle size in a cycle-dependent manner; and in the third set of experiments, they confirmed that the freeze–thaw process resulted in the rupture of the sEVs films, which were subsequently re-gelatinized into new particles (Gelibter et al., 2022). Gorgens et al. (2022) also confirmed that the −80°C storage method in PBS is suboptimal, and instead found that PBS supplemented with human albumin and trehalose (PBS-HAT) significantly improved the short- and long-term preservation of sEVs in samples stored at −80°C, maintaining stability over multiple freeze–thaw cycles.
Therefore, fresh sEVs should be used whenever possible, but if long-term storage is required, repeated freeze–thawing of samples must be avoided, and storage in PBS-HAT is preferred.
To date, numerous researchers have explored sEV-based DDS for therapeutic purposes. For example, Sun et al. (2010) found that sEV-containing curcumin enhanced the anti-inflammatory activity of curcumin by intraperitoneal injection in a lipopolysaccharide (LPS)-induced septic shock mouse model, which may be attributed to the increased stability and concentration of curcumin in the blood by sEVs. Lai et al. (2020) demonstrated that peripherally injected sEVs can deliver miRNAs to the brains of mice with subarachnoid hemorrhage (SAH), the underlying mechanism of which is regulated by neuroinflammatory regulation. Thomas et al. (2021) assembled WNT3a on sEVs, which actively penetrated intact cartilage for efficient delivery into cartilage, contributing to the healing of osteochondral defects for therapeutic purposes.
The properties of sEVs make them ideal for drug delivery; however, how to effectively load drugs into sEVs is a challenge that remains to be addressed for targeted therapeutic applications. For this purpose, researchers have established many platforms, including incubation, electroporation, ultrasound treatment, extrusion, freeze–thaw cycling, and saponins (Table 3). For example, Sun et al. (2010) found that sEV-containing curcumin, which was obtained by incubating sEVs with curcumin, ameliorated LPS-induced septic shock and suggested that this method can be used to elevate the concentration of curcumin in sEVs. Yan et al. (2022) successfully encapsulated miR-31-5p mimics into milk-derived sEVs by electroporation and demonstrated that miR-31-5p loaded into sEVs achieved higher cellular uptake and was degradation-resistant. Hajipour et al. (2021) used ultrasound treatment to effectively (40.55 ± 4.21%) load human chorionic gonadotropin into sEVs isolated from uterine fluid. It is important to note that the results of drug delivery studies are difficult to generalize because the results are highly dependent on sEVs sources, isolation techniques, therapeutic agents, and drug delivery protocols (Walker et al., 2019). A study has been found that electroporation and transfection of sEVs to load the short interfering RNA (siRNA) results in the disruption of sEVs, precipitation of siRNA on membranes, and a loss of cargo delivery capability (McCann et al., 2022). Recently, a new technique was described to integrate siRNA sequences into a Dicer-independent pre-miRNA stem loop (pre-miR-451), using a miRNA sorting mechanism that improves the loading efficiency of sEVs. The therapeutic dose required by this siRNA delivery method is at least ten times less than that required for siRNA delivered via lipid nanoparticles, greatly reducing the possible toxicity (Reshke et al., 2020). This suggests that effective drug packaging has a multiplier effect on therapy. Furthermore, putting the desired small-molecule or nucleic-acid drugs into sEVs in a way that does not disrupt the cell membrane or the biological activity of the cargo is the main challenge at hand. Meanwhile, common sEVs loading strategies often result in low loading efficiency (<30%) compared to synthetic nanomedicines (Guo et al., 2021). Kooijmans et al. (2013) suggested that when electroporated, siRNA forms extensive aggregates and has a substantial retention rate of less than 0.05% in sEVs. To avoid this, Guo et al. (2021) developed a facile magnetic extrusion method for the preparation of endosome-derived vesicles. The high yield and consistency of this method somewhat helped to overcome the difficulties faced when sEVs were loaded.
How to prolong the residence time in circulation and increase sEVs targeting ability are remaining challenges to be addressed (Xu and Xu, 2021). The intact transmembrane proteins (CD81 and CD9) and integrins (CD51 and CD61) in sEVs are thought to have some homing and targeting functions, but their targeting ability remains too weak for application (Fitzner et al., 2011). Furthermore, some components of naturally isolated sEVs are not essential for drug delivery, and synthetic therapeutic biomaterials (e.g., modified and synthetic sEVs) are necessary for a more pure and efficient characterization of nanocarriers (Garcia-Manrique et al., 2018; Claridge et al., 2021). Hence, modification of sEVs is essential for their biodistribution, half-life, ability to target specific cells, and therapeutic potential (Man et al., 2020). Various engineering approaches can be used to modify homing peptides or ligands on the surface of sEVs, which can confer targeting capabilities to sEVs and thus increase their therapeutic efficiency (Luarte et al., 2016; Xu et al., 2021). Modification strategies include genetic engineering and chemical modifications (Alvarez-Erviti et al., 2011). For example, researchers have found that the RVG peptide (TIWMPENPRPGTPCDIFTNSRGKRASNG) selectively binds to acetylcholine receptors and can be used to develop neuro-specific sEVs for drug delivery to the CNS (El-Andaloussi et al., 2012). Moreover, Yu et al. (2021) used genetic engineering techniques to target donor cells to the RVG peptide of α7-nAChR and added highly specific variants capable of degrading amyloid beta (Aβ) to the surface of sEVs. Didiot et al. (2016) demonstrated that sEVs loaded with hydrophobically modified small interfering RNA (hsiRNA) targeting Huntington protein mRNA were effectively internalized by mouse primary cortical neurons and promoted dose-dependent silencing of Huntington mRNA and protein. Cui et al. (2019) constructed RVG-coupled sEVs derived from MSCs by chemical coupling. They found that this approach enhanced the binding capacity of sEVs to the cortical and hippocampal region of the AD mouse model and prevented memory deficits by inactivating astrocytes and balancing the inflammatory response.
In addition, sEVs may have some risks as drug carriers for ND. Indeed, some studies have shown that sEVs accelerate the fibrosis of proteins such as a-synuclein and Aβ, depending on the composition of the lipids (Grey et al., 2015; Stuendl et al., 2016; Lindberg et al., 2017). Furthermore, Grey et al. (2015) used mass spectrometry to identify several phospholipids in sEVs, including phosphatidylserine, phosphatidylcholine, phosphatidylinositol, phosphatidylethanolamine, and the gangliosides GM2 and GM3. Vesicles containing ganglioside GM1 or GM3 were found to accelerate α-synuclein aggregation, and α-synuclein is highly sensitive to pH. At the resting state, α-synuclein aggregation is very slow at neutral pH, while at weakly acidic pH (<6), α-synuclein aggregation is significantly accelerated. Therefore, we believe that caution should be taken to maintain a neutral pH when using sEVs as drug carriers for treating ND.
Although these studies have explored the use of sEVs as DDS, sEVs still cannot meet the demand as DDS. Indeed, sEV-based drug delivery lacks appropriate guidelines pertaining to isolation and purification; in addition, there are deficiencies in the perception of sEVs as a DDS mechanism, inadequate clinical-grade production, and the fact that cell types that could be ideal sources of drug-delivery-grade sEVs have not been identified (Meng et al., 2020). Furthermore, a protein corona is formed by the adsorption of serum proteins onto vesicles during circulation, limiting vesicle migration and affecting DDS targeting. It has been shown that the formation of a protein corona alters the physicochemical properties of vesicles, giving them biological properties that differ from their synthetic properties (Walkey and Chan, 2012; Hadjidemetriou et al., 2015; Skliar et al., 2018; Toth et al., 2021). Therefore, in the future, nanomedicines must be designed that are either completely resistant to plasma components or promote the binding of specific plasma components, thereby improving circulatory or specific targeting properties, respectively.
The route of administration also has an important impact on the biodistribution, therapeutic efficacy, and short/long-term biological effects of sEVs. Currently, the main methods of administration for treating ND include intravenous injection, nasal administration, oral administration, and stereotactic injection (Xu et al., 2021). The first three modalities have high patient compliance, whereas stereotactic injections are often not accepted by the general public because of their invasive nature. In this regard, several researchers have also conducted relevant studies. For example, Cui et al. (2019) found that sEVs could be tracked in the brain by intravenous injection and significantly improve learning and memory capacity, reduce plaque deposition and Aβ levels, and normalize inflammatory cytokine levels. Peng et al. (2022) designed a nanocarrier with a targeting function that combines therapeutic MSC-derived sEVs with curcumin to target drug transport into the cytoplasm of target cells after intranasal administration, thereby significantly improving the motor and coordination abilities of PD model mice. Although oral administration is also well accepted by the public, it is rarely used in research because of the first-pass effect, the enzymatic disruption of the structural integrity of sEVs, and the difficulty of diffusing sEVs to the brain (Agrawal et al., 2017). Here, we propose a scenario: if peptides capable of targeting the brain and sEVs are coupled to make emulsifiers, could the drawbacks be solved and used in clinical applications? Aqil et al. (2017) developed sEVs containing curcumin by loading curcumin into milk-derived sEVs. sEVs curcumin increased the drug content by 3–5 times compared with free curcumin when administered orally. Recently, there have been some reports on sEVs of plant origin. For example, some researchers have reported that broccoli-derived EVs have therapeutic potential due to their ability to transport exogenous miRNA (Del Pozo-Acebo et al., 2022). However, it has only been possible to demonstrate that miRNA can be taken up by human colorectal adenocarcinoma cells (Caco-2) in vitro, and whether EV uptake is altered upon digestion when cells are exposed to digested EVs is not yet known and requires further study (Del Pozo-Acebo et al., 2022). Nonetheless, it cannot be denied that this study holds great promise for pharmacological applications. Interestingly, Zhao P. et al. (2020) found that the amount of indocyanine green (ICG) PLGA nanoparticles in the brain following subcutaneous injection was more than 44-fold higher than the intravenous route. Additionally, Nazimek et al. (2020) compared the therapeutic effects of sEVs administrated via intravenous (iv), intraperitoneal (ip), intradermal (id), and oral administration on delayed-type hypersensitivity (DTH) induced by ovalbumin, and found that the strongest effect was observed with orally administered sEVs. This result partially reflects the importance of the route of administration.
