- 1Manchester Centre for Audiology and Deafness, University of Manchester, Manchester, United Kingdom
- 2Department of Audiology and Speech Therapy, Birzeit University, Birzeit, Palestine
- 3Department of Psychology, Lancaster University, Lancaster, United Kingdom
Animal studies have shown that noise exposure and aging cause a reduction in the number of synapses between low and medium spontaneous rate auditory nerve fibers and inner hair cells before outer hair cell deterioration. This noise-induced and age-related cochlear synaptopathy (CS) is hypothesized to compromise speech recognition at moderate-to-high suprathreshold levels in humans. This paper evaluates the evidence on the relative and combined effects of noise exposure and aging on CS, in both animals and humans, using histopathological and proxy measures. In animal studies, noise exposure seems to result in a higher proportion of CS (up to 70% synapse loss) compared to aging (up to 48% synapse loss). Following noise exposure, older animals, depending on their species, seem to either exhibit significant or little further synapse loss compared to their younger counterparts. In humans, temporal bone studies suggest a possible age- and noise-related auditory nerve fiber loss. Based on the animal data obtained from different species, we predict that noise exposure may accelerate age-related CS to at least some extent in humans. In animals, noise-induced and age-related CS in separation have been consistently associated with a decreased amplitude of wave 1 of the auditory brainstem response, reduced middle ear muscle reflex strength, and degraded temporal processing as demonstrated by lower amplitudes of the envelope following response. In humans, the individual effects of noise exposure and aging do not seem to translate clearly into deficits in electrophysiological, middle ear muscle reflex, and behavioral measures of CS. Moreover, the evidence on the combined effects of noise exposure and aging on peripheral neural deafferentation in humans using electrophysiological and behavioral measures is even more sparse and inconclusive. Further research is necessary to establish the individual and combined effects of CS in humans using temporal bone, objective, and behavioral measures.
Introduction
Noise exposure during work and/or leisure activities is associated with a range of disorders including noise-induced hearing loss (NIHL), tinnitus, hyperacusis, temporary threshold shift, compromised sleep, increased stress, and hypertension (Concha-Barrientos et al., 2004; Nelson et al., 2005). The effect of aging on the human auditory system is often described as presbycusis or age-related hearing loss (ARHL); (Huang and Tang, 2010). In ARHL, peripheral and central auditory deterioration takes place which results in a wide variety of auditory symptoms including high-frequency sensorineural hearing loss, impaired sound localization, speech-perception-in-noise (SPiN) difficulties, poor central auditory processing, and impaired temporal processing (Mazelova et al., 2003; Gates and Mills, 2005; Jayakody et al., 2018). Although there is no agreement on a single etiology of ARHL, factors such as genetic predisposition, cumulative lifetime noise exposure, intake of ototoxic medications, and past auditory pathologies may be potential underlying causes (Gates and Mills, 2005; Dubno et al., 2013).
Excessive noise exposure and aging are both associated with major damage to cochlear outer hair cells (OHCs) and their stereocilia, with a lesser impact on inner hair cells (IHCs) (Wang et al., 2002; Gates and Mills, 2005; Popelar et al., 2006; Sergeyenko et al., 2013; Jayakody et al., 2018; Wu et al., 2021). This cochlear hair cell loss often results in a deterioration in hearing sensitivity, loss in frequency selectivity, and worse temporal precision of neural coding (Schuknecht and Gacek, 1993; Ashmore et al., 2010; Salvi et al., 2017). Moreover, atrophy of the cochlear stria vascularis was shown to occur as part of ARHL (Gates and Mills, 2005; Popelar et al., 2006).
In all studied rodent and non-human primate animal species, the synapses between IHCs and afferent auditory nerve fibers (ANFs) degenerate, due to both acoustic over-exposure and aging, before OHCs and IHCs are lost (Kujawa and Liberman, 2015; Valero et al., 2017). This cochlear synaptopathy (CS) has been shown to result in degraded neural temporal processing (Parthasarathy and Kujawa, 2018). Following the loss of cochlear synapses, primary deterioration of afferent ANFs and their spiral ganglion cells (SGCs) occurs (for a review, see Kujawa and Liberman, 2015). Some animal evidence suggests that the majority of lost ANFs are low- to medium spontaneous rate (SR) high-threshold fibers (Schmiedt et al., 1996; Furman et al., 2013), which, in humans, are thought to code moderate-to-high-level sounds, such as speech (Bharadwaj et al., 2014; Kujawa and Liberman, 2015; Huet et al., 2016). However, recent findings by Suthakar and Liberman (2021) have shown that a substantial proportion of high-SR ANFs were lost alongside low-SR ANFs in CBA/CaJ mouse following exposure to intense noise.
The extent to which lifetime noise exposure exacerbates age-related hearing difficulties has been under debate for decades and is generally poorly understood (Shone et al., 1991; Kujawa and Liberman, 2006, 2015; Ciorba et al., 2011). The majority of animal and human research has focused on how each factor separately affects cochlear hair cells and hearing thresholds, with several studies providing evidence that noise exposure may accelerate age-related threshold loss when both factors combine (Shone et al., 1991; Gates and Mills, 2005; Kujawa and Liberman, 2006; Ciorba et al., 2011; Alvarado et al., 2019; Wu et al., 2021; Fetoni et al., 2022).
Recently, consistent research efforts have been made to better understand noise-induced and age-related CS in separation using non-invasive auditory proxy measures. Animal studies have shown a clear relation between noise-induced and age-related synapse loss (occurring in separation) and objective proxy measures such as the amplitude of wave 1 of the auditory brainstem response (ABR) (Kujawa and Liberman, 2009), the middle ear muscle reflex (MEMR) threshold and amplitude (Valero et al., 2016, 2018), the envelope following response (EFR; Shaheen et al., 2015), and the ratio of the summating potential (SP) of the cochlear hair cells to the action potential (AP) of the auditory nerve (SP:AP ratio; Sergeyenko et al., 2013). A large number of human studies have investigated the effects of noise exposure and aging using objective proxy measures of CS, by employing different sample demographics, measurement techniques, and sample sizes. The findings of these studies were generally mixed and inconclusive, making it difficult to draw firm conclusions (Bramhall et al., 2017, 2019, 2021; Prendergast et al., 2017a, 2019; Valderrama et al., 2018; Carcagno and Plack, 2020, 2021; Fernandez et al., 2020).
In this narrative review paper, we will evaluate how noise exposure and aging affect peripheral auditory neural deafferentation independently using: (1) histopathological and neurophysiological; (2) electrophysiological; and (3) behavioral evidence from both animals and humans. For each type of evidence, we will discuss and compare the potential relative and combined effects between these two factors, noise exposure and aging, in relation to CS. All papers included in this review are peer-reviewed published journal articles.
Histopathological and Neurophysiological Aspects
In this section, the histopathological and neurophysiological aspects of noise exposure, aging, and the combined effects of noise exposure and aging, will be discussed in relation to CS in both animals and humans.
Histopathological and Neurophysiological Aspects: Noise Exposure
Animal Studies
Histopathological evidence from several animal species shows that acoustic over-exposure can result in significant CS in basal cochlear regions despite a near-complete recovery of hearing thresholds (Kujawa and Liberman, 2009, 2015; Lin et al., 2011; Furman et al., 2013; Maison et al., 2013; Jensen et al., 2015; Shaheen et al., 2015; Song et al., 2016; Valero et al., 2017; Hickman et al., 2018; Fernandez et al., 2020). Loss of ANFs and SGCs was noted to only be observable several months following the synapse loss in rodents (Kujawa and Liberman, 2015).
Table 1 shows a summary of key studies that investigated the proportion of synapse loss and ABR wave 1 amplitude reductions (which is a proxy measure of CS) related to noise exposure across different animal species, for which there were no permanent ABR threshold shifts. Studies suggest that different animal species exhibit variable susceptibility to noise-induced synapse loss. In these studies, the sound pressure level to which animals were exposed was selected such that it was intense enough to produce a temporary threshold shift but not result in permanent threshold elevation.
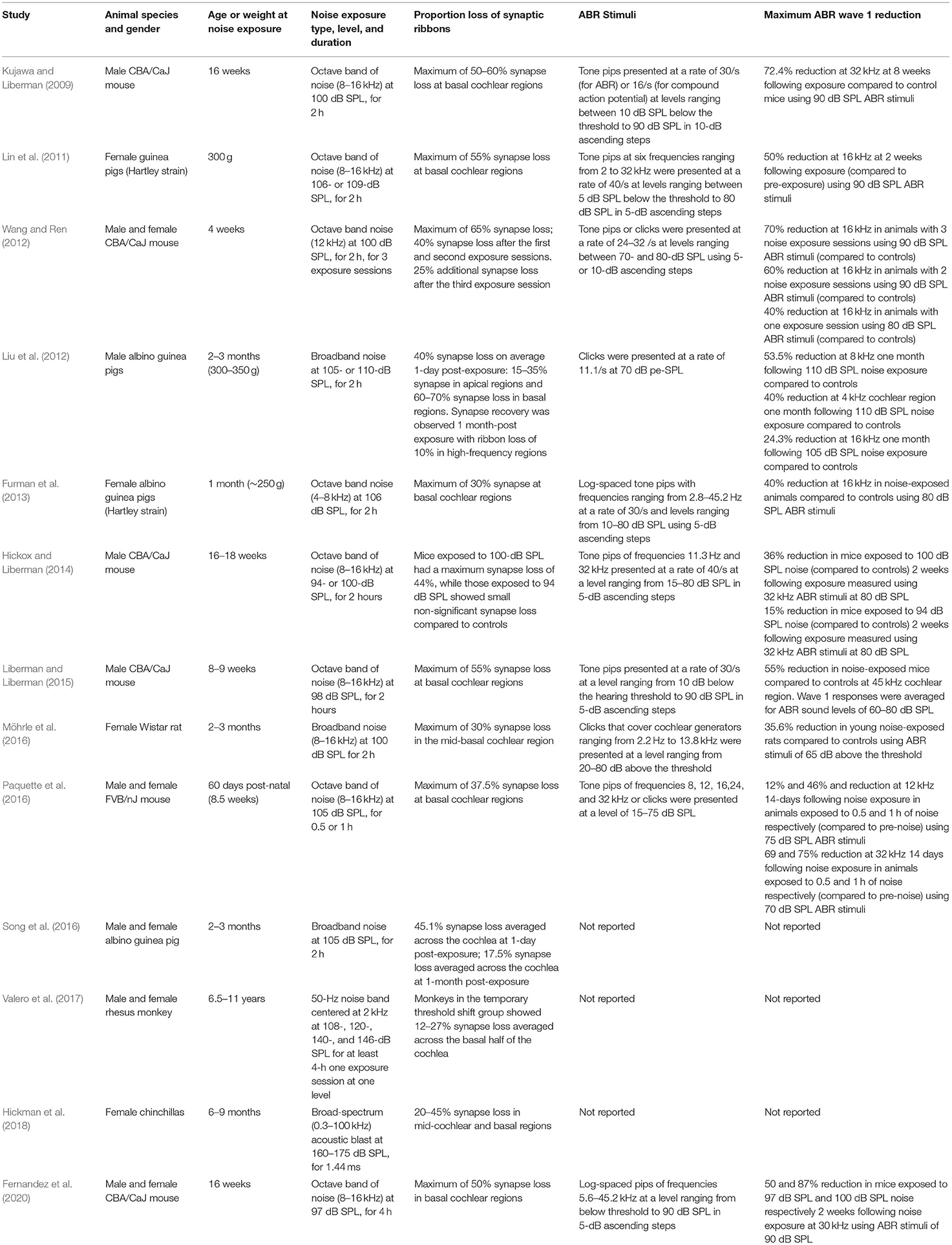
Table 1. Summary of key studies on the effect of noise exposure on synapse loss and ABR wave 1 amplitude across different animal species. Data reported were either explicitly mentioned in the manuscript text or were derived from the relevant figures in the respective publications using the online tool of WebPlotDigitizer version 4.5 (Rohatgi, 2021).
As shown in Table 1, acoustic-over exposure resulted in synapse loss ranging from 12 to 70% primarily in basal regions rather than across the entire cochlea in the absence of threshold elevation in different animal species. Although the majority of the animal literature summarized in Table 1 employed octave-band noise centered at high frequencies, with few of them using broadband and blast noise insults, the differences in synapse loss could be essentially explained by the fact that the different authors investigated different types of animal species. The left panel of Figure 1 shows a scatterplot of the proportion of the remaining synapses vs. the maximum noise exposure (standardized as noise intensity in dB of equivalent continuous sound level for 8 h) considered in each study in Table 1. The different numbers, shapes, and colors of the data points in the left panel of Figure 1 reflect the different animal species that were examined in the studies in Table 1.
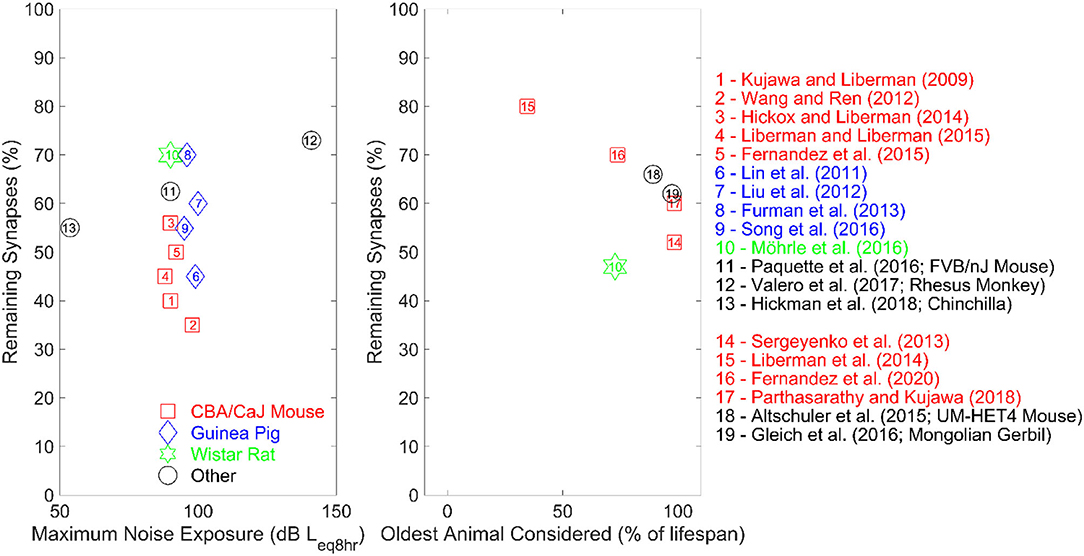
Figure 1. The left panel represents the proportion of remaining synapses as a function of the maximum average noise exposure of the studies summarized in Table 1. All studies exposed their subjects to octave-band noise, except for studies numbered 7, 10, and 13 employed broadband noise (study 13 only used blast noise). Studies number 2 and 12 involved multiple noise-exposure session, while all other studies exposed their subjects during one session only. The right panel shows the proportion of remaining synapses as a function of the age of the oldest animals in percent lifespan for the studies summarized in Table 2. The reference lifespan for the animals is 25 months for the Wistar rat, 36 months for the Mongolian gerbil and 30 months for both CBA and UMHET4 mouse.
As inferred from the left panel of Figure 1, even for very similar noise exposure levels and durations, a wide range of synaptopathic effects were reported across the different animal species. Although animal subjects used were genetically similar in each study (which minimizes inter-subject variability due to genetic makeup), different animal species seem to exhibit different physiologic susceptibility to noise-induced CS. Interestingly, rhesus monkeys, which are physiologically closer to humans than rodents, exhibited the lowest noise-induced synapse loss compared to rodent models, which may be helpful to infer the effect of acoustic over-exposure in humans (Valero et al., 2017). Furthermore, this synapse loss in rhesus monkeys was elicited at much higher intensities than those used in rodent studies (see Figure 1), which supports the hypothesis that rhesus monkeys are less susceptible to CS. Dobie and Humes (2017) suggest that humans may be less susceptible to temporary threshold shifts following acoustic overexposure compared to rodents. These findings support the hypothesized variability in auditory system susceptibility to noise damage across different species.
Single-unit recordings suggest that the majority of ANFs lost following CS as a result of acoustic over-exposure in guinea pigs are low- and medium-SR fibers (Furman et al., 2013; Bourien et al., 2014; Song et al., 2016) which are found to represent around 40% of type I ANFs in cats and guinea pigs (Liberman, 1978; Tsuji and Liberman, 1997). In CBA/CaJ mice, significant loss of both low- and high-SR ANFs was seen following intense noise exposure (Suthakar and Liberman, 2021). Low-SR ANFs are observed to have high thresholds in several animal species such as mice, guinea pigs, cats, and gerbils; thus, they are thought to encode suprathreshold, higher-level, acoustic stimuli (Liberman, 1978; Evans and Palmer, 1980; Huet et al., 2016). However, in rhesus monkeys, Joris et al. (2011) found no evidence that low-SR fibers have higher thresholds than high-SR ANFs. This finding may therefore challenge the assumption that the loss of low-SR ANFs in humans translates into perceptual consequences at higher acoustic stimulus levels, such as SPiN difficulties (Hickox et al., 2017).
Human Studies
In the absence of post-mortum temporal bone data from young noise-exposed humans, it is difficult to precisely predict and quantify the extent to which CS occurs, and the noise levels, types, and duration that may produce CS before hearing thresholds are elevated. However, a recent temporal bone study by Wu et al. (2021) reported that middle-aged human subjects with a documented history of significant occupational noise exposure exhibited an additional 25% ANF loss compared to their low-noise counterparts. Moreover, OHC loss in middle-aged and older human adults with and without occupational noise exposure was highly correlated with ANF loss. Hence, the authors argued that CS may not necessarily be significant and noticeable in humans with minimal OHC loss (i.e., with normal or near-normal hearing thresholds). Instead, the effects of CS may only be clear in individuals with elevated hearing thresholds. Hence, these findings may explain the mixed and inconclusive outcomes produced by CS proxy measures in young normal-hearing humans with a history of acoustic over-exposure as discussed below.
Carney (2018) argues that although low- and medium-SR fibers may not necessarily be involved in the coding of suprathreshold stimuli in humans, their loss may still contribute to deficits in the processing of high-level acoustic stimuli through their involvement in an efferent auditory feedback loop. When this efferent feedback loop is compromised due to either noise exposure or aging, it is thought that it can no longer effectively maintain and enhance signal functional profiles at a wide range of levels and hence would not improve suprathreshold hearing in background noise (Carney, 2018).
Histopathological and Neurophysiological Aspects: Aging
Animal Studies
A progressive loss of cochlear synapses and afferent ANF degeneration is observed in aging rodent models (Sergeyenko et al., 2013; Altschuler et al., 2015; Fernandez et al., 2015; Gleich et al., 2016; Möhrle et al., 2016; Parthasarathy and Kujawa, 2018; Peineau et al., 2021). Table 2 shows a summary of key animal studies which investigated the proportion of synapse loss and the reduction in the amplitude of wave 1 of the ABR in relation to aging across different rodent species. The right panel of Figure 1 shows a scatterplot of the proportion of remaining synapses as a function of the age of the oldest age of animals (in percent lifespan) considered in the studies summarized in Table 2. The different numbers, shapes, and colors of the data points in the right panel of Figure 1 reflect the different animal species that were examined in the studies in Table 2.
