- 1Convergence Research Center for Brain Science, Brain Science Institute, Korea Institute of Science and Technology (KIST), Seoul, South Korea
- 2Division of Bio-Medical Science and Technology, Korea Institute of Science and Technology (KIST) School, University of Science and Technology (UST), Seoul, South Korea
- 3Department of Biological Engineering, Massachusetts Institute of Technology (MIT), Cambridge, MA, United States
Neuronal accumulation of mis-folded tau is the pathological hallmark of multiple neurodegenerative disorders, including Alzheimer’s disease. Distinct from amyloid plaques, which appear simultaneously throughout the brain, tau pathology develops first in a specific brain region and then propagates to neuroanatomically connected brain regions, exacerbating the disease. Due to the implication in disease progression, prevention of tau transmission is recognized as an important therapeutic strategy that can halt disease progression in the brain. Recently, accumulating studies have demonstrated diverse cellular mechanisms associated with cell-to-cell transmission of tau. Once transmitted, mis-folded tau species act as a prion-like seed for native tau aggregation in the recipient neuron. In this review, we summarize the diverse cellular mechanisms associated with the secretion and uptake of tau, and highlight tau-trafficking receptors, which mediate tau clearance or cell-to-cell tau transmission.
Introduction
Tau is a microtubule-associated protein, which is extremely soluble in physiological condition (Barbier et al., 2019; Iwata et al., 2019). Full-length human tau contains a microtubule binding domain, which contain 18 lysine residues. The positive charges of lysine residues drive tau to bind a microtubule, a negatively charged polymer (Jho et al., 2010). Upon binding, tau stabilizes microtubules promoting microtubule assembly, which is critical for axonal outgrowth (Fischer et al., 2009; Rodríguez-Martín et al., 2013). Tau also plays a role in anchoring microtubules to other cytoskeletal filaments and cellular organelles for maintaining axonal structure and function (Miyata et al., 1986; Jung et al., 1993). More recent studies have elucidated new physiological roles of tau as a synaptic protein in accelerating spine formation, dendritic elongation, and synaptic plasticity (Chen et al., 2012; Kimura et al., 2014; Zempel et al., 2017; Velazquez et al., 2018). Despite the expected role of tau in neuronal structure and function, tau-deficient mice appeared to be normal presenting with no overt phenotype or malformations in the nervous system (Harada et al., 1994). Depletion of tau did not affect axonal elongation in cultured neurons (Tint et al., 1998). These observations suggest that other microtubule-associated proteins could play the role of tau in the absence of tau protein (Takei et al., 2000). While the reported tau knockout strains presented without obvious phenotype when young, one tau knockout strain showed muscle weakness and motor deficits when old, suggesting the pathological role of tau in age-related diseases (Ikegami et al., 2000; Lei et al., 2012).
Under pathological conditions, tau hyperphosphorylation occurs, and the charge balance between tau and microtubules becomes disrupted (Gong et al., 2005; Alonso et al., 2018). Consequently, hyperphosphorylated tau dissociates from microtubules and accumulates in the cytosol (Figure 1A). In the cytoplasm, mis-localized tau undergoes various post-translational modifications, such as acetylation, sumoylation, ubiquitination, glycosylation, methylation, nitration, truncation, and inter-molecular bond formation (Martin et al., 2011; Haque et al., 2016). Tau acetylation prevents the degradation of phosphorylated tau (Min et al., 2010). Disulfide-bond formation between tau molecules generates structurally stable tau oligomers that play a critical role in tau aggregation and transmission (Kim et al., 2015; Prifti et al., 2021). Proteolytic cleavage of tau is also crucial in the generation of compact filaments by removing the N-and C-terminal flanking regions of tau (Friedhoff et al., 2000). The complex pattern of tau modifications would change the physical and chemical properties of tau as the infectious, aggregation-prone form. The accumulation of filamentous tau aggregates is a pathological hallmark of tauopathies, including Alzheimer’s disease (AD), frontotemporal dementia (FTDP-17), Pick’s disease, and progressive supranuclear palsy (PSP) (Iqbal et al., 2010; Orr et al., 2017). In tauopathy brains, tau pathology develops first in a specific brain region, and then propagates into anatomically connected brain regions. For example, in the brains of AD patients, tau pathology appears first in the trans-entorhinal cortex and propagates into the neuroanatomically connected hippocampus, causing cognitive impairments (Braak and Braak, 1991). In PSP brains, tau pathology develops in the basal ganglia at an early stage, causing motor symptoms, and later spreads to the cerebellum and brainstem, resulting in motor failure (Williams et al., 2007).
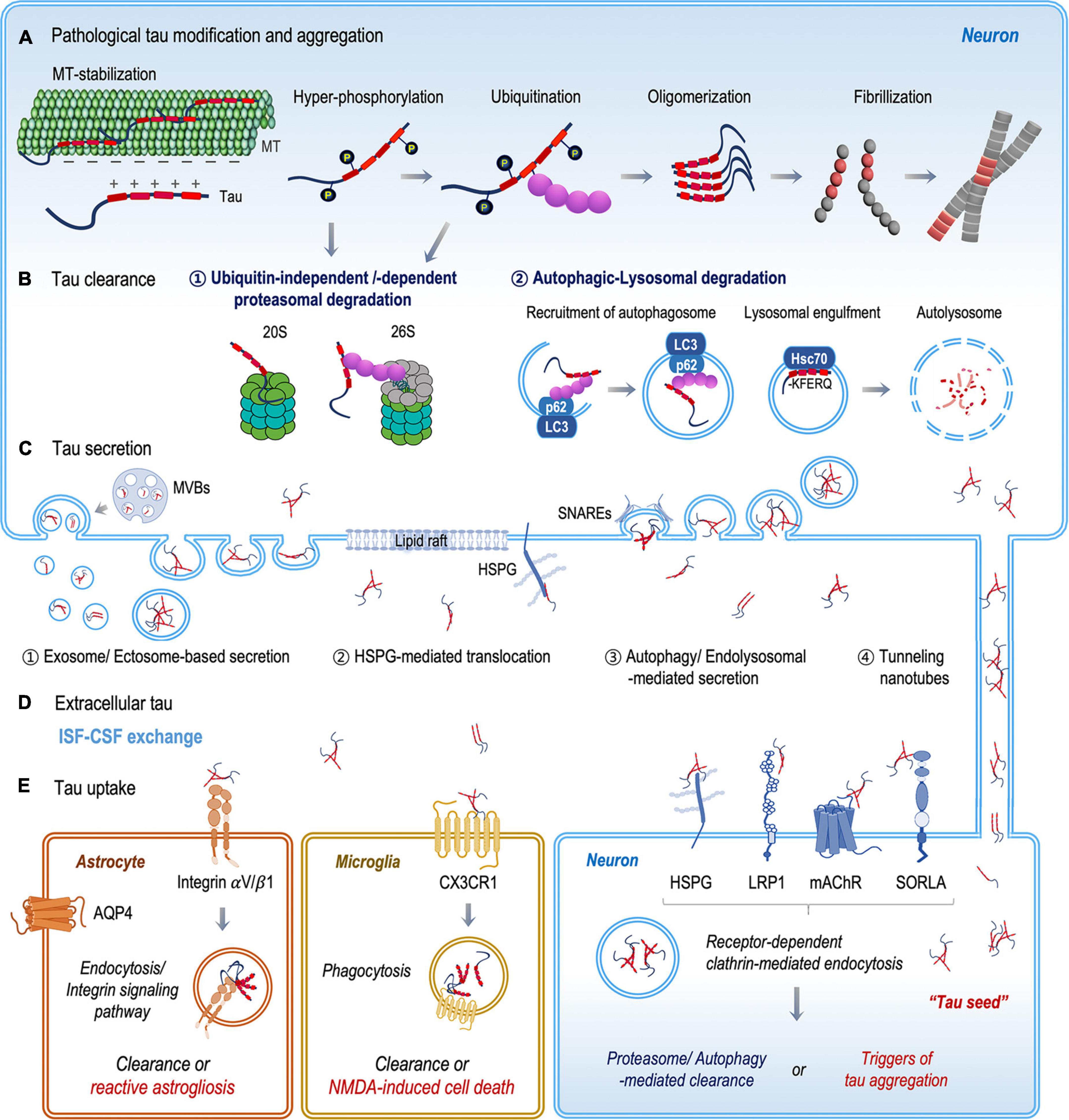
Figure 1. Schematic illustration of the fate of tau protein between clearance and transmission. (A) Mis-localized tau undergoes various post-translational modifications, such as phosphorylation and ubiquitination. Chemically and structurally modified tau becomes aggregated and accumulated in the cytoplasm. (B) Mis-folded and aggregated tau proteins are degraded by the ubiquitin–proteasome and autophagosome-lysosomal systems. (C) Tau proteins are released into the extracellular space both as a free protein and in vesicles, through (1) vesicular and (2–4) non-vesicular secretion pathways. (D) Extracellular tau proteins are eliminated through ISF–CSF exchange within the glymphatic system or are taken up by neurons, microglia, and astrocytes. (E) In recipient neurons, extracellular tau proteins bind specific tau receptors (HSPG, LRP1, mAChRs, and SORLA) and are internalized by receptor-dependent clathrin-mediated endocytosis. Extracellular tau clearance by microglia and astrocytes includes the binding and internalization of tau via the CX3CR1 receptor in microglia and the integrin αV/β1 receptor in astrocytes.