Although these routes of administration have been studied, some shortcomings still need to be addressed. For example, the half-life index of sEVs with intravenous administration is too short and they are readily cleared by macrophages (Liu et al., 2017). Nasal administration also has some disadvantages including relatively small doses, limited olfactory epithelial surface area, the short retention time for drug absorption, and its effect on nasal secretions (Erdo et al., 2018). The choice of a more appropriate route of drug delivery still needs to be explored.
Numerous articles have shown that neuronal cells in the CNS regulate the function of each other by releasing large amounts of sEVs (Fruhbeis et al., 2012; Sharma et al., 2019). These suggests that sEVs may play an important role to maintain the communication and homeostasis in the CNS. For example, sEVs are involved in controlling neurogenesis (Sharma et al., 2019), regulating myelin biogenesis (Bakhti et al., 2011), and repairing damaged neurons (Pei et al., 2019). Fruhbeis et al. (2013) found that activity-dependent release of the neurotransmitter glutamate triggered the secretion of oligodendrocyte-derived sEVs and that neurons could internalize these sEVs via endocytosis to improve neuronal viability under cellular stress. This suggests that oligodendrocyte-derived sEVs are involved in a novel mode of bidirectional neuron-glia communication that contributes to neuronal integrity. Wang et al. (2011) suggested that synaptophysin, a glycoprotein associated with astrocyte sEVs, may play a role in mediating neuroprotection and stimulating axonal growth. In addition, a number of articles have shown that sEVs can have a neuroprotective effect. For example, Haney et al. (2015) administrated sEVs-containing peroxidase to the brain of the PD mouse model and found that these sEVs could protect SNpc neurons from acute oxidative stress. Kalani et al. (2016) found that embryonic stem cell-derived sEVs loaded with curcumin could also induce neuroprotective effects in an ischemia-reperfusion injury model. Similarly, sEVs secreted by adipose-derived stem cells are neuroprotective. In AD, sEVs secreted by adipose-derived MSCs have potent neuroprotective effects against Aβ-induced neuronal toxicity, reducing neuronal damage, and promoting neurogenesis (Ma et al., 2020). Adipose-derived stem cells secreting sEVs improved motor performance and reduced glial cell activation in an animal model of amyotrophic lateral sclerosis (ALS) (Bonafede et al., 2020). Although sEVs are involved in neuroprotective processes, they are also involved in the pathogenesis of CNS disease (Venturini et al., 2019). For example, sEVs delivered some pathogenic proteins such as Aβ, α-synuclein, and mutant Huntington’s protein (mHTT) into healthy neuronal cells and induced dysfunction of receipt cells (Eitan et al., 2016; Stuendl et al., 2016; Xia et al., 2022). Therefore, it is important to fully understand the role of sEVs in the physiology and pathology of CNS. In parallel, these studies also indicated that sEVs can be used to treatment of NDD by regulating the biosynthesis, released or modified the contents in sEVs with gene editing.
Due to the presence of the BBB and the complexity of the CNS, treatment of ND often requires consideration of whether the drug can effectively reach the brain tissue (Figure 3). As sEVs have the advantages of low immunogenicity and stable and long distance transport, they are considered the most promising tool for ND treatment at present (Loch-Neckel et al., 2022). sEVs also have great potential as non-invasive diagnostic biomarkers for ND and have been extensively reported in the literature. However, the current review focuses on the therapeutic application of sEVs rather than their diagnostic potential. The following sections focus on treatment methods that use sEVs loaded with therapeutic ingredients for ND.
Figure 3. Schematic diagram of the clinical application of small extracellular vesicles as a drug delivery system in neurodegenerative diseases. Small extracellular vesicles have greater advantages as drug delivery systems due to their biological properties, which protect their contents from phagocytosis, and their ability to cross the blood–brain barrier. Small extracellular vesicles are isolated by ultracentrifugation, density gradient centrifugation, and other methods. Small extracellular vesicles can be targeted to cross the blood–brain barrier for treating neurodegenerative diseases through surface modification and drug loading.
Alzheimer’s disease is a neurodegenerative disorder that usually develops in old age and is the most common form of dementia worldwide. The number of people with AD is increasing rapidly owing to the aging of the population (2022). The main clinical manifestations of AD are memory loss, cognitive decline, behavioral disturbances, and death from complications 10–20 years after the onset of the disease (Bartus et al., 1982). Three major pathological features are present in the brains of patients with AD patients, including Aβ-containing protein, neurofibrillary tangles containing tau protein, and plaque accumulation with progressive synaptic and neuronal loss (Castello et al., 2012).
BACE1 is a protease responsible for the N-terminal cleavage of amyloid precursor protein (APP), which produces aggregates to form Aβ polypeptides (Taylor et al., 2022). In view of this, researchers delivered sEVs-containing siRNA into the brains of AD mice via tail vein injection and found a significant decrease in the level of mRNA and protein of the therapeutic target BACE1, representing the first study to explore sEV-based drug delivery for CNS diseases and demonstrating the validity of using siRNA to deliver sEVs to treat ND (Alvarez-Erviti et al., 2011). Subsequently, scientists conducted numerous studies on the effects of sEVs in AD. For example, Morel et al. (2013) found that miR-124a delivered via sEVs enhanced the expression of excitatory amino acid transporter protein 2 (GLT1) on the surface of astrocytes, thereby regulating synaptic activity and improving glutamate uptake. This approach is expected to alleviate neuronal apoptosis in AD. Improving Aβ clearance is an effective strategy for AD treatment. Yuyama et al. (2012) found that increased secretion of sEVs with sphingomyelin synthase 2 (SMS2) siRNA significantly reduced extracellular levels of Aβ by enhancing Aβ uptake into microglia. Falker et al. (2016) found that PrP(C) enriched on sEVs from SH-SY5Y reduced the neurotoxicity of Aβ via promoting Aβ fibrosis. Jahangard et al. (2020) injected miR-29-containing sEVs to the CA1 (cornu ammonis area) of the hippocampus in a rat model of AD and found that miR-29-containing sEVs could partially recover cognitive deficits. Based on these studies, we suggested that sEVs that are modified by genetic engineering may be of potential therapeutic value for treating AD. Likewise, there are also some studies of sEVs loaded with drugs. Indeed, Wang et al. (2019) designed sEVs as carriers of the anti-inflammatory agent curcumin and effectively delivered curcumin to the BBB to inhibit Tau phosphorylation and prevent neuronal death via the AKT/GSK-3β pathway, thereby alleviating AD symptoms. Qi et al. (2020) treated AD models with free quercetin and quercetin-loaded sEVs, and found that the sEV delivery system improved quercetin permeability in the brain parenchymal tissue and improved cognitive function more than free quercetin. Tang et al. (2022) reported that sEVs encapsulated with neferine reduced the levels of Aβ in the brain and eased motor deficits in APP/presenilin1 (PS1) double transgenic mice.
Parkinson’s disease is a neurological disorder caused by degenerating dopaminergic neurons in the striatum nigra and the formation of Lewy bodies, with the main clinical manifestations being motor symptoms such as myotonia, resting tremor, bradykinesia, postural balance disorders, and non-motor symptoms such as autonomic dysfunction, mood disorders, and sleep disorders (Samii et al., 2004; Zhang et al., 2005).
Small extracellular vesicles have also been used in the treatment of PD via different pathways. For example, Chen H. X. et al. (2020) found that sEVs from umbilical cord MSCs could inhibit apoptosis of DA neurons by inducing autophagy in a model of PD, with potential therapeutic effects. In addition to sEVs of natural origin, modified sEVs can be used for treating PD. For example, Cooper et al. (2014) and Izco et al. (2019) modified sEVs by combining brain-specific RVG with siRNA or shRNA minicircles to treat PD. They found that these sEVs reduced the levels of α-synuclein mRNA and protein throughout a mouse brain 7 days after peripheral injection, and downregulated α-synuclein aggregation in a mouse model of PD. Haney et al. (2015) and Kojima et al. (2018) found that peroxidase-loaded sEVs induced neuroprotective effects in vitro and in vivo models of PD. Additionally, sEVs-containing drugs can significantly enhance the effects of existing drugs. For example, Qu et al. (2018b) loaded dopamine into blood sEVs by incubation and delivered to the brain, demonstrating that the dopamine distribution in the brain was increased more than 15-fold, with good therapeutic efficacy. Liu et al. (2020) designed a curcumin analog-based nanoscavenger (NanoCA) that stimulates nuclear translocation of the major autophagy regulator transcription factor EB (TFEB), triggering autophagy and calcium-dependent secretion of sEVs to clear α-synuclein.
Huntington’s disease is inherited in an autosomal dominant manner and is caused by the amplification of the cytosine-adenine-guanine (CAG) trinucleotide repeat sequence in the short arm of the coding region of the HD gene (located at 4p16.3) in exon 1 of the Huntington gene (MacDonald et al., 1993). A higher number of CAG repeats is also thought to be associated with earlier disease onset, faster clinical progression, and increased disease severity (Aziz et al., 2009). Clinical features of HD include progressive motor dysfunction (mainly chorea), cognitive decline, and psychiatric disorders, such as personality changes and depression (Walker, 2007). Dysphagia is one of the most common and important complications of HD, often leading to aspiration pneumonia and death (Schumann-Werner et al., 2021).