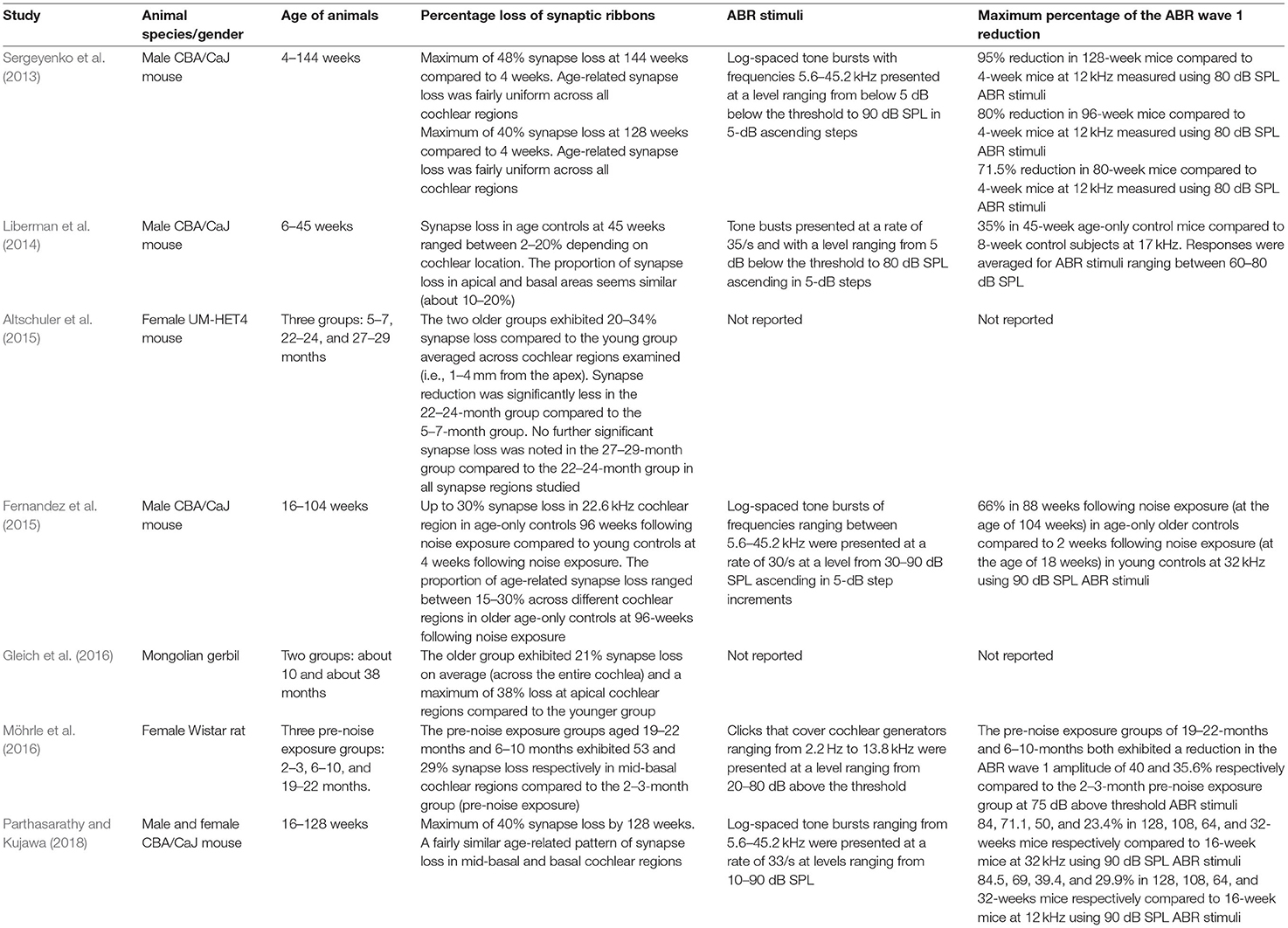
Table 2. Summary of the key studies on the effect of aging on synapse loss and ABR wave 1 amplitude across different animal species. Data reported were either explicitly mentioned in the manuscript text or were derived from the relevant figures in the respective publications using the online tool of WebPlotDigitizer version 4.5 (Rohatgi, 2021).
Unlike acute noise-induced CS, which primarily manifests in basal cochlear regions, Fernandez et al. (2015) provided evidence that the cochlear region of noise-induced CS broadens over time to have a widespread impact after a single acoustic trauma. Moreover, age-related synapse loss did not exceed 50% across the different rodent species, whereas acoustic over-exposure seems to account for a higher proportion of synapse loss in some animal studies (Kujawa and Liberman, 2009; Lin et al., 2011; Liu et al., 2012; Singer et al., 2013; Liberman and Liberman, 2015). Furthermore, unlike noise-exposure studies, evidence from aging studies suggests progressive age-related OHC loss that occurs in parallel with synapse loss. A minimal loss of IHCs took place as age progressed and SGC deterioration was slow and uniform across the different cochlear regions (Sergeyenko et al., 2013; Parthasarathy and Kujawa, 2018). Similar to noise-induced CS, the ANFs lost as a result of aging are thought to be predominantly low- to medium-SR fibers (Schmiedt et al., 1996; Kujawa and Liberman, 2015).
Human Studies
Post-mortem human temporal bone studies have confirmed a significant age-related degeneration of SGCs (Otte et al., 1978; Kusunoki et al., 2004; Makary et al., 2011; Nayagam et al., 2011). The percentage of SGC loss seems to be greater in humans with a higher proportion of degenerated cochlear hair cells. For instance, Makary et al. (2011) estimated the rate of SGC loss at around 1,000 per decade in human subjects with normal counts of cochlear hair cells. Otte et al. (1978) reported that this SGC loss rate was doubled (i.e. around 2,000 per decade) in subjects with varying degrees of sensorineural hearing loss compared to subjects with normal cochlear hair cells as shown in the data of Makary et al. (2011). The process of aging seems to affect type I ANFs in humans (Felder and Schrott-fischer, 1995; Chen et al., 2006) such that older adults with high-frequency sensorineural hearing loss were found to exhibit 30–40% type I ANF neuronal degeneration in the absence of significant IHC or SGC loss (Felder and Schrott-fischer, 1995).
More recently, Wu et al. (2019) found that the degeneration of type I ANF peripheral axons due to aging in humans took place well before the loss of OHCs, IHCs, and SGCs. Hence, this is consistent with the primary nature of age-related ANF deafferentation in humans. More than 60% ANF loss (as averaged across the entire standard audiometric range) was estimated to have occurred in human subjects aged over 50 years (Wu et al., 2019). ANF deafferentation was hypothesized to result in the loss of auditory neural information channels, which may render it more difficult for older adults to centrally process speech in the presence of background noise, even when hearing thresholds are within normal limits (as reflected by the normal counts of OHCs) (Wu et al., 2019). However, a caveat to this assumption could be that the relative proportion of low- to medium SR fibers, and their role in higher-level speech perception, are poorly understood in humans.
Wu et al. (2021) determined ANF loss in post-mortum human temporal bones of subjects aged 43–104. The authors estimated age-related ANF loss at 6.3% per decade. This was noted to take place across the entire human cochlea with more pronounced effects in basal cochlear regions. However, unlike the data reported by Wu et al. (2019), Wu et al. (2021) showed a strong positive correlation between OHC and ANF loss. According to the authors, this positive correlation between OHC and ANF loss contradicts the hypothesized primary nature of ANF loss in humans and hence adds more uncertainty to how age-related CS manifests perceptually in humans with normal/near-normal audiometric profiles. This is because most ANFs that are affected by CS are thought to make contact with IHCs and histopathological animal studies have demonstrated that the loss of CS and afferent ANFs occurs well before OHCs are lost (as discussed earlier). More temporal bone evidence is therefore necessary to establish the relation between ANF and OHC loss over the entire human lifespan.
Viana et al. (2015) counted synaptic ribbons connected with IHCs in older humans and reported that aged ears had no more than 2.0 synapses per IHC at basal cochlear regions (i.e., at about 2 kHz) compared to 11.3–13.3 synapses per IHC in young controls. This translates to approximately 85% age-related basal synapse loss in humans. At more apical cochlear regions (e.g., 0.25 kHz), synapses per IHC did not exceed 7.6 in older ears (i.e., about 40% synapse loss), which suggests that age-related synapse loss in humans may have a bigger impact at basal rather than apical cochlear regions. Synapse loss was reported to take place well before cochlear hair cells were lost. This is thus consistent with Wu et al. (2019) findings concerning the primary nature of peripheral neural deafferntiation. Bharadwaj et al. (2014) predicted that age-related synapse loss most likely occurs at a minimum of 30% in aged humans. This prediction was inferred from mouse data which showed that SGC degeneration occurred 1–2 years following synapse loss. Moreover, this prediction is consistent with the findings of Viana et al. (2015) and with rodent studies summarized in Table 2 which documented age-related synapse loss of up to 50%. Hence, significant synapse loss may well occur over a human's lifespan given the existing evidence from temporal bones on age-related ANF and SGC degeneration in older humans.
Histopathological and Neurophysiological Aspects: Combined Effects of Noise Exposure and Aging
Animal Studies
In a few animal models, the combined impact of aging and noise exposure on synapse loss has been investigated. Fernandez et al. (2015) determined the pattern of auditory neural degeneration following acute noise exposure across the lifespan of CBA/CaJ mice. Synapse loss was estimated at a maximum of about 55% in older animals aged 96 weeks following exposure to 100 dB SPL noise for 2 h at the age of 16 weeks compared to up to 30% in non-exposed older counterparts. Synapse loss was most significant in basal cochlear regions in both young and older mice. As noise-exposed mice aged further, synapse counts in more apical cochlear regions were found to deteriorate as well. The authors noted, however, that cochlear regions with the most significant noise-induced synapse loss exhibited less synapse degeneration per year (throughout the 96 weeks following the noise exposure) compared to cochlear areas with the lowest noise-induced CS. The authors proposed that this decrease in synapse loss is consistent with the assumption that only a proportion of afferent auditory ANFs may be vulnerable to both noise exposure and aging (Schmiedt et al., 1996; Furman et al., 2013).
Möhrle et al. (2016) reported that young rats exposed to 100 dB SPL noise for 2 h exhibited about 30% synaptic loss in mid-basal cochlear regions compared to controls. The synapse populations following the same noise exposure event in middle-aged and old rats were not significantly different from controls in each age group. Moreover, synaptic counts in middle-aged noise-exposed rats were similar to young noise-exposed animals. Old noise-exposed rats had about 15% fewer mid-basal IHC-ANF synapses compared to their young noise-exposed counterparts.
Human Studies
By assuming either a regular constant acoustic over-exposure throughout the lifespan or exposure to one single event of intense noise, we propose two simple models for the combined effects of noise exposure and aging on CS in basal cochlear regions as shown in Figure 2. In this figure, the proportion of remaining synapses is expressed as a function of age ranging from 0 to 100 years. Figures 2A,B represent the effects of age and the combined effects of age and constant acoustic overexposure on the proportion of synapse loss, while Figures 2C,D illustrate the effects of age and the combined effects of age and a single event of intense noise exposure. For both instances of noise exposure scenarios, we assume that either all IHC-ANF synapses (Figures 2A,C) or only low- and medium-SR ANFs (Figures 2B,D), which are thought to comprise 40% of type I ANFs in cats and guinea pigs (Liberman, 1978; Tsuji and Liberman, 1997), are vulnerable. It is assumed in the models that age causes the loss of a constant proportion of the remaining vulnerable synapses per unit of time. Similarly, noise exposure is assumed to cause a constant proportional loss of the remaining vulnerable synapses (for a given exposure). In other words, for a given vulnerable synapse, there is assumed to be a constant risk of loss for a given unit of time, or a given exposure. This is why the plots are asymptotic curves, rather than straight lines.
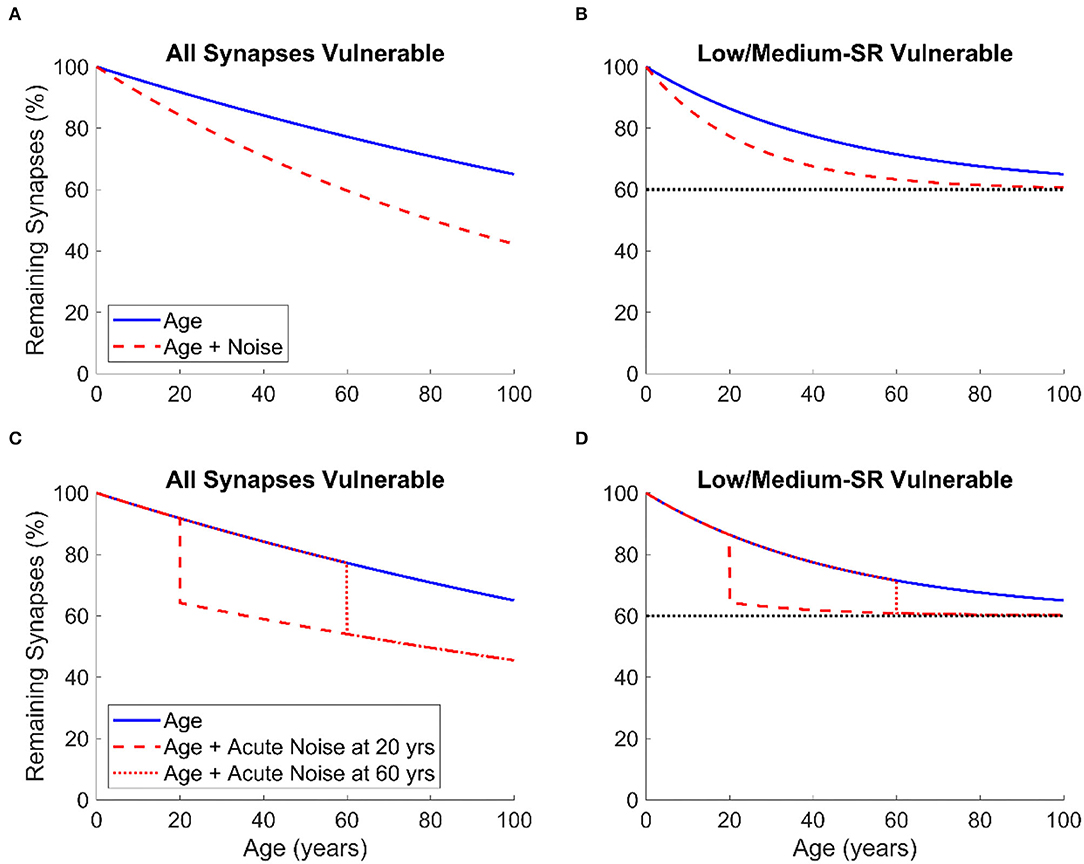
Figure 2. The proportion of remaining IHC-ANF synapses at basal cochlear regions as a function of age in humans given two models of synapse/ANF vulnerability: All synapses vulnerable (A,C) and only low- and medium-SR ANFs vulnerable (B,D). The two models are based on two assumptions: regular constant lifetime acoustic over-exposure (A,B) and one single event of intense noise exposure occurring at age 20 or 60 (C,D). In (B,D), the dashed line is an asymptotic line defining the percentage of synapse loss beyond which no further CS occurs.
For both noise exposure scenarios of our models, we predict that, although human temporal bone studies have shown that age-related ANF loss may occur at a proportion of more than 60% (Wu et al., 2019), IHC-ANF synapse loss secondary to aging may take place at a more conservative proportion (i.e., 30% in basal cochlear regions) as suggested by Bharadwaj et al. (2014). It is important to acknowledge that the main limitation in temporal bone studies, which may reduce confidence in their findings, is that many human subjects were in poor health prior to death. This may result in over-estimating the effects of aging (since there may be factors other than age contributing to CS and the influence of these factors may increase with age). Moreover, these studies lack precise estimation of noise and ototoxic exposure. Individuals who were not identified as having an occupational noise history could still have had significant lifetime exposure to noise and/or ototoxins. Finally, this difference in ratios may be explained by factors other than synapse loss that may account for ANF degeneration such as age-related genetic susceptibility to ANF degeneration.
We also assume that about 30% further synapse loss occurs due to acoustic over-exposure for both noise exposure scenarios. This estimation is based on Valero et al. (2017) data which has shown that 12–27% synapse loss occurred in the non-human primates of macaque monkeys following one intense event of noise exposure. Unfortunately, no animal or human data are available on the proportion of synapse loss secondary to cumulative regular constant lifetime noise exposure. So, we arbitrarily extended the assumption of 30% synapse loss to the scenario of regular acoustic-over exposure across the entire human lifespan.
For the assumption in which all synapses are vulnerable and for both scenarios of noise exposure (Figures 2A,C), CS due to noise exposure has a greater overall effect as more synapses are vulnerable. In contrast, synapse loss, either due to aging only or to noise exposure and aging together, saturates to a maximum of 40% if only low- and medium-SR ANFs are vulnerable (assuming that humans have the same proportion of low- and medium-SR ANFs to cats and guinea pigs as discussed above) as shown in Figures 2B,D.
It is worth pointing out that this model (as proposed in Figure 2) is very simplistic and is intended to be primarily a schematic illustration of the patterns of synapse loss that may occur in human ears secondary to noise exposure throughout the lifespan. However, the model may be useful for relating the expected consequences of different combinations of noise exposure and aging to objective and behavioral proxy measures in animals and humans.
Recently, the combined impact of both occupational noise exposure and aging in post-mortum human temporal bones was assessed by Wu et al. (2021). Lifetime occupational noise exposure was found to uniformly exacerbate age-related ANF loss across the different cochlear regions in the middle-aged group (i.e., subjects aged 50–74) by 25%, but not in the older group (i.e., subjects aged 75–104). These results are broadly consistent with the assumption we made above that when only low- and medium SR ANFs are vulnerable to both noise exposure and aging, little further CS occurs at older ages once a specific proportion of IHC-ANF synapses has been lost (Figures 2B,D). It is important to point out, however, that for the highest cochlear frequency regions considered by Wu et al. (2021) almost all ANFs were lost where a near-complete degeneration of IHCs had occurred. Therefore, the primary cause of this high-frequency ANF loss may not necessarily be CS, but rather IHC loss. This is because the loss of an IHC will lead to degeneration of the associated ANFs, irrespective of the degree of CS.
Wu et al. (2021) reported that IHC loss due to occupational noise exposure was minimal. In contrast, a high correlation between ANF and OHC loss in both basal and apical cochlear regions across different subjects of varying ages and with and without documented occupational noise exposure was found. Hence, the authors suggest that the effects of CS may only be substantial in the presence of threshold elevation in humans. Furthermore, OHC loss, rather than IHC or ANF loss, was found to be the main predictor of subjects' word recognition in quiet.
Given the lack of human temporal bone studies on the effect of noise exposure in isolation, it is difficult to estimate precisely how a history of acoustic over-exposure may impact the populations of cochlear synapses and ANFs at an older age. Given the difficulty in planning and conducting temporal bone studies, it is likely some time before data are available on how noise exposure and aging interact. This lack of studies may stem in part from the fact that it is difficult to retrospectively quantify the extent of lifetime noise exposure in deceased humans. Moreover, such studies may not be successful in controlling for genetic factors and past exposure to ototoxic substances, which may influence the onset and progression of age-related cochlear degeneration as well as the vulnerability to noise exposure at both young and older ages (Pyykkö et al., 2007).
Objective Proxy Measures of Cochlear Synaptopathy
In this section, animal and human studies in relation to noise exposure, aging, and the combined effects of noise exposure and aging, will be discussed in the framework of the objective proxy measures of CS: ABR wave I, ABR wave I:V amplitude ratio, SP:AP ratio, EFR, and MEMR.
