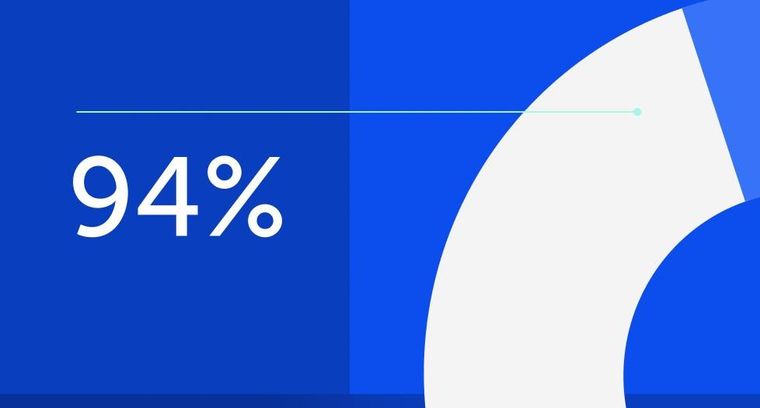
94% of researchers rate our articles as excellent or good
Learn more about the work of our research integrity team to safeguard the quality of each article we publish.
Find out more
REVIEW article
Front. Aging Neurosci., 17 February 2022
Sec. Cellular and Molecular Mechanisms of Brain-aging
Volume 14 - 2022 | https://doi.org/10.3389/fnagi.2022.814528
This article is part of the Research TopicCentral Nervous System: From Aging to Repair and RegenerationView all 10 articles
Age-related hearing loss (ARHL) is a common, increasing problem for older adults, affecting about 1 billion people by 2050. We aim to correlate the different reductions of hearing from cochlear hair cells (HCs), spiral ganglion neurons (SGNs), cochlear nuclei (CN), and superior olivary complex (SOC) with the analysis of various reasons for each one on the sensory deficit profiles. Outer HCs show a progressive loss in a basal-to-apical gradient, and inner HCs show a loss in a apex-to-base progression that results in ARHL at high frequencies after 70 years of age. In early neonates, SGNs innervation of cochlear HCs is maintained. Loss of SGNs results in a considerable decrease (~50% or more) of cochlear nuclei in neonates, though the loss is milder in older mice and humans. The dorsal cochlear nuclei (fusiform neurons) project directly to the inferior colliculi while most anterior cochlear nuclei reach the SOC. Reducing the number of neurons in the medial nucleus of the trapezoid body (MNTB) affects the interactions with the lateral superior olive to fine-tune ipsi- and contralateral projections that may remain normal in mice, possibly humans. The inferior colliculi receive direct cochlear fibers and second-order fibers from the superior olivary complex. Loss of the second-order fibers leads to hearing loss in mice and humans. Although ARHL may arise from many complex causes, HC degeneration remains the more significant problem of hearing restoration that would replace the cochlear implant. The review presents recent findings of older humans and mice with hearing loss.
Age-related hearing loss (ARHL) is a significant issue that leads to a reduced hearing perception in older humans. Half of the adult men over the age of 70 have ARHL (Roccio et al., 2020; Yamoah et al., 2020; Wu et al., 2021), which makes it a significant public health concern. The World Health Organization (WHO) predicts that there will be approximately 1 billion people over the age of 65 by the year 2050. Thus, ARHL is poised to be a substantial problem. ARHL involves a combination of conductive hearing loss (middle ear defects) and/or neurosensory hearing loss (loss of hair cells or neurons). It may also involve second-order neuron functional decline in the cochlear nuclei (CN) and associated superior olivary complex (SOC) (Makary et al., 2011; Lang, 2016; Caspary and Llano, 2018; Fattal et al., 2018; Dubno, 2019; Syka, 2020). Roughly 30% of women and men older than 65 have a reduced hearing sensitivity (Homans et al., 2017; Eggermont, 2019) affecting approximately 600 million people worldwide who may develop a progressive neurosensory hearing loss. Overall, one can imply that about 90% of people aged 90 years and older will eventually suffer from overt hearing loss unilaterally, affecting at least one ear (Sheffield and Smith, 2019). Aging also decreases sound localization accuracy and the ability to detect weak signals in noise.
Additionally, the repeated noise exposure begets noise-induced hearing loss (NIHL), which may exacerbate ARHL. These deficits may also indirectly affect cognition through decreased social activities and communication (Dubno, 2019; Eckert et al., 2019; Syka, 2020). A better understanding of the aging auditory system mechanisms and eventually replacing lost hair cells and sensory neurons to maintain hearing is required to help those with hearing replacement, including cochlear implants.
Currently, mouse models are the most common model of ARHL. The model appears to have limited transformation of supporting cells into HCs (Walters et al., 2017; Yamashita et al., 2018; Roccio et al., 2020; Li et al., 2020). In contrast, age-related hearing loss is understudied compared to earliest hair cell replacements (Zine et al., 2014, 2021; Devare et al., 2018; Yamoah et al., 2020). Notably, we realize that the stages of mouse development are different compared to humans (Lim and Brichta, 2016; Wang et al., 2018): a 2-year-old mouse is equivalent to a ~70-year-old human (Figure 1A). The corresponding human age to which hair cells are lost, leading to a particularly profound hearing loss in a mouse (Rauch et al., 2001; Kusunoki et al., 2004; Eggermont, 2019; Sheffield and Smith, 2019) depends upon the specific mouse strain (Zheng et al., 1999). Age-related hearing loss and changes in hearing of aged people have been identified at many levels across the entire auditory system, from the cochlear hair cells to the CN to the SOC. Reviews have reported central auditory dysfunction, including the downregulation of synaptic inhibition due to peripheral deafferentation and maladaptive plasticity. Aging-related decreases in synaptic inhibition (Stebbings et al., 2016; Caspary and Llano, 2018) may be triggered by high metabolic demands that lead to inhibitory neurons that being affected, while the relative to the decline of their excitatory counterparts (Kann et al., 2014), yielding an inhibitory-excitatory imbalance. Metabolic demands may be higher in the auditory brainstem, including the SOC, due to having high firing rates of neurons (Sanes and Rubel, 1988; Kujawa and Liberman, 2009), potentially making them more vulnerable to aging-related declines. Recent data suggest that lateral superior olivary neurons (LSON) are more resistant to metabolic stress than SOC overall (Brosel et al., 2018).
Figure 1. Panel (A) provides an overview of a 70-year-old human comparable to a 2-year-old mouse. The age group in humans displays profound hearing decay, shown as percent (%) of hearing loss (A, in red) follow-on hearing loss of 90-year-old humans. Limited restoration is attempted that has focused on 2-year or mostly younger mice, that is driven by the cost of keeping animals to old age and is providing a lack of decent models. Audiograms (B) and histology (C,D) demonstrated the loss of IHC (C), OHC (D), and the progressive threshold reduction in older people shown with the audiogram (B). Note that people exposed to noise have an additional loss in hair cells (C,D) and show a threshold reduction in the audiogram (B) in the middle age of 50–74 years old. In newborn mice, we demonstrate the phenotype of Atoh1 LacZ in control mice (E) Atoh1LacZ/LacZ null mice (F) is comparable (i.e., a single row of undifferentiated IHCs). Compared to Neurog1 null mice, a shorter cochlea has outliers in the GER area (G). Data were taken from the Jackson lab, WHF, and Fritzsch et al. (2005); Matei et al. (2005); Sheffield and Smith (2019); and Wu et al. (2021). Scale bars: 100 μm (E–G).
Hair cell loss is one of the early signs leading to the progressive worsening of the organ of Corti (Pauley et al., 2008; Taylor et al., 2012; Kersigo and Fritzsch, 2015; Herranen et al., 2020; Wu et al., 2021). The loss of spiral ganglion neurons (SGN), cochlear nuclei (AVCN, PVCN, DCN), and superior olivary complex (SOC) may follow or may occur in tandem. A common consequence of hair-cell loss is a flat epithelium (Shibata et al., 2010), which constitutes a predominated clinical situation of hearing loss that requires a future cure (Yamasoba et al., 2013; Yang et al., 2015; Homans et al., 2017; Revuelta et al., 2017; Dawson and Bowl, 2019; Dubno, 2019; Lopez-Juarez et al., 2019). Several studies have focused on various aspects of age-related hearing loss prevention, while others work toward the therapy (Schilder et al., 2019; Eshraghi et al., 2020; Roccio et al., 2020; Sekiya and Holley, 2021). For instance, cochlear implants (CI) are effectively used to functionally replace hair cells (Gantz et al., 2018, 2005). In addition, current approaches are attempting to define in detail the genetic basis of ARHL (Lewis M. A. et al., 2018). Improved hearing loss measurement (Simpson et al., 2019; Cassarly et al., 2020) will guide the restoration of hair cells to ameliorate hearing loss.
The presented review will describe mouse mutants to endorse a genetic model with a flat auditory epithelium and associated loss of sensory neurons of the ear and the brainstem. We aim through this approach to inspire innovative research and novel interventions by framing the hallmarks of aging (Campisi, 2013; López-Otín et al., 2013; Sekiya and Holley, 2021) to improve the upcoming translatability of present restoration challenges. Developing those leads to novel mouse models to deal with the strengths and weaknesses. It would be helpful to develop restoration strategies that are temporally mountable from postnatal to old age. Age-dependent dysregulation of the cochlear duct endolymph composition is a problem (Dubno, 2019) that aggravates neuronal loss (Lang, 2016; Elliott et al., 2021b) and are other avenues of investigation. Their possible restoration for hearing perfection is being explored (Gantz et al., 2018; Yamoah et al., 2020). We focus on hair cells, sensory neurons, cochlear nuclei, and the MOC innervation as late-age-related restoration targets.
Hair cells and supporting cells form the functional cellular assembly in the organ of Corti in the inner ear (Fritzsch et al., 2006; Groves and Fekete, 2017). In mice, hair-cells exit the cell cycle around embryonic days E12–14 in an apex to base progression (Ruben, 1967; Matei et al., 2005). In humans, this happens around gestational weeks GW12 (Locher et al., 2013). Many transcription factors and other genes are involved in cochlear hair cell development. For instance, Eya1/Six1 early gene expression (Zou et al., 2004; Ahmed et al., 2012; Li et al., 2020; Xu et al., 2021) is followed by Sox2 (Kiernan et al., 2005; Dvorakova et al., 2016, 2020). Other genes are needed such as Gata3 (Karis et al., 2001; Duncan and Fritzsch, 2013; Walters et al., 2017), Pax2 (Bouchard et al., 2010; Kempfle and Edge, 2014), Shh (Riccomagno et al., 2005; Muthu et al., 2019), and miR-183 (Pierce et al., 2008; Kersigo et al., 2011). In addition, we know of several Lim domain factors such as Isl1 (Huang et al., 2008; Chumak et al., 2016) and Lhx3 (Hertzano et al., 2007) that are needed for normal development of the cochlear hair cells. A unique loss of Lmx1a (Huang et al., 2008, 2018; Nichols et al., 2008, 2020) and its interaction with Lmx1b (Chizhikov et al., 2021) regulates the development of all cochlear hair cells, comparable to deletions of either Pax2 (Bouchard et al., 2010) or Shh (Muthu et al., 2019). Another subset of early transcription factors is known for proliferation, such as p27kip (Löwenheim et al., 1999; Zine et al., 2014), n-Myc (Kopecky et al., 2011), and Prox1 (Fritzsch et al., 2010).
Atoh1 expression is an essential early step of all inner ear hair cell differentiation (Bermingham et al., 1999; Fritzsch et al., 2005; Pan et al., 2012; Figures 1E–G). It is required for healthy sensory hair cell development in a cyclical sequential fashion (Fritzsch et al., 2006; Kageyama et al., 2019; Tateya et al., 2019). Atoh1 can induce the naïve epithelial cells into hair cells, but the viability of those cells is limited (Jahan et al., 2018; Atkinson et al., 2019). Beyond neonatal cell conversion (Mantela et al., 2005; Kelly et al., 2012; Koehler et al., 2017; McGovern et al., 2019) using pluripotent stem cells (van der Valk et al., 2021; Zine et al., 2021) to make novel cochlear hair cells that are inner- and outer hair cell-specific is beyond our current ability. Cellular deviations are regularly observed in aged materials and almost all aspects of the cell, including nuclear structure, cellular state, mitochondria, genetic material, and protein function that reduce the outer and inner hair cells with time (Kersigo and Fritzsch, 2015; Chessum et al., 2018; Wiwatpanit et al., 2018; Driver and Kelley, 2020; Filova et al., 2020; Herranen et al., 2020). Notably, several later transcription factors interact to maximize the generation of cochlear hair cells (Chen et al., 2021). Approximately 300 genes are identified and associated with ARHL (Bowl et al., 2017). TMC1/2 is vital for the normal formation of hearing function (Yoshimura et al., 2019; Erives and Fritzsch, 2020), as well as Cdh23 and Pcdh15, which together form the tip links of stereocilia (Elliott et al., 2018; Qiu and Müller, 2018).
