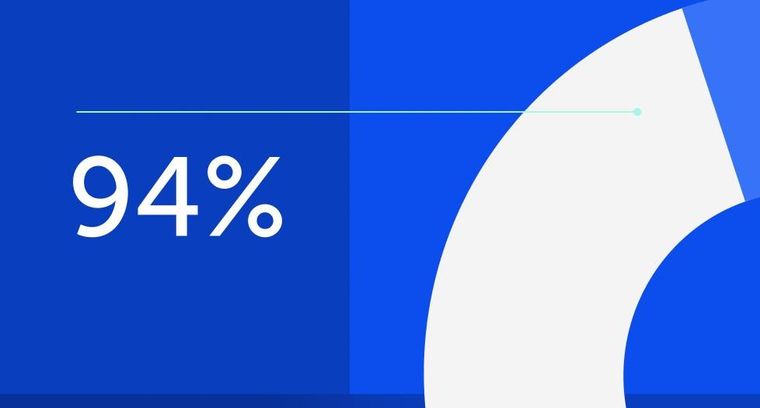
94% of researchers rate our articles as excellent or good
Learn more about the work of our research integrity team to safeguard the quality of each article we publish.
Find out more
REVIEW article
Front. Aging Neurosci., 02 November 2022
Sec. Cellular and Molecular Mechanisms of Brain-aging
Volume 14 - 2022 | https://doi.org/10.3389/fnagi.2022.1017299
This article is part of the Research TopicAdvances in Neural Reprogramming, Disease Modeling and Therapeutic InsightsView all 13 articles
Neurodegenerative disorders have been extremely challenging to treat with traditional drug-based approaches and curative therapies are lacking. Given continued progress in stem cell technologies, cell replacement strategies have emerged as concrete and potentially viable therapeutic options. In this review, we cover advances in methods used to differentiate human pluripotent stem cells into several highly specialized types of neurons, including cholinergic, dopaminergic, and motor neurons, and the potential clinical applications of stem cell-derived neurons for common neurodegenerative diseases, including Alzheimer’s disease, Parkinson’s disease, Huntington’s disease, ataxia, and amyotrophic lateral sclerosis. Additionally, we summarize cellular differentiation techniques for generating glial cell populations, including oligodendrocytes and microglia, and their conceivable translational roles in supporting neural function. Clinical trials of specific cell replacement therapies in the nervous system are already underway, and several attractive avenues in regenerative medicine warrant further investigation.
Age—it’s the one mountain you can’t overcome, and as the average life expectancy extends into the eighth decade, neurodegenerative diseases are becoming increasingly prevalent. Despite their increasing incidence, preventative or disease-modifying strategies for these emotionally and financially draining disorders are lacking. Due to the fundamental lack of regeneration within the central nervous system (CNS), neurodegenerative diseases relentlessly attacking discrete populations of neurons are excellent candidates for cell replacement therapies. Here, we review the current prospects on the application of pluripotent stem cell-derived cell types for the treatment of neurodegenerative disease.
Pluripotent stem cells provide a uniquely scalable source of functional somatic cells, including cells of the CNS, that can potentially replace damaged or diseased tissues. Although prospects for using stem cell derivatives seemed fanciful at the start of the millennium, approximately two decades later several clinical trials using cellular products of pluripotent stem cells are underway or about to reach the clinic (Gage and Temple, 2013; Kimbrel and Lanza, 2015; Steinbeck and Studer, 2015; Trounson and DeWitt, 2016). This progress has been facilitated through the development of robust methods for converting human pluripotent stem cells into the specific cell types that are lost in disease. Most techniques are based on fundamental principles learned from developmental biology and aim to recapitulate cell fate determination pathways in the culture dish, and these methods have been thoroughly reviewed elsewhere (Tao and Zhang, 2016). More recently, exogenous over-expression of transcription factors (TFs) has provided an alternative route to directed differentiation methodologies for generating specific classes of neurons. When appropriate, we will highlight both approaches that advance the field toward producing defined cellular populations, which are the ideal candidate for cell replacement therapies.
In this review, we summarize recent progress toward generating specific cell types from human pluripotent stem cells for regenerative medicine. The examples described herein are not intended to be all-inclusive, and readers are encouraged to examine other reviews on the clinical development of stem cell-based therapies (Gage and Temple, 2013; Kimbrel and Lanza, 2015; Steinbeck and Studer, 2015; Trounson and DeWitt, 2016). Rather, we focus on recent biotechnological advances in the derivation of human cells and their application as cell therapies in the field of neurodegeneration (Table 1). These selected studies illustrate the biological concepts, experimental approaches, and therapeutic possibilities of in vitro stem cell-derived cells of the neural (Figure 1) and glial (Figure 2) lineages. We conclude our review with a discussion of emerging technologies in the field, current limitations, and remaining challenges for regenerative medicine in translational neurosciences.
Figure 1. Pluripotent stem cell differentiation strategies for producing vulnerable neuronal cell types. Basal forebrain cholinergic: diff. 1–4 Bissonnette et al., 2011; Liu et al., 2013a,b; Hu et al., 2016; transpl. 2 Liu et al., 2013a. Cortical glutamatergic: diff. 5–9 Espuny-Camacho et al., 2013; Zhang et al., 2013; Cao et al., 2017; Qi et al., 2017; Nehme et al., 2018; transpl. 5–7, 10 Espuny-Camacho et al., 2013; Zhang et al., 2013; Espuny-Camacho et al., 2017; Qi et al., 2017. GABAergic inhibitory: diff. 11–15 Maroof et al., 2013; Nicholas et al., 2013; Chanda et al., 2014; Sun et al., 2016; Yuan et al., 2018; transpl. 16–17 Cunningham et al., 2014; Anderson et al., 2018. Dopaminergic: diff. 18–22 Cai et al., 2009; Caiazzo et al., 2011; Kriks et al., 2011; Pfisterer et al., 2011; Kim et al., 2021; transpl. 23–25 Grealish et al., 2014; Kikuchi et al., 2017; Wakeman et al., 2017. Medium Spiny: diff. 26–29 Aubry et al., 2008; Berkowitz et al., 2016; Ma et al., 2012; Victor et al., 2014; transpl. 26, 27, 29 Aubry et al., 2008; Berkowitz et al., 2016; Victor et al., 2014. Hypothalamic: diff. 30–33 Merkle et al., 2015; Wang et al., 2015b; Kirwan et al., 2017; Rajamani et al., 2018; transpl. 30 Merkle et al., 2015. Hippocampal: diff. 34–36 Yu et al., 2014; Sakaguchi et al., 2015; Hiragi et al., 2017; transpl. 34 Yu et al., 2014. Serotonergic: diff. 37–39 Lu et al., 2016; Vadodaria et al., 2016; Xu et al., 2016; transpl. 40 Carlsson et al., 2009. Purkinje: diff. 41–45 Muguruma et al., 2015; Wang et al., 2015a; Ishida et al., 2016; Watson et al., 2018; Silva et al., 2020; transpl. 46 Higuera et al., 2017. Motor: diff. 47–55 Amoroso et al., 2013; Hester et al., 2011; Son et al., 2011; Du et al., 2015; Lippmann et al., 2015; Maury et al., 2015; Goto et al., 2017; Klim et al., 2019; Limone et al., 2022; transpl. 55–56 Yohn et al., 2008; Corti et al., 2012.
Figure 2. Pluripotent stem cell differentiation strategies for producing glial cells. Astrocytes: diff. 1–8 Krencik and Zhang, 2011; Shaltouki et al., 2013; Tcw et al., 2017; Canals et al., 2018; Li et al., 2018; Tchieu et al., 2019; Barbar et al., 2020; transpl. 9–11 Lepore et al., 2008; Song et al., 2017; Baloh et al., 2022. Oligodendrocytes: diff. 12–17 Wang et al., 2013; Douvaras et al., 2014; Douvaras and Fossati, 2015; Ehrlich et al., 2017; García-León et al., 2018; Marton et al., 2019; transpl. 12, 14, 18–19 Wang et al., 2013; Douvaras et al., 2014; Thiruvalluvan et al., 2016; Windrem et al., 2020. Microglia: diff. 20–28 Muffat et al., 2016; Abud et al., 2017; Douvaras et al., 2017; Haenseler et al., 2017; Pandya et al., 2017; Takata et al., 2017; Chen et al., 2021; Limone et al., 2021; Dolan et al., 2022; transpl. 29–30 Svoboda et al., 2019; Xu et al., 2020.
Parkinson’s disease (PD) is characterized by the degeneration of several neuronal subtypes, most notably the dopaminergic neurons of the substantia nigra pars compacta (SNpc), located in the ventral midbrain. These neurons project to the dorsal striatum of the basal ganglia and function in motor control, and the loss of these neurons contributes to the movement symptoms observed in the initial stages of PD. Fetal-derived dopamine neurons have had promising clinical benefits for PD patients (Hallett et al., 2014). To avoid the ethical and logistical issues associated with fetal tissue transplants, the application of pluripotent stem cells to generate dopaminergic neurons has been a long-standing goal. Indeed, translational research to bring these specific neurons to the clinic has far exceeded the other cell replacement strategies discussed here and recent advances have extensively been discussed elsewhere (Barker et al., 2017; Kim et al., 2020). In this section we will provide a summary of the most relevant discoveries that led to the first transplantation studies with hiPSC-derived cells that established a road map for the field.
From the initial basic science studies that furnished the directed differentiation strategies of dopaminergic neurons to their large-scale production in GMP-facilities for transplantation studies, the research program for midbrain dopamine neurons has made excellent progress. Several groups developed methods to produce FOXA2/LMX1A-positive midbrain neurons capable of releasing dopamine (Arenas et al., 2015). For example, the Studer group has developed a highly efficient protocol for producing these neurons by combining dual-SMAD inhibition with activation of SHH and FGF8 signaling. The critical step in midbrain specification is the strong activation of WNT signaling achieved using a GSK3β inhibitor (Kriks et al., 2011; Kim et al., 2021). Transcription factors, such as LMX1A, can also be used to enhance directed differentiation approaches (Cai et al., 2009), or for the direct reprogramming of fibroblasts into dopaminergic neurons (Caiazzo et al., 2011; Pfisterer et al., 2011), and combined with cell sorting methods to further enrich for midbrain dopaminergic neurons (Arenas et al., 2015). Preclinical studies demonstrate that human iPS cell-derived dopaminergic neurons are safe and efficacious in both rodent and primate PD models (Kikuchi et al., 2017; Wakeman et al., 2017) with similar efficacy to fetal-derived tissue (Grealish et al., 2014). A number of clinical trials with stem cell-based therapies are currently being planned with their details summarized at a recent consortium meeting (Barker et al., 2017). Although PD patients receiving the stem cell-derived dopaminergic neurons will likely show improvements in movement symptoms, their additional symptoms, including depression, fatigue, visual hallucinations, and sleep disturbances, might persist due to continued degeneration of other neuronal types. This has led to some to propose serotonergic neurons (Lu et al., 2016; Vadodaria et al., 2016; Xu et al., 2016) as an additive cellular therapy for PD (Politis and Loane, 2011). A delicate balance must be struck between dopaminergic and serotonergic neurons, however, as fetal grafts with high levels of serotonergic neurons have been associated with graft-induced dyskinesias in parkinsonian rats (Carlsson et al., 2009).