Auditory Brainstem Response Wave I
Auditory Brainstem Response Wave I: Noise Exposure
Animal Studies
Across different animal species, noise-induced CS, primarily in the absence of hair cell loss, is associated with a 12–72.4% decrease in the amplitude of wave 1 of the ABR to moderate-high level stimuli, as summarized in Table 1. In addition to the fact that these studies involved different animal species (which likely exhibit different susceptibility to noise-induced CS), different studies used an exposure of different levels, durations, and spectra of noise. Moreover, the effect of noise exposure was investigated using different ABR stimuli, and measures were made at different frequencies (which may be affected by CS to differing extents). These methodological differences, highlighted in Table 1, could at least partially explain the high variability in the percentage of the ABR wave 1 reduction found across the different animal studies. Finally, since the majority of the animal literature summarized in Table 1 employed animals of single-sex, it is difficult to draw firm conclusions on whether the amplitude of ABR wave 1 varies, and to what extent, as a function of sex.
Human Studies
The effect of excessive noise exposure on the amplitude of wave I of young normal-hearing human adults has been inconclusive. Some studies have reported that a smaller amplitude of wave I of the ABR is associated with high noise exposure in young subjects (Stamper and Johnson, 2015a,b; Liberman et al., 2016; Bramhall et al., 2017, 2021; Valderrama et al., 2018; Buran et al., 2022), while several other studies failed to document such an effect (Grinn et al., 2017; Grose et al., 2017; Prendergast et al., 2017a, 2018; Skoe and Tufts, 2018; Couth et al., 2020). Table 3 shows a summary of studies that investigated the effect of noise exposure on ABR wave I amplitude in humans. It is worth highlighting that Bramhall et al. (2017, 2021) investigated firearm exposure among military veterans, which is primarily an impulsive type of noise and may hence be different in effect from the recreational exposures considered by the majority of the other human literature (for reviews, see Bramhall et al., 2019; Le Prell, 2019). As highlighted in Table 3, the amplitude of ABR wave I of female participants was larger than that of males (Stamper and Johnson, 2015a,b; Bramhall et al., 2017; Grose et al., 2017; Prendergast et al., 2017a; Valderrama et al., 2018). ABR wave amplitudes seem to be influenced by the sex of participants due to the potential variability in lifetime noise exposure (i.e., males may exhibit higher noise exposure than females; Stamper and Johnson, 2015b), and anatomical differences between sexes (such as differences in cochlear dispersion, head size, and bone density; Mitchell et al., 1989; Don et al., 1993). The influence of sex on ABR wave I was not quantified and controlled in all human CS studies. Future studies on CS in humans could be more explicit in considering this factor.
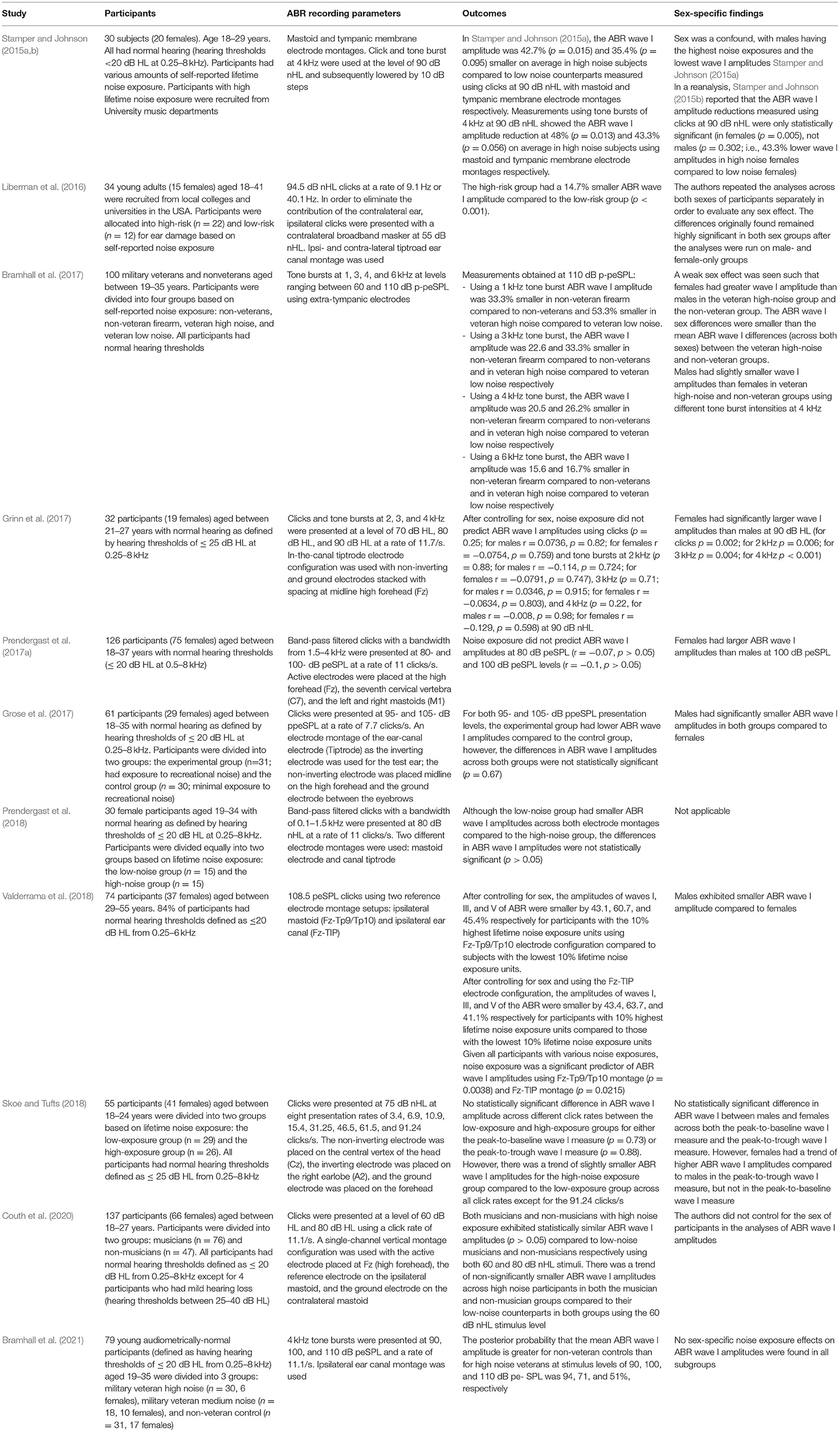
Table 3. Summary of the methods and findings of the studies that investigated the effect of noise exposure on the amplitude of wave I of the ABR in humans. Data reported were either explicitly mentioned in the manuscript text or were derived from the relevant figures in the respective publications using the online tool of WebPlotDigitizer version 4.5 (Rohatgi, 2021).
Auditory Brainstem Response Wave I: Aging
Animal Studies
Rodent studies suggest that age-related CS, in the absence of significant lifetime noise exposure, results in reduced amplitude of wave 1 of the ABR as documented in Table 2. The maximum age-related decline in wave 1 amplitude ranged between 70 and 90% (Sergeyenko et al., 2013; Parthasarathy and Kujawa, 2018), which is generally greater than that seen in studies investigating the effect of noise exposure in young animals (summarized in Table 1). This difference could be explained by the fact that age-related OHC loss had occurred in older animal subjects (which was not the case in young noise-exposed animals) especially in basal cochlear regions as documented by studies such as Sergeyenko et al. (2013), Liberman et al. (2014), Fernandez et al. (2015), and Parthasarathy and Kujawa (2018). Moreover, it is possible that aging and noise exposure result in different degrees of synapse and ANF loss depending on cochlear location and spontaneous rate level.
Since the ABR wave 1 amplitudes evoked by frequency-specific tone bursts are highly dependent on basal cochlear generators, as data from guinea pigs have shown (Eggermont, 1976), age-related basal OHC loss may further decrease the magnitude of the ABR wave 1 and thus obscure the effect caused by CS. It is worth pointing out that the ABR wave 1 amplitude reductions were seen to take place across all stimulation frequencies (i.e., low- and high-frequency tone bursts) in the animal studies summarized in Table 2. Based on this assumption, the pure effect of CS on the ABR wave 1 amplitude evoked by frequency-specific tone bursts can therefore only be determined once age-related basal OHC loss has been controlled for. However, computational modeling data from Verhulst et al. (2018a) suggest that OHC loss may have a limited impact on ABR wave 1 amplitudes for stimuli of 90 dB peSPL since the response growth of the OHCs is linear at high stimulus intensities. The computational modeling found that OHC loss even slightly increased ABR wave 1 amplitude for stimulus levels above 90 dB peSPL (Verhulst et al., 2018a). Moreover, Buran et al. (2022) also showed that accounting for cochlear gain loss (based on pure tone thresholds or distortion product otoacoustic emissions) in a computational modeling algorithm had a small effect on synapse predictions generated by the model from the ABR wave I amplitude measurements.
A strong correlation has been reported between the proportion of age-related synapse loss and ABR wave 1 amplitude in mice (Sergeyenko et al., 2013; Parthasarathy and Kujawa, 2018). Figure 3A illustrates the relationship from the results of Sergeyenko et al. (2013). It is important to point out that in this correlation analysis age-related OHC loss was never accounted for, and thus, the reductions in the ABR wave 1 amplitudes could be confounded by age-related threshold shifts. Further research is necessary to establish the effect of OHC loss on ABR wave 1 amplitude reduction secondary to CS (for the reasons discussed above) in order to establish whether ABR wave 1 amplitude may be a robust proxy measure of age-related CS with/without accounting for OHC loss.
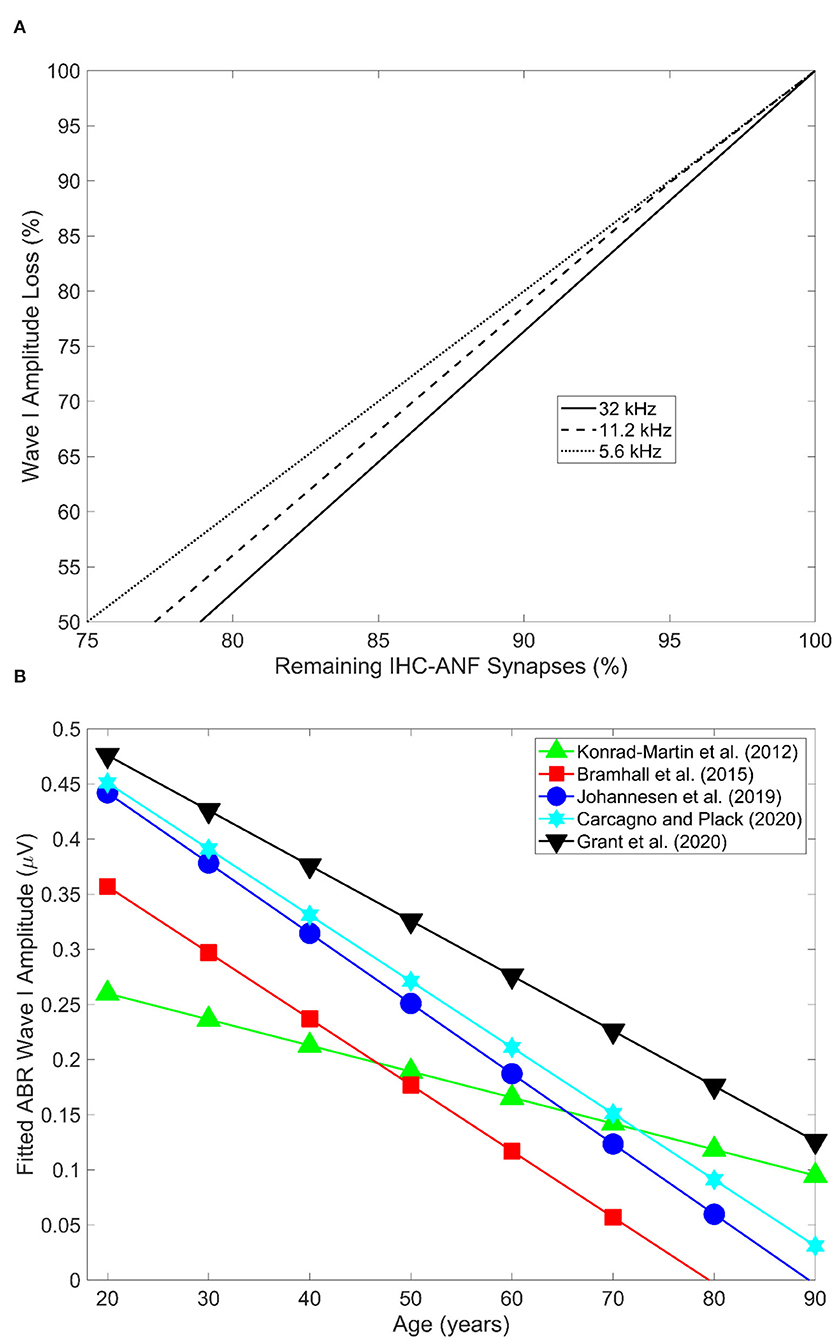
Figure 3. (A) Shows the relation between age-related decline in wave 1 amplitude and remaining IHC-ANF synapses as estimated in the 5.6, 11.2, and 32 kHz cochlear regions in CBA/CaJ mice. Redrawn from the data reported in panel D of Figure 5 in Sergeyenko et al. (2013) using the online tool of WebPlotDigitizer version 4.5 (Rohatgi, 2021). (B) Illustrates ABR wave I amplitude as a function of age across five different human studies. Redrawn from the data reported in Figure 4 in Bramhall (2021) using the online tool of WebPlotDigitizer version 4.5 (Rohatgi, 2021).
Human Studies
Otologically normal older adult humans have consistently been shown to exhibit smaller ABR amplitudes for waves I to V compared to their younger counterparts (Rowe, 1978; Maurizi et al., 1982; Allison et al., 1983; Costa et al., 1991; Konrad-Martin et al., 2012; Grose et al., 2019; Johannesen et al., 2019; Grant et al., 2020). Figure 3B shows the ABR wave I amplitude as a function of age in five different human studies (redrawn from Bramhall, 2021). An age-related decrease in the ABR amplitude measured at 110 dB peSPL at low click rates (i.e., 11 clicks/second) has been estimated at 38, 43, and 34% reduction for waves I, III, and V respectively for audiometrically normal-hearing individuals. This translates into 9.5, 10.8, and 8.5% amplitude reduction per decade for waves I, III, and V respectively (Konrad-Martin et al., 2012). The authors accounted for age-related increases in the audiometric thresholds, and thus the reduction in ABR wave I may not be attributed to OHC loss.
Bramhall et al. (2015) investigated the effect of age on ABR wave I amplitude by recruiting 57 adults (35 females) aged 19–90 with average pure tone audiometric thresholds at 0.5, 1, 2, and 4 kHz ranging between −1.25 to 38.75 dB HL. The ABR wave I amplitudes obtained using a 4 kHz tone burst presented at 80 dB nHL at a rate of 13.3/second were not influenced by the sex of the participants in the statistical model. After controlling for audiometric threshold loss, ABR wave I amplitude was found to decrease by about 17.8% per decade. Buran et al. (2022) provided a re-analysis of the Bramhall et al. (2017) data (n = 64; age range: 19–35; summarized in Table 3). After the potential confounds of sex and OHC function (as reflected by distortion product otoacoustic emission levels) were accounted for, ABR wave I amplitude measured at 110 dB peSPL was found to decrease by about 6.1% per decade.
Carcagno and Plack (2020) attempted to minimize the contribution of basal cochlear generators to ABR wave I (Eggermont and Don, 1978), which may be reduced by the effects of age, by band-pass filtering the click stimulus at 0.35–3 kHz and by presenting the click in a high-pass masking noise of 3.5–8 kHz (study summarized in Table 4). The authors reported an age-related reduction in wave I amplitude when high-pass masking noise was employed, at a rate of 12% reduction per decade (ages of subjects ranged from 18–70 years), with clicks presented at 80 dB p-peSPL. However, no age-related reduction was seen at 105 dB p-peSPL. This is the opposite pattern to that expected based on CS affecting low-SR fibers. In contrast, they observed an age-related wave I reduction of 17% per decade when no masking noise was used at 105 dB p-peSPL click level (but no reduction at 80 dB p-peSPL) even when controlling for high-frequency hearing loss in the statistical model. This latter result is consistent with CS in high-frequency cochlear regions (i.e., above the 3.5 kHz cut-off of the high pass masker). It is worth highlighting that this sort of masking paradigm has not been investigated in animal models of CS, so this approach has not been validated.
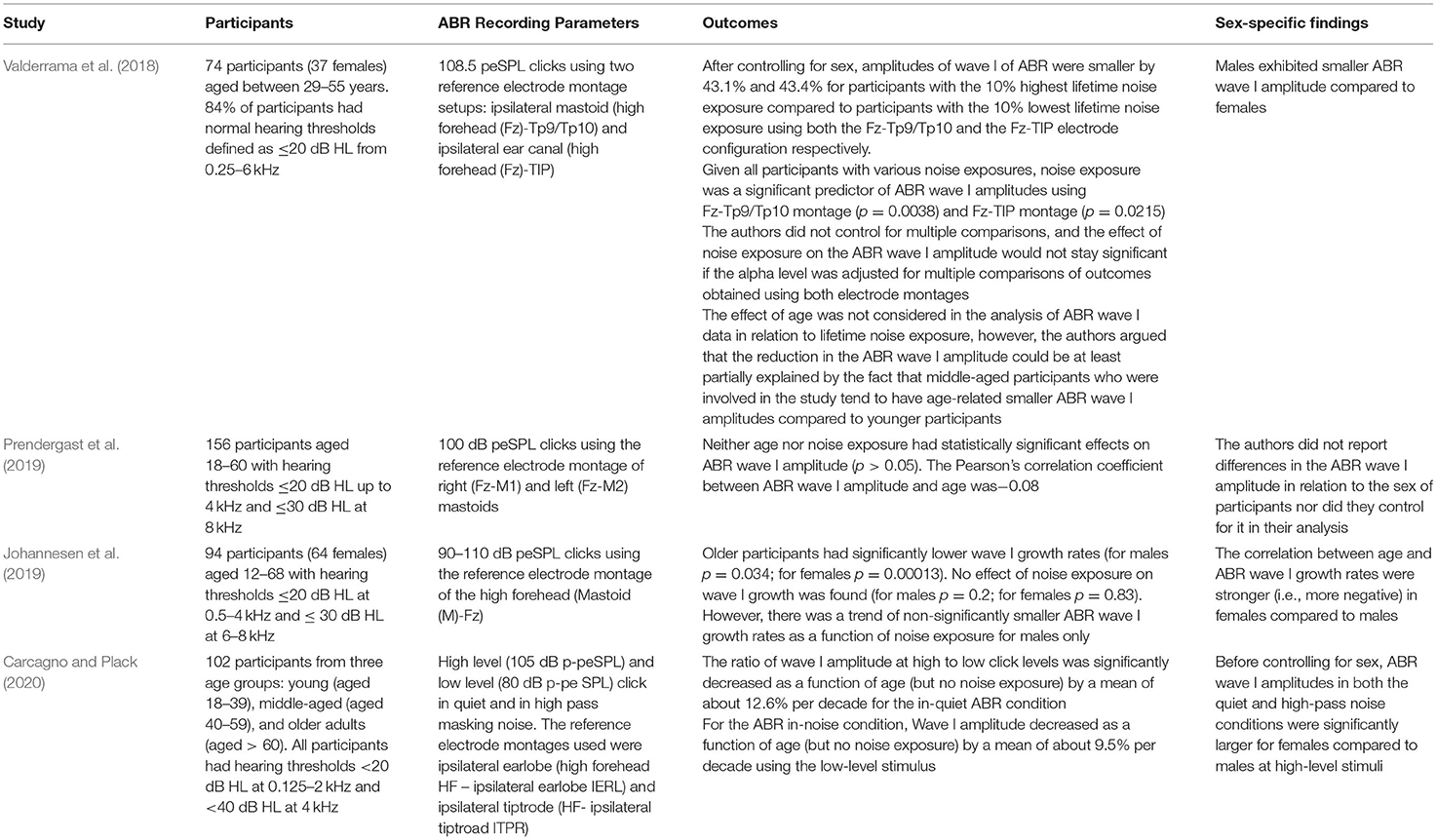
Table 4. Summary of the findings of key studies that investigated the combined effects of aging and noise exposure on wave I of ABR in humans. Data reported were either explicitly mentioned in the manuscript text or were derived from the relevant figures in the respective publications using the online tool of WebPlotDigitizer version 4.5 (Rohatgi, 2021).
