- 1Department of Physiology, University of Toronto, Toronto, ON, Canada
- 2Department of Anesthesiology and Pain Medicine, Chungnam National University, Daejeon, South Korea
- 3Department of Anesthesiology and Pain Medicine, University of Toronto, Toronto, ON, Canada
- 4Department of Anesthesia, Sunnybrook Health Sciences Centre, Toronto, ON, Canada
Background: Perioperative neurocognitive disorders (PNDs) occur commonly in older patients after anesthesia and surgery. Treating astrocytes with general anesthetic drugs stimulates the release of soluble factors that increase the cell-surface expression and function of GABAA receptors in neurons. Such crosstalk may contribute to PNDs; however, the receptor targets in astrocytes for anesthetic drugs have not been identified. GABAA receptors, which are the major targets of general anesthetic drugs in neurons, are also expressed in astrocytes, raising the possibility that these drugs act on GABAA receptors in astrocytes to trigger the release of soluble factors. To date, no study has directly examined the sensitivity of GABAA receptors in astrocytes to general anesthetic drugs that are frequently used in clinical practice. Thus, the goal of this study was to determine whether the function of GABAA receptors in astrocytes was modulated by the intravenous anesthetic etomidate and the inhaled anesthetic sevoflurane.
Methods: Whole-cell voltage-clamp recordings were performed in astrocytes in the stratum radiatum of the CA1 region of hippocampal slices isolated from C57BL/6 male mice. Astrocytes were identified by their morphologic and electrophysiologic properties. Focal puff application of GABA (300 μM) was applied with a Picospritzer system to evoke GABA responses. Currents were studied before and during the application of the non-competitive GABAA receptor antagonist picrotoxin (0.5 mM), or etomidate (100 μM) or sevoflurane (532 μM).
Results: GABA consistently evoked inward currents that were inhibited by picrotoxin. Etomidate increased the amplitude of the peak current by 35.0 ± 24.4% and prolonged the decay time by 27.2 ± 24.3% (n = 7, P < 0.05). Sevoflurane prolonged current decay by 28.3 ± 23.1% (n = 7, P < 0.05) but did not alter the peak amplitude. Etomidate and sevoflurane increased charge transfer (area) by 71.2 ± 45.9% and 51.8 ± 48.9% (n = 7, P < 0.05), respectively.
Conclusion: The function of astrocytic GABAA receptors in the hippocampus was increased by etomidate and sevoflurane. Future studies will determine whether these general anesthetic drugs act on astrocytic GABAA receptors to stimulate the release of soluble factors that may contribute to PNDs.
Introduction
Astrocytes, which are among the most abundant cells in the mammalian brain, play an essential role in numerous functions, from the maintenance of molecular, cellular, and metabolic homeostasis to the regulation of cognition and behavior (Verkhratsky and Nedergaard, 2018; Santello et al., 2019). Not surprisingly, astrocytes are implicated in a variety of neurological disorders, including neurodegenerative diseases, ischemic stroke, epilepsy, and depression (Liu and Chopp, 2016; Wang et al., 2017; Santello et al., 2019; Siracusa et al., 2019; De Majo et al., 2020; Zhang et al., 2021).
One group of cognitive conditions that is of particular interest to the fields of anesthesia and critical care is perioperative neurocognitive disorders (PNDs) (Evered et al., 2018). PNDs occur most commonly in older patients after anesthesia and surgery. These patients may experience a range of symptoms, including delirium, confusion, inattention, and cognitive deficits, that can persist for days to months (Evered et al., 2018). The incidence of PNDs is remarkably high, ranging from 10 to 60%; and these disorders are associated with poor long-term outcomes, increased healthcare costs, loss of independence, and increased mortality (Witlox et al., 2010; Moskowitz et al., 2017; Sprung et al., 2017; Boone et al., 2020). Few effective prevention and treatment strategies are currently available (Berger et al., 2018; Mahanna-Gabrielli et al., 2019). Therefore, PNDs represent a major unmet health concern.