Neurological conditions involving both memory loss and impaired judgment are classified as dementia (Yue and Jing, 2015). Alzheimer’s disease (AD) is the most common type of dementia in individuals older than 65 years old and the most prevalent neurodegenerative disease (Table 1). The incidence of AD dramatically increases with age, and with the aging US population, it is estimated that approximately 14 million individuals will be affected by 2050. AD often first manifests clinically as impairments with short-term memory, and later affects behavior and language. Current treatments are aimed at ameliorating these symptoms without substantially affecting disease course. Cognitive decline is associated with progressive degeneration of neurons in the limbic system (especially the hippocampus and connected entorhinal cortex), the basal forebrain, and neocortical areas. Histologically, patient brains are characterized by the accumulation of extracellular beta-amyloid depositions and intracellular tau-positive neurofibrillary tangles as well as neuritic plaques that contain both tau within dystrophic neurites and beta-amyloid. Neuropathological studies strongly suggest that AD has well-defined and consistent spatiotemporal pattern of neurofibrillary degeneration, in most cases, that begins in the entorhinal cortex and spreads to pyramidal neurons in the hippocampus and then neocortical areas, with association areas affected sooner and more severely. Currently, there is no effective therapy to block the progression of AD making it a major looming public heath challenge.
One of the earliest cell types perturbed by AD is the basal forebrain cholinergic neuron (BFCN). These neurons, which arise from the median ganglionic eminence (MGE) during development, are responsible for various aspects of cognition including learning, memory, and attention. At the molecular level, BFCNs are primary cholinergic neurons and innervate the cerebral cortex, hippocampus, and amygdala, and play critical roles in processing information related to cognitive function (Martinez et al., 2021). Transplantation of fetal cholinergic tissue from rats into the cortex of lesioned primates has been shown to restore memory deficits suggesting a potentially therapeutic roles for these cells (Ridley et al., 1994).
Several methods to differentiate pluripotent stem cells into BFCNs have been described (Bissonnette et al., 2011; Liu et al., 2013a,b; Hu et al., 2016). Typically, first forebrain neural progenitors are obtained and then treated with a SHH agonist and FGF8 to coax the cells into expressing the transcription factors Nkx2.1, consistent with a ventral medial ganglionic eminence (MGE) neural progenitor identity. Subsequent culture of these progenitor cells on glia or treatment with BMP9 then yields a mixture of neurons containing BFCNs (Bissonnette et al., 2011; Liu et al., 2013a,b; Hu et al., 2016). Alternatively, overexpression of the transcription factors Lhx8 and Gbx1 can convert the progenitors into BFCNs (Bissonnette et al., 2011). Cells produced using these methods express markers consistent with a cholinergic identity and exhibit expected electrophysiological profiles. In one study, MGE-progenitor cells transplanted into mouse brains differentiated into neurons, including BFCNs, and formed synaptic connections (Liu et al., 2013a). More importantly, injection of these precursor cells led to learning and memory improvements in lesioned mice (Liu et al., 2013a). Whether these improvements were the specific result of the BFCNs or other cell types remains to be determined but this study provides an important proof-of-principle for the use of stem cell-based therapy to improve cognition.
Cerebral cortex development consists of three major processes: cell proliferation, neuronal migration, and cortical organization into multiple well-defined layers. The cerebral cortex contains two major classes of neurons; a majority population of excitatory glutamatergic projections neurons that arise during development from the dorsal telencephalon, which is the developmental precursor to the cerebral cortex, and a minor population of inhibitory interneurons. Through successive waves of neurogenesis, these neurons generate the six layers of the neocortex, which can be further functionally divided based on specific patterns of axonal output and dendritic input. Due to their abundance and ability to project long distances, cortical pyramidal neurons, named for their shape, are able to integrate and send information across the entire nervous system (Bekkers, 2011).
The production of pyramidal neurons from pluripotent stem cells is considered to be a default differentiation fate because it occurs in the absence of exogenous signaling factors (Espuny-Camacho et al., 2013). Inhibiting certain signaling pathways, however, can enhance the yield of cortical glutamatergic neurons by suppressing the emergence of inhibitory interneurons (Cao et al., 2017). More recently, accelerated methods for generating cortical neurons have been reported. One method relies on a cocktail of molecules to both pattern the cells to dorsal forebrain lineage and then inhibit neural stem cell self-renewal to drive neurogenesis, which preliminary data suggests can be timed to achieve the production of neurons of different cortical layers (Qi et al., 2017). Forced expression of the transcription factor Ngn2 in stem cells further accelerates the differentiation to yield very pure populations of glutamatergic neurons (Zhang et al., 2013) that can be enhanced with the addition of developmental cues (Nehme et al., 2018). Transcriptional studies suggest this method favors the production of upper layer neurons, therefore additional methods to achieve the full diversity of cortical layers may still be necessary. After injecting into the postnatal mouse brain, human cortical neurons generated using the methodologies described above displayed proper, long-distance projection patterns and integrated functionally within the host’s circuitry (Espuny-Camacho et al., 2013; Zhang et al., 2013; Qi et al., 2017). Whether they can ameliorate disease phenotypes in animal models remains an unanswered question, but neurons transplanted into a murine AD model display pathological hallmarks of the disease including altered tau biochemistry (Espuny-Camacho et al., 2017).
In both the brain and spinal cord, gamma-aminobutyric acid (GABA)-releasing interneurons are the major class of inhibitory neurons and play crucial roles in modulating neural circuits. There are many distinct subtypes of interneurons that differ in their synaptic connections, expression of neuropeptides, neurotransmitter machinery, and developmental origin with some immature interneurons having the remarkable ability to migrate and disperse long distances to integrate throughout the CNS (Southwell et al., 2014). This integrative property makes interneurons a promising candidate for cell replacement therapies.
Several groups have developed directed differentiation approaches for producing interneurons from human pluripotent stem cells (Liu et al., 2013b; Maroof et al., 2013; Nicholas et al., 2013). These approaches typically inhibit both branches of SMAD signaling as well as WNT signaling using small molecules to achieve robust forebrain induction into cells resembling the MGE, as suggested by expression of the transcription factor Nkx2.1. Careful timing of SHH activation then allows for induction of ventral cell fate in these progenitor cells that develop into GABAergic interneurons as opposed to basal forebrain cholinergic neurons (Liu et al., 2013b). In addition to directed differentiation approaches, transcription factor-mediated inductions of interneurons from stem cells have also been described (Chanda et al., 2014; Sun et al., 2016; Yuan et al., 2018). Minimally, transient expression of ASCL1 and DLX2 can convert stem cells into GABAergic interneurons. When injected into the mouse brain, these cells, migrated, integrated, and matured into a variety of interneuronal subtypes, including expression of the mature subtype markers parvalbumin or somatostatin. Further studies, such as single-cell transcriptomic approaches, are needed to characterize the full repertoire of subtypes of interneurons that can be obtained from pluripotent stem cells. Impressive studies have gone on to show that transplanted interneurons were capable of improving memory (Anderson et al., 2018) and in some cases suppressing seizures and abnormal behaviors in an epileptic mouse model (Cunningham et al., 2014). Based on these promising studies, one biotech company, Neurona Therapeutics, is pioneering the clinical uses for interneuron-based cell therapies for epilepsy and neuropathic pain.
Composed of granule and pyramidal neurons, the hippocampus plays a critical role in learning and memory. It is also an area of the brain that deteriorates in AD, additional forms of dementia, and other age-related cognitive declines of distinct etiologies. Interestingly, in addition to the subventricular zone, the dentate gyrus of the hippocampus is a unique site of adult neurogenesis (although the absolute rate of neurogenesis remains controversial). Therefore, incorporation of immature stem cell-derived neurons into existing neural circuity beyond embryonic development is a hopeful prospect.
To generate hippocampal neurons, stem cells are patterned to dorsal forebrain progenitors by inhibiting both branches of the SMAD signaling as well as factors to promote WNT and SHH signaling. Subsequently, WNT3a is applied along with BDNF to drive the neurogenesis of hippocampal granule neurons (Yu et al., 2014; Sakaguchi et al., 2015; Hiragi et al., 2017). Initial findings indicate concurrent WNT and BMP activation can drive the differentiation of the dorsal forebrain progenitors into pyramidal neurons (Sakaguchi et al., 2015). Rodent transplantation studies with hippocampal neural precursors revealed that the human neurons could integrate into the dentate gyrus (Yu et al., 2014), but it remains to be determined if these xenografts can affect disease-related phenotypes in animal models.
Huntington’s disease (HD) is caused by a CAG trinucleotide repeat expansion within the coding region of the HTT gene, resulting in an extended polyglutamine (polyQ) tract within the Huntingtin protein. The progressive loss of neurons and gross atrophy in the neostriatum (caudate nucleus and putamen) disrupts neuronal circuits involving the basal ganglia and leads to gradually worsening motor impairment and, as additional brain regions are affected, significant cognitive and psychiatric symptoms.
Medium spiny neurons that reside in the striatum, contribute to the complex circuits that control movement and are particularly vulnerable in HD. During development, these inhibitory neurons arise from the lateral ganglionic eminence (LGE) and are marked by the expression of DARPP32 (dopamine- and cAMP-regulated phosphoprotein Mr∼32 kDa) (Fjodorova et al., 2015). The relatively specific loss of DARPP32+ medium spiny class of neurons in the neostriatum makes HD a strong candidate for cell replacement therapies. Like for PD, fetal transplants have paved the way for stem cell-derived therapies for HD (Freeman et al., 2000).
Numerous groups have validated directed differentiation approaches for producing medium spiny neurons from stem cells (Aubry et al., 2008; Carri et al., 2012; Ma et al., 2012). Like the methods for producing other inhibitory neurons from the neighboring MGE, combinatorial SHH/WNT signaling modulation induces an anterior-ventral fate. Of note, reduced activation of SHH signaling and the addition of Activin A can favor a LGE fate while inhibiting a MGE fate (Fjodorova et al., 2015). A direct conversion method has also recently been described for transforming fibroblasts into medium spiny neurons, specifically, with a combination of 4 transcription factors (CTIP2, DLX1, DLX2, and MYT1L) and two microRNAs (miR-9/9 and miR-124) (Victor et al., 2014). Whether these direct programming methods can be applied to pluripotent stem cells remains to be determined but could be used to improve the yield of medium spiny neurons from stem cells, which are at best ∼50%. When transplanted into a murine striatum, the neurons integrate into the host circuit and project to the proper anatomical targets. In some cases, the transplanted cells neurons can rescue motor deficits in quinolinic acid, an excitotoxin, striatal-lesioned mice, a model of HD (Carri et al., 2012; Victor et al., 2014). In another study, however, the transplanted cells also resulted in cellular overgrowth (Aubry et al., 2008). Based on these studies, refined purification methods to yield more homogenous neuron populations followed by additional animal model studies seem warranted.