As a possible mechanism of tau propagation in the brain, a cell-to-cell transmission hypothesis has been raised. Tau transmission occurs through the trans-synaptic transfer of mis-folded tau aggregates from a donor cell to a recipient cell. The process can be divided into three basic steps: (1) secretion of pathological tau aggregates from donor cells, (2) uptake of the secreted tau species by recipient cells, and (3) initiation of tau pathology in the recipient cells (Gibbons et al., 2019; Brunello et al., 2020). Accumulating evidence has demonstrated that tau protein is actively released into the extracellular space in the brain. Higher levels of tau (453.6 ± 343.5 pg/mL) and tau phosphorylated at T181 residue (67.8 ± 18.0 pg/mL) were detected in cerebrospinal fluid (CSF) of AD patients (Haense et al., 2008; Kandimalla et al., 2013). Phosphorylated tau at T181, T217, and T205 residues were detected in the CSF of AD patients, and the levels of phosphorylated tau increased during the disease progression, positively correlating with neuronal dysfunction (Fagan et al., 2007; Barthélemy et al., 2020). Increased levels of N-224 tau fragments were also observed in the CSF of AD patients and in conditioned medium of neuron cell cultures (Ramcharitar et al., 2013; Zhang et al., 2014; Cicognola et al., 2019). Although transmittable tau species were not fully characterized, several recent studies have shown that phosphorylated tau can be translocated through the plasma membrane (Katsinelos et al., 2018; Merezhko et al., 2018). Also, various forms of tau aggregates were detected in the extracellular space (Lasagna-Reeves et al., 2011; Goedert, 2016). Significantly increased levels of tau oligomers and a mix of dimers, trimers, and tetramers were detected in the CSF of AD patients (Takeda et al., 2015; Sengupta et al., 2017). A line of evidence suggests that soluble tau oligomers, before forming fibrils, induce neuritic degeneration, perturb fast axonal transport of membranous organelles (Swanson et al., 2017), and increase reactive oxygen species leading to neuronal cell death (Lasagna-Reeves et al., 2012; Kim et al., 2015; Shafiei et al., 2017). In addition, intra-hippocampal injection of tau oligomers, rather than tau fibrils, were effective in inducing memory impairments in mice (Fá et al., 2016; Gerson et al., 2016a; Puzzo et al., 2017). Alongside neurotoxicity, tau oligomers were actively internalized into cells to a greater extent than tau monomers or insoluble aggregates, and the internalized tau oligomers could act as a structural seed to initiate native tau aggregation (Usenovic et al., 2015; Gerson et al., 2016b; Manassero et al., 2017; Swanson et al., 2017). The presence of extracellular tau has long been considered the result of neuronal cell death and the release of intracellular contents (Fricker et al., 2018). However, recent accumulating studies have reported extracellular tau also exists along with healthy and mature neurons (Pooler et al., 2013; Yamada et al., 2014; Wu et al., 2016). Upon stimulation of neuronal activity, tau binds to the cytosolic side of synaptic vesicles and is secreted with neurotransmitters (McInnes et al., 2018). An increased level of tau was detected in the interstitial fluid (ISF) of tau transgenic mice stimulated with optogenetic activation (Yamada et al., 2014b). Moreover, the treatment of extracellular tau induces neuronal hyperactivity leading to the secretion of endogenous tau suggesting the bidirectional relationship between neuronal activity and tau release (Bright et al., 2015).
While the amount and types of extracellular tau vary depending on the disease status, it is clear that tau is constantly secreted into the extracellular space (Wattmo et al., 2020). Then, adjacent neurons uptake extracellular tau species through diverse endocytosis mechanisms. In the recipient neurons, pathogenic tau aggregates can initiate tau pathology as a “structural seed” for native tau aggregation (Clavaguera et al., 2009, 2013; Lasagna-Reeves et al., 2012). In 2013, an in vitro model of tau propagation, in which somatodendritic and axonal compartments were separated in microfluidic chambers, showed that tau aggregates were internalized by both compartments and transported along the axon in either the anterograde or the retrograde direction (Wu et al., 2013). In 2014, an in vivo model of tau propagation demonstrated contralateral and anterior/posterior spread of tau pathology in the brain of tau-transgenic mice (Ahmed et al., 2014). In the brain, the spread of tau pathology was dependent on synaptic connectivity rather than spatial proximity. Accordingly, extracellular tau has emerged as an important target for tau-targeted immunotherapy. Toward this, efforts have been made to characterize prion-like tau species and tau trafficking-receptors, which are critical in tau transmission. A comprehensive understanding of tau transmission would provide new perspectives for the diagnosis and prevention of tau pathology. Here, we review the diverse cellular mechanisms associated with tau clearance and transmission.
Molecular Mechanisms of Tau Secretion
In classical secretory pathways, secretory proteins contain signal peptides, which are well-known sequence motifs targeting proteins for translocation across the endoplasmic reticulum membrane (Cohen et al., 2020). Since tau protein does not contain an apparent signal peptide, tau was thought to be released from dying or dead neurons. However, proteins without a signal peptide can also be secreted into extracellular space via non-conventional pathways. Over the last decade, mounting evidence has demonstrated that tau secretion occurs through diverse non-conventional pathways. Due to the implication in tau pathology, extensive studies have investigated the pathological roles of the extracellular tau. In comparison, the physiological role of tau in the extracellular space remains largely unknown. A few preliminary studies suggested novel functions of tau in the extracellular space. For example, the treatment of extracellular tau increases the electrical activity of primary neuron culture (Bright et al., 2015) and the treatment of tau fibrils increased the formation of tunneling nanotubes (TNTs) between neurons (Tardivel et al., 2016). Although the role of tau is not clear, tau proteins are actively secreted into the extracellular space in both physiological and disease conditions. In this section, we will review the diverse non-conventional mechanisms associated with tau secretion (Figures 1B,C).
Heparan Sulfate Proteoglycan-Mediated Translocation of Tau
Tau translocation across the plasma membrane is recently being highlighted as a key mechanism of non-conventional tau secretion. Tau translocation is a two-step process: (1) recruitment of tau to the plasma membrane and (2) translocation mediated by heparan sulfate proteoglycans (HSPGs) (Katsinelos et al., 2018; Merezhko et al., 2018). Lipid microdomains containing cholesterol, sphingomyelin, phosphatidyl serine, and PI(4,5)P2 are the major trans-elements responsible for recruiting tau to the plasma membrane. Katsinelos et al. (2018) showed that PI(4,5)P2 could initiate the translocation of tau. In their study, lysine residues in microtubule-binding domain of tau can form an interaction surface with the negatively charged PI(4,5)P2 on the plasma membrane. Lipid rafts containing cholesterol and sphingomyelin are necessary for the recruitment of pathological tau aggregates (Lasagna-Reeves et al., 2014; Patel et al., 2015). Merezhko et al. (2018) demonstrated that the disruption of lipid rafts in the plasma membrane decreased tau secretion by 90%. Once tau is recruited to plasma membrane, HSPG-mediated translocation occurs. HSPGs are complex carbohydrate-modified proteins carrying heparan sulfate (HS) and are ubiquitously expressed on the transmembrane. HSPGs can recruit and cluster molecules in transmembrane domains, mediating their translocation (Condomitti and de Wit, 2018). Accumulating evidence suggests that HSPGs anchor tau and mediate bi-directional translocation of tau through the plasma membrane. Xu and Esko (2014) showed that a decrease of HSPGs in the plasma membrane suppressed tau secretion significantly. Altogether, tau protein can be secreted into the extracellular space via direct translocation through the plasma membrane.
Autophagy/Endolysosomal-Mediated Secretion
In cells, proteins that are no longer needed or are mis-folded/damaged are degraded by the ubiquitin–proteasome system (UPS) and the autophagy-lysosome system (Wong and Cuervo, 2010; Dikic, 2017). In the early stage of tau pathology, mis-localized and mis-folded tau proteins start to accumulate in the cytosol. The UPS mediates the clearance of soluble tau monomers and aggregates. In the UPS, tau proteins are tagged with ubiquitin and the ubiquitinylated tau proteins are digested by the 26S proteasome (Schmidt and Finley, 2014). As tau pathology progresses, tau proteins assemble into large, insoluble fibrils, which exceed the capacity of the UPS and lead to proteasome dysfunction (Morishima-Kawashima et al., 1993). As a result, polyubiquitinated tau proteins are accumulated in the cytosol and become a compartment of insoluble tau aggregates such as paired helical filaments (Morishima-Kawashima et al., 1993).
As an alternative to the UPS, autophagy plays a critical role in the clearance of pathological tau aggregates. Depending on the cargoes, autophagy is divided into three types: (1) chaperon-mediated autophagy (CMA), (2) endosomal-microautophagy (e-MI), and (3) macroautophagy. Soluble tau monomers and oligomers are cleared by CMA and e-MI, whereas large, insoluble tau aggregates are cleared by macroautophagy (Wang et al., 2009; Krüger et al., 2012; Caballero et al., 2018; Uytterhoeven et al., 2018). In macroautophagy, tau aggregates are first wrapped inside a specialized organelle, called an autophagosome, and the autophagosomes fuse with lysosomal vesicles to form autolysosomes, enabling the degradation of their cargoes (Dikic and Elazar, 2018). However, in a degenerating neuron, the axonal supply of lysosomes is restricted, resulting in the accumulation of autophagosomes (Farfel-Becker et al., 2019). Recent accumulating evidence has demonstrated that the deficiency of lysosomes drives the autophagosomes to fuse with the plasma membrane, leading to the secretion of their cargos into the extracellular milieu (Tang et al., 2015; Lonati et al., 2018; Kang et al., 2019). Tang et al. (2015) reported electron microscopic images of autophagic vacuoles containing tau approaching the plasma membrane. The fusion of autophagosomes with the plasma membrane is mediated by the assembly of soluble N-ethyl maleimide sensitive factor attachment protein receptors (SNAREs) (Wang et al., 2016). SNARE proteins (Sec22b) on autophagosomes interact with SNAREs (syntaxin 3/4 and SNAP-23/29) on the plasma membrane for membrane fusion and cargo secretion (Kimura et al., 2017a,b). CMA is also involved in the clearance and secretion of soluble tau monomers and oligomers. Tau protein contains KFERQ-like motifs, known as CMA-substrates, which are recognized by heat shock cognate 71 kDa protein (Hsc70) (Wang et al., 2009; Robert et al., 2019). Hsc70 mediates the sorting of tau proteins to lysosomes or late-endosomes (Wang et al., 2009). Cargo-loaded endosomes and lysosomes are transported to the cell periphery and fuse with the plasma membrane, releasing tau proteins into the extracellular space (Fontaine et al., 2016; Lee and Ye, 2018).
