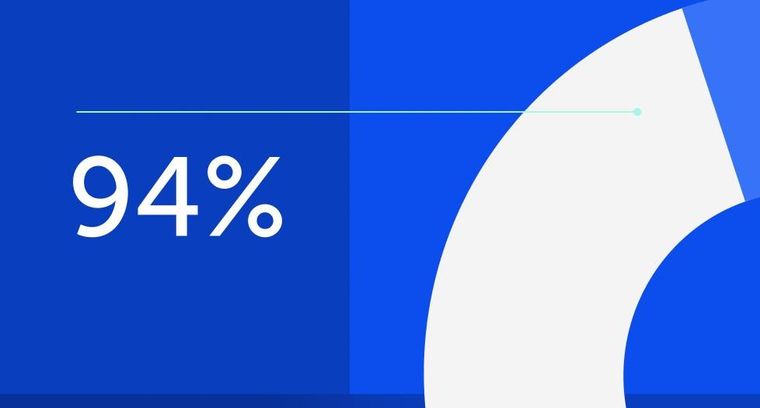
94% of researchers rate our articles as excellent or good
Learn more about the work of our research integrity team to safeguard the quality of each article we publish.
Find out more
REVIEW article
Front. Aging Neurosci., 11 August 2022
Sec. Neuroinflammation and Neuropathy
Volume 14 - 2022 | https://doi.org/10.3389/fnagi.2022.965169
This article is part of the Research TopicVascular Inflammation in Aging and NeurodegenerationView all 13 articles
Leukocyte migration into the central nervous system (CNS) represents a central process in the development of neurological diseases with a detrimental inflammatory component. Infiltrating neutrophils have been detected inside the brain of patients with several neuroinflammatory disorders, including stroke, multiple sclerosis and Alzheimer’s disease. During inflammatory responses, these highly reactive innate immune cells can rapidly extravasate and release a plethora of pro-inflammatory and cytotoxic factors, potentially inducing significant collateral tissue damage. Indeed, several studies have shown that neutrophils promote blood-brain barrier damage and increased vascular permeability during neuroinflammatory diseases. Recent studies have shown that neutrophils migrate into the meninges and choroid plexus, suggesting these cells can also damage the blood-cerebrospinal fluid barrier (BCSFB). In this review, we discuss the emerging role of neutrophils in the dysfunction of brain barriers across different neuroinflammatory conditions and describe the molecular basis and cellular interplays involved in neutrophil-mediated injury of the CNS borders.
The central nervous system (CNS) is physically separated from the peripheral milieu by specialized barriers ensuring a constant state of homeostasis and efficient neuronal function. It was previously considered an immune-privileged site, and this view was attributed to the low number of CNS immune cells and the presence of physical barriers limiting the communication between the systemic circulation and neural tissue (Bechmann et al., 2007; Galea et al., 2007). Over the past decades, this view has been challenged by several studies showing the presence of immune cells, including T and B lymphocytes, in the healthy brain (Anthony et al., 2003; Smolders et al., 2018). Additionally, recent reports describing CNS lymphatics, meningeal tissue and skull bone marrow as active immune hubs contributed to a broader vision of the immune cell dynamics within the healthy CNS (Louveau et al., 2015; Ribeiro et al., 2019; Alves de Lima et al., 2020; Brioschi et al., 2021; Cugurra et al., 2021; Herz et al., 2021; Rustenhoven et al., 2021). Particularly, under physiological conditions, meningeal immunity plays a key role in modulating cognition and behavior in a cytokine-dependent manner, suggesting that brain borders play an active role in brain functions (Filiano et al., 2016; Ribeiro et al., 2019; Alves de Lima et al., 2020; Herz et al., 2021). During CNS inflammatory pathologies, peripheral immune cells migrate into the brain and meninges and play a pathogenic role in several neuropathological disorders (Prinz and Priller, 2017; Rossi et al., 2021). Neutrophils constitute the most abundant immune cell population in the human peripheral blood (Juul et al., 1984), and their role in disease development has been shown in several CNS disorders such as stroke, multiple sclerosis (MS) and Alzheimer’s disease (AD) (Rossi et al., 2020, 2021). Their vast granule storage of enzymes potentially inducing collateral tissue damage, the capacity to rapidly extravasate and increase vascular permeability and the ability to favor tissue remodeling, suggest neutrophils may have a key role in the development CNS inflammatory disorders (Rossi et al., 2020; Fischer et al., 2022). This review summarizes recent data on the contribution of neutrophils to brain injury focusing on pathological mechanisms occurring at CNS borders. We discuss what is known about the involvement of neutrophils in the induction of endothelial injury, increased vascular permeability and degradation of extracellular matrix promoting neuroinflammation and neurodegeneration.
The CNS is physically separated from the peripheral milieu by two main barriers: the blood-brain barrier (BBB) and blood-cerebrospinal fluid barrier (BCSFB) (Figure 1). Although both barriers are characterized by high expression of junctional proteins and selective permeability capacities, they also have unique anatomical and molecular characteristics. The BBB is an endothelial structure of brain microvessels, which limits the passage of plasma molecules and blood cells into the brain and maintains the chemical composition of the neuronal “milieu” (Zlokovic, 2008). On the other hand, the BCSFB separates the blood from the CSF and was classically associated with the epithelium of the choroid plexus (ChP) and arachnoid matter (Brøchner et al., 2015; Engelhardt et al., 2017) (Figure 1). Overall, the CNS barriers not only protect the parenchyma, restricting the diffusion of pathogens and harmful blood molecules, but also play a critical role in the uptake of nutrients and excretion of byproducts of brain metabolism (Ballabh et al., 2004). The BBB consists of a monolayer of tightly packed and specialized non-fenestrated endothelial cells (ECs) brought together by junctional complexes, covering brain capillaries and postcapillary venules (Figure 1). It is fenced by the glia limitans formed by perivascular astrocyte endfeet and parenchymal basement membrane, which further restricts BBB permeability. This specialized and dynamic structure, together with the surveying microglia, astrocytes, pericytes, neuronal branches, and the acellular basement membrane, form the neurovascular unit (NVU) (Villabona-Rueda et al., 2019). Furthermore, the BBB represents a high-resistance electrical barrier, expressing transporters and efflux pumps for the regulation of ion fluctuations and solutes, and limiting the paracellular and transcellular movement of molecules. The inter-endothelial junctional structures are responsible for the “gate” function of ECs and consist of tight junction proteins (TJ), adherens junctions (AJs) and gap junctions (Chow and Gu, 2015; Tietz and Engelhardt, 2015; Sweeney et al., 2019; Saint-Pol et al., 2020). Brain endothelial TJs are the most apical cell-cell junctional complexes and constitute the core elements of the BBB with a crucial role in regulating paracellular permeability and maintaining cell polarity. They contain transmembrane proteins, including occludin claudins and junctional adhesion molecules, anchored to intracellular scaffolding proteins of the membrane-associated guanylate kinase family, also known as zonula occludens (ZO-1, -2, -3) (Zlokovic, 2008). The integral membrane proteins provide support to the TJs structure, with occludin contributing to the regulation and stabilization of the junctional structures (Cummins, 2012), whereas claudins are critical sealing determinants of paracellular “tightness” between adjacent ECs (Alahmari, 2021). Particularly, claudin-5, the most abundant transmembrane TJ protein at the BBB, plays a prominent role in maintaining the structural integrity of the vasculature and preventing the diffusion of small molecules through the intercellular space (Figure 2; Greene et al., 2019). Reduced expression of occludin, claudin-5, and ZO-1 is considered a sensitive indicator of BBB alterations associated with increased permeability during CNS diseases (Zlokovic, 2008; Cummins, 2012; Greene et al., 2019). Among the junctional complexes between the cerebral ECs, vascular endothelial (VE)-cadherin represents the major AJ transmembrane protein. VE-cadherin is linked to the cytoskeleton via catenins such as p120, β-catenin, plakoglobin, and γ-catenin, ensuring AJ assembly and BBB stability (Zlokovic, 2008; Li et al., 2018). Besides the organized junctional complexes, additional independent molecules are present at ECs junctions, including platelet-endothelial cell adhesion molecule-1 (PECAM-1 or CD31) and CD99. These proteins have homophilic and heterophilic binding capacity and have a role in the context of leukocyte transmigration during inflammatory responses (Lou et al., 2007; Zlokovic, 2008; Sullivan and Muller, 2014). Alterations in junctional molecules facilitate leukocyte transmigration during brain inflammation. Indeed, the binding of α4β1 integrin expressed on lymphocytes to the endothelial vascular adhesion molecule (VCAM-1) induces the phosphorylation of VE-cadherin and leads to the opening of AJ contacts, facilitating leukocyte transmigration (Vockel and Vestweber, 2013).
Figure 1. Schematic representation of neutrophil migration through the CNS barriers. CNS barriers limit the accessibility of circulating cells and molecules and can be divided into two main components: the Blood-Brain-Barrier (BBB) situated within the brain parenchyma and the blood-CSF-barrier (BCSFB) present in the choroid plexus (ChP) and in the meninges. Starting from the outer part of the CNS (depicted on the top left), the meninges represent a series of connective layers surrounding the brain, including dura mater and leptomeninges (arachnoid and pia mater). During CNS inflammatory diseases, neutrophils adhere in pial vessels and migrate into the SAS; these cells may then cross pia matter and migrate into the underlying brain parenchyma. Within the leptomeningeal vessels, neutrophils release MPO, potentially damaging endothelial cells and the extracellular matrix. On the top right, a representation of the choroid plexus shows the production of neutrophil chemoattractants CXCL1 and CXCL2 by the epithelial cells leading to neutrophil infiltration across the BCSFB. In both ChP stroma and within the CSF, neutrophils have been shown to produce LCN2. On the lower panel, a simplified model of the BBB is depicted, along with the classical neutrophil migration cascade. Inflamed BBB endothelial cells express adhesion molecules such as P- and E-selectins mediating capture and rolling through interaction with neutrophil PSGL-1 and CD44. Endothelial ICAM-1 and VCAM-1 mediate the arrest phase by binding neutrophil integrins LFA-1 and VLA-4, respectively. Firm adhesion and transmigration may contribute to increased BBB permeability (created with BioRender.com).
Figure 2. Molecular mechanisms mediating neutrophil-dependent BBB dysfunction. The BBB is a complex structure mainly composed of endothelial cells tightly bound together by junctional molecules, astrocytic endfeet and pericytes. During neuroinflammation, microglia and astrocytes produce IL-1b, which is a potent inducer of endothelial ICAM-1 and VCAM-1 and promotor of neutrophil infiltration. Engagement of neutrophil integrins as well as other stimuli, lead to the production of neutrophil extracellular traps (NETs) and release of inflammatory mediators including reactive oxygen species (ROS), lipocalin 2 (LCN2), myeloperoxidase (MPO) and matrix metalloproteinase 9 (MMP-9). These neutrophil-derived factors contribute to the reduction of tight junction proteins (claudin-5, occludin and ZO-1) and BBB breakdown. (Created with BioRender.com).
The BBB together with the BCSFB are considered part of the neurovascular system, providing active immune surveillance and supporting coordinated immune responses (Engelhardt et al., 2017; Mastorakos and McGavern, 2019). Even though the BCSFB is more accessible and permeable than the BBB, the latter has received more attention for its involvement in CNS pathologies (Felgenhauer, 1995; Tumani et al., 2017). Nevertheless, growing evidence suggests the BCSFB also plays a significant role in the communication between the periphery and the CNS during inflammatory neurological disorders. The BCSFB was mainly associated with two areas of the CNS: the ChP and the arachnoid membrane covering the subarachnoid space (SAS). The ChP is located in the lateral and fourth ventricles and is an epithelial tissue surrounding fenestrated vessels. It represents a major site of CSF production in the brain and strictly regulates the exchange of substances between the blood and the CSF (Lun et al., 2015). ChP capillaries do not have TJs, allowing the free movement of molecules through fenestrations and intercellular gaps. However, the choroidal BCSFB is characterized by the presence of junctional molecules in the epithelial lining of ChP, allowing selective permeability of blood components. Despite functional similarities between the BBB and the BSCFB, the choroidal BCSFB shows mainly the expression of claudins 1–3, 9, 12 and 22 and almost no expression of claudin-5, suggesting morphological differences that may lead to a more permeable barrier to small molecules, macromolecules and circulating immune cells (Kratzer et al., 2012; Tietz and Engelhardt, 2015). The pial microvessel blood-CSF barrier across pial microvessels is also considered part of the BCSFB (Brøchner et al., 2015). Endothelial cells isolated from the pial vessels show similarities with the BBB for some general ultrastructural features and transendothelial electrical resistance (Allt and Lawrenson, 1997). However, pial microvessels show some differences in the junctional systems compared to the BBB and lack astrocyte and NVU proximity, suggesting these vessels are more permeable and easier to cross by leukocytes during inflammatory responses (Allt and Lawrenson, 1997; Cassella et al., 1997).