Spinocerebellar ataxias (SCAs) are a clinically and genetically heterogenous group of neurological disorders associated with impairments in motor coordination due to degeneration of the cerebellum and connected neuronal pathways. Many SCAs are caused by CAG nucleotide repeat expansions within certain genes leading to the production of polyglutamine (polyQ)-containing proteins with putative toxic gain-of-function effects. For instance, an autosomal dominantly-inherited, abnormally long (>33 CAG repeats) trinucleotide repeat expansion within ATXN-2 results in SCA2 that can manifest with ataxia, loss of neurological reflexes, and Parkinsonian symptoms. Ataxias can be associated with other inherited disorders. For examples, an autosomal recessively-inherited GAA trinucleotide repeat expansions in FXN, encoding frataxin, cause Friedrich’s ataxia, which is characterized by progressive ataxia, impaired speech, loss of vibratory and proprioceptive sensation due to degeneration of spinal cord neurons and nerve fiber tracts connecting to the cerebellum. There are no effective treatments for these debilitating and often fatal diseases.
Purkinje cells are large inhibitory GABAergic neurons with extensive dendritic arbors that reside within the hindbrain structure of the cerebellum. As the output neurons of the cerebellar cortex, they project to neurons within deep cerebellar nuclei and play an important role in motor coordination. Until recently, the differentiation of human PSCs into Purkinje neurons remained elusive, perhaps due to their late emergence during development. An initial directed differentiation approach for this cell type required several steps and many factors. First, exogenous factors were employed to stimulate endogenous Wnt1 and FGF8 signaling and promote a midbrain/hindbrain identity, and inhibition of SHH signaling was used to pattern cells toward a dorsal identity (Muguruma et al., 2015; Wang et al., 2015a). Then, the maturation process could be accomplished through several methods: plating precursors on mouse cerebellar slice cultures (Watson et al., 2018), within self-organizing, polarized cerebellar structures (Muguruma et al., 2015), or more recently in a defined basal medium optimized for cell culture (Bardy et al., 2015; Silva et al., 2020). Studies indicate that the stem cell-derived Purkinje cells are susceptible to genetic insults, such as the trinucleotide CAG repeat in CACNA1A associated with SCA6 (Ishida et al., 2016), that trigger their selective demise, and that they can also engraft into the mouse cerebellum (Wang et al., 2015a). Although more defined and robust methods are needed before cell replacement therapies should be considered clinically, the initial findings have paved the way for producing this neuronal type that is relevant to many neurological disorders.
The specific loss of motor neurons underlies several devastating neurological diseases including amyotrophic lateral sclerosis (ALS) and spinal muscular atrophy (SMA). Both diseases involve the progressive loss of motor function, eventually progressing to fatal paralysis. In nearly all (∼97%) of cases of ALS, motor neurons in both the brain and spinal exhibit pathological changes in the cellular localization of the RNA binding protein TDP-43, which include loss of the normal nuclear localization and the formation of cytoplasmic inclusions (Klim et al., 2021).
Motor neurons represent a diverse group of neuronal subtypes and provide the pivotal link between mind and the animation of the body. Generally, there are two types of motor neurons; upper motor neurons that reside in the frontal cortex and project to lower motor neurons, found in the ventral brainstem and spinal cord, which in turn form synapses with the musculature. Decades of developmental studies and genetic analyses have illuminated the molecular underpinnings of lower motor neuron specification during embryo development (Dasen and Jessell, 2009) with the morphological gradients well established (Davis-Dusenbery et al., 2014).
Leveraging this knowledge, stem cell scientists developed methods to generate motor neurons from mouse embryonic stem cells by applying retinoic acid (RA) to caudalize the cells toward a spinal cord (the distal or tail end of the neural tube) identity and activating SHH to ventralize them toward a motor, rather than sensory, identity (Wichterle et al., 2002). Several research groups have advanced these earlier findings to reproducibly convert human pluripotent stem cells into vast quantities of motor neurons (Amoroso et al., 2013; Du et al., 2015; Maury et al., 2015; Klim et al., 2019). These approaches typically rely on neural induction through small molecule dual-SMAD signaling inhibition, in some cases activation of WNT signaling, accelerated neurogenesis through inhibition of FGF or NOTCH signaling, all coupled with MN patterning described above (RA and SHH). Son et al. (2011) have used a large cadre of MN-related transcription factors (Isl1, Ascl1, Myt1l, Brn2, Ngn2, Lhx3, and Neurod1) to directly convert fibroblasts into induced motor neurons. Alternatively, simpler protocols were achieved that used a subset these factors to transform human stem cells into motor neurons (Hester et al., 2011; Goto et al., 2017). Recently, we have also shown that transcription factor-based and small molecule approaches could be combined to yield a highly pure population of cervical-like motor neurons from iPSCs with 100% efficiency through the inducible expression of Ngn2 (neurogenin-2) alone coupled with RA and SHH treatments (Limone et al., 2022). Interestingly, carefully varying the timing of retinoid application has been demonstrated to afford more caudal motor neuron fates (Lippmann et al., 2015), but methodologies for producing upper motor neurons, also known as cortical spinal motor neurons (CSMNs), are still lacking. As degeneration of cortical and spinal cord motor regions occur in ALS, a full array of motor neuron subtypes might be needed as a cell replacement herapy.
So far, motor neuron transplant results have been encouraging. For example pioneering transplant studies demonstrate that mES-derived motor neurons injected into tibial nerve of adult mice can form functional NMJs and ameliorate muscle atrophy (Yohn et al., 2008). Another notable study was able to transplant human iPS cell-derived motor neurons into the ventral horns of an SMA mouse model (Corti et al., 2012). The transplanted motor neurons could survive and engraft into the murine spinal cord and could even ameliorate disease phenotypes and extend the life span relative to those receiving a fibroblast transplant (Corti et al., 2012). These exciting initial studies highlight the need for large animal models for testing motor neuron-based cell therapies.
Although glia are more abundant than neurons, nuances remain in our understanding of how their exact cellular identities are established and how glial developmental pathways can be recapitulated in vitro for cell replacement approaches. Three main types of glia exist in the CNS: astrocytes, oligodendrocytes (OLs), and microglia. In brief, astrocytes are responsible for forming and modulating the blood–brain barrier (BBB) and modifying the chemical microenvironment governing synaptic function. Microglia are the resident immune cells of the CNS that function in synaptic pruning during development, immune surveillance, debris clearance and defense from pathogens. Oligodendrocytes are responsible for myelinating axons in the CNS, thereby maintaining strong electrical connectivity of brain circuitry. Glia have been implicated in almost all neurodegenerative diseases, and their dysfunction in this context are more extensively reviewed elsewhere (Zheng et al., 2018). Glial transplantation for the treatment of neurodegenerative diseases has been explored much less than for neurons, though might be advantageous for ameliorating glial dysfunction as well as mitigating the loss of degenerating neurons by engaging in supportive roles. Like neurons, glial cells can be generated by activating development cues or overexpression of cell type-specific transcription factors. We will discuss a selection of strategies to generate glia and their most promising applications to neurodegenerative diseases.
Astrocytes are star-shaped glial cells that reside in both the brain and spinal cord to maintain BBB integrity, regulate nutrient flow, and govern neuronal function. They arise relatively early in neuronal development from radial glial progenitor cells usually after these cells have generated neurons. Broadly, differentiation protocols recapitulate developmental cues (Krencik et al., 2011; Shaltouki et al., 2013) by promoting neuronal stem cell (NSC) identity via dual SMAD inhibition and then gliogenesis with morphogens (Krencik and Zhang, 2011). Promoting gliogenesis after NSC differentiation has traditionally been a slow rate-limiting step in the generation of astrocytes, but recent transgenic and chemical strategies have greatly accelerated this process. Expansion of NSCs with Activin A, Heregulin 1β (Neuregulin1), and IGFI (Shaltouki et al., 2013; Tcw et al., 2017), flow cytometry-based enrichment strategies (Barbar et al., 2020) or overexpression of TFs NFIA and SOX9 can dramatically shorten differentiation protocols (Canals et al., 2018; Tchieu et al., 2019). hPSC-derived astrocyte-like cells can be generated in as little as 30 days and show functional properties similar to primary astrocytes in that they uptake glutamate, promote neurite outgrowth, propagate calcium waves, and retain their identity in vivo (Krencik and Zhang, 2011; Shaltouki et al., 2013; Li et al., 2018). Many groups have recently developed methods to increase maturity and function of these cells by differentiating them from 3D structures coupled with cell sorting methods (Barbar et al., 2020).
Studies on ALS and PD animal models are laying the foundation for astrocyte transplantation therapies. In ALS models, astrocytes exert toxic gain-of-function effects that can act in a cell non-autonomous manner to contribute to motor neuron degeneration (Di Giorgio et al., 2007, 2008; Meyer et al., 2014; Hall et al., 2017). For instance, mice expressing human mutant SOD1 in astrocytes in addition to neurons had reduced to survival compared to mice only expressing mutant SOD1 in neurons, in other words, a wild-type astrocyte microenvironment may promote motor neuron survival (Bataveljić et al., 2012). Focal transplantation of glial-restricted NPCs (Neuronal Progenitor Cells) into the cervical spinal cord of SOD1 transgenic rats during disease progression extended survival and decreased motor neuron death, in part due to the partial rescue of GLT1 expression in astrocytes (Clement et al., 2003). Clinical trials are ongoing to prove the efficacy of transplanted PSC-derived astrocytes to boost neuronal survival and slow disease progression. For instance, a phase 1/2a trial in a small cohort of ALS patients (NCT02943850) has shown that a single injection of human NPCs engineered to produce glial cell line-derived neurotrophic factor (GDNF) into the spinal cord is safe, and viable grafts differentiated into astrocytes that may be neuroprotective through increased GDNF production (Baloh et al., 2022).
Transplantation studies for PD also showed promising results. Co-transplantation of primary fetal NPCs and rat astrocytes increased long-term engraftment of mature midbrain dopaminergic neurons and increase anti-inflammatory markers in the brains of PD rats (Lepore et al., 2008). Transplantation of primary astrocytes into the SNpc increase synaptosomal dopamine uptake in the striatum, reduce ROS stress, and improved motor deficits of pharmacologically-induced PD rats (Song et al., 2017). These observations suggest hPSC-derived astrocytes may be used to slow disease progression and complement dopaminergic neuron transplantation.
Similar to astrocytes, oligodendrocytes are derived in development after neurogenesis. In both the forebrain and the spinal cord, oligodendroglial progenitor cells (OPCs) are generated from Nkx2.1+, SHH-derived progenitors and their differentiation is regulated by TFs Olig1 and Olig2. OPCs have an immense ability to migrate and populate the entire brain and spinal cord where most of them further differentiate into committed, myelinating oligodendrocytes (OLs) while a small subset of them are maintained in a progenitor state. Their great migratory abilities, plasticity and pivotal role in neuronal support render these cells ideal for transplantation studies and replacement therapies.
To generate OLs, hPSCs are first converted to a neural stem cell with small molecules or a neural epithelial identity through SHH activation and are then pushed toward an oligodendrocyte progenitors (OPCs) identity by the addition of PDGF-AA. These OPCs can be matured into OLs by various cocktails of small molecules, often containing IGF-1 and T3 (Wang et al., 2013; Douvaras et al., 2014; Douvaras and Fossati, 2015). Several groups have shown that complete maturation of OPCs into highly myelinating oligodendrocytes can be achieved either by injecting these cells in vivo (Douvaras et al., 2014) or by differentiating these cells in 3D structures (Marton et al., 2019). Protocols relying on overexpression of several transcription factors, including OLIG2, NKX6.2, and SOX10, were developed to be faster and similarly efficient (Ehrlich et al., 2017; García-León et al., 2018). García-León et al. (2018) found, however, that overexpression of SOX10 alone in NSCs was the most efficient at generating OLs in as little as 20 days, and the generated OLs were capable of myelinating cortical neurons both in vitro and in vivo.