Exosome/Ectosome-Based Secretion of Vesicular Tau
Intercellular communication can occur in multiple forms. With these diverse mechanisms, cells exchange their cellular information through the secretion and uptake of extracellular vesicles, which contain diverse cellular agents (proteins, lipids, reactive oxygen species, and genetic information) (Cocucci and Meldolesi, 2015). Mounting evidence has suggested that tau is one of the cellular components delivered by extracellular vesicles. The uptake of extracellular vesicles containing pathogenic tau aggregates can initiate tau pathology in recipient cells (Polanco et al., 2016; Wang et al., 2017). Extracellular vesicles are heterogeneous, with sizes ranging broadly from 50 to 1000 nm. Exosomes (30–150 nm) and ectosomes (100 and 1000 nm) are known to mediate tau transmission (DeLeo and Ikezu, 2018).
Exosomes are nano-sized vesicles derived from endosomal compartments called multivesicular bodies (MVBs) (Yáñez-Mó et al., 2015). Hsc70 delivers tau proteins into exosomes through interaction with the endosomal sorting complex required for transport (ESCRT) proteins (Wang et al., 2017). ESCRTs play a critical role in sorting exosomal cargos and secreting exosomes into the extracellular space. Once secreted into the extracellular space, exosomes are not only circulating in the brain-specific ISF, but also travel into the CSF and blood in humans (Caby et al., 2005; Fiandaca et al., 2015; Welton et al., 2017; Wu et al., 2017). Tau has been detected in the exosomes collected from the CSF and blood of AD patients (Saman et al., 2012; Jia et al., 2019). Particularly, in Jia et al. (2019) study, the level of exosomal tau was significantly higher in the CSF of AD patients than that of the healthy controls.
Ectosomes are microvesicles, released from the plasma membrane. Ectosome shedding is induced by intracellular calcium levels, inflammatory molecules, or oxidative stress (Piccin et al., 2007; Doeuvre et al., 2009). Ectosomal tau has also been found in neuroblastoma cell culture, the ISF of mice, and the CSF of AD patients and healthy controls (Dujardin et al., 2014; Spitzer et al., 2019). Recent evidence shows that neuron-derived exosomes or extracellular vesicles extracted from the AD patients had the potential to promote tau pathology when injected into the brains of wild-type mice (Winston et al., 2016; Ikezu et al., 2020).
Molecular Mechanisms of Extracellular Tau Uptake
In the extracellular space, the level of tau is maintained by the balance between secretion and clearance. In peripheral organs, the lymphatic system mediates the clearance of cellular wastes in the ISF (Aspelund et al., 2015). However, in the brain, lymphatic vessels are found only in the brain meninges, not in the deep brain where tau aggregates are secreted. Instead, the glymphatic system has been highlighted due to its role in clearing extracellular wastes, including amyloid-β and tau, through paravascular ISF–CSF exchange (Iliff et al., 2012). Accordingly, the breakdown of the glymphatic system is associated with various neurodegenerative diseases, including AD and traumatic brain injury (Rasmussen et al., 2018). Microglia and astrocytes also play a role in eliminating extracellular tau (Bolós et al., 2016; Piacentini et al., 2017; Hopp et al., 2018). However, the prolonged activation of microglia and astrocytes could stimulate neuroinflammatory responses that facilitate neuronal degeneration. Therefore, microglia- and astrocyte-mediated clearance might be effective particularly at the early stage of AD (Kinney et al., 2018). In AD brains, extracellular tau interacts with amyloid precursor protein (APP) and β-amyloid. Takahashi et al. (2015) showed that the extracellular region of APP is involved in the uptake of tau fibrils into a cell.
Also, several studies have shown that the spreading of tau pathology was enhanced by the injection of β-amyloid into mouse brain. Actually, the binding affinity of tau for β-amyloid is almost 1000-fold higher than for tau (Guo et al., 2006). The mutual interaction between β-amyloid and tau exaggerates the cross-seeding effects of tau, spreading tau pathology in the brain (Vergara et al., 2019; Vogel et al., 2020). Extracellular tau proteins are also taken up by neurons via diverse cellular mechanisms. In recipient neurons, most of the internalized proteins are degraded through the endosome–lysosome route, but a portion of internalized tau could stimulate tau pathology, acting as a seed for native tau aggregation. In this section, we will review the diverse cellular mechanisms of tau uptake (Figures 1D,E).
Heparan Sulfate Proteoglycan-Associated Macropinocytosis
Macropinocytosis is the bulk-endocytosis mediating the non-specific internalization of large amounts of extracellular fluid. Macropinocytosis plays a central role in tau pathology by mediating the internalization of large, insoluble tau aggregates (Wu et al., 2013; Evans et al., 2018). Tau aggregates bind to HSPGs on the neuronal surface, triggering macropinocytosis (Holmes et al., 2013; Rauch et al., 2018; Annadurai et al., 2021). Then, the extension of actin-dependent membrane ruffles engulfs tau aggregates, leading to the formation of macropinosomes. Macropinosomes are large vacuoles with an approximate diameter of up to 5 μm (Swanson, 2008). The maturation of macropinosomes involves membrane rearrangement for concentrating cargo proteins and then trafficking the cargos to the lysosome for degradation and recycling (Donaldson, 2019). The modification of HS chains on HSPGs is critical for tau binding and internalization. Rauch et al. (2018) reported that 6-O-sulfation of the HS sidechains is important for tau binding. Another study demonstrated that 3-O-sulfation enhances tau binding to the cell surface (Zhao et al., 2020).
Receptor-Dependent Clathrin-Mediated Endocytosis
Clathrin-mediated endocytosis mediates the internalization of a wide range of cargo molecules from the cell surface to the interior. The process is characterized by the recruitment of cargo molecules by various transmembrane receptors and the formation of the clathrin-coated pit (CCP) for internalization (Doherty and McMahon, 2009). Recent studies have shown several endocytic receptors binding to extracellular tau. Lipoprotein receptor-related protein 1 (LRP1) (Lane-Donovan et al., 2014; Rauch et al., 2020), muscarinic acetylcholine receptors (mAChRs) (Morozova et al., 2019), and sorting protein-related receptor (SORLA) (Bok et al., 2021) are known to mediate tau internalization.
LRP1 is considered a major neuronal receptor that is involved in the internalization of extracellular tau. Rauch et al. (2020) reported that LRP1 is a master receptor mediating clathrin-mediated endocytosis of tau aggregates, playing a critical role in tau transmission. In their study, the lysine-rich microtubule binding domain of tau interacts with the cysteine-rich ligand binding domain of LRP1 (Rauch et al., 2020). The genetic silencing of LRP1 inhibited the uptake of various forms of tau, including monomers, oligomers, and fibrils, almost completely in H4 neuroglioma cells. The knockdown of LRP1 also suppressed the spreading of tau in the brain of mice expressing human tau P301L mutant. Cooper et al. (2021) also demonstrated LRP1-mediated internalization of tau aggregates extracted from AD patients. In their study, internalized tau monomers were degraded in lysosomes, but internalized tau aggregates induced tau seeding in the recipient cells. A member of the LDL-receptor superfamily, SORLA, was also identified as a receptor of extracellular tau (Cooper et al., 2021). mAChRs are also known to mediate clathrin-mediated internalization of tau. Gomez-Ramos et al. (2008)Gómez-Ramos et al. (2009) identified that the C-terminal domain of tau binds to M1/M3 subtypes of mAChR as a receptor agonist, increasing intracellular calcium concentration, and leading to neuronal cell toxicity. Morozova et al. (2019) demonstrated that mAChRs not only activate tau-induced calcium influx but also mediate the internalization of extracellular tau.
Recent genome-wide association studies (GWASs) have revealed genetic factors associated with sporadic AD. Specifically, APOE, bridging integrator 1 (BIN1), and phosphatidylinositol binding clathrin assembly protein (PICALM) showed a strong association with AD. PICALM binds to clathrin and its adaptor proteins, initiating CCP assembly (Meyerholz et al., 2005). Ando et al. (2016) reported the co-localization of phosphorylated tau with PICALM in the brains of AD patients. BIN1 is known to be involved in clathrin-mediated endocytosis through its interaction with clathrin and dynamin (Doherty and McMahon, 2009). However, the role of BIN1 in AD pathology remains largely unclear. Significantly decreased levels of BIN1 were observed in the brains of late-onset AD patients (Holler et al., 2014). The knock-down of BIN1 is known to promote endocytosis of extracellular tau aggregates (Calafate et al., 2016).
Tau Clearance by Microglia and Astrocytes
Microglia, the professional phagocytes of the brain, mediates the clearance of extracellular tau aggregates, as well as dead or dying neurons bearing tau aggregates (Kofler and Wiley, 2011; Arcuri et al., 2017). In AD brains, extracellular tau proteins activate microglia, promoting phagocytosis of tau aggregates (Das et al., 2020). During this process, the extracellular tau binds CX3CR1, a chemokine receptor expressed on the surface of microglia (Bolós et al., 2016; Perea et al., 2020). Under physiological conditions, CX3CR1 interacts with fractalkines (CX3CL1), which are constitutively expressed by neuronal cells in either a membrane-bound or soluble form (Hatori et al., 2002). The CX3CR1/CX3CL1 axis plays a significant role in maintaining the homeostasis of the central nervous system. In the AD brain, the expression of fractalkines is reduced, disrupting the CX3CR1/CX3CL1 axis (Finneran and Nash, 2019). Additionally, tau aggregates compete to bind CX3CR1, mediating the phagocytosis of tau aggregates into microglia (Perea et al., 2020).