The meningeal tissue is now considered an active and complex immune hub (Alves De Lima et al., 2020). From a structural point of view, the meninges are divided into three main layers: dura, arachnoid and pia. Dura mater is the outermost layer and is securely attached to the periosteum of the skull (Protasoni et al., 2011). It is composed of a dense and thick fibrous tissue supplied with lymphatic vessels, meningeal arteries and veins (Protasoni et al., 2011). The arachnoid mater is an avascular, thin and translucent membrane forming a barrier between the dura mater and the CSF flowing in the SAS (Yasuda et al., 2013). This space includes trabeculae and collagen bundles generated by fibroblast-like cells, which connect the arachnoid to the pia mater (Alcolado et al., 1988; Saboori and Sadegh, 2015). The deepest layer of the meninges is the pia mater, a thin and transparent membrane composed of connective tissue permeable to solutes and containing a capillary network that nourishes the brain (Adeeb et al., 2013). The pia mater is anchored on the brain surface by astrocyte processes and together with the arachnoid matter forms the leptomeninges (Derk et al., 2021). In addition to their protective role, the meninges also constitute a route of drainage for brain interstitial fluid to lymphatic vessels and deep cervical lymph nodes (Natale et al., 2021), providing communication between the CNS and the immune system. The bona fide barriers from the meningeal tissue can be subdivided into three main interfaces: (1) the BCSFB associated with the arachnoid layer separating the dura and its fenestrated vessels from the CSF; (2) the BCSFB at the level of SAS pial microvessels; and (3) the pial surface layer, which together with glia limitans is considered a brain-CSF barrier (Brøchner et al., 2015). The junctional components of the meningeal barriers are less studied, but recent work identified claudin-11 as a marker for the arachnoid BCSFB (Brøchner et al., 2015). However, further studies are needed to better understand the structural and molecular features of meningeal barriers and how they regulate brain function during health and disease.
Neuroinflammation is a well-defined pathological feature of several neurodegenerative disorders and associates with BBB structural changes and increased vascular permeability (Zlokovic, 2008; Rossi et al., 2011; Zenaro et al., 2017; Liebner et al., 2018). For example, studies performed in stroke models and experimental autoimmune encephalomyelitis (EAE), the most common model of MS, have shown increased BBB permeability due to a reduction of claudin-5, occludin, and ZO-1 levels in the mouse brain (Jiao et al., 2011; Wang et al., 2016). Similar results were obtained in cerebral amyloid-beta angiopathy in AD post-mortem brains, suggesting the reduction of TJ molecules represents a general feature of BBB dysfunction across several brain diseases (Carrano et al., 2012). The redistribution of inter-endothelial junctional proteins during BBB dysfunction depends on their phosphorylation state, which can be affected by growth factors and inflammatory cytokines (Van Itallie and Anderson, 2018). Vascular endothelial growth factor (VEGF) is a potent angiogenic factor that induces rapid phosphorylation of occludin and ZO-1, disrupting the TJs organization and promoting endothelial permeability (Antonetti et al., 1999; Wang et al., 2001; Storkebaum and Carmeliet, 2004; Murakami et al., 2009). Moreover, pro-inflammatory cytokines, including interferon (IFN)-γ, tumor necrosis factor (TNF)-α and interleukin (IL)-1β, also affect the molecular mechanisms associated with TJs integrity (Capaldo and Nusrat, 2009). Particularly, IFN-γ can directly modify the barrier function of TJs in cultured brain ECs by inducing Rho kinase activity, leading to actin cytoskeletal contractions and junctional disruption (Bonney et al., 2019). In addition, IL-1β reduces the expression of ZO-1 and transendothelial electrical resistance, increasing the paracellular permeability, the expression of adhesion molecules, and leukocyte migration across BBB models in vitro (Labus et al., 2014). During neuroinflammation, the release of IL-1β by activated microglia increases the BBB leakage and suppresses the capacity of astrocytes to maintain BBB integrity. Furthermore, IL-1β stimulates astrocytes to produce pro-inflammatory cytokines, promoting BBB breakdown and increased leukocyte migration (Wang et al., 2014). Exposure of brain ECs to pro-inflammatory cytokines also triggers the expression of adhesion molecules, such as P- and E- selectins, VCAM-1 and intercellular adhesion molecule-1 (ICAM-1), which may bind their counter-ligands on activated circulating immune cells, allowing leukocyte extravasation in the inflamed brain (Wong and Dorovini-Zis, 1992; Rahman et al., 2000; Hauptmann et al., 2020). Despite being constitutively expressed on brain ECs, ICAM-1 is further up-regulated in response to pro-inflammatory mediators, and its engagement activates signal transduction pathways leading to increased BBB permeability (Adamson et al., 2002). BBB integrity can also be affected by oxidative stress, particularly by the imbalance between the generation of reactive oxygen species (ROS) and their elimination by scavenger systems. High levels of ROS trigger intracellular pathways leading to TJ rearrangement and matrix metalloproteinase (MMP) activation, which directly compromise BBB integrity. In vitro studies demonstrated that H2O2 induces cytoskeleton dysfunction and alters the localization of occludin and ZO-1, increasing paracellular permeability of brain endothelial cells (Lee et al., 2004; Anasooya Shaji et al., 2019). ROS also enhances the release of MMPs by brain endothelial cells, acting directly on TJ proteins and degrading the extracellular matrix (Aexander et al., 2002; Harada et al., 2012). Large amounts of ROS can be released by parenchymal-resident cells, such as activated microglia, leading to a loss of BBB integrity. Particularly, the microglial NADPH oxidase system is the main producer of superoxide anion (O2–), which damages ECs and increases BBB permeability (Kahles et al., 2007; Rojo et al., 2014). The principal source of ROS from CNS infiltrating peripheral immune system cells is represented by activated neutrophils, which are professional phagocytes with a strong NADPH oxidase machinery and may also contribute to BBB damage. Neutrophil adhesion to inflamed cerebral vasculature engages integrins leading to ROS production and release of both granule enzymes and complex web-like structures of decondensed DNA fibers and antimicrobial molecules, called neutrophil extracellular traps (NET) (Figure 2; Pietronigro et al., 2017). Thus, migrating neutrophils across the CNS barriers release inflammatory and cytotoxic factors promoting endothelial injury and vascular permeability.
Leukocytes receive a variety of signals upon interaction with inflamed endothelial cells, facilitating a cascade of adhesive events, including tethering and rolling, integrin activation, arrest (or firm adhesion), luminal crawling and diapedesis (also called transmigration) (Ley et al., 2007; Vestweber, 2015). Tethering and rolling of circulating leukocytes in inflamed CNS venules are mainly mediated by endothelial P- and E-selectin and their counter-ligands P-selectin glycoprotein ligand-1 (PSGL-1), TIM (T cell immunoglobulin and mucin domain)-1 glycoprotein and CD44 (Siegelman et al., 2000; Piccio et al., 2002, 2005; Battistini et al., 2003; Fabene et al., 2008; Rossi et al., 2011; Angiari and Constantin, 2014; Angiari et al., 2014; Pietronigro et al., 2016; Zenaro et al., 2017; Figure 1). Leukocyte arrest in CNS venules is mediated by the VLA (very late antigen)-4 integrin binding to VCAM-1 and LFA (lymphocyte functional antigen)-1 and Mac-1 integrins binding to ICAM-1 and ICAM-2 (Figure 1; Engelhardt and Ransohoff, 2005; Rossi et al., 2011). Whereas activated T cells mainly rely on VLA-4 (α4β1 integrin) for their intravascular arrest, neutrophils highly depend on β2 integrins (LFA-1 and Mac-1) to arrest and crawl on inflamed brain endothelium (Rossi et al., 2011; Gorina et al., 2014; Zenaro et al., 2015; Pietronigro et al., 2019). However, VLA-4 integrin represents an alternative pathway for neutrophil adhesion, whereas VCAM-1 is a marker of CNS vascular inflammation, suggesting that VLA-4-VCAM-1 interactions may also control neutrophil adhesion during CNS inflammatory diseases (Johnston and Kubes, 1999).
The capacity of neutrophils to increase vascular permeability has been known for four decades, and the interplay between neutrophil adhesion and vascular damage has been studied in several pathological conditions, including brain diseases (Wedmore and Williams, 1981; Wang and Doerschuk, 2002; DiStasi and Ley, 2009; Rossi et al., 2020). In stroke models, neutrophils adhere to cerebral microvessels one hour after stroke induction, suggesting they are early contributors to vascular dysfunction (Hallenbeck et al., 1986; Kataoka et al., 2004; Sienel et al., 2022). Neutrophils can also rapidly adhere in cerebral vessels after status epilepticus induction and contribute to endothelial damage promoting chronic recurrent seizures (Fabene et al., 2008). Interestingly, in both focal ischemia and epilepsy models, it has been suggested that intravascular neutrophil adhesion per se without transmigration is sufficient to induce BBB damage and neuronal dysfunction and death, pointing to neutrophil adhesion mechanisms as drug targets for CNS diseases (Fabene et al., 2008; Sienel et al., 2022).
Studies in EAE models also demonstrated the pathogenic role of neutrophils and the contribution of these cells to blood-spinal cord barrier (BSCB) disruption (Wu et al., 2010; Aubé et al., 2014). These data were confirmed by the analysis of postmortem CNS samples of MS patients showing infiltrated neutrophils in areas of BBB or BSCB leakage. However, whereas ICAM-1 and VCAM-1 have been shown to be upregulated on CNS microvascular endothelial cells during EAE, the molecular mechanisms controlling neutrophil adhesive interactions with the BBB or BSCB are still unclear (Steffen et al., 1994). A role for neutrophils has also been shown in AD, and our group has demonstrated that LFA-1 integrin mediates neutrophil interaction with brain ECs expressing ICAM-1 in transgenic mice with AD-like disease (Zenaro et al., 2015). In addition to the adhesion capacity on the vascular wall, neutrophils can also stall in brain capillaries, obstructing blood flow. Indeed, neutrophil depletion induces capillary reperfusion and reduces brain damage in experimental models of stroke and AD (Cruz Hernández et al., 2019; El Amki et al., 2020). Together, these studies suggest a complex detrimental role for neutrophils in CNS inflammatory diseases by increasing vascular permeability following adhesion to the ECs and inducing ischemic phenomena by plugging cerebral capillaries.
During inflammatory responses, neutrophils can release a plethora of factors potentially contributing to tissue injury. Furthermore, it has been known that adhesion on vascular endothelium via engagement of β2 integrins leads to neutrophil activation and consequent secretion of inflammation mediators such as ROS and cytotoxic granule enzymes (Figure 2; Richter et al., 1990; Cheung et al., 1993; DiStasi and Ley, 2009). Indeed, in animal models of stroke, NET formation and release of neutrophil elastase (NE) have been reported to induce increased BBB permeability, and the inhibition of these two factors reduces brain injury and promotes recovery (Kang et al., 2020). Neutrophils can also produce MMPs, which have been previously shown to represent a key factor in the induction of vascular damage (Aexander et al., 2002; Kolaczkowska and Kubes, 2013). MMPs were shown to be increased in the CNS during several neuroinflammatory conditions, including MS, stroke, epilepsy, and AD, although their presence was not clearly associated to neutrophil infiltration (Backstrom et al., 1996; Clark et al., 1997; Kieseier et al., 1998; Nygårdas and Hinkkanen, 2002; Solé et al., 2004; Wilczynski et al., 2008; Takács et al., 2010; Quirico-Santos et al., 2013; Py et al., 2014). However, among all MMPs produced by neutrophils, MMP-9 was highly correlated with vascular damage during diseases such as tuberculosis, myocardial infarction and cystic fibrosis (Kurihara et al., 2012; Halade et al., 2013; Garratt et al., 2015; Ong et al., 2017; Gelzo et al., 2022). MMP-9 was also associated with BBB dysfunction, and its expression is involved in the disruption of BBB TJs and degradation of basal lamina (Figure 2; Mun-bryce et al., 1998; Asahi et al., 2001; Moxon-Emre and Schlichter, 2011; Li et al., 2013). Furthermore, MMP-9 expression was clearly correlated with the presence of granulocytes in models of stroke and ischemia-reperfusion injury, in which neutrophils were considered the main source of MMP-9 (Romanic et al., 1998; Gidday et al., 2005; Turner and Sharp, 2016). In addition, by using MMP-9–/– mice, several studies confirmed the critical role of this enzyme in the induction of BBB permeability in stroke models and EAE (Dubois et al., 1999; Asahi et al., 2001; Agrawal et al., 2006; Svedin et al., 2007). Finally, MMP-9-positive neutrophils have been found surrounding brain microvessels with severe type IV collagen degradation and BBB breakdown in patients with stroke, clearly showing a strong association between neutrophils, MMP-9 and BBB damage also during human CNS disease (Rosell et al., 2008). Interestingly, the C-1562T polymorphism in the MMP-9 gene, which is associated with an elevated MMP-9 expression, increases the susceptibility to neuropsychiatric conditions, suggesting that neutrophil MMP-9 may represent a pathogenic factor also in mental disorders (Rybakowski et al., 2009a,b).
Human MMP-9 is covalently linked to lipocalin (LCN2), which increases its stability and confers protection from proteolytic degradation (Tschesche et al., 2001). LCN2 expression is increased during aging and several neuroinflammatory conditions, including AD, MS and stroke suggesting a role for LCN2 in CNS disorders (Anwaar et al., 1998; Marques et al., 2012; Chou et al., 2015; Weng and Chou, 2015; Al Nimer et al., 2016; Dekens et al., 2017). However, whereas in vitro data suggested a possible role for LCN2 in preserving BBB integrity, other studies clearly showed that LCN2 expression was found in cerebral endothelial cells and neutrophils in a mouse model of stroke, and its inhibition significantly reduced BBB leakage in vivo (Du et al., 2019; Wang et al., 2020). In support of these data, LCN2 was increased in the CNS and CSF in patients with vascular dementia, correlating with reduced expression of TJ proteins and increased BBB permeability, thus suggesting a role for LCN2 in BBB dysfunction (Kim et al., 2017; Llorens et al., 2020).