Auditory Brainstem Response Wave I: Combined Effects of Noise Exposure and Aging
Animal Studies
Fernandez et al. (2015) reported that the ABR wave 1 amplitude in 88-week old CBA/CaJ mice exposed to the noise of 8–16 kHz at 100 dB SPL for 2 h at 16 weeks of age was 35, 65, and 80% smaller compared to 88-week old unexposed counterparts, 24-week-old young exposed animals, and 24-week-old young unexposed mice respectively. These findings imply that noise exposure at a young age in CBA/CaJ mice may cause a further reduction in the amplitude of the ABR wave 1 as animals become older (compared to unexposed aged counterparts). The authors have shown that a slower rate of IHC-ANF synapse loss as a result of aging has occurred in cochlear regions with the most CS due to noise exposure (compared to control cochleae without noise exposure). This is consistent with our saturative noise exposure-aging CS model which proposes the vulnerability of low- and medium-SR ANFs only. Nonetheless, this 35% decrease in the ABR wave 1 amplitude in exposed older mice (compared to unexposed older counterparts) may stem from the fact that the ABR wave 1 amplitude may be influenced by other noise- and age-related factors that were not controlled for such OHC and IHC loss.
Möhrle et al. (2016) reported that pre-noise-exposed middle-aged (6–10 months) and older (19–22 months) rats exhibited a 40% smaller amplitude of wave 1 compared to pre-exposed young (2–3 months) rats. However, no further significant decrease in the amplitude of wave 1 of ABR in post-exposed middle-aged and older rats was noted compared to their pre-exposed middle-aged and older subject counterparts (animals were exposed to 8–16 kHz broadband noise at 100 dB SPL for 2 h). The key difference in methodology between Fernandez et al. (2015) and Möhrle et al. (2016) is that the animals in the Möhrle et al. (2016) study were not exposed to noise and then aged. Rather, they were aged and then noise exposed. In line with the patterns of synapse loss across the different age groups in this study (as discussed earlier in the histopathological section), the authors hypothesized that, as most vulnerable ANFs are lost as a result of aging, little further reduction in the amplitude of wave 1 of ABR is seen when noise exposure is added to middle-aged and older animals. This is consistent with our saturative model of CS which suggests that when only low- and medium-SR ANFs are vulnerable to noise exposure and aging, less CS loss may occur once the majority of vulnerable IHC-ANF synapses have been lost.
Although Fernandez et al. (2015) and Möhrle et al. (2016) employed different rodent species, with major methodological differences as highlighted above, their findings shed light on the potentially different patterns of noise-induced CS when noise exposure occurs at a young or old age. These differences should inform future human studies investigating the interaction of aging and noise exposure.
Human Studies
The contribution of both noise exposure and aging to the amplitude of ABR wave I in humans with normal/near-normal hearing was investigated by some studies, which have reported mixed results. Table 4 summarizes the methods and outcomes of these studies. Only Valderrama et al. (2018) reported that lifetime noise exposure may exacerbate an age-related decrease in the amplitude of wave I of the ABR. In contrast, other studies which considered the effects of noise exposure and aging found no correlation between lifetime noise exposure and ABR wave I amplitude (Prendergast et al., 2019; Carcagno and Plack, 2020). Similarly, Johannesen et al. (2019) reported no significant correlation between lifetime noise exposure and ABR wave I amplitude growth.
Several explanations have been proposed to justify the lack of consistency in the findings of the ABR wave I in relation to detecting CS across the different human studies. For instance, Bramhall et al. (2019) stated that the between-subject factors, which are difficult to control in human research, include the type (e.g., recreational vs. occupational/firearm noise) and duration of noise exposure as well as the tools used to retrospectively quantify them. Moreover, it could be difficult to rule out the presence of CS in the human control groups recruited based on self-reports of lifetime noise exposure. This is because noise exposure history is usually quantified using self-report questionnaires that primarily rely on subjects' ability to recall their history of noise exposure, which may not be optimally reliable and accurate (Bramhall et al., 2019). Another major concern with regards to the use of the ABR wave I amplitude is its potential lack of sensitivity to detect CS in humans due to the possibility that low-and medium-SR ANF responses may not contribute to ABR wave I amplitude (Versnel et al., 1990; Bourien et al., 2014). Rather, high-SR ANF activity may primarily dominate the ABR wave I amplitude (Bourien et al., 2014).
It has also been hypothesized that a noise-induced decrease in the amplitude of wave I of the ABR in normal-hearing humans could be so marginal that the current ABR wave I techniques may not be sensitive enough to detect it (Hickox et al., 2017). Prendergast et al. (2018) estimated that the coefficient of variation (CoV) of the ABR wave I amplitude was comparable to the wave V amplitude (i.e., CoV < 0.35). This may be in favor of detecting the effect of noise exposure on the ABR wave I amplitude. However, if this variance does not directly relate to noise exposure, then many hundreds of participants may be needed to detect small noise-induced changes, even at a group level.
Both Prendergast et al. (2018) and Guest et al. (2019b) estimated that the amplitude of wave I in young normal-hearing adults exhibits high test-retest reliability (intraclass correlation coefficient of 0.85). So by assuming that humans exhibit a similar proportion of synapse loss as the non-human primates of macaque monkeys (i.e., up to 27%), a reduction in the ABR wave I amplitude should be evident in humans in longitudinal studies. However, data from guinea pigs suggests that some cochlear synapses damaged following noise exposure were partially repaired (Song et al., 2016). A similar effect could happen in humans, and thus ABR wave I amplitude recovers to some extent. This recovery may also be variable across humans, which adds a further source of variability in the measurement of ABR wave I amplitude in CS studies. It should also be noted that humans could exhibit different genetic susceptibility to noise- and age-related CS. Hence, this could be another major source of variability that may influence ABR wave I amplitude reductions.
Finally, since both noise exposure and aging are thought to be associated with worse hearing thresholds in the extended high frequency (EHF) range (Matthews et al., 1997; Somma et al., 2008; Liberman et al., 2016; Bramhall et al., 2017), ABR wave I amplitude reduction may be confounded by the involvement of basal high-frequency cochlear generators such that smaller ABR wave I amplitude is recorded secondary to basal OHC loss (Eggermont and Don, 1978). As discussed earlier, it is important to establish the extent to which hearing threshold loss affects ABR wave I reduction, especially at high stimulus levels, in order to determine the efficacy of ABR wave I amplitude as a proxy measure of CS in the presence of noise-induced or age-related threshold elevations.
Auditory Brainstem Response Wave I:V Amplitude Ratio
In addition to the amplitude of wave I of the ABR, other electrophysiological objective metrics have been used to assess CS in both animal and human research. For instance, the ratio of ABR wave I amplitude to wave V amplitude (wave I:V amplitude ratio) is thought to reflect the compensatory central gain that is hypothesized to take place as a result of the ANF deafferentation (Schaette and McAlpine, 2011). As a result, the amplitude of wave V could remain the same (as a result of central neural compensation) or even increase (in case of over-compensation), hence reflecting increased neural activity at the level of the mid-brain where wave V is generated. This may therefore translate into tinnitus and hyperacusis in humans (Gu et al., 2012; Hickox and Liberman, 2014). A potential limitation with the use of ABR wave I:V amplitude ratio as a proxy tool to detect and quantify CS is that the degree of central gain in response to reduced peripheral input (as indicated by wave V amplitude) may vary. This means that two individuals with identical ABR wave I amplitudes could have different wave I:V ratios depending on the degree of central gain.
It is important to note that the wave I:V amplitude ratio was found to exhibit high test-retest reliability in young normal-hearing adults (Prendergast et al., 2018). This suggests that this synaptopathy metric is probably still worth considering in future research. However, as described above in the discussion of wave I amplitude, it is not clear whether the wave I:V amplitude ratio is sensitive enough to detect and quantify CS cross-sectionally.
Auditory Brainstem Response Wave I:V Amplitude Ratio: Noise Exposure
The effect of noise exposure on the ABR wave I:V amplitude ratio is inconsistent across the literature. On the one hand, a few studies documented evidence for the central gain hypothesis such that no change to the amplitude of wave V was found while the amplitude of wave I was decreased in young human and rodent subjects with a history of noise exposure (Schaette and McAlpine, 2011; Hickox and Liberman, 2014; Bramhall et al., 2017). Megarbane and Fuente (2020) reported that a smaller wave I:V amplitude ratio is associated with worse SPiN performance (which is considered as a potential perceptual consequence of CS) in one ear only of audiometrically normal young adults with variable self-reported SPiN abilities. On the other hand, Guest et al. (2017) and Prendergast et al. (2017a) reported no evidence of a smaller wave I:V amplitude ratio in noise-exposed young normal-hearing human subjects compared to controls with minimal noise exposure. Grose et al. (2017) found a significantly smaller wave I:V amplitude ratio in subjects with high noise exposure compared to low-noise control subjects. However, the reduction in wave I:V amplitude ratio was not correlated with tinnitus, and primarily occurred due to a reduction in wave I amplitude alongside no statistically significant change in wave V amplitude.
Auditory Brainstem Response Wave I:V Amplitude Ratio: Aging
In older CBA/CaJ mice with already documented basal OHC loss, Sergeyenko et al. (2013) reported a decreased amplitude of wave 1 of ABR with no evidence for reduced wave 5 amplitude, thus the authors suggested that the ratio of wave 1:5 amplitudes may decrease as a function of age. Verhulst et al. (2016) predicted that high-frequency sloping sensorineural hearing loss, typically accompanying ARHL (and potentially associated with noise exposure), may contribute to a smaller ABR wave I:V amplitude ratio when ABR click stimuli are used. This is because damage to basal cochlear generators may reduce wave I amplitude but have a much smaller impact on the amplitude of wave V (Eggermont, 1976; Eggermont and Don, 1978; Verhulst et al., 2016).
Normal-hearing older human adults were found to exhibit a diminished wave I:V amplitude ratio compared to their younger counterparts (Grose et al., 2019). Likewise, Carcagno and Plack (2020) reported no age-related decrease in the amplitude of wave V evoked using 105- and 80- dB p-peSPL clicks in quiet. In contrast, when clicks were presented at 80 dB p-peSPL with high-pass masking noise, the median of wave V reduction was estimated at 14% per decade. Interestingly, the changes in the ABR wave I and V amplitudes reported by Konrad-Martin et al. (2012) as indicated in Figure 3B show constant age-related decline in the amplitudes of both waves I and V evoked using 110 dB p-peSPL clicks in quiet. The data by Konrad-Martin et al. (2012) are therefore inconsistent with those reported by Grose et al. (2019) and Carcagno and Plack (2020) in quiet, and go against the hypothesis that a central compensation secondary to age-related peripheral neural deafferentation results in little change or even enhanced ABR wave V amplitude secondary to aging.
Auditory Brainstem Response Wave I:V Amplitude Ratio: Combined Effects of Noise Exposure and Aging
Möhrle et al. (2016) reported that after young and middle-aged rats were exposed to moderately loud noise, wave 1 amplitude significantly decreased while wave 5 amplitude remained intact in both age groups. Following a similar noise exposure pattern in older rats, both wave 1 and wave 5 amplitudes were reduced, which may indicate a decreased neuronal gain as a result of central auditory aging. These findings may explain the reduced ABR wave V amplitudes reported by Konrad-Martin et al. (2012) who tested military veterans (who were likely exposed to significant firearm noise), in that the ABR wave I:V amplitude ratio could be affected by central aging, apart from CS itself.
Recent human studies measured the wave I:V amplitude ratio as a function of age while taking into account noise exposure history (Valderrama et al., 2018; Prendergast et al., 2019; Carcagno and Plack, 2020). These studies found no evidence for reduced wave I:V in middle-aged and older adults. It is worth pointing out that Valderrama et al. (2018) reported that middle-aged subjects with tinnitus had a statistically significantly lower wave I:V amplitude ratio compared to their non-tinnitus counterparts. However, the authors did not take into account the extent of audiometric threshold loss in their analyses, which could at least partially account for lower wave I:V amplitude ratios. These mixed findings add further uncertainty to whether the combined effects of aging and noise exposure result in CS-related compensatory central gain, and thus perceptually translate into tinnitus in humans.
Summating Potential to Action Potential Ratio
Animal Studies
The SP:AP ratio has also been used as a metric of CS. The normalization of the auditory nerve AP (related to wave 1 of ABR) to the SP of hair cells is hypothesized to help in distinguishing presynaptic and postsynaptic damage at the IHC-ANF synapse (Sergeyenko et al., 2013). In aging CBA/CaJ mice with documented synapse loss, a large SP:AP ratio was found after age-related OHC loss was accounted for statistically. CS, in the absence of OHC loss, may hence compromise AP of the auditory nerve, while the SP remains intact (Sergeyenko et al., 2013).
Human Studies
In human studies, the rationale for the use of the SP:AP ratio is to control for possible sources of measurement variability, such as differences in head anatomy (Liberman et al., 2016). Liberman et al. (2016) found that the SP:AP ratio was increased in noise-exposed young normal-hearing adults compared to low-noise controls, although this was primarily due to greater SP rather than smaller AP. Similarly, Grant et al. (2020) reported increased SP and decreased AP in audiometrically-normal adults with the worst word recognition scores (as defined by the lower 25th percentile of word recognition scores) compared to their best-performing counterparts (i.e., those with the highest 75th percentile of word recognition scores). Chen et al. (2021) studied the SP:AP ratio in older adults with a confirmed age-related threshold elevation. The authors found that AP amplitudes were significantly reduced in participants with SP:AP ratios that were deemed abnormal (i.e., ≥ 34%) while the SP amplitudes were similar across the normal and abnormal SP:AP groups. These findings provide evidence that CS may occur as part of ARHL.
It is worth highlighting the poor test-retest reliability of the SP:AP metric reported by Prendergast et al. (2018), at least for the click level of 115.5 dB peSPL tested in that study. Hence, the SP:AP ratio may not be reliable enough to determine the combined effects of aging and noise exposure on CS. Additionally, the use of SP:AP metric in older adults might be complicated by age-related hair cell loss, which will require careful control, as performed by Sergeyenko et al. (2013) in their mouse study. Finally, it may be worth considering the approach proposed by Kamerer et al. (2020) in future studies. This method employs validated Gaussian functions to estimate the SP and the AP and is thought to provide a more reliable measure than visual inspection and determination (Kamerer et al., 2020).
Envelope Following Response
The EFR is an objective auditory evoked potential characterized by neural responses that are phase-locked with the stimulus envelope modulation (Dolphin and Mountain, 1992). EFRs elicited with high-level stimuli with low modulation depths and high-frequency envelopes are thought to be sensitive to CS (Bharadwaj et al., 2014). This is because saturated high-SR fibers do not phase lock when presented with such stimuli, but low-SR fibers do (Bharadwaj et al., 2014, 2015; Shaheen et al., 2015; Verhulst et al., 2018b). Consequently, EFRs may be more sensitive to CS than ABR wave I amplitudes, not only because ABR measures are highly variable in humans and thus difficult to control for, but also because EFRs reflect phase locking to temporal envelopes in which low-SR fibers are strongly involved (Bharadwaj et al., 2014). Conversely, the computational model provided by Encina-Llamas et al. (2019) showed that the levels typically used to elicit EFRs (i.e., 70–80 dB SPL) may not be very specific to low-SR ANFs since, at these high intensities, the EFR responses are dominated by basal off-frequency high-SR ANFs that have not yet reached saturation. The computational model showed a minimal effect of subclinical OHC loss (which typically is associated with normal audiogram) on EFR amplitudes using the stimuli commonly presented at 70–80 dB SPL.
More recently, Vasilkov et al. (2021) provided evidence that the use of a stimulus with a rectangular envelope, with modulation rate, modulation depth, and duty cycles of 120 Hz, 95 and 25% respectively, presented at a fixed root mean square level of 70 dB SPL, may provide more sensitivity to CS while being minimally affected by co-existing OHC loss compared to sinusoidally amplitude-modulated tones that are commonly used. Moreover, Mepani et al. (2021) assessed the correlation between word recognition scores (words were presented in background noise) and EFR amplitudes using sinusoidally vs. rectangular-modulated carrier tones in otologically-normal adults aged 18–63. The sinusoidally amplitude-modulated tones were presented at 85 dB SPL using carrier frequencies of 1 or 8 kHz and were 100% amplitude-modulated at modulation frequencies of 128 or 750 Hz. The rectangular-modulated carrier tones were presented at 70 dB SPL at a modulation frequency of 120 Hz with a 25% duty cycle and 100% modulation depth. The word recognition scores were significantly positively correlated with EFR amplitudes evoked using rectangular-modulated tones, but not with sinusoidally modulated tones.
Envelope Following Response: Noise Exposure
Animal Studies
Shaheen et al. (2015) employed moderate stimulus levels (up to 90 dB SPL) with a carrier frequency of 11.3 kHz and 32 kHz and modulation frequencies ranging from 0.4–1.99 kHz to elicit EFRs in CBA/CaJ mice. EFR amplitudes were significantly reduced (by up to 55%) in noise-induced synaptopathic mice compared to non-synaptopathic controls at modulation frequencies near 1 kHz. For these high modulation frequencies, the EFR is thought to originate from the auditory nerve. This reduction, however, was not as large at lower modulation frequencies.
Human Studies
In humans, since EFRs obtained using a 1 kHz modulation frequency exhibit smaller amplitudes than in animal studies, lower modulation frequencies are often used which are thought to reflect neural generators from the midbrain rather than from more peripheral sources (Bharadwaj et al., 2015). For instance, Bharadwaj et al. (2015) assessed EFRs in young normal-hearing adults using a 4 kHz carrier tone modulated at 100 Hz, at a fixed level of 75 dB SPL with different modulation depths, presented in notched noise to restrict the cochlear region associated with the response. Subjects who showed the greatest decrease in EFR amplitude as a function of decreasing the modulation depth of the stimuli from 0 to −8 dB had the worst behavioral amplitude modulation thresholds (r = 0.53, p = 0.008). Moreover, the group of subjects who reported high past noise exposure had marginally significantly steeper positive EFR slopes (i.e., the slope of the line fit of EFR magnitudes in relation to modulation depths) compared to the low noise group (p = 0.034).