Stem cell-derived OLs hold promise for both demyelinating diseases and spinal cord injury. Multiple sclerosis (MS) is a chronic, autoimmune disease characterized by the loss of myelin and associated oligodendrocytes, often in a remitting and relapsing clinical course that results in gradual neurological decline. MS-iPSC-derived OPCs can myelinate the corpus callosum of immunocompromised hypomyelinated (shiver) mice (Wang et al., 2013; Douvaras et al., 2014), offering a potential regenerative route for re-myelination for cases of MS that are resistant to immune-suppressant treatment. Strikingly, human iPSC-derived OPCs can myelinate axons in a non-human primate marmoset model (Thiruvalluvan et al., 2016). Long term transplantation studies in both shiver mice and demyelinating cuprizone treatment also showed that these cells can not only migrate to distal regions of the CNS farther than previously believed but can also improve behavior and motor function in murine models (Windrem et al., 2020). These results highlight the feasibility of an iPSC-derived OL transplantation therapy for MS and perhaps for other demyelinating diseases.
Unlike other glial cells, microglia are immune cells not derived from the neuroectoderm but originate from the embryonic yolk sac in early stages of development and then migrate to the neural tube (Ginhoux et al., 2010; Kierdorf et al., 2013). Chemical differentiation strategies generally generate early myeloid progenitors by isolation of delaminating cells from so-called yolk-sac embryoid bodies (Muffat et al., 2016; Haenseler et al., 2017) or by promoting hematopoiesis with hypoxic conditions and defined medias (Abud et al., 2017). Initial studies used co-cultures of these immature myeloid cells with human neurons or murine brain extracts to generate resident brain-like microglia (Takata et al., 2017). These protocols made scalability challenging so others have devised ways to further push immature myeloid progenitors toward microglia-like cells (MGLs) with defined medias containing M-CSF to generate myeloid cells coupled with CNS-enriched TGF-beta and CNS-specific, CSF1-receptor ligand IL34 to promote a brain-like specification of these myeloid progenitors. Generated MGLs show competence to phagocytose (Muffat et al., 2016; Abud et al., 2017; Douvaras et al., 2017; Haenseler et al., 2017; Pandya et al., 2017; Limone et al., 2021; Dolan et al., 2022) respond to IFN-γ and LPS stimulation via secretion of pro-inflammatory cytokines (Muffat et al., 2016; Abud et al., 2017), and migrate to sites of injury (Muffat et al., 2016). When co-cultured with neurons, MGLs have also been observed to secrete anti-inflammatory and pro-remodeling cytokines (Haenseler et al., 2017). Like for other glial cells, transcription factor-based protocols may offer increased efficiency and decreased time for the generation of microglial-like cells. One study has shown that overexpression of transcription factors CEBPA and PU.1 coupled with CNS-patterning molecules described above can generate Microglia-like cells from human iPSC (Chen et al., 2021) with a second one showing improved efficiency by overexpressing PU.1 from primitive hematopoietic progenitors (Sonn et al., 2022). A recent study has defined a set of six transcription factors for the generation of microglia-like cells at a scale sufficient for genetic screening (Drager et al., 2022). Following the progress in the derivation of specific neuronal populations, it is plausible that newer approaches might find that just a few transcriptional factors could be sufficient, when coupled with small molecules, for the generation of this cell type.
Long term engraftment studies have been rendered difficult by the lack of homology between murine and human CSF1, which is pivotal for long term microglial survival. However, initial studies have shown the feasibility of transplantation of hiPSC-derived iMGLs in humanized mouse models (Svoboda et al., 2019; Xu et al., 2020).
Directed differentiation approaches have evolved considerably since the initial derivation of neurons from human embryonic stem cells (Zhang et al., 2001). Although defined culture conditions that primarily employ small molecules instead of poorly defined co-culture systems are more robust, modern directed differentiation approaches still tend to yield highly heterogeneous cultures containing the cell type of interest along with developmentally related cells. Direct conversion strategies like the ones described above typically yield more homogenous cell populations, but viral integration could disrupt normal gene expression and thus might not be amenable to clinical applications. Alternatively, the use of cell surface antibodies for sorting different neural populations has been pioneered to enrich for more defined cell populations (Yuan et al., 2011), or dyes that are selectively taken up by specific cells could theoretically also be used to mark specific cell types as has been demonstrated for neural precursor cells (Yun et al., 2012). These advances have led to several of these differentiation protocols being used for modeling neurodegeneration in different cell types in vitro (Giacomelli et al., 2022), opening the door to their adaptation to transplantation studies in the future. Additionally, several groups have made significant progress in the development of protocols for the generation of 3D structures containing various CNS cell types (known as brain organoids) that can enhance cell type specification and maturation (Del Dosso et al., 2020). Whether this technology can be translated into reproducible, manufacturable products for transplantation studies remains unclear, though it does offer a myriad of intriguing possibilities for the field.
It is unclear whether nascent, immature neurons or elaborate, mature neurons will integrate more successfully into a degenerating brain to provide therapeutic benefit. Either way, the ability to control the functional maturation of stem cell-derived neurons would benefit many applications. For in vitro disease modeling studies, we have found that co-culture of human neurons with murine glial cells effectively increased neuronal activity, but co-culture with non-human cells is not an ideal strategy for cell replacement therapies. Instead, Gage and colleagues have developed a defined neuronal medium, BrainPhys, which better mimics the environment present in healthy human brains and enhances both spontaneous electrical and synaptic activity of human neurons (Bardy et al., 2015). Whether increased activity translates into increased survival after transplantation remains an unanswered but fascinating question.
The process of reprogramming adult cells back to the pluripotent state erases many aspects of aging that put vulnerable cells at risk in the first place (Mertens et al., 2018). Although resetting the biological clock makes disease modeling more challenging, it might rid the newly derived cells from the neurodegenerative stimuli of aging when transplanted. Still, there might be aspects of maturation that are critical for neuronal integration or function. Unlike stem cell-derived neurons, for example, neurons directly converted from adult fibroblasts capture the faithful expression of all tau isoforms detected in adult brains at the proper ratios (Capano et al., 2022). Direct conversion of adult cells to replace lost neurons might therefore be an alternative technology to consider and has even been shown to reverse symptoms of PD in a rodent model by converting midbrain astrocytes to dopaminergic neurons (Qian et al., 2020).
Induced pluripotent stem cell technology marshaled in the possibility of personalized regenerative medicine using therapies based on an individual’s own cells. To this end, investigators in Japan started a clinical trial to treat age-related macular degeneration using autologous transplants, however, the trial was eventually suspended after treating one patient (Mandai et al., 2017). Several hurdles generate significant headwinds for this type of approach including (1) the time and effort needed to generate iPS cells, (2) genomic instability of pluripotent stem cells, and (3) the cost of personalized therapeutics. Most of these hurdles have several potential solutions that we will describe here briefly.
Despite recent advances, the overall time to move from the collection of fibroblasts via skin biopsy in the clinic, the reprogramming of fibroblasts into PSCs with completion of appropriate quality controls, to the differentiation of individualized stem cells into a personal population of a specific cell type, such as mature motor neurons, remains extensive, and hence possibly beyond the therapeutic window for rapidly progressive neurodegenerative diseases like ALS. To meet the demands of future clinical applications, state-of-the-art technologies for the cryopreservation of differentiated cell types are being tested to provide a ready to go off-the-shelf product (Holm et al., 2010; Nishiyama et al., 2016). Indeed, this approach is being pioneered within the PD cell replacement field, which has demonstrated that cryopreserved iPSC-derived neurons can maintain high viability and the molecular properties of a dopaminergic neuron. Moreover, these cryopreserved cells can be directly transplanted into a rat model of PD to reverse functional deficits (Wakeman et al., 2017).
For cell replacement therapies, even rare proliferating cells are especially worrisome because they could ultimately lead to the growth of tumors. Moreover, genomic instability of pluripotent stem cells has long been a concern for the field as aneuploid cells have readily been observed (Draper et al., 2004). To identify more subtle genetic changes, groups have performed whole-exome sequencing on many of the hES cell lines listed on the US National Institutes of Health registry and reported the acquisition of dominant negative p53 mutations, a mutation associated with many cancers, for several hES cell lines (Merkle et al., 2017), and other genomic changes associated with cancer and tumorigenesis (Merkle et al., 2022). Similar studies have also identified recurrent mutations that can occur during the reprogramming process and subsequent propagation (Pera, 2011). Therefore, thoughtful genetic characterization should be standard before stem cells or any of their derivatives are used in the clinic. This analysis will not only be useful to rule out stem cell lines with potentially dangerous mutations but could also be used after transplant to retrospectively identify the distribution of the donor cells.
To overcome the laborious nature of converting somatic cells into pluripotent stem cells, the New York Stem Cell Foundation has developed an automated platform for the high throughput conversion of skin biopsies into iPS cells (Paull et al., 2015). This high throughput platform can be used in conjunction with synthetic modified RNA to reprogram cells and avoid viral transduction (Warren et al., 2010). Finally, xenofree culture conditions have been developed and are now commercially available for deriving and propagating human pluripotent stem cells (Klim et al., 2010; Chen et al., 2011). Collectively, these innovations will help expedite the large-scale generation of clinical grade iPS cells.
Finally, widely applicable and efficient cell banking methods are needed to meet the demand of cell transplantation therapies. There are ongoing efforts in both Japan and the United States to screen and bank cells for allogeneic transplantations. Estimates from Cellular Dynamics International suggest that top 183 haplotypes could cover 95% of the US population. To gain maximum population coverage and provide social justice (Ellison, 2016), a universal stem cell donor could be part of the banking effort. This tactic proposes to use genetic engineering to reduce immunogenicity by removing the MHC molecules from the surface of the cells while also introducing well-established tolerance-inducing molecules (Riolobos et al., 2013; Han et al., 2019). Ultimately, stem cell banking will facilitate regenerative therapies by providing a common and less costly off-the-shelf cellular materials that can be thoroughly characterized before regular and repeated clinical use.
It’s an incredibly exciting time for stem cell-based regenerative medicine with a number of clinical trials started and more just on the horizon for neurodegenerative diseases, including one for PD (Kimbrel and Lanza, 2015). The International Society for Stem Cell Research (ISSCR) has established an updated set of guidelines (Daley et al., 2016) for the clinical translation of stem cell research to ensure safety and appropriate rigor while avoiding the real and present dangers of unregulated stem cell therapies (Berkowitz et al., 2016).
The demand for neurodegenerative disease therapeutics continues to grow as populations around the globe age. Currently, no pharmacological strategies exist that can significantly alter disease course for neurodegenerative diseases, thus cell replacement therapies remain an attractive avenue of exploration. Although the prospect of using stem cell-derived neurons to treat many of the diseases discussed above remains abstract, the PD clinical trials, grounded on years of fetal transplant studies and animal models with high fidelity, will provide important guideposts as others venture into these uncharted territories. In this review, we highlighted current methodologies for generating therapeutically relevant neuronal and glial cell types. Although directed differentiation strategies for some of these CNS cell types are in their nascent stage, they represent important first steps toward heralding in a new era of cellular therapeutics.
