- 1Laboratory of Ischemic and Neurodegenerative Brain Research, Mossakowski Medical Research Institute, Polish Academy of Sciences, Warsaw, Poland
- 2Department of Rehabilitation and Orthopedics, Medical University of Lublin, Lublin, Poland
- 3Department of Pathophysiology, Medical University of Lublin, Lublin, Poland
Aging is an inevitable phenomenon experienced by animals and humans, and its intensity varies from one individual to another. Aging has been identified as a risk factor for neurodegenerative disorders by influencing the composition of the gut microbiota, microglia activity and cognitive performance. The microbiota-gut-brain axis is a two-way communication path between the gut microbes and the host brain. The aging intestinal microbiota communicates with the brain through secreted metabolites (neurotransmitters), and this phenomenon leads to the destruction of neuronal cells. Numerous external factors, such as living conditions and internal factors related to the age of the host, affect the condition of the intestinal microflora in the form of dysbiosis. Dysbiosis is defined as changes in the composition and function of the gut microflora that affect the pathogenesis, progress, and response to treatment of a disease entity. Dysbiosis occurs when changes in the composition and function of the microbiota exceed the ability of the microflora and its host to restore equilibrium. Dysbiosis leading to dysfunction of the microbiota-gut-brain axis regulates the development and functioning of the host’s nervous, immune, and metabolic systems. Dysbiosis, which causes disturbances in the microbiota-gut-brain axis, is seen with age and with the onset of stroke, and is closely related to the development of risk factors for stroke. The review presents and summarizes the basic elements of the microbiota-gut-brain axis to better understand age-related changes in signaling along the microbiota-gut-brain axis and its dysfunction after stroke. We focused on the relationship between the microbiota-gut-brain axis and aging, emphasizing that all elements of the microbiota-gut-brain axis are subject to age-related changes. We also discuss the interaction between microbiota, microglia and neurons in the aged individuals in the brain after ischemic stroke. Finally, we presented preclinical and clinical studies on the role of the aged microbiota-gut-brain axis in the development of risk factors for stroke and changes in the post-stroke microflora.
Introduction
Aging is defined as the time-related diminution and disappearance of the physical activities that all animals and humans on Earth pass through. With the development of modern society, people in both Eastern and Western communities are contemplating finding a way to live longer. In recent decades, due to the improvement of living conditions and the increase in living standards in developed and developing countries, the life span of people has significantly increased. As a consequence, the number of people aging is much greater than before, and the percentage of people over 60 will rise to 22% by the end of 2050 (Zhou et al., 2022). Life expectancy is increasing worldwide and is expected to further improve in most countries beyond 80 by 2040 (Foreman et al., 2018; Bleve et al., 2022). This means a potential increase in the morbidity and prevalence of aging-related diseases, including cardiovascular, musculoskeletal, oncological, and neurological diseases, such as ischemic stroke (Foreman et al., 2018; Bleve et al., 2022). Due to the progressive limitation of physical activity during aging, the human body undergoes a number of pathological changes, such as genomic and proteomic instability, mitochondrial dysfunction, or inflammatory processes (Green et al., 2011; Moskalev et al., 2013; Zhou et al., 2022). Moreover, in today’s society with an inactive lifestyle and an unhealthy diet, the number of diabetics among aging people has increased dramatically with obesity, which has a significant impact on the incidence of strokes (Popa-Wagner et al., 2020). Lifestyle, including addiction to tobacco and alcohol, high-sugar, and fatty diets, as well as some of the internal factors presented above, negatively affect the occurrence, progression, severity, and duration of neurodegenerative changes after stroke (Popa-Wagner et al., 2020).
Metabolic, molecular and structural changes also occur in the brain with age (Fjell and Walhovd, 2010). The hallmarks of an aging brain: are deregulated metabolic state, impaired activity of the neural network, dysregulated activity of neuroglial cells, depletion of stem cells, loss of neurons and cognitive impairment (Mattson and Arumugam, 2018; Baldwin and Greenwood, 2020; Honarpisheh et al., 2022; Zhou et al., 2022). These accelerated phenomena have cognitive consequences in the elderly, which can lead to neurodegenerative diseases such as Alzheimer’s disease and post-stroke brain injury (Fjell and Walhovd, 2010; Baldwin and Greenwood, 2020; Honarpisheh et al., 2022; Zhou et al., 2022). Age-related cognitive decline is a risk factor for developing dementia, depriving the elderly of wellbeing and shortening their lives (Baldwin and Greenwood, 2020).
Recent investigations have shown that changes in the gut microflora in the elderly are associated with neurodegenerative disorders (Honarpisheh et al., 2022; Zhou et al., 2022). The gut-brain axis shows tight connections between the gut and the brain, which is important for the maturation and function of the microglia (Erny et al., 2015; Honarpisheh et al., 2022). Microglia is a group of neuroglial cells that make about 15% of all brain cells (Zhou et al., 2022). As resident macrophage cells, microglia functions as the primary immune defense in the brain. In order to maintain brain homeostasis, the microglia continuously oversees the brain’s microenvironment through its connections with neighboring cells and many factors (Li et al., 2018). During aging, microglia changes from a resting state to an active state and, together with astrocytes, contributes to the development of neurodegenerative diseases (Johnson et al., 2020). Activated microglial cells act in amoeboid stage, produce pro-inflammatory cytokines, blocking amyloid clearance, and are involved in regulating blood-brain barrier integrity and synaptic plasticity in the older brain (Daria et al., 2017; Yousef et al., 2019; Nguyen et al., 2020). These findings point to a new way to slow and even reverse cognitive aging by influencing the microbiota-microglia cooperation. In this review, we will look at the changes in the gut microflora in the elderly, show the age-related microglia changes, and discuss role of neuroinflammation in an aging brain. Next, we will summarize the influence of microflora on microglia behavior in the aging brain and highlight the importance of the microbiota-microglia relationship in neurodegenerative disorders. This knowledge will certainly enrich our understanding of the relationship between age-related cognitive decline and the microbiota-microglia cooperation, pointing to a possible impact on age-related cognitive decline.
Aging
Due to limited research, little is currently known about the changes in the gut-brain axis with age (Spychala et al., 2018). Aging is associated with loss of nutrient absorption, malnutrition, anorexia, and weakness (Moss et al., 2012). Aging has been shown to be associated with impairment of the intestinal epithelial barrier, loss of intestinal neurons, and altered immune function of the mucosa, resulting in an imbalance in the secretion of pro-inflammatory cytokines (Durgan et al., 2019). Such changes, often referred to as “inflammaging,” can reduce the ability of the elderly to cope with toxic, antigenic, physical and ischemic stress (Durgan et al., 2019). A review of eighteen studies concluded that aging, inflammation, and different microbial compositions may unequivocally contribute to the development of ischemic stroke (Lee et al., 2021). Older mice with a high Firmicutes/Bacteroidetes ratio were documented to have a higher risk of ischemic stroke and were unable to recover from neurological deficits, contributing to higher mortality (Spychala et al., 2018; Sinagra et al., 2021). Aging increases the risk of neurodegenerative, cerebrovascular and cardiovascular diseases and cancer. Mechanisms underlying biological aging include increased DNA damage, impaired autophagy, telomere shortening, and immunesenescence (Lejault et al., 2020; Conway et al., 2022). More recently, gut microbiota has emerged as a key mediator of aging and age-related pathologies.
Aging and intestinal homeostasis
The function of the intestinal barrier deteriorates with age, which is associated with an increased incidence of gastrointestinal infections in the advanced years (Qi et al., 2017). Loss of barrier normal function is due to a decrease in tight junction proteins and age-related mucus production which are regulated by the microbiota (Parrish, 2017). An example of this is Akkermansia muciniphila which is lower in patients with age-related diseases than in healthy people of the same age (Singh et al., 2019). Akkermansia muciniphila supplementation relieves the age-related reduction in the thickness of the colon mucus (van der Lugt et al., 2019). A probiotic cocktail of human origin isolated from healthy infants, administered to older mice with dysbiosis reduced intestinal permeability by increased activity of bile salt hydrolase and by modulation of the microbiota/taurine/tight junction axis (Ahmadi et al., 2020). These observations suggest that age-related intestinal barrier dysfunction can be prevented by using microbiota-based therapies.
Secretion of interleukin-22, which stimulates the regenerative abilities of crypt stem cells, decreases with age (Lindemans et al., 2015; de Haan and Lazare, 2018). Generation of interleukin-22 by innate intestinal lymphoid cells is induced by microbial metabolites such as indole-3-carboxaldehyde and aryl hydrocarbon receptor ligand derived from tryptophan (Zelante et al., 2013; Lindemans et al., 2015). The ability of the microflora to synthesize indoles gradually decreases with age (Ruiz-Ruiz et al., 2020). These observations indicate that the deficiency of indole-based metabolites noted with age reduces the production of interleukin-22 dependent on innate lymphoid cells, which limits the regenerative capacity of crypt stem cells (Honarpisheh et al., 2022). Short-chain fatty acids inhibit stem cell proliferation by inhibiting FoxP3-dependent histone deacetylase (Kaiko et al., 2016). Of course, colon crypts protect stem cells from metabolites derived from the microflora (Kaiko et al., 2016). Fecal short-chain fatty acids levels decline with age, which explains the increase in crypt stem cells seen with age (Salazar et al., 2019). Aging mice have been found to have decreased mucus barrier thickness, impaired M-cell antigen processing, and decreased number of specialized plasma cells (Sovran et al., 2019). Thus, as a result of age-related changes and stroke-induced dysbiosis, the microflora can penetrate the epithelium and initiate inflammation (Honarpisheh et al., 2022).
Aging is associated with remodeling of the immune system and low-grade chronic inflammation (inflammaging) resulting in uncontrolled immunosenescence and the development of chronic and degenerative diseases (Bleve et al., 2022). Immunosenescence is characterized by decreased immune efficacy against infection, impaired effector response to new antigens, increased autoimmunity and poor wound healing (Bleve et al., 2022). Inflammaging is often viewed as a consequence of inflammation often associated with lifestyle and unhealthy diet during the living period (Popa-Wagner et al., 2020), characterized by elevated levels of pro-inflammatory cytokines in the serum such as IL-1β, IL-6, IL-8, and TNF-α, and decreased levels of anti-inflammatory cytokines such as IL-10 (Conway et al., 2022).
Consistent with documented increased proliferation and tissue-redistribution of myeloid cells with aging, it was shown that peripherally sourced myeloid antigen-presenting cells significantly increase in the brain with advancing age (Honarpisheh et al., 2020a). The accumulation of myelocytes in the brain positively correlated with reduced Akkermansia muciniphila, age-related changes in the intestinal microflora and behavioral deficits (Honarpisheh et al., 2020a). Although the proportion of neutrophils in the peripheral circulation does not change, their functions such as phagocytosis, chemotaxis, and the production of reactive oxygen species are disturbed with age (Roy-O’Reilly et al., 2020; Conway et al., 2022). Aging exacerbates neutrophil pathogenicity in ischemic stroke (Roy-O’Reilly et al., 2020). Microbiota enhances neutrophil aging, and depletion of microflora reduced circulating older neutrophils and improved survival in animal model of endotoxin-induced septic shock (Zhang et al., 2015).
Age-related changes in the T cell population are characterized by a decreased number of naive T and Treg cells and increased CD8+ memory cells (McKenna et al., 2001). Age-related changes in B cells have been noted to be associated with a decrease in the precursors of the B cell lineage (McKenna et al., 2001). With age, peripheral B cell phenotypes shift from naive B cells toward B cells experiencing an antigen of limited clonal diversity with impaired ability to generate new high affinity immunoglobulins (Frasca and Blomberg, 2011). B lymphocytes accumulate with age in the blood and meninges and undergo an excessive proliferative response upon stimulation with toll-like receptor 9 and toll-like receptor 7 ligands (Rubtsov et al., 2011; Brioschi et al., 2021). Studies have shown that the marked shift in lymphocyte proliferation toward marrow cell proliferation with aging in pathogen-free mice is abolished (Krambs et al., 2021). There is an expansion of B cells in germ free mice, suggesting that microbiota signals suppress B lymphopoiesis with aging in mice (Krambs et al., 2021). Also, Akkermansia muciniphila supplementation in the accelerated aging model reduced the number of activated B lymphocytes in Peyer’s patches (van der Lugt et al., 2019). This suggests an involvement of the gut microbiota in the regulation of lymphocyte proliferation and suggests a possible therapy for lymphocyte dominant diseases in combination with approved B-cell depleting drugs (Honarpisheh et al., 2022).