Many studies have investigated the use of sEVs as DDS for HD treatment. For instance, Lee et al. (2016) found that adipose-derived stem cell sEVs (ASC-sEVs) significantly reduced the aggregation of mHTT in neuronal cells, upregulated the expression of PGC-1 and phosphorylated CREB, and reduce mitochondrial dysfunction and apoptosis, suggesting that ASC-sEVs have potential for treating HD. Hong et al. (2017) injected astrocytic-derived sEVs into the striatum of HD 140Q knock-in (KI) mice, demonstrating a reduced density of mHTT aggregates. Interestingly, the presence of mHTT protein was not detected in sEVs released from primary astrocytes in 140Q KI mice, highlighting the potential use of astrocyte-derived sEVs in HD treatment (Hong et al., 2017). In addition to studies on sEVs of natural origin, numerous studies have investigated engineered sEVs. For example, Didiot et al. (2016) found that hydrophobic modification of sEVs mediated siRNA silencing of Huntington mRNA, which is expected to facilitate the development of therapeutic approaches for HD and other NDs. Zhang et al. (2021) designed a cytomegalovirus promoter-directed genetic circuit encoding the RVG tag and mHTT siRNA targeting sEVs and delivered the mHTT siRNA to the cortex and striatum via the sEVs circulatory system, showing that levels of mHTT protein and toxic aggregates were successfully reduced in the cortex and striatum of a mouse model of HD, providing a novel idea for the treatment of HD.
Other types of NDs, such as epilepsy, ALS, multiple sclerosis (MS), and spinal cerebellar ataxia (SCA), especially SCA, have been poorly studied.
Mesenchymal stem cell-derived sEVs administered intranasally are capable of treating the symptoms of LPS-induced epilepsy in mice. Long et al. (2017) found that the administration of sEVs following status epilepticus greatly reduced microglial activation, decreased overall neuronal losses in the hippocampus, and prevented cognitive and memory declines in the chronic phase. ALS is most commonly studied using transgenic mice overexpressing a reported G93A mutation of the human SOD1 gene [SOD1 (G93A)] (Vinsant et al., 2013). Bonafede et al. (2020) found that repeated administration of ASC-sEVs significantly improved motor function in treated SOD1 (G93A) mice and protected motor neurons, neuromuscular junctions, and muscles as well as reduced glial cell activation, demonstrating the potential therapeutic role of ASC-sEVs in human ALS. Wu et al. (2022) isolated sEVs from mouse neural stem cells and modified them to enable targeted delivery to the lesioned areas of MS model mice. The encapsulation of Bryostatin-1 (Bryo) in sEVs exhibited potent therapeutic effects compared to Bryo alone, suggesting that targeted delivery of Bryo based on sEVs significantly improves myelin protection and promotes myelin regeneration. You et al. (2020) found that MSC-derived sEVs reduced the loss of Purkinje cells and cerebellar myelin sheaths, alleviated neuroinflammation, and improved motor functions in SCA3 mice, which is expected to facilitate the development of SCA3 and other types of SCA.
Stem cell therapy has been a hot research topic in disease treatment recently, with several reports on this therapy. Coenzyme Q10 (CoQ10) is an electron donor in the mitochondrial electron transport chain and acts as an antioxidant to enhance mitochondrial function and prevent oxidative stress (Manzar et al., 2020). It has been demonstrated that CoQ10 has neuroprotective effects in ND and inhibits the pathological progression of neuroinflammation-related diseases (Ghasemloo et al., 2021). Sheykhhasan et al. (2022) found that sEVs obtained from adipose-derived stem cells could act as carriers of CoQ10. CoQ10 delivered by sEVs enhanced cognitive and memory deficits in AD by increasing Brain-derived neurotrophic factor (BDNF) and sex-determining region Y-box 2 (SOX2) levels in the hippocampus, thereby increasing the therapeutic effect of CoQ10. This therapeutic effect is not only due to the delivery of CoQ10 but also to the potential of stem cell-driven sEVs in tissue regeneration. Additionally, Losurdo et al. (2020) demonstrated that the intranasal delivery route of sEVs from cytokine-preconditioned MSCs could induce immunomodulatory and neuroprotective effects in triple-transgenic 3xTg mice. As a result, sEVs derived from stem cells can perform a dual protective function when used as drug delivery vehicles, aiding healing via their own therapeutic properties, thus creating a microenvironment that promotes healing while also serving as a targeting vehicle for the delivery of appropriate drugs.
The use of sEVs as a therapeutic approach for ND has largely focused on their functional aspects, but their negative effects have rarely been studied. Although sEVs can pass through the BBB, the amount that can pass is limited (about 5%) and the remaining 95% may remain at unknown sites of action and cause systemic adverse effects (Sonvico et al., 2018). Safety is also a key consideration, as nanomaterials may cause oxidative stress, apoptosis, and inflammation in the brain (Vega-Villa et al., 2008). Therefore, future designs should consider the biodegradability of sEVs from the brain. Additionally, the protein corona formed on the surface of sEVs may shield its surface from targeting molecules and lead to targeting errors (Salvati et al., 2013). The development of sEVs with long blood circulation times is also extremely challenging. Most of the targeted sEVs take a long time to penetrate the BBB and reach the target site, so they tend to accumulate in the liver and spleen (Chopra et al., 2022).
Many studies on sEVs have been limited to in vitro or animal model-level studies, and no further in vivo studies have been conducted to assess the safety of sEV therapeutic approaches. In previous studies, sEVs have been shown to play a role in promoting neurogenesis, inhibiting neuroinflammation, promoting angiogenesis, and promoting synaptic plasticity. However, not all effects are relevant for treating a disease, and some even pose significant risks, such as whether the treatment of non-oncological diseases increases the risk of cancer promotion. Despite the great therapeutic potential of sEV-based technologies, they are still in the growth phase. Most current research on sEVs is basic research and rarely used in the clinic, due to the fact that bench-to-bedside translational techniques typically require reasonable scalability, throughput, and thorough validation to screen large numbers of clinical samples (Li P. et al., 2017). For example, the amount of sEVs needed to be injected into a patient to achieve a therapeutic dose depends on several factors, such as the source, type and amount of sEVs, the purpose of the treatment, and the patient’s condition. Usually, sEVs injections require strict dose calculation and control, with individualized adjustments based on individual differences in patient weight, age, and condition. In addition, sEVs treatment also requires rigorous safety assessment and monitoring to ensure treatment efficacy and safety (Chen et al., 2023). To ensure the safe and effective clinical use of sEVs for treating major diseases, their safety and long-term effects should be further explored. Therefore, a comprehensive testing program for sEVs, including pharmacokinetic, biodistribution, and acute and chronic toxicity testing, is necessary prior to their introduction into clinical trials.
Small extracellular vesicles are widely used in brain-targeted delivery systems due to their low toxicity, low immunogenicity, high stability, high delivery efficiency, high biocompatibility, and trans-BBB function. sEVs can participate in inter-neuronal information exchange through self-delivery, endocytosis, or donor-receptor-specific binding for brain-targeted drug delivery. sEVs can also be chemically or genetically modified for efficient brain targeting. Today, sEVs are widely used in the diagnosis and treatment of ND and have become the most promising DDS.
However, the clinical application of sEVs in ND is still challenged by many factors, such as the lack of standardization and clinical utility of purification and detection methods, lack of sensitivity and specificity of screening indicators, the incomplete elucidation of the mechanism of action of sEVs targeting into the brain for expression and regulation, and the safety and efficiency of drug delivery. Although engineered sEVs have shown great potential in ND, clinical mass production remains a pressing issue. Furthermore, to better understand the in vivo behavior of sEVs, assessment of their pharmacokinetic properties is crucial. Analysis of the in vivo distribution of sEVs is a prerequisite for developing sEVs-based therapeutics and drug delivery vehicles that can accurately predict therapeutic doses and potential side effects. Therefore, the comprehensive screening of sEVs using sensitive and reliable measurement methods, the precise analysis of the relevant signaling pathways and molecular mechanisms, the pharmacokinetic properties of sEVs, and the selection of the correct and effective markers and therapeutic targets remain the focus of future research.
Today, many biotech companies are contributing to the field of sEVs, such as Evox therapeutics, which is developing modified sEVs to improve their ability to deliver siRNA across the BBB (Cully, 2021). Additionally, Evox is optimizing the sEVs delivery of proteins or mRNA to rare diseases within the CNS and other organs (Cully, 2021). Researchers have been exploring and continuously optimizing sEVs as potential therapies for various diseases. For example, Liang et al. (2022) employed an engineering strategy to decorate albumin onto the surface of the sEVs through surface display of albumin binding domains to prolong the circulation time of sEVs in the body and to reduce the frequency of sEVs administration. In conclusion, sEVs still offer hope for patients with ND and have great clinical research value, providing a new approach to the diagnosis and treatment of ND and better elucidating the pathophysiological mechanisms.
ZJ and RH designed the structure of the manuscript and finalized the manuscript. RP and DC drafted the manuscript. RP, DC, and LH provided critical revisions and improvements to the manuscript. All authors approved the final version of the manuscript.
This work was supported by the Key Laboratory of Prevention and Treatment of Cardiovascular and Cerebrovascular Diseases, Ministry of Education (XN202014).
The authors deeply appreciate the support from all participants.
The authors declare that the research was conducted in the absence of any commercial or financial relationships that could be construed as a potential conflict of interest.
All claims expressed in this article are solely those of the authors and do not necessarily represent those of their affiliated organizations, or those of the publisher, the editors and the reviewers. Any product that may be evaluated in this article, or claim that may be made by its manufacturer, is not guaranteed or endorsed by the publisher.