Outer hair cells degenerate before inner hair cells in older humans and mice (Spongr et al., 1997; Kane et al., 2012). Specifically, early loss of OHC is pronounced in specific mutations, such as inactivated Manf or loss of Atg7 (Herranen et al., 2020; Perkins et al., 2020; Zhou et al., 2020). Loss of OHCs occurs at different degrees depending on mouse strains. For example, ~80% of OHCs are lost by 26 months of age in C57bl6 mice. In contrast, only ~50% are lost in CBA mice by 26 months. Indeed, in CBA strains, ~100% of hair cells are intact until 18 months. Among the first early loss that affects IHCs, are specific mutants such as Srrm3/4 (Nakano et al., 2012, 2020) followed by loss of Cdc42 (Ueyama et al., 2014) and Arhgef6 (Zhu et al., 2018). Loss of OHC, followed by IHC using a novel diphtheria toxin, was shown in postnatal mice (Tong et al., 2015).
In humans, a loss of OHCs is followed by IHCs that progresses around 65 years of age (Nadol Jr and Xu, 1992; Rauch et al., 2001; Liu et al., 2021). A most detailed description was recently provided that shows a comparable loss of OHCs and IHCs (Wu et al., 2021) that reduces IHCs ~50% in the base of ~75-year-old humans, compared to ~40% in the same region of OHCs (Figures 1C,D). Interestingly enough, in older people with noise-induced hearing loss (NIHL) and/or age-related hearing loss (ARHL), there is a progressive loss of OHC in the base. In contrast, the nearby IHCs remain near normal. Compared to the high-frequency loss, progressive ~50 dB SPL at about 75-year-old to ~60 dB SPL affects nearly 85-year-old or older humans (Figure 1B). Unfortunately, much of the current biological restorative strategies focus on the earliest induction of new hair cells, knowing well that the delayed loss of cochlear hair cells happens about 60 years later (Figure 1A).
Generating vestibular and cochlear hair cells from human pluripotent stem cells have begun the earliest major steps, as mentioned above (Koehler et al., 2017; Lahlou et al., 2018; Patel et al., 2018; Romano et al., 2021; van der Valk et al., 2021; Zine et al., 2021). To date, no one has succeeded in producing both types of the organ of Corti sensory hair cells in vitro in the correct proportion and disparity distribution needed for the restoration of hair cells within the organ of Corti. Many issues remain open, but we have no solution at hand, turning the feasibility of producing hair cells with following repopulation of the damaged organ of Corti into a future option (Liu et al., 2021). Restoration of the flat auditory non-sensory epithelium between the lingering patches of hair cells (Pirvola et al., 2002; Kiernan et al., 2005; Soukup et al., 2009; Pan et al., 2012; Duncan and Fritzsch, 2013; Herranen et al., 2020) also requires restoration of innervation to the newly molded hair cells. Concentrating on the proliferation and transdifferentiating of undistinguishable epithelial cells of the flat epithelium requires several steps that need to be taken before precursor cells can respond with genes like Sox2 and Atoh1 (Dabdoub et al., 2008; Nyberg et al., 2019; Dvorakova et al., 2020; Yamoah et al., 2020). Appropriate hair cell differentiation in the aging cochlea is the prime objective of hair cell regeneration. Artificial gene governing networks (Ausländer et al., 2012; Sedlmayer et al., 2018; Xie and Fussenegger, 2018; Krawczyk et al., 2020) can be used to drive Sox2 and Atoh1 in an oscillatory dynamic manner in BMP4-positive cells. We predict a cargo load of 25 kb is required for this oscillatory gene regulation, well within the choice of the helper-dependent adenovirus (HdAd; 37 kb). It assumes that BMP4 expressing cells can activate and the generation of the LuxR protein will enable the activation of the LuxI, AiiA, and Sox2 proteins. Oscillation will initiate the level of Sox2 gene expression, activating Atoh1 gene (Neves et al., 2012; Alonso et al., 2018). The expression of the Atoh1 protein can act as positive feedback on its enhancer (Pan et al., 2012) and can provide a negative feedback loop back onto Sox2 (Dabdoub et al., 2008; Kempfle et al., 2016; Yamoah et al., 2020). Once hair cell differentiation has initiated, BMP4 expression can be reduced using a negative feedback loop (Lewis R. M. et al., 2018). Eventually, the gene regulation system will shut down after time, leaving feedback loops in the differentiating hair cells to complete the process. Hair cell formation is required to be induced in all age and classes of mice, from young to adult, requiring a scalable expression to take advantage of using synthetic biology to directly repopulate the flat epithelium from undifferentiated supporting cells into cochlear hair cells.
In summary, the progressive loss of cochlear hair cells depends on a large number of genes (Liu et al., 2021) that results in the flat auditory epithelium and requires a re-establishment of normal hair cells for hearing restoration. Alternative approaches, such as incorporating induced hair cells into the cochlea, are challenging. In this case, the hair cells would die once they are exposed to the endolymphatic fluid containing high potassium levels (~140 mM). Early development of hair cells is possible, but the induction of novel hair cells to replace lost hair cells in old mice and humans remains a future biological treatment option.
The sensory neurons of the cochlea, the spiral ganglion neurons (SGNs), depend on Neurog1 (Ma et al., 1998, 2000; Elliott et al., 2021b) and are myelinated by Schwann cells (Mao et al., 2014; Kersigo et al., 2021). Neurons begin proliferation in a base-to-apex progression around embryonic days E10–12 in mice (Ruben, 1967; Matei et al., 2005), equivalent to gestational week (GW10) in humans (Locher et al., 2013). Central fibers reach the cochlear nuclei at about E12.5, followed by peripheral innervation of hair cells at E14.5 (Yang et al., 2011; Schmidt and Fritzsch, 2019).
The genes, Eya1 (Xu et al., 2021), Sox2 (Dvorakova et al., 2020), Shh (Muthu et al., 2019), Pax2 (Bouchard et al., 2010), Lmx1a/b (Chizhikov et al., 2021), Dicer/miR-183 (Kersigo et al., 2011), Fgf7/10 (Pauley et al., 2003; Urness et al., 2018), and Neurog1 (Ma et al., 1998, 2000; Elliott et al., 2021b), among others, are required to induce the formation of spiral ganglion neurons, as well as cochlear nuclei. Downstream of Neurog1, a subset of transcription factors are upregulated to promote SGN differentiation, including Neurod1 (Jahan et al., 2010a; Macova et al., 2019), Pou4f1 (Huang et al., 2001), Gfi1 (Matern et al., 2020), Isl1 (Radde-Gallwitz et al., 2004; Chumak et al., 2016), and others. Additional factors are also required to maintain spiral ganglion neurons as they project to the cochlear nuclei (Brooks et al., 2020). Expression of the neurotrophins, Bdnf and Ntf3, are needed for maintaining SGNs (Yang et al., 2011; Fritzsch et al., 2016), and in their absence, no SGNs survive. In the absence of SGNS, the ear initially develops near normally; however, after approximately 2 months, cochlear hair cells degenerate (Kersigo and Fritzsch, 2015). In addition, an early loss of SGNs results in a reduced number of cochlear nuclei neurons (Figures 2B,C) (Dvorakova et al., 2020; Filova et al., 2020), consistent with an early loss in chicken (Levi-Montalcini, 1949). However, unlike in neonates, loss of the ear has less of an effect on cochlear nuclei survival in older mice, suggesting a critical window (Rubel and Fritzsch, 2002; Harris and Rubel, 2006). Our recent data show that vestibular afferents from transplanted mouse ears can innervate the vestibular nuclei of chickens, thus providing a novel approach to eventually replace ears (Elliott and Fritzsch, 2018). Currently, though, cochlear implants are the only means to restore hearing following a sensorineural hearing loss, so long as enough SGNs survive in humans to transmit the sound stimulation from the ear to the cochlear nucleus (Gantz et al., 2005, 2018). In cats, stimulating the ears will reform the cochlear nuclei and develop large endbulbs of Held (Ryugo et al., 2005) and this likely also happens in humans (Kral et al., 2013). Interestingly enough, a set of miR’s show a progressive reduction in cochlear nuclei (Krohs et al., 2021) that acts in parallel to Dicer dependent mutations (Kersigo et al., 2011).
Figure 2. A loss of neurons with time reduces the ~ 28,000 SGNs in ~40-year-old humans to ~25% in 80-year-old humans (A, purple). A similar loss is shown for ~1,300 SGNs and reduces to ~40 in 18 month-old mice (A, green). Immunoreaction of labeling for neuron markers validates the inner ear afferents in control mice (B,B’). This is compared with Atoh-cre;Atoh1f/f ‘self-termination mice (C), Bronx waltzer (bv/bv) (D), Atoh-cre;Atoh1ki/f (E), and Pax2-cre;Neurod1 (F). Central projections reach the anterior (AVCN) and dorsal (DCN) cochlear compared to controls (G) and Atoh1 CKO mice (H) that show a tonotopic organization of SGN central projection, despite the absence of cochlear hair cells (H’)—modified after Nadol Jr (1990); White et al. (2000); Jahan et al. (2018); and Filova et al. (2020). Scale bars: 10 μm (B–F) and 100 μm (G–H).
The development of SGNs is independent of both cochlear nuclei and cochlear hair cells (Rask-Andersen et al., 2005). Studying the growth and differentiation of SGNs could inform the steps needed to induce otic progenitors to replace lost neurons (Song et al., 2017, 2019; Karlsson et al., 2019). SGN projections follow a simple tonotopic organization (Figures 2G,H,H’): basal projection of the cochlea reaches the most dorsal part of the cochlear nuclei, whereas the apex reaches the ventral aspect of the cochlear nuclei (Muniak et al., 2016; Fritzsch et al., 2019). Following the loss of Neurod1, there is a reduction in the number of SGNs and a reorganization of these neurons that result in mixed innervation of auditory and vestibular targets and also a loss of tonotopic organization in the cochlear nuclei (Macova et al., 2019; Filova et al., 2020). A somewhat similar reorganization results from the loss of Nrp2 that shows a disorganized central cochlear projection (Lu et al., 2014; Schmidt and Fritzsch, 2019). Interestingly, SGNs project to the cochlear nuclei in the absence of Atoh1 (Fritzsch et al., 2005) where they maintain their tonotopic representation of the periphery (Elliott et al., 2017). In addition, SGNs project peripherally in the absence of Atoh1 and Pou4f1 (Xiang et al., 2003) to reach the flat epithelium in the absence of cochlear hair cells (Pauley et al., 2008). The latter demonstrates that SGNs form in the absence of hair cells, suggesting long-term retention of the ’de-innervated’ neurons (Figure 2A) in humans (Nadol Jr, 1990; Nadol Jr and Xu, 1992), cats (Stakhovskaya et al., 2008), and mice (White et al., 2000) whereas neurons of rats degenerate faster within months after the loss of all hair cells (Alam et al., 2007).
Two types of SGNs neurons are known, type I and type II, to innervate the inner hair cells (Petitpré et al., 2018; Shrestha et al., 2018; Sun et al., 2021) and the outer hair cells (Shrestha and Goodrich, 2019; Elliott et al., 2021a), respectively. Innervation of the hair cells occurs in a progression, starting at E18 (Fritzsch et al., 1997) and finishing at about P14 (Petitpré et al., 2018; Shrestha and Goodrich, 2019). In the absence of cochlear hair cells, projecting peripheral neurites of SGNs reach out (Xiang et al., 2003; Fritzsch et al., 2005; Pauley et al., 2008; Pan et al., 2012; Tong et al., 2015), but do not expand beyond the flat auditory epithelium (Shibata et al., 2010). As mentioned above, there is a reduced number of neurons after Neurod1 deletion and an effect on hair cells in the cochlear apex (Jahan et al., 2010b; Macova et al., 2019). In addition, loss of Neurod1 results in the conversion of some neurons into hair cells after upregulation of Atoh1 (Elliott et al., 2021b). Furthermore, in Neurod1 mutant mice, some OHC are converted into IHC-like cells (Figure 2F) and are innervated by putative type I SGNs (Jahan et al., 2018; Filova et al., 2020). Reorganization of innervation has been shown in Srrm4 mutant mice. In these mice, IHCs are primarily absent (Figure 2D), and type I SGNs innervate the OHCs instead can enforce some of the type I to innervate IHC instead of OHC (Figures 2B,C) (Nakano et al., 2012; Jahan et al., 2018). Replacement of one allele of Atoh1 with Neurog1, in which the other Atoh1 allele is conditionally knocked out, can lead to the formation of near-normal hair cells (Figure 2E) that also receive innervation (Jahan et al., 2015). A unique defect of SGNs is observed at a certain reduction level of specific stimulation in mice (Kujawa and Liberman, 2009) that requires additional information in humans (Wu et al., 2021).