The loss of microflora caused by antibiotics or in germ free models showed a harmful role of microflora in ischemic stroke (Pluta et al., 2021a, Honarpisheh et al., 2022). Lymphatic drainage of brain antigens decreases with age, suggesting that exposure of brain-derived antigens to border-bound macrophages and other antigen-presenting cells may be prolonged in old age compared to young stroke patients (Louveau et al., 2018; Rustenhoven et al., 2021; Honarpisheh et al., 2022). Metagenomic data indicate that increased abundance of Akkermansia muciniphila and butyrate production are responsible for the rejuvenation of the immune system seen in older mice (Shin et al., 2021). This suggests that the microflora can stimulate age-related inflammation of the central nervous system, regardless of the age of the host, and may contribute to the onset of neuroinflammation and reduce amyloid and tau protein pathology after ischemic stroke (Spychala et al., 2018; Kim et al., 2020; Pluta et al., 2021a). There is evidence that some Enterobacter species and fungi produce amyloid peptides or amyloid fibers associated with seed formation for amyloid aggregation in the brain (Lundmark et al., 2005; Zhou et al., 2012; Evans et al., 2015; Pluta et al., 2021a). Microbial amyloids induce nucleation of amyloid aggregates with high accumulation and an inflammatory response (Chen et al., 2016; Friedland and Chapman, 2017). In the absence of gut microflora, a reduction in amyloid accumulation was observed in the transgenic mice (Harach et al., 2017). It has also been noted that in vitro amyloid aggregation is inhibited by short chain fatty acids produced by the intestinal microflora (Ho et al., 2018). Additionally, bacterial endotoxin is responsible for inflammation of the central nervous system, causing the development of amyloid fibrils (Asti and Gioglio, 2014; Syed and Boles, 2014). Certain bacteria, such as Escherichia coli, produce amyloid, but the link between bacterial amyloid origin and post-ischemic neurodegeneration in the brain has not been definitively elucidated (Syed and Boles, 2014; Pluta et al., 2021a). Bacterial gram-negative lipopolysaccharide has been found to promote amyloid deposition in the brains of mice, adversely affecting cognition (Lee et al., 2008). It is not known how battery amyloids interact with other post-ischemic neuropathological elements such as post-translational changes in tau protein, production of β-amyloid peptides, neuroinflammation, and development of cerebral amyloid angiopathy (Kato et al., 1988; Pluta et al., 1994a, 2016a,b, 2018a,b, 2020a, 2021b, Pluta, 2000; Wen et al., 2007; Kocki et al., 2015; Ułamek-Kozioł et al., 2016, 2017, 2019; Khan et al., 2018; Hatsuta et al., 2019; Radenovic et al., 2020; Rost et al., 2022). As the permeability of the intestinal barrier increases with age, bacterial-derived amyloids enter the systemic circulation, aggravating inflammation in the brain and causing memory loss in mice (Jang et al., 2018; Pluta et al., 2020b). These data suggest that bacterial-derived amyloids play a significant role in enhancing the immune response and nucleation of amyloid aggregates in the brain following ischemia (Pluta et al., 2021a). Microbiome generated amyloid has a potential effect on amyloidogenesis in neurodegenerative disorders such as post-ischemic brain injury (Zhao and Lukiw, 2015; Pluta et al., 2021a). These suggestions have recently been strongly supported by in vivo and in vitro data (Lee et al., 2018; Zhao et al., 2018). By acting on brain gliosis, microbial amyloids are able to control brain neuroinflammation and levels of β-amyloid peptides (Zhao and Lukiw, 2015; Lee et al., 2018; Zhao et al., 2018). The altered bacterial flora influences the level of bacterial amyloid and metabolites in the blood and therefore may act as a trigger for the onset and exacerbation of neurodegeneration in the brain following ischemia (Pluta et al., 2020b, 2021a, Honarpisheh et al., 2022). Data from meta-analysis indicate that intestinal metabolites may increase inflammation, amyloid and tau protein aggregation in neurodegenerative diseases of the brain (Xu and Wang, 2016). The gut microbiota is involved in the secretion of over 100 metabolites, but their involvement in post-ischemic brain neuropathogenesis has not been definitively proven (Bostanciklioglu, 2019; Pluta et al., 2021a, Honarpisheh et al., 2022). It has been shown that valerian, isovaleric, isobutyric, butyric, propionic, acetic, and formic acids influence the development of neurodegenerative disorders by affecting the activity of astrocytes and microglia and reducing inflammation, amyloid and tau protein aggregation (Cummings et al., 1987; Macfarlane and Macfarlane, 2012). The host gut microflora has been found to influence microglial homeostasis in the brain, resulting in an immature microglial phenotype in germ-free animals, ultimately weakening the innate immune response (Erny et al., 2015). Attempts to re-colonize by the complex microflora partially restored the physiological features of the microglia (Erny et al., 2015). Taken together, these observations suggest that the gut microflora can control microglia maturation, function and activation, and therefore, in the case of impaired intestinal microflora, the maturation of the microglia and its phagocytic potential for amyloid and tau protein that accumulate after ischemia are limited (Kato et al., 1988; Pluta et al., 1994a, 2009, 2021a, Pluta, 2000; van Groen et al., 2005; Khan et al., 2018; Hatsuta et al., 2019; Honarpisheh et al., 2022). In vitro studies have shown that propionic, valeric and butyric acids inhibit the oligomerization of β-amyloid peptide 1-40 (Ho et al., 2018). After assessing the effect of intestinal microflora metabolites on β-amyloid peptide 1-42 aggregation, it was shown that only valeric acid inhibited the development of amyloid oligomers (Ho et al., 2018). Regarding the conversion of β-amyloid peptides into β-amyloid peptide fibrils, it was shown that both butyric and valeric acid inhibited the conversion of β-amyloid peptide 1-40 monomer to filamentous β-amyloid peptide (Ho et al., 2018). The dependence on the type of intestinal metabolite indicates that an increase in the amount of beneficial metabolites produced by the gut flora, especially anti-inflammatory bacteria, may aid in the removal of tau protein and amyloid from brain tissue following ischemia (Pluta et al., 2021a).
As much as 50% of the aging population suffer from chronic constipation and gastrointestinal problems (Honarpisheh et al., 2022). A 7-year clinical trial with over 3 million veterans found that a higher rate of constipation is independently associated with a higher risk of all-cause mortality, including ischemic stroke (Sumida et al., 2019). Investigations have shown that aging reduces the contractility of the intestinal smooth muscles (O’Mahony et al., 2002), their innervation (Phillips and Powley, 2007), and slows the intestinal transit time (Kim, 2017), suggesting age-related dysfunction of the autonomic nervous system that may be a consequence of changes in the microbiota and exacerbate complications after a stroke, such as a bowel obstruction.
Age-related changes in the functioning of the autonomic nervous system include diminished neurotransmitter activity, leading to impaired cerebral blood flow regulation and poorly controlled autonomic secretion, e.g., bladder function (Hotta and Uchida, 2010). Resting sympathetic tone increases with age, which is associated with increased blood levels of norepinephrine and decreased sensitivity of catecholamine and cholinergic receptors (Hotta and Uchida, 2010), indicating age-related dysfunction of the cholinergic anti-inflammatory pathway post-stroke (Honarpisheh et al., 2022). Fecal microflora transplantation from rats with spontaneous hypertension to rats with normal blood pressure increases sympathetic activity, blood pressure and inflammation of the central nervous system (Toral et al., 2019), suggesting that the microflora may mediate sympathetic pathophysiology observed with aging and post-ischemic stroke (Honarpisheh et al., 2022).
The neurons of the intestinal nervous system undergo age-related neurodegeneration (Saffrey, 2013). The loss of intestinal neurons with age reduces the density of the nerve fibers in the intestinal mucosa (Peck et al., 2009). Aging gut neurons show swollen and dystrophic features, contain excessive accumulation of lipofuscin, and have a higher generation of reactive oxygen species in the muscle plexus (Jurk et al., 2012). Age-related reductions in intestinal glial cells, proportional to neuronal loss, have also been demonstrated (Phillips et al., 2004). Although there are communication pathways between the microbiota and the intestinal nervous system, the direct involvement of age-related dysbiosis in the neurodegeneration of the intestinal nervous system has not been definitively elucidated (Honarpisheh et al., 2022).
In summary, all components of the microbiota-gut-brain axis undergo significant age-related changes, many of which may be directly influenced or even stimulated by microflora (Honarpisheh et al., 2022). Given the above data and the fact that ischemic stroke is a disease of the elderly, studies of the pathophysiology and sequence of stroke must take into account age-related changes in the host and its microflora, including dysbiosis, gastrointestinal dysfunction, immune remodeling, and other existing pathologies of central nervous system and systemic such as diabetes, atherosclerosis, and obesity (Popa-Wagner et al., 2020; Honarpisheh et al., 2022).
Influence of the gut microbiome on brain homeostasis and aging
It has been shown that, in addition to healthy neuronal development, there is increasing evidence that the physiological gut microflora also strongly influences brain function and its architecture under homeostatic conditions and during normal aging (Honarpisheh et al., 2022). It has been shown that the integrity of the blood-brain barrier in the hippocampus, striatum and frontal cortex is influenced by the physiological gut microflora (Honarpisheh et al., 2022). It was noted that germ-free mice have an increased permeability of the blood-brain barrier, a phenomenon that is already visible during embryonic development due to the weakening of tight junctions connections due to reduced occludin and claudin 5 activity (Braniste et al., 2014). Re-colonization of germ-free mice with physiological gut microflora or the use of the short-chain fatty acid, butyrate, restored the integrity of the blood-brain barrier. Recently, the host microbiota has been shown to control microglia maturation and the brain’s innate immune function (Honarpisheh et al., 2022). Germ-free adult mice show inhibition of microglia growth under homeostatic conditions compared to microglia in pathogen-free mice (Erny et al., 2015). In addition, it is known that short-chain fatty acids derived from microbes can cross the blood-brain barrier (Huuskonen et al., 2004). The oral administration of a mixture of butyrate, acetate and propionate stimulated the maturation of the microglial cells, indicating that they can directly affect the microglia (Erny et al., 2015). In addition, physiological microbiota have been shown to influence neurogenesis in the hippocampus of adult mice, leading to more immature neuronal cells in germ-free mice, but not in conventionally colonized mice (Marín-Burgin and Schinder, 2012; Ogbonnaya et al., 2015). Normal aging has been found to be characterized by a progressive decline in cognitive function associated with changes in the gut microflora of these individuals (Partridge, 2001; Claesson et al., 2011). It is still not fully understood how aging and dysbiosis of the intestinal microflora, the characteristic elements of aging, change the plasticity and homeostasis of immune cells in the brain. The plasticity of brain immune cells influences the homeostasis of brain parenchyma as well as diseases and is strongly dependent on the microenvironment and systemic factors. Blood metabolites and cytokines have been shown to be altered by intestinal dysbiosis leading to dysregulation of the peripheral immune system (Arpaia et al., 2013; Bachem et al., 2019; Lehallier et al., 2019). Brain immunity and central nervous system inflammation are also indirectly altered by dysbiosis via signaling metabolites (neurotransmitters) produced by the intestinal microflora (Table 1; Erny et al., 2015; Dinan and Cryan, 2017; Ma et al., 2019). Some of the major microbial metabolites (neurotransmitters) and their role in brain health are presented in Table 1. There is evidence to suggest that the gut microflora plays an important role in gut-brain communication, ultimately shaping the aging of the central nervous system while helping to maintain nervous system function in old age, or promoting pathology by placing the gut microflora as a judge of age-related neurological deterioration (Madison and Kiecolt-Glaser, 2021). Dysbiosis of the gut microflora can induce abnormal immune responses, which in turn disrupt systemic and local homeostasis of the host, and existing evidence points to the importance of the gut microflora in age-related central nervous system disease such as ischemic stroke (Pluta et al., 2021a, Honarpisheh et al., 2022). Various research systems have used the manipulation of the senile microbiome through fecal microbiota transplantation, administration of prebiotics and probiotics, and dietary interventions to reduce age-related dysbiosis (Holmes et al., 2020). Cellular indexing of transcriptomes and epitopes by sequencing revealed transcriptional changes among inflammatory Ly6C+ monocytes and innate lymphoid cells associated with the central nervous system (Honarpisheh et al., 2022). Demonstrating the plasticity of immune cells during aging and intestinal dysbiosis can help learn about the critical components that determine the brain’s immunity during aging, and determine the onset of age-related neurodegenerative disorders (Golomb et al., 2020). Studies on the effect of aging intestinal microflora on cognitive decline by transplanting fecal microflora from older to juvenile rats have shown that transplanting fecal microflora impairs cognitive behavior in young recipient rats, leading to reduced regional homogeneity in the medial prefrontal cortex and the hippocampus, decreased neurotrophic expression factor of brain origin, N-methyl-D-aspartate receptor NR1 subunit and synaptophysin in synaptic structures (Li et al., 2020). In addition, young rats showed elevated levels of oxidative stress and pro-inflammatory cytokines following fecal microflora transplantation, indicating that oxidative stress and neuroinflammation may underlie gut-related cognitive decline during aging (Schächtle and Rosshart, 2021).
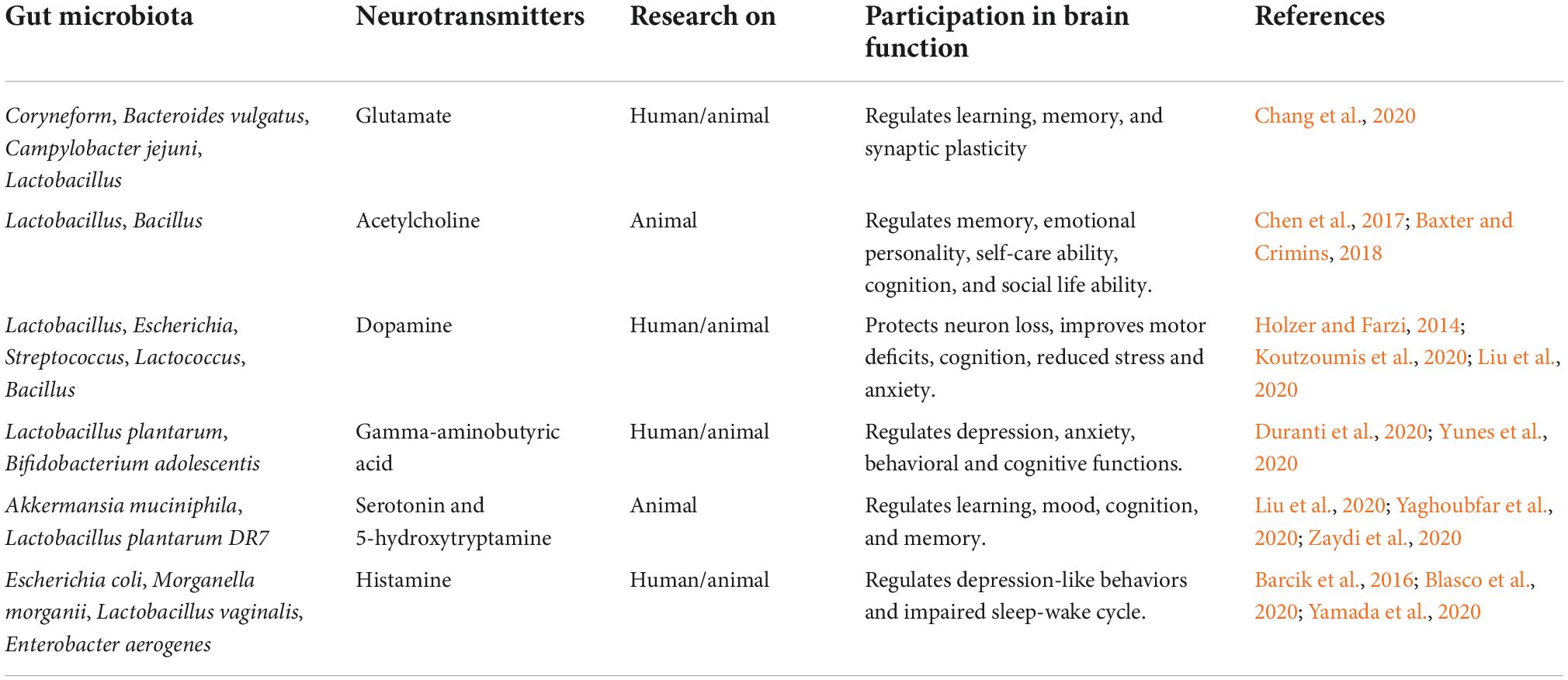
Table 1. Neurotransmitters (metabolites) made by the intestinal microbiota and their role in the functioning of the brain.