Astrocytes are specialized glial cells that are involved in the recycling and clearance of unnecessary synapses, synaptic debris, and extracellular protein aggregates in the brain (Sofroniew and Vinters, 2010; Jung and Chung, 2018). Astrocytes express a low amount of endogenous tau (Perea et al., 2019). However, elevated levels of tau have been observed in astrocytes of AD brains (Ferrer et al., 2018). Astrocytes are known to uptake extracellular tau proteins via distinct mechanisms depending on their aggregation states. Tau monomers are internalized by HSPGs-mediated translocation (Perea et al., 2019), and tau aggregates are internalized by endocytosis (de Calignon et al., 2012; Martini-Stoica et al., 2018). Tau aggregates bind to integrins on the surface of astrocytes, resulting in the activation of the integrin signaling pathway, which triggers tau endocytosis (Perea et al., 2020). In the early stage of tauopathies, the activation of astrocytes plays a critical role in neuroprotection, secreting pro-inflammatory cytokines and chemokines. However, prolonged activation of astrocytes eventually stimulates neuroinflammatory responses that facilitate neuronal degeneration (Zhang and Jiang, 2015; Ransohoff, 2016).
Tunneling Nanotubes
Tunneling nanotubes are cell–cell communication bridges composed of filamentous-actin positive and tubulin negative membranous structures with a diameter of 50–800 nm (Davis and Sowinski, 2008). TNTs, discovered in 2004, indicate a novel mechanism for long-range intercellular communication in various cell types, such as immune cells and neuronal cells (Rustom et al., 2004). Recent in vivo imaging studies have shown the occurrence of TNTs in the mouse retina and cerebral cortex. Alarcon-Martinez et al. (2020) reported the occurrence of actin-positive TNTs between retinal pericytes using two-photon imaging. Chen and Cao (2021) reported actin-positive TNTs between astrocytes and neurons in the cerebral cortex of mice and showed EGFP transport from astrocytes to neurons in the cortex through TNTs. In addition, TNTs can be used as a pathway for transmitting various pathogens, including prions and prion-like proteins (Gousset et al., 2009). Among diverse prion-like proteins, tau is known as an extrinsic factor that increases TNT formation. Tardivel et al. (2016) showed that treatment of tau fibrils increased the TNT population in neuronal cells by almost three times. Additionally, Abounit et al. (2016) showed that tau fibril treatment increased TNT formation not only in neuronal cells but also in Hela cells. Based on these results, tau fibrils lead to an increase in TNT formation and, facilitate the intercellular spread of pathological tau.
Conclusion
Tau pathology includes tau modification, aggregation, and transmission, which are associated with multiple cellular pathways. Accordingly, it is extremely challenging to identify therapeutic targets for tau-targeted drug discovery. During early trials, tau phosphorylation was considered as a key initiating event regulating tau pathology, and great effort was made for the development of kinase inhibitors. Unfortunately, all trials targeting kinases have failed to demonstrate clinical efficacy in patients with tauopathy, suggesting that tau phosphorylation is merely a part of tau pathology. There are also efforts to develop anti-tau agents inhibiting tau aggregation, or autophagy activators boosting the clearance of tau aggregates. More recently, extracellular tau is recognized as an important therapeutic target to prevent disease progression (Gomez-Ramos et al., 2006; Simon et al., 2012). Bepranemab, an anti-tau IgG4 antibody developed by Hoffmann-La Roche, is currently undergoing phase 2 clinical trials in AD patients (NCT04867616) (Albert et al., 2019). JNJ-63733657, an anti-tau IgG1 antibody developed by Janssen, is undergoing phase 2 trials in AD patients (NCT04619420) (Beshir et al., 2022). In the extracellular space, antibody-tau complexes are expected to be cleared as extracellular waste by microglia, astrocytes or the glymphatic system. However, the clearing efficiency of antibody-tau complexes has not been reported. So far, fragmented understandings of tau pathology cause repeated failures in tau-targeted drug discovery. We believe that comprehensive understanding of the fate of tau in physiological and pathological condition is necessary for the success of tau-targeted drug development.
Author Contributions
SL, AS, KK, EF, and YK contributed to the search and assessment of the available literature. SL, AS, KK, and YK interpreted the results of previous studies and wrote the manuscript. SL, KK, YS, and YK edited and commented upon the final draft. All authors reviewed the manuscript.
Funding
This research was supported by the Korea Health Technology R and D Project through the Korea Health Industry Development Institute (KHIDI) and Korea Dementia Research Center (KDRC), funded by the Ministry of Health and Welfare and Ministry of Science and ICT, Republic of Korea (No. HU21C0223); the National Research Foundation of Korea (NRF) (2017R1A6A3A0401238422, 2021R1A2C2093734, and 2022R1C1C100714611); and the Korea Institute Science and Technology (KIST) Institutional Program (2V08250).
Conflict of Interest
The authors declare that the research was conducted in the absence of any commercial or financial relationships that could be construed as a potential conflict of interest.
Publisher’s Note
All claims expressed in this article are solely those of the authors and do not necessarily represent those of their affiliated organizations, or those of the publisher, the editors and the reviewers. Any product that may be evaluated in this article, or claim that may be made by its manufacturer, is not guaranteed or endorsed by the publisher.
References
Abounit, S., Wu, J. W., Duff, K., Victoria, G. S., and Zurzolo, C. (2016). Tunneling nanotubes: a possible highway in the spreading of tau and other prion-like proteins in neurodegenerative diseases. Prion 10, 344–351. doi: 10.1080/19336896.2016.1223003
Ahmed, Z., Cooper, J., Murray, T. K., Garn, K., Mcnaughton, E., Clarke, H., et al. (2014). A novel in vivo model of tau propagation with rapid and progressive neurofibrillary tangle pathology: the pattern of spread is determined by connectivity, not proximity. Acta Neuropathol. 127, 667–683. doi: 10.1007/s00401-014-1254-6
Alarcon-Martinez, L., Villafranca-Baughman, D., Quintero, H., Kacerovsky, J. B., Dotigny, F., Murai, K. K., et al. (2020). Interpericyte tunnelling nanotubes regulate neurovascular coupling. Nature 585, 91–95.
Albert, M., Mairet-Coello, G., Danis, C., Lieger, S., Caillierez, R., Carrier, S., et al. (2019). Prevention of tau seeding and propagation by immunotherapy with a central tau epitope antibody. Brain 142, 1736–1750. doi: 10.1093/brain/awz100
Alonso, A. D., Cohen, L. S., Corbo, C., Morozova, V., Elidrissi, A., Phillips, G., et al. (2018). Hyperphosphorylation of tau associates with changes in its function beyond microtubule stability. Front. Cell. Neurosci. 12:338. doi: 10.3389/fncel.2018.00338
Ando, K., Tomimura, K., Sazdovitch, V., Suain, V., Yilmaz, Z., Authelet, M., et al. (2016). Level of PICALM, a key component of clathrin-mediated endocytosis, is correlated with levels of phosphotau and autophagy-related proteins and is associated with tau inclusions in AD. PSP and Pick disease. Neurobiol. Dis. 94, 32–43. doi: 10.1016/j.nbd.2016.05.017
Annadurai, N., De Sanctis, J. B., Hajduch, M., and Das, V. (2021). Tau secretion and propagation: perspectives for potential preventive interventions in Alzheimer’s disease and other tauopathies. Exp. Neurol. 343:113756. doi: 10.1016/j.expneurol.2021.113756
Arcuri, C., Mecca, C., Bianchi, R., Giambanco, I., and Donato, R. (2017). The pathophysiological role of microglia in dynamic surveillance, phagocytosis and structural remodeling of the developing CNS. Front. Mol. Neurosci. 10:191. doi: 10.3389/fnmol.2017.00191
Aspelund, A., Antila, S., Proulx, S. T., Karlsen, T. V., Karaman, S., Detmar, M., et al. (2015). A dural lymphatic vascular system that drains brain interstitial fluid and macromolecules. J. Exp. Med. 212, 991–999.
Barbier, P., Zejneli, O., Martinho, M., Lasorsa, A., Belle, V., Smet-Nocca, C., et al. (2019). Role of Tau as a microtubule associated protein: structural and functional aspects. Front. Aging Neurosci. 11:204. doi: 10.3389/fnagi.2019.00204
Barthélemy, N. R., Li, Y., Joseph-Mathurin, N., Gordon, B. A., Hassenstab, J., Benzinger, T. L., et al. (2020). A soluble phosphorylated tau signature links tau, amyloid and the evolution of stages of dominantly inherited Alzheimer’s disease. Nat. Med. 26, 398–407. doi: 10.1038/s41591-020-0781-z
Beshir, S. A., Aadithsoorya, A., Parveen, A., Goh, S. S. L., Hussain, N., and Menon, V. B. (2022). Aducanumab therapy to Treat Alzheimer’s disease: a narrative review. Int. J. Alzheimers Dis. 2022:9343514.
Bok, E., Leem, E., Lee, B.-R., Lee, J. M., Yoo, C. J., Lee, E. M., et al. (2021). Role of the lipid membrane and membrane proteins in Tau pathology. Front. Cell Dev. Biol. 9:653815. doi: 10.3389/fcell.2021.653815
Bolós, M., Llorens-Martin, M., Jurado-Arjona, J., Hernandez, F., Rábano, A., and Avila, J. (2016). Direct evidence of internalization of tau by microglia in vitro and in vivo. J. Alzheimers Dis. 50, 77–87.