Abdik, H., Avsar Abdik, E., Hizli Deniz, A. A., Tasli, P. N., and Sahin, F. (2019). A novel virtue in stem cell research: exosomes and their role in differentiation. Adv. Exp. Med. Biol. 1144, 133–146. doi: 10.1007/5584_2019_339
Agrawal, A. K., Aqil, F., Jeyabalan, J., Spencer, W. A., Beck, J., Gachuki, B. W., et al. (2017). Milk-derived exosomes for oral delivery of paclitaxel. Nanomedicine 13, 1627–1636. doi: 10.1016/j.nano.2017.03.001
Alvarez-Erviti, L., Seow, Y., Yin, H., Betts, C., Lakhal, S., and Wood, M. J. (2011). Delivery of siRNA to the mouse brain by systemic injection of targeted exosomes. Nat. Biotechnol. 29, 341–345. doi: 10.1038/nbt.1807
Aqil, F., Munagala, R., Jeyabalan, J., Agrawal, A. K., and Gupta, R. (2017). Exosomes for the enhanced tissue bioavailability and efficacy of curcumin. AAPS J. 19, 1691–1702. doi: 10.1208/s12248-017-0154-9
Aziz, N. A., Jurgens, C. K., Landwehrmeyer, G. B., Group, E. R. S., van Roon-Mom, W. M., van Ommen, G. J., et al. (2009). Normal and mutant HTT interact to affect clinical severity and progression in Huntington disease. Neurology 73, 1280–1285. doi: 10.1212/WNL.0b013e3181bd1121
Bakhti, M., Winter, C., and Simons, M. (2011). Inhibition of myelin membrane sheath formation by oligodendrocyte-derived exosome-like vesicles. J. Biol. Chem. 286, 787–796. doi: 10.1074/jbc.M110.190009
Bartus, R. T., Dean, R. L. III, Beer, B., and Lippa, A. S. (1982). The cholinergic hypothesis of geriatric memory dysfunction. Science 217, 408–414. doi: 10.1126/science.7046051
Bonafede, R., Turano, E., Scambi, I., Busato, A., Bontempi, P., Virla, F., et al. (2020). ASC-exosomes ameliorate the disease progression in SOD1(G93A) murine model underlining their potential therapeutic use in human ALS. Int. J. Mol. Sci. 21:3651. doi: 10.3390/ijms21103651
Burgio, S., Noori, L., Marino Gammazza, A., Campanella, C., Logozzi, M., Fais, S., et al. (2020). Extracellular vesicles-based drug delivery systems: a new challenge and the exemplum of malignant pleural mesothelioma. Int. J. Mol. Sci. 21:5432. doi: 10.3390/ijms21155432
Castello, N. A., Green, K. N., and LaFerla, F. M. (2012). Genetic knockdown of brain-derived neurotrophic factor in 3xTg-AD mice does not alter Abeta or tau pathology. PLoS One 7:e39566. doi: 10.1371/journal.pone.0039566
Chan, J. M., Valencia, P. M., Zhang, L., Langer, R., and Farokhzad, O. C. (2010). Polymeric nanoparticles for drug delivery. Methods Mol. Biol. 624, 163–175. doi: 10.1007/978-1-60761-609-2_11
Chen, B. Y., Sung, C. W., Chen, C., Cheng, C. M., Lin, D. P., Huang, C. T., et al. (2019). Advances in exosomes technology. Clin. Chim. Acta 493, 14–19. doi: 10.1016/j.cca.2019.02.021
Chen, H. X., Liang, F. C., Gu, P., Xu, B. L., Xu, H. J., Wang, W. T., et al. (2020). Exosomes derived from mesenchymal stem cells repair a Parkinson’s disease model by inducing autophagy. Cell Death Dis. 11:288. doi: 10.1038/s41419-020-2473-5
Chen, J. J., Yang, G., Yan, Q. Q., Zhao, J., and Li, S. (2019). Exosome-encapsulated microRNAs as promising biomarkers for Alzheimer’s disease. Rev. Neurosci. 31, 77–87. doi: 10.1515/revneuro-2019-0001
Chen, L. Y., Kao, T. W., Chen, C. C., Niaz, N., Lee, H. L., Chen, Y. H., et al. (2023). Frontier review of the molecular mechanisms and current approaches of stem cell-derived exosomes. Cells 12:1018. doi: 10.3390/cells12071018
Chen, Z. J., Yang, S. C., Liu, X. L., Gao, Y., Dong, X., Lai, X., et al. (2020). Nanobowl-supported liposomes improve drug loading and delivery. Nano Lett. 20, 4177–4187. doi: 10.1021/acs.nanolett.0c00495
Chopra, H., Bibi, S., Singh, I., Kamal, M. A., Islam, F., Alhumaydhi, F. A., et al. (2022). Nanomedicines in the management of Alzheimer’s disease: current view and future prospects. Front. Aging Neurosci. 14:879114. doi: 10.3389/fnagi.2022.879114
Chu, Y., Zhang, J., Pan, H., Shi, J., Wang, J., and Chen, L. (2020). Preparation and evaluation of long circulating erythrocyte membrane-cloaked anti-cancer drug delivery system. Drug Deliv. Transl. Res. 10, 1278–1287. doi: 10.1007/s13346-020-00780-x
Claridge, B., Lozano, J., Poh, Q. H., and Greening, D. W. (2021). Development of extracellular vesicle therapeutics: challenges, considerations, and opportunities. Front. Cell Dev. Biol. 9:734720. doi: 10.3389/fcell.2021.734720
Cooper, J. M., Wiklander, P. B., Nordin, J. Z., Al-Shawi, R., Wood, M. J., Vithlani, M., et al. (2014). Systemic exosomal siRNA delivery reduced alpha-synuclein aggregates in brains of transgenic mice. Mov. Disord. 29, 1476–1485. doi: 10.1002/mds.25978
Cui, G. H., Guo, H. D., Li, H., Zhai, Y., Gong, Z. B., Wu, J., et al. (2019). RVG-modified exosomes derived from mesenchymal stem cells rescue memory deficits by regulating inflammatory responses in a mouse model of Alzheimer’s disease. Immun Ageing 16:10. doi: 10.1186/s12979-019-0150-2
Cully, M. (2021). Exosome-based candidates move into the clinic. Nat. Rev. Drug Discov. 20, 6–7. doi: 10.1038/d41573-020-00220-y
Dal Magro, R., Ornaghi, F., Cambianica, I., Beretta, S., Re, F., Musicanti, C., et al. (2017). ApoE-modified solid lipid nanoparticles: a feasible strategy to cross the blood-brain barrier. J. Control Release 249, 103–110. doi: 10.1016/j.jconrel.2017.01.039
De Jong, W. H., and Borm, P. J. (2008). Drug delivery and nanoparticles:applications and hazards. Int. J. Nanomed. 3, 133–149. doi: 10.2147/ijn.s596
Del Pozo-Acebo, L., Lopez de Las Hazas, M. C., Tome-Carneiro, J., Del Saz-Lara, A., Gil-Zamorano, J., Balaguer, L., et al. (2022). Therapeutic potential of broccoli-derived extracellular vesicles as nanocarriers of exogenous miRNAs. Pharmacol. Res. 185:106472. doi: 10.1016/j.phrs.2022.106472
Didiot, M. C., Hall, L. M., Coles, A. H., Haraszti, R. A., Godinho, B. M., Chase, K., et al. (2016). Exosome-mediated delivery of hydrophobically modified siRNA for Huntingtin mRNA silencing. Mol. Ther. 24, 1836–1847. doi: 10.1038/mt.2016.126
Doyle, L. M., and Wang, M. Z. (2019). Overview of extracellular vesicles, their origin, composition, purpose, and methods for exosome isolation and analysis. Cells 8:727. doi: 10.3390/cells8070727
Eitan, E., Hutchison, E. R., Marosi, K., Comotto, J., Mustapic, M., Nigam, S. M., et al. (2016). Extracellular vesicle-associated abeta mediates trans-neuronal bioenergetic and Ca(2+)-handling deficits in Alzheimer’s disease models. NPJ Aging Mech. Dis. 2:16019. doi: 10.1038/npjamd.2016.19
El-Andaloussi, S., Lee, Y., Lakhal-Littleton, S., Li, J., Seow, Y., Gardiner, C., et al. (2012). Exosome-mediated delivery of siRNA in vitro and in vivo. Nat. Protoc. 7, 2112–2126. doi: 10.1038/nprot.2012.131
Elsharkasy, O. M., Nordin, J. Z., Hagey, D. W., de Jong, O. G., Schiffelers, R. M., Andaloussi, S. E., et al. (2020). Extracellular vesicles as drug delivery systems: why and how? Adv. Drug Deliv. Rev. 159, 332–343. doi: 10.1016/j.addr.2020.04.004
Erdo, F., Bors, L. A., Farkas, D., Bajza, A., and Gizurarson, S. (2018). Evaluation of intranasal delivery route of drug administration for brain targeting. Brain Res. Bull. 143, 155–170. doi: 10.1016/j.brainresbull.2018.10.009
Falker, C., Hartmann, A., Guett, I., Dohler, F., Altmeppen, H., Betzel, C., et al. (2016). Exosomal cellular prion protein drives fibrillization of amyloid beta and counteracts amyloid beta-mediated neurotoxicity. J. Neurochem. 137, 88–100. doi: 10.1111/jnc.13514
Fitzner, D., Schnaars, M., van Rossum, D., Krishnamoorthy, G., Dibaj, P., Bakhti, M., et al. (2011). Selective transfer of exosomes from oligodendrocytes to microglia by macropinocytosis. J. Cell Sci. 124(Pt. 3), 447–458. doi: 10.1242/jcs.074088
Frank, M. M. (1993). The reticuloendothelial system and bloodstream clearance. J. Lab. Clin. Med. 122, 487–488.