In summary, these studies indicate a significant role of the intestinal microflora in the maintenance of neuronal homeostasis as well as in normal and pathological aging. A well-balanced equilibrium between the gut microflora and the host seems to be essential for maintaining the health of neuronal cells, while meaningful disturbances in this relationship can lead to the development of various mental and neurological disease entities.
Post-ischemic pathology of the brain
Post-ischemic brain neurodegeneration in rodents and humans is characterized by the accumulation and deposition of misfolded amyloid in the form of amyloid plaques and the aggregation of misfolded tau protein in the form of neurofibrillary tangles, neuroinflammation as well as brain atrophy, ultimately leading to cognitive decline and dementia (Kato et al., 1988; Pluta et al., 1994a, 2009, 2018b, 2021b; Jendroska et al., 1995, 1997; Pluta, 2000; van Groen et al., 2005; Qi et al., 2007; Wen et al., 2007; Gemmell et al., 2012, 2014; Sekeljic et al., 2012; Khan et al., 2018; Hatsuta et al., 2019; Radenovic et al., 2020; Rost et al., 2022). Amyloid monomers aggregate into soluble, low molecular weight oligomers that spread throughout the brain and then aggregate into insoluble amyloid fibrils, gradually forming amyloid plaques (van Groen et al., 2005). Aggregation of the tau protein into intracellular neurofibrillary tangles is supported by various post-translational modifications such as hyperphosphorylation (Kato et al., 1988; Wen et al., 2007; Khan et al., 2018; Pluta et al., 2018b, 2021b; Hatsuta et al., 2019). An imbalance in the production and removal of amyloid from the brain following ischemia indicates that the brain favors the pathological process of amyloid aggregation (Pluta et al., 1994a, 2009; Pluta, 2000). Since the interstitial fluid, cerebral spinal fluid contributes to amyloid removal, amyloid and tau protein can be observed in both cerebral spinal fluid and peripheral blood serum (Pluta et al., 2011; Rickenbach and Gericke, 2022). In the brain, amyloid is locally degraded by enzymes in cells residing in the brain, including microglia and astrocytes, which help to reduce the amyloid load in the brain (Rickenbach and Gericke, 2022). Since the amyloid protein precursor is also present in the cell membranes of other organs and tissues, peripherally produced amyloid contributes to the total amyloid pool (Pluta et al., 1996; Rickenbach and Gericke, 2022). At the periphery, amyloid is cleared by monocytes, macrophages, and neutrophils through phagocytosis and endocytosis (Rickenbach and Gericke, 2022). The brain amyloid load is also reduced by sequestration of soluble amyloid in the blood by anti-amyloid antibodies and low density lipoprotein receptor-related protein 1 through a peripheral “sink” effect (Rickenbach and Gericke, 2022).
Experimental data show the involvement of the adaptive immune system in the post-ischemic brain, which is associated with an increase in CD4 and CD8 of T cells in the brain (Sekeljic et al., 2012). To date, it is not fully understood whether the extravasation of T lymphocytes to the brain is the result of ischemic damage to the brain-blood barrier and microbleeds, or whether T lymphocytes are specially recruited to the brain by recognizing brain-derived antigens (Rost et al., 2022). Studies in murine models of β-amyloidosis showed that although microhemorrhages contributed to non-specific T cell extravasation, specific infiltration of T cells into the parenchyma of the brain was mainly associated with amyloidosis and tau protein pathology (Rickenbach and Gericke, 2022). Alternatively, new T-cell antigens, so-called neo-epitopes, can be created by splicing mechanisms during processing in antigen presenting cells (Rickenbach and Gericke, 2022).
The immune receptor TREM2 is required for microglia responses, including microglia accumulation in proximity to amyloid deposition and amyloid plaque phagocytosis. Treatment with an anti-TREM2 agonist antibody has been shown to promote microglia activation and induce phagocytosis of amyloid in murine models of β-amyloidosis, reducing the amount of amyloid plaques and neuronal damage (Wang et al., 2020). Anti-amyloid and tau protein autoantibodies are naturally present in the serum and cerebrospinal fluid of Alzheimer’s disease patients (Rickenbach and Gericke, 2022) and should be expected to after cerebral ischemia. A potential neuroprotective role and a relationship between autoantibody levels and the progression of brain neurodegeneration following ischemia are to be expected.
Ischemic stroke and the intestinal microflora
Currently, ischemic stroke is the leading cause of death and long-term disability worldwide (Benjamin et al., 2018). A stroke is caused by a blood clot or embolism in the arteries that supply the brain with blood (Pluta et al., 1994b, Benjamin et al., 2018). Ischemic stroke survival has dramatically improved with advances in emergency treatment with intravenous thrombolysis or intravascular thrombectomy (Powers et al., 2019). Due to the exclusion criteria for post-stroke time, risk of hemorrhage, and the need for specialized resources, less than 10% of stroke patients receive this treatment (Rinaldo et al., 2019). Poor long-term functional outcomes in stroke survivors are a major factor in lowering quality of life and increasing the socioeconomic burden post-stroke (Gbd 2013 DALYs and Hale Collaborators et al., 2015).
The post-stroke infection rate is around 30%, and the development of post-stroke infection is associated with poor recovery outcomes (Popović et al., 2013). About 50% of post-stroke patients also experience gastrointestinal complications, including gastrointestinal bleeding, dysbiosis and loss of peristalsis resulting in longer hospital stays, higher mortality, and poorer neurological outcomes (Camara-Lemarroy et al., 2014). Signaling phenomena caused by stroke do not end with disturbing gastrointestinal homeostasis and microflora (Honarpisheh et al., 2022). The signaling wave from the gastrointestinal tract spreads toward the brain, causing the subacute and chronic phase of the response to the stroke (Honarpisheh et al., 2022). Above, it was shown how dysbiosis and aging affect microbiota-gut-brain axis signaling, playing a detrimental but potentially reversible role in stroke neurological outcomes. Although stroke is a disease of aging, but the majority of preclinical research has been conducted in young animals.
Numerous studies have shown that infarct volume is smaller after reversible middle cerebral artery occlusion in older animals compared to matched young, even with the same duration of ischemia and post-ischemic survival (Shapira et al., 2002; Liu et al., 2009; Liu and McCullough, 2011; Sieber et al., 2011). Despite having a smaller volume of infarction, older rodents have poorer neurological outcomes and higher mortality compared to younger rodents (Shapira et al., 2002; Liu et al., 2009; Liu and McCullough, 2011; Sieber et al., 2011). On the other hand, the few studies using photochemically induced local cerebral ischemia have shown larger infarcts in older animals (Kharlamov et al., 2000; Wang et al., 2003). In addition to age, the infarct volume after an experimental stroke is influenced by many factors, including the species studied, reperfusion after ischemia vs. permanent ischemia, and existing differences in collateral circulation. Thus, in most studies that used the reversible middle cerebral artery occlusion model, older rodents had less severe brain edema and smaller infarcts, but showed higher mortality and poorer neurological outcomes post-stroke compared to young controls (Shapira et al., 2002; Liu et al., 2009; Liu and McCullough, 2011; Sieber et al., 2011).
Stroke risk factors include both intra- and inter-individual changes in the intestinal microflora (Fei et al., 2019). It has been shown that a metabolic shift of tryptophan metabolism from a microbiota-regulated indole pathway toward a host-regulated kynurenine pathway may have a detrimental effect on the development of cerebral atherosclerosis, a major risk factor for stroke (Wikoff et al., 2009; Cason et al., 2018).
Risk factors for ischemic stroke such as obesity and diabetes (Popa-Wagner et al., 2020) are transmitted through fecal microflora transplantation (Honarpisheh et al., 2022). Targeting the microflora to reduce metabolic disturbances can be used for the clinical control of metabolic risk factors for ischemic stroke. Dysfunction of the microbiota-gut-brain axis contributes to arterial hypertension through metabolites, bacteria in the circulatory system and autonomic system dysregulation (Richards et al., 2022). Evidence suggests that the gut microflora may independently contribute to the development of significant risk factors for ischemic stroke which are modulated or transmitted by the gut microflora (Honarpisheh et al., 2022).
A significant proportion of older post-stroke patients are weak, linked to cerebrovascular disease, and weakness predicts shorter post-stroke survival (Palmer et al., 2019). General weakness is characterized by decreased grip strength, reduced energy, slowness of movement, decreased physical activity, and unintentional weight loss (Fried et al., 2001). A specific feature of the weakness is the loss of microflora diversity and taxonomic changes, including an increase in the number of Eubacterium dolichum and Eggerthella lenta, a decrease in the number of Faecalibacterium prausnitzii, a loss of butyrate producers, a decrease in the metabolism of tryptophan and an increased production of peptidoglycans and lipopolysaccharides (Jackson et al., 2016). There are strong positive associations between microflora diversity and less fragility, which improves immune function and increased cognitive performance (Claesson et al., 2012). Oral administration of Akkermansia muciniphila changed the aging phenotype in the intestines of older mice and prolonged health, as evidenced by the recovery of atrophied muscles (Shin et al., 2021). These observations suggest that identifying microbial changes during aging could be the basis for developing bacteriotherapy for healthy aging and recovery from stroke. A placebo-controlled clinical trial in 55 patients with an overgrowth of bacteria in the small intestine showed that a 4-week treatment with fecal transplant capsules prepared from healthy donors resulted in a significant increase in bacterial diversity and a reduction in gastrointestinal symptoms (Xu et al., 2021a).
An ischemic stroke is a type of focal cerebral ischemia with an ischemic core where neurons die quickly and there is a penumbra on the periphery of that core that remains at risk but can be saved. A damaged core initiates inflammation and apoptosis through glutamate excitotoxicity, changes in calcium levels and oxidative stress (Spychala et al., 2017). Disruption of cerebral blood flow activates endothelial cells and initiates the coagulation cascade and vessel spasm (Pluta et al., 1994b, Wisniewski et al., 1995). Once the blood-brain barrier is opened after stroke, blood-borne immune cells are transmigrated within minutes into ischemic focus (Yilmaz and Granger, 2010; Iadecola et al., 2020).
Age influences the processes involved in the early response to stroke, including the age-related increase in the level of coagulation factors in the blood which leads to procoagulation in the elderly and increases the risk of post-stroke complications (Tschan and Bolliger, 2021). Hemorrhagic transformation of stroke is more common in older mice than in young mice compared to the size of the infarction, which is related to the earlier influx of neutrophils into the aging brain that produce metalloproteinases and this leads to damage of the blood-brain barrier (Ritzel et al., 2018). Subsequently, the recruitment of phagocytes and granulocytes initiates the removal of damaged neuroglia after ischemia (Schilling et al., 2003). Aging alters the immune response to ischemic stroke, so manipulating peripheral immunity by acting on the bone marrow or the microbiota can reverse the age-related response to stroke (Ritzel et al., 2018; Iadecola et al., 2020).
Complete removal of microglial cells worsens post-stroke outcomes, suggesting that microglia plays a beneficial role after stroke, possibly by suppressing neuroinflammation caused by neuroglial damage (Jin et al., 2017). Aging has a significant influence on the microglia response after a stroke (Ritzel et al., 2018; Honarpisheh et al., 2020b, Conway et al., 2022). Aging microglial cells produce less TNF-α but more ROS than young microglia, and old mice with a young bone marrow transplant showed a reduced microglia response after stroke (Ritzel et al., 2018). Also, transplantation of the post-stroke fecal flora of young healthy mice to older mice reduced microglia activation (Lee et al., 2020). These observations indicate that the age-dependent role of the microglia after stroke may be influenced by the manipulation of the intestinal microflora.