JK conceived of the review and drafted the figures. DM drafted the table. All authors drafted the original manuscript and read, edited, and approved the submitted version.
We would like to thank Katrina M. Wilbur and Renate G. E. Hellmiss from Harvard’s MCB Graphics for their graphic design skills in preparing the figures, and Andrew Castillo for assisting in researching glial-related topics. DM acknowledges support from the NINDS (K08NS104270).
JK is an employee of Faze Medicines and a shareholder of Faze Medicines as well as QurAlis, and is an author on patent that describes surfaces for the long-term culture of pluripotent cells (US patent 8648170). JK and FL are authors on a patent application that describes methods and compositions for restoring STMN2 levels (US patent application 20220133848). DM is an author on a pending patent that describes compounds and methods for treating neurodegenerative diseases (WO2020107037).
All claims expressed in this article are solely those of the authors and do not necessarily represent those of their affiliated organizations, or those of the publisher, the editors and the reviewers. Any product that may be evaluated in this article, or claim that may be made by its manufacturer, is not guaranteed or endorsed by the publisher.
Abud, E. M., Ramirez, R. N., Martinez, E. S., Healy, L. M., Nguyen, C. H. H., Newman, S. A., et al. (2017). iPSC-Derived human microglia-like cells to study neurological diseases. Neuron 94, 278–293. doi: 10.1016/j.neuron.2017.03.042
Amoroso, M. W., Croft, G. F., Williams, D. J., O’Keeffe, S., Carrasco, M. A., Davis, A. R., et al. (2013). Accelerated high-yield generation of limb-innervating motor neurons from human stem cells. J. Neurosci. 33, 574–586. doi: 10.1523/jneurosci.0906-12.2013
Anderson, N. C., Zandt, M. A. V., Shrestha, S., Lawrence, D. B., Gupta, J., Chen, C. Y., et al. (2018). Pluripotent stem cell-derived interneuron progenitors mature and restore memory deficits but do not suppress seizures in the epileptic mouse brain. Stem Cell Res. 33, 83–94. doi: 10.1016/j.scr.2018.10.007
Arenas, E., Denham, M., and Villaescusa, J. C. (2015). How to make a midbrain dopaminergic neuron. Development 142, 1918–1936. doi: 10.1242/dev.097394
Aubry, L., Bugi, A., Lefort, N., Rousseau, F., Peschanski, M., and Perrier, A. L. (2008). Striatal progenitors derived from human ES cells mature into DARPP32 neurons in vitro and in quinolinic acid-lesioned rats. Proc. Natl. Acad. Sci. U. S. A. 105, 16707–16712. doi: 10.1073/pnas.0808488105
Baloh, R. H., Johnson, J. P., Avalos, P., Allred, P., Svendsen, S., Gowing, G., et al. (2022). Transplantation of human neural progenitor cells secreting GDNF into the spinal cord of patients with ALS: a phase 1/2a trial. Nat. Med. 28, 1813–1822. doi: 10.1038/s41591-022-01956-3
Barbar, L., Jain, T., Zimmer, M., Kruglikov, I., Sadick, J. S., Wang, M., et al. (2020). CD49f Is a novel marker of functional and reactive human iPSC-derived astrocytes. Neuron 107, 436–453. doi: 10.1016/j.neuron.2020.05.014
Bardy, C., van den Hurk, M., Eames, T., Marchand, C., Hernandez, R. V., Kellogg, M., et al. (2015). Neuronal medium that supports basic synaptic functions and activity of human neurons in vitro. Proc. Natl. Acad. Sci. U.S.A. 112, E2725–E2734. doi: 10.1073/pnas.1504393112
Barker, R. A., Parmar, M., Studer, L., and Takahashi, J. (2017). Human trials of stem cell-derived dopamine neurons for Parkinson’s Disease: Dawn of a New Era. Cell Stem Cell 21, 569–573. doi: 10.1016/j.stem.2017.09.014
Bataveljić, D., Nikolić, L., Milosević, M., Todorović, N., and Andjus, P. R. (2012). Changes in the astrocytic aquaporin-4 and inwardly rectifying potassium channel expression in the brain of the amyotrophic lateral sclerosis SOD1 G93A rat model. Glia 60, 1991–2003. doi: 10.1002/glia.22414
Berkowitz, A. L., Miller, M. B., Mir, S. A., Cagney, D., Chavakula, V., Guleria, I., et al. (2016). Glioproliferative lesion of the spinal cord as a complication of “stem-cell tourism”. N. Engl. J. Med. 375, 196–198. doi: 10.1056/nejmc1600188
Bissonnette, C. J., Lyass, L., Bhattacharyya, B. J., Belmadani, A., Miller, R. J., and Kessler, J. A. (2011). The controlled generation of functional basal forebrain cholinergic neurons from human embryonic stem cells. Stem Cells 29, 802–811. doi: 10.1002/stem.626
Cai, J., Donaldson, A., Yang, M., German, M. S., Enikolopov, G., and Iacovitti, L. (2009). The role of mx1a in the differentiation of human embryonic stem cells into midbrain dopamine neurons in culture and after transplantation into a Parkinson’s Disease Model. Stem Cells 27, 220–229. doi: 10.1634/stemcells.2008-0734
Caiazzo, M., Dell’Anno, M. T., Dvoretskova, E., Lazarevic, D., Taverna, S., Leo, D., et al. (2011). Direct generation of functional dopaminergic neurons from mouse and human fibroblasts. Nature 476, 224–227. doi: 10.1038/nature10284
Canals, I., Ginisty, A., Quist, E., Timmerman, R., Fritze, J., Miskinyte, G., et al. (2018). Rapid and efficient induction of functional astrocytes from human pluripotent stem cells. Nat. Methods 15, 693–696. doi: 10.1038/s41592-018-0103-2
Cao, S.-Y., Hu, Y., Chen, C., Yuan, F., Xu, M., Li, Q., et al. (2017). Enhanced derivation of human pluripotent stem cell-derived cortical glutamatergic neurons by a small molecule. Sci. Rep. 7:3282. doi: 10.1038/s41598-017-03519-w
Capano, L. S., Sato, C., Ficulle, E., Yu, A., Horie, K., Kwon, J. S., et al. (2022). Recapitulation of endogenous 4R tau expression and formation of insoluble tau in directly reprogrammed human neurons. Cell Stem Cell 29, 918–932 e918. doi: 10.1016/j.stem.2022.04.018
Carlsson, T., Carta, M., Muñoz, A., Mattsson, B., Winkler, C., Kirik, D., et al. (2009). Impact of grafted serotonin and dopamine neurons on development of l-DOPA-induced dyskinesias in parkinsonian rats is determined by the extent of dopamine neuron degeneration. Brain 132, 319–335. doi: 10.1093/brain/awn305
Carri, A. D., Onorati, M., Lelos, M. J., Castiglioni, V., Faedo, A., Menon, R., et al. (2012). Developmentally coordinated extrinsic signals drive human pluripotent stem cell differentiation toward authentic DARPP-32+ medium-sized spiny neurons. Development 140, 301–312. doi: 10.1242/dev.084608
Chanda, S., Ang, C. E., Davila, J., Pak, C., Mall, M., Lee, Q. Y., et al. (2014). Generation of induced neuronal cells by the single reprogramming factor ASCL1. Stem Cell Rep. 3, 282–296. doi: 10.1016/j.stemcr.2014.05.020
Chen, G., Gulbranson, D. R., Hou, Z., Bolin, J. M., Ruotti, V., Probasco, M. D., et al. (2011). Chemically defined conditions for human iPSC derivation and culture. Nat. Methods 8, 424–429. doi: 10.1038/nmeth.1593
Chen, S.-W., Hung, Y.-S., Fuh, J.-L., Chen, N.-J., Chu, Y.-S., Chen, S.-C., et al. (2021). Efficient conversion of human induced pluripotent stem cells into microglia by defined transcription factors. Stem Cell Rep. 16, 1363–1380. doi: 10.1016/j.stemcr.2021.03.010
Clement, A. M., Nguyen, M. D., Roberts, E. A., Garcia, M. L., Boilleìe, S., Rule, M., et al. (2003). Wild-type nonneuronal cells extend survival of SOD1 mutant motor neurons in ALS Mice. Science 302, 113–117. doi: 10.1126/science.1086071
Corti, S., Nizzardo, M., Simone, C., Falcone, M., Nardini, M., Ronchi, D., et al. (2012). Genetic correction of human induced pluripotent stem cells from patients with spinal muscular atrophy. Sci. Transl. Med. 4:165ra162. doi: 10.1126/scitranslmed.3004108
Cunningham, M., Cho, J.-H., Leung, A., Savvidis, G., Ahn, S., Moon, M., et al. (2014). hPSC-Derived maturing GABAergic interneurons ameliorate seizures and abnormal behavior in epileptic mice. Cell Stem Cell 15, 559–573. doi: 10.1016/j.stem.2014.10.006
Daley, G. Q., Hyun, I., Apperley, J. F., Barker, R. A., Benvenisty, N., and Bredenoord, A. L. (2016). Setting global standards for stem cell research and clinical translation: The 2016 ISSCR Guidelines. Stem Cell Rep. 6, 787–797. doi: 10.1016/j.stemcr.2016.05.001
Dasen, J. S., and Jessell, T. M. (2009). Hox networks and the origins of motor neuron diversity. Curr. Topics Dev. Biol. 88, 169–200. doi: 10.1016/s0070-2153(09)88006-x
Davis-Dusenbery, B. N., Williams, L. A., Klim, J. R., and Eggan, K. (2014). How to make spinal motor neurons. Development 141, 491–501. doi: 10.1242/dev.097410
Del Dosso, A., Urenda, J. P., Nguyen, T., and Quadrato, G. (2020). Upgrading the physiological relevance of human brain organoids. Neuron 107, 1014–1028. doi: 10.1016/j.neuron.2020.08.029
Di Giorgio, F. P., Boulting, G. L., Bobrowicz, S., and Eggan, K. C. (2008). Human embryonic stem cell-derived motor neurons are sensitive to the toxic effect of glial cells carrying an ALS-causing mutation. Cell Stem Cell 3, 637–648. doi: 10.1016/j.stem.2008.09.017
Di Giorgio, F. P., Carrasco, M. A., Siao, M. C., Maniatis, T., and Eggan, K. (2007). Non–cell autonomous effect of glia on motor neurons in an embryonic stem cell–based ALS model. Nat. Neurosci. 10, 608–614. doi: 10.1038/nn1885
Dolan, M. J., Therrien, M., Jereb, S., Kamath, T., Atkenson, T., Marsh, S., et al. (2022). A resource for generating and manipulating human microglial states in vitro. bioRxiv [Preprint] doi: 10.1101/2022.05.02.490100
Douvaras, P., and Fossati, V. (2015). Generation and isolation of oligodendrocyte progenitor cells from human pluripotent stem cells. Nat. Protoc. 10, 1143–1154. doi: 10.1038/nprot.2015.075