More recently, Bramhall et al. (2021) measured EFR amplitude in young audiometrically normal military veterans and non-veterans using a 4 kHz sinusoidally amplitude-modulated carrier tone presented at 80 dB SPL. The authors found that EFR amplitudes were 2.7, 2.5, and 3.4 dB smaller in the military veteran high-noise group at 100, 63, and 40% modulation depths respectively compared to the non-veteran control group. After adjustment for sex and OHC function, as reflected by the average distortion-product otoacoustic emission levels at 3–8 kHz, smaller EFR amplitudes were found at all modulation depths in high-noise military veteran male and female participants compared to their non-veteran counterparts.
Paul et al. (2017b) presented a 5 kHz carrier tone modulated at 86 Hz (with 0 dB modulation depth) at 75 dB SPL to two groups of young normal-hearing 18- and 19-year-old adults with and without significant noise exposure history. EFRs were measured both in quiet and in narrow band noise (NBN). The authors found reduced EFR magnitude for the high noise group compared with the low noise group. In a correction to the findings in the original publication, Paul et al. (2018) subsequently reported no statistically significant differences in the EFR amplitudes between the low and high noise groups across all measurement conditions (p > 0.05). Further studies such as those by Grose et al. (2017), Guest et al. (2017, 2018), Prendergast et al. (2017a) and Carcagno and Plack (2020) failed to find any significant relation between EFR amplitudes and lifetime noise exposure, tinnitus, or listening difficulties in young audiometrically normal adults. For the relation between EFR amplitudes and lifetime noise exposure, Grose et al. (2017) reported a p-value of 0.0664, while Guest et al. (2017) noted a correlation coefficient (r) of 0.01 between lifetime noise exposure and EFR amplitudes (p = 0.94). Prendergast et al. (2017a) found that the correlation coefficient (r) between lifetime noise exposure and EFR amplitudes obtained using 262 Hz pure tones was 0.08 (p > 0.05), while r was −0.16 (p > 0.05) when EFRs were elicited by 4 kHz pure tones. Guest et al. (2017) found that the tinnitus group had non-significantly lower EFR amplitudes than the control group (p = 0.1). Finally, Guest et al. (2018) reported similar EFR amplitudes across two groups of audiometrically-normal adults with and without listening difficulties (p = 0.99).
Paul et al. (2017a) assessed EFRs in young normal-hearing adults with and without chronic tinnitus using a 5 kHz carrier tone modulated at 85 Hz and presented at 75 dB SPL at three modulation depths of 0 dB (in quiet and in NBN), −2.5 dB with NBN, and −6 dB with NBN. In an erratum to the original publication, although no statistically significant difference in EFR amplitude was found between the tinnitus and control groups (p = 0.207), there was a trend toward lower EFR amplitudes for the tinnitus group compared to the control group (Roberts et al., 2018).
Other human studies based on computational simulation models of the peripheral and central auditory system predicted reduced EFR amplitudes in synaptopathic normal-hearing listeners (Verhulst et al., 2018a,b). The decreased EFR amplitudes were significantly associated with poor performance on psychoacoustic amplitude modulation tasks (p < 0.05; Verhulst et al., 2018a,b). Given the mixed findings using low modulation frequency stimuli in human studies, it is not clear whether the EFR at these frequencies is sensitive to noise-induced CS.
Envelope Following Response: Aging
Animal Studies
Progressive age-related CS has been associated with decreased EFRs to 1,024 Hz amplitude-modulated tones in older CBA/CaJ mice (Parthasarathy and Kujawa, 2018). This aging-EFR correlation was found significant across different tone levels and modulation depths. At lower modulation rates, which are dependent on more basal generators, decreased EFRs in older adults may arise not only from peripheral synapse loss but also from age-related deterioration in the central auditory system due to neural fiber loss and demyelination (Walton, 2010; Bharadwaj et al., 2014; Parthasarathy and Kujawa, 2018).
Lai et al. (2017) measured EFR amplitudes in young and aged Fischer-344 rats, using 8 kHz carrier tones modulated at frequencies of 45, 128, and 456 Hz and modulation depths ranging from 3.125% (−30 dB) to 100% (0 dB). The authors accounted for age-related peripheral hair cell and neural degeneration, which may manifest as poorer central neural responses, by adjusting the EFR stimulus level presented to the age groups so that the ABR amplitudes for these levels were similar. After this peripheral activation matching, the authors reported enhanced EFR amplitudes at 100% modulation depth (but not at 25% modulation depth) in the aged animals compared to their young counterparts. This was found when tones were modulated at 16–90 Hz (which are thought to generate EFRs originating from central auditory neural generators) were presented at 85 dB SPL. This age-related EFR amplitude enhancement suggests that older subjects had increased compensatory central gain as a result of decreased peripheral ANF neural activity.
To emphasize the differences in EFR while taking into account age-related central gain, the authors performed an additional “central” activation matching to the EFR stimuli. This was done by measuring the EFR amplitudes of old rats using 85 dB SPL tones that are 100% amplitude modulated at 45, 128, and 256 Hz with a carrier frequency of 8 kHz (which would stimulate the cochlear region with the least age-related changes in hearing thresholds). The median EFR amplitude in aged rats for each of the amplitude-modulated tones was measured. The authors then identified the EFR stimulus intensities to be used in the cental matching by measuring the EFR amplitudes in young rats using sinusoidally amplitude-modulated tones presented at 85–60 dB SPL (in 5-dB descending steps). The EFR stimulus intensity that produced equivalent central activation across the young and older rats was subsequently employed in EFR amplitude measurements. For both types of peripheral and central matching independently, no significant age-related differences in EFR amplitudes at different modulation depths and frequencies between the young and aged animals were reported, which suggests that peripheral and central auditory temporal coding was not different between the two age groups.
Human Studies
In humans, Prendergast et al. (2019) employed four low-frequency tones of 240–285 Hz to modulate a carrier frequency of 4 kHz at an intensity of 80 dB SPL in young and middle-aged audiometrically normal (up to 4 kHz) adults. The authors reported that participants' age did not predict EFR amplitudes (adjusted r2 = −0.004, p = 0.495). Patro et al. (2021) measured EFR amplitudes in audiometrically normal adults using a carrier frequency of either 2 or 4 kHz modulated at a rate of 91.42 Hz presented either in quiet (70 dB SPL at modulation depths of −8 or 0 dB) or in notched-noise (presented at an overall level of 60 dB SPL at modulation depths of −8, −4, and 0 dB). For the 2 kHz carrier frequency, the oldest adults had significantly reduced phase-locking value (PLV) of the EFR at 0 dB modulation depth in quiet compared to their youngest counterparts (p = 0.048). The oldest group produced the lowest PLV compared to the middle-aged and youngest adult group for the carrier frequency of 4 kHz at modulation depths of 0 dB in quiet (p = 0.031) and −8 dB in noise (p = 0.009).
More recently, Vasilkov et al. (2021) found that EFR amplitudes evoked by rectangular modulated stimuli presented at 70 dB SPL at a modulation rate of 120 Hz, a modulation depth of 95%, and a duty cycle of 25%, were significantly reduced in older adults with suspected age-related CS (p < 0.0001). Moreover, the authors found that their single-unit ANF simulation model suggested that ANFs fired more synchronously with this type of EFR stimulus compared to the commonly used sinusoidally amplitude-modulated stimuli (Vasilkov et al., 2021).
Envelope Following Response: Combined Effects of Noise Exposure and Aging
Carcagno and Plack (2020) measured EFR amplitudes in young, middle-aged, and older adults using two carrier tones of 0.6 and 2 kHz, modulated at around 100 Hz using two modulation depths of 100 and 70%, embedded in pink noise (to minimize the contribution of high-SR fibers) and using band-pass noise at 3–8 kHz (to minimize the contribution of high-frequency cochlear regions). The authors reported a significant age-related reduction in EFR amplitudes using a 0.6 kHz carrier at both modulation depths, while no effect was noted for the 2 kHz carrier at either modulation depth. No correlation between EFR amplitudes and lifetime noise exposure was found for either 0.6 or 2 kHz carrier tones. These findings are consistent with earlier studies such as those by Leigh-Paffenroth and Fowler (2006), Grose et al. (2009), and Garrett and Verhulst (2019) which documented an age-related decline in electrophysiological measures of phase-locking at subcortical levels using modulation rates of about 100 Hz.
Given the above studies, there is some evidence that aging may degrade EFR amplitudes, potentially due in part to the deterioration of central auditory pathways in older adults. However, the evidence on the effect of noise exposure on EFRs has been generally mixed and inconclusive. It is not yet clear whether EFRs are sufficiently sensitive, at least using the currently used research paradigms in humans, to capture CS and peripheral ANF loss. This is because human studies employed much lower modulation frequencies to elicit EFRs, unlike animal studies which mainly used higher modulation frequencies that are believed to reflect the function of more peripheral auditory neural generators (Parthasarathy and Kujawa, 2018). Moreover, EFR amplitudes in the aged population may be influenced by enhanced central gain, central neural dysfunction, and high-frequency cochlear damage, which may add further ambiguity to identifying CS in the low–mid-frequency range (Lai et al., 2017). Furthermore, Hesse et al. (2016) suggest that EFRs could be primarily mediated by high-SR rather than low-SR fibers at high levels and may not hence be effective in the search for low-SR fiber loss.
Middle Ear Muscle Reflex
The MEMR, which in clinical terms is known as acoustic reflex (AR), is an objective measure of change in middle ear immittance that occurs as a result of an efferent feedback mechanism to the middle ear stapedial muscle in response to intense acoustic stimulation. Low- to medium-SR type I fibers may be involved in the afferent branch of the MEMR pathway (Kobler et al., 1992). Two types of MEMR approaches have been used in CS research: the standard tonal probe approach and the wideband probe approach. The standard tonal MEMR probe approach is widely used in clinical settings and measures middle ear admittance at one probe tone of 226 Hz or 1,000 Hz (Schairer et al., 2013). In contrast, the wideband probe MEMR determines middle ear admittance, power reflectance, and absorbance over a broad frequency range typically between 0.25 and 8 kHz (Schairer et al., 2013). Prendergast et al. (2018) and Guest et al. (2019b) reported that the MEMR thresholds obtained using the standard tonal probe approach exhibited high test-retest reliability in young audiometrically-normal human adults. This provides some promise to using the MEMR in the search for CS in humans.
Middle Ear Muscle Reflex: Noise Exposure
Animal Studies
In mice with a histologically verified noise-induced CS, MEMR thresholds obtained using wideband probe and broadband elicitors were significantly increased while MEMR growth functions (i.e. MEMR magnitudes as a function of elicitor level) were considerably decreased at frequencies corresponding to the affected cochlear regions compared to non-synaptopathic areas (Valero et al., 2016, 2018). Therefore, the MEMR has been suggested as a good proxy for CS (Bharadwaj et al., 2019). Figure 4 shows a schematic representation of MEMR thresholds and growth functions in mice with verified CS compared to control mice respectively as measured at contralateral noise onset and offset (redrawn from Valero et al., 2016).
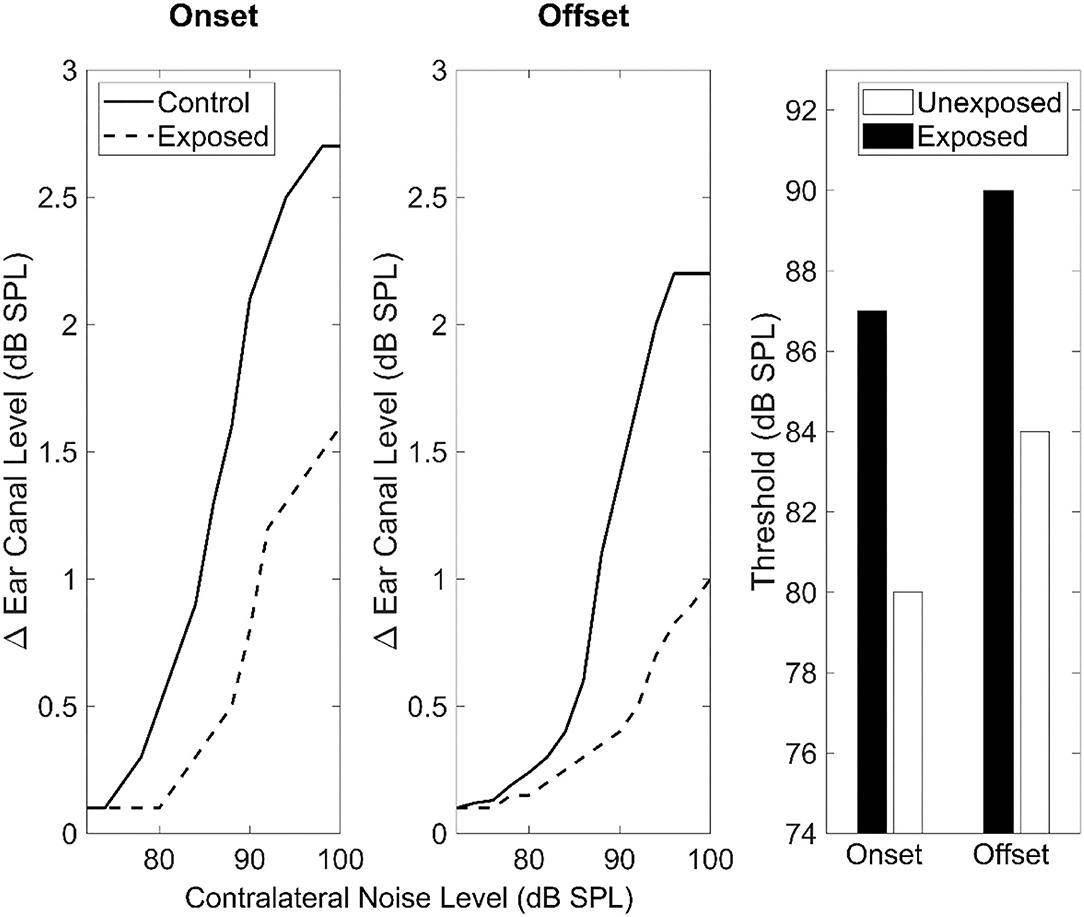
Figure 4. MEMR thresholds and growth functions (expressed as the difference in-ear canal SPL as a function of contralateral noise level) in noise-exposed and control mice measured at stimulus onset and offset. A wideband chirp covering a range of 4–64 kHz was presented contralaterally. This figure is redrawn from the data reported in panels A, B, and C of Figure 7 in Valero et al. (2016) using the online tool of WebPlotDigitizer version 4.5 (Rohatgi, 2021).
Human Studies
In humans, some recent studies have suggested a relation between MEMR amplitude and noise-induced CS. For instance, Shehorn et al. (2020) reported that high lifetime noise exposure is associated with lower ipsilateral broadband MEMR amplitude in normal-hearing young and middle-aged adults. Recently, Bramhall et al. (2022) measured the contralateral MEMR growth functions in 92 audiometrically-normal military veterans (who are typically exposed to firearm noise) and non-veterans aged 19–35 using a wideband probe and a broadband elicitor. The authors reported a trend of reduced MEMR growth functions in military veterans with high noise exposure compared to their non-veteran control counterparts. The mean difference in MEMR magnitude was lower by 0.29 dB in the veteran high noise group compared to the non-veteran control group. Other studies which involved normal-hearing young adults found a correlation between the presumed perceptual consequences of CS, such as poorer speech perception in noise and tinnitus, and reduced MEMR strength using the wideband probe approach (Wojtczak et al., 2017; Mepani et al., 2019; Shehorn et al., 2020). In contrast, Guest et al. (2019a) failed to find an association between MEMR thresholds (using the standard tonal probe and elicitors) and noise exposure, tinnitus, and coordinate response measure (CRM) SPiN thresholds. Moreover, Causon et al. (2020) failed to document a relationship between lifetime noise exposure in young normal-hearing subjects and MEMR thresholds and growth functions obtained using the clinical standard probe tone of 226 Hz and tonal elicitors. These negative findings may be potentially explained by the lack of sensitivity of the clinically MEMR protocol (which employs tonal elicitors and 226 Hz probe tone) to detect CS compared to the wideband probe and broadband noise elicitors employed by the other studies (Causon et al., 2020; Shehorn et al., 2020).
Middle Ear Muscle Reflex: Aging
Earlier studies suggest increased MEMR thresholds in normal-hearing older adults compared to their younger counterparts when measured by the standard clinical probe tone approach using broadband elicitors, but not low-to-mid frequency tonal elicitors (i.e., 0.5, 1, and 2 kHz), after controlling for the differences in audiometric thresholds (Silman, 1979; Gelfand and Piper, 1981). Wilson (1981) reported that older adults may show higher MEMR thresholds using the standard clinical probe tone approach, not only using broadband noise elicitors but also using tonal elicitors of 4 kHz and 6 kHz. Moreover, MEMR growth has been observed to decrease as a function of age (Thompson et al., 1980). In contrast, Unsal et al. (2016) found no differences in either the MEMR thresholds (obtained by the standard clinical probe tone approach) using 4 kHz tonal elicitors, or the MEMR decay, between older and younger adults. The correlation between MEMR thresholds/growth functions and aging in the above studies could be at least partially explained by age-related declines in central auditory neural pathways (Ouda et al., 2015), which need to be accounted for in the investigation of age-related CS using MEMR measures.
Middle Ear Muscle Reflex: Combined Effects of Noise Exposure and Aging
MEMR thresholds and growth functions using broadband noise elicitors may have promise as a measure of synaptopathy given the studies discussed above. However, it is not yet known whether lifetime noise exposure compounds the effect of age on MEMR strength.
Behavioral Proxy Measures in Humans
In this section, the evidence from human studies on noise exposure, aging, and the combined effects of noise exposure and aging using behavioral proxy measures of CS will be discussed.
Behavioral Proxy Measures in Humans: Noise Exposure
Based on the hypothesis that low- to medium-SR high threshold ANF fiber loss may affect speech perception at moderate-to-high levels (Liberman and Liberman, 2015), human studies have considered SPiN performance, and other proxy behavioral measures, concerning noise exposure in young normal-hearing adults. SPiN outcomes have been mixed and inconclusive (for reviews see Bramhall et al., 2019 and Le Prell, 2019).
Some studies have measured the effect of noise exposure on non-speech auditory psychoacoustic perceptual tasks in young normal-hearing adults. Measures such as interaural phase difference (IPD) discrimination, frequency and intensity difference limens, sound localization, and amplitude modulation detection have been used. Findings have been generally mixed and inconclusive. For instance, some studies reported that noise-exposed normal hearing adults exhibited poorer detection of temporal fine structure (e.g. discrimination of Gaussian noise from low-level noise with minimal envelope fluctuations) (Stone et al., 2008), worse amplitude modulation detection (Kumar et al., 2012; Stone and Moore, 2014; Verhulst et al., 2018b), and poorer IPD discrimination (Shehorn et al., 2020). In contrast, other studies failed to document a correlation between noise exposure and IPD discrimination, frequency, and intensity difference limens, sound localization, and amplitude modulation detection in young normal-hearing adults (Grose et al., 2017; Prendergast et al., 2017b, 2019; Yeend et al., 2017).