Braak, H., and Braak, E. (1991). Neuropathological stageing of Alzheimer-related changes. Acta Neuropathol. 82, 239–259. doi: 10.1007/BF00308809
Bright, J., Hussain, S., Dang, V., Wright, S., Cooper, B., Byun, T., et al. (2015). Human secreted tau increases amyloid-beta production. Neurobiol. Aging 36, 693–709.
Brunello, C. A., Merezhko, M., Uronen, R.-L., and Huttunen, H. J. (2020). Mechanisms of secretion and spreading of pathological tau protein. Cell. Mol. Life Sci. 77, 1721–1744.
Caballero, B., Wang, Y., Diaz, A., Tasset, I., Juste, Y. R., Stiller, B., et al. (2018). Interplay of pathogenic forms of human tau with different autophagic pathways. Aging Cell 17:e12692. doi: 10.1111/acel.12692
Caby, M.-P., Lankar, D., Vincendeau-Scherrer, C., Raposo, G., and Bonnerot, C. (2005). Exosomal-like vesicles are present in human blood plasma. Int. Immunol. 17, 879–887.
Calafate, S., Flavin, W., Verstreken, P., and Moechars, D. (2016). Loss of Bin1 promotes the propagation of tau pathology. Cell Rep. 17, 931–940. doi: 10.1016/j.celrep.2016.09.063
Chen, J., and Cao, J. (2021). Astrocyte-to-neuron transportation of enhanced green fluorescent protein in cerebral cortex requires F-actin dependent tunneling nanotubes. Sci. Rep. 11:16798. doi: 10.1038/s41598-021-96332-5
Chen, Q., Zhou, Z., Zhang, L., Wang, Y., Zhang, Y.-W., Zhong, M., et al. (2012). Tau protein is involved in morphological plasticity in hippocampal neurons in response to BDNF. Neurochem. Int. 60, 233–242. doi: 10.1016/j.neuint.2011.12.013
Cicognola, C., Brinkmalm, G., Wahlgren, J., Portelius, E., Gobom, J., Cullen, N. C., et al. (2019). Novel tau fragments in cerebrospinal fluid: relation to tangle pathology and cognitive decline in Alzheimer’s disease. Acta Neuropathol. 137, 279–296.
Clavaguera, F., Akatsu, H., Fraser, G., Crowther, R. A., Frank, S., Hench, J., et al. (2013). Brain homogenates from human tauopathies induce tau inclusions in mouse brain. Proc. Natl. Acad. Sci. U.S.A. 110, 9535–9540.
Clavaguera, F., Bolmont, T., Crowther, R. A., Abramowski, D., Frank, S., Probst, A., et al. (2009). Transmission and spreading of tauopathy in transgenic mouse brain. Nat. Cell Biol. 11, 909–913.
Cocucci, E., and Meldolesi, J. (2015). Ectosomes and exosomes: shedding the confusion between extracellular vesicles. Trends Cell Biol. 25, 364–372. doi: 10.1016/j.tcb.2015.01.004
Cohen, M. J., Chirico, W. J., and Lipke, P. N. (2020). Through the back door: unconventional protein secretion. Cell Surf. 6:100045. doi: 10.1016/j.tcsw.2020.100045
Condomitti, G., and de Wit, J. (2018). Heparan sulfate proteoglycans as emerging players in synaptic specificity. Front. Mol. Neurosci. 11:14. doi: 10.3389/fnmol.2018.00014
Cooper, J. M., Lathuiliere, A., Migliorini, M., Arai, A. L., Wani, M. M., Dujardin, S., et al. (2021). Regulation of tau internalization, degradation, and seeding by LRP1 reveals multiple pathways for tau catabolism. J. Biol. Chem. 296. doi: 10.1016/j.jbc.2021.100715
Das, R., Balmik, A. A., and Chinnathambi, S. (2020). Phagocytosis of full-length Tau oligomers by Actin-remodeling of activated microglia. J. Neuroinflammation 17, 1–15.
Davis, D. M., and Sowinski, S. (2008). Membrane nanotubes: dynamic long-distance connections between animal cells. Nat. Rev. Mol. Cell Biol. 9, 431–436.
de Calignon, A., Polydoro, M., Suarez-Calvet, M., William, C., Adamowicz, D. H., Kopeikina, K. J., et al. (2012). Propagation of tau pathology in a model of early Alzheimer’s disease. Neuron 73, 685–697. doi: 10.1016/j.neuron.2011.11.033
DeLeo, A. M., and Ikezu, T. (2018). Extracellular vesicle biology in Alzheimer’s disease and related tauopathy. J. Neuroimmune Pharmacol. 13, 292–308.
Dikic, I., and Elazar, Z. (2018). Mechanism and medical implications of mammalian autophagy. Nat. Rev. Mol. Cell Biol. 19, 349–364.
Doeuvre, L., Plawinski, L., Toti, F., and Anglés-Cano, E. (2009). Cell-derived microparticles: a new challenge in neuroscience. J. Neurochem. 110, 457–468.
Doherty, G. J., and McMahon, H. T. (2009). Mechanisms of endocytosis. Annu. Rev. Biochem. 78, 857–902.
Donaldson, J. G. (2019). Macropinosome formation, maturation and membrane recycling: lessons from clathrin-independent endosomal membrane systems. Philos. Trans. R. Soc. B 374:20180148. doi: 10.1098/rstb.2018.0148
Dujardin, S., Bégard, S., Caillierez, R., Lachaud, C., Delattre, L., Carrier, S., et al. (2014). Ectosomes: a new mechanism for non-exosomal secretion of tau protein. PloS one 9:e100760. doi: 10.1371/journal.pone.0100760
Evans, L. D., Wassmer, T., Fraser, G., Smith, J., Perkinton, M., Billinton, A., et al. (2018). Extracellular monomeric and aggregated tau efficiently enter human neurons through overlapping but distinct pathways. Cell Rep. 22, 3612–3624. doi: 10.1016/j.celrep.2018.03.021
Fá, M., Puzzo, D., Piacentini, R., Staniszewski, A., Zhang, H., Baltrons, M. A., et al. (2016). Extracellular tau oligomers produce an immediate impairment of LTP and memory. Sci. Rep. 6:19393. doi: 10.1038/srep19393
Fagan, A. M., Roe, C. M., Xiong, C., Mintun, M. A., Morris, J. C., and Holtzman, D. M. (2007). Cerebrospinal fluid tau/β-amyloid42 ratio as a prediction of cognitive decline in nondemented older adults. Arch. Neurol. 64, 343–349.
Farfel-Becker, T., Roney, J. C., Cheng, X.-T., Li, S., Cuddy, S. R., and Sheng, Z.-H. (2019). Neuronal soma-derived degradative lysosomes are continuously delivered to distal axons to maintain local degradation capacity. Cell Rep. 28, 51–64.e4. doi: 10.1016/j.celrep.2019.06.013
Ferrer, I., Garcia, M. A., Gonzalez, I. L., Lucena, D. D., Villalonga, A. R., Tech, M. C., et al. (2018). Aging-related tau astrogliopathy (ARTAG): not only tau phosphorylation in astrocytes. Brain Pathol. 28, 965–985.
Fiandaca, M. S., Kapogiannis, D., Mapstone, M., Boxer, A., Eitan, E., Schwartz, J. B., et al. (2015). Identification of preclinical Alzheimer’s disease by a profile of pathogenic proteins in neurally derived blood exosomes: a case-control study. Alzheimers Dement. 11, 600–607.e1. doi: 10.1016/j.jalz.2014.06.008
Finneran, D. J., and Nash, K. R. (2019). Neuroinflammation and fractalkine signaling in Alzheimer’s disease. J. Neuroinflammation 16:11.
Fischer, D., Mukrasch, M. D., Biernat, J., Bibow, S., Blackledge, M., Griesinger, C., et al. (2009). Conformational changes specific for pseudophosphorylation at serine 262 selectively impair binding of tau to microtubules. Biochemistry 48, 10047–10055. doi: 10.1021/bi901090m
Fontaine, S. N., Zheng, D., Sabbagh, J. J., Martin, M. D., Chaput, D., Darling, A., et al. (2016). DnaJ/Hsc70 chaperone complexes control the extracellular release of neurodegenerative-associated proteins. EMBO J. 35, 1537–1549. doi: 10.15252/embj.201593489
Fricker, M., Tolkovsky, A. M., Borutaite, V., Coleman, M., and Brown, G. C. (2018). Neuronal cell death. Physiol. Rev. 98, 813–880.
Friedhoff, P., Von Bergen, M., Mandelkow, E.-M., and Mandelkow, E. (2000). Structure of tau protein and assembly into paired helical filaments. Biochim. Biophys. Acta 1502, 122–132.
Gerson, J., Castillo-Carranza, D. L., Sengupta, U., Bodani, R., Prough, D. S., Dewitt, D. S., et al. (2016a). Tau oligomers derived from traumatic brain injury cause cognitive impairment and accelerate onset of pathology in Htau mice. J. Neurotrauma 33, 2034–2043. doi: 10.1089/neu.2015.4262
Gerson, J. E., Mudher, A., and Kayed, R. (2016b). Potential mechanisms and implications for the formation of tau oligomeric strains. Crit. Rev. Biochem. Mol. Biol. 51, 482–496. doi: 10.1080/10409238.2016.1226251
Gibbons, G. S., Lee, V. M., and Trojanowski, J. Q. (2019). Mechanisms of cell-to-cell transmission of pathological tau: a review. JAMA Neurol. 76, 101–108. doi: 10.1001/jamaneurol.2018.2505
Goedert, M. (2016). The ordered assembly of tau is the gain-of-toxic function that causes human tauopathies. Alzheimers Dement. 12, 1040–1050.
Gomez-Ramos, A., Diaz-Hernandez, M., Cuadros, R., Hernandez, F., and Avila, J. (2006). Extracellular tau is toxic to neuronal cells. FEBS Lett. 580, 4842–4850.