Douvaras, P., Sun, B., Wang, M., Kruglikov, I., Lallos, G., Zimmer, M., et al. (2017). Directed Differentiation of human pluripotent stem cells to microglia. Stem Cell Rep. 8, 1516–1524. doi: 10.1016/j.stemcr.2017.04.023
Douvaras, P., Wang, J., Zimmer, M., Hanchuk, S., O’Bara, M. A., Sadiq, S., et al. (2014). Efficient generation of myelinating oligodendrocytes from primary progressive multiple sclerosis patients by induced pluripotent stem cells. Stem Cell Rep. 3, 250–259. doi: 10.1016/j.stemcr.2014.06.012
Drager, N. M., Sattler, S. M., Huang, C. T., Teter, O. M., Leng, K., Hashemi, S. H., et al. (2022). A CRISPRi/a platform in human iPSC-derived microglia uncovers regulators of disease states. Nat. Neurosci. 25, 1149–1162. doi: 10.1038/s41593-022-01131-4
Draper, J. S., Smith, K., Gokhale, P., Moore, H. D., Maltby, E., Johnson, J., et al. (2004). Recurrent gain of chromosomes 17q and 12 in cultured human embryonic stem cells. Nat. Biotechnol. 22, 53—-54. doi: 10.1038/nbt922
Du, Z.-W., Chen, H., Liu, H., Lu, J., Qian, K., Huang, C.-L., et al. (2015). Generation and expansion of highly pure motor neuron progenitors from human pluripotent stem cells. Nat. Commun. 6:6626. doi: 10.1038/ncomms7626
Ehrlich, M., Mozafari, S., Glatza, M., Starost, L., Velychko, S., Hallmann, A.-L., et al. (2017). Rapid and efficient generation of oligodendrocytes from human induced pluripotent stem cells using transcription factors. Proc. Natl. Acad. Sci. U. S. A. 114, E2243–E2252. doi: 10.1073/pnas.1614412114
Ellison, B. (2016). Stem Cell Research and Social Justice: Aligning Scientific Progress with Social Need. Current Stem Cell Rep. 2, 328–335. doi: 10.1007/s40778-016-0063-3
Espuny-Camacho, I., Arranz, A. M., Fiers, M., Snellinx, A., Ando, K., Munck, S., et al. (2017). Hallmarks of Alzheimer’s Disease in stem-cell-derived human neurons transplanted into mouse brain. Neuron 93, 1066–1081.e1068. doi: 10.1016/j.neuron.2017.02.001
Espuny-Camacho, I., Michelsen, K. A., Gall, D., Linaro, D., Hasche, A., Bonnefont, J., et al. (2013). Pyramidal neurons derived from human pluripotent stem cells integrate efficiently into mouse brain circuits in vivo. Neuron 77, 440–456. doi: 10.1016/j.neuron.2012.12.011
Fjodorova, M., Noakes, Z., and Li, M. (2015). How to make striatal projection neurons. Neurogenesis 2:e1100227. doi: 10.1080/23262133.2015.1100227
Freeman, T. B., Cicchetti, F., Hauser, R. A., Deacon, T. W., Li, X.-J., Hersch, S. M., et al. (2000). Transplanted fetal striatum in Huntington’s disease: Phenotypic development and lack of pathology. Proc. Natl. Acad. Sci. U. S. A. 97, 13877–13882. doi: 10.1073/pnas.97.25.13877
Gage, F. H., and Temple, S. (2013). Neural stem cells: Generating and regenerating the brain. Neuron 80, 588–601. doi: 10.1016/j.neuron.2013.10.037
García-León, J. A., Kumar, M., Boon, R., Chau, D., One, J., Wolfs, E., et al. (2018). SOX10 Single transcription factor-based fast and efficient generation of oligodendrocytes from human pluripotent stem cells. Stem Cell Rep. 10, 655–672. doi: 10.1016/j.stemcr.2017.12.014
Giacomelli, E., Vahsen, B. F., Calder, E. L., Xu, Y., Scaber, J., Gray, E., et al. (2022). Human stem cell models of neurodegeneration: From basic science of amyotrophic lateral sclerosis to clinical translation. Cell Stem Cell 29, 11–35. doi: 10.1016/j.stem.2021.12.008
Ginhoux, F., Greter, M., Leboeuf, M., Nandi, S., See, P., Gokhan, S., et al. (2010). Fate mapping analysis reveals that adult microglia derive from primitive macrophages. Science 330, 841–845. doi: 10.1126/science.1194637
Goto, K., Imamura, K., Komatsu, K., Mitani, K., Aiba, K., Nakatsuji, N., et al. (2017). Simple derivation of spinal motor neurons from escs/ipscs using sendai virus vectors. Mol. Ther. Methods Clin. Dev. 4, 115–125. doi: 10.1016/j.omtm.2016.12.007
Grealish, S., Diguet, E., Kirkeby, A., Mattsson, B., Heuer, A., Bramoulle, Y., et al. (2014). Human ESC-derived dopamine neurons show similar preclinical efficacy and potency to fetal neurons when grafted in a rat model of Parkinson’s Disease. Cell Stem Cell 15, 653–665. doi: 10.1016/j.stem.2014.09.017
Haenseler, W., Sansom, S. N., Buchrieser, J., Newey, S. E., Moore, C. S., Nicholls, F. J., et al. (2017). A Highly efficient human pluripotent stem cell microglia model displays a neuronal-co-culture-specific expression profile and inflammatory response. Stem Cell Rep. 8, 1727–1742. doi: 10.1016/j.stemcr.2017.05.017
Hall, C. E., Yao, Z., Choi, M., Tyzack, G. E., Serio, A., Luisier, R., et al. (2017). Progressive motor neuron pathology and the role of astrocytes in a human stem cell model of VCP-Related ALS. Cell Rep. 19, 1739–1749. doi: 10.1016/j.celrep.2017.05.024
Hallett, P. J., Cooper, O., Sadi, D., Robertson, H., Mendez, I., and Isacson, O. (2014). Long-Term health of dopaminergic neuron transplants in Parkinson’s Disease Patients. Cell Rep. 7, 1755–1761. doi: 10.1016/j.celrep.2014.05.027
Han, X., Wang, M., Duan, S., Franco, P. J., Kenty, J. H.-R., Hedrick, P., et al. (2019). Generation of hypoimmunogenic human pluripotent stem cells. Proc. Natl. Acad. Sci. U. S. A. 116, 10441–10446. doi: 10.1073/pnas.1902566116
Hester, M. E., Murtha, M. J., Song, S., Rao, M., Miranda, C. J., Meyer, K., et al. (2011). Rapid and efficient generation of functional motor neurons from human pluripotent stem cells using gene delivered transcription factor codes. Mol. Ther. 19, 1905–1912. doi: 10.1038/mt.2011.135
Higuera, G. A., Iaffaldano, G., Bedar, M., Shpak, G., Broersen, R., Munshi, S. T., et al. (2017). An expandable embryonic stem cell-derived Purkinje neuron progenitor population that exhibits in vivo maturation in the adult mouse cerebellum. Sci. Rep. 7:8863. doi: 10.1038/s41598-017-09348-1
Hiragi, T., Andoh, M., Araki, T., Shirakawa, T., Ono, T., Koyama, R., et al. (2017). Differentiation of human induced pluripotent stem cell (hiPSC)-derived neurons in mouse hippocampal slice cultures. Front. Cell. Neurosci. 11:143. doi: 10.3389/fncel.2017.00143
Holm, F., Ström, S., Inzunza, J., Baker, D., Strömberg, A.-M., Rozell, B., et al. (2010). An effective serum- and xeno-free chemically defined freezing procedure for human embryonic and induced pluripotent stem cells. Hum. Reprod. 25, 1271–1279. doi: 10.1093/humrep/deq040
Hu, Y., Qu, Z.-Y., Cao, S.-Y., Li, Q., Ma, L., Krencik, R., et al. (2016). Directed differentiation of basal forebrain cholinergic neurons from human pluripotent stem cells. J. Neurosci. Methods 266, 42–49. doi: 10.1016/j.jneumeth.2016.03.017
Ishida, Y., Kawakami, H., Kitajima, H., Nishiyama, A., Sasai, Y., Inoue, H., et al. (2016). Vulnerability of purkinje cells generated from spinocerebellar ataxia type 6 patient-derived iPSCs. Cell Rep. 17, 1482–1490. doi: 10.1016/j.celrep.2016.10.026
Kierdorf, K., Erny, D., Goldmann, T., Sander, V., Schulz, C., Perdiguero, E. G., et al. (2013). Microglia emerge from erythromyeloid precursors via Pu.1- and Irf8-dependent pathways. Nat. Neurosci. 16, 273–280. doi: 10.1038/nn.3318
Kikuchi, T., Morizane, A., Doi, D., Magotani, H., Onoe, H., Hayashi, T., et al. (2017). Human iPS cell-derived dopaminergic neurons function in a primate Parkinson’s disease model. Nature 548, 592–596. doi: 10.1038/nature23664
Kim, T. W., Koo, S. Y., and Studer, L. (2020). Pluripotent stem cell therapies for Parkinson Disease: Present challenges and future opportunities. Front. Cell Dev. Biol. 8:729. doi: 10.3389/fcell.2020.00729
Kim, T. W., Piao, J., Koo, S. Y., Kriks, S., Chung, S. Y., Betel, D., et al. (2021). Biphasic Activation of WNT Signaling Facilitates the Derivation of Midbrain Dopamine Neurons from hESCs for Translational Use. Cell Stem Cell 28, 343–355. doi: 10.1016/j.stem.2021.01.005
Kimbrel, E. A., and Lanza, R. (2015). Current status of pluripotent stem cells: moving the first therapies to the clinic. Nat. Rev. Drug Discov. 14, 681–692. doi: 10.1038/nrd4738
Kirwan, P., Jura, M., and Merkle, F. T. (2017). Generation and Characterization of Functional Human Hypothalamic Neurons. Curr. Protoc. Neurosci. 81, 33331–333324. doi: 10.1002/cpns.40
Klim, J. R., Li, L., Wrighton, P. J., Piekarczyk, M. S., and Kiessling, L. L. (2010). A defined glycosaminoglycan-binding substratum for human pluripotent stem cells. Nat. Methods 7, 989–994. doi: 10.1038/nmeth.1532
Klim, J. R., Pintacuda, G., Nash, L. A., Guerra San Juan, I., and Eggan, K. (2021). Connecting TDP-43 Pathology with Neuropathy. Trends Neurosci. 44, 424–440. doi: 10.1016/j.tins.2021.02.008
Klim, J. R., Williams, L. A., Limone, F., Juan, I. G. S., Davis-Dusenbery, B. N., and Mordes, D. A. (2019). ALS-implicated protein TDP-43 sustains levels of STMN2, a mediator of motor neuron growth and repair. Nat. Neurosci. 22, 167–179. doi: 10.1038/s41593-018-0300-4
Krencik, R., and Zhang, S.-C. (2011). Directed differentiation of functional astroglial subtypes from human pluripotent stem cells. Nat. Protoc. 6, 1710–1717. doi: 10.1038/nprot.2011.405
Krencik, R., Weick, J. P., Liu, Y., Zhang, Z.-J., and Zhang, S.-C. (2011). Specification of transplantable astroglial subtypes from human pluripotent stem cells. Nat. Biotechnol. 29, 528–534. doi: 10.1038/nbt.1877
Kriks, S., Shim, J.-W., Piao, J., Ganat, Y. M., Wakeman, D. R., Xie, Z., et al. (2011). Dopamine neurons derived from human ES cells efficiently engraft in animal models of Parkinson’s disease. Nature 480, 547–551. doi: 10.1038/nature10648