These mixed outcomes for behavioral proxy measures of CS in young noise-exposed humans with normal audiometric profiles could potentially be explained in three ways (Guest et al., 2018). Firstly, Noise-induced CS could not be as widespread in young normal-hearing adult humans as it is in rodent models. Secondly, the current behavioral measures in humans may not be particularly sensitive to CS. Based on signal detection theory, Oxenham (2016) showed that a synapse loss in humans up to 50% may not necessarily translate into measurable effects on behavioral tasks. Furthermore, the different behavioral tools used in human CS studies place variable sensory, perceptual, and central/cognitive demands (such as attention and memory), which likely contribute to inter-subject variability (Bramhall et al., 2019; DiNino et al., 2022). Thirdly, noise-induced CS in humans might not preferentially impair low- to medium-SR ANFs (as discussed in Section Histopathological and Neurophysiological Aspects: Noise Exposure). Moreover, low- to medium-SR ANFs might not have high thresholds in humans, consistent with evidence from non-human primates (Hickox et al., 2017). Hence, CS may not cause differential effects on performance as a function of stimulus level, as assumed by some measures.
Behavioral Proxy Measures in Humans: Aging
Audiometrically normal/near-normal older adults with no cognitive decline have consistently been shown to exhibit poorer SPiN performance using different types of speech stimuli and competing background noises compared to their younger counterparts (Pichora-fuller et al., 1995; Kim et al., 2006; Füllgrabe et al., 2015; Vermeire et al., 2016; Babkoff and Fostick, 2017). Compromised temporal processing, which may arise due to age-related central neural degeneration as well as CS, has been suggested to explain the difference in performance (Gordon-Salant and Fitzgibbons, 1993; Füllgrabe et al., 2015; Babkoff and Fostick, 2017). It is worth highlighting that not all studies which found an age-related decline in SPiN performance controlled for cognitive performance when comparing outcomes to younger adults. While the effect of age-related CS on SPiN tasks cannot be ruled out, it is possible that age-related deterioration in the EHF (i.e., frequencies above the standard clinical range of 8 kHz) thresholds (Stelrnachowicz et al., 1989; Snell et al., 2002), central auditory processing (Caspary et al., 2008; Ouda et al., 2015) and cognitive decline (Humes and Dubno, 2009; Kamerer et al., 2019) may contribute to the observed differences. Moreover, the variability in audiometric hearing thresholds and OHC function was not controlled for in the studies investigating the age-related auditory perceptual deficits in audiometrically normal/near-normal adults as discussed above. This may partially influence SPiN/psychophysical outcomes in favor of the younger population, which generally has better OHC function and hearing thresholds.
Some studies have tried to isolate the effects of CS by measuring performance as a function of level, under the assumption that CS will differentially affect higher levels due to low- and medium-SR ANF loss. Prendergast et al. (2019) found that, for audiometrically normal adults, age did not predict performance on the CRM task in either the 40 and 80 dB SPL stimulus presentation conditions while hearing thresholds at 2 and 16 kHz were accounted for. However, older participants performed significantly better than their younger counterparts in the 40 dB SPL condition of the digits in noise (DIN) task while older age was associated with worse performance on the 80 dB SPL condition. This is in line with the hypothesis that older subjects with age-related CS affecting low- to medium-SR ANFs perform worse with higher-level SPiN stimuli, but not lower-level stimuli, compared to their younger counterparts. The effects of the hearing thresholds at 0.5 kHz and EHF threshold at 16 kHz were controlled for in two separate statistical models and they were shown to be significant predictors of DIN thresholds.
Carcagno and Plack (2021) measured CRM and DIN thresholds using low-pass filtered speech stimuli (at a cut-off frequency of 3 kHz) presented at low and high levels to audiometrically normal adults of various ages. The authors employed pink band-pass filtered noise at 3–8 kHz in both tasks to reduce the contribution of basal cochlear generators. No credible age-related declines were found in the CRM task (using both collocated and spatially separated maskers) or in the DIN task at either level. Likewise, Johannesen et al. (2019) attempted to isolate the effects of age-related CS by employing both sentences from the hearing in noise test (HINT) fixed at 65 dB SPL and disyllabic words at 50, 65, and 75 dB SPL, while the masking noise (which was either speech shaped noise SSN or the international female fluctuating masker IFFM) was varied adaptively. Authors found that age was a significant predictor of HINT thresholds using both SSN and IFFM maskers, but not of the disyllabic words in noise thresholds (using either masker). The effect of differntial speech level used in the HINT test was not a significant predictor of SPiN performance as a function of age, even after the variability in hearing thresholds across subjects is accounted for.
Patro et al. (2021) employed sentence target stimuli presented either as full-spectrum or lowpass filtered signal (presented at a fixed level of 75 dB SPL in both conditions) embedded in a speech masker of either the same or different F0. The proportion of correct scores was measured in two spatial conditions: co-located (i.e., target and masker at 0° azimuth) and non-colocated (target and masker at ±15° azimuth). A significant age effect was reported for both conditions of the full-spectrum and lowpass-filtered speech target embedded with the same/different F0 speech maskers, however, no significant interaction between the spatial condition and age group was found.
Age-related declines in performance in psychoacoustic tasks in audiometrically normal older adults are inconsistent across the literature. For instance, on the one hand, decreased performance on amplitude modulation tasks (He et al., 2008; Füllgrabe et al., 2015; Wallaert et al., 2016; Carcagno and Plack, 2021), IPD discrimination (King et al., 2014; Füllgrabe et al., 2015; Carcagno and Plack, 2021), gap detection thresholds for a tone in noise (Patro et al., 2021), and frequency discrimination (He et al., 1998; Clinard et al., 2010) has been found in older adults compared to their younger counterparts. On the other hand, data from Grose et al. (2019), Paraouty et al. (2016), Patro et al. (2021), Prendergast et al. (2019) and Schoof and Rosen (2014) (amplitude modulation detection), Carcagno and Plack (2021) and Patro et al. (2021) (low-frequency carrier IPD discrimination task), Prendergast et al. (2019) and Patro et al. (2021) (high-frequency carrier IPD discrimination task) and Bianchi et al. (2019) and Carcagno and Plack (2021) (for frequency discrimination) provide no evidence for age-related declines in these psychophysical tasks. This inconsistency in findings may be partly explained by the fact that not all studies accounted for the variability in hearing thresholds, EHF thresholds, cognitive factors, past musical training, as well as central auditory processing ability in the analysis of their psychoacoustic data.
A few studies have attempted to isolate the effects of age-related CS on psychoacoustic tasks by presenting the psychophysical stimuli at different levels such as those by Prendergast et al. (2019) and Carcagno and Plack (2021). Yet, the outcomes of these studies provide little evidence of poorer performance at higher stimulus levels.
Behavioral Proxy Measures in Humans: Combined Effects of Noise Exposure and Aging
A few recent studies have attempted to evaluate the combined effects of aging and lifetime noise exposure on SPiN tasks. For instance, Valderrama et al. (2018) found that SPiN performance (using the high cue LiSN-S test) in young and middle-aged normal hearing adults was neither predicted by their age nor by their lifetime noise exposure. Similarly, Johannesen et al. (2019) showed that while noise exposure did not seem to influence the SPiN scores, older normal hearing subjects performed worse on a SPiN task involving words presented in steady and fluctuating noises compared to their younger counterparts. However, age (which ranged from 12 to 68 years in Johannesen et al., 2019 study) did not seem to influence the performance of participants in a different SPiN task involving sentences embedded in the same types of noises. Furthermore, Prendergast et al. (2019) and Carcagno and Plack (2021) reported that neither age nor lifetime noise exposure predicted the SPiN performance of subjects using the CRM task. However, the authors had conflicting findings concerning the effect of age using the DIN task, such that Prendergast et al. (2019) reported that older age was unexpectedly associated with better DIN thresholds at low stimulus levels while higher lifetime noise exposure was associated with better scores at high stimulus levels. In contrast, Carcagno and Plack (2021) found that neither age nor noise exposure had effects on DIN thresholds using their band-limited stimuli.
The evidence on the combined effects of aging and lifetime noise exposure on psychoacoustic tasks is sparse and inconclusive. Prendergast et al. (2019) and Carcagno and Plack (2021) have recently found that neither aging nor lifetime noise exposure was correlated with performance on a high-frequency carrier IPD task (Prendergast et al., 2019) and low-frequency carrier IPD task (Carcagno and Plack, 2021). Moreover, Carcagno and Plack (2021) found no interaction between lifetime noise exposure and aging on the amplitude modulation detection and frequency discrimination tasks. These inconsistent and mainly negative findings add further doubt to the sensitivity of these psychoacoustic tasks in detecting CS.
Summary and Recommendations for Future Research
In summary, animal histopathological studies have shown that both noise exposure and aging result in a substantial, yet highly variable, degree of synapse and ANF loss across several species. Rodent studies on the combined effects of noise exposure and aging suggest that animals who experience intense noise exposure at a young age may exhibit substantial noise-induced CS, and then go on to exhibit further CS as they age. However, the impact of noise exposure on older animals tends to be reduced, suggesting a saturation-like effect.
In young adult humans, histopathological studies are still lacking on the effects of noise exposure on synapse loss. Recently, Wu et al. (2021) have confirmed noise-related ANF loss in middle-aged and older human subjects. With regards to aging, human temporal bone studies suggest an age-related loss of synapses and ANFs, but these could not ascertain whether the lost fibers were primarily low-to-medium-SR ANFs, as is the case in rodent models, due to the lack of methods for classifying ANFs based on their SR in humans. The current human temporal bone data seem to be consistent with a model that assumes that only a portion of synapses (perhaps those with low- and medium-SR ANFs) are vulnerable to aging and noise exposure. While noise exposure was associated with a reduction in ANFs for middle-aged adults, older adults, who had a reduced baseline number of ANFs, did not show an additional effect of noise exposure (Wu et al., 2021). There are two possible explanations for the observed effect: first, these older adults may have reached the maximum extent of synapse loss, due to the effects of age alone, thus no further CS has taken place due to noise exposure; alternatively, the older “unexposed” adults may have had considerable undocumented noise exposure that eventually resulted in a similar extent of CS compared to their “exposed” counterparts.
Animal studies have consistently shown that noise-induced and age-related synapse and ANF loss are related to reductions in objective metrics (i.e., ABR wave 1, EFR, and MEMR amplitudes). In humans, objective and behavioral measures have produced inconsistent outcomes in relation to noise-induced CS, with some studies showing effects consistent with CS and others not. It is worth pointing out that estimates of the effect of noise exposure on physiological proxy measures of CS vary, with some studies showing large effects and others showing small non-significant effects. Some of this variability may be due to variability in study design and the type of noise exposure (e.g., military noise vs. recreational noise) as discussed earlier. In contrast, age-related changes in objective (e.g., wave I of ABR, EFR, and MEMR) and behavioral metrics are generally consistent across the human literature. However, it is not clear whether these changes relate directly to the synapse loss or are brought about by the age-related changes that occur across the entire auditory neural pathways. Only a few behavioral studies have attempted to isolate the effects of CS by comparing outcomes across levels, and these have not shown any clear differential effects. Future research will also need to account for the age-related loss of basal hair cells when investigating electrophysiologic neural responses (e.g., wave I of ABR and EFR) as well as the effects of cognitive decline when measuring behavioral performance in older adults.
Most of the current evidence in humans is based on observational cross-sectional studies that involve proxy objective or behavioral measures. Future research may need to employ longitudinal study designs and focus on the development and employment of more sensitive objective and behavioral tools based on a gold-standard measure of CS in living humans that relies on more robust CS models derived from animal and human temporal bone data. In particular, wideband MEMR thresholds and growth functions when measured using broadband elicitors are promising as sensitive measures of CS in humans. It may also be critical to establish more sensitive estimation tools of lifetime noise exposure such as by developing noise exposure metrics validated to objective measures (e.g., dosimetry). The need to control for differences in genetic susceptibility to noise- and age-related CS may still be a challenge in future research studies.
Although we recognize that it may be difficult to disentangle and control for all the different factors that may influence peripheral neural auditory aging, we recommend that future research focuses on the effects of noise exposure and aging in combination, rather than in separation, by determining when in the human lifespan noise exposure has occurred and the rate of progression of CS in ARHL using both histopathological and proxy approaches. This could be potentially achieved by controlling for past exposure to ototoxic substances and carefully screening and accounting for pathologic history, particularly some common chronic conditions among older adults that may affect peripheral hearing such as diabetes, blood hypertension, as well as genetic factors that may accelerate ARHL. Longitudinal study designs may be particularly useful in this regard, for instance studying cohorts of humans who are noise-exposed in occupational settings, compared to controls with a quiet lifestyle.
Author Contributions
All authors listed have made a substantial, direct, and intellectual contribution to the work and approved it for publication.
Funding
This work was supported by an internal Ph.D. grant from the Faculty of Biology, Medicine, and Health at the University of Manchester, the Medical Research Council (MR/V01272X/1), and the NIHR Manchester Biomedical Research Centre.
Conflict of Interest
The authors declare that the research was conducted in the absence of any commercial or financial relationships that could be construed as a potential conflict of interest.
Publisher's Note
All claims expressed in this article are solely those of the authors and do not necessarily represent those of their affiliated organizations, or those of the publisher, the editors and the reviewers. Any product that may be evaluated in this article, or claim that may be made by its manufacturer, is not guaranteed or endorsed by the publisher.
Acknowledgments
We would like to thank the reviewers and the associate editors for their contributions to this paper.
References
Allison, T., Wood, C. C., and Goff, W. R. (1983). Brain stem auditory, pattern-reversal visual, and short-latency somatosensory evoked potentials: latencies in relation to age, sex, and brain and body size. Electroencephalogr. Clin. Neurophysiol. 55, 619–636. doi: 10.1016/0013-4694(83)90272-9
Altschuler, R. A., Dolan, D. F., Halsey, K., Kanicki, A., Deng, N., Martin, C., et al. (2015). Age-related changes in auditory nerve-inner hair cell connections, hair cell numbers, auditory brain stem response and gap detection in UM-HET4 mice. Neuroscience 292, 22–33. doi: 10.1016/j.neuroscience.2015.01.068
Alvarado, J. C., Fuentes-Santamaría, V., Gabaldón-Ull, M. C., and Juiz, J. M. (2019). Age-related hearing loss is accelerated by repeated short-duration loud sound stimulation. Front. Neurosci. 13, 1–14. doi: 10.3389/fnins.2019.00077
Ashmore, J., Avan, P., Brownell, W. E., Dallos, P., Dierkes, K., Fettiplace, R., et al. (2010). The remarkable cochlear amplifier. Hear. Res. 266, 1–17. doi: 10.1016/j.heares.2010.05.001
Babkoff, H., and Fostick, L. (2017). Age-related changes in auditory processing and speech perception : cross-sectional and longitudinal analyses. Eur. J. Ageing 14, 269–281. doi: 10.1007/s10433-017-0410-y
Bharadwaj, H. M., Mai, A. R., Simpson, J. M., Choi, I., Heinz, M. G., and Shinn-Cunningham, B. G. (2019). Non-invasive assays of cochlear synaptopathy – candidates and considerations. Neuroscience 407, 53–66. doi: 10.1016/j.neuroscience.2019.02.031
Bharadwaj, H. M., Masud, S., Mehraei, G., Verhulst, S., and Shinn-Cunningham, B. G. (2015). Individual differences reveal correlates of hidden hearing deficits. J. Neurosci. 35, 2161–2172. doi: 10.1523/JNEUROSCI.3915-14.2015
Bharadwaj, H. M., Verhulst, S., Shaheen, L., Charles Liberman, M., and Shinn-Cunningham, B. G. (2014). Cochlear neuropathy and the coding of supra-threshold sound. Front. Syst. Neurosci. 8, 1–18. doi: 10.3389/fnsys.2014.00026
Bianchi, F., Carney, L. H., Dau, T., and Santurette, S. (2019). Effects of musical training and hearing loss on fundamental frequency discrimination and temporal fine structure processing: psychophysics and modeling. J. Assoc. Res. Otolaryngol. 20, 263–277. doi: 10.1007/s10162-018-00710-2
Bourien, J., Tang, Y., Batrel, C., Huet, A., Lenoir, M., Ladrech, S., et al. (2014). Contribution of auditory nerve fibers to compound action potential of the auditory nerve. J. Neurophysiol. 112, 1025–1039. doi: 10.1152/jn.00738.2013
Bramhall, N., Beach, E. F., Epp, B., Le Prell, C. G., Lopez-Poveda, E. A., Plack, C. J., et al. (2019). The search for noise-induced cochlear synaptopathy in humans: Mission impossible? Hear. Res. 377, 88–103. doi: 10.1016/j.heares.2019.02.016
Bramhall, N., Ong, B., Ko, J., and Parker, M. (2015). Speech perception ability in noise is correlated with auditory brainstem response wave I amplitude. J. Am. Acad. Audiol. 26, 509–517. doi: 10.3766/jaaa.14100
Bramhall, N. F. (2021). Use of the auditory brainstem response for assessment of cochlear synaptopathy in humans. J. Acoust. Soc. Am. 150, 4440–4451. doi: 10.1121/10.0007484
Bramhall, N. F., Konard-Martin, D., McMillan, G. P., and Griest, S. E. (2017). Auditory brainstem response altered in humans With noise exposure despite normal outer hair cell function. Ear. Hear. 38, e1–e12. doi: 10.1097/AUD.0000000000000370
Bramhall, N. F., McMillan, G. P., and Kampel, S. D. (2021). Envelope following response measurements in young veterans are consistent with noise-induced cochlear synaptopathy. Hear. Res. 408, 1–12. doi: 10.1016/j.heares.2021.108310
Bramhall, N. F., Reavis, K. M., Feeney, M. P., and Kampel, S. D. (2022). The impacts of noise exposure on the middle ear muscle reflex in a veteran population. Am. J. Audiol. 31, 126–142. doi: 10.1044/2021_AJA-21-00133
Buran, B. N., McMillan, G. P., Keshishzadeh, S., Verhulst, S., and Bramhall, N. F. (2022). Predicting synapse counts in living humans by combining computational models with auditory physiology. J. Acoust. Soc. Am. 151, 561–576. doi: 10.1121/10.0009238
Carcagno, S., and Plack, C. J. (2020). Effects of age on electrophysiological measures of cochlear synaptopathy in humans. Hear. Res. 396, 1–15. doi: 10.1016/j.heares.2020.108068
Carcagno, S., and Plack, C. J. (2021). Effects of age on psychophysical measures of auditory temporal processing and speech reception at low and high levels. Hear. Res. 400, 1–18. doi: 10.1016/j.heares.2020.108117
Carney, L. H. (2018). Supra-threshold hearing and fluctuation profiles: Implications for sensorineural and hidden hearing loss. J. Assoc. Res. Otolaryngol. 19, 331–352. doi: 10.1007/s10162-018-0669-5
Caspary, D. M., Ling, L., Turner, J. G., and Hughes, L. F. (2008). Inhibitory neurotransmission, plasticity and aging in the mammalian central auditory system. J. Exp. Biol. 211, 1781–1791. doi: 10.1242/jeb.013581
Causon, A., Munro, K. J., Plack, C. J., and Prendergast, G. (2020). The role of the clinically obtained acoustic reflex as a research tool for subclinical hearing pathologies. Trends Hear. 24, 1–14. doi: 10.1177/2331216520972860
Chen, M. A., Webster, P., Yang, E., and Linthicum, F. H. (2006). Presbycusic neuritic degeneration within the osseous spiral lamina. Otol. Neurotol. 27, 316–322. doi: 10.1097/00129492-200604000-00005
Chen, Z., Zhang, Y., Zhang, J., Zhou, R., Zhong, Z., Wei, C., et al. (2021). Cochlear synaptopathy: A primary factor affecting speech recognition performance in presbycusis. Biomed Res. Int. 2021, 1–7. doi: 10.1155/2021/6568477
Ciorba, A., Benatti, A., Bianchini, C., Aimoni, C., Volpato, S., Bovo, R., et al. (2011). High frequency hearing loss in the elderly: Effect of age and noise exposure in an Italian group. J. Laryngol. Otol. 125, 776–780. doi: 10.1017/S0022215111001101
Clinard, C. G., Tremblay, K. L., and Krishnan, A. R. (2010). Aging alters the perception and physiological representation of frequency: Evidence from human frequency-following response recordings. Hear. Res. 264, 48–55. doi: 10.1016/j.heares.2009.11.010
Concha-Barrientos, M., Campbell-Lendrum, D., and Steenland, K. (2004). Occupational Noise: Assessing the Burden of Disease From Work-Related Hearing Impairment at National And Local Levels. WHO Environmental Burden of Disease Series, Geneva.