In summary, SGNs project centrally to the cochlear nucleus and peripherally to the hair cells. SGNs show a tonotopic organization to the cochlear nuclei even in the absence of hair cells, which is beneficial for cochlear implants. Likewise, in the absence of hair cells, long-term retention of SGNs is demonstrated and ramified within the “flat auditory epithelium” that could possibly be re-connected with novel hair cells in the near future.
The cochlear nucleus is the first information processing center for auditory information within the central nervous system and provides output to the superior olivary cells (SOC) and the inferior colliculi (IC) (Malmierca and Ryugo, 2011; Oertel and Cao, 2020). It comprises three subnuclei: anteroventral cochlear nucleus (AVCN) from rhombomere 2 and 3 (r2, 3), posteroventral cochlear nucleus (PVCN; r4), and dorsal cochlear nucleus (DCN, r5). Most cochlear nuclei neurons exit the cell cycle around E10–14 except for the delayed formation of granule cells that finish at E18 in mice (Pierce, 1967). Atoh1 is a major bHLH gene that defines the cochlear nuclei. It is expressed in regions of the rhombic lip of the hindbrain that contribute cells to the cochlear nucleus. Conditional deletion of Atoh1 in r3/5 impaired cochlear nucleus formation in those regions (Maricich et al., 2009)., without Atoh1, there is no formation of cochlear nuclei (Wang et al., 2005; Fritzsch et al., 2006; Ray and Dymecki, 2009). However, as mentioned earlier, in the absence of Atoh1, a near-normal central projection develops (Figures 3E,F) of SGNs (Elliott et al., 2017). Notably, a delayed loss of Atoh1 can be driven by Pax2 (Figures 3C,D). Upstream of Atoh1 is the expression of Lmx1a/b (Chizhikov et al., 2021). Without expression of Lmx1a/b, there is a loss of Gdf7 (Lee et al., 2000), Atoh1, and Wnt3a expression, and there is no formation of the choroid plexus (Chizhikov et al., 2021; Elliott et al., 2021b). In addition, without Lmx1a/b, all aspects of the cochlea are eliminated beyond an undifferentiated sac, including no differentiation of cochlear hair cells or SGNs (Figures 3A,B). Additional transcription factors have been identified for cochlear nuclei formation (Milinkeviciute and Cramer, 2020). For instance, Hoxb1 is expressed in r4 in the PVCN and parts of the DCN (Di Bonito et al., 2017).
Figure 3. Cochlear nuclei depend on Lmx1a/b, upstream of Gdf7, Atoh1, and Wnt3a (A). In the absence of Lmx1a/b neither cochlear hair cells, spiral ganglion neurons, nor cochlear nuclei develop (B). The choroid plexus is replaced by a roof plate that allows fibers to cross to the contralateral side (A,B). Cochlear nuclei are smaller in a conditional deletion of Atoh1 using Pax2-cre (C,D). In Atoh1-null mice, there is a normal central projection of SGNs without either peripheral hair cells or cochlear nuclei (E,F). Expression of Parvalbumin, Calbindin and Calretinin showing upregulation in the cochlear nuclei at the level of DCN and PVCN areas that increase in some but not all neurons (G). Modified after Idrizbegovic et al. (2006); Elliott et al. (2017); Elliott et al. (2021b); and Chizhikov et al. (2021). Scale bars: 100 μm (C–F). *** indicates significance.
The cochlear nuclei have defined numerous cell types based on morphological and physiological criteria (Oertel and Cao, 2020). Synapses from type I SGNs onto bushy cells in the AVCN have structural adaptations that receive the endbulbs of Held; the endbulb of Held permits highly secure signaling between the auditory periphery of SGN and bushy cells (Manis et al., 2012; Caspary and Llano, 2018; Syka, 2020; Wang et al., 2021). Losing proper auditory innervation that leads to a simplification of the endbulb termination (Muniak et al., 2016). Local inhibitory cells in the cochlear nucleus are known as either glycinergic (e.g., D-multipolar, vertical, and cartwheel) or as GABAergic [stellate and Golgi; (Young and Oertel, 1998; Oertel and Cao, 2020)] that receive the SGN projections. Genomically are clustered as miR-183 and miR-96 that reduces the volumes of auditory hindbrain nuclei. Electrophysiological analysis shows that the calyx of Held synapses on the medial nucleus of the trapezoid body (MNTB) demonstrated strongly altered synaptic transmission in young-adult mice (Krohs et al., 2021).
Aging-related changes are known to a certain extent, followed in neuronal morphology and biochemistry in the cochlear nucleus and SOC. The size of the AVCN remains constant with aging, whereas its cell density decreases with age. Moreover, cellular density correlates with aging-related loss of cochlear sensory neuron input. In addition, the cellular density diminishes by about 15% at 7 months of age in certain mice (C57BL/6). Still, it does not show a reduction of cellular density until the 2nd year of life in CBA/CaJ mice (Willott et al., 1987). The reduction of cellular density is complemented by a drop in the size of synaptic terminals by AVCN neurons (Briner and Willott, 1989; Helfert et al., 2003). The volume of the DCN and cell number and size of aging-related decreases have been detected in C57BL/J, suggesting that the losses are related to hearing loss. Changes have been detected in the octopus cell area of the PVCN. Interestingly enough, changes seen in both C57BL/J and CBA/CaJ mice indicate a different aging-related effect (Willott and Bross, 1990; Jalenques et al., 1995). The morphology of the cochlear nucleus in humans with presbycusis suggests an increase in the total cell number and number of multipolar and granule neurons in the cochlear nucleus with aging (Hinojosa and Nelson, 2011), a contradictory result that requires additional work (Syka, 2020).
The calcium-binding proteins parvalbumin, calbindin, and calretinin play a leading role in buffering intracellular calcium during cellular stress in all cochlear nuclei (Lohmann and Friauf, 1996). For example, calretinin-, calbindin-, and parvalbumin-positive cells are perceived in the DCN and PVCN of aged mice; this increase of the three proteins is correlated with hair cell loss (Idrizbegovic et al., 2003; Caspary and Llano, 2018). Recent data suggest the total number of neurons in the DCN and PVCN is decreasing while the proportion of parvalbumin- and calretinin-positive neurons (Figure 3G) is increasing (Idrizbegovic et al., 2006; Syka, 2020). Hearing loss goes beyond the three proteins that are the only factor in these increases. Moreover, induced hearing loss may cause a lessened effect of calretinin-positive neurons in layer III of the aged DCN, innervating the fusiform neurons (Zettel et al., 2003; Syka, 2020) in the human cochlear nucleus. Note that all three calcium-binding proteins decrease progressively from adult to old age (Sharma et al., 2014). The differences in intracellular calcium mobilization may play a role to confirm the differences between the human and mouse data (Ibrahim and Llano, 2019).
Changes with aging in the cochlear nucleus are known to degrade the spiking activity in opposition to temporally patterned impetuses. The Fischer 344 rat showed aging-related decreases in glycine binding sites. In addition, receptor subunits in the cochlear nucleus are declining (Wang et al., 2009; Caspary and Llano, 2018). Reduction and loss of inhibition are most likely mirrored in cochlear nucleus neurons’ firing possessions. Thus, cartwheel and fusiform cells show increased acoustically driven responses in the aging cochlear nucleus. This activity will reflect the loss of inhibition (Caspary et al., 2006; Caspary and Llano, 2018). Physiologically, numerous studies show the temporal fidelity that breaks down at the synapse between cochlear nerve fibers and bushy cells in old mice (Xie, 2016; Xie and Manis, 2017; Wang et al., 2021). These data suggest multiple instruments may be responsible for the aging-related temporal spreading of cochlear nucleus neuronal responses from temporally patterned sounds. Moreover, the first longitudinal investigation of different type Ia is positive for calretinin and innervates the bushy neurons.
In summary, we show that cochlear nuclei depend on Lmx1a/b and Atoh1 and require further studies with heterozygotes to identify the long-term defects in mice. Preliminary data suggest a distinct effect of upregulation of parvalbumin and calretinin that could upregulate the relative calbindin expression and suggest a long-term reduction of calretinin that innervates the bushy cells. No new cochlear nuclei are needed as they survive in the absence of SGNs.
The superior olivary complex (SOC) is the second major station in the ascending auditory pathway, which consists of the first input at which information from the two ears is combined for binaural information in the central auditory system. The SOC nuclei involved in processing ascending information are the medial superior olive (MSO), lateral superior olive (LSO), and medial nucleus of the trapezoid body (MNTB; Marrs et al., 2013). These three major SOC nuclei play a critical role in sound localization ability, which is known to diminish with age (Caspary and Llano, 2018; Syka, 2020). In mice, neurons are born between embryonic days E9–14 with the latest differentiated neurons in the LSO (Pierce, 1973).
Recent data suggest that the SOC complex is generated in part by dorsal neurons from Atoh1-positive cells that identify their contribution to distinct migrating SOC cells (Maricich et al., 2009; Marrs et al., 2013; Di Bonito et al., 2017; Lipovsek and Wingate, 2018). In addition to Atoh1, there is a combined expression of Wnt3a and En1 (Jalabi et al., 2013; Altieri et al., 2015; Chizhikov et al., 2021) that are important for the SOC. A noticeable difference is in the SOC of the chicken that generates more caudal nuclei (Lipovsek and Wingate, 2018). A clear progression in Calbindin, Calretinin, and Parvalbumin expression that require a generation of SOCs is demonstrated by tracing and immunological data (Lohmann and Friauf, 1996; Kandler et al., 2020).
Very few studies have investigated variations in the MSO, LSO, and MNTB, particularly with aging. For example, Godfrey et al. found that there were no changes in the dry weight of the superior olive complex, but that was significantly reduced in glutamate, glycine, and GABA (Godfrey et al., 2017). Moreover, there is a reduction of about 25% in the level of neurotransmitters with aging in the auditory system. The size of glycinergic and GABAergic cell size has become smaller in the LSO of gerbils of aging (Gleich et al., 2004). Furthermore, decreases in synaptic terminals have been reduced in MNTB in Sprague-Dawley rat with age (Casey and Feldman, 1982, 1985, 1988). An increase in parvalbumin staining in the MSO, but not LSO or MNTB, is reported in primates with aging (Gray et al., 2013). Changes seem to correlate with deviations in peripheral hearing, implying that parvalbumin upregulation may be a part of the mechanism to compensate for the loss of peripheral input from neurons. Beyond significant changes in the synaptic organization in superior olives that have provided few aging-related physiological changes in vivo compared with changes in the ABR threshold (Ibrahim and Llano, 2019; Syka, 2020). Older miR-183/96 require studying in much older mice to study the long-term defects (Krohs et al., 2021).
Our initial set of calretinin and calbindin data suggests a slight reduction in the number of MNTB cells (Figure 4) consistent with data in rats (Casey and Feldman, 1988). Using a gene regulation of two distinct nuclei showing a fast up- and down-regulation for three nuclei that are all positive for calbindin (Figures 4A–C). Whereas the MNTB is calbindin-positive early and keeps this expression, the SPN and LSO show a strong early up-regulation of calbindin followed by near regular expression of calbindin at 1 year of age. An interesting size of MNTB differs between 1 year-old mice: more significant more lateral neurons are becoming more smaller in the medial MNTB (Figures 4C,C””). Individual neurons can be traced by calretinin to form a calyx of Held (Figure 4C”) that resembles the endbulb of Held of bushy cells (Wang et al., 2021). While all calyx receives innervation from the contralateral cochlea, the size of innervation suggests that smaller MNTB neurons have fewer calyces (Figures 4C”’,C””). Further work is needed to detail the loss in mice using calretinin, calbindin, and parvalbumin to investigate the effects in mice 24 months or older.