Myeloperoxidase (MPO) is the most abundant protein stored in neutrophil azurophilic granules. It can be released upon β2 integrin cross-linking in neutrophils and its expression was associated with areas of myeloid cell infiltration in stroke and MS (Figure 2; Matsuo et al., 1994; Walzog et al., 1994; Breckwoldt et al., 2008; Chen et al., 2008; Sajad et al., 2009; Forghani et al., 2012, 2015; Pulli et al., 2015). The link between MPO secretion and endothelial damage has also been reported. Indeed, pharmacological inhibition of MPO attenuates endothelial dysfunction in a mouse model of atherosclerosis (Cheng et al., 2019). Notably, MPO-deficient mice showed decreased leakage in LPS-induced BBB inflammation compared to wild-type littermates (Üllen et al., 2013). These results were supported by in vitro data showing that the MPO-H2O2-Cl2 system causes barrier dysfunction in primary brain microvascular endothelial cells by inducing alterations of TJs and AJs (Üllen et al., 2013). Using the MPO inhibitor 4-aminobenzoic acid hydrazide, BBB dysfunction was partially rescued, demonstrating a direct link between BBB increased permeability and MPO release (Üllen et al., 2013). Moreover, in EAE and stroke mouse models, inhibition of MPO with N-acetyl lysyltyrosylcysteine amide prevented BBB breakdown and reduced disease severity, further demonstrating a role for MPO in CNS barrier breakdown (Zhang et al., 2016; Yu et al., 2018). Of note, high expression of MPO was associated with ischemic stroke, and MPO inhibition had beneficial effects, suggesting that MPO may represent a therapeutic target in stroke (Malle et al., 2007; Kim et al., 2016; Kim H. J. et al., 2019; Wang et al., 2022). Interestingly, epidemiological studies associated MPO polymorphisms with an increased risk of neurodegenerative diseases (Reynolds et al., 1999; Crawford et al., 2001; Combarros et al., 2002; Zappia et al., 2004; Pope et al., 2006). Moreover, MPO+ cells (probably neutrophils) were increased in the brain in subjects with AD and Parkinson’s disease (PD) (Zenaro et al., 2015; Gellhaar et al., 2017), thus supporting a role for this enzyme in neurodegeneration. Importantly, recent studies by Smyth et al. have shown that MPO staining is mainly associated with infiltrating neutrophils in the AD brain, further providing evidence of a role for neutrophil-derived inflammatory factors in BBB dysfunction (Smyth et al., 2022).
The ChP constitutively expresses adhesion molecules and cytokines supporting continuous immune surveillance of the CNS (Steffen et al., 1996; Meeker et al., 2012; Kunis et al., 2013; Lun et al., 2015; Strominger et al., 2018). Indeed, the stromal compartment of the ChP is populated by several types of cells, including immune cells of peripheral origin (Marques et al., 2012; Schmitt et al., 2012; Szmydynger-Chodobska et al., 2012; Marques and Sousa, 2015). During inflammatory responses, the ChP epithelium and blood vessels further upregulate adhesion receptors and chemokines, facilitating the migration of blood leukocytes into the CSF (Steffen et al., 1994; Vercellino et al., 2008; Marques et al., 2009, 2012; Kunis et al., 2013). In addition to immune cell migration, structural and functional changes at the level of ChP are present during various brain disorders, and BCSFB breakdown may precede clinical symptoms (Schwartz and Baruch, 2014; Alicioglu et al., 2017; Saul et al., 2020; Gião et al., 2022; Mold and Exley, 2022). During AD, the dysfunctional ChP is characterized by alterations of secretory, barrier, transport, and immune functions. Moreover, several imaging studies in AD subjects showed premature ChP aging with increased lipofuscin vacuoles and Biondi bodies compared to age-matched healthy controls (Miklossy et al., 1998; Wen et al., 1999; Serot et al., 2000; Krzyzanowska and Carro, 2012; Tadayon et al., 2020). In addition, the ChP of AD patients have a lower rate of CSF production (Silverberg et al., 2001), show an increase of several inflammatory transcripts and downregulation of claudin-5, claudin-11, and claudin-18, pointing to a role for ChP breakdown in disease development (Bergen et al., 2015; Kant et al., 2018). Expression of TJ proteins in ChP epithelial cells has also been studied in MS. Although the specific functions of individual TJ proteins are unclear, the analysis of post-mortem brain tissues from MS patients showed a selective loss of claudin-3 compared to control tissues. Claudin-3 displays ChP selectivity and is expressed at the apical parts of epithelial cells, acting as a sealant in a similar manner as claudin-5 at the BBB level (Steinemann et al., 2016). Confirming these data, mice lacking claudin-3 showed impaired BCSFB function and a faster onset and increased EAE severity, clearly indicating a role for claudin-3 in ChP breakdown in CNS autoimmune diseases (Kooij et al., 2014).
Neutrophils can migrate into the CSF through a dysfunctional ChP in several CNS inflammatory conditions. Peripheral administration of the TLR2 ligand PAM3CSK4, a prototypic Gram-positive bacterial lipopeptide, induces the migration of neutrophils through the ChP barrier (Mottahedin et al., 2017). Neutrophils can also infiltrate the ChP in EAE mice and represent a source of CSF LCN2 during early disease phases (Figure 1; Marques et al., 2012). Supporting these data, neutrophils migrate into the ChP stroma in MS patients, and this process is probably favored by a high expression of adhesion molecules on ChP blood vessels (Vercellino et al., 2008; Rodríguez-Lorenzo et al., 2020). Similarly, in models of traumatic brain injury (TBI), the choroidal epithelium produces CXCL1 and CXCL2 chemokines, which promote neutrophil migration, supporting the view that ChP represents an entry point for neutrophils invasion of the damaged brain (Figure 1; Szmydynger-Chodobska et al., 2009). Also, neutrophils migrate in the ChP in stroke models, further showing that ChP is a key site for neutrophil infiltration (Otxoa-De-Amezaga et al., 2019). Despite a clear demonstration of neutrophil migration into the ChP during CNS diseases, the molecular mechanisms promoting neutrophil-dependent ChP damage are unclear. However, previous studies have shown a correlation between ChP dysfunction and increased expression of MMP-8 and MMP-9 (Batra et al., 2010). These two enzymes have been shown to mediate neutrophil-dependent damage in other pathological contexts, suggesting this may be the case also during CNS inflammatory conditions. Collectively, these data suggest that ChP is a gateway for neutrophil entry into the brain, and further studies are needed to better understand neutrophil contribution to BCSFB breakdown.
The meninges also serve as an important route for immune cell entry into the CNS (Ransohoff et al., 2003; Derk et al., 2021). Indeed, dural venous sinuses were recently shown to be active sites of immune cell trafficking and contain both adaptive and innate immune cells (Mrdjen et al., 2018; Jordão et al., 2019; Van Hove et al., 2019; Rustenhoven et al., 2021). Particularly, recent results have shown that dural sinuses regulate T cell trafficking and contain APCs presenting CSF antigens to patrolling T cells, demonstrating a role for dura in CNS immune surveillance (Rustenhoven et al., 2021). Furthermore, by releasing cytokines, meningeal immune cells regulate several brain functions, including spatial learning (Derecki et al., 2010; Radjavi et al., 2014; Brombacher et al., 2021), short-term memory (Ribeiro et al., 2019), sensory responses (Oetjen et al., 2017) and adult hippocampal neurogenesis (Wolf et al., 2009). Notably, recent studies described meningeal-bone marrow channels directly feeding the meninges with immune cells (Herisson et al., 2018; Brioschi et al., 2021; Cugurra et al., 2021), suggesting that a dysfunctional meningeal immunity may contribute to CNS disorders, such as MS, AD, and PD (Russi and Brown, 2015; Silva and Ferrari, 2019; Zou et al., 2019; Mentis et al., 2021). The meningeal BSCFB is more permissive to the entry of immune cells and large molecules compared to the highly restrictive BBB (Ransohoff et al., 2003). The permeability changes of the arachnoid barrier were less studied in CNS diseases. However, recent studies showed that claudin 11, a TJ protein enriched in the arachnoid barrier, is downregulated in EAE mice (Uchida et al., 2019), indicating a disruption of the arachnoid BCSFB. Increased permeability of the leptomeningeal vessels has also been shown in MRI studies in several brain disorders, including stroke, CNS infections and cerebral amyloid angiopathy (Ineichen et al., 2022). Leptomeninges are now recognized as key players in the development of MS and EAE (Pikor et al., 2015; Russi and Brown, 2015; Rua and McGavern, 2018; Wicken et al., 2018). Indeed, subpial lesions are considered the most common type of lesions in MS patients and autoreactive effector T cells have been shown to infiltrate the leptomeninges via pial microvessels, demonstrating a fundamental immunological role for this vascular district in CNS autoimmunity (Bartholomäus et al., 2009; Schläger et al., 2016; Filippi et al., 2018). Accordingly, reports of gadolinium enhancement in the leptomeningeal compartment have been described in MS, confirming a role for meningeal inflammation in MS (Absinta et al., 2015).
Several studies performed in animal models of AD, MS, TBI, stroke and systemic inflammation have shown increased expression of the adhesion molecules, such as ICAM-1, VCAM-1 and P-selectin, as well as consequent leukocyte adhesion in pial microvasculature (Kerfoot and Kubes, 2002; Piccio et al., 2002; Mel’nikova, 2009; Zhou et al., 2009; Zenaro et al., 2015; Szmydynger-Chodobska et al., 2016; Dusi et al., 2019; Lodygin et al., 2019; Pietronigro et al., 2019). These findings support the idea that meningeal tissue represents a gateway for immune cell access into the CNS and open the possibility of a neutrophil contribution to BCSFB dysfunction (Walker-Caulfield et al., 2015). Indeed, studies performed in patients with stroke and its animal model demonstrated the presence of extravasated neutrophils in the leptomeninges, suggesting a role for neutrophils in meningeal inflammation (Perez-de-Puig et al., 2015; Kim S. W. et al., 2019). Also, in a mouse model of subarachnoid hemorrhage, neutrophils infiltrated the meninges and were associated with neuronal damage (Coulibaly et al., 2021). Notably, mice deficient for MPO lacked neuronal dysfunction suggesting this lysosomal enzyme represents a putative mechanism of neutrophil-dependent damage to the underlying brain tissue in subarachnoid hemorrhage (Coulibaly et al., 2021). Furthermore, studies performed by our group in mouse models of AD showed that neutrophils migrate in pial vessels and brain parenchyma during early disease stages via an LFA-1-dependent mechanism promoting cognitive deficit and neuropathological hallmarks of AD (Zenaro et al., 2015). Furthermore, it has been suggested that neutrophil influx in the leptomeninges of EAE mice during pre-clinical stages of the disease promotes early BCSFB breakdown facilitating the access of other immune cells into the CNS (Christy et al., 2013). Overall, although current data provide evidence of neutrophil accumulation in the leptomeninges in models of CNS diseases, how neutrophils promote BCSFB dysfunction and neuronal damage is still largely unknown.
The role of neutrophils in the induction of BBB damage is now well documented in animal models of neuroinflammation and patients with CNS diseases. Although less investigated, the contribution of neutrophils to BSCFB breakdown is now emerging from recent studies on stroke, AD and MS. Neutrophil-mediated damage to CNS barriers since early disease stages may pave the way for the recruitment of other leukocyte populations during brain inflammatory diseases, and further studies are needed to clarify this interesting aspect (Christy et al., 2013; Rossi et al., 2020, 2021). However, whereas neutrophil accumulation in the meninges and ChP was clearly demonstrated, the intravascular adhesion mechanisms mediating neutrophil-endothelial interactions and the interplay between neutrophils and other barrier cells, such as ChP epithelial cells, are still unknown. Indeed, in vivo tracking of migrating neutrophils in animal models of CNS disorders using advanced microscopy together with non-invasive imaging of radioactive leukocyte tracers may improve our future understanding of neutrophil interaction with CNS borders (Mel’nikova, 2009; Zhou et al., 2009; Szmydynger-Chodobska et al., 2016; Lodygin et al., 2019; Pietronigro et al., 2019). Neutrophils release their arsenal of cytotoxic molecules during inflammatory responses, potentially causing significant collateral tissue damage (Kolaczkowska and Kubes, 2013). However, the molecular mechanisms contributing to neutrophil-dependent inflammation of CNS barriers leading to neuronal and glial cell dysfunction are largely unknown and may represent a new field of investigation. Finally, the elucidation of the molecular pathways underlying neutrophil contribution to CNS diseases may help design new therapies for neurological disorders such as stroke, MS and AD.
All authors contributed to the literature search and writing the review.
This work was supported in part by the European Research Council (ERC) advanced (grant no. 695714) IMMUNOALZHEIMER and the ERC Proof of Concept (grant no. 693606) IMPEDE and (grant no. 101069397) NeutrAD (to GC), Italian Ministry of University and Research, PNRR National Centers Program (National Biodiversity Future Center – NBFC) (to GC); “Alzheimer’s Drug Discovery Foundation (ADDF), USA (to GC), Fondazione Italiana Sclerosi Multipla (FISM), Genoa, Italy project 2019/PR-single/044 (to EZ), INVITE European Union’s Horizon 2020 research and innovation program under the Marie Skłodowska-Curie (grant agreement no. 754345) to BS-L. Fondazione Umberto Veronesi post-doctoral fellowship 2019 and 2020 (to EZ).
The authors declare that the research was conducted in the absence of any commercial or financial relationships that could be construed as a potential conflict of interest.
All claims expressed in this article are solely those of the authors and do not necessarily represent those of their affiliated organizations, or those of the publisher, the editors and the reviewers. Any product that may be evaluated in this article, or claim that may be made by its manufacturer, is not guaranteed or endorsed by the publisher.