Gómez-Ramos, A., Díaz-Hernández, M., Rubio, A., Díaz-Hernández, J. I., Miras-Portugal, M. T., and Avila, J. (2009). Characteristics and consequences of muscarinic receptor activation by tau protein. Eur. Neuropsychopharmacol. 19, 708–717. doi: 10.1016/j.euroneuro.2009.04.006
Gomez-Ramos, A., Diaz-Hernandez, M., Rubio, A., Miras-Portugal, M., and Avila, J. (2008). Extracellular tau promotes intracellular calcium increase through M1 and M3 muscarinic receptors in neuronal cells. Mol. Cell. Neurosci. 37, 673–681.
Gong, C.-X., Liu, F., Grundke-Iqbal, I., and Iqbal, K. (2005). Post-translational modifications of tau protein in Alzheimer’s disease. J. Neural Transm. 112, 813–838.
Gousset, K., Schiff, E., Langevin, C., Marijanovic, Z., Caputo, A., Browman, D. T., et al. (2009). Prions hijack tunnelling nanotubes for intercellular spread. Nat. Cell Biol. 11, 328–336. doi: 10.1038/ncb1841
Guo, J.-P., Arai, T., Miklossy, J., and Mcgeer, P. L. (2006). Aβ and tau form soluble complexes that may promote self aggregation of both into the insoluble forms observed in Alzheimer’s disease. Proc. Natl. Acad. Sci. U.S.A. 103, 1953–1958. doi: 10.1073/pnas.0509386103
Haense, C., Buerger, K., Kalbe, E., Drzezga, A., Teipel, S., Markiewicz, P., et al. (2008). CSF total and phosphorylated tau protein, regional glucose metabolism and dementia severity in Alzheimer’s disease. Eur. J. Neurol. 15, 1155–1162.
Haque, M., Lim, S., Kim, D., Kim, D. J., and Kim, Y. K. (2016). “Intracellular tau modifications and cell-based sensors for monitoring tau aggregation,” in Protein Purification: Principles and Trends, ed. iConcept Press (Hong Kong: iConcept Press).
Harada, A., Oguchi, K., Okabe, S., Kuno, J., Terada, S., Ohshima, T., et al. (1994). Altered microtubule organization in small-calibre axons of mice lacking tau protein. Nature 369, 488–491. doi: 10.1038/369488a0
Hatori, K., Nagai, A., Heisel, R., Ryu, J. K., and Kim, S. U. (2002). Fractalkine and fractalkine receptors in human neurons and glial cells. J. Neurosci. Res. 69, 418–426.
Holler, C. J., Davis, P. R., Beckett, T. L., Platt, T. L., Webb, R. L., Head, E., et al. (2014). Bridging integrator 1 (BIN1) protein expression increases in the Alzheimer’s disease brain and correlates with neurofibrillary tangle pathology. J. Alzheimers Dis. 42, 1221–1227.
Holmes, B. B., Devos, S. L., Kfoury, N., Li, M., Jacks, R., Yanamandra, K., et al. (2013). Heparan sulfate proteoglycans mediate internalization and propagation of specific proteopathic seeds. Proc. Natl. Acad. Sci. U.S.A. 110, E3138–E3147. doi: 10.1073/pnas.1301440110
Hopp, S. C., Lin, Y., Oakley, D., Roe, A. D., Devos, S. L., Hanlon, D., et al. (2018). The role of microglia in processing and spreading of bioactive tau seeds in Alzheimer’s disease. J. Neuroinflammation 15:269. doi: 10.1186/s12974-018-1309-z
Ikegami, S., Harada, A., and Hirokawa, N. (2000). Muscle weakness, hyperactivity, and impairment in fear conditioning in tau-deficient mice. Neurosci. Lett. 279, 129–132. doi: 10.1016/s0304-3940(99)00964-7
Ikezu, T., Ruan, Z., Pathak, D., Muraoka, S., Deleo, A. M., Kayed, R., et al. (2020). Elucidating the pathogenic mechanisms of AD brain-derived, tau-containing extracellular vesicles: Highly transmissible and preferential propagation to GABAergic neurons: EV or not EV? Extracellular propagation of AD pathogenic molecules and evaluation of brain cell-derived extracellular vesicles from patient plasma as EV biomarkers. Alzheimers Dement. 16:e037316.
Iliff, J. J., Wang, M., Liao, Y., Plogg, B. A., Peng, W., Gundersen, G. A., et al. (2012). A paravascular pathway facilitates CSF flow through the brain parenchyma and the clearance of interstitial solutes, including amyloid β. Sci. Transl. Med. 4:147ra111. doi: 10.1126/scitranslmed.3003748
Iqbal, K., Liu, F., Gong, C.-X., and Grundke-Iqbal, I. (2010). Tau in Alzheimer disease and related tauopathies. Curr. Alzheimer Res. 7, 656–664.
Iwata, M., Watanabe, S., Yamane, A., Miyasaka, T., and Misonou, H. (2019). Regulatory mechanisms for the axonal localization of tau protein in neurons. Mol. Biol. Cell 30, 2441–2457.
Jho, Y., Zhulina, E., Kim, M.-W., and Pincus, P. (2010). Monte carlo simulations of tau proteins: effect of phosphorylation. Biophys. J. 99, 2387–2397.
Jia, L., Qiu, Q., Zhang, H., Chu, L., Du, Y., Zhang, J., et al. (2019). Concordance between the assessment of Aβ42, T-tau, and P-T181-tau in peripheral blood neuronal-derived exosomes and cerebrospinal fluid. Alzheimers Dement. 15, 1071–1080. doi: 10.1016/j.jalz.2019.05.002
Jung, D., Filliol, D., Miehe, M., and Rendon, A. (1993). Interaction of brain mitochondria with microtubules reconstituted from brain tubulin and MAP2 or TAU. Cell Motil. Cytoskeleton 24, 245–255.
Jung, Y.-J., and Chung, W.-S. (2018). Phagocytic roles of glial cells in healthy and diseased brains. Biomol. Ther. 26, 350–357.
Kandimalla, R. J., Prabhakar, S., Wani, W. Y., Kaushal, A., Gupta, N., Sharma, D. R., et al. (2013). CSF p-Tau levels in the prediction of Alzheimer’s disease. Biol. Open 2, 1119–1124.
Kang, S., Son, S. M., Baik, S. H., Yang, J., and Mook-Jung, I. (2019). Autophagy-mediated secretory pathway is responsible for both normal and pathological tau in neurons. J. Alzheimers Dis. 70, 667–680. doi: 10.3233/JAD-190180
Katsinelos, T., Zeitler, M., Dimou, E., Karakatsani, A., Muller, H. M., Nachman, E., et al. (2018). Unconventional secretion mediates the trans-cellular spreading of Tau. Cell Rep. 23, 2039–2055. doi: 10.1016/j.celrep.2018.04.056
Kim, D., Lim, S., Haque, M. M., Ryoo, N., Hong, H. S., Rhim, H., et al. (2015). Identification of disulfide cross-linked tau dimer responsible for tau propagation. Sci. Rep. 5:15231. doi: 10.1038/srep15231
Kimura, T., Jia, J., Claude-Taupin, A., Kumar, S., Choi, S. W., Gu, Y., et al. (2017a). Cellular and molecular mechanism for secretory autophagy. Autophagy 13, 1084–1085.
Kimura, T., Jia, J., Kumar, S., Choi, S. W., Gu, Y., Mudd, M., et al. (2017b). Dedicated SNARE s and specialized TRIM cargo receptors mediate secretory autophagy. EMBO J. 36, 42–60. doi: 10.15252/embj.201695081
Kimura, T., Whitcomb, D. J., Jo, J., Regan, P., Piers, T., Heo, S., et al. (2014). Microtubule-associated protein tau is essential for long-term depression in the hippocampus. Philos. Trans. R. Soc. B Biol. Sci. 369:20130144.
Kinney, J. W., Bemiller, S. M., Murtishaw, A. S., Leisgang, A. M., Salazar, A. M., and Lamb, B. T. (2018). Inflammation as a central mechanism in Alzheimer’s disease. Alzheimers Dement. 4, 575–590.
Kofler, J., and Wiley, C. A. (2011). Microglia: key innate immune cells of the brain. Toxicol. Pathol. 39, 103–114.
Krüger, U., Wang, Y., Kumar, S., and Mandelkow, E.-M. (2012). Autophagic degradation of tau in primary neurons and its enhancement by trehalose. Neurobiol. Aging 33, 2291–2305. doi: 10.1016/j.neurobiolaging.2011.11.009
Lane-Donovan, C., Philips, G. T., and Herz, J. (2014). More than cholesterol transporters: lipoprotein receptors in CNS function and neurodegeneration. Neuron 83, 771–787.
Lasagna-Reeves, C. A., Castillo-Carranza, D. L., Sengupta, U., Clos, A. L., Jackson, G. R., and Kayed, R. (2011). Tau oligomers impair memory and induce synaptic and mitochondrial dysfunction in wild-type mice. Mol. Neurodegener. 6:39. doi: 10.1186/1750-1326-6-39
Lasagna-Reeves, C. A., Castillo-Carranza, D. L., Sengupta, U., Guerrero-Munoz, M. J., Kiritoshi, T., Neugebauer, V., et al. (2012). Alzheimer brain-derived tau oligomers propagate pathology from endogenous tau. Sci. Rep. 2:700.
Lasagna-Reeves, C. A., Sengupta, U., Castillo-Carranza, D., Gerson, J. E., Guerrero-Munoz, M., Troncoso, J. C., et al. (2014). The formation of tau pore-like structures is prevalent and cell specific: possible implications for the disease phenotypes. Acta Neuropathol. Commun. 2:56. doi: 10.1186/2051-5960-2-56
Lee, J., and Ye, Y. (2018). The roles of endo-lysosomes in unconventional protein secretion. Cells 7:198.