Figure 4. Early expression of Calbindin (Calb1) and Calretinin (Calb2) expression in the MNTB at P8 (A,A’). Calb1 expression increases in the LSO by P46 (B,B’). At 1 year of age, there is minimal Calb1 expression in the LSO and SPN. Calb2-positive fibers form a calyx of Held in the MNTB (C–C””). Within the MNTB, there are larger Calb1-positive cells close to the cochlear nucleus (~229 μm2) compared to much smaller cells closer to the midline (~132 μm2). Our expression shows upregulation of Calb2 in the MNTB (compare P8 with P46) that progressively upregulates with Calb1. Note that fewer MNTB are shown in a single section that likely comes about through lateral distributions. P: postnatal, Bar indicates 100 μm in (A–C,C’), 10 μm in (C”’,C””).
In summary, we know that the migrated SOC nuclei are derived from the dorsal rhombomere. Our preliminary set of calretinin and calbindin data suggest that different complexity and size of MNTB neurons correlate with the size of neurons with aging.
Our overview shows the earliest loss of cochlear hair cells, spiral ganglion neurons, cochlear nuclei, and associated superior olivary complex: Nearly all four auditory systems depend on Lmx1a/b, Atoh1, and Neurog1 expression. Later, a progressive loss of hair cells occurs with aging, followed by a spiral ganglion neuron reduction to ~40% in humans and mice. In contrast, early losses of cochlear nuclei have been well described, showing changes in different proteins for survival. How much of the changes are related to compensation for peripheral hearing loss remains an open question, and the impact of adaptation on sound perception remains a subject for future investigations. Auditory brainstem neurons may be less vulnerable to oxidative stress, which may have high metabolic demands based on neurons’ high firing rates. Thus, different mechanisms may interpret for aging declines, in particular, inhibition that affects the auditory pathway. We suggest that the loss of inhibition may, in part, be responsible for temporal fidelity or may be affected by sound localization or may produce dysregulated plasticity. Future work will be of help to explain the causes of changes of aging that ultimately affect the central auditory system that will lead to novel therapeutic objectives to perfect such changes by adding new hair cells and connecting them with remaining SGNs to connect the cochlear nuclei. How much cochlear nuclei and SOC and IC neurons can plastically revert in sizes and complexity of innervation remains to be seen. Additional investigations to uncover the complex reasons for changes in the central auditory system will contribute to developing new healing targets to alleviate sensory ARHL and associated cognitive decline.
This work was conceived by AZ and BF, final revision was drafted by KE, ENY and BF, reviewed by AZ and ENY. All authors edited the final draft in collaboration and approved the submitted version. All authors contributed to the article and approved the submitted version.
Grants to ENY and BF supported this work from the National Institutes of Health (DC016099, DC015252, DC015135, AG060504, AG051443).
The authors declare that the research was conducted in the absence of any commercial or financial relationships that could be construed as a potential conflict of interest.
All claims expressed in this article are solely those of the authors and do not necessarily represent those of their affiliated organizations, or those of the publisher, the editors and the reviewers. Any product that may be evaluated in this article, or claim that may be made by its manufacturer, is not guaranteed or endorsed by the publisher.
Ahmed, M., Wong, E. Y., Sun, J., Xu, J., Wang, F., and Xu, P.-X. (2012). Eya1-Six1 interaction is sufficient to induce hair cell fate in the cochlea by activating Atoh1 expression in cooperation with Sox2. Dev. Cell 22, 377–390. doi: 10.1016/j.devcel.2011.12.006
Alam, S. A., Robinson, B. K., Huang, J., and Green, S.H. (2007). Prosurvival and proapoptotic intracellular signaling in rat spiral ganglion neurons in vivo after the loss of hair cells. J. Comp. Neurol. 503, 832–852. doi: 10.1002/cne.21430
Alonso, M. B. D., Hernandez, I. L., de la Fuente, M. A., Garcia-Sancho, J., Giraldez, F., and Schimmang, T. (2018). Transcription factor induced conversion of human fibroblasts towards the hair cell lineage. PLoS One 13:e0200210. doi: 10.1371/journal.pone.0200210
Altieri, S. C., Jalabi, W., Zhao, T., Romito-DiGiacomo, R. R., and Maricich, S. M. (2015). En1 directs superior olivary complex neuron positioning, survival and expression of FoxP1. Dev. Biol. 408, 99–108. doi: 10.1016/j.ydbio.2015.10.008
Atkinson, P. J., Kim, G. S., and Cheng, A. G. (2019). Direct cellular reprogramming and inner ear regeneration. Expert Opin. Biol. Ther. 19, 129–139. doi: 10.1080/14712598.2019.1564035
Ausländer, S., Ausländer, D., Müller, M., Wieland, M., and Fussenegger, M. (2012). Programmable single-cell mammalian biocomputers. Nature 487, 123–127. doi: 10.1038/nature11149
Bermingham, N. A., Hassan, B. A., Price, S. D., Vollrath, M. A., Ben-Arie, N., Eatock, R. A., et al. (1999). Math1: an essential gene for the generation of inner ear hair cells. Science 284, 1837–1841. doi: 10.1126/science.284.5421.1837
Bouchard, M., de Caprona, D., Busslinger, M., Xu, P., and Fritzsch, B. (2010). Pax2 and Pax8 cooperate in mouse inner ear morphogenesis and innervation. BMC Dev. Biol. 10:89. doi: 10.1186/1471-213X-10-89
Bowl, M. R., Simon, M. M., Ingham, N. J., Greenaway, S., Santos, L., Cater, H., et al. (2017). A large scale hearing loss screen reveals an extensive unexplored genetic landscape for auditory dysfunction. Nat. Commun. 8:886. doi: 10.1038/s41467-017-00595-4
Briner, W., and Willott, J. F. (1989). Ultrastructural features of neurons in the C57BL/6J mouse anteroventral cochlear nucleus: young mice versus old mice with chronic presbycusis. Neurobiol. Aging 10, 295–303. doi: 10.1016/0197-4580(89)90039-0
Brooks, P. M., Rose, K. P., MacRae, M. L., Rangoussis, K. M., Gurjar, M., Hertzano, R., et al. (2020). Pou3f4-expressing otic mesenchyme cells promote spiral ganglion neuron survival in the postnatal mouse cochlea. J. Comp. Neurol. 528, 1967–1985. doi: 10.1002/cne.24867
Brosel, S., Grothe, B., and Kunz, L. (2018). An auditory brainstem nucleus as a model system for neuronal metabolic demands. Eur. J. Neurosci. 47, 222–235. doi: 10.1111/ejn.13789
Campisi, J. (2013). Aging, cellular senescence and cancer. Ann. Rev. Physiol. 75, 685–705. doi: 10.1146/annurev-physiol-030212-183653
Casey, M., and Feldman, M. (1988). Age-related loss of synaptic terminals in the rat medial nucleus of the trapezoid body. Neuroscience 24, 189–194. doi: 10.1016/0306-4522(88)90322-3
Casey, M. A., and Feldman, M. L. (1982). Aging in the rat medial nucleus of the trapezoid body I. Light microscopy. Neurobiol. Aging 3, 187–195. doi: 10.1016/0197-4580(82)90039-2
Casey, M. A., and Feldman, M. L. (1985). Aging in the rat medial nucleus of the trapezoid body. II. Electron microscopy. J. Comp. Neurol. 232, 401–413. doi: 10.1002/cne.902320311
Caspary, D. M., Hughes, L. F., Schatteman, T. A., and Turner, J. G. (2006). Age-related changes in the response properties of cartwheel cells in rat dorsal cochlear nucleus. Hear. Res. 216, 207–215. doi: 10.1016/j.heares.2006.03.005
Caspary, D. M., and Llano, D. A. (2018). “Aging processes in the subcortical auditory system,” in The Oxford Handbook of the Auditory Brainstem, ed K. Kandler (London: Oxford University Press), 639–679. doi: 10.1093/oxfordhb/9780190849061.013.16
Cassarly, C., Matthews, L. J., Simpson, A. N., and Dubno, J. R. (2020). The revised hearing handicap inventory and screening tool based on psychometric reevaluation of the hearing handicap inventories for the elderly and adults. Ear Hear. 41, 95–105. doi: 10.1097/AUD.0000000000000746
Chen, Y., Gu, Y., Li, Y., Li, G.-L., Chai, R., Li, W., et al. (2021). Generation of mature and functional hair cells by co-expression of Gfi1, Pou4f3 and Atoh1 in the postnatal mouse cochlea. Cell Rep. 35:109016. doi: 10.1016/j.celrep.2021.109016
Chessum, L., Matern, M. S., Kelly, M. C., Johnson, S. L., Ogawa, Y., Milon, B., et al. (2018). Helios is a key transcriptional regulator of outer hair cell maturation. Nature 563, 696–700. doi: 10.1038/s41586-018-0728-4
Chizhikov, V. V., Iskusnykh, I. Y., Fattakhov, N., and Fritzsch, B. (2021). Lmx1a and Lmx1b are redundantly required for the development of multiple components of the mammalian auditory system. Neuroscience 452, 247–264. doi: 10.1016/j.neuroscience.2020.11.013
Chumak, T., Bohuslavova, R., Macova, I., Dodd, N., Buckiova, D., Fritzsch, B., et al. (2016). Deterioration of the medial olivocochlear efferent system accelerates age-related hearing loss in Pax2-Isl1 transgenic mice. Mol. Neurobiol. 53, 2368–2383. doi: 10.1007/s12035-015-9215-1
Dabdoub, A., Puligilla, C., Jones, J. M., Fritzsch, B., Cheah, K. S., Pevny, L. H., et al. (2008). Sox2 signaling in prosensory domain specification and subsequent hair cell differentiation in the developing cochlea. Proc. Natl. Acad. Sci. 105, 18396–18401. doi: 10.1073/pnas.0808175105
Dawson, S., and Bowl, M. (2019). Age-related hearing loss. Cold Spring Harb. Perspect. Med. 9:a033217. doi: 10.1101/cshperspect.a033217
Devare, J., Gubbels, S., and Raphael, Y. (2018). Outlook and future of inner ear therapy. Hear. Res. 368, 127–135. doi: 10.1016/j.heares.2018.05.009
Di Bonito, M., Studer, M., and Puelles, L. (2017). Nuclear derivatives and axonal projections originating from rhombomere 4 in the mouse hindbrain. Brain Struct. Funct. 222, 3509–3542. doi: 10.1007/s00429-017-1416-0
Driver, E. C., and Kelley, M. W. (2020). Development of the cochlea. Development 147:dev162263. doi: 10.1242/dev.162263
Dubno, J. R. (2019). New insights on age-related hearing loss. J. South Carolina Acad. Sci. 17, 3–11.
Duncan, J. S., and Fritzsch, B. (2013). Continued expression of GATA3 is necessary for cochlear neurosensory development. PLoS One 8:e62046. doi: 10.1371/journal.pone.0062046
Dvorakova, M., Jahan, I., Macova, I., Chumak, T., Bohuslavova, R., Syka, J., et al. (2016). Incomplete and delayed Sox2 deletion defines residual ear neurosensory development and maintenance. Sci. Rep. 6:38253. doi: 10.1038/srep38253
Dvorakova, M., Macova, I., Bohuslavova, R., Anderova, M., Fritzsch, B., and Pavlinkova, G. (2020). Early ear neuronal development, but not olfactory or lens development, can proceed without SOX2. Dev. Biol. 457, 43–56. doi: 10.1016/j.ydbio.2019.09.003
Eckert, M. A., Vaden Jr, K. I., and Dubno, J. R. (2019). Age-related hearing loss associations with changes in brain morphology. Trends Hear. 23:2331216519857267. doi: 10.1177/2331216519857267
Eggermont, J. J. (2019). The Auditory Brain and Age-related Hearing Impairment. London; San Diego: Academic Press, Elsevier. pp. 269.