Congenital immune response following a stroke of old age
Increased neutrophil infiltration into the brain following stroke was noted in older animals (Ritzel et al., 2018). Aging neutrophils have decreased phagocytosis but produce more reactive oxygen species and metaloproteinaze-9 following stroke (Ritzel et al., 2018; Roy-O’Reilly et al., 2020). The response of macrophages related to the brain environment, blood-derived monocytes and dendritic cells is among the early responses to ischemic stroke. Recruitment of monocyte-derived macrophages via the C-C chemokine receptor 2 is protective in the early stages after stroke, contributing to phagocytic activity and debris removal, however, their continued presence contributes to chronic neuroinflammation (Sekeljic et al., 2012; Radenovic et al., 2020; Honarpisheh et al., 2022). The bone marrow and spleen are the main sources of monocytes and have been shown to have a significant influence on the development of systemic inflammation, including enteritis (Chauhan et al., 2018). But splenectomy protects older mice from ischemic stroke injuries (Chauhan et al., 2018). The observed elevated expression of toll-like receptor 4 on peripheral blood monocytes of stroke patients aggravated stroke and inflammatory lesions, and the same monocytes were also involved in systemic inflammation following stroke (Yang et al., 2008). It is not fully understood whether age-related changes in the microbiota affect neutrophils and monocytes of peripheral origin following stroke. Dendritic cells have been shown to arrive early in the focus of stroke and remain there for several days (Felger et al., 2010). Studies have shown that, under the control of the gut microflora, dendritic cells are involved in the development of inflammation by secreting IL-23, inducing IL-17 expression by γδ T cells, and promoting neutrophil infiltration (Benakis et al., 2016). The data show that the number of antigen presenting cells in the brain before stroke is significantly higher in older mice and is correlated with specific age-related changes in microflora (Honarpisheh et al., 2020b). Studies have shown that more neutrophils are moving into the older brain during 3 days after the stroke (Ritzel et al., 2018). The data indicate that the composition of the myeloid populations in the bone marrow of the skull bones changes with age with a significant increase in the number of neutrophils in the aging skull, which may explain the increased influx of neutrophils into the stroke area in older mice compared to young mice (Cugurra et al., 2021).
Adaptive immune response following a stroke of old age
As a result of an episode of reversible cerebral ischemia with inflammation, the blood-brain barrier, the lymphatic system of the meninges and the blood-cerebrospinal fluid barrier are damaged, which allows the passage of antigens and cytokines of brain origin into the systemic circulation (Bernardo-Castro et al., 2020; Iadecola et al., 2020). In addition, an increased number of circulating lymphocytes has been associated with a higher risk of recurrent stroke and its mortality (Nadareishvili et al., 2004). Increased numbers of T helper 17 cells in the blood are associated with increased cognitive deficits after stroke, suggesting an involvement of antigenic lymphocyte activation in the chronic sequelae of stroke, such as cognitive decline and autoimmunity (Yin and Li, 2011; Swardfager et al., 2014). Indeed, autoreactive B and T lymphocytes are present in the stroke focus as early as 4 days after stroke, while circulating lymphocytes from patients collected 2 weeks after stroke show stronger immunoreactivity to brain antigens than those collected from the control group (Youngchaiyud et al., 1974).
More research is needed to determine whether this age-dependent heterogeneity of myeloid cells and lymphocytes differently contributes to the post-stroke response (Singh et al., 2018). Their proximity, temporal involvement, and specific cellular regulation by the gut microbiota following acute cerebral ischemia, combined with the slower lymph clearance seen with aging, may result in prolonged exposure to new brain antigens, which may contribute to an increased incidence of autoimmunity and long-term, irreversible cognitive decline observed in patients after ischemic stroke (Doyle et al., 2015; Ma et al., 2017; Javidi and Magnus, 2019).
Age worsens post-stroke dysbiosis
The systemic phenomena are involved in post-stroke injury: systemic immune answer, e.g., migration of monocytes and neutrophils in reply to brain signals, activation of the hypothalamic-pituitary-adrenal axis affecting the intestinal barrier function, and activation of the sympathetic system and loss of parasympathetic drive, disrupting intestinal motility by intestinal nervous system and gastrointestinal secretions by enteroendocrine and Paneth cells (Honarpisheh et al., 2020a,b). These phenomena trigger after stroke dysbiosis, often superimposed on age-related dysbiosis and immunosenescence (Benakis et al., 2016; Xia et al., 2019; Lee et al., 2020). Dysbiosis following stroke exacerbates systemic inflammation, by creating a vicious cycle of damage between the brain and gut.
Experimental research on the role of aging gut microflora in stroke
In preclinical investigations, the complete absence of microflora in germ-free and antibiotic-treated mice leads to poorer post-stroke outcomes (Winek et al., 2016). Moreover, pre-stroke bacterial colonization from non-stroke-specific pathogen-free mice into germ-free mice increases microglia/macrophage counts and brain cytokines (IL-1β and TNF-α) and reduces infarct volume in germ-free mice, indicating that pre-stroke microbiota from young healthy donors may play a neuroprotective role post-stroke (Singh et al., 2016, 2018). On the other hand, transplanting pre-stroke fecal microflora from specific pathogen-free mice with stroke increased infarct volume and worsened post-stroke neurological deficits in germ-free mice (Singh et al., 2016, 2018). Consequently, transplantation of pre-stroke fecal microflora from stroke patients to antibiotic-treated mice increased infarct volume and neurological deficits in recipient mice (Xia et al., 2019). These results suggest that pre-stroke transplantation with microflora from healthy donors has a neuroprotective effect, but transplantation with age-related dysbiotic microbiota and from patients with prior stroke has a detrimental effect on the outcome of stroke (Honarpisheh et al., 2022). The effect of a prior stroke on the immune response to a next/new stroke is clinically important and requires further research. Importantly, older mice not only have an underlying dysbiotic state, but are also more prone to stroke-induced dysbiosis, as manifested by increased intestinal permeability and subsequent septicemia due to bacterial translocation (Table 2; Crapser et al., 2016). Aging mice receiving fecal transplant prior to stroke from young donors have better survival rates, decreased circulating cytokines, and better behavioral outcomes even though infarct size has not changed after stroke (Table 2; Spychala et al., 2018). This suggests that rejuvenating the microflora in elderly patients at risk of stroke may be a promising preventive therapy. It should be emphasized that, with the exception of a few studies, most of the preclinical studies were carried out in young mice, and microflora transplants were performed before stroke, which significantly reduces the translational value of the results (Lee et al., 2020).
Clinical studies on the role of aging gut microflora in stroke
Clinical trials have shown a reduction in the diversity of bacteria in samples taken from stroke patients compared with healthy patients (Yin et al., 2015; Haak et al., 2021). In Japanese stroke patients, an increase in Lactobacillus ruminis and a decrease in acetate and valerate were found to positively correlate with markers of systemic inflammation after stroke (Yamashiro et al., 2017). Other studies found that Oscillospira, Enterobacteriaceae, and Parabacteroides were enriched in stroke patients, while Faecalibacterium, Roseburia, and Prevotella were reduced (Xia et al., 2019). Moreover, it was shown that higher Enterobacteriaceae numbers were correlated with poor neurological outcomes (Xu et al., 2021b).
Conclusion
The effect of the aging gut microflora on ischemic stroke is unique as it affects both the risk and outcome of a stroke (Panther et al., 2022). Clinical studies have shown that patients with the most known risk factors (e.g., unhealthy eating habits, diabetes, obesity, and stimulants) (Popa-Wagner et al., 2020) of ischemic stroke have a significantly altered composition of the intestinal microflora (Zeng et al., 2019). In addition, it has been proven that high-risk patients have a reduced number of butyrate-producing bacteria and a lower concentration of butyrate in the stool (Honarpisheh et al., 2022). Also clinical investigations performed following ischemic stroke have shown reduced levels of short-chain fatty acids in stroke patients compared to healthy persons (Tan et al., 2021). Fecal short-chain fatty acids levels are inversely related to functional outcomes ninety days after stroke (Tan et al., 2021; Honarpisheh et al., 2022). However, it should be remembered that these changes may rather be a secondary phenomenon co-occurring with the basic phenomenon (stroke), but not significantly influencing its course (Honarpisheh et al., 2022). An inadequate diet and limited mobility after an ischemic stroke can lead to a poor microbiome. Nevertheless, these evidences suggest that not only the gut-brain axis is an important risk and outcome factor for stroke, but that metabolites such as short-chain fatty acids, glutamate, acetylcholine, dopamine, GABA, serotonin, and histamine may be important functional components in this relationship (Table 1). The main difference between the microbiota of young and old mice is the reduction of bacteria that produce short-chain fatty acids as neurotransmitters (Coman and Vodnar, 2020; Honarpisheh et al., 2022). Old mice that received a fecal transplant from young mice after occlusion of the middle cerebral artery showed better functional regeneration than those that received a fecal transplant from older mice (Lee et al., 2020). Changes in specific types of bacteria are also consistent with the observation that different experimental antibiotic treatment regimens have different effects on stroke outcome. Treatment of mice with clavulanic acid and amoxicillin reduces the infarct volume post-stroke, while a broad-spectrum cocktail of ciprofloxacin, ampicillin, metronidazole, imipenem and vancomycin reduces survival (Benakis et al., 2016; Winek et al., 2016).
Also these observations indicate that aging alters the innate and adaptive immune response following stroke with increased neutrophil infiltration, prior involvement of peripherally derived antigen presenting cells, and a potentially different proportion of cranial bone marrow-derived immune cells compared to peripheral lymphoid immune cells. It can be concluded that the age-related decrease in the number of protective bacteria, e.g., Akkermansia muciniphila, may lead to disturbances in the intestinal barrier function. Thus, γδ T cells move into the aging brain after stroke due to increased blood-brain barrier permeability and the damaged intestinal barrier as a result of stimulation by activated dendritic cells, increasing the production of IL-17, ultimately leading to additional recruitment of neutrophils from the plasma or cranial bone marrow. Research indicates that it is not only the presence, absence, or complete microbial load that governs the gut-brain axis, but rather the abundance and interactions between bacterial groups are important. Future research is needed to elucidate the role of age-related changes in the gut microbiota in cell-specific immune responses following stroke and in the long-term effects of stroke such as cognitive decline with development dementia.
Author contributions
RP and MJ: idea of the manuscript preparation and editing. SJ: searching for literature and preparation of the tables. SC: preparation and editing of the manuscript. All authors contributed to the article and approved the submitted version.
Acknowledgments
We acknowledge financial support from the following institutions: the Mossakowski Medical Research Institute, Polish Academy of Sciences, Warsaw, Poland (T3-RP), and the Medical University of Lublin, Lublin, Poland (DS 475/22-SJC).
Conflict of interest
The authors declare that the research was conducted in the absence of any commercial or financial relationships that could be construed as a potential conflict of interest.
Publisher’s note
All claims expressed in this article are solely those of the authors and do not necessarily represent those of their affiliated organizations, or those of the publisher, the editors and the reviewers. Any product that may be evaluated in this article, or claim that may be made by its manufacturer, is not guaranteed or endorsed by the publisher.