Costa, P., Benna, P., Bianco, C., Ferrero, P., and Bergamasco, B. (1991). Aging effects on brainstem auditory evoked potentials. Electromyogr. Clin. Neurophysiol. 30, 495–500.
Couth, S., Prendergast, G., Guest, H., Munro, K. J., Moore, D. R., Plack, C. J., et al. (2020). Investigating the effects of noise exposure on self-report, behavioral and electrophysiological indices of hearing damage in musicians with normal audiometric thresholds. Hear. Res. 395, 1–19. doi: 10.1016/j.heares.2020.108021
DiNino, M., Holt, L. L., and Shinn-Cunningham, B. G. (2022). Cutting through the noise: noise-induced cochlear synaptopathy and individual differences in speech understanding among listeners with normal audiograms. Ear Hear. 43, 9–22. doi: 10.1097/AUD.0000000000001147
Dobie, R. A., and Humes, L. E. (2017). Commentary on the regulatory implications of noise-induced cochlear neuropathy. Int. J. Audiol. 56, S74–S78. doi: 10.1080/14992027.2016.1255359
Dolphin, W. F., and Mountain, D. C. (1992). The envelope following response: Scalp potentials elicited in the mongolian gerbil using sinusoidally AM acoustic signals. Hear. Res. 58, 70–78. doi: 10.1016/0378-5955(92)90010-K
Don, M., Ponton, C. W., Eggermont, J. J., and Masuda, A. (1993). Gender differences in cochlear response time: An explanation for gender amplitude differences in the unmasked auditory brain-stem response. J. Acoust. Soc. Am. 94, 2135–2148. doi: 10.1121/1.407485
Dubno, J. R., Eckert, M. A., Lee, F. S., Matthews, L. J., and Schmiedt, R. A. (2013). Classifying human audiometric phenotypes of age-related hearing loss from animal models. J. Assoc. Res. Otolaryngol. 14, 687–701. doi: 10.1007/s10162-013-0396-x
Eggermont, J. J. (1976). Analysis of compound action potential responses to tone bursts in the human and guinea pig cochlea. J. Acoust. Soc. Am. 60, 1132–1139. doi: 10.1121/1.381214
Eggermont, J. J., and Don, M. (1978). Analysis of the click-evoked brainstem potentials in humans using high-pass noise masking. J. Acoust. Soc. Am. 63, 1084–1092. doi: 10.1121/1.381816
Encina-Llamas, G., Harte, J. M., Dau, T., Shinn-Cunningham, B., and Epp, B. (2019). Investigating the effect of cochlear synaptopathy on envelope following responses using a model of the auditory nerve. J. Assoc. Res. Otolaryngol. 20, 363–382. doi: 10.1007/s10162-019-00721-7
Evans, E. F., and Palmer, A. R. (1980). Relationship between the dynamic range of cochlear nerve fibres and their spontaneous activity. Exp. Brain Res. 40, 115–118. doi: 10.1007/BF00236671
Felder, E., and Schrott-fischer, A. (1995). Quantitative evaluation of myelinated nerve fibres and hair cells in cochleae of humans with age-related high-tone hearing loss. Hear. Res. 91, 19–32. doi: 10.1016/0378-5955(95)00158-1
Fernandez, K. A., Guo, D., Micucci, S., De Gruttola, V., Liberman, M. C., and Kujawa, S. G. (2020). Noise-induced cochlear synaptopathy with and without sensory cell loss. Neuroscience 427, 43–57. doi: 10.1016/j.neuroscience.2019.11.051
Fernandez, K. A., Jeffers, P. W. C., Lall, K., Liberman, M. C., and Kujawa, S. G. (2015). Aging after noise exposure: Acceleration of cochlear synaptopathy in “recovered” ears. J. Neurosci. 35, 7509–7520. doi: 10.1523/JNEUROSCI.5138-14.2015
Fetoni, A. R., Pisani, A., Rolesi, R., Paciello, F., Viziano, A., Moleti, A., et al. (2022). Early noise-induced hearing loss accelerates presbycusis altering aging processes in the cochlea. Front. Aging Neurosci. 4, 2022. doi: 10.3389/fnagi.2022.803973
Füllgrabe, C., Moore, B. C. J., and Stone, M. A. (2015). Age-group differences in speech identification despite matched audiometrically normal hearing: Contributions from auditory temporal processing and cognition. Front. Aging Neurosci. 6, 1–25. doi: 10.3389/fnagi.2014.00347
Furman, A. C., Kujawa, S. G., and Liberman, C. M. (2013). Noise-induced cochlear neuropathy is selective for fibers with low spontaneous rates. J. Neurophysiol. 110, 577–586. doi: 10.1152/jn.00164.2013
Garrett, M., and Verhulst, S. (2019). Applicability of subcortical EEG metrics of synaptopathy to older listeners with impaired audiograms. Hear. Res. 380, 150–165. doi: 10.1016/j.heares.2019.07.001
Gates, G. A., and Mills, J. H. (2005). Presbyacusis. Lancet 336, 1111–1120. doi: 10.1016/S0140-6736(05)67423-5
Gelfand, S. A., and Piper, N. (1981). Acoustic reflex thresholds in young and elderly subjects with normal hearing. J. Acoust. Soc. Am. 69, 295–297. doi: 10.1121/1.385352
Gleich, O., Semmler, P., and Strutz, J. (2016). Behavioral auditory thresholds and loss of ribbon synapses at inner hair cells in aged gerbils. Exp. Gerontol. 84, 61–70. doi: 10.1016/j.exger.2016.08.011
Gordon-Salant, S., and Fitzgibbons, P. J. (1993). Temporal factors and speech recognition performance in young and elderly listeners. J. Speech Hear. Res. 36, 1276–1285. doi: 10.1044/jshr.3606.1276
Grant, K. J., Mepani, A. M., Wu, P., Hancock, K. E., Gruttola, V., De, L.iberman, M. C., et al. (2020). Electrophysiological markers of cochlear function correlate with hearing-innoise performance among audiometrically normal subjects. J. Neurophysiol. 124, 418–431. doi: 10.1152/jn.00016.2020
Grinn, S. K., Wiseman, K. B., Baker, J. A., and Le Prell, C. G. (2017). Hidden hearing loss? No effect of common recreational noise exposure on cochlear nerve response amplitude in humans. Front. Neurosci. 11, 1–24. doi: 10.3389/fnins.2017.00465
Grose, J. H., Buss, E., and Elmore, H. (2019). Age-related changes in the auditory brainstem response and suprathreshold processing of temporal and spectral modulation. Trends Hear. 23, 1–11. doi: 10.1177/2331216519839615
Grose, J. H., Buss, E., and Hall, J. W. (2017). Loud music exposure and cochlear synaptopathy in young adults: isolated auditory brainstem response effects but no perceptual consequences. Trends Hear. 21, 1–18. doi: 10.1177/2331216517737417
Grose, J. H., Mamo, S. K., and Hall, J. W. (2009). Age effects in temporal envelope processing: Speech unmasking and auditory steady state responses. Ear Hear. 30, 568–575. doi: 10.1097/AUD.0b013e3181ac128f
Gu, J. W., Herrmann, B. S., Levine, R. A., and Melcher, J. R. (2012). Brainstem auditory evoked potentials suggest a role for the ventral cochlear nucleus in tinnitus. J. Assoc. Res. Otolaryngol. 13, 819–833. doi: 10.1007/s10162-012-0344-1
Guest, H., Munro, K. J., and Plack, C. J. (2019a). Acoustic middle-ear-muscle-reflex thresholds in humans with normal audiograms: no relations to tinnitus, speech perception in noise, or noise exposure. Neuroscience 407, 75–82. doi: 10.1016/j.neuroscience.2018.12.019
Guest, H., Munro, K. J., Prendergast, G., Howe, S., and Plack, C. J. (2017). Tinnitus with a normal audiogram: Relation to noise exposure but no evidence for cochlear synaptopathy. Hear. Res. 344, 265–274. doi: 10.1016/j.heares.2016.12.002
Guest, H., Munro, K. J., Prendergast, G., Millman, R. E., and Plack, C. J. (2018). Impaired speech perception in noise with a normal audiogram: No evidence for cochlear synaptopathy and no relation to lifetime noise exposure. Hear. Res. 364, 142–151. doi: 10.1016/j.heares.2018.03.008
Guest, H., Munro, K. J., Prendergast, G., and Plack, C. J. (2019b). Reliability and interrelations of seven proxy measures of cochlear synaptopathy. Hear. Res. 375, 34–43. doi: 10.1016/j.heares.2019.01.018
He, N., Dubno, J. R., and Mills, J. H. (1998). Frequency and intensity discrimination measured in a maximum-likelihood procedure from young and aged normal-hearing subjects. J. Acoust. Soc. Am. 103, 553–565. doi: 10.1121/1.421127
He, N., Mills, J. H., Ahlstrom, J. B., and Dubno, J. R. (2008). Age-related differences in the temporal modulation transfer function with pure-tone carriers. J. Acoust. Soc. Am. 124, 3841–3849. doi: 10.1121/1.2998779
Hesse, L. L., Bakay, W., Ong, H. C., Anderson, L., Ashmore, J., McAlpine, D., et al. (2016). Non-monotonic relation between noise exposure severity and neuronal hyperactivity in the auditory midbrain. Front. Neurol. 7, 1–13. doi: 10.3389/fneur.2016.00133
Hickman, T. T., Smalt, C., Bobrow, J., Quatieri, T., and Liberman, M. C. (2018). Blast-induced cochlear synaptopathy in chinchillas. Sci. Rep. 8, 1–12. doi: 10.1038/s41598-018-28924-7
Hickox, A. E., Larsen, E., Heinz, M. G., Shinobu, L., and Whitton, J. P. (2017). Translational issues in cochlear synaptopathy. Hear. Res. 349, 164–171. doi: 10.1016/j.heares.2016.12.010
Hickox, A. E., and Liberman, M. C. (2014). Is noise-induced cochlear neuropathy key to the generation of hyperacusis or tinnitus? J. Neurophysiol. 111, 552–564. doi: 10.1152/jn.00184.2013
Huang, Q., and Tang, J. (2010). Age-related hearing loss or presbycusis. Eur. Arch. Otorhinolaryngol. 267, 1179–1191. doi: 10.1007/s00405-010-1270-7
Huet, A., Batrel, C., Tang, Y., Desmadryl, G., Wang, J., Puel, J. L., et al. (2016). Sound coding in the auditory nerve of gerbils. Hear. Res. 338, 32–39. doi: 10.1016/j.heares.2016.05.006
Humes, L. E., and Dubno, J. R. (2009). “Factors affecting speech understanding in older adults”, in: Springer Handbook of Auditory Research. New York, NY: Springer. p. 211–257. doi: 10.1007/978-1-4419-0993-0_8
Jayakody, D. M. P., Friedland, P. L., Martins, R. N., and Sohrabi, H. R. (2018). Impact of aging on the auditory system and related cognitive functions: a narrative review. Front. Neurosci. 12, 1–16. doi: 10.3389/fnins.2018.00125
Jensen, J. B., Lysaght, A. C., Liberman, M. C., Qvortrup, K., and Stankovic, K. M. (2015). Immediate and delayed cochlear neuropathy after noise exposure in pubescent mice. PLoS ONE 10, 1–17. doi: 10.1371/journal.pone.0125160
Johannesen, P. T., Buzo, B. C., and Lopez-Poveda, E. A. (2019). Evidence for age-related cochlear synaptopathy in humans unconnected to speech-in-noise intelligibility deficits. Hear. Res. 374, 35–48. doi: 10.1016/j.heares.2019.01.017
Joris, P. X., Bergevin, C., Kalluri, R., Laughlin, M. M., Michelet, P., Van Der Heijden, M., et al. (2011). Frequency selectivity in old-world monkeys corroborates sharp cochlear tuning in humans. Proc. Natl. Acad. Sci. U. S. A. 108, 17516–17520. doi: 10.1073/pnas.1105867108
Kamerer, A. M., Aubuchon, A., Fultz, S. E., Kopun, J. G., Neely, S. T., and Rasetshwane, D. M. (2019). The role of cognition in common measures of peripheral synaptopathy and hidden hearing loss. Am. J. Audiol. 28, 843–856. doi: 10.1044/2019_AJA-19-0063
Kamerer, A. M., Neely, S. T., and Rasetshwane, D. M. (2020). A model of auditory brainstem response wave I morphology. J. Acoust. Soc. Am. 147, 25–31. doi: 10.1121/10.0000493
Kim, S., Frisina, R. D., Mapes, F. M., Hickman, E. D., and Frisina, D. R. (2006). Effect of age on binaural speech intelligibility in normal hearing adults. Speech Commun. 48, 591–597. doi: 10.1016/j.specom.2005.09.004
King, A., Hopkins, K., and Plack, C. J. (2014). The effects of age and hearing loss on interaural phase difference discrimination. J. Acoust. Soc. Am. 135, 342–351. doi: 10.1121/1.4838995
Kobler, J. B., Guinan, J. J., Vacher, S. R., and Norris, B. E. (1992). Acoustic reflex frequency selectivity in single stapedius motoneurons of the cat. J. Neurophysiol. 68, 807–817. doi: 10.1152/jn.1992.68.3.807
Konrad-Martin, D., Dille, M. F., McMillan, G., Griest, S., McDermott, D., Fausti, S. A., et al. (2012). Age-related changes in the auditory brainstem response. J. Am. Acad. Audiol. 23, 18–35. doi: 10.3766/jaaa.23.1.3
Kujawa, S. G., and Liberman, M. C. (2006). Acceleration of age-related hearing loss by early noise exposure: Evidence of a misspent youth. J. Neurosci. 26, 2115–2123. doi: 10.1523/JNEUROSCI.4985-05.2006
Kujawa, S. G., and Liberman, M. C. (2009). Adding insult to injury: Cochlear nerve degeneration after “temporary” noise-induced hearing loss. J. Neurosci. 29, 14077–14085. doi: 10.1523/JNEUROSCI.2845-09.2009
Kujawa, S. G., and Liberman, M. C. (2015). Synaptopathy in the noise-exposed and aging cochlea: Primary neural degeneration in acquired sensorineural hearing loss. Hear. Res. 330, 191–199. doi: 10.1016/j.heares.2015.02.009
Kumar, U., Ameenudin, S., and Sangamanatha, A. (2012). Temporal and speech processing skills in normal hearing individuals exposed to occupational noise. Noise Heal. 14, 100–105.