Elliott, K. L., and Fritzsch, B. (2018). Ear transplantations reveal conservation of inner ear afferent pathfinding cues. Sci. Rep. 8:13819. doi: 10.1038/s41598-018-31952-y
Elliott, K. L., Fritzsch, B., and Duncan, J. S. (2018). Evolutionary and developmental biology provide insights into the regeneration of organ of corti hair cells. Front. Cell. Neurosci. 12:252. doi: 10.3389/fncel.2018.00252
Elliott, K. L., Kersigo, J., Lee, J. H., Jahan, I., Pavlinkova, G., Fritzsch, B., et al. (2021a). Developmental changes in peripherin-eGFP expression in spiral ganglion neurons. Front. Cell. Neurosci. 15:678113. doi: 10.3389/fncel.2021.678113
Elliott, K. L., Pavlínková, G., Chizhikov, V. V., Yamoah, E. N., and Fritzsch, B. (2021b). Development in the mammalian auditory system depends on transcription factors. Int. J. Mol. Sci. 22:4189. doi: 10.3390/ijms2208418
Elliott, K. L., Kersigo, J., Pan, N., Jahan, I., and Fritzsch, B. (2017). Spiral ganglion neuron projection development to the hindbrain in mice lacking peripheral and/or central target differentiation. Front. Neural Circuits 11:25. doi: 10.3389/fncir.2017.00025
Erives, A., and Fritzsch, B. (2020). A screen for gene paralogies delineating evolutionary branching order of early metazoa. G3 (Bethesda) 10, 811–826. doi: 10.1534/g3.119.400951
Eshraghi, A. A., Jung, H. D., and Mittal, R. (2020). Recent advancements in gene and stem cell based treatment modalities: potential implications in noise induced hearing loss. Anat. Rec. (Hoboken) 303, 516–526. doi: 10.1002/ar.24107
Fattal, D., Hansen, M., and Fritzsch, B. (2018). “Aging-related balance impairment and hearing Loss,” in The Wiley Handbook on the Aging Mind and Brain, eds M. Rizzo, S. Anderson, and B. Fritzsch (Hoboken, NJ: John Wiley and Sons), 315–336. doi: 10.1002/9781118772034.ch16
Filova, I., Dvorakova, M., Bohuslavova, R., Pavlinek, A., Elliott, K. L., Vochyanova, S., et al. (2020). Combined Atoh1 and neurod1 deletion reveals autonomous growth of auditory nerve fibers. Mol. Neurobiol. 57, 5307–5323. doi: 10.1007/s12035-020-02092-0
Fritzsch, B., Beisel, K. W., and Hansen, L. A. (2006). The molecular basis of neurosensory cell formation in ear development: a blueprint for hair cell and sensory neuron regeneration? Bioessays 28, 1181–1193. doi: 10.1002/bies.20502
Fritzsch, B., Dillard, M., Lavado, A., Harvey, N. L., and Jahan, I. (2010). Canal cristae growth and fiber extension to the outer hair cells of the mouse ear require Prox1 activity. PLoS One 5:e9377. doi: 10.1371/journal.pone.0009377
Fritzsch, B., Elliott, K. L., and Pavlinkova, G. (2019). Primary sensory map formations reflect unique needs and molecular cues specific to each sensory system. F1000Res. 8:F1000. doi: 10.12688/f1000research.17717.1
Fritzsch, B., Fariñas, I., and Reichardt, L. F. (1997). Lack of neurotrophin 3 causes losses of both classes of spiral ganglion neurons in the cochlea in a region-specific fashion. J. Neurosci. 17, 6213–6225. doi: 10.1523/JNEUROSCI.17-16-06213.1997
Fritzsch, B., Kersigo, J., Yang, T., Jahan, I., and Pan, N. (2016). “Neurotrophic factor function during ear development: expression changes define critical phases for neuronal viability”, in The Primary Auditory Neurons of the Mammalian Cochlea, eds A. Dabdoub, B. Fritzsch, A. Popper, and R. Fay (New York, NY: Springer), 49–84. doi: 10.1007/978-1-4939-3031-9_3
Fritzsch, B., Matei, V., Nichols, D., Bermingham, N., Jones, K., Beisel, K., et al. (2005). Atoh1 null mice show directed afferent fiber growth to undifferentiated ear sensory epithelia followed by incomplete fiber retention. Dev Dyn. 233, 570–583. doi: 10.1002/dvdy.20370
Gantz, B. J., Dunn, C. C., Oleson, J., and Hansen, M. R. (2018). Acoustic plus electric speech processing: long-term results. Laryngoscope 128, 473–481. doi: 10.1002/lary.26669
Gantz, B. J., Turner, C., Gfeller, K. E., and Lowder, M. W. (2005). Preservation of hearing in cochlear implant surgery: advantages of combined electrical and acoustical speech processing. Laryngoscope 115, 796–802. doi: 10.1097/01.MLG.0000157695.07536.D2
Gleich, O., Weiss, M., and Strutz, J. (2004). Age-dependent changes in the lateral superior olive of the gerbil (Meriones unguiculatus). Hear. Res. 194, 47–59. doi: 10.1016/j.heares.2004.03.016
Godfrey, D. A., Chen, K., O’Toole, T. R., and Mustapha, A. I. (2017). Amino acid and acetylcholine chemistry in the central auditory system of young, middle-aged and old rats. Hear. Res. 350, 173–188. doi: 10.1016/j.heares.2017.05.002
Gray, D. T., Engle, J. R., and Recanzone, G. H. (2013). Age-related neurochemical changes in the rhesus macaque superior olivary complex. J. Comp. Neurol. 522, 573–591. doi: 10.1002/cne.23427
Groves, A. K., and Fekete, D. M. (2017). “New directions in cochlear development,” in Understanding the Cochlea, eds G. Manley, A. Gummer, A. Popper, and R. Fay (Cham: Springer), 33–73.
Harris, J. A., and Rubel, E. W. (2006). Afferent regulation of neuron number in the cochlear nucleus: cellular and molecular analyses of a critical period. Hear. Res. 216, 127–137. doi: 10.1016/j.heares.2006.03.016
Helfert, R. H., Krenning, J., Wilson, T. S., and Hughes, L. F. (2003). Age-related synaptic changes in the anteroventral cochlear nucleus of Fischer-344 rats. Hear. Res. 183, 18–28. doi: 10.1016/s0378-5955(03)00194-1
Herranen, A., Ikäheimo, K., Lankinen, T., Pakarinen, E., Fritzsch, B., Saarma, M., et al. (2020). Deficiency of the ER-stress-regulator MANF triggers progressive outer hair cell death and hearing loss. Cell Death Dis. 11:100. doi: 10.1038/s41419-020-2286-6
Hertzano, R., Dror, A. A., Montcouquiol, M., Ahmed, Z. M., Ellsworth, B., Camper, S., et al. (2007). Lhx3, a LIM domain transcription factor, is regulated by Pou4f3 in the auditory but not in the vestibular system. Eur. J. Neurosci. 25, 999–1005. doi: 10.1111/j.1460-9568.2007.05332.x
Hinojosa, R., and Nelson, E. G. (2011). Cochlear nucleus neuron analysis in individuals with presbycusis. Laryngoscope 121, 2641–2648. doi: 10.1002/lary.22383
Homans, N. C., Metselaar, R. M., Dingemanse, J. G., van der Schroeff, M. P., Brocaar, M. P., Wieringa, M. H., et al. (2017). Prevalence of age-related hearing loss, including sex differences, in older adults in a large cohort study. Laryngoscope 127, 725–730. doi: 10.1002/lary.26150
Huang, Y., Hill, J., Yatteau, A., Wong, L., Jiang, T., Petrovic, J., et al. (2018). Reciprocal negative regulation between Lmx1a and Lmo4 is required for inner ear formation. J. Neurosci. 38, 5429–5440. doi: 10.1523/JNEUROSCI.2484-17.2018
Huang, E. J., Liu, W., Fritzsch, B., Bianchi, L. M., Reichardt, L. F., and Xiang, M. (2001). Brn3a is a transcriptional regulator of soma size, target field innervation and axon pathfinding of inner ear sensory neurons. Development 128, 2421–2432. doi: 10.1242/dev.128.13.2421
Huang, M., Sage, C., Li, H., Xiang, M., Heller, S., and Chen, Z. Y. (2008). Diverse expression patterns of LIM-homeodomain transcription factors (LIM-HDs) in mammalian inner ear development. Dev. Dyn. 237, 3305–3312. doi: 10.1002/dvdy.21735
Ibrahim, B. A., and Llano, D. A. (2019). Aging and central auditory disinhibition: is it a reflection of homeostatic downregulation or metabolic vulnerability? Brain Sci. 9:351. doi: 10.3390/brainsci9120351
Idrizbegovic, E., Bogdanovic, N., Viberg, A., and Canlon, B. (2003). Auditory peripheral influences on calcium binding protein immunoreactivity in the cochlear nucleus during aging in the C57BL/6J mouse. Hear. Res. 179, 33–42. doi: 10.1016/s0378-5955(03)00076-5
Idrizbegovic, E., Salman, H., Niu, X., and Canlon, B. (2006). Presbyacusis and calcium-binding protein immunoreactivity in the cochlear nucleus of BALB/c mice. Hear. Res. 216–217, 198–206. doi: 10.1016/j.heares.2006.01.009
Jahan, I., Elliott, K. L., and Fritzsch, B. (2018). Understanding molecular evolution and development of the organ of corti can provide clues for hearing restoration. Integr. Comp. Biol. 58, 351–365. doi: 10.1093/icb/icy019
Jahan, I., Kersigo, J., Pan, N., and Fritzsch, B. (2010a). Neurod1 regulates survival and formation of connections in mouse ear and brain. Cell Tissue Res. 341, 95–110. doi: 10.1007/s00441-010-0984-6
Jahan, I., Pan, N., Kersigo, J., and Fritzsch, B. (2010b). Neurod1 suppresses hair cell differentiation in ear ganglia and regulates hair cell subtype development in the cochlea. PLoS One 5:e11661. doi: 10.1371/journal.pone.0011661
Jahan, I., Pan, N., Kersigo, J., and Fritzsch, B. J. D. (2015). Neurog1 can partially substitute for Atoh1 function in hair cell differentiation and maintenance during organ of Corti development. Development 142, 2810–2821. doi: 10.1242/dev.123091
Jalabi, W., Kopp-Scheinpflug, C., Allen, P. D., Schiavon, E., DiGiacomo, R. R., Forsythe, I. D., et al. (2013). Sound localization ability and glycinergic innervation of the superior olivary complex persist after genetic deletion of the medial nucleus of the trapezoid body. J. Neurosci. 33, 15044–15049. doi: 10.1523/JNEUROSCI.2604-13.2013
Jalenques, I., Albuisson, E., Despres, G., and Romand, R. (1995). Distribution of glial fibrillary acidic protein (GFAP) in the cochlear nucleus of adult and aged rats. Brain Res. 686, 223–232. doi: 10.1016/0006-8993(95)00463-z
Kageyama, R., Shimojo, H., and Ohtsuka, T. (2019). Dynamic control of neural stem cells by bHLH factors. Neurosci. Res. 138, 12–18. doi: 10.1016/j.neures.2018.09.005
Kandler, K., Lee, J., and Pecka, M. (2020). “2.28 - The superior olivary complex,” in The Senses: A Comprehensive Reference (Second Edition), ed B. Fritzsch (Oxford: Elsevier), 533–555.