References
Ahmadi, S., Wang, S., Nagpal, R., Wang, B., Jain, S., Razazan, A., et al. (2020). A human-origin probiotic cocktail ameliorates aging-related leaky gut and inflammation via modulating the microbiota/taurine/tight junction axis. JCI Insight 5:e132055. doi: 10.1172/jci.insight.132055
Arpaia, N., Campbell, C., Fan, X., Dikiy, S., van der Veeken, J., deRoos, P., et al. (2013). Metabolites produced by commensal bacteria promote peripheral regulatory T-cell generation. Nature 504, 451–455. doi: 10.1038/nature12726
Asti, A., and Gioglio, L. (2014). Can a bacterial endotoxin be a key factor in the kinetics of amyloid fibril formation? J. Alzheimers Dis. 39, 169–179. doi: 10.3233/JAD-131394
Bachem, A., Makhlouf, C., Binger, K. J., de Souza, D. P., Tull, D., Hochheiser, K., et al. (2019). Microbiota-derived short-chain fatty acids promote the memory potential of antigen-activated CD8. Immunity 51, 285–297.e5. doi: 10.1016/j.immuni.2019.06.002
Baldwin, C. L., and Greenwood, P. M. (2020). Editorial: Cognitive and brain aging: interventions to promote well-being in old age. Front. Aging Neurosci. 11:353. doi: 10.3389/fnagi.2019.00353
Barcik, W., Pugin, B., Westermann, P., Perez, N. R., Ferstl, R., Wawrzyniak, M., et al. (2016). Histamine-secreting microbes are increased in the gut of adult asthma patients. J. Allergy Clin. Immunol. 138, 1491–1494.e7. doi: 10.1016/j.jaci.2016.05.049
Baxter, M. G., and Crimins, J. L. (2018). Acetylcholine receptor stimulation for cognitive enhancement: Better the devil you know? Neuron 98, 1064–1066. doi: 10.1016/j.neuron.2018.06.018
Benakis, C., Brea, D., Caballero, S., Faraco, G., Moore, J., Murphy, M., et al. (2016). Commensal microbiota affects ischemic stroke outcome by regulating nitestinal Γδ T cells. Nat. Med. 22, 516–523. doi: 10.1038/nm.4068
Benjamin, E. J., Virani, S. S., Callaway, C. W., Chamberlain, A. M., Chang, A. R., Cheng, S., et al. (2018). Heart disease and stroke statistics-2018 update: a report from the American Heart Association. Circulation 137, e67–e492. doi: 10.1161/CIR.0000000000000558
Bernardo-Castro, S., Sousa, J. A., Brás, A., Cecília, C., Rodrigues, B., Almendra, L., et al. (2020). Pathophysiology of blood-brain barrier permeability throughout the different stages of ischemic stroke and its implication on hemorrhagic transformation and recovery. Front. Neurol. 11:594672. doi: 10.3389/fneur.2020.594672
Blasco, M. P., Chauhan, A., Honarpisheh, P., Ahnstedt, H., d’Aigle, J., Ganesan, A., et al. (2020). Age-dependent involvement of gut mast cells and histamine in post-stroke inflammation. J. Neuroinflamm. 17:160. doi: 10.1186/s12974-020-01833-1
Bleve, A., Motta, F., Durante, B., Pandolfo, C., Selmi, C., and Sica, A. (2022). Immunosenescence, inflammaging, and frailty: Role of myeloid cells in age-related diseases. Clin. Rev. Allergy Immunol. 15, 1–22. doi: 10.1007/s12016-021-08909-7
Bostanciklioglu, M. (2019). The role of gut microbiota in pathogenesis of Alzheimer’s disease. J. Appl. Microbiol. 127, 954–967. doi: 10.1111/jam.14264
Braniste, V., Al-Asmakh, M., Kowal, C., Anuar, F., Abbaspour, A., Tóth, M., et al. (2014). The gut microbiota influences blood-brain barrier permeability in mice. Sci. Transl. Med. 6:263ra158. doi: 10.1126/scitranslmed.3009759
Brioschi, S., Wang, W. L., Peng, V., Wang, M., Shchukina, I., Greenberg, Z. J., et al. (2021). Heterogeneity of meningeal B cells reveals a lymphopoietic niche at the CNS borders. Science 373:eabf9277. doi: 10.1126/science.abf9277
Camara-Lemarroy, C. R., Ibarra-Yruegas, B. E., and Gongora-Rivera, F. (2014). Gastrointestinal complications after ischemic stroke. J. Neurol. Sci. 346, 20–25. doi: 10.1016/j.jns.2014.08.027
Cason, C. A., Dolan, K. T., Sharma, G., Tao, M., Kulkarni, R., Helenowski, I. B., et al. (2018). Plasma microbiome-modulated indole- and phenyl-derived metabolites associate with advanced atherosclerosis and postoperative outcomes. J Vasc Surg. 68, 1552–1562.e7. doi: 10.1016/j.jvs.2017.09.029
Chang, C.-H., Lin, C.-H., and Lane, H.-Y. (2020). D-glutamate and gut microbiota in Alzheimer’s disease. Int. J. Mol. Sci. 21:2676. doi: 10.3390/ijms21082676
Chauhan, A., Al Mamun, A., Spiegel, G., Harris, N., Zhu, L., and McCullough, L. D. (2018). Splenectomy protects aged mice from injury after experimental stroke. Neurobiol. Aging. 61, 102–111. doi: 10.1016/j.neurobiolaging.2017.09.022
Chen, D., Yang, X., Yang, J., Lai, G., Yong, T., Tang, X., et al. (2017). Prebiotic effect of fructooligosaccharides from Morinda officinalis on Alzheimer’s disease in rodent models by targeting the microbiota-gut-brain axis. Front. Aging Neurosci. 29:403. doi: 10.3389/fnagi.2017.00403
Chen, S. G., Stribinskis, V., Rane, M. J., Demuth, D. R., Gozal, E., Roberts, A. M., et al. (2016). Exposure to the functional bacterial amyloid protein curli enhances alpha-synuclein aggregation in aged fischer 344 rats and Caenorhabditis elegans. Sci. Rep. 6:34477. doi: 10.1038/srep34477
Claesson, M. J., Cusack, S., O’Sullivan, O., Greene-Diniz, R., de Weerd, H., Flannery, E., et al. (2011). Composition, variability, and temporal stability of the intestinal microbiota of the elderly. Proc. Natl. Acad. Sci. U.S.A. 108(Suppl. 1), 4586–4591. doi: 10.1073/pnas.1000097107
Claesson, M. J., Jeffery, I. B., Conde, S., Power, S. E., O’Connor, E. M., Cusack, S., et al. (2012). Gut microbiota composition correlates with diet and health in the elderly. Nature 488, 178–184. doi: 10.1038/nature11319
Coman, V., and Vodnar, D. C. (2020). Gut microbiota and old age: Modulating factors and interventions for healthy longevity. Exp. Gerontol. 141:111095. doi: 10.1016/j.exger.2020.111095
Conway, J., Certo, M., Lord, J. M., Mauro, C., and Duggal, N. A. (2022). Understanding the role of host metabolites in the induction of immunesenescence: future strategies for keeping the ageing population healthy. Br. J. Pharmacol. 179, 1808–1824. doi: 10.1111/bph.15671
Crapser, J., Ritzel, R., Verma, R., Venna, V. R., Liu, F., Chauhan, A., et al. (2016). Ischemic stroke induces gut permeability and enhances bacterial translocation leading to sepsis in aged mice. Aging 8, 1049–1063. doi: 10.18632/aging.100952
Cugurra, A., Mamuladze, T., Rustenhoven, J., Dykstra, T., Beroshvili, G., Greenberg, Z. J., et al. (2021). Skull and vertebral bone marrow are myeloid cell reservoirs for the meninges and CNS parenchyma. Science 373:eabf7844. doi: 10.1126/science.abf7844
Cummings, J. H., Pomare, E. W., Branch, W. J., Naylor, C. P., and Macfarlane, G. T. (1987). Short chain fatty acids in human large intestine, portal, hepatic and venous blood. Gut 28, 1221–1227. doi: 10.1136/gut.28.10.1221
Daria, A., Colombo, A., Llovera, G., Hampel, H., Willem, M., Liesz, A., et al. (2017). Young microglia restore amyloid plaque clearance of aged microglia. EMBO J. 36, 583–603. doi: 10.15252/embj.201694591
de Haan, G., and Lazare, S. S. (2018). Aging of hematopoietic stem cells. Blood 131, 479–487. doi: 10.1182/blood-2017-06-746412
Dinan, T. G., and Cryan, J. F. (2017). Gut instincts: microbiota as a key regulator of brain development, ageing and neurodegeneration. J. Physiol. 595, 489–503. doi: 10.1113/JP273106
Doyle, K. P., Quach, L. N., Solé, M., Axtell, R. C., Nguyen, T. V., Soler-Llavina, G. J., et al. (2015). B-lymphocyte-mediated delayed cognitive impairment following stroke. J. Neurosci. 35, 2133–2145. doi: 10.1523/JNEUROSCI.4098-14.2015
Duranti, S., Ruiz, L., Lugli, G. A., Tames, H., Milani, C., Mancabelli, L., et al. (2020). Bifidobacterium adolescentis as a key member of the human gut microbiota in the production of GABA. Sci. Rep. 10:14112. doi: 10.1038/s41598-020-70986-z
Durgan, D. J., Lee, J., McCullough, L. D., and Bryan, R. M. Jr. (2019). Examining the role of the microbiota-gut-brain axis in stroke. Stroke 50, 2270–2277. doi: 10.1161/STROKEAHA.119.025140
Erny, D., Hrabe de Angelis, A. L., Jaitin, D., Wieghofer, P., Staszewski, O., David, E., et al. (2015). Host microbiota constantly control maturation and function of microglia in the CNS. Nat. Neurosci. 18, 965–977. doi: 10.1038/nn.4030
Evans, M. L., Chorell, E., Taylor, J. D., Åden, J., Götheson, A., Li, F., et al. (2015). The bacterial curli system possesses a potent and selective inhibitor of amyloid formation. Mol. Cell 57, 445–455. doi: 10.1016/j.molcel.2014.12.025
Fei, N., Bernabé, B. P., Lie, L., Baghdan, D., Bedu-Addo, K., Plange-Rhule, J., et al. (2019). The human microbiota is associated with cardiometabolic risk across the epidemiologic transition. PLoS One 14:e0215262. doi: 10.1371/journal.pone.0215262
Felger, J. C., Abe, T., Kaunzner, U. W., Gottfried-Blackmore, A., Gal-Toth, J., McEwen, B. S., et al. (2010). Brain dendritic cells in ischemic stroke: time course, activation state, and origin. Brain Behav. Immun. 24, 724–737. doi: 10.1016/j.bbi.2009.11.002
Fjell, A. M., and Walhovd, K. B. (2010). Structural brain changes in aging: courses, causes and cognitive consequences. Rev. Neurosci. 21, 187–221. doi: 10.1515/REVNEURO.2010.21.3.187
Foreman, K. J., Marquez, N., Dolgert, A., Fukutaki, K., Fullman, N., McGaughey, M., et al. (2018). Forecasting life expectancy, years of life lost, and all-cause and cause-specific mortality for 250 causes of death: reference and alternative scenarios for 2016-40 for 195 countries and territories. Lancet 392, 2052–2090. doi: 10.1016/S0140-6736(18)31694-5
Frasca, D., and Blomberg, B. B. (2011). Aging affects human B cell responses. J. Clin. Immunol. 31, 430–435. doi: 10.1007/s10875-010-9501-7
Fried, L. P., Tangen, C. M., Walston, J., Newman, A. B., Hirsch, C., Gottdiener, J., et al. (2001). Frailty in older adults: evidence for a phenotype. J. Gerontol. A Biol. Sci. Med. Sci. 56, M146–M156. doi: 10.1093/gerona/56.3.M146
Friedland, R. P., and Chapman, M. R. (2017). The role of microbial amyloid in neurodegeneration. PLoS Pathog. 13:e1006654. doi: 10.1371/journal.ppat.1006654
Gbd 2013 DALYs and Hale Collaborators, Murray, C. J. L., Barber, R. M., Foreman, K. J., Abbasoglu Ozgoren, A., Abd-Allah, F., et al. (2015). Global, regional, and national disability-adjusted life years (DALYs) for 306 diseases and injuries and healthy life expectancy (HALE) for 188 countries, 1990-2013: quantifying the epidemiological transition. Lancet 386, 2145–2191. doi: 10.1016/S0140-6736(15)61340-X
Gemmell, E., Bosomworth, H., Allan, L., Hall, R., Khundakar, A., Oakley, A. E., et al. (2012). Hippocampal neuronal atrophy and cognitive function in delayed poststroke and aging-related dementias. Stroke 43, 808–814. doi: 10.1161/STROKEAHA.111.636498
Gemmell, E., Tam, E., Allan, L., Hall, R., Khundakar, A., Oakley, A. E., et al. (2014). Neuron volumes in hippocampal subfields in delayed poststroke and aging-related dementias. J. Neuropathol. Exp. Neurol. 73, 305–311. doi: 10.1097/NEN.0000000000000054
Golomb, S. M. I., Guldner, H., Zhao, A., Wang, Q., Palakurthi, B., Aleksandrovic, E. A., et al. (2020). Multi-modal single-cell analysis reveals brain immune landscape plasticity during aging and gut microbiota dysbiosis. Cell Rep. 33:108438. doi: 10.1016/j.celrep.2020.108438
Green, D. R., Galluzzi, L., and Kroemer, G. (2011). Mitochondria and the autophagy–inflammation– cell death axis in organismal aging. Science 333, 1109–1112. doi: 10.1126/science.1201940
Haak, B. W., Westendorp, W. F., van Engelen, T. S. R., Brands, X., Brouwer, M. C., Vermeij, J. D., et al. (2021). Disruptions of anaerobic gut bacteria are associated with stroke and poststroke infection: a prospective case-control study. Transl. Stroke Res. 12, 581–592. doi: 10.1007/s12975-020-00863-4
Harach, T., Marungruang, N., Duthilleul, N., Cheatham, V., Mc Coy, K. D., Frisoni, G., et al. (2017). Reduction of Abeta amyloid pathology in APPPS1 transgenic mice in the absence of gut microbiota. Sci. Rep. 7:41802. doi: 10.1038/srep46856
Hatsuta, H., Takao, M., Nogami, A., Uchino, A., Sumikura, H., Takata, T., et al. (2019). Tau and TDP-43 accumulation of the basal nucleus of Meynert in individuals with cerebral lobar infarcts or hemorrhage. Acta Neuropathol. Commun. 7:49. doi: 10.1186/s40478-019-0700-z
Ho, L., Ono, K., Tsuji, M., Mazzola, P., Singh, R., and Pasinetti, G. M. (2018). Protective roles of intestinal microbiota derived short chain fatty acids in Alzheimer’s disease-type beta amyloid neuropathological mechanisms. Expert. Rev. Neurother. 18, 83–90. doi: 10.1080/14737175.2018.1400909
Holmes, A., Finger, C., Morales-Scheihing, D., Lee, J., and McCullough, L. D. (2020). Gut dysbiosis and age-related neurological diseases; an innovative approach for therapeutic interventions. Transl. Res. 226, 39–56. doi: 10.1016/j.trsl.2020.07.012
Holzer, P., and Farzi, A. (2014). Neuropeptides and the microbiota-gut-brain axis. Adv. Exp. Med. Biol. 817, 195–219. doi: 10.1007/978-1-4939-0897-4_9
Honarpisheh, P., Blixt, F. W., Blasco Conesa, M. P., Won, W., d’Aigle, J., Munshi, Y., et al. (2020a). Peripherally-sourced myeloid antigen presenting cells increase with advanced aging. Brain Behav. Immun. 90, 235–247. doi: 10.1016/j.bbi.2020.08.023
Honarpisheh, P., Lee, J., Banerjee, A., Blasco-Conesa, M. P., Honarpisheh, P., d’Aigle, J., et al. (2020b). Potential caveats of putative microglia-specific markers for assessment of age-related cerebrovascular neuroinflammation. J. Neuroinflam. 17:366. doi: 10.1186/s12974-020-02019-5
Honarpisheh, P., Bryan, R. M., and McCullough, L. D. (2022). Aging microbiota-gut-brain axis in stroke risk and outcome. Circ. Res. 130, 1112–1144. doi: 10.1161/CIRCRESAHA.122.319983
Hotta, H., and Uchida, S. (2010). Aging of the autonomic nervous system and possible improvements in autonomic activity using somatic afferent stimulation. Geriatr. Gerontol. Int. 10(Suppl. 1), S127–S136. doi: 10.1111/j.1447-0594.2010.00592.x
Huuskonen, J., Suuronen, T., Nuutinen, T., Kyrylenko, S., and Salminen, A. (2004). Regulation of microglial inflammatory response by sodium butyrate and short-chain fatty acids. Br. J. Pharmacol. 141, 874–880. doi: 10.1038/sj.bjp.0705682