The causes of PNDs are complex and multifactorial, with general anesthetic drugs likely being one of several key contributing factors (Weinstein et al., 2018; Memtsoudis et al., 2019). Interestingly, we and others have postulated that astrocytes may play a causal role in PNDs (Terrando et al., 2013; Zurek et al., 2014; Wang et al., 2018; Li et al., 2020). Our previous studies using primary cultures of astrocytes, neurons, and astrocyte-neuron co-cultures have suggested that astrocytes contribute to the cognitive deficits that persist after brief exposure to general anesthetic drugs (Zurek et al., 2014; Wang et al., 2018). Indeed, we refer to these in vitro cell culture models that have demonstrated a crosstalk between astrocytes and neurons following exposure to anesthetic drugs as “PND in a dish.” Specifically, both an intravenous anesthetic drug (etomidate) and an inhalational agent (isoflurane) trigger a sustained increase in cell-surface expression and hence function of a subtype of γ-aminobutyric acid type A (GABAA) receptors in neurons (Zurek et al., 2014; Wang et al., 2018). Such an increase in GABAA receptor function is sustained after the anesthetic drug is eliminated and is associated with long-lasting cognitive deficits (Zurek et al., 2014; Li and Zhang, 2021; Zuo et al., 2021). Furthermore, in vitro studies have shown that anesthetic drugs act on astrocytes to stimulate the release of one or more soluble factors that crosstalk with neurons, triggering a persistent increase in GABAA receptor function in those neurons (Zurek et al., 2014; Wang et al., 2018). However, the receptors in astrocytes that act as targets for general anesthetic drugs have not yet been identified.
Astrocytes express a wide range of neurotransmitter receptors and transporters, including GABAA receptors, which allow them to sense and respond to their surroundings (Verkhratsky and Nedergaard, 2018; Mederos and Perea, 2019). In contrast to what typically occurs in neurons, the activation of GABAA receptors in astrocytes induces membrane depolarization, rather than hyperpolarization, and an increase in intracellular Ca2+ (Meier et al., 2008; Egawa et al., 2013; Mederos and Perea, 2019). These changes stimulate the release of various signaling molecules (Verkhratsky and Nedergaard, 2018). Because GABAA receptors in neurons represent the primary target of most general anesthetic drugs (Garcia et al., 2010), GABAA receptors in astrocytes may also be sensitive to commonly used drugs. These drugs may act upon GABAA receptors in astrocytes to depolarize the membrane potential and trigger the release of soluble factors. Indeed, studies showing that pentobarbital, and the benzodiazepine agonists flunitrazepam and midazolam, increased the activity of GABAA receptors in astrocytes were first reported in the 1980s and 1990s (Backus et al., 1988; Bormann and Kettenmann, 1988; Macvicar et al., 1989; Muller et al., 1994; Fraser et al., 1995). However, to date, no subsequent studies have directly examined the sensitivity of GABAA receptors in astrocytes to modern general anesthetic drugs that are now in common use.
The goal of this study was to determine whether two representative general anesthetic drugs, etomidate and sevoflurane, modulate the function of astrocytic GABAA receptors in hippocampal slices from mice. Etomidate is an intravenous agent that is often used for the induction of general anesthesia in critically ill patients because of its favorable hemodynamic profile (Hannam et al., 2019). Sevoflurane is one of the most commonly used inhalational anesthetic drugs (Brioni et al., 2017). Both these drugs have been shown to trigger the persistent increase in GABAA receptor function in neurons (Zurek et al., 2014; Wang et al., 2018). Our results show that etomidate and sevoflurane increase the function of GABAA receptors in astrocytes. These results provide the foundation for future studies, which will define the role of astrocytic GABAA receptors in the pathophysiology of PNDs and assist in the development of potential new treatments for these disorders.
Materials and Methods
Experimental Animals
All experiments were performed with C57BL/6 male mice at postnatal days 21–27 (Charles River, Montreal, QC, Canada). This age was selected because astrocytes have reached maturity (Zhou et al., 2006), and the quality of brain slices significantly reduces with further aging (Lipton et al., 1995; Ting et al., 2014). Mice were housed in the animal care facility at the University of Toronto (Toronto, Ontario, Canada).