Fruhbeis, C., Frohlich, D., and Kramer-Albers, E. M. (2012). Emerging roles of exosomes in neuron-glia communication. Front. Physiol. 3:119. doi: 10.3389/fphys.2012.00119
Fruhbeis, C., Frohlich, D., Kuo, W. P., Amphornrat, J., Thilemann, S., Saab, A. S., et al. (2013). Neurotransmitter-triggered transfer of exosomes mediates oligodendrocyte-neuron communication. PLoS Biol. 11:e1001604. doi: 10.1371/journal.pbio.1001604
Fuhrmann, G., Serio, A., Mazo, M., Nair, R., and Stevens, M. M. (2015). Active loading into extracellular vesicles significantly improves the cellular uptake and photodynamic effect of porphyrins. J. Control Release 205, 35–44. doi: 10.1016/j.jconrel.2014.11.029
Garcia-Manrique, P., Matos, M., Gutierrez, G., Pazos, C., and Blanco-Lopez, M. C. (2018). Therapeutic biomaterials based on extracellular vesicles: classification of bio-engineering and mimetic preparation routes. J. Extracell. Vesicles 7:1422676. doi: 10.1080/20013078.2017.1422676
Gelibter, S., Marostica, G., Mandelli, A., Siciliani, S., Podini, P., Finardi, A., et al. (2022). The impact of storage on extracellular vesicles: a systematic study. J. Extracell. Vesicles 11:e12162. doi: 10.1002/jev2.12162
Ghasemloo, E., Mostafavi, H., Hosseini, M., Forouzandeh, M., Eskandari, M., and Mousavi, S. S. (2021). Neuroprotective effects of coenzyme Q10 in Parkinson’s model via a novel Q10/miR-149-5p/MMPs pathway. Metab. Brain Dis. 36, 2089–2100. doi: 10.1007/s11011-021-00795-4
Gorgens, A., Corso, G., Hagey, D. W., Jawad Wiklander, R., Gustafsson, M. O., Felldin, U., et al. (2022). Identification of storage conditions stabilizing extracellular vesicles preparations. J. Extracell. Vesicles 11:e12238. doi: 10.1002/jev2.12238
Grey, M., Dunning, C. J., Gaspar, R., Grey, C., Brundin, P., Sparr, E., et al. (2015). Acceleration of alpha-synuclein aggregation by exosomes. J. Biol. Chem. 290, 2969–2982. doi: 10.1074/jbc.M114.585703
Guo, P., Busatto, S., Huang, J., Morad, G., and Moses, M. A. (2021). A facile magnetic extrusion method for preparing endosome-derived vesicles for cancer drug delivery. Adv. Funct. Mater. 31:2008326. doi: 10.1002/adfm.202008326
Gurung, S., Perocheau, D., Touramanidou, L., and Baruteau, J. (2021). The exosome journey: from biogenesis to uptake and intracellular signalling. Cell Commun. Signal. 19:47. doi: 10.1186/s12964-021-00730-1
Hadjidemetriou, M., Al-Ahmady, Z., Mazza, M., Collins, R. F., Dawson, K., and Kostarelos, K. (2015). In Vivo Biomolecule Corona around Blood-Circulating, Clinically Used and Antibody-Targeted Lipid Bilayer Nanoscale Vesicles. ACS Nano 9, 8142–8156. doi: 10.1021/acsnano.5b03300
Hajipour, H., Farzadi, L., Roshangar, L., Latifi, Z., Kahroba, H., Shahnazi, V., et al. (2021). A human chorionic gonadotropin (hCG) delivery platform using engineered uterine exosomes to improve endometrial receptivity. Life Sci. 275:119351. doi: 10.1016/j.lfs.2021.119351
Han, C., Yang, J., Sun, J., and Qin, G. (2022). Extracellular vesicles in cardiovascular disease: biological functions and therapeutic implications. Pharmacol. Ther. 233:108025. doi: 10.1016/j.pharmthera.2021.108025
Haney, M. J., Klyachko, N. L., Zhao, Y., Gupta, R., Plotnikova, E. G., He, Z., et al. (2015). Exosomes as drug delivery vehicles for Parkinson’s disease therapy. J. Control Release 207, 18–30. doi: 10.1016/j.jconrel.2015.03.033
Hong, Y., Zhao, T., Li, X. J., and Li, S. (2017). Mutant huntingtin inhibits alphaB-crystallin expression and impairs exosome secretion from astrocytes. J. Neurosci. 37, 9550–9563. doi: 10.1523/JNEUROSCI.1418-17.2017
Izco, M., Blesa, J., Schleef, M., Schmeer, M., Porcari, R., Al-Shawi, R., et al. (2019). Systemic exosomal delivery of shRNA minicircles prevents parkinsonian pathology. Mol. Ther. 27, 2111–2122. doi: 10.1016/j.ymthe.2019.08.010
Jahangard, Y., Monfared, H., Moradi, A., Zare, M., Mirnajafi-Zadeh, J., and Mowla, S. J. (2020). Therapeutic effects of transplanted exosomes containing miR-29b to a rat model of Alzheimer’s disease. Front. Neurosci. 14:564. doi: 10.3389/fnins.2020.00564
Kalani, A., Chaturvedi, P., Kamat, P. K., Maldonado, C., Bauer, P., Joshua, I. G., et al. (2016). Curcumin-loaded embryonic stem cell exosomes restored neurovascular unit following ischemia-reperfusion injury. Int. J. Biochem. Cell Biol. 79, 360–369. doi: 10.1016/j.biocel.2016.09.002
Kalluri, R., and LeBleu, V. S. (2020). The biology, function, and biomedical applications of exosomes. Science 367:eaau6977. doi: 10.1126/science.aau6977
Kim, M. S., Haney, M. J., Zhao, Y., Mahajan, V., Deygen, I., Klyachko, N. L., et al. (2016). Development of exosome-encapsulated paclitaxel to overcome MDR in cancer cells. Nanomedicine 12, 655–664. doi: 10.1016/j.nano.2015.10.012
Kimiz-Gebologlu, I., and Oncel, S. S. (2022). Exosomes: large-scale production, isolation, drug loading efficiency, and biodistribution and uptake. J. Control Release 347, 533–543. doi: 10.1016/j.jconrel.2022.05.027
Koh, Y. Q., Almughlliq, F. B., Vaswani, K., Peiris, H. N., and Mitchell, M. D. (2018). Exosome enrichment by ultracentrifugation and size exclusion chromatography. Front. Biosci. 23:865–874. doi: 10.2741/4621
Kojima, R., Bojar, D., Rizzi, G., Hamri, G. C., El-Baba, M. D., Saxena, P., et al. (2018). Designer exosomes produced by implanted cells intracerebrally deliver therapeutic cargo for Parkinson’s disease treatment. Nat. Commun. 9:1305. doi: 10.1038/s41467-018-03733-8
Kooijmans, S. A., Vader, P., van Dommelen, S. M., van Solinge, W. W., and Schiffelers, R. M. (2012). Exosome mimetics: a novel class of drug delivery systems. Int. J. Nanomed. 7, 1525–1541. doi: 10.2147/IJN.S29661
Kooijmans, S. A. A., Stremersch, S., Braeckmans, K., de Smedt, S. C., Hendrix, A., Wood, M. J. A., et al. (2013). Electroporation-induced siRNA precipitation obscures the efficiency of siRNA loading into extracellular vesicles. J. Control Release 172, 229–238. doi: 10.1016/j.jconrel.2013.08.014
Kurtjak, M., Kereiche, S., Klepac, D., Krizan, H., Percic, M., Krusic Alic, V., et al. (2022). Unveiling the native morphology of extracellular vesicles from human cerebrospinal fluid by atomic force and cryogenic electron microscopy. Biomedicines 10:1251. doi: 10.3390/biomedicines10061251
Lai, N., Wu, D., Liang, T., Pan, P., Yuan, G., Li, X., et al. (2020). Systemic exosomal miR-193b-3p delivery attenuates neuroinflammation in early brain injury after subarachnoid hemorrhage in mice. J. Neuroinflamm. 17:74. doi: 10.1186/s12974-020-01745-0
Lee, M., Liu, T., Im, W., and Kim, M. (2016). Exosomes from adipose-derived stem cells ameliorate phenotype of Huntington’s disease in vitro model. Eur. J. Neurosci. 44, 2114–2119. doi: 10.1111/ejn.13275
Lee, S. T., Im, W., Ban, J. J., Lee, M., Jung, K. H., Lee, S. K., et al. (2017). Exosome-based delivery of miR-124 in a Huntington’s disease model. J. Mov. Disord. 10, 45–52. doi: 10.14802/jmd.16054
Li, C., Qin, S., Wen, Y., Zhao, W., Huang, Y., and Liu, J. (2022). Overcoming the blood-brain barrier: Exosomes as theranostic nanocarriers for precision neuroimaging. J. Control Release 349, 902–916. doi: 10.1016/j.jconrel.2022.08.002
Li, M., Fang, F., Sun, M., Zhang, Y., Hu, M., and Zhang, J. (2022). Extracellular vesicles as bioactive nanotherapeutics: an emerging paradigm for regenerative medicine. Theranostics 12, 4879–4903. doi: 10.7150/thno.72812
Li, P., Kaslan, M., Lee, S. H., Yao, J., and Gao, Z. (2017). Progress in exosome isolation techniques. Theranostics 7, 789–804. doi: 10.7150/thno.18133
Li, X., Tsibouklis, J., Weng, T., Zhang, B., Yin, G., Feng, G., et al. (2017). Nano carriers for drug transport across the blood-brain barrier. J. Drug Target 25, 17–28. doi: 10.1080/1061186X.2016.1184272
Liang, X., Niu, Z., Galli, V., Howe, N., Zhao, Y., Wiklander, O. P. B., et al. (2022). Extracellular vesicles engineered to bind albumin demonstrate extended circulation time and lymph node accumulation in mouse models. J. Extracell. Vesicles 11:e12248. doi: 10.1002/jev2.12248
Lindberg, D. J., Wesen, E., Bjorkeroth, J., Rocha, S., and Esbjorner, E. K. (2017). Lipid membranes catalyse the fibril formation of the amyloid-beta (1-42) peptide through lipid-fibril interactions that reinforce secondary pathways. Biochim. Biophys. Acta Biomembr. 1859, 1921–1929. doi: 10.1016/j.bbamem.2017.05.012
Liu, J., Liu, C., Zhang, J., Zhang, Y., Liu, K., Song, J. X., et al. (2020). A Self-Assembled alpha-Synuclein Nanoscavenger for Parkinson’s Disease. ACS Nano 14, 1533–1549. doi: 10.1021/acsnano.9b06453
Liu, X., Yang, Y., Li, Y., Niu, X., Zhao, B., Wang, Y., et al. (2017). Integration of stem cell-derived exosomes with in situ hydrogel glue as a promising tissue patch for articular cartilage regeneration. Nanoscale 9, 4430–4438. doi: 10.1039/c7nr00352h
Loch-Neckel, G., Matos, A. T., Vaz, A. R., and Brites, D. (2022). Challenges in the development of drug delivery systems based on small extracellular vesicles for therapy of brain diseases. Front. Pharmacol. 13:839790. doi: 10.3389/fphar.2022.839790
Long, Q., Upadhya, D., Hattiangady, B., Kim, D. K., An, S. Y., Shuai, B., et al. (2017). Intranasal MSC-derived A1-exosomes ease inflammation, and prevent abnormal neurogenesis and memory dysfunction after status epilepticus. Proc. Natl. Acad. Sci. U.S.A. 114, E3536–E3545. doi: 10.1073/pnas.1703920114
Losurdo, M., Pedrazzoli, M., D’Agostino, C., Elia, C. A., Massenzio, F., Lonati, E., et al. (2020). Intranasal delivery of mesenchymal stem cell-derived extracellular vesicles exerts immunomodulatory and neuroprotective effects in a 3xTg model of Alzheimer’s disease. Stem Cells Transl. Med. 9, 1068–1084. doi: 10.1002/sctm.19-0327
Lu, Y., Wang, L., Zhang, M., and Chen, Z. (2022). Mesenchymal stem cell-derived small extracellular vesicles: a novel approach for kidney disease treatment. Int. J. Nanomed. 17, 3603–3618. doi: 10.2147/IJN.S372254
Luan, X., Sansanaphongpricha, K., Myers, I., Chen, H., Yuan, H., and Sun, D. (2017). Engineering exosomes as refined biological nanoplatforms for drug delivery. Acta Pharmacol. Sin. 38, 754–763. doi: 10.1038/aps.2017.12
Luarte, A., Batiz, L. F., Wyneken, U., and Lafourcade, C. (2016). Potential therapies by stem cell-derived exosomes in CNS diseases: focusing on the neurogenic niche. Stem Cells Int. 2016:5736059. doi: 10.1155/2016/5736059
Ma, X., Huang, M., Zheng, M., Dai, C., Song, Q., Zhang, Q., et al. (2020). ADSCs-derived extracellular vesicles alleviate neuronal damage, promote neurogenesis and rescue memory loss in mice with Alzheimer’s disease. J. Control Release 327, 688–702. doi: 10.1016/j.jconrel.2020.09.019
MacDonald, M. E., Ambrose, C. M., Duyao, M. P., Myers, R. H., Lin, C., and Srinidhi, L. (1993). A novel gene containing a trinucleotide repeat that is expanded and unstable on Huntington’s disease chromosomes. Cell 72, 971–983.