Lei, P., Ayton, S., Finkelstein, D. I., Spoerri, L., Ciccotosto, G. D., Wright, D. K., et al. (2012). Tau deficiency induces parkinsonism with dementia by impairing APP-mediated iron export. Nat. Med. 18, 291–295. doi: 10.1038/nm.2613
Lonati, E., Sala, G., Tresoldi, V., Coco, S., Salerno, D., Milani, C., et al. (2018). Ischemic conditions affect rerouting of tau protein levels: evidences for alteration in tau processing and secretion in hippocampal neurons. J. Mol. Neurosci. 66, 604–616. doi: 10.1007/s12031-018-1199-7
Manassero, G., Guglielmotto, M., Monteleone, D., Vasciaveo, V., Butenko, O., Tamagno, E., et al. (2017). Dual mechanism of toxicity for extracellular injection of tau oligomers versus monomers in human tau mice. J. Alzheimers Dis. 59, 743–751. doi: 10.3233/JAD-170298
Martin, L., Latypova, X., and Terro, F. (2011). Post-translational modifications of tau protein: implications for Alzheimer’s disease. Neurochem. Int. 58, 458–471.
Martini-Stoica, H., Cole, A. L., Swartzlander, D. B., Chen, F., Wan, Y. W., Bajaj, L., et al. (2018). TFEB enhances astroglial uptake of extracellular tau species and reduces tau spreading. J. Exp. Med. 215, 2355–2377. doi: 10.1084/jem.20172158
McInnes, J., Wierda, K., Snellinx, A., Bounti, L., Wang, Y.-C., Stancu, I.-C., et al. (2018). Synaptogyrin-3 mediates presynaptic dysfunction induced by tau. Neuron 97, 823–835.e8. doi: 10.1016/j.neuron.2018.01.022
Merezhko, M., Brunello, C. A., Yan, X., Vihinen, H., Jokitalo, E., Uronen, R.-L., et al. (2018). Secretion of tau via an unconventional non-vesicular mechanism. Cell Rep. 25, 2027–2035.e4. doi: 10.1016/j.celrep.2018.10.078
Meyerholz, A., Hinrichsen, L., Groos, S., Esk, P. C., Brandes, G., and Ungewickell, E. J. (2005). Effect of clathrin assembly lymphoid myeloid leukemia protein depletion on clathrin coat formation. Traffic 6, 1225–1234. doi: 10.1111/j.1600-0854.2005.00355.x
Min, S.-W., Cho, S.-H., Zhou, Y., Schroeder, S., Haroutunian, V., Seeley, W. W., et al. (2010). Acetylation of tau inhibits its degradation and contributes to tauopathy. Neuron 67, 953–966.
Miyata, Y., Hoshi, M., Nishida, E., Minami, Y., and Sakai, H. (1986). Binding of microtubule-associated protein 2 and tau to the intermediate filament reassembled from neurofilament 70-kDa subunit protein. Its regulation by calmodulin. J. Biol. Chem. 261, 13026–13030.
Morishima-Kawashima, M., Hasegawa, M., Takio, K., Suzuki, M., Titani, K., and Ihara, Y. (1993). Ubiquitin is conjugated with amino-terminally processed tau in paired helical filaments. Neuron 10, 1151–1160. doi: 10.1016/0896-6273(93)90063-w
Morozova, V., Cohen, L. S., Makki, A. E.-H., Shur, A., Pilar, G., El Idrissi, A., et al. (2019). Normal and pathological tau uptake mediated by M1/M3 muscarinic receptors promotes opposite neuronal changes. Front. Cell. Neurosci. 13:403. doi: 10.3389/fncel.2019.00403
Orr, M. E., Sullivan, A. C., and Frost, B. (2017). A brief overview of tauopathy: causes, consequences, and therapeutic strategies. Trends Pharmacol. Sci. 38, 637–648. doi: 10.1016/j.tips.2017.03.011
Patel, N., Ramachandran, S., Azimov, R., Kagan, B. L., and Lal, R. (2015). Ion channel formation by tau protein: implications for Alzheimer’s disease and tauopathies. Biochemistry 54, 7320–7325.
Perea, J. R., Bolós, M., and Avila, J. (2020). Microglia in Alzheimer’s disease in the context of tau pathology. Biomolecules 10:1439.
Perea, J. R., López, E., Díez-Ballesteros, J. C., Ávila, J., Hernández, F., and Bolós, M. (2019). Extracellular monomeric tau is internalized by astrocytes. Front. Neurosci. 13:442. doi: 10.3389/fnins.2019.00442
Piacentini, R., Li Puma, D. D., Mainardi, M., Lazzarino, G., Tavazzi, B., Arancio, O., et al. (2017). Reduced gliotransmitter release from astrocytes mediates tau-induced synaptic dysfunction in cultured hippocampal neurons. Glia 65, 1302–1316. doi: 10.1002/glia.23163
Piccin, A., Murphy, W. G., and Smith, O. P. (2007). Circulating microparticles: pathophysiology and clinical implications. Blood Rev. 21, 157–171.
Polanco, J. C., Scicluna, B. J., Hill, A. F., and Götz, J. (2016). Extracellular vesicles isolated from the brains of rTg4510 mice seed tau protein aggregation in a threshold-dependent manner. J. Biol. Chem. 291, 12445–12466. doi: 10.1074/jbc.M115.709485
Pooler, A. M., Phillips, E. C., Lau, D. H., Noble, W., and Hanger, D. P. (2013). Physiological release of endogenous tau is stimulated by neuronal activity. EMBO Rep. 14, 389–394.
Prifti, E., Tsakiri, E. N., Vourkou, E., Stamatakis, G., Samiotaki, M., and Papanikolopoulou, K. (2021). The two cysteines of tau protein are functionally distinct and contribute differentially to its pathogenicity in vivo. J. Neurosci. 41, 797–810. doi: 10.1523/JNEUROSCI.1920-20.2020
Puzzo, D., Piacentini, R., Fa, M., Gulisano, W., Puma, D. D. L., Staniszewski, A., et al. (2017). LTP and memory impairment caused by extracellular Aβ and Tau oligomers is APP-dependent. eLife 6:e26991. doi: 10.7554/eLife.26991
Ramcharitar, J., Albrecht, S., Afonso, V. M., Kaushal, V., Bennett, D. A., and Leblanc, A. C. (2013). Cerebrospinal fluid tau cleaved by caspase-6 reflects brain levels and cognition in aging and Alzheimer disease. J. Neuropathol. Exp. Neurol. 72, 824–832. doi: 10.1097/NEN.0b013e3182a0a39f
Ransohoff, R. M. (2016). How neuroinflammation contributes to neurodegeneration. Science 353, 777–783.
Rasmussen, M. K., Mestre, H., and Nedergaard, M. (2018). The glymphatic pathway in neurological disorders. Lancet Neurol. 17, 1016–1024.
Rauch, J. N., Chen, J. J., Sorum, A. W., Miller, G. M., Sharf, T., See, S. K., et al. (2018). Tau Internalization is Regulated by 6-O Sulfation on Heparan Sulfate Proteoglycans (HSPGs). Sci. Rep. 8:6382. doi: 10.1038/s41598-018-24904-z
Rauch, J. N., Luna, G., Guzman, E., Audouard, M., Challis, C., Sibih, Y. E., et al. (2020). LRP1 is a master regulator of tau uptake and spread. Nature 580, 381–385.
Robert, G., Jacquel, A., and Auberger, P. (2019). Chaperone-Mediated autophagy and its emerging role in hematological malignancies. Cells 8:1260. doi: 10.3390/cells8101260
Rodríguez-Martín, T., Cuchillo-Ibáñez, I., Noble, W., Nyenya, F., Anderton, B. H., and Hanger, D. P. (2013). Tau phosphorylation affects its axonal transport and degradation. Neurobiol. Aging 34, 2146–2157.
Rustom, A., Saffrich, R., Markovic, I., Walther, P., and Gerdes, H.-H. (2004). Nanotubular highways for intercellular organelle transport. Science 303, 1007–1010. doi: 10.1126/science.1093133
Saman, S., Kim, W., Raya, M., Visnick, Y., Miro, S., Saman, S., et al. (2012). Exosome-associated tau is secreted in tauopathy models and is selectively phosphorylated in cerebrospinal fluid in early Alzheimer disease. J. Biol. Chem. 287, 3842–3849. doi: 10.1074/jbc.M111.277061
Schmidt, M., and Finley, D. (2014). Regulation of proteasome activity in health and disease. Biochim. Biophys. Acta 1843, 13–25.
Sengupta, U., Portelius, E., Hansson, O., Farmer, K., Castillo-Carranza, D., Woltjer, R., et al. (2017). Tau oligomers in cerebrospinal fluid in Alzheimer’s disease. Ann. Clin. Transl. Neurol. 4, 226–235.
Shafiei, S. S., Guerrero-Muñoz, M. J., and Castillo-Carranza, D. L. (2017). Tau oligomers: cytotoxicity, propagation, and mitochondrial damage. Front. Aging Neurosci. 9:83. doi: 10.3389/fnagi.2017.00083
Simon, D., Garcia-Garcia, E., Gomez-Ramos, A., Falcon-Perez, J. M., Diaz-Hernandez, M., Hernandez, F., et al. (2012). Tau overexpression results in its secretion via membrane vesicles. Neurodegener. Dis. 10, 73–75.
Sofroniew, M. V., and Vinters, H. V. (2010). Astrocytes: biology and pathology. Acta Neuropathol. 119, 7–35.