Kane, K. L., Longo-Guess, C. M., Gagnon, L. H., Ding, D., Salvi, R. J., and Johnson, K. R. (2012). Genetic background effects on age-related hearing loss associated with Cdh23 variants in mice. Hear. Res. 283, 80–88. doi: 10.1016/j.heares.2011.11.007
Kann, O., Papageorgiou, I. E., and Draguhn, A. (2014). Highly energized inhibitory interneurons are a central element for information processing in cortical networks. J. Cereb. Blood Flow Metab. 34, 1270–1282. doi: 10.1038/jcbfm.2014.104
Karis, A., Pata, I., van Doorninck, J. H., Grosveld, F., de Zeeuw, C. I., de Caprona, D., et al. (2001). Transcription factor GATA-3 alters pathway selection of olivocochlear neurons and affects morphogenesis of the ear. J. Comp. Neurol. 429, 615–630. doi: 10.1002/1096-9861(20010122)429:4<615::aid-cne8>3.0.co;2-f
Karlsson, T., Rask-Andersen, M., Pan, G., Höglund, J., Wadelius, C., Ek, W. E., et al. (2019). Contribution of genetics to visceral adiposity and its relation to cardiovascular and metabolic disease. Nat. Med. 25, 1390–1395. doi: 10.1038/s41591-019-0563-7
Kelly, M. C., Chang, Q., Pan, A., Lin, X., and Chen, P. (2012). Atoh1 directs the formation of sensory mosaics and induces cell proliferation in the postnatal mammalian cochlea in vivo. J. Neurosci. 32, 6699–6710. doi: 10.1523/JNEUROSCI.5420-11.2012
Kempfle, J. S., and Edge, A. S. (2014). Pax2 and Sox2 cooperate to promote hair cell fate in inner ear stem cells. Otolaryngol. Head Neck Surg. 151, P221–P221. doi: 10.1177/0194599814541629a266
Kempfle, J. S., Turban, J. L., and Edge, A. S. (2016). Sox2 in the differentiation of cochlear progenitor cells. Sci. Rep. 6:23293. doi: 10.1038/srep23293
Kersigo, J., D’Angelo, A., Gray, B. D., Soukup, G. A., and Fritzsch, B. (2011). The role of sensory organs and the forebrain for the development of the craniofacial shape as revealed by Foxg1-cre-mediated microRNA loss. Genesis 49, 326–341. doi: 10.1002/dvg.20714
Kersigo, J., and Fritzsch, B. (2015). Inner ear hair cells deteriorate in mice engineered to have no or diminished innervation. Front. Aging Neurosci. 7:33. doi: 10.3389/fnagi.2015.00033
Kersigo, J., Gu, L., Xu, L., Pan, N., Vijayakuma, S., Jones, T., et al. (2021). Effects of Neurod1 expression on mouse and human schwannoma cells. Laryngoscope 131, E259–E270. doi: 10.1002/lary.28671
Kiernan, A. E., Pelling, A. L., Leung, K. K., Tang, A. S., Bell, D. M., Tease, C., et al. (2005). Sox2 is required for sensory organ development in the mammalian inner ear. Nature 434, 1031–1035. doi: 10.1038/nature03487
Koehler, K. R., Nie, J., Longworth-Mills, E., Liu, X.-P., Lee, J., Holt, J. R., et al. (2017). Generation of inner ear organoids containing functional hair cells from human pluripotent stem cells. Nat. Biotechnol. 35, 583–589. doi: 10.1038/nbt.3840
Kopecky, B., Santi, P., Johnson, S., Schmitz, H., and Fritzsch, B. (2011). Conditional deletion of N-Myc disrupts neurosensory and non-sensory development of the ear. Dev. Dyn. 240, 1373–1390. doi: 10.1002/dvdy.22620
Kral, A., Popper, A. N., and Fay, R. R. Eds. (2013). Deafness. New York, NY: Springer. doi: 10.1007/978-1-4614-7840-9
Krawczyk, K., Scheller, L., Kim, H., and Fussenegger, M. (2020). Rewiring of endogenous signaling pathways to genomic targets for therapeutic cell reprogramming. Nat. Commun. 11:608. doi: 10.1038/s41467-020-14397-8
Krohs, C., Körber, C., Ebbers, L., Altaf, F., Hollje, G., Hoppe, S., et al. (2021). Loss of miR-183/96 alters synaptic strength via presynaptic and postsynaptic mechanisms at a central synapse. J. Neurosci. 41, 6796–6811. doi: 10.1523/JNEUROSCI.0139-20.2021
Kujawa, S. G., and Liberman, M. C. (2009). Adding insult to injury: cochlear nerve degeneration after “temporary” noise-induced hearing loss. J. Neurosci. 29, 14077–14085. doi: 10.1523/JNEUROSCI.2845-09.2009
Kusunoki, T., Cureoglu, S., Schachern, P. A., Baba, K., Kariya, S., and Paparella, M. M. (2004). Age-related histopathologic changes in the human cochlea: a temporal bone study. Otolaryngol. Head Neck Surg. 131, 897–903. doi: 10.1016/j.otohns.2004.05.022
Lahlou, H., Nivet, E., Lopez-Juarez, A., Fontbonne, A., Assou, S., and Zine, A. (2018). Enriched differentiation of human otic sensory progenitor cells derived from induced pluripotent stem cells. Front. Mol. Neurosci. 11:452. doi: 10.3389/fnmol.2018.00452
Lang, H. (2016). “Loss, degeneration and preservation of the spiral ganglion neurons and their processes,” in The Primary Auditory Neurons of the Mammalian Cochlea, eds A. Dabdoub, B. Fritzsch, A. N. Popper, and R. R. Fay (New York, NY: Springer New York), 229–262. doi: 10.1007/978-1-4939-3031-9_8
Lee, K. J., Dietrich, P., and Jessell, T. M. (2000). Genetic ablation reveals that the roof plate is essential for dorsal interneuron specification. Nature 403, 734–740. doi: 10.1038/35001507
Levi-Montalcini, R. (1949). The development of the acoustico-vestibular centres in the chick embryo in the absence of the afferent root fibers and of descending fiber tracts. J. Comp. Neurol. 91, 209–241. doi: 10.1002/cne.900910204
Lewis, M. A., Nolan, L. S., Cadge, B. A., Matthews, L. J., Schulte, B. A., Dubno, J. R., et al. (2018). Whole exome sequencing in adult-onset hearing loss reveals a high load of predicted pathogenic variants in known deafness-associated genes and identifies new candidate genes. BMC Med. Genomics 11:77. doi: 10.1186/s12920-018-0395-1
Lewis, R. M., Keller, J. J., Wan, L., and Stone, J. S. (2018). Bone morphogenetic protein 4 antagonizes hair cell regeneration in the avian auditory epithelium. Hear. Res. 364, 1–11. doi: 10.1016/j.heares.2018.04.008
Li, J., Zhang, T., Ramakrishnan, A., Fritzsch, B., Xu, J., Wong, E. Y., et al. (2020). Dynamic changes in cis-regulatory occupancy by Six1 and its cooperative interactions with distinct cofactors drive lineage-specific gene expression programs during progressive differentiation of the auditory sensory epithelium. Nucleic Acids Res. 48, 2880–2896. doi: 10.1093/nar/gkaa012
Lim, R., and Brichta, A. M. (2016). Anatomical and physiological development of the human inner ear. Hear. Res. 338, 9–21. doi: 10.1016/j.heares.2016.02.004
Lipovsek, M., and Wingate, R. J. (2018). Conserved and divergent development of brainstem vestibular and auditory nuclei. eLife 7:e40232. doi: 10.7554/eLife.40232
Liu, W., Johansson, Å., Rask-Andersen, H., and Rask-Andersen, M. (2021). A combined genome-wide association and molecular study of age-related hearing loss in H. sapiens. BMC Med. 19:302. doi: 10.1186/s12916-021-02169-0
Locher, H., Frijns, J. H., van Iperen, L., de Groot, J. C., Huisman, M. A., and de Sousa Lopes, S. M. C. (2013). Neurosensory development and cell fate determination in the human cochlea. Neural Dev. 8:20. doi: 10.1186/1749-8104-8-20
Lohmann, C., and Friauf, E. (1996). Distribution of the calcium-binding proteins parvalbumin and calretinin in the auditory brainstem of adult and developing rats. J. Comp. Neurol. 367, 90–109. doi: 10.1002/(SICI)1096-9861(19960325)367:1<90::AID-CNE7>3.0.CO;2-E
Lopez-Juarez, A., Lahlou, H., Ripoll, C., Cazals, Y., Brezun, J. M., Wang, Q., et al. (2019). Engraftment of human stem cell-derived otic progenitors in the damaged cochlea. Mol. Ther. 27, 1101–1113. doi: 10.1016/j.ymthe.2019.03.018
López-Otín, C., Blasco, M. A., Partridge, L., Serrano, M., and Kroemer, G. (2013). The hallmarks of aging. Cell 153, 1194–1217. doi: 10.1016/j.cell.2013.05.039
Löwenheim, H., Furness, D. N., Kil, J., Zinn, C., Gültig, K., Fero, M. L., et al. (1999). Gene disruption of p27Kip1 allows cell proliferation in the postnatal and adult organ of corti. Proc. Natl. Acad. Sci. U S A 96, 4084–4088. doi: 10.1073/pnas.96.7.4084
Lu, C. C., Cao, X.-J., Wright, S., Ma, L., Oertel, D., and Goodrich, L. V. (2014). Mutation of Npr2 leads to blurred tonotopic organization of central auditory circuits in mice. PLoS Genet. 10:e1004823. doi: 10.1371/journal.pgen.1004823
Ma, Q., Anderson, D. J., and Fritzsch, B. (2000). Neurogenin 1 null mutant ears develop fewer, morphologically normal hair cells in smaller sensory epithelia devoid of innervation. J. Assoc. Res. Otolaryngol. 1, 129–143. doi: 10.1007/s101620010017
Ma, Q., Chen, Z., del Barco Barrantes, I., De La Pompa, J. L., and Anderson, D. J. (1998). neurogenin1 is essential for the determination of neuronal precursors for proximal cranial sensory ganglia. Neuron 20, 469–482. doi: 10.1016/s0896-6273(00)80988-5
Macova, I., Pysanenko, K., Chumak, T., Dvorakova, M., Bohuslavova, R., Syka, J., et al. (2019). Neurod1 is essential for the primary tonotopic organization and related auditory information processing in the midbrain. J. Neurosci. 39, 984–1004. doi: 10.1523/JNEUROSCI.2557-18.2018
Makary, C. A., Shin, J., Kujawa, S. G., Liberman, M. C., and Merchant, S. N. (2011). Age-related primary cochlear neuronal degeneration in human temporal bones. J. Assoc. Res. Otolaryngol. 12, 711–717. doi: 10.1007/s10162-011-0283-2
Malmierca, M. S., and Ryugo, D. K. (2011). “Descending connections of auditory cortex to the midbrain and brain stem,” in The Auditory Cortex, eds J. A. Winer, and C. E. Schreiner (Boston, MA: Springer), 189–208. doi: 10.1007/978-1-4419-0074-6_9
Manis, P. B., Xie, R., Wang, Y., Marrs, G. S., and Spirou, G. A. (2012). “The endbulbs of held,” in Synaptic Mechanisms in the Auditory System, eds L. O. Trussell, A. N. Popper, and R. R. Fay (New York, NY: Springer New York), 61–93. doi: 10.1007/978-1-4419-9517-9_4
Mantela, J., Jiang, Z., Ylikoski, J., Fritzsch, B., Zacksenhaus, E., and Pirvola, U. (2005). The retinoblastoma gene pathway regulates the postmitotic state of hair cells of the mouse inner ear. Development 132, 2377–2388. doi: 10.1242/dev.01834
Mao, Y., Reiprich, S., Wegner, M., and Fritzsch, B. (2014). Targeted deletion of Sox10 by Wnt1-cre defects neuronal migration and projection in the mouse inner ear. PLoS One 9:e94580. doi: 10.1371/journal.pone.0094580
Maricich, S. M., Xia, A., Mathes, E. L., Wang, V. Y., Oghalai, J. S., Fritzsch, B., et al. (2009). Atoh1-lineal neurons are required for hearing and for the survival of neurons in the spiral ganglion and brainstem accessory auditory nuclei. J. Neurosci. 29, 11123–11133. doi: 10.1523/JNEUROSCI.2232-09.2009
Marrs, G. S., Morgan, W. J., Howell, D. M., Spirou, G. A., and Mathers, P. H. (2013). Embryonic origins of the mouse superior olivary complex. Dev. Neurobiol. 73, 384–398. doi: 10.1002/dneu.22069
Matei, V., Pauley, S., Kaing, S., Rowitch, D., Beisel, K., Morris, K., et al. (2005). Smaller inner ear sensory epithelia in Neurog1 null mice are related to earlier hair cell cycle exit. Dev. Dyn. 234, 633–650. doi: 10.1002/dvdy.20551
Matern, M. S., Milon, B., Lipford, E. L., McMurray, M., Ogawa, Y., Tkaczuk, A., et al. (2020). GFI1 functions to repress neuronal gene expression in the developing inner ear hair cells. Development 147:dev186015. doi: 10.1242/dev.186015
McGovern, M. M., Randle, M. R., Cuppini, C. L., Graves, K. A., and Cox, B. C. (2019). Multiple supporting cell subtypes are capable of spontaneous hair cell regeneration in the neonatal mouse cochlea. Development 146:dev171009. doi: 10.1242/dev.171009
Milinkeviciute, G., and Cramer, K. S. (2020). “2.18 - Development of the ascending auditory pathway,” in The Senses: A Comprehensive Reference (Second Edition), ed B. Fritzsch (Oxford: Elsevier), 337–353.
Muniak, M. A., Connelly, C. J., Suthakar, K., Milinkeviciute, G., Ayeni, F. E., and Ryugo, D. K. (2016). “Central projections of spiral ganglion neurons,” in The Primary Auditory Neurons of the Mammalian Cochlea, eds A. Dabdoub, B. Fritzsch, A. Popper, and R. Fay (New York, NY: Springer), 157–190.