Absinta, M., Vuolo, L., Rao, A., Nair, G., Sati, P., and Cortese, I. C. M. (2015). Gadolinium-based MRI characterization of leptomeningeal inflammation in multiple sclerosis. Neurology 85, 18–28. doi: 10.1212/WNL.0000000000001587
Adamson, P., Wilbourn, B., Etienne-Manneville, S., Calder, V., Beraud, E., and Milligan, G. (2002). Lymphocyte trafficking through the blood–brain barrier is dependent on endothelial cell heterotrimeric G-protein signaling. FASEB J. 16, 1185–1194. doi: 10.1096/fj.02-0035com
Adeeb, N., Deep, A., Griessenauer, C. J., Mortazavi, M. M., Watanabe, K., and Loukas, M. (2013). The intracranial arachnoid mater: A comprehensive review of its history, anatomy, imaging, and pathology. Child’s Nervous Syst. 29, 17–33. doi: 10.1007/s00381-012-1910-x
Aexander, J. S., Elrod, J. W., Alexander, J. S., and Elrod, J. W. (2002). Extracellular matrix, junctional integrity and matrix metalloproteinase interactions in endothelial permeability regulation. J. Anatomy 200, 561–574. doi: 10.1046/j.1469-7580.2002.00057.x
Agrawal, S., Anderson, P., Durbeej, M., van Rooijen, N., Ivars, F., Opdenakker, G., et al. (2006). Dystroglycan is selectively cleaved at the parenchymal basement membrane at sites of leukocyte extravasation in experimental autoimmune encephalomyelitis. J. Exp. Med. 203, 1007–1016. doi: 10.1084/jem.20051342
Al Nimer, F., Elliott, C., Bergman, J., Khademi, M., Dring, A. M., and Aeinehband, S. (2016). Lipocalin-2 is increased in progressive multiple sclerosis and inhibits remyelination. Neurol. Neuroimmunol. Neuroinflamm. 3:e191. doi: 10.1212/nxi.0000000000000191
Alahmari, A. (2021). Blood-Brain Barrier Overview: Structural and Functional Correlation. Neural Plast. 2021:6564585. doi: 10.1155/2021/6564585
Alcolado, R., Weller, R. O., Parrish, E. P., and Garrod, D. (1988). The cranial arachnoid and pia mater in man: Anatomical and ultrastructural observations. Neuropathol. Appl. Neurobiol. 14, 1–17. doi: 10.1111/J.1365-2990.1988.TB00862.X
Alicioglu, B., Yilmaz, G., Tosun, O., and Bulakbasi, N. (2017). Diffusion-weighted magnetic resonance imaging in the assessment of choroid plexus aging. Neuroradiol. J. 30, 490–495. doi: 10.1177/1971400917714280
Allt, G., and Lawrenson, J. G. (1997). Short (or topical). review. Is the pial microvessel a good model for blood-brain barrier studies? Brain Res. Rev. 24, 67–76. doi: 10.1016/s0165-0173(97)00011-8
Alves de Lima, K., Rustenhoven, J., da Mesquita, S., Wall, M., Salvador, A. F., and Smirnov, I. (2020). Meningeal γδ T cells regulate anxiety-like behavior via IL-17a signaling in neurons. Nat. Immunol. 21, 1421–1429. doi: 10.1038/S41590-020-0776-4
Alves De Lima, K., Rustenhoven, J., and Kipnis, J. (2020). Meningeal immunity and its function in maintenance of the central nervous system in health and disease. Annu. Rev. Immunol. 38, 597–620. doi: 10.1146/annurev-immunol-102319-103410
Anasooya Shaji, C., Robinson, B. D., Yeager, A., Beeram, M. R., Davis, M. L., and Isbell, C. L. (2019). The tri-phasic role of hydrogen peroxide in blood-brain barrier endothelial cells. Sci. Rep. 9:133. doi: 10.1038/s41598-018-36769-3
Angiari, S., and Constantin, G. (2014). Regulation of T cell trafficking by the T cell immunoglobulin and mucin domain 1 glycoprotein. Trends Mol. Med. 20, 675–684. doi: 10.1016/j.molmed.2014.10.003
Angiari, S., Donnarumma, T., Rossi, B., Dusi, S., Pietronigro, E., and Zenaro, E. (2014). TIM-1 glycoprotein binds the adhesion receptor P-selectin and mediates T cell trafficking during inflammation and autoimmunity. Immunity 40, 542–553. doi: 10.1016/j.immuni.2014.03.004
Anthony, I. C., Crawford, D. H., and Bell, J. E. (2003). B lymphocytes in the normal brain: Contrasts with HIV-associated lymphoid infiltrates and lymphomas. Brain 126, 1058–1067. doi: 10.1093/BRAIN/AWG118
Antonetti, D. A., Barber, A. J., Ann Hollinger, L., Wolpert, E. B., and Gardner, T. W. (1999). Vascular endothelial growth factor induces rapid phosphorylation of tight junction proteins occludin and zonula occluden 1 a potential mechanism for vascular permeability in diabetic retinopathy and tumors. J. Biol. Chem. 274, 23463–23467. doi: 10.1074/jbc.274.33.23463
Anwaar, I., Gottsäter, A., Ohlsson, K., Mattiasson, I., Lindgärde, F., and Anwaar, I. (1998). Increasing levels of leukocyte-derived inflammatory mediators in plasma and cAMP in platelets during follow-up after acute cerebral ischemia. Cerebrovasc. Dis. 8, 310–317. doi: 10.1159/000015873
Asahi, M., Wang, X., Mori, T., Sumii, T., Jung, J.-C., Moskowitz, M. A., et al. (2001). Effects of matrix metalloproteinase-9 gene knock-out on the proteolysis of blood-brain barrier and white matter components after cerebral ischemia. J. Neurosci. 21, 7724–7732. doi: 10.1523/jneurosci.21-19-07724.2001
Aubé, B., Lévesque, S. A., Paré, A., Chamma, É, Kébir, H., Gorina, R., et al. (2014). Neutrophils mediate blood–spinal cord barrier disruption in demyelinating neuroinflammatory diseases. J. Immunol. 193, 2438–2454. doi: 10.4049/jimmunol.1400401
Backstrom, J. R., Lim, G. P., Cullen, M. J., and Tökés, Z. A. (1996). Matrix metalloproteinase-9 (MMP-9). is synthesized in neurons of the human hippocampus and is capable of degrading the amyloid-β peptide (1–40). J. Neurosci. 16:7910. doi: 10.1523/JNEUROSCI.16-24-07910.1996
Ballabh, P., Braun, A., and Nedergaard, M. (2004). The blood-brain barrier: An overview: Structure, regulation, and clinical implications. Neurobiol. Dis. 16, 1–13. doi: 10.1016/J.NBD.2003.12.016
Bartholomäus, I., Kawakami, N., Odoardi, F., Schläger, C., Miljkovic, D., and Ellwart, J. W. (2009). Effector T cell interactions with meningeal vascular structures in nascent autoimmune CNS lesions. Nature 462, 94–98. doi: 10.1038/nature08478
Batra, A., Latour, L. L., Ruetzler, C. A., Hallenbeck, J. M., Spatz, M., Warach, S., et al. (2010). Increased plasma and tissue MMP levels are associated with BCSFB and BBB disruption evident on post-contrast FLAIR after experimental stroke. J. Cerebral Blood Flow Metabolism 30, 1188–1199. doi: 10.1038/jcbfm.2010.1
Battistini, L., Piccio, L., Rossi, B., Bach, S., Galgani, S., and Gasperini, C. (2003). CD8 + T cells from patients with acute multiple sclerosis display selective increase of adhesiveness in brain venules: A critical role for P-selectin glycoprotein ligand-1. Blood 101, 4775–4782. doi: 10.1182/BLOOD-2002-10-3309
Bechmann, I., Galea, I., and Perry, V. H. (2007). What is the blood-brain barrier (not)? Trends Immunol. 28, 5–11. doi: 10.1016/j.it.2006.11.007
Bergen, A. A., Kaing, S., ten Brink, J. B., Gorgels, T. G., and Janssen, S. F. (2015). Gene expression and functional annotation of human choroid plexus epithelium failure in Alzheimer’s disease. BMC Genomics 16:956. doi: 10.1186/s12864-015-2159-z
Bonney, S., Seitz, S., Ryan, C. A., Jones, K. L., Clarke, P., Tyler, K. L., et al. (2019). Gamma interferon alters junctional integrity via rho kinase, resulting in blood-brain barrier leakage in experimental viral encephalitis. mBio 10:e01675-19. doi: 10.1128/MBIO.01675-19
Breckwoldt, M. O., Chen, J. W., Stangenberg, L., Aikawa, E., Rodriguez, E., Qiu, S., et al. (2008). Tracking the inflammatory response in stroke in vivo by sensing the enzyme myeloperoxidase. Proc. Natl. Acad. Sci. U.S.A. 105, 18584–18589. doi: 10.1073/pnas.0803945105
Brioschi, S., Wang, W. L., Peng, V., Wang, M., Shchukina, I., and Greenberg, Z. J. (2021). Heterogeneity of meningeal B cells reveals a lymphopoietic niche at the CNS borders. Science 373:eabf9277. doi: 10.1126/science.abf9277
Brøchner, C. B., Holst, C. B., and Møllgård, K. (2015). Outer brain barriers in rat and human development. Front. Neurosci. 9:75. doi: 10.3389/fnins.2015.00075
Brombacher, T. M., Ajonijebu, D. C., Scibiorek, M., Berkiks, I., Moses, B. O., Mpotje, T., et al. (2021). IL-4Rα deletion disrupts psychomotor performance and reference memory in mice while sparing behavioural phenotype associated with spatial learning. Brain Behav. Immunity 92, 157–164. doi: 10.1016/J.BBI.2020.12.003
Capaldo, C. T., and Nusrat, A. (2009). Cytokine regulation of tight junctions. Biochim. et Biophysica Acta - Biomembranes 1788, 864–871. doi: 10.1016/j.bbamem.2008.08.027
Carrano, A., Hoozemans, J. J. M., van der Vies, S. M., van Horssen, J., de Vries, H. E., and Rozemuller, A. J. M. (2012). Neuroinflammation and blood-brain barrier changes in capillary amyloid angiopathy. Neurodegener. Dis. 10, 329–331. doi: 10.1159/000334916
Cassella, J. P., Lawrenson, J. G., and Firth, J. A. (1997). Development of endothelial paracellular clefts and their tight junctions in the pial microvessels of the rat. J. Neurocytol. 26, 567–575. doi: 10.1023/a:1015438624949
Chen, J. W., Breckwoldt, M. O., Aikawa, E., Chiang, G., and Weissleder, R. (2008). Myeloperoxidase-targeted imaging of active inflammatory lesions in murine experimental autoimmune encephalomyelitis. Brain 131, 1123–1133. doi: 10.1093/brain/awn004
Cheng, D., Talib, J., Stanley, C. P., Rashid, I., Michaëlsson, E., and Lindstedt, E. L. (2019). Inhibition of MPO (myeloperoxidase). attenuates endothelial dysfunction in mouse models of vascular inflammation and atherosclerosis. Arterioscler. Thromb. Vasc. Biol. 39, 1448–1457. doi: 10.1161/ATVBAHA.119.312725
Cheung, A. K., Parker, C. J., and Hohnholt, M. (1993). Beta2 integrins are required for neutrophil degranulation induced by hemodialysis membranes. Kidney Int. 43, 649–660. doi: 10.1038/ki.1993.94
Chou, W.-H., Wang, G., Kumar, V., and Weng, Y.-C. (2015). Lipocalin-2 in Stroke. Neuro Open J. 2, 38–41. doi: 10.17140/NOJ-2-109
Chow, B. W., and Gu, C. (2015). The molecular constituents of the blood-brain barrier. Trends Neurosci. 38, 598–608. doi: 10.1016/J.TINS.2015.08.003
Christy, A. L., Walker, M. E., Hessner, M. J., and Brown, M. A. (2013). Mast cell activation and neutrophil recruitment promotes early and robust inflammation in the meninges in EAE. J. Autoimmun. 42, 50–61. doi: 10.1016/j.jaut.2012.11.003
Clark, A. W., Krekoski, C. A., Bou, S. S., Chapman, K. R., and Edwards, D. R. (1997). Increased gelatinase A (MMP-2). and gelatinase B (MMP-9). activities in human brain after focal ischemia. Neurosci. Lett. 238, 53–56. doi: 10.1016/S0304-3940(97)00859-8
Combarros, O., Infante, J., Llorca, J., Pea, N., Fernández-Viadero, C., and Berciano, J. (2002). The myeloperoxidase gene in Alzheimer’s disease: A case-control study and meta-analysis. Neurosci. Lett. 326, 33–36. doi: 10.1016/S0304-3940(02)00303-8
Coulibaly, A. P., Pezuk, P., Varghese, P., Gartman, W., Triebwasser, D., and Kulas, J. A. (2021). Neutrophil enzyme myeloperoxidase modulates neuronal response in a model of subarachnoid hemorrhage by venous injury. Stroke 52, 3374–3384. doi: 10.1161/STROKEAHA.120.033513