Spitzer, P., Mulzer, L.-M., Oberstein, T. J., Munoz, L. E., Lewczuk, P., Kornhuber, J., et al. (2019). Microvesicles from cerebrospinal fluid of patients with Alzheimer’s disease display reduced concentrations of tau and APP protein. Sci. Rep. 9:7089. doi: 10.1038/s41598-019-43607-7
Swanson, E., Breckenridge, L., Mcmahon, L., Som, S., Mcconnell, I., and Bloom, G. S. (2017). Extracellular tau oligomers induce invasion of endogenous tau into the somatodendritic compartment and axonal transport dysfunction. J. Alzheimers Dis. 58, 803–820. doi: 10.3233/JAD-170168
Swanson, J. A. (2008). Shaping cups into phagosomes and macropinosomes. Nat. Rev. Mol. Cell Biol. 9, 639–649. doi: 10.1038/nrm2447
Takahashi, M., Miyata, H., Kametani, F., Nonaka, T., Akiyama, H., Hisanaga, S.-I., et al. (2015). Extracellular association of APP and tau fibrils induces intracellular aggregate formation of tau. Acta Neuropathol. 129, 895–907.
Takeda, S., Wegmann, S., Cho, H., Devos, S. L., Commins, C., Roe, A. D., et al. (2015). Neuronal uptake and propagation of a rare phosphorylated high-molecular-weight tau derived from Alzheimer’s disease brain. Nat. Commun. 6:8490. doi: 10.1038/ncomms9490
Takei, Y., Teng, J., Harada, A., and Hirokawa, N. (2000). Defects in axonal elongation and neuronal migration in mice with disrupted tau and map1b genes. J. Cell Biol. 150, 989–1000. doi: 10.1083/jcb.150.5.989
Tang, Z., Ioja, E., Bereczki, E., Hultenby, K., Li, C., Guan, Z., et al. (2015). mTor mediates tau localization and secretion: implication for Alzheimer’s disease. Biochim. Biophys. Acta 1853, 1646–1657.
Tardivel, M., Begard, S., Bousset, L., Dujardin, S., Coens, A., Melki, R., et al. (2016). Tunneling nanotube (TNT)-mediated neuron-to neuron transfer of pathological Tau protein assemblies. Acta Neuropathol. Commun. 4:117. doi: 10.1186/s40478-016-0386-4
Tint, I., Slaughter, T., Fischer, I., and Black, M. M. (1998). Acute inactivation of tau has no effect on dynamics of microtubules in growing axons of cultured sympathetic neurons. J. Neurosci. 18, 8660–8673. doi: 10.1523/JNEUROSCI.18-21-08660.1998
Usenovic, M., Niroomand, S., Drolet, R. E., Yao, L., Gaspar, R. C., Hatcher, N. G., et al. (2015). Internalized tau oligomers cause neurodegeneration by inducing accumulation of pathogenic tau in human neurons derived from induced pluripotent stem cells. J. Neurosci. 35, 14234–14250. doi: 10.1523/JNEUROSCI.1523-15.2015
Uytterhoeven, V., Deaulmerie, L., and Verstreken, P. (2018). Increased endosomal microautophagy reduces Tau driven synaptic dysfunction. Front. Neurosci 12:10.3389.
Velazquez, R., Ferreira, E., Tran, A., Turner, E. C., Belfiore, R., Branca, C., et al. (2018). Acute tau knockdown in the hippocampus of adult mice causes learning and memory deficits. Aging Cell 17:e12775. doi: 10.1111/acel.12775
Vergara, C., Houben, S., Suain, V., Yilmaz, Z., De Decker, R., Vanden Dries, V., et al. (2019). Amyloid-beta pathology enhances pathological fibrillary tau seeding induced by Alzheimer PHF in vivo. Acta Neuropathol. 137, 397–412. doi: 10.1007/s00401-018-1953-5
Vogel, J. W., Iturria-Medina, Y., Strandberg, O. T., Smith, R., Levitis, E., Evans, A. C., et al. (2020). Spread of pathological tau proteins through communicating neurons in human Alzheimer’s disease. Nat. Commun. 11:2612.
Wang, Y., Balaji, V., Kaniyappan, S., Krüger, L., Irsen, S., Tepper, K., et al. (2017). The release and trans-synaptic transmission of Tau via exosomes. Mol. Neurodegener. 12:5. doi: 10.1186/s13024-016-0143-y
Wang, Y., Li, L., Hou, C., Lai, Y., Long, J., Liu, J., et al. (2016). “SNARE-mediated membrane fusion in autophagy. Semin. Cell Dev. Biol. 60, 97–104.
Wang, Y., Martinez-Vicente, M., Krüger, U., Kaushik, S., Wong, E., Mandelkow, E.-M., et al. (2009). Tau fragmentation, aggregation and clearance: the dual role of lysosomal processing. Hum. Mol. Genet. 18, 4153–4170. doi: 10.1093/hmg/ddp367
Wattmo, C., Blennow, K., and Hansson, O. (2020). Cerebro-spinal fluid biomarker levels: phosphorylated tau (T) and total tau (N) as markers for rate of progression in Alzheimer’s disease. BMC Neurol. 20:10. doi: 10.1186/s12883-019-1591-0
Welton, J. L., Loveless, S., Stone, T., Von Ruhland, C., Robertson, N. P., and Clayton, A. (2017). Cerebrospinal fluid extracellular vesicle enrichment for protein biomarker discovery in neurological disease; multiple sclerosis. J. Extracell. Vesicles 6:1369805. doi: 10.1080/20013078.2017.1369805
Williams, D. R., Holton, J. L., Strand, C., Pittman, A., De Silva, R., Lees, A. J., et al. (2007). Pathological tau burden and distribution distinguishes progressive supranuclear palsy-parkinsonism from Richardson’s syndrome. Brain 130, 1566–1576. doi: 10.1093/brain/awm104
Winston, C. N., Goetzl, E. J., Akers, J. C., Carter, B. S., Rockenstein, E. M., Galasko, D., et al. (2016). Prediction of conversion from mild cognitive impairment to dementia with neuronally derived blood exosome protein profile. Alzheimers Dement. 3, 63–72. doi: 10.1016/j.dadm.2016.04.001
Wong, E., and Cuervo, A. M. (2010). Integration of clearance mechanisms: the proteasome and autophagy. Cold Spring Harb. Perspecti. Biol. 2:a006734.
Wu, J. W., Herman, M., Liu, L., Simoes, S., Acker, C. M., Figueroa, H., et al. (2013). Small misfolded Tau species are internalized via bulk endocytosis and anterogradely and retrogradely transported in neurons. J. Biol. Chem. 288, 1856–1870. doi: 10.1074/jbc.M112.394528
Wu, J. W., Hussaini, S. A., Bastille, I. M., Rodriguez, G. A., Mrejeru, A., Rilett, K., et al. (2016). Neuronal activity enhances tau propagation and tau pathology in vivo. Nat. Neurosci. 19, 1085–1092. doi: 10.1038/nn.4328
Wu, M., Ouyang, Y., Wang, Z., Zhang, R., Huang, P.-H., Chen, C., et al. (2017). Isolation of exosomes from whole blood by integrating acoustics and microfluidics. Proc. Natl. Acad. Sci. U.S.A. 114, 10584–10589. doi: 10.1073/pnas.1709210114
Xu, D., and Esko, J. D. (2014). Demystifying heparan sulfate-protein interactions. Annu. Rev. Biochem. 83, 129–157. doi: 10.1146/annurev-biochem-060713-035314
Yamada, K., Holth, J. K., Liao, F., Stewart, F. R., Mahan, T. E., Jiang, H., et al. (2014). Neuronal activity regulates extracellular tau in vivo. J. Exp. Med. 211, 387–393.
Yáñez-Mó, M., Siljander, P. R.-M., Andreu, Z., Bedina Zavec, A., Borràs, F. E., Buzas, E. I., et al. (2015). Biological properties of extracellular vesicles and their physiological functions. J. Extracell. Vesicles 4:27066.
Zempel, H., Dennissen, F. J., Kumar, Y., Luedtke, J., Biernat, J., Mandelkow, E.-M., et al. (2017). Axodendritic sorting and pathological missorting of Tau are isoform-specific and determined by axon initial segment architecture. J. Biol. Chem. 292, 12192–12207. doi: 10.1074/jbc.M117.784702
Zhang, F., and Jiang, L. (2015). Neuroinflammation in Alzheimer’s disease. Neuropsychiatr. Dis. Treat. 11, 243–256.
Zhang, Z., Song, M., Liu, X., Kang, S. S., Kwon, I.-S., Duong, D. M., et al. (2014). Cleavage of tau by asparagine endopeptidase mediates the neurofibrillary pathology in Alzheimer’s disease. Nat. Med. 20, 1254–1262.
Keywords: tau, tau clearance, tau transmission, tauopathy, pathological tau
Citation: Seitkazina A, Kim KH, Fagan E, Sung Y, Kim YK and Lim S (2022) The Fate of Tau Aggregates Between Clearance and Transmission. Front. Aging Neurosci. 14:932541. doi: 10.3389/fnagi.2022.932541
Received: 29 April 2022; Accepted: 22 June 2022;
Published: 18 July 2022.
Edited by:
Jia Liu, Capital Medical University, ChinaReviewed by:
Simon Dujardin, Massachusetts General Hospital and Harvard Medical School, United StatesDavid R. Borchelt, University of Florida, United States
Copyright © 2022 Seitkazina, Kim, Fagan, Sung, Kim and Lim. This is an open-access article distributed under the terms of the Creative Commons Attribution License (CC BY). The use, distribution or reproduction in other forums is permitted, provided the original author(s) and the copyright owner(s) are credited and that the original publication in this journal is cited, in accordance with accepted academic practice. No use, distribution or reproduction is permitted which does not comply with these terms.
*Correspondence: Yun Kyung Kim, yunkyungkim@kist.re.kr; Sungsu Lim, sungsulim@kist.re.kr
†These authors have contributed equally to this work