Man, K., Brunet, M. Y., Jones, M. C., and Cox, S. C. (2020). Engineered extracellular vesicles: tailored-made nanomaterials for medical applications. Nanomaterials 10:1838. doi: 10.3390/nano10091838
Manzar, H., Abdulhussein, D., Yap, T. E., and Cordeiro, M. F. (2020). Cellular consequences of coenzyme Q10 deficiency in neurodegeneration of the retina and brain. Int. J. Mol. Sci. 21:9299. doi: 10.3390/ijms21239299
Martinez-Greene, J. A., Hernandez-Ortega, K., Quiroz-Baez, R., Resendis-Antonio, O., Pichardo-Casas, I., Sinclair, D. A., et al. (2021). Quantitative proteomic analysis of extracellular vesicle subgroups isolated by an optimized method combining polymer-based precipitation and size exclusion chromatography. J. Extracell. Vesicles 10:e12087. doi: 10.1002/jev2.12087
McCann, J., Sosa-Miranda, C. D., Guo, H., Reshke, R., Savard, A., Zardini Buzatto, A., et al. (2022). Contaminating transfection complexes can masquerade as small extracellular vesicles and impair their delivery of RNA. J. Extracell. Vesicles 11:e12220. doi: 10.1002/jev2.12220
Mehryab, F., Rabbani, S., Shahhosseini, S., Shekari, F., Fatahi, Y., Baharvand, H., et al. (2020). Exosomes as a next-generation drug delivery system: an update on drug loading approaches, characterization, and clinical application challenges. Acta Biomater. 113, 42–62. doi: 10.1016/j.actbio.2020.06.036
Men, Y., Yelick, J., Jin, S., Tian, Y., Chiang, M. S. R., Higashimori, H., et al. (2019). Exosome reporter mice reveal the involvement of exosomes in mediating neuron to astroglia communication in the CNS. Nat. Commun. 10:4136. doi: 10.1038/s41467-019-11534-w
Meng, W., He, C., Hao, Y., Wang, L., Li, L., and Zhu, G. (2020). Prospects and challenges of extracellular vesicle-based drug delivery system: considering cell source. Drug Deliv. 27, 585–598. doi: 10.1080/10717544.2020.1748758
Mondal, S. K., and Whiteside, T. L. (2021). Immunoaffinity-based isolation of melanoma cell-derived AND T cell-derived exosomes from plasma of melanoma patients. Methods Mol. Biol. 2265, 305–321. doi: 10.1007/978-1-0716-1205-7_23
Morales-Kastresana, A., Musich, T. A., Welsh, J. A., Telford, W., Demberg, T., Wood, J. C. S., et al. (2019). High-fidelity detection and sorting of nanoscale vesicles in viral disease and cancer. J. Extracell. Vesicles 8:1597603. doi: 10.1080/20013078.2019.1597603
Morel, L., Regan, M., Higashimori, H., Ng, S. K., Esau, C., Vidensky, S., et al. (2013). Neuronal exosomal miRNA-dependent translational regulation of astroglial glutamate transporter GLT1. J. Biol. Chem. 288, 7105–7116. doi: 10.1074/jbc.M112.410944
Nazimek, K., Bryniarski, K., Ptak, W., Groot Kormelink, T., and Askenase, P. W. (2020). Orally administered exosomes suppress mouse delayed-type hypersensitivity by delivering miRNA-150 to antigen-primed macrophage APC targeted by exosome-surface anti-peptide antibody light chains. Int. J. Mol. Sci. 21:5540. doi: 10.3390/ijms21155540
Neamtu, I., Rusu, A. G., Diaconu, A., Nita, L. E., and Chiriac, A. P. (2017). Basic concepts and recent advances in nanogels as carriers for medical applications. Drug Deliv. 24, 539–557. doi: 10.1080/10717544.2016.1276232
Nissen, M., Forster, R., Wieduwilt, T., Lorenz, A., Jiang, S., Hauswald, W., et al. (2022). Nanoparticle tracking in single-antiresonant-element fiber for high-precision size distribution analysis of mono- and polydisperse samples. Small 18, e2202024. doi: 10.1002/smll.202202024
Niu, X., Chen, J., and Gao, J. (2019). Nanocarriers as a powerful vehicle to overcome blood-brain barrier in treating neurodegenerative diseases: focus on recent advances. Asian J. Pharm. Sci. 14, 480–496. doi: 10.1016/j.ajps.2018.09.005
Noble, J. M., Roberts, L. M., Vidavsky, N., Chiou, A. E., Fischbach, C., Paszek, M. J., et al. (2020). Direct comparison of optical and electron microscopy methods for structural characterization of extracellular vesicles. J. Struct. Biol. 210:107474. doi: 10.1016/j.jsb.2020.107474
Padda, R. S., Deng, F. K., Brett, S. I., Biggs, C. N., Durfee, P. N., Brinker, C. J., et al. (2019). Nanoscale flow cytometry to distinguish subpopulations of prostate extracellular vesicles in patient plasma. Prostate 79, 592–603. doi: 10.1002/pros.23764
Parada, N., Romero-Trujillo, A., Georges, N., and Alcayaga-Miranda, F. (2021). Camouflage strategies for therapeutic exosomes evasion from phagocytosis. J. Adv. Res. 31, 61–74. doi: 10.1016/j.jare.2021.01.001
Pei, X., Li, Y., Zhu, L., and Zhou, Z. (2019). Astrocyte-derived exosomes suppress autophagy and ameliorate neuronal damage in experimental ischemic stroke. Exp. Cell Res. 382, 111474. doi: 10.1016/j.yexcr.2019.06.019
Peng, H., Li, Y., Ji, W., Zhao, R., Lu, Z., Shen, J., et al. (2022). Intranasal administration of self-oriented nanocarriers based on therapeutic exosomes for synergistic treatment of Parkinson’s disease. ACS Nano 16, 869–884. doi: 10.1021/acsnano.1c08473
Pinnell, J. R., Cui, M., and Tieu, K. (2021). Exosomes in Parkinson disease. J. Neurochem. 157, 413–428. doi: 10.1111/jnc.15288
Prikhozhdenko, E. S., Gusliakova, O. I., Kulikov, O. A., Mayorova, O. A., Shushunova, N. A., Abdurashitov, A. S., et al. (2021). Target delivery of drug carriers in mice kidney glomeruli via renal artery. Balance between efficiency and safety. J. Control Release 329, 175–190. doi: 10.1016/j.jconrel.2020.11.051
Qi, Y., Guo, L., Jiang, Y., Shi, Y., Sui, H., and Zhao, L. (2020). Brain delivery of quercetin-loaded exosomes improved cognitive function in AD mice by inhibiting phosphorylated tau-mediated neurofibrillary tangles. Drug Deliv. 27, 745–755. doi: 10.1080/10717544.2020.1762262
Qu, M., Lin, Q., He, S., Wang, L., Fu, Y., Zhang, Z., et al. (2018a). A brain targeting functionalized liposomes of the dopamine derivative N-3,4-bis(pivaloyloxy)-dopamine for treatment of Parkinson’s disease. J. Control Release 277, 173–182. doi: 10.1016/j.jconrel.2018.03.019
Qu, M., Lin, Q., Huang, L., Fu, Y., Wang, L., He, S., et al. (2018b). Dopamine-loaded blood exosomes targeted to brain for better treatment of Parkinson’s disease. J. Control Release 287, 156–166. doi: 10.1016/j.jconrel.2018.08.035
Ren, Z., Wang, J., Wang, S., Zou, C., Li, X., Guan, Y., et al. (2013). Autologous transplantation of GDNF-expressing mesenchymal stem cells protects against MPTP-induced damage in cynomolgus monkeys. Sci. Rep. 3:2786. doi: 10.1038/srep02786
Reshke, R., Taylor, J. A., Savard, A., Guo, H., Rhym, L. H., Kowalski, P. S., et al. (2020). Reduction of the therapeutic dose of silencing RNA by packaging it in extracellular vesicles via a pre-microRNA backbone. Nat. Biomed. Eng. 4, 52–68. doi: 10.1038/s41551-019-0502-4
Ryu, K. J., Lee, J. Y., Park, C., Cho, D., and Kim, S. J. (2020). Isolation of small extracellular vesicles from human serum using a combination of ultracentrifugation with polymer-based precipitation. Ann. Lab. Med. 40, 253–258. doi: 10.3343/alm.2020.40.3.253
Salvati, A., Pitek, A. S., Monopoli, M. P., Prapainop, K., Bombelli, F. B., Hristov, D. R., et al. (2013). Transferrin-functionalized nanoparticles lose their targeting capabilities when a biomolecule corona adsorbs on the surface. Nat. Nanotechnol. 8, 137–143. doi: 10.1038/nnano.2012.237
Samii, A., Nutt, J. G., and Ransom, B. R. (2004). Parkinson’s disease. Lancet 363, 1783–1793. doi: 10.1016/S0140-6736(04)16305-8
Schumann-Werner, B., Dogan, I., Mirzazade, S., Mall, B., Overbeck, R., Honrath, P., et al. (2021). Clinical predictors and neural correlates for compromised swallowing safety in Huntington disease. Eur. J. Neurol. 28, 2855–2862. doi: 10.1111/ene.14953