Hippocampal Slice Preparation
Mice brains were obtained by decapitation after the mice were euthanized with a brief exposure to isoflurane. Sagittal brain slices (300 μm) containing hippocampus were prepared using a VT1200S vibratome (Leica, Deerfield, Illinois). Hippocampal slices were prepared in ice-cold sucrose-based cutting solution that contained (in mM): 212 sucrose, 25 NaHCO3, 5 KCl, 1.25 NaH2PO4, 10 glucose, 2 sodium pyruvate, 1.2 sodium ascorbate, 3.5 MgCl2, and 0.5 CaCl2. Slices were immediately transferred to a chamber containing artificial cerebrospinal fluid (aCSF) that contained (in mM): 125 NaCl, 25 NaHCO3, 2.5 KCl, 1.25 NaH2PO4, 10 glucose, 1.3 MgCl2, and 2.5 CaCl2. The slice chamber was first placed in a water bath (32°C, 30 min) for recovery of neuronal activities, and later placed at room temperature. All solutions were aerated with 95% O2/5% CO2 throughout the procedures.
Whole-Cell Recordings of Astrocytes
Whole-cell patch-clamp recordings were performed at room temperature from astrocytes located in the stratum radiatum of the CA1 region of the hippocampus. Slices were transferred to a submersion recording chamber, where they were perfused with aCSF at 3–4 ml/min and were visualized using a 400x microscope (BX50WI; Olympus, Tokyo, Japan). Glass pipette resistance ranged between 3 and 5 MΩ. All recordings were performed using a MultiClamp 700B amplifier (Molecular Devices, Sunnyvale, California, United States), and data were acquired with pCLAMP 10.6 (Molecular Devices) via a Digidata 1550A interface (Molecular Devices).
Recordings were conducted with a KCl-based internal solution that contained (in mM): 140 KCl, 0.5 CaCl2, 1 MgCl2, 5 EGTA, 10 HEPES, 3 Mg2+-ATP (pH 7.3 using KOH at 290 mOsm). Upon achieving the whole-cell patch configuration, cells were confirmed as astrocytes based on the unique electrophysiological properties including a low membrane resistance (RM < 15 MΩ), a low resting membrane potential (VM < -70 mV), and a unique linear I-V relationship (Zhou et al., 2006, 2009; Du et al., 2016). The RM and Ra were measured with “membrane test” protocol that is built into the pCLAMP 10.6 software (Molecular Devices). The resting membrane potential was measured in “I = 0” mode. The I-V relationship was tested by measuring currents that were generated in response to voltage steps from holding potentials that ranged from −180 to 0 mV, in 20 mV increments. Astrocytes were then voltage-clamped at their resting membrane potentials.
All slices were continuously perfused with aCSF that contained TTX (tetrodotoxin, 0.5 μM), APV ([2R]-amino-5-phosphonovaleric acid, 20 μM), and CNQX (6-Cyano-7-nitroquinoxaline-2,3-dione, 10 μM). Only recordings with an initial Ra less than 25 MΩ that varied less than 20% throughout the experiments were included in the analyses.
Drugs and Chemicals
TTX was purchased from Alomone Labs (Jerusalem, Israel). APV and CNQX were obtained from Hello Bio Inc. (Princeton, NJ, United States). GABA and picrotoxin were from Sigma–Aldrich (Oakville, ON, Canada), while etomidate was purchased from US Pharmacopeia (Rockville, MD, United States) and sevoflurane was obtained from Abbott Laboratories (North Chicago, IL, United States).
Stock solutions of etomidate (100 mM) were prepared by dissolving etomidate powder in propylene glycol (35% v/v in physiological saline) and were stored at 4°C (Sprung et al., 2000). A final concentration of etomidate at 100 μM was used for the studies. Sevoflurane (532 μM) was diluted from the saturated aqueous phase of sevoflurane and was prepared at room temperature, as previously described (Lecker et al., 2013). This concentration of sevoflurane is twice the MAC (Minimum Alveolar Concentration of anesthetics) value for sevoflurane and was selected to ensure adequate drug levels in the slices (Nishikawa and MacIver, 2001; Lecker et al., 2013). In brief, 50 ml of sevoflurane was mixed with 100 ml of aCSF in a gas-tight glass bottle and stored at 4°C overnight. Sevoflurane at the saturated aqueous phase was measured at 11.8 mM (Lecker et al., 2013).
GABA Puff Application
Focal puff applications of GABA (300 μM) were applied to the soma of the astrocytes using a Picospritzer system (Picospritzer II, Parker Hannifin, United States). A glass pipette (tip diameter 3–5 μm) that was filled with aCSF containing GABA (300 μM) was placed approximately 50–100 μm away from the cell soma before performing whole-cell configuration. The concentration of GABA was chosen based on a previous study (EC50 = 300 μM) (Ma et al., 2012). Puff pressure was set at 15–20 psi, and puff duration between 20 and 150 ms to obtain a baseline current amplitude of approximately 50–100 pA.