Lepore, A. C., Rauck, B., Dejea, C., Pardo, A. C., Rao, M. S., Rothstein, J. D., et al. (2008). Focal transplantation–based astrocyte replacement is neuroprotective in a model of motor neuron disease. Nat. Neurosci. 11, 1294–1301. doi: 10.1038/nn.2210
Li, X., Tao, Y., Bradley, R., Du, Z., Tao, Y., Kong, L., et al. (2018). Fast generation of functional subtype astrocytes from human pluripotent stem cells. Stem Cell Rep. 11, 998–1008. doi: 10.1016/j.stemcr.2018.08.019
Limone, F., Mitchell, J. M., Juan, I. G. S., Smith, J. L. M., Raghunathan, K., Couto, A., et al. (2022). Efficient generation of lower induced Motor Neurons by coupling Ngn2 expression with developmental cues. bioRxiv [Preprint] doi: 10.1101/2022.01.12.476020
Limone, F., Mordes, D. A., Couto, A., Pietilainen, O., Joseph, B. J., Burberry, A., et al. (2021). Single-nucleus sequencing reveals enriched expression of genetic risk factors sensitises Motor Neurons to degeneration in ALS. bioRxiv [Preprint] doi: 10.1101/2021.07.12.452054
Lippmann, E. S., Williams, C. E., Ruhl, D. A., Estevez-Silva, M. C., Chapman, E. R., Coon, J. J., et al. (2015). Deterministic HOX patterning in human pluripotent stem cell-derived neuroectoderm. Stem Cell Rep. 4, 632–644. doi: 10.1016/j.stemcr.2015.02.018
Liu, Y., Liu, H., Sauvey, C., Yao, L., Zarnowska, E. D., and Zhang, S.-C. (2013b). Directed differentiation of forebrain GABA interneurons from human pluripotent stem cells. Nat. Protoc. 8, 1670–1679. doi: 10.1038/nprot.2013.106
Liu, Y., Weick, J. P., Liu, H., Krencik, R., Zhang, X., Ma, L., et al. (2013a). Medial ganglionic eminence–like cells derived from human embryonic stem cells correct learning and memory deficits. Nat. Biotechnol. 31, 440–447. doi: 10.1038/nbt.2565
Lu, J., Zhong, X., Liu, H., Hao, L., Huang, C. T.-L., Sherafat, M. A., et al. (2016). Generation of serotonin neurons from human pluripotent stem cells. Nature Biotechnol. 34, 89–94. doi: 10.1038/nbt.3435
Ma, L., Hu, B., Liu, Y., Vermilyea, S. C., Liu, H., Gao, L., et al. (2012). Human embryonic stem cell-derived GABA neurons correct locomotion deficits in quinolinic acid-lesioned mice. Cell Stem Cell 10, 455–464. doi: 10.1016/j.stem.2012.01.021
Mandai, M., Watanabe, A., Kurimoto, Y., Hirami, Y., Morinaga, C., Daimon, T., et al. (2017). Autologous induced stem-cell–derived retinal cells for macular degeneration. N. Engl. J. Med. 376, 1038—-1046. doi: 10.1056/nejmoa1608368
Maroof, A. M., Keros, S., Tyson, J. A., Ying, S.-W., Ganat, Y. M., Merkle, F. T., et al. (2013). Directed differentiation and functional maturation of cortical interneurons from human embryonic stem cells. Cell Stem Cell 12, 559–572. doi: 10.1016/j.stem.2013.04.008
Martinez, J. L., Zammit, M. D., West, N. R., Christian, B. T., and Bhattacharyya, A. (2021). Corrigendum: basal forebrain cholinergic neurons: Linking down syndrome and Alzheimer’s Disease. Front. Aging Neurosci. 13:742233. doi: 10.3389/fnagi.2021.742233
Marton, R. M., Miura, Y., Sloan, S. A., Li, Q., Revah, O., Levy, R. J., et al. (2019). Differentiation and maturation of oligodendrocytes in human three-dimensional neural cultures. Nat. Neurosci. 22, 484–491. doi: 10.1038/s41593-018-0316-9
Maury, Y., Côme, J., Piskorowski, R. A., Salah-Mohellibi, N., Chevaleyre, V., Peschanski, M., et al. (2015). Combinatorial analysis of developmental cues efficiently converts human pluripotent stem cells into multiple neuronal subtypes. Nat. Biotechnol. 33, 89–96. doi: 10.1038/nbt.3049
Merkle, F. T., Ghosh, S., Genovese, G., Handsaker, R. E., Kashin, S., Meyer, D., et al. (2022). Whole-genome analysis of human embryonic stem cells enables rational line selection based on genetic variation. Cell Stem Cell 29, 472–486. doi: 10.1016/j.stem.2022.01.011
Merkle, F. T., Ghosh, S., Kamitaki, N., Mitchell, J., Avior, Y., Mello, C., et al. (2017). Human pluripotent stem cells recurrently acquire and expand dominant negative P53 mutations. Nature 545, 229–233. doi: 10.1038/nature22312
Merkle, F. T., Maroof, A., Wataya, T., Sasai, Y., Studer, L., Eggan, K., et al. (2015). Generation of neuropeptidergic hypothalamic neurons from human pluripotent stem cells. Development 142, 633–643. doi: 10.1242/dev.117978
Mertens, J., Reid, D., Lau, S., Kim, Y., and Gage, F. H. (2018). Aging in a Dish: iPSC-Derived and directly induced neurons for studying brain aging and age-related neurodegenerative Diseases. Annu. Rev. Genet. 52, 271–293. doi: 10.1146/annurev-genet-120417-031534
Meyer, K., Ferraiuolo, L., Miranda, C. J., Likhite, S., McElroy, S., Renusch, S., et al. (2014). Direct conversion of patient fibroblasts demonstrates non-cell autonomous toxicity of astrocytes to motor neurons in familial and sporadic ALS. Proc. Natl. Acad. Sci. U. S. A. 111, 829–832. doi: 10.1073/pnas.1314085111
Muffat, J., Li, Y., Yuan, B., Mitalipova, M., Omer, A., Corcoran, S., et al. (2016). Efficient derivation of microglia-like cells from human pluripotent stem cells. Nat. Med. 22, 1358–1367. doi: 10.1038/nm.4189
Muguruma, K., Nishiyama, A., Kawakami, H., Hashimoto, K., and Sasai, Y. (2015). Self-Organization of polarized cerebellar tissue in 3d culture of human pluripotent stem cells. Cell Rep. 10, 537–550. doi: 10.1016/j.celrep.2014.12.051
Nehme, R., Zuccaro, E., Ghosh, S. D., Li, C., Sherwood, J. L., Pietilainen, O., et al. (2018). Combining NGN2 programming with developmental patterning generates human excitatory neurons with NMDAR-mediated synaptic transmission. Cell Rep. 23, 2509–2523. doi: 10.1016/j.celrep.2018.04.066
Nicholas, C. R., Chen, J., Tang, Y., Southwell, D. G., Chalmers, N., Vogt, D., et al. (2013). Functional maturation of hpsc-derived forebrain interneurons requires an extended timeline and mimics human neural development. Cell Stem Cell 12, 573–586. doi: 10.1016/j.stem.2013.04.005
Nishiyama, Y., Iwanami, A., Kohyama, J., Itakura, G., Kawabata, S., Sugai, K., et al. (2016). Safe and efficient method for cryopreservation of human induced pluripotent stem cell-derived neural stem and progenitor cells by a programmed freezer with a magnetic field. Neurosci. Res. 107, 20–29. doi: 10.1016/j.neures.2015.11.011
Pandya, H., Shen, M. J., Ichikawa, D. M., Sedlock, A. B., Choi, Y., Johnson, K. R., et al. (2017). Differentiation of human and murine induced pluripotent stem cells to microglia-like cells. Nat. Neurosci. 20, 753–759. doi: 10.1038/nn.4534
Paull, D., Sevilla, A., Zhou, H., Hahn, A. K., Kim, H., Napolitano, C., et al. (2015). Automated, high-throughput derivation, characterization and differentiation of induced pluripotent stem cells. Nat. Methods 12, 885–892. doi: 10.1038/nmeth.3507
Pera, M. F. (2011). Stem cells: The dark side of induced pluripotency. Nature 471, 46–47. doi: 10.1038/471046a
Pfisterer, U., Kirkeby, A., Torper, O., Wood, J., Nelander, J., Dufour, A., et al. (2011). Direct conversion of human fibroblasts to dopaminergic neurons. Proc. Natl. Acad. Sci. U. S. A. 108, 10343–10348. doi: 10.1073/pnas.1105135108
Politis, M., and Loane, C. (2011). Serotonergic dysfunction in Parkinson’s Disease and its relevance to disability. Sci. World J. 11, 1726–1734. doi: 10.1100/2011/172893
Qi, Y., Zhang, X.-J., Renier, N., Wu, Z., Atkin, T., Sun, Z., et al. (2017). Combined small-molecule inhibition accelerates the derivation of functional cortical neurons from human pluripotent stem cells. Nat. Biotechnol. 35, 154–163. doi: 10.1038/nbt.3777
Qian, H., Kang, X., Hu, J., Zhang, D., Liang, Z., Meng, F., et al. (2020). Reversing a model of Parkinson’s disease with in situ converted nigral neurons. Nature 582, 550–556. doi: 10.1038/s41586-020-2388-4
Rajamani, U., Gross, A. R., Hjelm, B. E., Sequeira, A., Vawter, M. P., Tang, J., et al. (2018). Super-Obese patient-derived iPSC hypothalamic neurons exhibit obesogenic signatures and hormone responses. Cell Stem Cell 22, 698–712. doi: 10.1016/j.stem.2018.03.009
Ridley, R. M., Baker, J. A., Baker, H. F., and MacLean, C. J. (1994). Restoration of cognitive abilities by cholinergic grafts in cortex of monkeys with lesions of the basal nucleus of meynert. Neuroscience 63, 653–666. doi: 10.1016/0306-4522(94)90512-6
Riolobos, L., Hirata, R. K., Turtle, C. J., Wang, P.-R., Gornalusse, G. G., Zavajlevski, M., et al. (2013). HLA Engineering of Human Pluripotent Stem Cells. Molecular Therapy 21, 1232–1241. doi: 10.1038/mt.2013.59
Sakaguchi, H., Kadoshima, T., Soen, M., Narii, N., Ishida, Y., Ohgushi, M., et al. (2015). Generation of functional hippocampal neurons from self-organizing human embryonic stem cell-derived dorsomedial telencephalic tissue. Nat. Commun. 6:8896. doi: 10.1038/ncomms9896
Shaltouki, A., Peng, J., Liu, Q., Rao, M. S., and Zeng, X. (2013). Efficient generation of astrocytes from human pluripotent stem cells in defined conditions. Stem Cells 31, 941–952. doi: 10.1002/stem.1334
Silva, T. P., Bekman, E. P., Fernandes, T. G., Vaz, S. H., Rodrigues, C. A. V., Diogo, M. M., et al. (2020). Maturation of human pluripotent stem cell-derived cerebellar neurons in the absence of co-culture. Front. Bioeng. Biotechnol. 8:70. doi: 10.3389/fbioe.2020.00070
Son, E. Y., Ichida, J. K., Wainger, B. J., Toma, J. S., Rafuse, V. F., Woolf, C. J., et al. (2011). Conversion of mouse and human fibroblasts into functional spinal motor neurons. Cell Stem Cell 9, 205–218. doi: 10.1016/j.stem.2011.07.014