Muthu, V., Rohacek, A. M., Yao, Y., Rakowiecki, S. M., Brown, A. S., Zhao, Y.-T., et al. (2019). Genomic architecture of Shh-dependent cochlear morphogenesis. Development 146:dev181339. doi: 10.1242/dev.181339
Nadol Jr, J. B. (1990). Degeneration of cochlear neurons as seen in the spiral ganglion of man. Hear. Res. 49, 141–154. doi: 10.1016/0378-5955(90)90101-t
Nadol Jr, J. B., and Xu, W.-Z. (1992). Diameter of the cochlear nerve in deaf humans: implications for cochlear implantation. Ann. Otol. Rhinol. Laryngol. 101, 988–993. doi: 10.1177/000348949210101205
Nakano, Y., Jahan, I., Bonde, G., Sun, X., Hildebrand, M. S., Engelhardt, J. F., et al. (2012). A mutation in the Srrm4 gene causes alternative splicing defects and deafness in the Bronx waltzer mouse. PLoS Genet. 8:e1002966. doi: 10.1371/journal.pgen.1002966
Nakano, Y., Wiechert, S., Fritzsch, B., and Bánfi, B. (2020). Inhibition of a transcriptional repressor rescues hearing in a splicing factor-deficient mouse. Life Sci. Alliance 3:e202000841. doi: 10.26508/lsa.202000841
Neves, J., Uchikawa, M., Bigas, A., and Giraldez, F. (2012). The prosensory function of Sox2 in the chicken inner ear relies on the direct regulation of Atoh1. PLoS One 7:e30871. doi: 10.1371/journal.pone.0030871
Nichols, D. H., Bouma, J. E., Kopecky, B. J., Jahan, I., Beisel, K. W., He, D. Z., et al. (2020). Interaction with ectopic cochlear crista sensory epithelium disrupts basal cochlear sensory epithelium development in Lmx1a mutant mice. Cell Tissue Res. 380, 435–448. doi: 10.1007/s00441-019-03163-y
Nichols, D. H., Pauley, S., Jahan, I., Beisel, K. W., Millen, K. J., and Fritzsch, B. (2008). Lmx1a is required for segregation of sensory epithelia and normal ear histogenesis and morphogenesis. Cell Tissue Res. 334, 339–358. doi: 10.1007/s00441-008-0709-2
Nyberg, S., Abbott, N. J., Shi, X., Steyger, P. S., and Dabdoub, A. (2019). Delivery of therapeutics to the inner ear: the challenge of the blood-labyrinth barrier. Sci. Transl. Med. 11:eaao0935. doi: 10.1126/scitranslmed.aao0935
Oertel, D., and Cao, X.-J. (2020). “2.27 - The ventral cochlear nucleus,” in The Senses: A Comprehensive Reference (Second Edition), ed B. Fritzsch (Oxford: Elsevier), 517–532.
Pan, N., Jahan, I., Kersigo, J., Duncan, J. S., Kopecky, B., and Fritzsch, B. (2012). A novel Atoh1 “self-terminating” mouse model reveals the necessity of proper Atoh1 level and duration for hair cell differentiation and viability. PLoS One 7:e30358. doi: 10.1371/journal.pone.0030358
Patel, D., Shimomura, A., Majumdar, S., Holley, M. C., and Hashino, E. (2018). The histone demethylase LSD1 regulates inner ear progenitor differentiation through interactions with Pax2 and the NuRD repressor complex. PLoS One 13:e0191689. doi: 10.1371/journal.pone.0191689
Pauley, S., Kopecky, B., Beisel, K., Soukup, G., and Fritzsch, B. (2008). Stem cells and molecular strategies to restore hearing. Panminerva Medica 50, 41–65.
Pauley, S., Wright, T. J., Pirvola, U., Ornitz, D., Beisel, K., and Fritzsch, B. (2003). Expression and function of FGF10 in mammalian inner ear development. Dev. Dyn. 227, 203–215. doi: 10.1002/dvdy.10297
Perkins, G., Lee, J. H., Park, S., Kang, M., Perez-Flores, M. C., Ju, S., et al. (2020). Altered outer hair cell mitochondrial and subsurface cisternae connectomics are candidate mechanisms for hearing loss in mice. J. Neurosci. 40, 8556–8572. doi: 10.1523/JNEUROSCI.2901-19.2020
Petitpré, C., Wu, H., Sharma, A., Tokarska, A., Fontanet, P., Wang, Y., et al. (2018). Neuronal heterogeneity and stereotyped connectivity in the auditory afferent system. Nat. Commun. 9:3691. doi: 10.1038/s41467-018-06033-3
Pierce, E. T. (1967). Histogenesis of the dorsal and ventral cochlear nuclei in the mouse. An autoradiographic study. J. Comp. Neurol. 131, 27–54. doi: 10.1002/cne.901310104
Pierce, E. T. (1973). Time of origin of neurons in the brain stem of the mouse. Prog. Brain Res. 40, 53–65. doi: 10.1016/S0079-6123(08)60679-2
Pierce, M. L., Weston, M. D., Fritzsch, B., Gabel, H. W., Ruvkun, G., and Soukup, G. A. (2008). MicroRNA-183 family conservation and ciliated neurosensory organ expression. Evol. Dev. 10, 106–113. doi: 10.1111/j.1525-142X.2007.00217.x
Pirvola, U., Ylikoski, J., Trokovic, R., Hébert, J. M., McConnell, S. K., and Partanen, J. (2002). FGFR1 is required for the development of the auditory sensory epithelium. Neuron 35, 671–680. doi: 10.1016/s0896-6273(02)00824-3
Qiu, X., and Müller, U. (2018). Mechanically gated ion channels in mammalian hair cells. Front. Cell. Neurosci. 12:100. doi: 10.3389/fncel.2018.00100
Radde-Gallwitz, K., Pan, L., Gan, L., Lin, X., Segil, N., and Chen, P. (2004). Expression of Islet1 marks the sensory and neuronal lineages in the mammalian inner ear. J. Comp. Neurol. 477, 412–421. doi: 10.1002/cne.20257
Rask-Andersen, H., Boström, M., Gerdin, B., Kinnefors, A., Nyberg, G., Engstrand, T., et al. (2005). Regeneration of human auditory nerve. In vitro/in video demonstration of neural progenitor cells in adult human and guinea pig spiral ganglion. Hear. Res. 203, 180–191. doi: 10.1016/j.heares.2004.12.005
Rauch, S. D., Velazquez-VillaseÑor, L., Dimitri, P. S., and Merchant, S. N. (2001). Decreasing hair cell counts in aging humans. Ann. N Y Acad. Sci. 942, 220–227. doi: 10.1111/j.1749-6632.2001.tb03748.x
Ray, R. S., and Dymecki, S. M. (2009). Rautenlippe Redux—toward a unified view of the precerebellar rhombic lip. Curr. Opin. Cell Biol. 21, 741–747. doi: 10.1016/j.ceb.2009.10.003
Revuelta, M., Santaolalla, F., Arteaga, O., Alvarez, A., Sanchez-del-Rey, A., and Hilario, E. (2017). Recent advances in cochlear hair cell regeneration—a promising opportunity for the treatment of age-related hearing loss. Ageing Res. Rev. 36, 149–155. doi: 10.1016/j.arr.2017.04.002
Riccomagno, M. M., Takada, S., and Epstein, D. J. (2005). Wnt-dependent regulation of inner ear morphogenesis is balanced by the opposing and supporting roles of Shh. Genes Dev. 19, 1612–1623. doi: 10.1101/gad.1303905
Roccio, M., Senn, P., and Heller, S. (2020). Novel insights into inner ear development and regeneration for refined hearing loss therapies. Hear. Res. 397:107859. doi: 10.1016/j.heares.2019.107859
Romano, D. R., Hashino, E., and Nelson, R. F. (2021). Deafness-in-a-dish: modeling hereditary deafness with inner ear organoids. Hum. Genet. . [Online ahead of print]. doi: 10.1007/s00439-021-02325-9
Rubel, E. W., and Fritzsch, B. (2002). Auditory system development: primary auditory neurons and their targets. Ann. Rev. Neurosci. 25, 51–101. doi: 10.1146/annurev.neuro.25.112701.142849
Ruben, R. J. (1967). Development of the inner ear of the mouse: a radioautographic study of terminal mitoses. Acta Otolaryngol. (Stockh.) 220, 1–44.
Ryugo, D., Kretzmer, E., and Niparko, J. (2005). Restoration of auditory nerve synapses in cats by cochlear implants. Science 310, 1490–1492. doi: 10.1126/science.1119419
Sanes, D. H., and Rubel, E. (1988). The ontogeny of inhibition and excitation in the gerbil lateral superior olive. J. Neurosci. 8, 682–700. doi: 10.1523/JNEUROSCI.08-02-00682.1988
Schilder, A. G., Su, M. P., Blackshaw, H., Lustig, L., Staecker, H., Lenarz, T., et al. (2019). Hearing protection, restoration and regeneration: an overview of emerging therapeutics for inner ear and central hearing disorders. Otol. Neurotol. 40, 559–570. doi: 10.1097/MAO.0000000000002194
Schmidt, H., and Fritzsch, B. (2019). Npr2 null mutants show initial overshooting followed by reduction of spiral ganglion axon projections combined with near-normal cochleotopic projection. Cell Tissue Res. 378, 15–32. doi: 10.1007/s00441-019-03050-6
Sedlmayer, F., Aubel, D., and Fussenegger, M. (2018). Synthetic gene circuits for the detection, elimination and prevention of disease. Nat. Biomed. Eng. 2, 399–415. doi: 10.1038/s41551-018-0215-0
Sekiya, T., and Holley, M. C. (2021). Cell transplantation to restore lost auditory nerve function is a realistic clinical opportunity. Cell Transplant. 30:09636897211035076. doi: 10.1177/09636897211035076
Sharma, S., Nag, T. C., Thakar, A., Bhardwaj, D. N., and Roy, T. S. (2014). The aging human cochlear nucleus: changes in the glial fibrillary acidic protein, intracellular calcium regulatory proteins, GABA neurotransmitter and cholinergic receptor. J. Chem. Neuroanat. 56, 1–12. doi: 10.1016/j.jchemneu.2013.12.001
Sheffield, A. M., and Smith, R. J. (2019). The epidemiology of deafness. Cold Spring Harb. Perspect. Med. 9:a033258. doi: 10.1101/cshperspect.a033258
Shibata, S. B., Cortez, S. R., Beyer, L. A., Wiler, J. A., Di Polo, A., Pfingst, B. E., et al. (2010). Transgenic BDNF induces nerve fiber regrowth into the auditory epithelium in deaf cochleae. Exp. Neurol. 223, 464–472. doi: 10.1016/j.expneurol.2010.01.011
Shrestha, B. R., Chia, C., Wu, L., Kujawa, S. G., Liberman, M. C., and Goodrich, L. V. (2018). Sensory neuron diversity in the inner ear is shaped by activity. Cell 174, 1229–1246.e17. doi: 10.1016/j.cell.2018.07.007
Shrestha, B. R., and Goodrich, L. V. (2019). Wiring the Cochlea for Sound Perception. London: Oxford University Press. pp. 5–25.