Seo, N., Nakamura, J., Kaneda, T., Tateno, H., Shimoda, A., Ichiki, T., et al. (2022). Distinguishing functional exosomes and other extracellular vesicles as a nucleic acid cargo by the anion-exchange method. J. Extracell. Vesicles 11:e12205. doi: 10.1002/jev2.12205
Sharma, P., Mesci, P., Carromeu, C., McClatchy, D. R., Schiapparelli, L., and Yates, J. R. III, et al. (2019). Exosomes regulate neurogenesis and circuit assembly. Proc. Natl. Acad. Sci. U.S.A. 116, 16086–16094. doi: 10.1073/pnas.1902513116
Sheykhhasan, M., Amini, R., Soleimani Asl, S., Saidijam, M., Hashemi, S. M., and Najafi, R. (2022). Neuroprotective effects of coenzyme Q10-loaded exosomes obtained from adipose-derived stem cells in a rat model of Alzheimer’s disease. Biomed. Pharmacother. 152:113224. doi: 10.1016/j.biopha.2022.113224
Shu, S., Allen, C. L., Benjamin-Davalos, S., Koroleva, M., MacFarland, D., Minderman, H., et al. (2021). A Rapid exosome isolation using ultrafiltration and size exclusion chromatography (REIUS) method for exosome isolation from melanoma cell lines. Methods Mol. Biol. 2265, 289–304. doi: 10.1007/978-1-0716-1205-7_22
Shu, S., Yang, Y., Allen, C. L., Hurley, E., Tung, K. H., Minderman, H., et al. (2020). Purity and yield of melanoma exosomes are dependent on isolation method. J. Extracell. Vesicles 9:1692401. doi: 10.1080/20013078.2019.1692401
Skliar, M., Chernyshev, V. S., Belnap, D. M., Sergey, G. V., Al-Hakami, S. M., Bernard, P. S., et al. (2018). Membrane proteins significantly restrict exosome mobility. Biochem. Biophys. Res. Commun. 501, 1055–1059. doi: 10.1016/j.bbrc.2018.05.107
Smith, Z. J., Lee, C., Rojalin, T., Carney, R. P., Hazari, S., Knudson, A., et al. (2015). Single exosome study reveals subpopulations distributed among cell lines with variability related to membrane content. J. Extracell. Vesicles 4:28533. doi: 10.3402/jev.v4.28533
Song, Y., Li, Z., He, T., Qu, M., Jiang, L., Li, W., et al. (2019). M2 microglia-derived exosomes protect the mouse brain from ischemia-reperfusion injury via exosomal miR-124. Theranostics 9, 2910–2923. doi: 10.7150/thno.30879
Sonvico, F., Clementino, A., Buttini, F., Colombo, G., Pescina, S., Staniscuaski Guterres, S., et al. (2018). Surface-modified nanocarriers for nose-to-brain delivery: from bioadhesion to targeting. Pharmaceutics 10:34. doi: 10.3390/pharmaceutics10010034
Stuendl, A., Kunadt, M., Kruse, N., Bartels, C., Moebius, W., Danzer, K. M., et al. (2016). Induction of alpha-synuclein aggregate formation by CSF exosomes from patients with Parkinson’s disease and dementia with Lewy bodies. Brain 139(Pt. 2), 481–494. doi: 10.1093/brain/awv346
Subra, C., Laulagnier, K., Perret, B., and Record, M. (2007). Exosome lipidomics unravels lipid sorting at the level of multivesicular bodies. Biochimie 89, 205–212. doi: 10.1016/j.biochi.2006.10.014
Sun, D., Zhuang, X., Xiang, X., Liu, Y., Zhang, S., Liu, C., et al. (2010). A novel nanoparticle drug delivery system: the anti-inflammatory activity of curcumin is enhanced when encapsulated in exosomes. Mol. Ther. 18, 1606–1614. doi: 10.1038/mt.2010.105
Szatanek, R., Baj-Krzyworzeka, M., Zimoch, J., Lekka, M., Siedlar, M., and Baran, J. (2017). The methods of choice for extracellular vesicles (EVs) characterization. Int. J. Mol. Sci. 18:1153. doi: 10.3390/ijms18061153
Tang, B., Zeng, W., Song, L. L., Wang, H. M., Qu, L. Q., Lo, H. H., et al. (2022). Extracellular vesicle delivery of neferine for the attenuation of neurodegenerative disease proteins and motor deficit in an Alzheimer’s disease mouse model. Pharmaceuticals 15:83. doi: 10.3390/ph15010083
Taylor, H. A., Przemylska, L., Clavane, E. M., and Meakin, P. J. (2022). BACE1: more than just a beta-secretase. Obes. Rev. 23:e13430. doi: 10.1111/obr.13430
Teng, F., and Fussenegger, M. (2020). Shedding light on extracellular vesicle biogenesis and bioengineering. Adv. Sci. 8:2003505. doi: 10.1002/advs.202003505
Thery, C., Witwer, K. W., Aikawa, E., Alcaraz, M. J., Anderson, J. D., Andriantsitohaina, R., et al. (2018). Minimal information for studies of extracellular vesicles 2018 (MISEV2018): a position statement of the international society for extracellular vesicles and update of the MISEV2014 guidelines. J. Extracell. Vesicles 7:1535750. doi: 10.1080/20013078.2018.1535750
Thery, C., Zitvogel, L., and Amigorena, S. (2002). Exosomes: composition, biogenesis and function. Nat. Rev. Immunol. 2, 569–579. doi: 10.1038/nri855
Thomas, B. L., Eldridge, S. E., Nosrati, B., Alvarez, M., Thorup, A. S., Nalesso, G., et al. (2021). WNT3A-loaded exosomes enable cartilage repair. J. Extracell. Vesicles 10:e12088. doi: 10.1002/jev2.12088
Toth, E. A., Turiak, L., Visnovitz, T., Cserep, C., Mazlo, A., Sodar, B. W., et al. (2021). Formation of a protein corona on the surface of extracellular vesicles in blood plasma. J. Extracell. Vesicles 10:e12140. doi: 10.1002/jev2.12140
Vader, P., Mol, E. A., Pasterkamp, G., and Schiffelers, R. M. (2016). Extracellular vesicles for drug delivery. Adv. Drug Deliv. Rev. 106(Pt. A), 148–156. doi: 10.1016/j.addr.2016.02.006
van der Pol, E., Coumans, F. A., Grootemaat, A. E., Gardiner, C., Sargent, I. L., Harrison, P., et al. (2014). Particle size distribution of exosomes and microvesicles determined by transmission electron microscopy, flow cytometry, nanoparticle tracking analysis, and resistive pulse sensing. J. Thromb. Haemost. 12, 1182–1192. doi: 10.1111/jth.12602
van Niel, G., D’Angelo, G., and Raposo, G. (2018). Shedding light on the cell biology of extracellular vesicles. Nat. Rev. Mol. Cell Biol. 19, 213–228. doi: 10.1038/nrm.2017.125
Veerman, R. E., Teeuwen, L., Czarnewski, P., Gucluler Akpinar, G., Sandberg, A., Cao, X., et al. (2021). Molecular evaluation of five different isolation methods for extracellular vesicles reveals different clinical applicability and subcellular origin. J. Extracell. Vesicles 10:e12128. doi: 10.1002/jev2.12128
Vega-Villa, K. R., Takemoto, J. K., Yanez, J. A., Remsberg, C. M., Forrest, M. L., and Davies, N. M. (2008). Clinical toxicities of nanocarrier systems. Adv. Drug Deliv. Rev. 60, 929–938. doi: 10.1016/j.addr.2007.11.007
Venturini, A., Passalacqua, M., Pelassa, S., Pastorino, F., Tedesco, M., Cortese, K., et al. (2019). Exosomes from astrocyte processes: signaling to neurons. Front. Pharmacol. 10:1452. doi: 10.3389/fphar.2019.01452
Vinsant, S., Mansfield, C., Jimenez-Moreno, R., Del Gaizo Moore, V., Yoshikawa, M., Hampton, T. G., et al. (2013). Characterization of early pathogenesis in the SOD1(G93A) mouse model of ALS: part I, background and methods. Brain Behav. 3, 335–350. doi: 10.1002/brb3.143
Visan, K. S., Lobb, R. J., Ham, S., Lima, L. G., Palma, C., Edna, C. P. Z., et al. (2022). Comparative analysis of tangential flow filtration and ultracentrifugation, both combined with subsequent size exclusion chromatography, for the isolation of small extracellular vesicles. J. Extracell. Vesicles 11:e12266. doi: 10.1002/jev2.12266
Walker, S., Busatto, S., Pham, A., Tian, M., Suh, A., Carson, K., et al. (2019). Extracellular vesicle-based drug delivery systems for cancer treatment. Theranostics 9, 8001–8017. doi: 10.7150/thno.37097
Walkey, C. D., and Chan, W. C. (2012). Understanding and controlling the interaction of nanomaterials with proteins in a physiological environment. Chem. Soc. Rev. 41, 2780–2799. doi: 10.1039/c1cs15233e