Crawford, F. C., Freeman, M. J., Schinka, J. A., Morris, M. D., Abdullah, L. I., and Richards, D. (2001). Association between Alzheimer’s disease and a functional polymorphism in the myeloperoxidase gene. Exp. Neurol. 167, 456–459. doi: 10.1006/exnr.2000.7560
Cruz Hernández, J. C., Bracko, O., Kersbergen, C. J., Muse, V., Haft-Javaherian, M., and Berg, M. (2019). Neutrophil adhesion in brain capillaries reduces cortical blood flow and impairs memory function in Alzheimer’s disease mouse models. Nat. Neurosci. 22, 413–420. doi: 10.1038/s41593-018-0329-4
Cugurra, A., Mamuladze, T., Rustenhoven, J., Dykstra, T., Beroshvili, G., and Greenberg, Z. J. (2021). Skull and vertebral bone marrow are myeloid cell reservoirs for the meninges and CNS parenchyma. Science 373:eabf7844. doi: 10.1126/science.abf7844
Cummins, M. (2012). Occludin: One Protein. Many Forms, Mol. Cell. Biol. 32, 242–250. doi: 10.1128/mcb.06029-11
Dekens, D. W., Naudé, J. W., Engelborghs, S., Vermeiren, Y., van Dam, D., and Oude Voshaar, R. C. (2017). Neutrophil gelatinase-associated lipocalin and its receptors in Alzheimer’s Disease (AD). brain regions: Differential findings in AD with and without depression. J. Alzheimer’s Dis. 55, 763–776. doi: 10.3233/JAD-160330
Derecki, N. C., Cardani, A. N., Yang, C. H., Quinnies, K. M., Crihfield, A., Lynch, K. R., et al. (2010). Regulation of learning and memory by meningeal immunity: A key role for IL-4. J. Exp. Med. 207, 1067–1080. doi: 10.1084/jem.20091419
Derk, J., Jones, H. E., Como, C., Pawlikowski, B., and Siegenthaler, J. A. (2021). Living on the edge of the cns: Meninges cell diversity in health and disease. Front. Cell. Neurosci. 15:703944. doi: 10.3389/fncel.2021.703944
DiStasi, M. R., and Ley, K. (2009). Opening the flood-gates: How neutrophil-endothelial interactions regulate permeability. Trends Immunol 30, 547–556. doi: 10.1016/j.it.2009.07.012
Du, Y., Li, W., Lin, L., Lo, E. H., and Xing, C. (2019). Effects of lipocalin-2 on brain endothelial adhesion and permeability. PLoS One 14:e0218965. doi: 10.1371/journal.pone.0218965
Dubois, B., Masure, S., Hurtenbach, U., Paemen, L., Heremans, H., and van den Oord, J. (1999). Resistance of young gelatinase B-deficient mice to experimental autoimmune encephalomyelitis and necrotizing tail lesions. J. Clin. Inves. 104, 1507–1515. doi: 10.1172/JCI6886
Dusi, S., Angiari, S., Pietronigro, E. C., Lopez, N., Angelini, G., and Zenaro, E. (2019). LFA-1 controls Th1 and Th17 motility behavior in the inflamed central nervous system. Front. Immunol. 10:2436. doi: 10.3389/FIMMU.2019.02436/BIBTEX
El Amki, M., Glück, C., Binder, N., Middleham, W., Wyss, M. T., and Weiss, T. (2020). Neutrophils Obstructing Brain Capillaries Are a Major Cause of No-Reflow in Ischemic Stroke. Cell Rep. 33:108260. doi: 10.1016/J.CELREP.2020.108260
Engelhardt, B., and Ransohoff, R. M. (2005). The ins and outs of T-lymphocyte trafficking to the CNS: Anatomical sites and molecular mechanisms. Trends Immunol. 26, 485–495. doi: 10.1016/j.it.2005.07.004
Engelhardt, B., Vajkoczy, P., and Weller, R. O. (2017). The movers and shapers in immune privilege of the CNS. Nat. Immunol. 18, 123–131. doi: 10.1038/ni.3666
Fabene, F., Mora, G. N., Martinello, M., Rossi, B., Merigo, F., and Ottoboni, L. (2008). A role for leukocyte-endothelial adhesion mechanisms in epilepsy. Nat. Med. 14, 1377–1383. doi: 10.1038/NM.1878
Felgenhauer, K. (1995). “The Filtration Concept of the Blood-CSF-Barrier as Basis for the Differentiation of CSF Proteins,” in New Concepts of a Blood—Brain Barrier**, eds J. Greenwood, D. J. Begley, and M. B. Segal (Boston, MA: Springer), doi: 10.1007/978-1-4899-1054-7_21
Filiano, A. J., Xu, Y., Tustison, N. J., Marsh, R. L., Baker, W., and Smirnov, I. (2016). Unexpected role of interferon-γ in regulating neuronal connectivity and social behaviour. Nature 535, 425–429. doi: 10.1038/NATURE18626
Filippi, M., Bar-Or, A., Piehl, F., Preziosa, P., Solari, A., Vukusic, S., et al. (2018). Multiple sclerosis. Nat. Rev. Dis. Primers 4:43. doi: 10.1038/s41572-018-0041-4
Fischer, A., Wannemacher, J., Christ, S., Koopmans, T., Kadri, S., and Zhao, J. (2022). Neutrophils direct preexisting matrix to initiate repair in damaged tissues. Nat. Immunol. 23, 518–531. doi: 10.1038/s41590-022-01166-6
Forghani, R., Kim, H. J., Wojtkiewicz, G. R., Bure, L., Wu, Y., and Hayase, M. (2015). Myeloperoxidase propagates damage and is a potential therapeutic target for subacute stroke. J. Perinatol. 35, 485–493. doi: 10.1038/jcbfm.2014.222
Forghani, R., Wojtkiewicz, G. R., Zhang, Y., Seeburg, D., Bautz, B. R. M., and Pulli, B. (2012). Demyelinating diseases: Myeloperoxidase as an imaging biomarker and therapeutic target. Radiol. 263, 451–460. doi: 10.1148/RADIOL.12111593
Galea, I., Bechmann, I., and Perry, V. H. (2007). What is immune privilege (not)? Trends Immunol. 28, 12–18. doi: 10.1016/j.it.2006.11.004
Garratt, L. W., Sutanto, E. N., Ling, K. M., Looi, K., Iosifidis, T., and Martinovich, K. M. (2015). Matrix metalloproteinase activation by free neutrophil elastase contributes to bronchiectasis progression in early cystic fibrosis. Eur. Respiratory J. 46, 384–394. doi: 10.1183/09031936.00212114
Gellhaar, S., Sunnemark, D., Eriksson, H., Olson, L., and Galter, D. (2017). Myeloperoxidase-immunoreactive cells are significantly increased in brain areas affected by neurodegeneration in Parkinson’s and Alzheimer’s disease. Cell Tissue Res. 369, 445–454. doi: 10.1007/s00441-017-2626-8
Gelzo, M., Cacciapuoti, S., Pinchera, B., de Rosa, A., Cernera, G., and Scialò, F. (2022). Matrix metalloproteinases (MMP). 3 and 9 as biomarkers of severity in COVID-19 patients. Sci. Rep. 12:1212. doi: 10.1038/s41598-021-04677-8
Gião, T., Teixeira, T., Almeida, M. R., and Cardoso, I. (2022). Choroid Plexus in Alzheimer’s Disease—The Current State of Knowledge. Biomedicines 10:224. doi: 10.3390/biomedicines10020224
Gidday, J. M., Gasche, Y. G., Copin, J.-C., Shah, A. R., Perez, R. S., Shapiro, S. D., et al. (2005). Leukocyte-derived matrix metalloproteinase-9 mediates blood-brain barrier breakdown and is proinflammatory after transient focal cerebral ischemia. Am. J. Physiol. Heart Circulatory Physiol. 289, 558–568. doi: 10.1152/ajpheart.01275.2004.-Results
Gorina, R., Lyck, R., Vestweber, D., and Engelhardt, B. (2014). β 2 Integrin–mediated crawling on endothelial ICAM-1 and ICAM-2 Is a prerequisite for transcellular neutrophil diapedesis across the inflamed blood–brain barrier. J. Immunol. 192, 324–337. doi: 10.4049/jimmunol.1300858
Greene, C., Hanley, N., and Campbell, M. (2019). Claudin-5: Gatekeeper of neurological function. Fluids Barriers of the CNS 16:3. doi: 10.1186/s12987-019-0123-z
Halade, G. V., Jin, Y. F., and Lindsey, M. L. (2013). Matrix metalloproteinase (MMP)-9: A proximal biomarker for cardiac remodeling and a distal biomarker for inflammation. Pharmacol. Ther. 139, 32–40. doi: 10.1016/j.pharmthera.2013.03.009
Hallenbeck, J. M., Dutka, A. J., Kochanek, M., Kumaroo, K. K., Thompson, C. B., and Obrenovitch, T. P. (1986). Polymorphonuclear leukocyte accumulation in brain regions with low blood flow during the early postischemic period. Stroke 17, 246–253. doi: 10.1161/01.str.17.2.246
Harada, K., Suzuki, Y., Yamakawa, K., Kawakami, J., and Umemura, K. (2012). Combination of reactive oxygen species and tissue-type plasminogen activator enhances the induction of gelatinase B in brain endothelial cells. Int. J. Neurosci. 122, 53–59. doi: 10.3109/00207454.2011.623808
Hauptmann, J., Johann, L., Marini, F., Kitic, M., Colombo, E., and Mufazalov, I. A. (2020). Interleukin-1 promotes autoimmune neuroinflammation by suppressing endothelial heme oxygenase-1 at the blood–brain barrier. Acta Neuropathol. 140, 549–567. doi: 10.1007/s00401-020-02187-x
Herisson, F., Frodermann, V., Courties, G., Rohde, D., Sun, Y., and Vandoorne, K. (2018). Direct vascular channels connect skull bone marrow and the brain surface enabling myeloid cell migration. Nat. Neurosci. 21, 1209–1217. doi: 10.1038/S41593-018-0213-2
Herz, J., Fu, Z., Kim, K., Dykstra, T., Wall, M., and Li, H. (2021). GABAergic neuronal IL-4R mediates T cell effect on memory. Neuron 109, 3609–3618.e9. doi: 10.1016/J.NEURON.2021.10.022
Ineichen, B. V., Tsagkas, C., Absinta, M., and Reich, D. S. (2022). Leptomeningeal enhancement in multiple sclerosis and other neurological diseases: A systematic review and Meta-Analysis. NeuroImage 33:102939. doi: 10.1016/j.nicl.2022.102939
Jiao, H., Wang, Z., Liu, Y., Wang, P., and Xue, Y. (2011). Specific role of tight junction proteins claudin-5, occludin, and ZO-1 of the blood-brain barrier in a focal cerebral ischemic insult. J. Mol. Neurosci. 44, 130–139. doi: 10.1007/S12031-011-9496-4
Johnston, B., and Kubes. (1999). The α4-integrin: An alternative pathway for neutrophil recruitment? Immunol. Today 20, 545–550. doi: 10.1016/S0167-5699(99)01544-3
Jordão, M. J. C., Sankowski, R., Brendecke, S. M., Sagar, L. G., Tai, Y. H., and Tay, T. L. (2019). Neuroimmunology: Single-cell profiling identifies myeloid cell subsets with distinct fates during neuroinflammation. Science 363:eaat7554. doi: 10.1126/science.aat7554
Juul, S., Pliskin, J. S., and Fineberg, H. V. (1984). Variation and information in white blood cell differential counts. Med. Dec. Making 4, 69–80. doi: 10.1177/0272989
Kahles, T., Luedike, P., Endres, M., Galla, H. J., Steinmetz, H., and Busse, R. (2007). NADPH oxidase plays a central role in blood-brain barrier damage in experimental stroke. Stroke 38, 3000–3006. doi: 10.1161/STROKEAHA.107.489765
Kang, L., Yu, H., Yang, X., Zhu, Y., Bai, X., and Wang, R. (2020). Neutrophil extracellular traps released by neutrophils impair revascularization and vascular remodeling after stroke. Nat. Commun. 11:2488. doi: 10.1038/s41467-020-16191-y
Kant, S., Stopa, E. G., Johanson, C. E., Baird, A., and Silverberg, G. D. (2018). Choroid plexus genes for CSF production and brain homeostasis are altered in Alzheimer’s disease. Fluids Barriers CNS 15:34. doi: 10.1186/s12987-018-0120-7
Kataoka, H., Kim, S. W., and Plesnila, N. (2004). Leukocyte-endothelium interactions during permanent focal cerebral ischemia in mice. J. Cerebral Blood Flow Mmetabolism 24, 668–676. doi: 10.1097/01.WCB.0000117812.35136.5B
Kerfoot, S. M., and Kubes. (2002). Overlapping roles of P-Selectin and α 4 integrin to recruit leukocytes to the central nervous system in experimental autoimmune encephalomyelitis. J. Immunol. 169, 1000–1006. doi: 10.4049/jimmunol.169.2.1000
Kieseier, B. C., Kiefer, R., Clements, J. M., Miller, K., Wells, G. M., Schweitzer, T., et al. (1998). Matrix metalloproteinase-9 and-7 are regulated in experimental autoimmune encephalomyelitis. Brain 121, 159–166. doi: 10.1093/brain/121.1.159