Iadecola, C., Buckwalter, M. S., and Anrather, J. (2020). Immune responses to stroke: mechanisms, modulation, and therapeutic potential. J. Clin. Invest. 130, 2777–2788. doi: 10.1172/JCI135530
Jackson, M. A., Jackson, M., Jeffery, I. B., Beaumont, M., Bell, J. T., Clark, A. G., et al. (2016). Signatures of early frailty in the gut microbiota. Genome Med. 8:8. doi: 10.1186/s13073-016-0262-7
Jang, S. E., Lim, S. M., Jeong, J. J., Jang, H. M., Lee, H. J., Han, M. J., et al. (2018). Gastrointestinal inflammation by gut microbiota disturbance induces memory impairment in mice. Mucosal. Immunol. 11, 369–379. doi: 10.1038/mi.2017.49
Javidi, E., and Magnus, T. (2019). Autoimmunity after ischemic stroke and brain injury. Front. Immunol. 10:686. doi: 10.3389/fimmu.2019.00686
Jendroska, K., Hoffmann, O. M., and Patt, S. (1997). Amyloid β peptide and precursor protein (APP) in mild and severe brain ischemia. Ann. N.Y. Acad. Sci. 826, 401–405. doi: 10.1111/j.1749-6632.1997.tb48492.x
Jendroska, K., Poewe, W., Daniel, S. E., Pluess, J., Iwerssen-Schmidt, H., Paulsen, J., et al. (1995). Ischemic stress induces deposition of amyloid beta immunoreactivity in human brain. Acta Neuropathol. 90, 461–466. doi: 10.1007/BF00294806
Jin, W. N., Shi, S. X., Li, Z., Li, M., Wood, K., Gonzales, R. J., et al. (2017). Depletion of microglia exacerbates postischemic inflammation and brain injury. J. Cereb. Blood Flow Metab. 37, 2224–2236. doi: 10.1177/0271678X17694185
Johnson, E. C. B., Dammer, E. B., Duong, D. M., Ping, L., Zhou, M., Yin, L., et al. (2020). Large scale proteomic analysis of Alzheimer’s disease brain and cerebrospinal fluid reveals early changes in energy metabolism associated with microglia and astrocyte activation. Nat. Med. 26, 769–780. doi: 10.1038/s41591-020-0815-6
Jurk, D., Wang, C., Miwa, S., Maddick, M., Korolchuk, V., Tsolou, A., et al. (2012). Postmitotic neurons develop a p21-dependent senescence-like phenotype driven by a DNA damage response. Aging Cell 11, 996–1004. doi: 10.1111/j.1474-9726.2012.00870.x
Kaiko, G. E., Ryu, S. H., Koues, O. I., Collins, P. L., Solnica-Krezel, L., Pearce, E. J., et al. (2016). The colonic crypt protects stem cells from microbiota-derived metabolites. Cell 165, 1708–1720. doi: 10.1016/j.cell.2016.05.018
Kato, T., Hirano, A., Katagiri, T., Sasaki, H., and Yamada, S. (1988). Neurofibrillary tangle formation in the nucleus basalis of Meynert ipsilateral to a massive cerebral infarct. Ann. Neurol. 23, 620–623. doi: 10.1002/ana.410230617
Khan, S., Yuldasheva, N. Y., Batten, T. F. C., Pickles, A. R., Kellett, K. A. B., and Saha, S. (2018). Tau pathology and neurochemical changes associated with memory dysfunction in an optimized murine model of global cerebral ischaemia—A potential model for vascular dementia? Neurochem. Int. 118, 134–144. doi: 10.1016/j.neuint.2018.04.004
Kharlamov, A., Kharlamov, E., and Armstrong, D. M. (2000). Age-dependent increase in infarct volume following photochemically induced cerebral infarction: putative role of astroglia. J. Gerontol. A Biol. Sci. Med. Sci. 55, B135–B141. doi: 10.1093/gerona/55.3.B135
Kim, M. S., Kim, Y., Choi, H., Kim, W., Park, S., Lee, D., et al. (2020). Transfer of a healthy microbiota reduces amyloid and tau pathology in an Alzheimer’s disease animal model. Gut 69, 283–294. doi: 10.1136/gutjnl-2018-317431
Kim, S. E. (2017). Colonic slow transit can cause changes in the gut environment observed in the elderly. J. Neurogastroenterol. Motil. 23, 3–4. doi: 10.5056/jnm16215
Kocki, J., Ułamek-Kozioł, M., Bogucka-Kocka, A., Januszewski, S., Jabłonski, M., Gil-Kulik, P., et al. (2015). Dysregulation of amyloid precursor protein, βsecretase, presenilin 1 and 2 genes in the rat selectively vulnerable CA1 subfield of hippocampus following transient global brain ischemia. J. Alzheimers Dis. 47, 1047–1056. doi: 10.3233/JAD-150299
Koutzoumis, D. N., Vergara, M., Pino, J., Buddendorff, J., Khoshbouei, H., Mandel, R. J., et al. (2020). Alterations of the gut microbiota with antibiotics protects dopamine neuron loss and improve motor deficits in a pharmacological rodent model of Parkinson’s disease. Exp. Neurol. 325:113159. doi: 10.1016/j.expneurol.2019.113159
Krambs, J. R., Monlish, D. A., Gao, F., Schuettpelz, L. G., and Link, D. C. (2021). Microbiota signals suppress B lymphopoiesis with aging in mice. Front. Immunol. 12:767267. doi: 10.3389/fimmu.2021.767267
Lee, C. Y. D., Daggett, A., Gu, X., Jiang, L. L., Langfelder, P., Li, X., et al. (2018). Elevated TREM2 gene dosage reprograms microglia responsivity and ameliorates pathological phenotypes in Alzheimer’s disease models. Neuron 97, 1032–1048.e5. doi: 10.1016/j.neuron.2018.02.002
Lee, J., D’Aigle, J., Atadja, L., Quaicoe, V., Honarpisheh, P., Ganesh, B. P., et al. (2020). Gut microbiota-derived short-chain fatty acids promote poststroke recovery in aged mice. Circ. Res. 127, 453–465. doi: 10.1161/CIRCRESAHA.119.316448
Lee, J. W., Lee, Y. K., Yuk, D. Y., Choi, D. Y., Ban, S. B., Oh, K. W., et al. (2008). Neuro-inflammation induced by lipopolysaccharide causes cognitive impairment through enhancement of beta-amyloid generation. J. Neuroinflamm. 5:37. doi: 10.1186/1742-2094-5-37
Lee, Y. T., Mohd Ismail, N. I., and Wei, L. K. (2021). Microbiome and ischemic stroke: A systematic review. PLoS One 16:e0245038. doi: 10.1371/journal.pone.0245038
Lehallier, B., Gate, D., Schaum, N., Nanasi, T., Lee, S. E., Yousef, H., et al. (2019). Undulating changes in human plasma proteome profiles across the lifespan. Nat. Med. 25, 1843–1850. doi: 10.1038/s41591-019-0673-2
Lejault, P., Moruno-Manchon, J. F., Vemu, S. M., Honarpisheh, P., Zhu, L., Kim, N., et al. (2020). Regulation of autophagy by DNA G-quadruplexes. Autophagy 16, 2252–2259. doi: 10.1080/15548627.2020.1769991
Li, D., Lang, W., Zhou, C., Wu, C., Zhang, F., Liu, Q., et al. (2018). Upregulation of microglial ZEB1 ameliorates brain damage after acute ischemic stroke. Cell Rep. 22, 3574–3586. doi: 10.1016/j.celrep.2018.03.011
Li, Y., Ning, L., Yin, Y., Wang, R., Zhang, Z., Hao, L., et al. (2020). Age-related shifts in gut microbiota contribute to cognitive decline in aged rats. Aging 12, 7801–7817. doi: 10.18632/aging.103093
Lindemans, C. A., Calafiore, M., Mertelsmann, A. M., O’Connor, M. H., Dudakov, J. A., Jenq, R. R., et al. (2015). Interleukin-22 promotes intestinal-stem-cell-mediated epithelial regeneration. Nature 528, 560–564. doi: 10.1038/nature16460
Liu, F., and McCullough, L. D. (2011). Middle cerebral artery occlusion model in rodents: methods and potential pitfalls. J. Biomed. Biotechnol. 2011:464701. doi: 10.1155/2011/464701
Liu, F., Yuan, R., Benashski, S. E., and McCullough, L. D. (2009). Changes in experimental stroke outcome across the life span. J. Cereb. Blood Flow Metab. 29, 792–802. doi: 10.1038/jcbfm.2009.5
Liu, G., Chong, H. X., Chung, F. Y. L., Li, Y., and Liong, M. T. (2020). Lactobacillus plantarum DR7 modulated bowel movement and gut microbiota associated with dopamine and serotonin pathways in stressed adults. Int. J. Mol. Sci. 21:4608. doi: 10.3390/ijms21134608
Louveau, A., Herz, J., Alme, M. N., Salvador, A. F., Dong, M. Q., Viar, K. E., et al. (2018). CNS lymphatic drainage and neuroinflammation are regulated by meningeal lymphatic vasculature. Nat. Neurosci. 21, 1380–1391. doi: 10.1038/s41593-018-0227-9
Lundmark, K., Westermark, G. T., Olsén, A., and Westermark, P. (2005). Protein fibrils in nature can enhance amyloid protein A amyloidosis in mice: Cross-seeding as a disease mechanism. Proc. Natl. Acad. Sci. U.S.A. 102, 6098–6102. doi: 10.1073/pnas.0501814102
Ma, Q., Ineichen, B. V., Detmar, M., and Proulx, S. T. (2017). Outflow of cerebrospinal fluid is predominantly through lymphatic vessels and is reduced in aged mice. Nat. Commun. 8:1434. doi: 10.1038/s41467-017-01484-6
Ma, Q., Xing, C., Long, W., Wang, H. Y., Liu, Q., and Wang, R. F. (2019). Impact of microbiota on central nervous system and neurological diseases: the gut-brain axis. J. Neuroinflamm. 16:53. doi: 10.1186/s12974-019-1434-3
Macfarlane, G. T., and Macfarlane, S. (2012). Bacteria, colonic fermentation, and gastrointestinal health. J. AOAC Int. 95, 50–60. doi: 10.5740/jaoacint.SGE_Macfarlane
Madison, A. A., and Kiecolt-Glaser, J. K. (2021). The gut microbiota and nervous system: Age-defined and age-defying. Semin. Cell Dev. Biol. 116, 98–107. doi: 10.1016/j.semcdb.2020.12.009
Marín-Burgin, A., and Schinder, A. F. (2012). Requirement of adult-born neurons for hippocampus-dependent learning. Behav. Brain Res. 227, 391–399. doi: 10.1016/j.bbr.2011.07.001
Mattson, M. P., and Arumugam, T. V. (2018). Hallmarks of brain aging: adaptive and pathological modification by metabolic states. Cell Metab. 27, 1176–1199. doi: 10.1016/j.cmet.2018.05.011
McKenna, R. W., Washington, L. T., Aquino, D. B., Picker, L. J., and Kroft, S. H. (2001). Immunophenotypic analysis of hematogones (B-lymphocyte precursors) in 662 consecutive bone marrow specimens by 4-color flow cytometry. Blood 98, 2498–2507. doi: 10.1182/blood.V98.8.2498
Moskalev, A. A., Shaposhnikov, M. V., Plyusnina, E. N., Zhavoronkov, A., Budovsky, A., Yanai, H., et al. (2013). The role of DNA damage and repair in aging through the prism of Koch-like criteria. Ageing Res. Rev. 12, 661–668. doi: 10.1016/j.arr.2012.02.001
Moss, C., Dhillo, W. S., Frost, G., and Hickson, M. (2012). Gastrointestinal hormones: the regulation of appetite and the anorexia of ageing. J. Hum. Nutr. Diet. 25, 3–15. doi: 10.1111/j.1365-277X.2011.01211.x
Nadareishvili, Z. G., Li, H., Wright, V., Maric, D., Warach, S., Hallenbeck, J. M., et al. (2004). Elevated pro-inflammatory CD4+CD28-lymphocytes and stroke recurrence and death. Neurology 63, 1446–1451. doi: 10.1212/01.WNL.0000142260.61443.7C
Nguyen, P. T., Dorman, L. C., Pan, S., Vainchtein, I. D., Han, R. T., Nakao-Inoue, H., et al. (2020). Microglial remodeling of the extracellular matrix promotes synapse plasticity. Cell 182, 388–403. doi: 10.1016/j.cell.2020.05.050
Ogbonnaya, E. S., Clarke, G., Shanahan, F., Dinan, T. G., Cryan, J. F., and O’Leary, O. F. (2015). Adult hippocampal neurogenesis is regulated by the microbiome. Biol. Psychiatry 78, e7–e9. doi: 10.1016/j.biopsych.2014.12.023
O’Mahony, D., O’Leary, P., and Quigley, E. M. (2002). Aging and intestinal motility: a re-view of factors that affect intestinal motility in the aged. Drugs Aging 19, 515–527. doi: 10.2165/00002512-200219070-00005
Palmer, K., Vetrano, D. L., Padua, L., Romano, V., Rivoiro, C., Scelfo, B., et al. (2019). Frailty syndromes in persons with cerebrovascular disease: a systematic review and meta-analysis. Front. Neurol. 10:1255. doi: 10.3389/fneur.2019.01255
Panther, E. J., Dodd, W., Clark, A., and Lucke-Wold, B. (2022). Gastrointestinal microbiome and neurologic injury. Biomedicines 10:500. doi: 10.3390/biomedicines10020500
Parrish, A. R. (2017). The impact of aging on epithelial barriers. Tissue Barriers 5:e1343172. doi: 10.1080/21688370.2017.1343172
Partridge, L. (2001). Evolutionary theories of ageing applied to long-lived organisms. Exp. Gerontol. 36, 641–650. doi: 10.1016/S0531-5565(00)00232-1
Peck, C. J., Samsuria, S. D., Harrington, A. M., King, S. K., Hutson, J. M., and Southwell, B. R. (2009). Fall in density, but not number of myenteric neurons and circular muscle nerve fibres in guinea-pig colon with ageing. Neurogastroenterol. Motil. 21, 1075–1090. doi: 10.1111/j.1365-2982.2009.01349.x
Phillips, R. J., Kieffer, E. J., and Powley, T. L. (2004). Loss of glia and neurons in the myenteric plexus of the aged Fischer 344 rat. Anat. Embryol. 209, 19–30. doi: 10.1007/s00429-004-0426-x
Phillips, R. J., and Powley, T. L. (2007). Innervation of the gastrointestinal tract: patterns of aging. Auton. Neurosci. 136, 1–19. doi: 10.1016/j.autneu.2007.04.005
Pluta, R. (2000). The role of apolipoprotein E in the deposition of β-amyloid peptide during ischemia–reperfusion brain injury. A model of early Alzheimer’s disease. Ann. N. Y. Acad. Sci. 903, 324–334. doi: 10.1111/j.1749-6632.2000.tb06383.x
Pluta, R., Barcikowska, M., Januszewski, S., Misicka, A., and Lipkowski, A. W. (1996). Evidence of blood-brain barrier permeability/leakage for circulating human Alzheimer’s beta-amyloid-(1-42)-peptide. NeuroReport 7, 1261–1265. doi: 10.1097/00001756-199605170-00008
Pluta, R., Bogucka-Kocka, A., Ułamek-Kozioł, M., Bogucki, J., and Czuczwar, S. J. (2018a). Ischemic tau protein gene induction as an additional key factor driving development of Alzheimer’s phenotype changes in CA1 area of hippocampus in an ischemic model of Alzheimer’s disease. Pharmacol. Rep. 70, 881–884. doi: 10.1016/j.pharep.2018.03.004
Pluta, R., Ułamek-Kozioł, M., Januszewski, S., and Czuczwar, S. J. (2018b). Tau protein dysfunction after brain ischemia. J. Alzheimers Dis. 66, 429–437. doi: 10.3233/JAD-180772
Pluta, R., Januszewski, S., and Czuczwar, S. J. (2021a). The role of gut microbiota in an ischemic stroke. Int. J. Mol. Sci. 22:915. doi: 10.3390/ijms22020915
Pluta, R., Czuczwar, S. J., Januszewski, S., and Jabłoński, M. (2021b). The many faces of post-ischemic tau protein in brain neurodegeneration of the Alzheimer’s disease type. Cells 10:2213. doi: 10.3390/cells10092213
Pluta, R., Jolkkonen, J., Cuzzocrea, S., Pedata, F., Cechetto, D., and Popa-Wagner, A. (2011). Cognitive impairment with vascular impairment and degeneration. Curr. Neurovasc. Res. 8, 342–350. doi: 10.2174/156720211798120981
Pluta, R., Kida, E., Lossinsky, A. S., Golabek, A. A., Mossakowski, M. J., and Wisniewski, H. M. (1994a). Complete cerebral ischemia with short-term survival in rats induced by cardiac arrest. I. Extracellular accumulation of Alzheimer’s beta-amyloid protein precursor in the brain. Brain Res. 649, 323–328. doi: 10.1016/0006-8993(94)91081-2
Pluta, R., Lossinsky, A. S., Walski, M., Wisniewski, H. M., and Mossakowski, M. J. (1994b). Platelet occlusion phenomenon after short- and long-term survival following complete cerebral ischemia in rats produced by cardiac arrest. J. Hirnforsch. 35, 463–471.