Data and Statistical Analyses
The peak amplitude, rise time, decay time, and area of GABA-evoked responses were analyzed with Clampfit 10.7 software (Molecular Devices). The rise time was measured as the time from 10 to 90% of peak amplitude, and the decay time was defined as the duration from 90 to 40% of peak amplitude due to fluctuations in late decay phase. The area of the current responses was measured to the point where currents returned to baseline.
Data are represented as mean ± SD (Standard Deviation). Statistical analyses were performed using R statistical software version 3.6.1 (R Foundation for Statistical Computing, Vienna, Austria). All continuous variables were tested to determine whether they met conditions of normality (Shapiro-Wilk test) and homogeneity of variance (Levene’s test). Paired Student’s t-test was performed to compare paired data. A two-tailed hypothesis test was used, and statistical significance was set at P < 0.05.
Results
Identification of Astrocytes in Hippocampal Slices
Astrocytes were identified in hippocampal slices based on their morphology and electrophysiological properties. We recorded from cells that were relatively small, as astrocytes have a diameter of approximately 10 μm with round or irregularly shaped somas (Zhou et al., 2006; Du et al., 2015). Morphology alone was not sufficient to identify the astrocytes as other cell types, such as interneurons, have similar structural properties (Zhou et al., 2006). Thus, we next examined the electrophysiological properties of each cell to confirm that the recorded cells were indeed astrocytes. Astrocytes have unique electrophysiological properties that allow them to be readily distinguished from other cell types including interneurons. Specifically, astrocytes have a low membrane resistance (RM < 15 MΩ), a relatively hyperpolarized resting membrane potential (VM < -70 mV), and they generate a linear current-to-voltage (I-V) relationship in response to voltage steps due to passive K+ membrane conductances (Zhou et al., 2006, 2009; Ma et al., 2014; Du et al., 2016). All the cells we recorded from had a low membrane resistance (3.2 ± 2.2 MΩ, n = 21) and a hyperpolarized resting membrane potential (−80.2 ± 3.7 mV, n = 21; Figure 1A). The cells also displayed a linear I-V relationship in response to voltage steps (−180–0 mV in 20 mV increments), as shown in Figures 1B,C.