Kim, H. J., Wei, Y., Lee, J. Y., Wu, Y., Zheng, Y., Moskowitz, M. A., et al. (2016). Myeloperoxidase inhibition increases neurogenesis after ischemic stroke. J. Pharmacol. Exp. Ther. 359, 262–272. doi: 10.1124/jpet.116.235127
Kim, H. J., Wei, Y., Wojtkiewicz, G. R., Lee, J. Y., Moskowitz, M. A., and Chen, J. W. (2019). Reducing myeloperoxidase activity decreases inflammation and increases cellular protection in ischemic stroke. J. Cerebral Blood Flow Metabolism 39, 1864–1877. doi: 10.1177/0271678
Kim, J. H., Ko, W., Lee, H. W., Jeong, J. Y., Lee, M. G., and Kim, J. H. (2017). Astrocyte-derived lipocalin-2 mediates hippocampal damage and cognitive deficits in experimental models of vascular dementia. Glia 65, 1471–1490. doi: 10.1002/glia.23174
Kim, S. W., Lee, H., Lee, H. K., Kim, I. D., and Lee, J. K. (2019). Neutrophil extracellular trap induced by HMGB1 exacerbates damages in the ischemic brain. Acta Neuropathol. Commun. 7:94. doi: 10.1186/s40478-019-0747-x
Kolaczkowska, E., and Kubes. (2013). Neutrophil recruitment and function in health and inflammation. Nat. Rev. Immunol. 13, 159–175. doi: 10.1038/nri3399
Kooij, G., Kopplin, K., Blasig, R., Stuiver, M., Koning, N., and Goverse, G. (2014). Disturbed function of the blood-cerebrospinal fluid barrier aggravates neuro-inflammation. Acta Neuropathol. 128, 267–277. doi: 10.1007/s00401-013-1227-1
Kratzer, I., Vasiljevic, A., Rey, C., Fevre-Montange, M., Saunders, N., Strazielle, N., et al. (2012). Complexity and developmental changes in the expression pattern of claudins at the blood-CSF barrier. Histochem. Cell Biol. 138, 861–879. doi: 10.1007/s00418-012-1001-9
Krzyzanowska, A., and Carro, E. (2012). Pathological alteration in the choroid plexus of Alzheimer’s disease: Implication for new therapy aroaches. Front. Pharmacol. 3:75. doi: 10.3389/fphar.2012.00075
Kunis, G., Baruch, K., Rosenzweig, N., Kertser, A., Miller, O., Berkutzki, T., et al. (2013). IFN-γ-dependent activation of the brain’s choroid plexus for CNS immune surveillance and repair. Brain 136, 3427–3440. doi: 10.1093/brain/awt259
Kurihara, T., Shimizu-Hirota, R., Shimoda, M., Adachi, T., Shimizu, H., and Weiss, S. J. (2012). Neutrophil-Derived Matrix Metalloproteinase 9 Triggers Acute Aortic Dissection. Circulation. 26, 3070–3080. doi: 10.1161/CIRCULATIONAHA.112.097097
Labus, J., Häckel, S., Lucka, L., and Danker, K. (2014). Interleukin-1β induces an inflammatory response and the breakdown of the endothelial cell layer in an improved human THBMEC-based in vitro blood-brain barrier model. J. Neurosci. Methods 228, 35–45. doi: 10.1016/J.JNEUMETH.2014.03.002
Lee, H. S., Namkoong, K., Kim, D. H., Kim, K. J., Cheong, Y. H., Kim, S. S., et al. (2004). Hydrogen peroxide-induced alterations of tight junction proteins in bovine brain microvascular endothelial cells. Microvasc. Res. 68, 231–238. doi: 10.1016/J.MVR.2004.07.005
Ley, K., Laudanna, C., Cybulsky, M. I., and Nourshargh, S. (2007). Getting to the site of inflammation: The leukocyte adhesion cascade updated. Nat. Rev. Immunol. 7, 678–689. doi: 10.1038/NRI2156
Li, W., Chen, Z., Chin, I., Chen, Z., and Dai, H. (2018). The Role of VE-cadherin in Blood-brain Barrier Integrity Under Central Nervous System Pathological Conditions Current Neuropharmacology. 16, 1375–1384. doi: 10.2174/1570159
Li, Y.-J., Wang, Z.-H., Zhang, B., Zhe, X., Wang, M.-J., and Shi, S.-T. (2013). Disruption of the blood-brain barrier after generalized tonic-clonic seizures correlates with cerebrospinal fluid MMP-9 levels. J. Neuroinflammation 10:80. doi: 10.1186/1742-2094-10-80
Liebner, S., Dijkhuizen, R. M., Reiss, Y., Plate, K. H., Agalliu, D., and Constantin, G. (2018). Functional morphology of the blood–brain barrier in health and disease. Acta Neuropathol. 135, 311–336. doi: 10.1007/s00401-018-1815-1
Llorens, F., Hermann, P., Villar-Piqué, A., Diaz-Lucena, D., Nägga, K., and Hansson, O. (2020). Cerebrospinal fluid lipocalin 2 as a novel biomarker for the differential diagnosis of vascular dementia. Nat. Commun. 11:619. doi: 10.1038/s41467-020-14373-2
Lodygin, D., Hermann, M., Schweingruber, N., Flügel-Koch, C., Watanabe, T., and Schlosser, C. (2019). β-Synuclein-reactive T cells induce autoimmune CNS grey matter degeneration. Nature 566, 503–508. doi: 10.1038/s41586-019-0964-2
Lou, O., Alcaide, P., Luscinskas, F. W., and Muller, W. A. (2007). CD99 Is a Key Mediator of the Transendothelial Migration of Neutrophils. J. Immunol. 178, 1136–1143. doi: 10.4049/jimmunol.178.2.1136
Louveau, A., Smirnov, I., Keyes, T. J., Eccles, J. D., Rouhani, S. J., and Peske, J. D. (2015). Structural and functional features of central nervous system lymphatic vessels. Nature 523, 337–341. doi: 10.1038/NATURE14432
Lun, M. P., Monuki, E. S., and Lehtinen, M. K. (2015). Development and functions of the choroid plexus-cerebrospinal fluid system. Nat. Rev. Neurosci. 6, 445–457. doi: 10.1038/nrn3921
Malle, E., Furtmüller, P. G., Sattler, W., and Obinger, C. (2007). Myeloperoxidase: A target for new drug development? Br. J. Pharmacol. 152, 838–854. doi: 10.1038/sj.bjp.0707358
Marques, F., and Sousa, J. C. (2015). The choroid plexus is modulated by various peripheral stimuli: Implications to diseases of the central nervous system. Front. Cell. Neurosci. 9:136. doi: 10.3389/FNCEL.2015.00136/BIBTEX
Marques, F., Mesquita, S. D., Sousa, J. C., Coola, G., Gao, F., and Geschwind, D. H. (2012). Lipocalin 2 is present in the EAE brain and is modulated by natalizumab. Front. Cell. Neurosci. 6:33. doi: 10.3389/fncel.2012.00033
Marques, F., Sousa, J. C., Coola, G., Falcao, A. M., Rodrigues, A. J., and Geschwind, D. H. (2009). Kinetic profile of the transcriptome changes induced in the choroid plexus by peripheral inflammation. J. Cerebral Blood Flow Metabolism 29, 921–932. doi: 10.1038/jcbfm.2009.15
Mastorakos, P., and McGavern, D. (2019). The anatomy and immunology of vasculature in the central nervous system. Sci. Immunol. 4:eaav0492. doi: 10.1126/sciimmunol.aav0492
Matsuo, Y., Onodera, H., Shiga, Y., Nakamura, M., Ninomiya, M., Kihara, T., et al. (1994). Correlation between myeloperoxidase-quantified neutrophil accumulation and ischemic brain injury in the rat effects of neutrophil depletion. Stroke 25, 1469–1475. doi: 10.1161/01.str.25.7.1469
Meeker, R. B., Williams, K., Killebrew, D. A., and Hudson, L. C. (2012). Cell trafficking through the choroid plexus. Cell Adhesion Migration 6, 390–396. doi: 10.4161/cam.21054
Mel’nikova, N. N. (2009). Leukocyte-endothelial interactions in pial arterioles and venules on development of cerebral ischemia in rats. Neurosci. Behavioral Physiol. 39, 167–171. doi: 10.1007/S11055-009-9110-1
Mentis, A. F. A., Dardiotis, E., and Chrousos, G. P. (2021). Apolipoprotein E4 and meningeal lymphatics in Alzheimer disease: A conceptual framework. Mol. Psychiatry 26, 1075–1097. doi: 10.1038/s41380-020-0731-7
Miklossy, J., Kraftsik, R., Pillevuit, O., Lepori, D., Genton, C., and Bosman, F. T. (1998). Curly fiber and tangle-like inclusions in the ependyma and choroid Plexus—A pathogenetic relationship with the cortical alzheimer-type changes? J. Neuropathol. Exp. Neurol. 57, 1202–1212. doi: 10.1097/00005072-199812000-00012
Mold, M. J., and Exley, C. (2022). Aluminium co-localises with Biondi ring tangles in Parkinson’s disease and epilepsy. Sci. Rep. 12:1465. doi: 10.1038/s41598-022-05627-8
Mottahedin, A., Smith, P. L. P., Hagberg, H., Ek, C. J., and Mallard, C. (2017). TLR2-mediated leukocyte trafficking to the developing brain. J. Leukocyte Biol. 101, 297–305. doi: 10.1189/jlb.3a1215-568r
Moxon-Emre, I., and Schlichter, L. C. (2011). Neutrophil depletion reduces blood-brain barrier breakdown, axon injury, and inflammation after intracerebral hemorrhage. J. Neuropathol. Exp. Neurol. 70, 218–235. doi: 10.1097/NEN.0B013E31820D94A5
Mrdjen, D., Pavlovic, A., Hartmann, F. J., Schreiner, B., Utz, S. G., and Leung, B. P. (2018). High-dimensional single-cell maing of central nervous system immune cells reveals distinct myeloid subsets in health, aging, and disease. Immunity 48, 380–395.e6. doi: 10.1016/j.immuni.2018.01.011
Mun-bryce, S., Rosenberg, G. A., and Rosenberg Gelatin, G. A. (1998). Gelatinase B modulates selective opening of the blood-brain barrier during inflammation. Am. J. Physiol. 274:R1203–R1211. doi: 10.1152/ajpregu.1998.274.5.R1203
Murakami, T., Felinski, E. A., and Antonetti, D. A. (2009). Occludin phosphorylation and ubiquitination regulate tight junction trafficking and vascular endothelial growth factor-induced permeability. J. Biol. Chem. 284, 21036–21046. doi: 10.1074/jbc.M109.016766
Natale, G., Limanaqi, F., Busceti, C. L., Mastroiacovo, F., Nicoletti, F., Puglisi-Allegra, S., et al. (2021). Glymphatic System as a Gateway to Connect Neurodegeneration From Periphery to CNS. Front. Neurosci. 15:639140. doi: 10.3389/fnins.2021.639140
Nygårdas, P. T., and Hinkkanen, A. E. (2002). Up-regulation of MMP-8 and MMP-9 activity in the BALB/c mouse spinal cord correlates with the severity of experimental autoimmune encephalomyelitis. Clin. Exp. Immunol. 128, 245–254. doi: 10.1046/J.1365-2249.2002.01855.X
Oetjen, L. K., Mack, M. R., Feng, J., Whelan, T. M., Niu, H., and Guo, C. J. (2017). Sensory neurons co-opt classical immune signaling pathways to mediate chronic itch. Cell 171, 217–228.e13. doi: 10.1016/J.CELL.2017.08.006
Ong, C. W. M., Pabisiak, P. J., Brilha, S., Singh, P., Roncaroli, F., Elkington, P. T., et al. (2017). Complex regulation of neutrophil-derived MMP-9 secretion in central nervous system tuberculosis. J. Neuroinflammation 14:13. doi: 10.1186/s12974-017-0801-1
Otxoa-De-Amezaga, A., Gallizioli, M., Pedragosa, J., Justicia, C., Miró-Mur, F., and Salas-Perdomo, A. (2019). Location of neutrophils in different compartments of the damaged mouse brain after severe ischemia/reperfusion. Stroke 50, 1548–1557. doi: 10.1161/STROKEAHA.118.023837
Perez-de-Puig, I., Miró-Mur, F., Ferrer-Ferrer, M., Gelpi, E., Pedragosa, J., and Justicia, C. (2015). Neutrophil recruitment to the brain in mouse and human ischemic stroke. Acta Neuropathol. 129, 239–257. doi: 10.1007/s00401-014-1381-0
Piccio, L., Rossi, B., Colantonio, L., Grenningloh, R., Gho, A., and Ottoboni, L. (2005). Efficient recruitment of lymphocytes in inflamed brain venules requires expression of cutaneous lymphocyte antigen and fucosyltransferase-VII. J. Immunol. 174, 5805–5813. doi: 10.4049/JIMMUNOL.174.9.5805
Piccio, L., Rossi, B., Scarpini, E., Laudanna, C., Giagulli, C., Issekutz, A. C., et al. (2002). Molecular mechanisms involved in lymphocyte recruitment in inflamed brain microvessels: Critical Roles for P-Selectin Glycoprotein Ligand-1 and Heterotrimeric Gi-Linked Receptors. J. Immunol. 168, 1940–1949. doi: 10.4049/JIMMUNOL.168.4.1940