Pluta, R., Kocki, J., Ułamek-Kozioł, M., Petniak, A., Gil-Kulik, P., Januszewski, S., et al. (2016a). Discrepancy in expression of β-secretase and amyloid-β protein precursor in Alzheimer-related genes in the rat medial temporal lobe cortex following transient global brain ischemia. J. Alzheimers Dis. 51, 1023–1031. doi: 10.3233/JAD-151102
Pluta, R., Kocki, J., Ułamek-Kozioł, M., Bogucka-Kocka, A., Gil-Kulik, P., Januszewski, S., et al. (2016b). Alzheimer-associated presenilin 2 gene is dysregulated in rat medial temporal lobe cortex after complete brain ischemia due to cardiac arrest. Pharmacol. Rep. 68, 155–161. doi: 10.1016/j.pharep.2015.08.002
Pluta, R., Ułamek, M., and Jabłoński, M. (2009). Alzheimer’s mechanisms in ischemic brain degeneration. Anat. Rec. 292, 1863–1881. doi: 10.1002/ar.21018
Pluta, R., Ułamek-Kozioł, M., Kocki, J., Bogucki, J., Januszewski, S., BoguckaKocka, A., et al. (2020a). Expression of the tau protein and amyloid protein precursor processing genes in the CA3 area of the hippocampus in the ischemic model of Alzheimer’s disease in the rat. Mol. Neurobiol. 57, 1281–1290. doi: 10.1007/s12035-019-01799-z
Pluta, R., Ułamek-Kozioł, M., Januszewski, S., and Czuczwar, S. J. (2020b). Gut microbiota and pro/prebiotics in Alzheimer’s disease. Aging 12, 5539–5550. doi: 10.18632/aging.102930
Popa-Wagner, A., Dumitrascu, D. I., Capitanescu, B., Petcu, E. B., Surugiu, R., Fang, W.-H., et al. (2020). Dietary habits, lifestyle factors and neurodegenerative diseases. Neural. Regen. Res. 15, 394–400. doi: 10.4103/1673-5374.266045
Popović, N., Stefanović-Budimkić, M., Mitrović, N., Urošević, A., Milošević, B., Pelemiš, M., et al. (2013). The frequency of post-stroke infections and their impact on early stroke outcome. J. Stroke Cerebrovasc. Dis. 22, 424–429. doi: 10.1016/j.jstrokecerebrovasdis.2013.03.003
Powers, W. J., Rabinstein, A. A., Ackerson, T., Adeoye, O. M., Bambakidis, N. C., Becker, K., et al. (2019). Guidelines for the early management of patients with acute ischemic stroke: 2019 update to the 2018 guidelines for the early management of acute ischemic stroke: a guideline for healthcare professionals from the American Heart Association/American Stroke Association. Stroke 50, e344–e418. doi: 10.1161/STR.0000000000000211
Qi, J., Wu, H., Yang, Y., Wand, D., Chen, Y., Gu, Y., et al. (2007). Cerebral ischemia and Alzheimer’s disease: the expression of amyloid-β and apolipoprotein E in human hippocampus. J. Alzheimers Dis. 12, 335–341. doi: 10.3233/JAD-2007-12406
Qi, Y., Goel, R., Kim, S., Richards, E. M., Carter, C. S., Pepine, C. J., et al. (2017). Intestinal permeability biomarker zonulin is elevated in healthy aging. J Am Med Dir Assoc. 18, 810.e1–810.e4. doi: 10.1016/j.jamda.2017.05.018
Radenovic, L., Nenadic, M., Ułamek-Kozioł, M., Januszewski, S., Czuczwar, S. J., Andjus, P. R., et al. (2020). Heterogeneity in brain distribution of activated microglia and astrocytes in a rat ischemic model of Alzheimer’s disease after 2 years of survival. Aging 12, 12251–12267. doi: 10.18632/aging.103411
Richards, E. M., Li, J., Stevens, B. R., Pepine, C. J., and Raizada, M. K. (2022). Gut microbiome and neuroinflammation in hypertension. Circ. Res. 130, 401–417. doi: 10.1161/CIRCRESAHA.121.319816
Rickenbach, C., and Gericke, C. (2022). Specificity of adaptive immune responses in central nervous system health, aging and diseases. Front. Neurosci. 15:806260. doi: 10.3389/fnins.2021.806260
Rinaldo, L., Rabinstein, A. A., Cloft, H., Knudsen, J. M., Castilla, L. R., and Brinjikji, W. (2019). Racial and ethnic disparities in the utilization of thrombectomy for acute stroke. Stroke 50, 2428–2432. doi: 10.1161/STROKEAHA.118.024651
Ritzel, R. M., Lai, Y. J., Crapser, J. D., Patel, A. R., Schrecengost, A., Grenier, J. M., et al. (2018). Aging alters the immunological response to ischemic stroke. Acta Neuropathol. 136, 89–110. doi: 10.1007/s00401-018-1859-2
Rost, N. S., Brodtmann, A., Pase, M. P., van Veluw, S. J., Biffi, A., Duering, M., et al. (2022). Post-stroke cognitive impairment and dementia. Circ. Res. 130, 1252–1271. doi: 10.1161/CIRCRESAHA.122.319951
Roy-O’Reilly, M. A., Ahnstedt, H., Spychala, M. S., Munshi, Y., Aronowski, J., Sansing, L. H., et al. (2020). Aging exacerbates neutrophil pathogenicity in ischemic stroke. Aging 12, 436–461. doi: 10.18632/aging.102632
Rubtsov, A. V., Rubtsova, K., Fischer, A., Meehan, R. T., Gillis, J. Z., Kappler, J. W., et al. (2011). Toll-like receptor 7 (TLR7)-driven accumulation of a novel CD11c+ B-cell population is important for the development of autoimmunity. Blood 118, 1305–1315. doi: 10.1182/blood-2011-01-331462
Ruiz-Ruiz, S., Sanchez-Carrillo, S., Ciordia, S., Mena, M. C., Méndez-García, C., Rojo, D., et al. (2020). Functional microbiome deficits associated with ageing: Chronological age threshold. Aging Cell 19:e13063. doi: 10.1111/acel.13063
Rustenhoven, J., Drieu, A., Mamuladze, T., de Lima, K. A., Dykstra, T., Wall, M., et al. (2021). Functional characterization of the dural sinuses as a neuroimmune interface. Cell 184, 1000–1016.e27. doi: 10.1016/j.cell.2020.12.040
Saffrey, M. J. (2013). Cellular changes in the enteric nervous system during ageing. Dev. Biol. 382, 344–355. doi: 10.1016/j.ydbio.2013.03.015
Salazar, N., Arboleya, S., Fernández-Navarro, T., de Los Reyes-Gavilán, C. G., Gonzalez, S., and Gueimonde, M. (2019). Age-associated changes in gut microbiota and dietary components related with the immune system in adulthood and old age: A Cross-Sectional Study. Nutrients 11:E1765. doi: 10.3390/nu11081765
Schächtle, M. A., and Rosshart, S. P. (2021). The microbiota-gut-brain axis in health and disease and its implications for translational research. Front. Cell. Neurosci. 15:698172. doi: 10.3389/fncel.2021.698172
Schilling, M., Besselmann, M., Leonhard, C., Mueller, M., Ringelstein, E. B., and Kiefer, R. (2003). Microglial activation precedes and predominates over macrophage infiltration in transient focal cerebral ischemia: a study in green fluorescent protein transgenic bone marrow chimeric mice. Exp. Neurol. 183, 25–33. doi: 10.1016/S0014-4886(03)00082-7
Sekeljic, V., Bataveljic, D., Stamenkovic, S., Ułamek, M., Jabłonski, M., Radenovic, L., et al. (2012). Cellular markers of neuroinflammation and neurogenesis after ischemic brain injury in the long-term survival rat model. Brain Struct. Funct. 217, 411–420. doi: 10.1007/s00429-011-0336-7
Shapira, S., Sapir, M., Wengier, A., Grauer, E., and Kadar, T. (2002). Aging has a complex effect on a rat model of ischemic stroke. Brain Res. 925, 148–158. doi: 10.1016/S0006-8993(01)03270-X
Shin, J., Noh, J. R., Choe, D., Lee, N., Song, Y., Cho, S., et al. (2021). Ageing and rejuvenation models reveal changes in key microbial communities associated with healthy ageing. Microbiome 9:240. doi: 10.1186/s40168-021-01189-5
Sieber, M. W., Claus, R. A., Witte, O. W., and Frahm, C. (2011). Attenuated inflammatory response in aged mice brains following stroke. PLoS One 6:e26288. doi: 10.1371/journal.pone.0026288
Sinagra, E., Pellegatta, G., Guarnotta, V., Maida, M., Rossi, F., Conoscenti, G., et al. (2021). Microbiota gut-brain axis in ischemic stroke: A narrative review with a focus about the relationship with inflammatory bowel disease. Life 11:715. doi: 10.3390/life11070715
Singh, H., Torralba, M. G., Moncera, K. J., DiLello, L., Petrini, J., Nelson, K. E., et al. (2019). Gastro-intestinal and oral microbiome signatures associated with healthy aging. Geroscience 41, 907–921. doi: 10.1007/s11357-019-00098-8
Singh, V., Roth, S., Llovera, G., Sadler, R., Garzetti, D., Stecher, B., et al. (2016). Microbiota dysbiosis controls the neuroinflammatory response after stroke. J. Neurosci. 36, 7428–7440. doi: 10.1523/JNEUROSCI.1114-16.2016
Singh, V., Sadler, R., Heindl, S., Llovera, G., Roth, S., Benakis, C., et al. (2018). The gut microbiome primes a cerebroprotective immune response after stroke. J. Cereb. Blood Flow Metab. 38, 1293–1298. doi: 10.1177/0271678X18780130
Sovran, B., Hugenholtz, F., Elderman, M., Van Beek, A. A., Graversen, K., Huijskes, M., et al. (2019). Age associated impairment of the mucus barrier function is associated with profound changes in microbiota and immunity. Sci. Rep. 9:1437. doi: 10.1038/s41598-018-35228-3
Spychala, M. S., Honarpisheh, P., and McCullough, L. D. (2017). Sex differences in neuroinflammation and neuroprotection in ischemic stroke. J. Neurosci. Res. 95, 462–471. doi: 10.1002/jnr.23962
Spychala, M. S., Venna, V. R., Jandzinski, M., Doran, S. J., Durgan, D. J., Ganesh, B. P., et al. (2018). Age-related changes in the gut microbiota influence systemic inflammation and stroke outcome. Ann. Neurol. 84, 23–36. doi: 10.1002/ana.25250
Sumida, K., Molnar, M. Z., Potukuchi, P. K., Thomas, F., Lu, J. L., Yamagata, K., et al. (2019). Constipation and risk of death and cardiovascular events. Atherosclerosis 281, 114–120. doi: 10.1016/j.atherosclerosis.2018.12.021
Swardfager, W., Herrmann, N., Andreazza, A. C., Swartz, R. H., Khan, M. M., Black, S. E., et al. (2014). Poststroke neuropsychiatric symptoms: relationships with IL-17 and oxidative stress. Biomed. Res. Int. 2014:245210. doi: 10.1155/2014/245210
Syed, A. K., and Boles, B. R. (2014). Fold modulating function: Bacterial toxins to functional amyloids. Front. Microbiol. 5:401. doi: 10.3389/fmicb.2014.00401
Tan, C., Wu, Q., Wang, H., Gao, X., Xu, R., Cui, Z., et al. (2021). Dysbiosis of gut microbiota and short-chain fatty acids in acute ischemic stroke and the subsequent risk for poor functional outcomes. J. Parenter. Enter. Nutr. 45, 518–529. doi: 10.1002/jpen.1861