Simpson, A. N., Matthews, L. J., Cassarly, C., and Dubno, J. R. (2019). Time from hearing aid candidacy to hearing aid adoption: a longitudinal cohort study. Ear Hear. 40, 468–476. doi: 10.1097/AUD.0000000000000641
Song, Z., Jadali, A., Fritzsch, B., and Kwan, K. Y. (2017). NEUROG1 regulates CDK2 to promote proliferation in otic progenitors. Stem Cell Rep. 9, 1516–1529. doi: 10.1016/j.stemcr.2017.09.011
Song, Z., Laureano, A. S., Patel, K., Yip, S., Jadali, A., and Kwan, K. Y. (2019). Single-cell fluorescence analysis of pseudotemporal ordered cells provides protein expression dynamics for neuronal differentiation. Front. Cell Dev. Biol. 7:87. doi: 10.3389/fcell.2019.00087
Soukup, G. A., Fritzsch, B., Pierce, M. L., Weston, M. D., Jahan, I., McManus, M. T., et al. (2009). Residual microRNA expression dictates the extent of inner ear development in conditional Dicer knockout mice. Dev. Biol. 328, 328–341. doi: 10.1016/j.ydbio.2009.01.037
Spongr, V. P., Flood, D. G., Frisina, R. D., and Salvi, R. J. (1997). Quantitative measures of hair cell loss in CBA and C57BL/6 mice throughout their life spans. J. Acoust. Soc. Am. 101, 3546–3553. doi: 10.1121/1.418315
Stakhovskaya, O., Hradek, G. T., Snyder, R. L., and Leake, P. A. (2008). Effects of age at onset of deafness and electrical stimulation on the developing cochlear nucleus in cats. Hear. Res. 243, 69–77. doi: 10.1016/j.heares.2008.05.007
Stebbings, K., Choi, H., Ravindra, A., Caspary, D., Turner, J., and Llano, D. (2016). Ageing-related changes in GABAergic inhibition in mouse auditory cortex, measured using in vitro flavoprotein autofluorescence imaging. J. Physiol. 594, 207–221. doi: 10.1113/JP271221
Sun, S., Siebald, C., and Müller, U. (2021). Subtype maturation of spiral ganglion neurons. Curr. Opin. Otolaryngol. Head Neck Surg. 29, 391–399. doi: 10.1097/MOO.0000000000000748
Syka, J. (2020). “Age-related changes in the auditory brainstem and inferior colliculus,” in Aging and Hearing. Causes and Consequences. Springer Handbook of Auditory Research Series, eds Karen S. Helfer, Edward L. Bartlett, Arthur N. Popper, and Richard R. Fay (Berlin: Springer) 72, 67–96.
Tateya, T., Sakamoto, S., Ishidate, F., Hirashima, T., Imayoshi, I., and Kageyama, R. (2019). Three-dimensional live imaging of Atoh1 reveals the dynamics of hair cell induction and organization in the developing cochlea. Development 146:dev177881. doi: 10.1242/dev.177881
Taylor, R. R., Jagger, D. J., and Forge, A. (2012). Defining the cellular environment in the organ of Corti following extensive hair cell loss: a basis for future sensory cell replacement in the Cochlea. PLoS One 7:e30577. doi: 10.1371/journal.pone.0030577
Tong, L., Strong, M. K., Kaur, T., Juiz, J. M., Oesterle, E. C., Hume, C., et al. (2015). Selective deletion of cochlear hair cells causes rapid age-dependent changes in spiral ganglion and cochlear nucleus neurons. J. Neurosci. 35, 7878–7891. doi: 10.1523/JNEUROSCI.2179-14.2015
Ueyama, T., Sakaguchi, H., Nakamura, T., Goto, A., Morioka, S., Shimizu, A., et al. (2014). Maintenance of stereocilia and apical junctional complexes by Cdc42 in cochlear hair cells. J. Cell Sci. 127, 2040–2052. doi: 10.1242/jcs.143602
Urness, L. D., Wang, X., Doan, H., Shumway, N., Noyes, C. A., Gutierrez-Magana, E., et al. (2018). Spatial and temporal inhibition of FGFR2b ligands reveals continuous requirements and novel targets in mouse inner ear morphogenesis. Development 145:dev170142. doi: 10.1242/dev.170142
van der Valk, W. H., Steinhart, M. R., Zhang, J., and Koehler, K. R. (2021). Building inner ears: recent advances and future challenges for in vitro organoid systems. Cell Death Differ. 28, 24–34. doi: 10.1038/s41418-020-00678-8
Walters, B. J., Coak, E., Dearman, J., Bailey, G., Yamashita, T., Kuo, B., et al. (2017). In vivo interplay between p27Kip1, GATA3, ATOH1 and POU4F3 converts non-sensory cells to hair cells in adult mice. Cell Rep. 19, 307–320. doi: 10.1016/j.celrep.2017.03.044
Wang, L., Kempton, J. B., and Brigande, J. V. (2018). Gene therapy in mouse models of deafness and balance dysfunction. Front. Mol. Neurosci. 11:300. doi: 10.3389/fnmol.2018.00300
Wang, V. Y., Rose, M. F., and Zoghbi, H. Y. (2005). Math1 expression redefines the rhombic lip derivatives and reveals novel lineages within the brainstem and cerebellum. Neuron 48, 31–43. doi: 10.1016/j.neuron.2005.08.024
Wang, H., Turner, J. G., Ling, L., Parrish, J. L., Hughes, L. F., and Caspary, D. M. (2009). Age-related changes in glycine receptor subunit composition and binding in dorsal cochlear nucleus. Neuroscience 160, 227–239. doi: 10.1016/j.neuroscience.2009.01.079
Wang, M., Zhang, C., Lin, S., Wang, Y., Seicol, B. J., Ariss, R. W., et al. (2021). Biased auditory nerve central synaptopathy is associated with age-related hearing loss. J. Physiol. 599, 1833–1854. doi: 10.1113/JP281014
White, J. A., Burgess, B. J., Hall, R. D., and Nadol, J. B. (2000). Pattern of degeneration of the spiral ganglion cell and its processes in the C57BL/6J mouse. Hear. Res. 141, 12–18. doi: 10.1016/s0378-5955(99)00204-x
Willott, J. F., and Bross, L. S. (1990). Morphology of the octopus cell area of the cochlear nucleus in young and aging C57BL/6J and CBA/J mice. J. Comp. Neurol. 300, 61–81. doi: 10.1002/cne.903000106
Willott, J. F., Jackson, L. M., and Hunter, K. P. (1987). Morphometric study of the anteroventral cochlear nucleus of two mouse models of presbycusis. J. Comp. Neurol. 260, 472–480. doi: 10.1002/cne.902600312
Wiwatpanit, T., Lorenzen, S. M., Cantú, J. A., Foo, C. Z., Hogan, A. K., Márquez, F., et al. (2018). Trans-differentiation of outer hair cells into inner hair cells in the absence of INSM1. Nature 563, 691–695. doi: 10.1038/s41586-018-0570-8
Wu, P.-Z., O’Malley, J. T., de Gruttola, V., and Liberman, M. C. (2021). Primary neural degeneration in noise-exposed human cochleas: correlations with outer hair cell loss and word-discrimination scores. J. Neurosci. 41, 4439–4447. doi: 10.1523/JNEUROSCI.3238-20.2021
Xiang, M., Maklad, A., Pirvola, U., and Fritzsch, B. (2003). Brn3c null mutant mice show long-term, incomplete retention of some afferent inner ear innervation. BMC Neurosci. 4:2. doi: 10.1186/1471-2202-4-2
Xie, R. (2016). Transmission of auditory sensory information decreases in rate and temporal precision at the endbulb of Held synapse during age-related hearing loss. J. Neurophysiol. 116, 2695–2705. doi: 10.1152/jn.00472.2016
Xie, M., and Fussenegger, M. (2018). Designing cell function: assembly of synthetic gene circuits for cell biology applications. Nat. Rev. Mol. Cell Biol. 19, 507–525. doi: 10.1038/s41580-018-0024-z
Xie, R., and Manis, P. B. (2017). Synaptic transmission at the endbulb of Held deteriorates during age-related hearing loss. J. Physiol. 595, 919–934. doi: 10.1113/JP272683
Xu, J., Li, J., Zhang, T., Jiang, H., Ramakrishnan, A., Fritzsch, B., et al. (2021). Chromatin remodelers and lineage-specific factors interact to target enhancers to establish proneurosensory fate within otic ectoderm. Proc. Natl. Acad. Sci. U S A 118:e2025196118. doi: 10.1073/pnas.2025196118
Yamashita, T., Zheng, F., Finkelstein, D., Kellard, Z., Robert, C., Rosencrance, C.D., et al. (2018). High-resolution transcriptional dissection of in vivo Atoh1-mediated hair cell conversion in mature cochleae identifies Isl1 as a co-reprogramming factor. PLoS Genet. 14:e1007552. doi: 10.1371/journal.pgen.1007552
Yamasoba, T., Lin, F. R., Someya, S., Kashio, A., Sakamoto, T., and Kondo, K. (2013). Current concepts in age-related hearing loss: epidemiology and mechanistic pathways. Hear. Res. 303, 30–38. doi: 10.1016/j.heares.2013.01.021
Yamoah, E. N., Li, M., Shah, A., Elliott, K. L., Cheah, K., Xu, P.-X., et al. (2020). Using Sox2 to alleviate the hallmarks of age-related hearing loss. Ageing Res. Rev. 59:101042. doi: 10.1016/j.arr.2020.101042
Yang, T., Kersigo, J., Jahan, I., Pan, N., and Fritzsch, B. (2011). The molecular basis of making spiral ganglion neurons and connecting them to hair cells of the organ of Corti. Hear. Res. 278, 21–33. doi: 10.1016/j.heares.2011.03.002
Yang, C.-H., Schrepfer, T., and Schacht, J. (2015). Age-related hearing impairment and the triad of acquired hearing loss. Front. Cell. Neurosci. 9:276. doi: 10.3389/fncel.2015.00276
Yoshimura, H., Shibata, S. B., Ranum, P. T., Moteki, H., and Smith, R. J. (2019). Targeted allele suppression prevents progressive hearing loss in the mature murine model of human TMC1 deafness. Mol. Ther. 27, 681–690. doi: 10.1016/j.ymthe.2018.12.014
Young, E. D., and Oertel, D. (1998). “Cochlear nucleus,” in The Synaptic Organization of the Brain. ed Gordon M. Shepherd (Oxford Scholarship Online). doi: 10.1093/acprof:oso/9780195159561.003.0004
Zettel, M. L., O’neill, W. E., Trang, T. T., and Frisina, R. D. (2003). The effects of early bilateral deafening on calretinin expression in the dorsal cochlear nucleus of aged CBA/CaJ mice. Hear. Res 183, 57–66. doi: 10.1016/s0378-5955(03)00216-8
Zheng, Q. Y., Johnson, K. R., and Erway, L. C. (1999). Assessment of hearing in 80 inbred strains of mice by ABR threshold analyses. Hear. Res. 130, 94–107. doi: 10.1016/s0378-5955(99)00003-9
Zhou, H., Q42an, X., Xu, N., Zhang, S., Zhu, G., Zhang, Y., et al. (2020). Disruption of Atg7-dependent autophagy causes electromotility disturbances, outer hair cell loss and deafness in mice. Cell Death Dis. 11:913. doi: 10.1038/s41419-020-03110-8
Zhu, C., Cheng, C., Wang, Y., Muhammad, W., Liu, S., Zhu, W., et al. (2018). Loss of ARHGEF6 causes hair cell stereocilia deficits and hearing loss in mice. Front. Mol. Neurosci. 11:362. doi: 10.3389/fnmol.2018.00362
Zine, A., Löwenheim, H., and Fritzsch, B. (2014). “Toward translating molecular ear development to generate hair cells from stem cells,” in Adult Stem Cells. Stem Cell Biology and Regenerative Medicine (New York: Springer), 111–161. doi: 10.1007/978-1-4614-9569-7_6
Zine, A., Messat, Y., and Fritzsch, B. (2021). A human induced pluripotent stem cell-based modular platform to challenge sensorineural hearing loss. Stem Cells 39, 697–706. doi: 10.1002/stem.3346
Keywords: OHC, IHC, SGN, cochlear nuclei, SOC
Citation: Elliott KL, Fritzsch B, Yamoah EN and Zine A (2022) Age-Related Hearing Loss: Sensory and Neural Etiology and Their Interdependence. Front. Aging Neurosci. 14:814528. doi: 10.3389/fnagi.2022.814528
Received: 13 November 2021; Accepted: 03 January 2022;
Published: 17 February 2022.
Edited by:
Benoit Laurent, Université de Sherbrooke, CanadaReviewed by:
Togay Muderris, Izmir Bakircay University, TurkeyCopyright © 2022 Elliott, Fritzsch, Yamoah and Zine. This is an open-access article distributed under the terms of the Creative Commons Attribution License (CC BY). The use, distribution or reproduction in other forums is permitted, provided the original author(s) and the copyright owner(s) are credited and that the original publication in this journal is cited, in accordance with accepted academic practice. No use, distribution or reproduction is permitted which does not comply with these terms.
*Correspondence: Bernd Fritzsch, YmVybmQtZnJpdHpzY2hAdWlvd2EuZWR1
Disclaimer: All claims expressed in this article are solely those of the authors and do not necessarily represent those of their affiliated organizations, or those of the publisher, the editors and the reviewers. Any product that may be evaluated in this article or claim that may be made by its manufacturer is not guaranteed or endorsed by the publisher.
Research integrity at Frontiers
Learn more about the work of our research integrity team to safeguard the quality of each article we publish.