Kusunoki, T., Cureoglu, S., Schachern, P. A., Baba, K., Kariya, S., and Paparella, M. M. (2004). Age-related histopathologic changes in the human cochlea: a temporal bone study. Otolaryngology 131, 897–903. doi: 10.1016/j.otohns.2004.05.022
Lai, J., Sommer, A. L., and Bartlett, E. L. (2017). Age-related changes in envelope-following responses at equalized peripheral or central activation. Neurobiol. Aging 58, 191–200. doi: 10.1016/j.neurobiolaging.2017.06.013
Le Prell, C. G. (2019). Effects of noise exposure on auditory brainstem response and speech-in-noise tasks: a review of the literature. Int. J. Audiol. 58, 1–28. doi: 10.1080/14992027.2018.1534010
Leigh-Paffenroth, E. D., and Fowler, C. G. (2006). Amplitude-modulated auditory steady-state responses in younger and older listeners. J. Am. Acad. Audiol. 17, 582–597. doi: 10.3766/jaaa.17.8.5
Liberman, L. D., and Liberman, M. C. (2015). Dynamics of cochlear synaptopathy after acoustic overexposure. J. Assoc. Res. Otolaryngol. 16, 205–219. doi: 10.1007/s10162-015-0510-3
Liberman, M. C. (1978). Auditory-nerve response from cats raised in a low-noise chamber. J. Acoust. Soc. Am. 63, 442–455. doi: 10.1121/1.381736
Liberman, M. C., Epstein, M. J., Cleveland, S. S., Wang, H., and Maison, S. F. (2016). Toward a differential diagnosis of hidden hearing loss in humans. PLoS ONE 11, 1–15. doi: 10.1371/journal.pone.0162726
Liberman, M. C., Liberman, L. D., and Maison, F. (2014). Efferent feedback slows cochlear aging. J. Neurosci. 34, 4599–4607. doi: 10.1523/JNEUROSCI.4923-13.2014
Lin, H. W., Furman, A. C., Kujawa, S. G., and Liberman, M. C. (2011). Primary neural degeneration in the guinea pig cochlea after reversible noise-induced threshold shift. J. Assoc. Res. Otolaryngol. 12, 605–616. doi: 10.1007/s10162-011-0277-0
Liu, L., Wang, H., Shi, L., Almuklass, A., He, T., Aiken, S., et al. (2012). Silent damage of noise on cochlear afferent innervation in guinea pigs and the impact on temporal processing. PLoS ONE 7, 1–11. doi: 10.1371/journal.pone.0049550
Maison, S. F., Usubuchi, H., and Liberman, M. C. (2013). Efferent feedback minimizes cochlear neuropathy from moderate noise exposure. J. Neurosci. 33, 5542–5552. doi: 10.1523/JNEUROSCI.5027-12.2013
Makary, C. A., Shin, J., Kujawa, S. G., Liberman, M. C., and Merchant, S. N. (2011). Age-related primary cochlear neuronal degeneration in human temporal bones. J. Assoc. Res. Otolaryngol. 12, 711–717. doi: 10.1007/s10162-011-0283-2
Matthews, L. J., Lee, F. S., Mills, J. H., and Dubno, J. R. (1997). Extended high-frequency thresholds in older adults. J. Speech, Lang. Hear. Res. 40, 208–14. doi: 10.1044/jslhr.4001.208
Maurizi, M., Altissimi, G., Ottaviani, F., Paludetti, G., and Bambini, M. (1982). Auditory brainstem responses (ABR) in the aged. Scand. Audiol. 11, 213–221. doi: 10.3109/01050398209087470
Mazelova, J., Popelar, J., and Syka, J. (2003). Auditory function in presbycusis: peripheral vs. central changes. Exp. Gerontol. 38, 87–94. doi: 10.1016/S0531-5565(02)00155-9
Megarbane, L., and Fuente, A. (2020). Association between speech perception in noise and electrophysiological measures: an exploratory study of possible techniques to evaluate cochlear synaptopathy in humans. Int. J. Audiol. 59, 427–433. doi: 10.1080/14992027.2020.1718783
Mepani, A. M., Kirk, S. A., Hancock, K. E., Bennett, K., de Gruttola, V., Liberman, M. C., et al. (2019). Middle ear muscle reflex and word recognition in “normal-hearing” adults: Evidence for cochlear synaptopathy? Ear. Hear. 41, 25–38. doi: 10.1097/AUD.0000000000000804
Mepani, A. M., Verhulst, S., Hancock, K. E., Garrett, M., Vasilkov, V., Bennett, K., et al. (2021). Envelope following responses predict speech-in-noise performance in normal-hearing listeners. J. Neurophysiol. 125, 1213–1222. doi: 10.1152/jn.00620.2020
Mitchell, C., Phillips, D. S., and Trune, D. R. (1989). Variables affecting the auditory brainstem response: Audiogram, age, gender and head size. Hear. Res. 40, 75–85. doi: 10.1016/0378-5955(89)90101-9
Möhrle, D., Ni, K., Varakina, K., Bing, D., Lee, S. C., Zimmermann, U., et al. (2016). Loss of auditory sensitivity from inner hair cell synaptopathy can be centrally compensated in the young but not old brain. Neurobiol. Aging 44, 173–184. doi: 10.1016/j.neurobiolaging.2016.05.001
Nayagam, B. A., Muniak, M. A., and Ryugo, D. K. (2011). The spiral ganglion: connecting the peripheral and central auditory systems. Hear. Res. 278, 2–20. doi: 10.1016/j.heares.2011.04.003
Nelson, D. I., Nelson, R. Y., Concha-Barrientos, M., and Fingerhut, M. (2005). The global burden of occupational noise-induced hearing loss. Am. J. Ind. Med. 48, 446–458. doi: 10.1002/ajim.20223
Otte, J., Schuknecht, H. F., and Kerr, A. G. (1978). Ganglion cell populations in normal and pathological human cochleae. Implications for cochlear implantation. Laryngoscope 8, 1231–1246. doi: 10.1288/00005537-197808000-00002
Ouda, L., Profant, O., and Syka, J. (2015). Age-related changes in the central auditory system. Cell Tissue Res. 361, 337–358. doi: 10.1007/s00441-014-2107-2
Oxenham, A. J. (2016). Predicting the perceptual consequences of hidden hearing loss. Trends Hear. 20, 1–6. doi: 10.1177/2331216516686768
Paquette, S. T., Gilels, F., and White, P. M. (2016). Noise exposure modulates cochlear inner hair cell ribbon volumes, correlating with changes in auditory measures in the FVB/nJ mouse. Sci. Rep. 6, 1–13. doi: 10.1038/srep25056
Paraouty, N., Ewert, S. D., Wallaert, N., and Lorenzi, C. (2016). Interactions between amplitude modulation and frequency modulation processing: Effects of age and hearing loss. J. Acoust. Soc. Am. 140, 121–131. doi: 10.1121/1.4955078
Parthasarathy, A., and Kujawa, S. G. (2018). Synaptopathy in the aging cochlea: Characterizing early-neural deficits in auditory temporal envelope processing. J. Neurosci. 38, 7108–7119. doi: 10.1523/JNEUROSCI.3240-17.2018
Patro, C., Kreft, H. A., and Wojtczak, M. (2021). The search for correlates of age-related cochlear synaptopathy: Measures of temporal envelope processing and spatial release from speech-on-speech masking. Hear. Res. 409, 108333. doi: 10.1016/j.heares.2021.108333
Paul, B. T., Bruce, I. C., and Roberts, L. E. (2017a). Evidence that hidden hearing loss underlies amplitude modulation encoding deficits in individuals with and without tinnitus. Hear. Res. 344, 170–182. doi: 10.1016/j.heares.2016.11.010
Paul, B. T., Bruce, I. C., and Roberts, L. E. (2018). Envelope following responses, noise exposure, and evidence of cochlear synaptopathy in humans: Correction and comment. J. Acoust. Soc. Am. 143, EL487–EL489. doi: 10.1121/1.5043082
Paul, B. T., Waheed, S., Bruce, I. C., and Roberts, L. E. (2017b). Subcortical amplitude modulation encoding deficits suggest evidence of cochlear synaptopathy in normal-hearing 18–19 year olds with higher lifetime noise exposure. J. Acoust. Soc. Am. 142, EL434–EL440. doi: 10.1121/1.5009603
Peineau, T., Belleudy, S., Pietropaolo, S., Bouleau, Y., and Dulon, D. (2021). Synaptic release potentiation at aging auditory ribbon synapses. Front. Aging Neurosci. 13, 1–20. doi: 10.3389/fnagi.2021.756449
Pichora-fuller, M. K., Schneider, B. A., and Daneman, M. (1995). How young and old adults listen to and remember speech in noise. J. Acoust. Soc. Am. 97, 593–608. doi: 10.1121/1.412282
Popelar, J., Groh, D., Pelánov,á, J., Canlon, B., and Syka, J. (2006). Age-related changes in cochlear and brainstem auditory functions in Fischer 344 rats. Neurobiol. Aging 27, 490–500. doi: 10.1016/j.neurobiolaging.2005.03.001
Prendergast, G., Couth, S., Millman, R. E., Guest, H., Kluk, K., Munro, K. J., et al. (2019). Effects of age and noise Exposure on proxy measures of cochlear synaptopathy. Trends Hear. 23, 1–16. doi: 10.1177/2331216519877301
Prendergast, G., Guest, H., Munro, K. J., Kluk, K., Léger, A., Hall, D. A., et al. (2017a). Effects of noise exposure on young adults with normal audiograms I: Electrophysiology. Hear. Res. 344, 68–81. doi: 10.1016/j.heares.2016.10.028
Prendergast, G., Millman, R. E., Guest, H., Munro, K. J., Kluk, K., Dewey, R. S., et al. (2017b). Effects of noise exposure on young adults with normal audiograms II: Behavioral measures. Hear. Res. 356, 74–86. doi: 10.1016/j.heares.2017.10.007
Prendergast, G., Tu, W., Guest, H., Millman, R. E., Kluk, K., Couth, S., et al. (2018). Supra-threshold auditory brainstem response amplitudes in humans: Test-retest reliability, electrode montage and noise exposure. Hear. Res. 364, 38–47. doi: 10.1016/j.heares.2018.04.002
Pyykkö, I., Toppila, E., Zou, J., and Kentala, E. (2007). Individual susceptibility to noise-induced hearing loss. Audiol. Med. 5, 41–53. doi: 10.1080/16513860601175998
Roberts, L. E., Paul, B. T., and Bruce, I. C. (2018). Erratum and comment: Envelope following responses in normal hearing and in tinnitus. Hear. Res. 361, 157–158. doi: 10.1016/j.heares.2018.01.011
Rohatgi, A. (2021). WebPlotDigitizer [WWW Document]. Available online at: https://automeris.io/WebPlotDigitizer (accessed Octomber 14, 2022).
Rowe, M. J. (1978). Normal variability of the brain-stem auditory evoked response in young and old adult subjects. Electroencephalogr. Clin. Neurophysiol. 44, 459–470. doi: 10.1016/0013-4694(78)90030-5
Salvi, R., Sun, W., Ding, D., Chen, G., Di, L.obarinas, E., Wang, J., et al. (2017). Inner hair cell loss disrupts hearing and cochlear function leading to sensory deprivation and enhanced central auditory gain. Front. Neurosci. 10, 1–14. doi: 10.3389/fnins.2016.00621
Schaette, R., and McAlpine, D. (2011). Tinnitus with a normal audiogram: Physiological evidence for hidden hearing loss and computational model. J. Neurosci. 31, 13452–13457. doi: 10.1523/JNEUROSCI.2156-11.2011
Schairer, K. S., Feeney, M. P., and Sanford, C. A. (2013). Acoustic reflex measurement. Ear. Hear. 34, 43S-47S. doi: 10.1097/AUD.0b013e31829c70d9
Schmiedt, R. A., Mills, J. H., and Boettcher, F. A. (1996). Age-related loss of activity of auditory-nerve fibers. J. Neurophysiol. 76, 2799–2803. doi: 10.1152/jn.1996.76.4.2799
Schoof, T., and Rosen, S. (2014). The role of auditory and cognitive factors in understanding speech in noise by normal-hearing older listeners. Front. Aging Neurosci. 6, 1–14. doi: 10.3389/fnagi.2014.00307
Schuknecht, H. F., and Gacek, M. R. (1993). Cochlear pathology in presbycusis. Ann. Otol. Rhinol. Laryngol. 102, 1–16. doi: 10.1177/00034894931020S101
Sergeyenko, Y., Lall, K., Liberman, M. C., and Kujawa, S. G. (2013). Age-related cochlear synaptopathy: An early-onset contributor to auditory functional decline. J. Neurosci. 33, 13686–13694. doi: 10.1523/JNEUROSCI.1783-13.2013
Shaheen, L. A., Valero, M. D., and Liberman, M. C. (2015). Towards a diagnosis of cochlear neuropathy with envelope following responses. J. Assoc. Res. Otolaryngol. 16, 727–745. doi: 10.1007/s10162-015-0539-3
Shehorn, J., Strelcyk, O., and Zahorik, P. (2020). Associations between speech recognition at high levels, the middle ear muscle reflex and noise exposure in individuals with normal audiograms. Hear. Res. 392, 1–11. doi: 10.1016/j.heares.2020.107982
Shone, G., Altschuler, R. A., Miller, J. M., and Nuttall, A. L. (1991). The effect of noise exposure on the aging ear. Hear. Res. 56, 173–178. doi: 10.1016/0378-5955(91)90167-8
Silman, S. (1979). The effects of aging on the stapedius reflex thresholds. J. Acoust. Soc. Am. 66, 735–738. doi: 10.1121/1.383675
Singer, W., Zuccotti, A., Jaumann, M., Lee, S. C., Panford-Walsh, R., Xiong, H., et al. (2013). Noise-induced inner hair cell ribbon loss disturbs central arc mobilization: A novel molecular paradigm for understanding tinnitus. Mol. Neurobiol. 47, 261–279. doi: 10.1007/s12035-012-8372-8
Skoe, E., and Tufts, J. (2018). Evidence of noise-induced subclinical hearing loss using auditory brainstem responses and objective measures of noise exposure in humans. Hear. Res. 361, 80–91. doi: 10.1016/j.heares.2018.01.005
Snell, K. B., Mapes, F. M., Hickman, E. D., and Frisina, D. R. (2002). Word recognition in competing babble and the effects of age, temporal processing, and absolute sensitivity. J. Acoust. Soc. Am. 112, 720–727. doi: 10.1121/1.1487841
Somma, G., Pietroiusti, A., Magrini, A., Coppeta, L., Ancona, C., Gardi, S., et al. (2008). Extended high-frequency audiometry and noise induced hearing loss in cement workers. Am. J. Ind. Med. 51, 452–462. doi: 10.1002/ajim.20580
Song, Q., Shen, P., Li, X., Shi, L., Liu, L., Wang, J., iping, Yu, Z., et al. (2016). Coding deficits in hidden hearing loss induced by noise: The nature and impacts. Sci. Rep. 6, 1–13. doi: 10.1038/srep25200
Stamper, G. C., and Johnson, T. (2015a). Auditory function in normal-hearing, noise-exposed human ears. Ear Hear. 36, 172–184. doi: 10.1097/AUD.0000000000000107
Stamper, G. C., and Johnson, T. (2015b). Letter to the editor: examination of potential sex onfluences in Stamper, G. C., and Johnson T.A. (2015). auditory function in normal-hearing, noise-exposed human ears, Ear Hear, 36, 172-184. Ear. Hear. 36, 738–740. doi: 10.1097/AUD.0000000000000228
Stelrnachowicz, P. G., Beauchaine, K. A., Kalberer, A., and Jesteadt, W. (1989). Normative thresholds in the 8- to 20-kHz range as a function of age. J. Acoust. Soc. Am. 86, 1384–1391. doi: 10.1121/1.398698
Stone, M. A., and Moore, B. C. J. (2014). Amplitude-modulation detection by recreational-noise-exposed humans with near-normal hearing thresholds and its medium-term progression. Hear. Res. 317, 50–62. doi: 10.1016/j.heares.2014.09.005
Stone, M. A., Moore, B. C. J., and Greenish, H. (2008). Discrimination of envelope statistics reveals evidence of sub-clinical hearing damage in a noise-exposed population with “normal” hearing thresholds. Int. J. Audiol. 47, 737–750. doi: 10.1080/14992020802290543
Suthakar, K., and Liberman, M. C. (2021). Auditory-nerve responses in mice with noise-induced cochlear synaptopathy. J. Neurophysiol. 126, 2027–2038. doi: 10.1152/jn.00342.2021
Thompson, D. J., Sills, J. A., Recke, K. R., and Bui, D. M. (1980). Acoustic reflex growth in the aging adult. J. Speech Hear. Res. doi: 10.1044/jshr.2302.405
Tsuji, J., and Liberman, M. C. (1997). Intracellular labeling of auditory nerve fibers in guinea pig: Central and peripheral projections. J. Comp. Neurol. 381, 188–202. doi: 10.1002/(SICI)1096-9861(19970505)381:2<188::AID-CNE6>3.0.CO;2-#
Unsal, S., Karatas, H., Kaya, M., Gumus, N. M., Temugan, E., Yuksel, M., et al. (2016). Evaluation of acoustic reflex and reflex decay tests in geriatric group. Turkish Arch. Otolaryngol. 54, 10–15. doi: 10.5152/tao.2016.1556
Valderrama, J. T., Beach, E. F., Yeend, I., Sharma, M., Van Dun, B., and Dillon, H. (2018). Effects of lifetime noise exposure on the middle-age human auditory brainstem response, tinnitus and speech-in-noise intelligibility. Hear. Res. 365, 36–48. doi: 10.1016/j.heares.2018.06.003
Valero, M. D., Burton, J. A., Hauser, S. N., Hackette, T. A., Ramachandran, R., and Liberman, M. C. (2017). Noise-induced cochlear synaptopathy in rhesus monkeys (Macaca mulatta). Hear. Res. 353, 213–223. doi: 10.1016/j.heares.2017.07.003
Valero, M. D., Hancock, K. E., and Liberman, M. C. (2016). The middle ear muscle reflex in the diagnosis of cochlear neuropathy. Hear. Res. 332, 29–38. doi: 10.1016/j.heares.2015.11.005
Valero, M. D., Hancock, K. E., Maison, S. F., and Liberman, M. C. (2018). Effects of cochlear synaptopathy on middle-ear muscle reflexes in unanesthetized mice. Hear. Res. 363, 109–118. doi: 10.1016/j.heares.2018.03.012
Vasilkov, V., Garrett, M., Mauermann, M., and Verhulst, S. (2021). Enhancing the sensitivity of the envelope-following response for cochlear synaptopathy screening in humans: The role of stimulus envelope. Hear. Res. 400, 1–17. doi: 10.1016/j.heares.2020.108132
Verhulst, S., Alto,è, A., and Vasilkov, V. (2018a). Computational modeling of the human auditory periphery: Auditory-nerve responses, evoked potentials and hearing loss. Hear. Res. 360, 55–75. doi: 10.1016/j.heares.2017.12.018
Verhulst, S., Ernst, F., Garrett, M., and Vasilkov, V. (2018b). Suprathreshold psychoacoustics and envelope-following response relations: Normal-hearing, synaptopathy and cochlear gain loss. Acta Acust. united with Acust. 104, 800–803. doi: 10.3813/AAA.919227
Verhulst, S., Jagadeesh, A., Mauermann, M., and Ernst, F. (2016). Individual differences in auditory brainstem response wave characteristics. Trends Hear. 20, 1–20. doi: 10.1177/2331216516672186
Vermeire, K., Knoop, A., Boel, C., Auwers, S., Schenus, L., Talaveron-rodriguez, M., et al. (2016). Speech recognition in noise by younger and older adults : effects of age, hearing loss, and temporal resolution. Ann. Otol. Rhinol. Laryngol. 125, 297–302. doi: 10.1177/0003489415611424
Versnel, H., Prijs, V. F., and Schoonhoven, R. (1990). Single-fibre responses to clicks in relationship to the compound action potential in the guinea pig. Hear. Res. 46, 147–160. doi: 10.1016/0378-5955(90)90145-F
Viana, L. M., O'Malley, J. T., Burgess, B. J., Jones, D. D., Oliveira, C. A. C. P., Santos, F., et al. (2015). Cochlear neuropathy in human presbycusis: Confocal analysis of hidden hearing loss in post-mortem tissue. Hear. Res. 327, 78–88. doi: 10.1016/j.heares.2015.04.014
Wallaert, N., Moore, B. C. J., and Lorenzi, C. (2016). Comparing the effects of age on amplitude modulation and frequency modulation detection. J. Acoust. Soc. Am. 139, 3088–3096. doi: 10.1121/1.4953019
Walton, J. P. (2010). Timing is everything: Temporal processing deficits in the aged auditory brainstem. Hear. Res. 264, 63–69. doi: 10.1016/j.heares.2010.03.002
Wang, Y., Hirose, K., and Liberman, M. C. (2002). Dynamics of noise-induced cellular injury and repair in the mouse cochlea. J. Assoc. Res. Otolaryngol. 3, 248–268. doi: 10.1007/s101620020028
Wang, Y., and Ren, C. (2012). Effects of repeated “benign” noise exposures in young cba mice: shedding light on age-related hearing loss. J. Assoc. Res. Otolaryngol. 13, 505–515. doi: 10.1007/s10162-012-0329-0
Wilson, R. H. (1981). The effects of aging on the magnitude of the acoustic reflex. J. Speech Hear. Res. 24, 406–14. doi: 10.1044/jshr.2403.406
Wojtczak, M., Beim, J. A., and Oxenham, A. J. (2017). Weak middle-ear-muscle reflex in humans with noise-induced tinnitus and normal hearing may reflect cochlear synaptopathy. eNeuro 4, 1–8. doi: 10.1523/ENEURO.0363-17.2017
Wu, P.-Z., O'Malley, J. T., de Gruttola, V., and Liberman, M. C. (2021). Primary neural degeneration in noise-exposed human cochleas: Correlations with outer hair cell loss and word-discrimination scores. J. Neurosci. 41, 4439–4447. doi: 10.1523/JNEUROSCI.3238-20.2021
Wu, P. Z., Liberman, L. D., Bennett, K., de Gruttola, V., O'Malley, J. T., and Liberman, M. C. (2019). Primary neural degeneration in the human cochlea: evidence for hidden hearing loss in the aging ear. Neuroscience 407, 8–20. doi: 10.1016/j.neuroscience.2018.07.053
Keywords: cochlear synaptopathy (CS), noise exposure, age-related hearing loss (ARHL), auditory brainstem response (ABR), summating potential to action potential ratio (SP:AP), envelope-following response (EFR), middle ear muscle reflex (MEMR), speech-perception-in-noise (SPiN)
Citation: Shehabi AM, Prendergast G and Plack CJ (2022) The Relative and Combined Effects of Noise Exposure and Aging on Auditory Peripheral Neural Deafferentation: A Narrative Review. Front. Aging Neurosci. 14:877588. doi: 10.3389/fnagi.2022.877588
Received: 16 February 2022; Accepted: 30 May 2022;
Published: 23 June 2022.
Edited by:
Yi Du, Institute of Psychology (CAS), ChinaReviewed by:
Naomi Bramhall, United States Department of Veterans Affairs, United StatesClaudio Grassi, Catholic University of the Sacred Heart, Italy
Copyright © 2022 Shehabi, Prendergast and Plack. This is an open-access article distributed under the terms of the Creative Commons Attribution License (CC BY). The use, distribution or reproduction in other forums is permitted, provided the original author(s) and the copyright owner(s) are credited and that the original publication in this journal is cited, in accordance with accepted academic practice. No use, distribution or reproduction is permitted which does not comply with these terms.
*Correspondence: Adnan M. Shehabi, adnan.shehabi@postgrad.manchester.ac.uk