Toral, M., Robles-Vera, I., de la Visitación, N., Romero, M., Yang, T., Sánchez, M., et al. (2019). Critical role of the interaction gut microbiota - sympathetic nervous system in the regulation of blood pressure. Front. Physiol. 10:231. doi: 10.3389/fphys.2019.00231
Tschan, S. L., and Bolliger, D. (2021). Coagulation and aging: implications for the anesthesiologist. Curr. Anesthesiol. Rep. 11, 387–395. doi: 10.1007/s40140-021-00498-7
Ułamek-Kozioł, M., Czuczwar, S. J., Kocki, J., Januszewski, S., Bogucki, J., Bogucka-Kocka, A., et al. (2019). Dysregulation of autophagy, mitophagy, and apoptosis genes in the CA3 region of the hippocampus in the ischemic model of Alzheimer’s disease in the rat. J. Alzheimer’s Dis. 72, 1279–1286. doi: 10.3233/JAD-190966
Ułamek-Kozioł, M., Kocki, J., Bogucka-Kocka, A., Januszewski, S., Bogucki, J., Czuczwar, S. J., et al. (2017). Autophagy, mitophagy and apoptotic gene changes in the hippocampal CA1 area in a rat ischemic model of Alzheimer’s disease. Pharmacol. Rep. 69, 1289–1294. doi: 10.1016/j.pharep.2017.07.015
Ułamek-Kozioł, M., Kocki, J., Bogucka-Kocka, A., Petniak, A., Gil-Kulik, P., Januszewski, S., et al. (2016). Dysregulation of autophagy, mitophagy and apoptotic genes in the medial temporal lobe cortex in an ischemic model of Alzheimer’s disease. J. Alzheimer’s Dis. 54, 113–121. doi: 10.3233/JAD-160387
van der Lugt, B., van Beek, A. A., Aalvink, S., Meijer, B., Sovran, B., Vermeij, W. P., et al. (2019). Akkermansia muciniphila ameliorates the age-related decline in colonic mucus thickness and attenuates immune activation in accelerated aging Ercc1 -/Δ7 mice. Immun. Ageing 16:6. doi: 10.1186/s12979-019-0145-z
van Groen, T., Puurunen, K., Maki, H. M., Sivenius, J., and Jolkkonen, J. (2005). Transformation of diffuse beta-amyloid precursor protein and beta-amyloid deposits to plaques in the thalamus after transient occlusion of the middle cerebral artery in rats. Stroke 36, 1551–1556. doi: 10.1161/01.STR.0000169933.88903.cf
Wang, R. Y., Wang, P. S., and Yang, Y. R. (2003). Effect of age in rats following middle cerebral artery occlusion. Gerontology 49, 27–32. doi: 10.1159/000066505
Wang, S., Mustafa, M., Yuede, C. M., Salazar, S. V., Kong, P., Long, H., et al. (2020). Anti-human TREM2 induces microglia proliferation and reduces pathology in an Alzheimer’s disease model. J. Exp. Med. 217:20200785. doi: 10.1084/jem.20200785
Wen, Y., Yang, S. H., Liu, R., Perez, E. J., Brun-Ziukemagel, A. M., Koulen, P., et al. (2007). Cdk5 is involved in NFT-like tauopathy induced by transient cerebral ischemia in female rats. Biochim. Biophys. Acta 1772, 473–483. doi: 10.1016/j.bbadis.2006.10.011
Wikoff, W. R., Anfora, A. T., Liu, J., Schultz, P. G., Lesley, S. A., Peters, E. C., et al. (2009). Metabolomics analysis reveals large effects of gut microflora on mammalian blood metabolites. Proc. Natl. Acad. Sci. U.S.A. 106, 3698–3703. doi: 10.1073/pnas.0812874106
Winek, K., Engel, O., Koduah, P., Heimesaat, M. M., Fischer, A., Bereswill, S., et al. (2016). Depletion of cultivatable gut microbiota by broad-spectrum antibiotic pretreatment worsens outcome after murine stroke. Stroke 47, 1354–1363. doi: 10.1161/STROKEAHA.115.011800
Wisniewski, H. M., Pluta, R., Lossinsky, A. S., and Mossakowski, M. J. (1995). Ultrastructural studies of cerebral vascular spasm after cardiac arrest-related global cerebral ischemia in rats. Acta Neuropathol. 90, 432–440. doi: 10.1007/BF00294802
Xia, G. H., You, C., Gao, X. X., Zeng, X. L., Zhu, J. J., Xu, K. Y., et al. (2019). Stroke dysbiosis index (SDI) in gut microbiome are associated with brain injury and prognosis of stroke. Front. Neurol. 10:397. doi: 10.3389/fneur.2019.00397
Xu, F., Li, N., Wang, C., Xing, H., Chen, D., and Wei, Y. (2021a). Clinical efficacy of fecal microbiota transplantation for patients with small intestinal bacterial overgrowth: a randomized, placebo-controlled clinic study. BMC Gastroenterol. 21:54. doi: 10.1186/s12876-021-01630-x
Xu, K., Gao, X., Xia, G., Chen, M., Zeng, N., Wan, G. S., et al. (2021b). Rapid gut dysbiosis induced by stroke exacerbates brain infarction in turn. Gut 2020:323263.
Xu, R., and Wang, Q. (2016). Towards understanding brain-gut microbiome connections in Alzheimer’s disease. BMC Syst. Biol. 10(Suppl. 3):63. doi: 10.1186/s12918-016-0307-y
Yaghoubfar, R., Behrouzi, A., Ashrafian, F., Shahryari, A., Moradi, H. R., Choopani, S., et al. (2020). Modulation of serotonin signaling/metabolism by Akkermansia muciniphila and its extracellular vesicles through the gut-brain axis in mice. Sci. Rep. 10:22119. doi: 10.1038/s41598-020-79171-8
Yamada, Y., Yoshikawa, T., Naganuma, F., Kikkawa, T., Osumi, N., and Yanai, K. (2020). Chronic brain histamine depletion in adult mice induced depression-like behaviours and impaired sleep-wake cycle. Neuropharmacology 175:108179. doi: 10.1016/j.neuropharm.2020.108179
Yamashiro, K., Tanaka, R., Urabe, T., Ueno, Y., Yamashiro, Y., Nomoto, K., et al. (2017). Gut dysbiosis is associated with metabolism and systemic inflammation in patients with ischemic stroke. PLoS One 12:e0171521. doi: 10.1371/journal.pone.0171521
Yang, Q. W., Li, J. C., Lu, F. L., Wen, A. Q., Xiang, J., Zhang, L. L., et al. (2008). Upregulated expression of toll-like receptor 4 in monocytes correlates with severity of acute cerebral infarction. J. Cereb. Blood Flow Metab. 28, 1588–1596. doi: 10.1038/jcbfm.2008.50
Yilmaz, G., and Granger, D. N. (2010). Leukocyte recruitment and ischemic brain injury. Neuromol. Med. 12, 193–204. doi: 10.1007/s12017-009-8074-1
Yin, J., Liao, S. X., He, Y., Wang, S., Xia, G. H., Liu, F. T., et al. (2015). Dysbiosis of gut microbiota with reduced trimethylamine N-oxide level in patients with large-artery atherosclerotic stroke or transient ischemic attack. J. Am. Heart Assoc. 4:e002699. doi: 10.1161/JAHA.115.002699
Yin, Y., and Li, G. (2011). Hypoxia induces T Helper 17 cell upregulation in cultured peripheral blood mononuclear cells from chronic stage patients of severe cerebral infarction. Microbiol. Immunol. 55, 130–134. doi: 10.1111/j.1348-0421.2010.00301.x
Youngchaiyud, U., Coates, A. S., Whittingham, S., and Mackay, I. R. (1974). Cellular-immune response to myelin protein: absence in multiple sclerosis and presence in cerebrovascular accidents. Aust. N. Z. J. Med. 4, 535–538. doi: 10.1111/j.1445-5994.1974.tb03233.x
Yousef, H., Czupalla, C. J., Lee, D., Chen, M. B., Burke, A. N., Zera, K. A., et al. (2019). Aged blood impairs hippocampal neural precursor activity and activates microglia via brain endothelial cell VCAM1. Nat. Med. 25, 988–1000. doi: 10.1038/s41591-019-0440-4
Yunes, R., Poluektova, E., Vasileva, E., Odorskaya, M., Marsova, M., Kovalev, G., et al. (2020). A multi-strain potential probiotic formulation of GABA-producing Lactobacillus plantarum 90sk and bifidobacterium adolescentis 150 with antidepressant effects. Probiotic. Antimicrob. Proteins 12, 973–979. doi: 10.1007/s12602-019-09601-1
Zaydi, A., Lew, L.-C., Hor, Y.-Y., Jaafar, M., Chuah, L.-O., Yap, K.-P., et al. (2020). Lactobacillus plantarum DR7 improved brain health in aging rats via the serotonin, inflammatory and apoptosis pathways. Benef. Microbes 11, 753–766. doi: 10.3920/BM2019.0200
Zelante, T., Iannitti, R. G., Cunha, C., De Luca, A., Giovannini, G., Pieraccini, G., et al. (2013). Tryptophan catabolites from microbiota engage aryl hydrocarbon receptor and balance mucosal reactivity via interleukin-22. Immunity 39, 372–385. doi: 10.1016/j.immuni.2013.08.003
Zeng, X., Gao, X., Peng, Y., Wu, Q., Zhu, J., Tan, C., et al. (2019). Higher risk of stroke is correlated with increased opportunistic pathogen load and reduced levels of butyrate-producing bacteria in the gut. Front. Cell. Infect. Microbiol. 9:4. doi: 10.3389/fcimb.2019.00004
Zhang, D., Chen, G., Manwani, D., Mortha, A., Xu, C., Faith, J. J., et al. (2015). Neutrophil ageing is regulated by the microbiome. Nature 525, 528–532. doi: 10.1038/nature15367
Zhao, Y., and Lukiw, W. J. (2015). Microbiome-generated amyloid and potential impact on amyloidogenesis in Alzheimer’s disease (AD). J. Nat. Sci. 1:e138.
Zhao, Y., Wu, X., Li, X., Jiang, L. L., Gui, X., Liu, Y., et al. (2018). TREM2 is a receptor for β-amyloid that mediates microglial function. Neuron 97, 1023–1031. doi: 10.1016/j.neuron.2018.01.031
Zhou, R., Qian, S., Cho, W. C. S., Zhou, J., Jin, C., Zhong, Y., et al. (2022). Microbiota-microglia connections in age-related cognition decline. Aging Cell 21:e13599. doi: 10.1111/acel.13599
Keywords: aging, gut-brain axis, stroke, brain ischemia, amyloid, tau protein, microglia, astrocytes
Citation: Pluta R, Jabłoński M, Januszewski S and Czuczwar SJ (2022) Crosstalk between the aging intestinal microflora and the brain in ischemic stroke. Front. Aging Neurosci. 14:998049. doi: 10.3389/fnagi.2022.998049
Received: 19 July 2022; Accepted: 22 September 2022;
Published: 06 October 2022.
Edited by:
Jolanta Dorszewska, Poznan University of Medical Sciences, PolandReviewed by:
Aurel Popa-Wagner, University of Medicine and Pharmacy of Craiova, RomaniaJan Lehotsky, Comenius University, Slovakia
Copyright © 2022 Pluta, Jabłoński, Januszewski and Czuczwar. This is an open-access article distributed under the terms of the Creative Commons Attribution License (CC BY). The use, distribution or reproduction in other forums is permitted, provided the original author(s) and the copyright owner(s) are credited and that the original publication in this journal is cited, in accordance with accepted academic practice. No use, distribution or reproduction is permitted which does not comply with these terms.
*Correspondence: Ryszard Pluta, cGx1dGFAaW1kaWsucGFuLnBs