Pietronigro, E. C., della Bianca, V., Zenaro, E., and Constantin, G. (2017). NETosis in Alzheimer’s disease. Front. Immunol. 8:211. doi: 10.3389/fimmu.2017.00211
Pietronigro, E., Zenaro, E., and Constantin, G. (2016). Imaging of leukocyte trafficking in Alzheimer’s disease. Front. Immunol. 7:33. doi: 10.3389/fimmu.2016.00033
Pietronigro, E., Zenaro, E., Bianca, V., della Dusi, S., Terrabuio, E., and Iannoto, G. (2019). Blockade of α4 integrins reduces leukocyte–endothelial interactions in cerebral vessels and improves memory in a mouse model of Alzheimer’s disease. Sci. Rep. 9:12055. doi: 10.1038/s41598-019-48538-x
Pikor, N. B., Astarita, J. L., Summers-Deluca, L., Galicia, G., Qu, J., and Ward, L. A. (2015). Integration of Th17- and Lymphotoxin-derived signals initiates meningeal-resident stromal cell remodeling to propagate neuroinflammation. Immunity 43, 1160–1173. doi: 10.1016/j.immuni.2015.11.010
Pope, S. K., Kritchevsky, S. B., Ambrosone, C., Yaffe, K., Tylavsky, F., and Simonsick, E. M. (2006). Myeloperoxidase polymorphism and cognitive decline in older adults in the health, aging, and body composition study. Am. J. Epidemiol. 163, 1084–1090. doi: 10.1093/aje/kwj146
Prinz, M., and Priller, J. (2017). The role of peripheral immune cells in the CNS in steady state and disease. Nat. Neurosci. 20, 136–144. doi: 10.1038/NN.4475
Protasoni, M., Sangiorgi, S., Cividini, A., Culuvaris, G. T., Tomei, G., Dell’Orbo, C., et al. (2011). The collagenic architecture of human dura mater: Laboratory investigation. J. Neurosurgery 114, 1723–1730. doi: 10.3171/2010.12.JNS101732
Pulli, B., Bure, L., Wojtkiewicz, G. R., Iwamoto, Y., Ali, M., Li, D., et al. (2015). Multiple sclerosis: Myeloperoxidase immunoradiology improves detection of acute and chronic disease in experimental model. Radiology 275, 480–489. doi: 10.1148/radiol.14141495
Py, N. A., Bonnet, A. E., Bernard, A., Marchalant, Y., Charrat, E., Checler, F., et al. (2014). Differential spatio-temporal regulation of MMPs in the 5xFAD mouse model of Alzheimer’s disease: Evidence for a pro-amyloidogenic role of MT1-MMP. Front. Aging Neurosci. 6:247. doi: 10.3389/fnagi.2014.00247
Quirico-Santos, T., Mello, N. A., Gomes, C. A., de Carvalho, L. P., de Souza, J. M., and Alves-Leon, S. (2013). Increased metalloprotease activity in the epileptogenic lesion - Lobectomy reduces metalloprotease activity and urokinase-type uPAR circulating levels. Brain Res. 1538, 172–181. doi: 10.1016/j.brainres.2013.09.044
Radjavi, A., Smirnov, I., Derecki, N., and Kipnis, J. (2014). Dynamics of the meningeal CD4 + T-cell repertoire are defined by the cervical lymph nodes and facilitate cognitive task performance in mice. Mol. Psychiatry 19, 531–533. doi: 10.1038/mp.2013.79
Rahman, A., Anwar, K. N., Malik, A. B., Rahman, K. N., and Anwar, A. B. (2000). Protein kinase C-mediates TNF-induced ICAM-1 gene transcription in endothelial cells. Am. J. physiol. 279:C906–C914. doi: 10.1152/ajpcell.2000.279.4.C906
Ransohoff, R. M., Kivisäkk, P., and Kidd, G. (2003). Three or more routes for leukocyte migration into the central nervous system. Nat. Rev. Immunol. 3, 569–581. doi: 10.1038/nri1130
Reynolds, W. F., Rhees, J., Maciejewski, D., Paladino, T., Sieburg, H., Maki, R. A., et al. (1999). Myeloperoxidase polymorphism Is associated with gender specific risk for alzheimer’s disease. Exp. Neurol. 155, 31–41. doi: 10.1006/exnr.1998.6977
Ribeiro, M., Brigas, H. C., Temido-Ferreira, M., Pousinha, P. A., Regen, T., and Santa, C. (2019). Meningeal γδ T cell-derived IL-17 controls synaptic plasticity and short-term memory. Sci. Immunol. 4:eaay5199. doi: 10.1126/sciimmunol.aay5199
Richter, J., Ng-Sikorskit, J., Olsson, I., and Anderssont, T. (1990). Tumor necrosis factor-induced degranulation in adherent human neutrophils is dependent on CD1lb/CD18-integrin-triggered oscillations of cytosolic free Ca2 +. Proc. Natl. Acad. Sci. U.S. A. 87, 9472–9476. doi: 10.1073/pnas.87.23.9472
Rodríguez-Lorenzo, S., Konings, J., van der Pol, S., Kamermans, A., Amor, S., and van Horssen, J. (2020). Inflammation of the choroid plexus in progressive multiple sclerosis: Accumulation of granulocytes and T cells. Acta Neuropathol. Commun. 8:9. doi: 10.1186/s40478-020-0885-1
Rojo, A. I., McBean, G., Cindric, M., Egea, J., López, M. G., Rada, P., et al. (2014). Redox control of microglial function: Molecular mechanisms and functional significance. Antioxidants Redox Signaling 21, 1766–1801. doi: 10.1089/ars.2013.5745
Romanic, A. M., White, R. F., Arleth, A. J., Ohlstein, E. H., and Barone, F. C. (1998). Matrix metalloproteinase expression increases after cerebral focal ischemia in rats inhibition of matrix metalloproteinase-9 reduces infarct size. Stroke 29, 1020–1030. doi: 10.1161/01.str.29.5.1020
Rosell, A., Cuadrado, E., Ortega-Aznar, A., Hernández-Guillamon, M., Lo, E. H., and Montaner, J. (2008). MMP-9-positive neutrophil infiltration is associated to blood-brain barrier breakdown and basal lamina type IV collagen degradation during hemorrhagic transformation after human ischemic stroke. Stroke 39, 1121–1126. doi: 10.1161/STROKEAHA.107.500868
Rossi, B., Angiari, S., Zenaro, E., Budui, S. L., and Constantin, G. (2011). Vascular inflammation in central nervous system diseases: Adhesion receptors controlling leukocyte-endothelial interactions. J. Leukocyte Biol. 89, 539–556. doi: 10.1189/jlb.0710432
Rossi, B., Constantin, G., and Zenaro, E. (2020). The emerging role of neutrophils in neurodegeneration. Immunobiology 225:151865. doi: 10.1016/j.imbio.2019.10.014
Rossi, B., Santos-Lima, B., Terrabuio, E., Zenaro, E., and Constantin, G. (2021). Common peripheral immunity mechanisms in multiple sclerosis and Alzheimer’s disease. Front. Immunol. 12:639369. doi: 10.3389/fimmu.2021.639369
Rua, R., and McGavern, D. B. (2018). Advances in Meningeal Immunity. Trends Mol. Med. 24, 542–559. doi: 10.1016/j.molmed.2018.04.003
Russi, A. E., and Brown, M. A. (2015). The meninges: New therapeutic targets for multiple sclerosis. Trans. Res. 165, 255–269. doi: 10.1016/j.trsl.2014.08.005
Rustenhoven, J., Drieu, A., Mamuladze, T., de Lima, K. A., Dykstra, T., and Wall, M. (2021). Functional characterization of the dural sinuses as a neuroimmune interface. Cell 184, 1000–1016.e27. doi: 10.1016/j.cell.2020.12.040
Rybakowski, J. K., Skibinska, M., Kapelski, P., Kaczmarek, L., and Hauser, J. (2009a). Functional polymorphism of the matrix metalloproteinase-9 (MMP-9). gene in schizophrenia. Schizophrenia Res. 109, 90–93. doi: 10.1016/j.schres.2009.02.005
Rybakowski, J. K., Skibinska, M., Leszczynska-Rodziewicz, A., Kaczmarek, L., and Hauser, J. (2009b). Matrix metalloproteinase-9 gene and bipolar mood disorder. Neuromolec. Med. 11, 128–132. doi: 10.1007/S12017-009-8072-3
Saboori, P., and Sadegh, A. (2015). Histology and morphology of the brain subarachnoid trabeculae. Anatomy Res. Int. 2015:279814. doi: 10.1155/2015/279814
Saint-Pol, J., Gosselet, F., Duban-Deweer, S., Pottiez, G., and Karamanos, Y. (2020). Targeting and Crossing the Blood-Brain Barrier with Extracellular Vesicles. Cells 9:851. doi: 10.3390/CELLS9040851
Sajad, M., Zargan, J., Chawla, R., Umar, S., Sadaqat, M., and Khan, H. A. (2009). Hiocampal neurodegeneration in experimental autoimmune encephalomyelitis (EAE): Potential role of inflammation activated myeloperoxidase. Mol. Cell. Biochem. 328, 183–188. doi: 10.1007/s11010-009-0088-3
Saul, J., Saul, J., Hutchins, E., Reiman, R., Saul, M., Ostrow, L. W., et al. (2020). Global alterations to the choroid plexus blood-CSF barrier in amyotrophic lateral sclerosis. Acta Neuropathol. Commun. 8:92. doi: 10.1186/s40478-020-00968-9
Schläger, C., Körner, H., Krueger, M., Vidoli, S., Haberl, M., and Mielke, D. (2016). Effector T-cell trafficking between the leptomeninges and the cerebrospinal fluid. Nature 530, 349–353. doi: 10.1038/nature16939
Schmitt, C., Strazielle, N., and Ghersi-Egea, J.-F. (2012). Brain leukocyte infiltration initiated by peripheral inflammation or experimental autoimmune encephalomyelitis occurs through pathways connected to the CSF-filled compartments of the forebrain and midbrain. J. Neuroinflammation 9:187. doi: 10.1186/1742-2094-9-187
Schwartz, M., and Baruch, K. (2014). The resolution of neuroinflammation in neurodegeneration: Leukocyte recruitment via the choroid plexus. EMBO J. 33, 7–22. doi: 10.1002/embj.201386609
Serot, J. M., Béné, M. C., Foliguet, B., and Faure, G. C. (2000). Morphological alterations of the choroid plexus in late-onset Alzheimer’s disease. Acta Neuropathol. 99, 105–108. doi: 10.1007/PL00007412
Siegelman, M. H., Stanescu, D., and Estess, P. (2000). The CD44-initiated pathway of T-cell extravasation uses VLA-4 but not LFA-1 for firm adhesion. J. Clin. Inves. 105, 683–691. doi: 10.1172/JCI8692
Sienel, R. I., Kataoka, H., Kim, S. W., Seker, F. B., and Plesnila, N. (2022). Adhesion of leukocytes to cerebral venules precedes neuronal cell death and is sufficient to trigger tissue damage after cerebral ischemia. Front. Neurol. 12:2544. doi: 10.3389/FNEUR.2021.807658/BIBTEX
Silva, B. A., and Ferrari, C. C. (2019). Cortical and meningeal pathology in progressive multiple sclerosis: A new therapeutic target? Rev. Neurosci. 30, 221–232. doi: 10.1515/revneuro-2018-0017
Silverberg, G. D., Heit, G., Huhn, S., Jaffe, R. A., Chang, S. D., Bronte-Stewart, H., et al. (2001). The cerebrospinal fluid production rate is reduced in dementia of the Alzheimer’s type. Neurology 57, 1763–1766. doi: 10.1212/WNL.57.10.1763
Smolders, J., Heutinck, K. M., Fransen, N. L., Remmerswaal, E. B. M., Hombrink, P., and ten Berge, I. J. M. (2018). Tissue-resident memory T cells populate the human brain. Nat. Commun. 9:4593. doi: 10.1038/s41467-018-07053-9
Smyth, L. C. D., Murray, H. C., Hill, M., van Leeuwen, E., Highet, B., and Magon, N. J. (2022). Neutrophil-vascular interactions drive myeloperoxidase accumulation in the brain in Alzheimer’s disease. Acta Neuropathol. Commun. 10:38. doi: 10.1186/s40478-022-01347-2
Solé, N., Petegnief, V, Gorina, R, Chamorro, A, Planas, AM. (2004). Activation of matrix metalloproteinase-3 and agrin cleavage in cerebral ischemia/reperfusion. J. Neuropathol. Exp. Neurol. 63, 338–349. doi: 10.1093/jnen/63.4.338
Steffen, B. J., Breier, G., Butcher, E. C., Schulz, M., and Engelhardtt, B. (1996). ICAM-1, VCAM-1, and MAdCAM-1 are expressed on choroid plexus epithelium but not endothelium and mediate binding of lymphocytes in vitro. Am. J. Pathol. 148, 1819–1838.
Steffen, B. J., Butcher, E. C., and Engelhardt, B. (1994). Evidence for Involvement of IAM-1 and VCAM-1 in Lymphocyte Interaction with Endothelium in Experimental Autoimmune Encephalomyelitis in the Central Nervous System in the SJL/J Mouse. Am. J. Pathol. 145, 189–201.