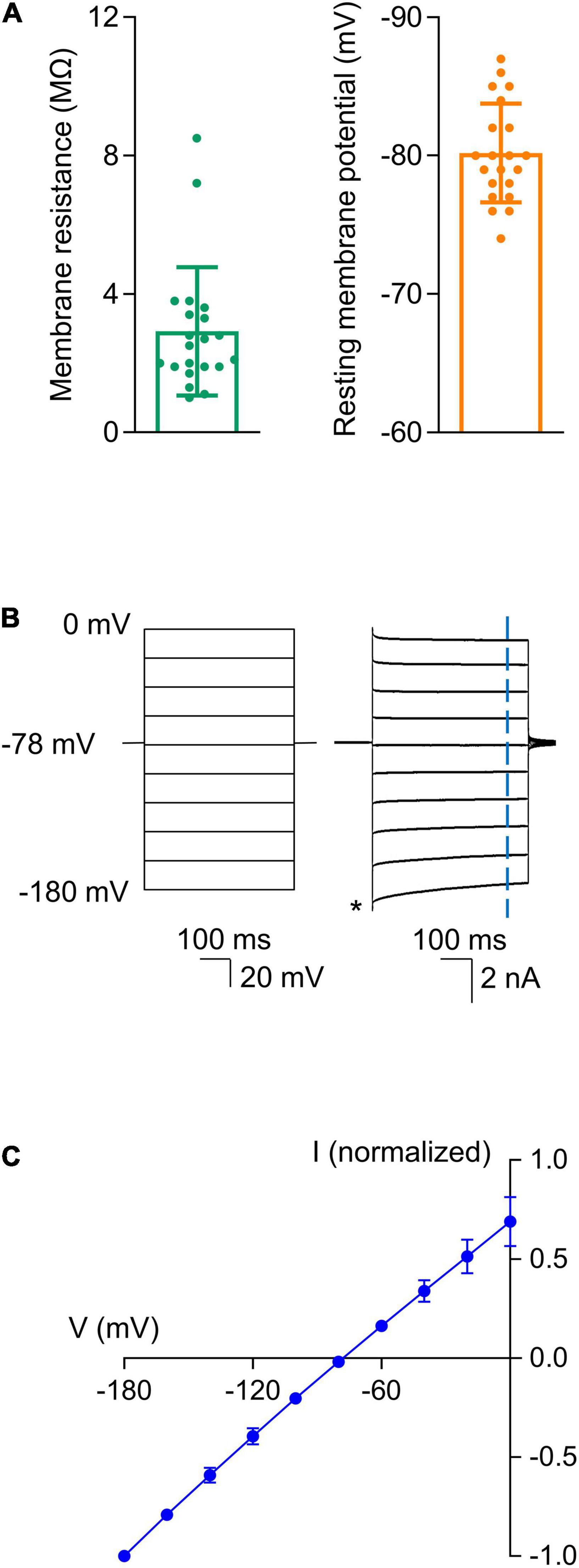
Figure 1. Astrocytes displayed unique electrophysiological properties. After the whole-cell patch configuration was achieved, cells were confirmed to be astrocytes based on their low membrane resistance, hyperpolarized resting membrane potentials, and linear I-V relationships. (A) Summarized data for membrane resistance and resting membrane potential (n = 21). (B) Linear I-V relationship of the astrocytes. Representative recordings show current responses (Right) to voltage steps (500 ms) that ranged from -180 to 0 mV in 20 mV increments (Left). The cell was held at -78 mV between voltage commands. Current amplitudes were measured 435 ms after initiation of each step voltage as indicated by the vertical blue dash line. The amplitudes were normalized to that at -180 mV as indicated by the asterisk. (C) Summarized I-V plot. Each data point represents the mean of values from 21 recorded astrocytes. The reversal potential obtained from the fitted I-V plot was -78 mV. Data are presented as mean ± SD.
GABA Activates γ-Aminobutyric Acid Type A Receptors in Astrocytes
To study the effects of general anesthetic drugs on the function of GABAA receptors in astrocytes, we first needed to record stable GABA-evoked responses. We used focal puff applications of GABA as previous studies have examined GABAA receptor-dependent currents using similar methods (Ma et al., 2012). A glass capillary that contained GABA (300 μM) was placed 50–100 μm away from the recorded cell (Figure 2A). After the whole-cell patch configuration was successfully established in astrocytes, a focal puff application of GABA was applied. The application of GABA consistently activated an inward current (Figure 2B). To confirm the GABA-evoked responses were generated by GABAA receptors, the non-competitive GABAA receptor antagonist picrotoxin (0.5 mM) was then added to the bath solution for 5–10 min and a subsequent puff of GABA was applied. The amplitude of the peak current was measured before and during the application of picrotoxin. The peak current was reduced to 28.9 ± 8.0% of the control (Figure 2B, 85.4 ± 12.0 pA for GABA vs. 24.0 ± 3.2 pA for GABA + picrotoxin, n = 3; P = 0.019, paired t-test). These results showed that the inward currents evoked by GABA were primarily generated by GABAA receptors.