Song, J.-J., Oh, S.-M., Kwon, O.-C., Wulnansari, N., Lee, H.-S., Chang, M.-Y., et al. (2017). Cografting astrocytes improves cell therapeutic outcomes in a Parkinson’s disease model. J. Clin. Invest. 128, 463–482. doi: 10.1172/jci93924
Sonn, I., Honda-Ozaki, F., Yoshimatsu, S., Morimoto, S., Watanabe, H., and Okano, H. (2022). Single transcription factor efficiently leads human induced pluripotent stem cells to functional microglia. Inflamm. Regen. 42:20. doi: 10.1186/s41232-022-00201-1
Southwell, D. G., Nicholas, C. R., Basbaum, A. I., Stryker, M. P., Kriegstein, A. R., Rubenstein, J. L., et al. (2014). Interneurons from Embryonic Development to Cell-Based Therapy. Science 344:1240622. doi: 10.1126/science.1240622
Steinbeck, J. A., and Studer, L. (2015). Moving stem cells to the clinic: potential and limitations for brain repair. Neuron 86, 187–206. doi: 10.1016/j.neuron.2015.03.002
Sun, A. X., Yuan, Q., Tan, S., Xiao, Y., Wang, D., Khoo, A. T., et al. (2016). Direct Induction and functional maturation of forebrain GABAergic neurons from human pluripotent stem cells. Cell Rep. 16, 1942–1953. doi: 10.1016/j.celrep.2016.07.035
Svoboda, D. S., Barrasa, M. I., Shu, J., Rietjens, R., Zhang, S., Mitalipova, M., et al. (2019). Human iPSC-derived microglia assume a primary microglia-like state after transplantation into the neonatal mouse brain. Proc. Natl. Acad. Sci. U. S. A. 116, 25293–25303. doi: 10.1073/pnas.1913541116
Takata, K., Kozaki, T., Lee, C. Z. W., Thion, M. S., Otsuka, M., Lim, S., et al. (2017). Induced-Pluripotent-stem-cell-derived primitive macrophages provide a platform for modeling tissue-resident macrophage differentiation and function. Immunity 47, 183–198. doi: 10.1016/j.immuni.2017.06.017
Tao, Y., and Zhang, S.-C. (2016). Neural subtype specification from human pluripotent stem cells. Cell Stem Cell 19, 573–586. doi: 10.1016/j.stem.2016.10.015
Tchieu, J., Calder, E. L., Guttikonda, S. R., Gutzwiller, E. M., Aromolaran, K. A., Steinbeck, J. A., et al. (2019). NFIA is a gliogenic switch enabling rapid derivation of functional human astrocytes from pluripotent stem cells. Nat. Biotechnol. 37, 267–275. doi: 10.1038/s41587-019-0035-0
Tcw, J., Wang, M., Pimenova, A. A., Bowles, K. R., Hartley, B. J., Lacin, E., et al. (2017). An efficient platform for astrocyte differentiation from human induced pluripotent stem cells. Stem Cell Rep. 9, 600–614. doi: 10.1016/j.stemcr.2017.06.018
Thiruvalluvan, A., Czepiel, M., Kap, Y. A., Mantingh-Otter, I., Vainchtein, I., Kuipers, J., et al. (2016). Survival and functionality of human induced pluripotent stem cell-derived oligodendrocytes in a nonhuman primate model for multiple sclerosis. Stem Cells Transl. Med. 5, 1550–1561. doi: 10.5966/sctm.2016-0024
Trounson, A., and DeWitt, N. D. (2016). Pluripotent stem cells progressing to the clinic. Nat. Rev. Mol. Cell Biol. 17, 194–200. doi: 10.1038/nrm.2016.10
Vadodaria, K. C., Mertens, J., Paquola, A., Bardy, C., Li, X., Jappelli, R., et al. (2016). Generation of functional human serotonergic neurons from fibroblasts. Mol. Psychiatry 21, 49–61. doi: 10.1038/mp.2015.161
Victor, M. B., Richner, M., Hermanstyne, T. O., Ransdell, J. L., Sobieski, C., Deng, P.-Y., et al. (2014). Generation of human striatal neurons by microrna-dependent direct conversion of fibroblasts. Neuron 84, 311–323. doi: 10.1016/j.neuron.2014.10.016
Wakeman, D. R., Hiller, B. M., Marmion, D. J., McMahon, C. W., Corbett, G. T., Mangan, K. P., et al. (2017). Cryopreservation Maintains Functionality of Human iPSC Dopamine Neurons and Rescues Parkinsonian Phenotypes In Vivo. Stem Cell Rep. 9, 149–161. doi: 10.1016/j.stemcr.2017.04.033
Wang, L., Meece, K., Williams, D. J., Lo, K. A., Zimmer, M., Heinrich, G., et al. (2015b). Differentiation of hypothalamic-like neurons from human pluripotent stem cells. J. Clin. Invest. 125, 796–808. doi: 10.1172/JCI79220
Wang, S., Bates, J., Li, X., Schanz, S., Chandler-Militello, D., Levine, C., et al. (2013). Human iPSC-derived oligodendrocyte progenitor cells can myelinate and rescue a mouse model of congenital hypomyelination. Cell Stem Cell 12, 252–264. doi: 10.1016/j.stem.2012.12.002
Wang, S., Wang, B., Pan, N., Fu, L., Wang, C., Song, G., et al. (2015a). Differentiation of human induced pluripotent stem cells to mature functional Purkinje neurons. Sci. Rep. 5:9232. doi: 10.1038/srep09232
Warren, L., Manos, P. D., Ahfeldt, T., Loh, Y.-H., Li, H., Lau, F., et al. (2010). Highly efficient reprogramming to pluripotency and directed differentiation of human cells with synthetic modified mRNA. Cell Stem Cell 7, 618–630. doi: 10.1016/j.stem.2010.08.012
Watson, L. M., Wong, M. M. K., Vowles, J., Cowley, S. A., and Becker, E. B. E. (2018). A Simplified method for generating purkinje cells from human-induced pluripotent stem cells. Cerebellum 17, 419–427. doi: 10.1007/s12311-017-0913-2
Wichterle, H., Lieberam, I., Porter, J. A., and Jessell, T. M. (2002). Directed differentiation of embryonic stem cells into motor neurons. Cell 110, 385–397. doi: 10.1016/s0092-8674(02)00835-8
Windrem, M. S., Schanz, S. J., Zou, L., Chandler-Militello, D., Kuypers, N. J., Nedergaard, M., et al. (2020). Human glial progenitor cells effectively remyelinate the demyelinated adult brain. Cell Rep. 31:107658. doi: 10.1016/j.celrep.2020.107658
Xu, R., Li, X., Boreland, A. J., Posyton, A., Kwan, K., Hart, R. P., et al. (2020). Human iPSC-derived mature microglia retain their identity and functionally integrate in the chimeric mouse brain. Nat. Commun. 11:1577. doi: 10.1038/s41467-020-15411-9
Xu, Z., Jiang, H., Zhong, P., Yan, Z., Chen, S., and Feng, J. (2016). Direct conversion of human fibroblasts to induced serotonergic neurons. Mol. Psychiatry 21, 62–70. doi: 10.1038/mp.2015.101
Yohn, D. C., Miles, G. B., Rafuse, V. F., and Brownstone, R. M. (2008). Transplanted mouse embryonic stem-cell-derived motoneurons form functional motor units and reduce muscle atrophy. J. Neurosci. 28, 12409–12418. doi: 10.1523/jneurosci.1761-08.2008
Yu, D. X., Di Giorgio, F. P., Yao, J., Marchetto, M. C., Brennand, K., Wright, R., et al. (2014). Modeling hippocampal neurogenesis using human pluripotent stem cells. Stem Cell Rep. 2, 295–310. doi: 10.1016/j.stemcr.2014.01.009
Yuan, F., Chen, X., Fang, K.-H., Wang, Y., Lin, M., Xu, S.-B., et al. (2018). Induction of human somatostatin and parvalbumin neurons by expressing a single transcription factor LIM homeobox 6. eLife 7:e37382. doi: 10.7554/elife.37382
Yuan, S. H., Martin, J., Elia, J., Flippin, J., Paramban, R. I., Hefferan, M. P., et al. (2011). Cell-Surface Marker Signatures for the Isolation of Neural Stem Cells, Glia and Neurons Derived from Human Pluripotent Stem Cells. PLoS One 6:e17540. doi: 10.1371/journal.pone.0017540
Yue, C., and Jing, N. (2015). The promise of stem cells in the therapy of Alzheimer’s disease. Transl. Neurodegener. 4:8. doi: 10.1186/s40035-015-0029-x
Yun, S.-W., Leong, C., Zhai, D., Tan, Y. L., Lim, L., Bi, X., et al. (2012). Neural stem cell specific fluorescent chemical probe binding to FABP7. Proc. Natl Acad. Sci. U. S. A. 109, 10214–10217. doi: 10.1073/pnas.1200817109
Zhang, S.-C., Wernig, M., Duncan, I. D., Brüstle, O., and Thomson, J. A. (2001). In vitro differentiation of transplantable neural precursors from human embryonic stem cells. Nat. Biotechnol. 19, 1129–1133. doi: 10.1038/nbt1201-1129
Zhang, Y., Pak, C., Han, Y., Ahlenius, H., Zhang, Z., Chanda, S., et al. (2013). Rapid single-step induction of functional neurons from human pluripotent stem cells. Neuron 78, 785–798. doi: 10.1016/j.neuron.2013.05.029
Keywords: neurodegeneration, aging, cell replacement therapies, regenerative medicine, pluripotent stem cells, developmental neuroscience, brain regeneration, neurological diseases
Citation: Limone F, Klim JR and Mordes DA (2022) Pluripotent stem cell strategies for rebuilding the human brain. Front. Aging Neurosci. 14:1017299. doi: 10.3389/fnagi.2022.1017299
Received: 11 August 2022; Accepted: 27 September 2022;
Published: 02 November 2022.
Edited by:
Shong Lau, Salk Institute for Biological Studies, United StatesReviewed by:
Adegbenro Omotuyi John Fakoya, All Saints University School of Medicine, DominicaCopyright © 2022 Limone, Klim and Mordes. This is an open-access article distributed under the terms of the Creative Commons Attribution License (CC BY). The use, distribution or reproduction in other forums is permitted, provided the original author(s) and the copyright owner(s) are credited and that the original publication in this journal is cited, in accordance with accepted academic practice. No use, distribution or reproduction is permitted which does not comply with these terms.
*Correspondence: Joseph R. Klim, amtsaW1AZmF6ZW1lZC5jb20=; Daniel A. Mordes, ZGFuaWVsLm1vcmRlc0B1Y3NmLmVkdQ==
Disclaimer: All claims expressed in this article are solely those of the authors and do not necessarily represent those of their affiliated organizations, or those of the publisher, the editors and the reviewers. Any product that may be evaluated in this article or claim that may be made by its manufacturer is not guaranteed or endorsed by the publisher.
Research integrity at Frontiers
Learn more about the work of our research integrity team to safeguard the quality of each article we publish.