Steinemann, A., Galm, I., Chip, S., Nitsch, C., and Maly, I. P. (2016). Claudin-1, -2 and -3 are selectively expressed in the epithelia of the choroid plexus of the mouse from early development and into adulthood while claudin-5 is restricted to endothelial cells. Front. Neuroanatomy 10:16. doi: 10.3389/fnana.2016.00016
Storkebaum, E., and Carmeliet, P. (2004). VEGF: A critical player in neurodegeneration. J. Clin. Inves. 113, 14–18. doi: 10.1172/JCI20682
Strominger, I., Elyahu, Y., Berner, O., Reckhow, J., Mittal, K., Nemirovsky, A., et al. (2018). The choroid plexus functions as a niche for T-cell stimulation within the central nervous system. Front. Immunol. 9:1066. doi: 10.3389/fimmu.2018.01066
Sullivan, D. P., and Muller, W. A. (2014). Neutrophil and monocyte recruitment by PECAM. CD99, and other molecules via the LBRC. Sem. Immunopathol. 36, 193–209. doi: 10.1007/s00281-013-0412-6
Svedin, P., Hagberg, H., Sävman, K., Zhu, C., and Mallard, C. (2007). Matrix metalloproteinase-9 gene knock-out protects the immature brain after cerebral hypoxia–ischemia. J. Neurosci. 27:1511. doi: 10.1523/JNEUROSCI.4391-06.2007
Sweeney, M. D., Zhao, Z., Montagne, A., Nelson, A. R., and Zlokovic, B. V. (2019). Blood-brain barrier: From physiology to disease and back. Physiol. Rev. 99, 21–78. doi: 10.1152/PHYSREV.00050.2017
Szmydynger-Chodobska, J., Shan, R., Thomasian, N., and Chodobski, A. (2016). The involvement of pial microvessels in leukocyte invasion after mild traumatic brain injury. PLoS One 11:e0167677. doi: 10.1371/journal.pone.0167677
Szmydynger-Chodobska, J., Strazielle, N., Gandy, J. R., Keefe, T. H., Zink, B. J., Ghersi-Egea, J. F., et al. (2012). Posttraumatic invasion of monocytes across the blood-cerebrospinal fluid barrier. J. Cerebral Blood Flow Metabolism 32, 93–104. doi: 10.1038/jcbfm.2011.111
Szmydynger-Chodobska, J., Strazielle, N., Zink, B. J., Ghersi-Egea, J. F., and Chodobski, A. (2009). The role of the choroid plexus in neutrophil invasion after traumatic brain injury. J. Cerebral Blood Metabolism 29, 1503–1516. doi: 10.1038/jcbfm.2009.71
Tadayon, E., Pascual-Leone, A., Press, D., and Santarnecchi, E. (2020). Choroid plexus volume is associated with levels of CSF proteins: Relevance for Alzheimer’s and Parkinson’s disease. Neurobiol. Aging 89, 108–117. doi: 10.1016/j.neurobiolaging.2020.01.005
Takács, E., Nyilas, R., Szepesi, Z., Baracskay, P., Karlsen, B., and Røsvold, T. (2010). Matrix metalloproteinase-9 activity increased by two different types of epileptic seizures that do not induce neuronal death: A possible role in homeostatic synaptic plasticity. Neurochem. Int. 56, 799–809. doi: 10.1016/j.neuint.2010.03.003
Tietz, S., and Engelhardt, B. (2015). Brain barriers: Crosstalk between complex tight junctions and adherens junctions. J. Cell Biol. 209, 493–506. doi: 10.1083/jcb.201412147
Tschesche, H., Zo, V., Lzer, È, Triebel, S., and Bartsch, S. (2001). The human neutrophil lipocalin suorts the allosteric activation of matrix metalloproteinases. Eur. J. Biochem. 268, 1918–1928. doi: 10.1046/j.1432-1327.2001.02066.x
Tumani, H., Huss, A., and Bachhuber, F. (2017). The cerebrospinal fluid and barriers - anatomic and physiologic considerations. Handbook Clin. Neurol. 146, 21–32. doi: 10.1016/B978-0-12-804279-3.00002-2
Turner, R. J., and Sharp, F. R. (2016). Implications of MMP9 for blood brain barrier disruption and hemorrhagic transformation following ischemic stroke. Front. Cell. Neurosci. 10:56. doi: 10.3389/fncel.2016.00056
Uchida, Y., Sumiya, T., Tachikawa, M., Yamakawa, T., Murata, S., and Yagi, Y. (2019). Involvement of claudin-11 in disruption of blood-brain, -spinal cord, and -arachnoid barriers in multiple sclerosis. Mol. Neurobiol. 56, 2039–2056. doi: 10.1007/s12035-018-1207-5
Üllen, A., Singewald, E., Konya, V., Fauler, G., Reicher, H., and Nusshold, C. (2013). Myeloperoxidase-derived oxidants induce blood-brain barrier dysfunction in vitro and in vivo. PLoS One 8:e64034. doi: 10.1371/journal.pone.0064034
Van Hove, H., Martens, L., Scheyltjens, I., de Vlaminck, K., Pombo Antunes, A. R., and de Prijck, S. (2019). A single-cell atlas of mouse brain macrophages reveals unique transcriptional identities shaped by ontogeny and tissue environment. Nat. Neurosci. 22, 1021–1035. doi: 10.1038/s41593-019-0393-4
Van Itallie, C. M., and Anderson, J. M. (2018). Phosphorylation of tight junction transmembrane proteins: Many sites, much to do. Tissue Barriers 6:e1382671. doi: 10.1080/21688370.2017.1382671
Vercellino, M., Votta, B., Condello, C., Piacentino, C., Romagnolo, A., and Merola, A. (2008). Involvement of the choroid plexus in multiple sclerosis autoimmune inflammation: A neuropathological study. J. Neuroimmunol. 199, 133–141. doi: 10.1016/j.jneuroim.2008.04.035
Vestweber, D. (2015). How leukocytes cross the vascular endothelium. Nat. Rev. Immunol. 15, 692–704. doi: 10.1038/NRI3908
Villabona-Rueda, A., Erice, C., Pardo, C. A., and Stins, M. F. (2019). The evolving concept of the blood brain barrier (BBB): From a single static barrier to a heterogeneous and dynamic relay center. Front. Cell. Neurosci. 13:405. doi: 10.3389/FNCEL.2019.00405/BIBTEX
Vockel, M., and Vestweber, D. (2013). How T cells trigger the dissociation of the endothelial receptor phosphatase VE-PTP from VE-cadherin. Blood 122, 2512–2522. doi: 10.1182/blood-2013-04-499228
Walker-Caulfield, M. E., Hatfield, J. K., and Brown, M. A. (2015). Dynamic changes in meningeal inflammation correspond to clinical exacerbations in a murine model of relapsing-remitting multiple sclerosis. J. Neuroimmunol. 278, 112–122. doi: 10.1016/j.jneuroim.2014.12.009
Walzog, B., Seifert, R., Zakrzewicz, A., Gaehtgens, P., and Ley, K. (1994). Cross-linking of CD18 in human neutrophils induces an increase of intracellular free Ca2 +, exocytosis of azurophilic granules, quantitative up-regulation of CD18, shedding of L-selectin, and actin polymerization. J. Leukocyte Biol. 56, 625–635. doi: 10.1002/jlb.56.5.625
Wang, D., Li, S.-P., Fu, J.-S., Zhang, S., Bai, L., and Guo, L. (2016). Resveratrol defends blood-brain barrier integrity in experimental autoimmune encephalomyelitis mice. J. Neurophysiol. 116, 2173–2179. doi: 10.1152/jn.00510.2016
Wang, G., Weng, Y. C., Chiang, I. C., Huang, Y. T., Liao, Y. C., and Chen, Y. C. (2020). Neutralization of lipocalin-2 diminishes stroke-reperfusion injury. Int. J. Mol. Sci. 21, 6253. doi: 10.3390/ijms21176253
Wang, Q., and Doerschuk, C. M. (2002). The signaling pathways induced by neutrophil-endothelial cell adhesion. Antioxidants Redox Signaling 4, 39–47. doi: 10.1089/152308602753625843
Wang, W., Dentler, W. L., and Borchardt, R. T. (2001). VEGF increases BMEC monolayer permeability by affecting occludin expression and tight junction assembly. Heart Circulatory physiol. 280:H434–H440. doi: 10.1152/ajpheart.2001.280.1.H434
Wang, Y. C., Lu, Y. B., Huang, X. L., Lao, Y. F., Zhang, L., and Yang, J. (2022). Myeloperoxidase: A new target for the treatment of stroke? Neural Regen. Res. 17, 1711–1716. doi: 10.4103/1673-5374.332130
Wang, Y., Jin, S., Sonobe, Y., Cheng, Y., Horiuchi, H., and Parajuli, B. (2014). Interleukin-1β induces blood-brain barrier disruption by downregulating sonic hedgehog in astrocytes. PLoS One 9:e110024. doi: 10.1371/journal.pone.0110024
Wedmore, C. V., and Williams, T. J. (1981). Control of vascular permeability by polymorphonuclear leukocytes in inflammation. Nature 289, 646–650. doi: 10.1038/289646A0
Wen, G. Y., Wisniewski, H. M., and Kascsak, R. J. (1999). Biondi ring tangles in the choroid plexus of Alzheimer’s disease and normal aging brains: A quantitative study. Brain Res. 832, 40–46. doi: 10.1016/s0006-899301466-3
Weng, Y.-C., and Chou, W.-H. (2015). Neutrophil gelatinase-associated lipocalin and matrix metalloproteinase-9 as potential biomarkers for stroke: A Pilot Study. J. Neurol. Neurophysiol. 6:278. doi: 10.4172/2155-9562.1000278
Wicken, C., Nguyen, J., Karna, R., and Bhargava, P. (2018). Leptomeningeal inflammation in multiple sclerosis: Insights from animal and human studies. Mult. Scler. Relat. Disord. 26, 173–182. doi: 10.1016/j.msard.2018.09.025
Wilczynski, G. M., Konopacki, F. A., Wilczek, E., Lasiecka, Z., Gorlewicz, A., and Michaluk, P. (2008). Important role of matrix metalloproteinase 9 in epileptogenesis. J. Cell Biol. 180, 1021–1035. doi: 10.1083/jcb.200708213
Wolf, S. A., Steiner, B., Akpinarli, A., Kammertoens, T., Nassenstein, C., and Braun, A. (2009). CD4-Positive T Lymphocytes Provide a Neuroimmunological Link in the Control of Adult Hiocampal Neurogenesis. J. Immunol. 182, 3979–3984. doi: 10.4049/jimmunol.0801218
Wong, D., and Dorovini-Zis, K. (1992). Upregulation of intercellular adhesion molecule-1 (ICAM-1). expression in primary cultures of human brain microvessel endothelial cells by cytokines and lipopolysaccharide. J. Neuroimmunol. 39, 11–21. doi: 10.1016/0165-572890170-p
Wu, F., Cao, W., Yang, Y., and Liu, A. (2010). Extensive infiltration of neutrophils in the acute phase of experimental autoimmune encephalomyelitis in C57BL/6 mice. Histochem. Cell Biol. 133, 313–322. doi: 10.1007/s00418-009-0673-2
Yasuda, K., Cline, C., Vogel, P., Onciu, M., Fatima, S., and Sorrentino, B. P. (2013). Drug transporters on arachnoid barrier cells contribute to the blood-cerebrospinal fluid barrier. Drug Metabolism Disposition 41, 923–931. doi: 10.1124/DMD.112.050344
Yu, G., Liang, Y., Zheng, S., and Zhang, H. (2018). Inhibition of myeloperoxidase by N-Acetyl lysyltyrosylcysteine amide reduces oxidative stress-mediated inflammation, neuronal damage, and neural stem cell injury in a murine model of stroke. J. Pharmacol. Exp. Ther. 364, 311–322. doi: 10.1124/jpet.117.245688
Zappia, M., Manna, I., Serra, P., Cittadella, R., Andreoli, V., and La Russa, A. (2004). Increased risk for Alzheimer disease with the interaction of MPO and A2M polymorphisms. Arch. Neurol. 61, 341–344. doi: 10.1001/ARCHNEUR.61.3.341
Zenaro, E., Piacentino, G., and Constantin, G. (2017). The blood-brain barrier in Alzheimer’s disease. Neurobiol. Dis. 107, 41–56. doi: 10.1016/j.nbd.2016.07.007
Zenaro, E., Pietronigro, E., Bianca, V., della Piacentino, G., Marongiu, L., and Budui, S. (2015). Neutrophils promote Alzheimer’s disease-like pathology and cognitive decline via LFA-1 integrin. Nat. Med. 21, 880–886. doi: 10.1038/nm.3913
Zhang, H., Ray, A., Miller, N. M., Hartwig, D., Pritchard, K. A., and Dittel, B. N. (2016). Inhibition of myeloperoxidase at the peak of experimental autoimmune encephalomyelitis restores blood-brain barrier integrity and ameliorates disease severity. J. Neurochem. 136, 826–836. doi: 10.1111/jnc.13426
Zhou, H., Andonegui, G., Wong, C. H. Y., and Kubes, P. (2009). Role of Endothelial TLR4 for Neutrophil Recruitment into Central Nervous System Microvessels in Systemic Inflammation. J. Immunol. 183, 5244–5250. doi: 10.4049/jimmunol.0901309
Zlokovic, B. V. (2008). The blood-brain barrier in health and chronic neurodegenerative disorders. Neuron 57, 178–201. doi: 10.1016/j.neuron.2008.01.003
Keywords: neutrophils, blood-brain barrier, blood-cerebrospinal fluid barrier (BCSFB), neurodegenerative diseases, neuroinflammation
Citation: Santos-Lima B, Pietronigro EC, Terrabuio E, Zenaro E and Constantin G (2022) The role of neutrophils in the dysfunction of central nervous system barriers. Front. Aging Neurosci. 14:965169. doi: 10.3389/fnagi.2022.965169
Received: 09 June 2022; Accepted: 21 July 2022;
Published: 11 August 2022.
Edited by:
Donghui Zhu, Stony Brook University, United StatesReviewed by:
Heather A. Ferris, University of Virginia, United StatesCopyright © 2022 Santos-Lima, Pietronigro, Terrabuio, Zenaro and Constantin. This is an open-access article distributed under the terms of the Creative Commons Attribution License (CC BY). The use, distribution or reproduction in other forums is permitted, provided the original author(s) and the copyright owner(s) are credited and that the original publication in this journal is cited, in accordance with accepted academic practice. No use, distribution or reproduction is permitted which does not comply with these terms.
*Correspondence: Elena Zenaro, ZWxlbmEuemVuYXJvQHVuaXZyLml0; Gabriela Constantin, Z2FicmllbGEuY29uc3RhbnRpbkB1bml2ci5pdA==
Disclaimer: All claims expressed in this article are solely those of the authors and do not necessarily represent those of their affiliated organizations, or those of the publisher, the editors and the reviewers. Any product that may be evaluated in this article or claim that may be made by its manufacturer is not guaranteed or endorsed by the publisher.
Research integrity at Frontiers
Learn more about the work of our research integrity team to safeguard the quality of each article we publish.