Wang, H., Sui, H., Zheng, Y., Jiang, Y., Shi, Y., Liang, J., et al. (2019). Curcumin-primed exosomes potently ameliorate cognitive function in AD mice by inhibiting hyperphosphorylation of the Tau protein through the AKT/GSK-3beta pathway. Nanoscale 11, 7481–7496. doi: 10.1039/c9nr01255a
Wang, S., Cesca, F., Loers, G., Schweizer, M., Buck, F., Benfenati, F., et al. (2011). Synapsin I is an oligomannose-carrying glycoprotein, acts as an oligomannose-binding lectin, and promotes neurite outgrowth and neuronal survival when released via glia-derived exosomes. J. Neurosci. 31, 7275–7290. doi: 10.1523/JNEUROSCI.6476-10.2011
Wong, H. L., Wu, X. Y., and Bendayan, R. (2012). Nanotechnological advances for the delivery of CNS therapeutics. Adv. Drug Deliv. Rev. 64, 686–700. doi: 10.1016/j.addr.2011.10.007
Wu, J. Y., Li, Y. J., Hu, X. B., Huang, S., and Xiang, D. X. (2021). Preservation of small extracellular vesicles for functional analysis and therapeutic applications: a comparative evaluation of storage conditions. Drug Deliv. 28, 162–170. doi: 10.1080/10717544.2020.1869866
Wu, X. Y., Liao, B. Y., Xiao, D., Wu, W. C., Xiao, Y., Alexander, T., et al. (2022). Encapsulation of bryostatin-1 by targeted exosomes enhances remyelination and neuroprotection effects in the cuprizone-induced demyelinating animal model of multiple sclerosis. Biomater. Sci. 10, 714–727. doi: 10.1039/d1bm01142a
Xi, X. M., Xia, S. J., and Lu, R. (2021). Drug loading techniques for exosome-based drug delivery systems. Pharmazie 76, 61–67. doi: 10.1691/ph.2021.0128
Xia, X., Wang, Y., and Zheng, J. C. (2022). Extracellular vesicles, from the pathogenesis to the therapy of neurodegenerative diseases. Transl. Neurodegener. 11:53. doi: 10.1186/s40035-022-00330-0
Xu, H., and Xu, B. (2021). BMSC-derived exosomes ameliorate osteoarthritis by inhibiting pyroptosis of cartilage via delivering miR-326 targeting HDAC3 and STAT1//NF-kappaB p65 to chondrocytes. Mediators Inflamm. 2021:9972805. doi: 10.1155/2021/9972805
Xu, M., Feng, T., Liu, B., Qiu, F., Xu, Y., Zhao, Y., et al. (2021). Engineered exosomes: desirable target-tracking characteristics for cerebrovascular and neurodegenerative disease therapies. Theranostics 11, 8926–8944. doi: 10.7150/thno.62330
Xu, W. M., Li, A., Chen, J. J., and Sun, E. J. (2022). Research development on exosome separation technology. J. Membr. Biol. 256, 25–34. doi: 10.1007/s00232-022-00260-y
Yan, C., Chen, J., Wang, C., Yuan, M., Kang, Y., Wu, Z., et al. (2022). Milk exosomes-mediated miR-31-5p delivery accelerates diabetic wound healing through promoting angiogenesis. Drug Deliv. 29, 214–228. doi: 10.1080/10717544.2021.2023699
Yang, D., Zhang, W., Zhang, H., Zhang, F., Chen, L., Ma, L., et al. (2020). Progress, opportunity, and perspective on exosome isolation - efforts for efficient exosome-based theranostics. Theranostics 10, 3684–3707. doi: 10.7150/thno.41580
You, H. J., Fang, S. B., Wu, T. T., Zhang, H., Feng, Y. K., Li, X. J., et al. (2020). Mesenchymal stem cell-derived exosomes improve motor function and attenuate neuropathology in a mouse model of Machado-Joseph disease. Stem Cell Res. Ther. 11:222. doi: 10.1186/s13287-020-01727-2
Yu, R., Li, J., Lin, Z., Ouyang, Z., Huang, X., Reglodi, D., et al. (2020). TAT-tagging of VIP exerts positive allosteric modulation of the PAC1 receptor and enhances VIP neuroprotective effect in the MPTP mouse model of Parkinson’s disease. Biochim. Biophys. Acta Gen. Subj. 1864:129626. doi: 10.1016/j.bbagen.2020.129626
Yu, Y., Li, W., Mao, L., Peng, W., Long, D., Li, D., et al. (2021). Genetically engineered exosomes display RVG peptide and selectively enrich a neprilysin variant: a potential formulation for the treatment of Alzheimer’s disease. J. Drug Target 29, 1128–1138. doi: 10.1080/1061186X.2021.1929257
Yukuyama, M. N., Kato, E. T., Lobenberg, R., and Bou-Chacra, N. A. (2017). Challenges and future prospects of nanoemulsion as a drug delivery system. Curr. Pharm. Des. 23, 495–508. doi: 10.2174/1381612822666161027111957
Yuyama, K., Sun, H., Mitsutake, S., and Igarashi, Y. (2012). Sphingolipid-modulated exosome secretion promotes clearance of amyloid-beta by microglia. J. Biol. Chem. 287, 10977–10989. doi: 10.1074/jbc.M111.324616
Zhang, L., Wu, T., Shan, Y., Li, G., Ni, X., Chen, X., et al. (2021). Therapeutic reversal of Huntington’s disease by in vivo self-assembled siRNAs. Brain 144, 3421–3435. doi: 10.1093/brain/awab354
Zhang, P., Yeo, J. C., and Lim, C. T. (2019). Advances in technologies for purification and enrichment of extracellular vesicles. SLAS Technol. 24, 477–488. doi: 10.1177/2472630319846877
Zhang, T. T., Li, W., Meng, G., Wang, P., and Liao, W. (2016). Strategies for transporting nanoparticles across the blood-brain barrier. Biomater. Sci. 4, 219–229. doi: 10.1039/c5bm00383k
Zhang, Z. X., Roman, G. C., Hong, Z., Wu, C. B., Qu, Q. M., Huang, J. B., et al. (2005). Parkinson’s disease in China: prevalence in Beijing, Xian, and Shanghai. Lancet 365, 595–597. doi: 10.1016/S0140-6736(05)17909-4
Zhao, C., Zhang, J., Hu, H., Qiao, M., Chen, D., Zhao, X., et al. (2018). Design of lactoferrin modified lipid nano-carriers for efficient brain-targeted delivery of nimodipine. Mater. Sci. Eng. C. Mater. Biol. Appl. 92, 1031–1040. doi: 10.1016/j.msec.2018.02.004
Zhao, P., Le, Z., Liu, L., and Chen, Y. (2020). Therapeutic delivery to the brain via the lymphatic vasculature. Nano Lett. 20, 5415–5420. doi: 10.1021/acs.nanolett.0c01806
Zhao, Y., Xiong, S., Liu, P., Liu, W., Wang, Q., Liu, Y., et al. (2020). Polymeric nanoparticles-based brain delivery with improved therapeutic efficacy of ginkgolide B in Parkinson’s disease. Int. J. Nanomed. 15, 10453–10467. doi: 10.2147/IJN.S272831
Zhao, Z., Yang, Y., Zeng, Y., and He, M. (2016). A microfluidic ExoSearch chip for multiplexed exosome detection towards blood-based ovarian cancer diagnosis. Lab. Chip 16, 489–496. doi: 10.1039/c5lc01117e
Keywords: small extracellular vesicles, drug delivery system, neurodegenerative diseases, brain targeting, blood–brain barrier, treatment
Citation: Pan R, Chen D, Hou L, Hu R and Jiao Z (2023) Small extracellular vesicles: a novel drug delivery system for neurodegenerative disorders. Front. Aging Neurosci. 15:1184435. doi: 10.3389/fnagi.2023.1184435
Received: 11 March 2023; Accepted: 30 May 2023;
Published: 19 June 2023.
Edited by:
Alok Raghav, Gachon University, Republic of KoreaReviewed by:
Hossein Mohammad-Beigi, Technical University of Denmark, DenmarkCopyright © 2023 Pan, Chen, Hou, Hu and Jiao. This is an open-access article distributed under the terms of the Creative Commons Attribution License (CC BY). The use, distribution or reproduction in other forums is permitted, provided the original author(s) and the copyright owner(s) are credited and that the original publication in this journal is cited, in accordance with accepted academic practice. No use, distribution or reproduction is permitted which does not comply with these terms.
*Correspondence: Rong Hu, MTM2MDc5NzkwODhAMTYzLmNvbQ==; Zhigang Jiao, amlhb3poaWdhbmc4MDQzQDE2My5jb20=
†These authors have contributed equally to this work and share first authorship
Disclaimer: All claims expressed in this article are solely those of the authors and do not necessarily represent those of their affiliated organizations, or those of the publisher, the editors and the reviewers. Any product that may be evaluated in this article or claim that may be made by its manufacturer is not guaranteed or endorsed by the publisher.
Research integrity at Frontiers
Learn more about the work of our research integrity team to safeguard the quality of each article we publish.