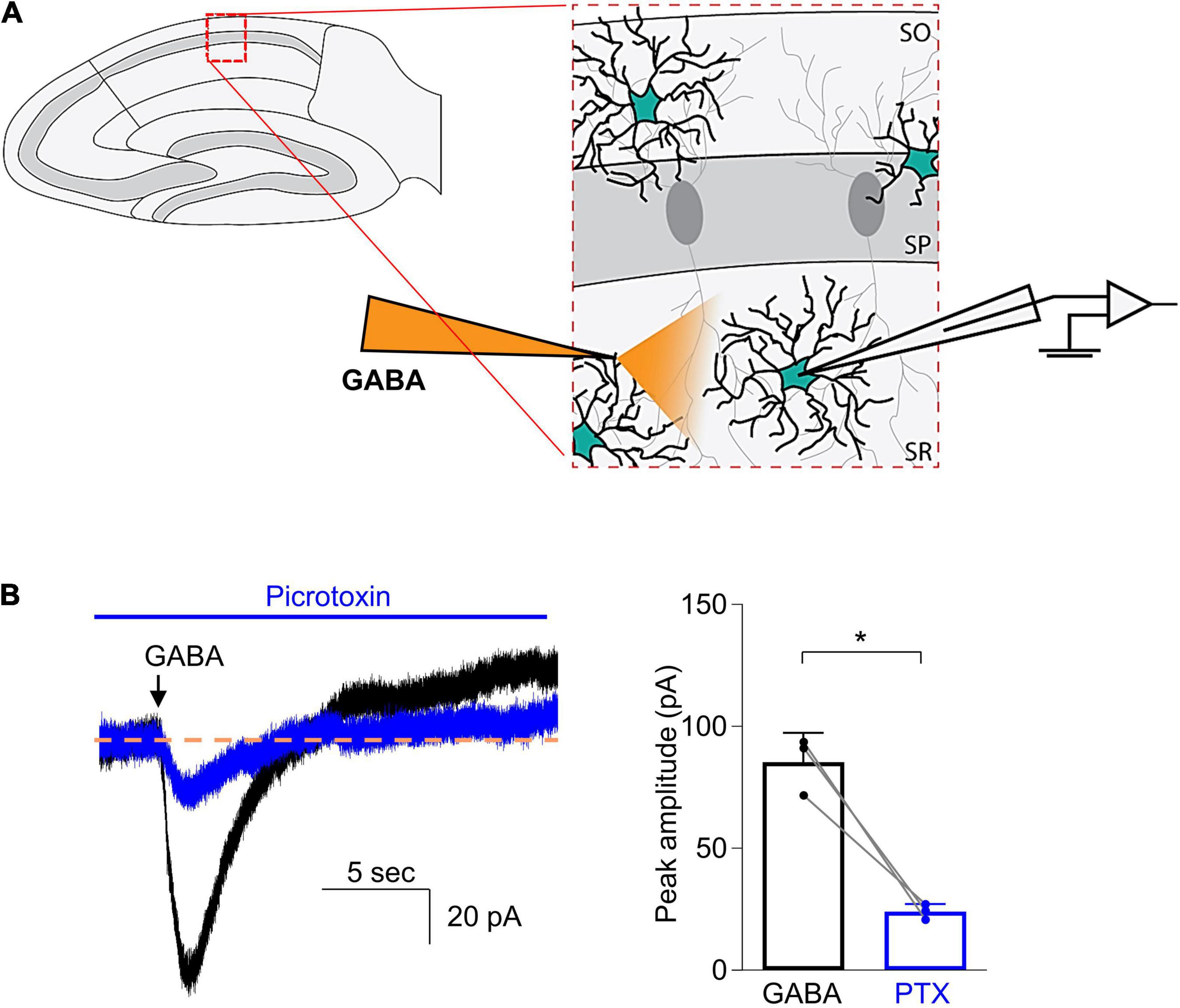
Figure 2. Focal puff application of GABA to astrocytes induced an inward current that was inhibited by picrotoxin. (A) Schematic drawing shows that a glass capillary containing GABA (300 μM) was placed 50–100 μm away from the recorded astrocyte in the stratum radiatum of CA1 region of a hippocampal slice. (B) Left: Representative traces demonstrate current responses to puff applications of GABA before (black) and during addition of picrotoxin (0.5 mM, blue), a non-competitive GABAA receptor antagonist. Right: Quantified data show the inhibitory effects of picrotoxin (PTX). n = 3, *P = 0.019, paired Student’s t-test. Data are presented as mean ± SD.
Etomidate Increases the Function of γ-Aminobutyric Acid Type A Receptors in Astrocytes
We next sought to examine the effects of etomidate (100 μM) on GABAA receptor-generated currents in astrocytes. After obtaining an initial baseline current in response to GABA (300 μM), the slices were perfused for 2 min with aCSF containing etomidate and a second puff of GABA was applied. The amplitude of the peak current (pA), the rise time and decay time (s) of the current, and the area (pA4⋅ms) under the current response curve were measured. Notably, we observed that the late decay phase of current evoked by GABA, both in the absence and the presence of etomidate, was somewhat unstable. Specifically, the decay current had several different undulating shapes, as shown in Figure 3A. This variability in the late phase of current decay differed from current recorded in hippocampal neurons, as observed by us and others (Bai et al., 1999; Caraiscos et al., 2004; Domínguez et al., 2016). To minimize the impact of the baseline instability on the analysis of current responses, the decay time of the current was measured from 90 to 40% of the peak amplitude.
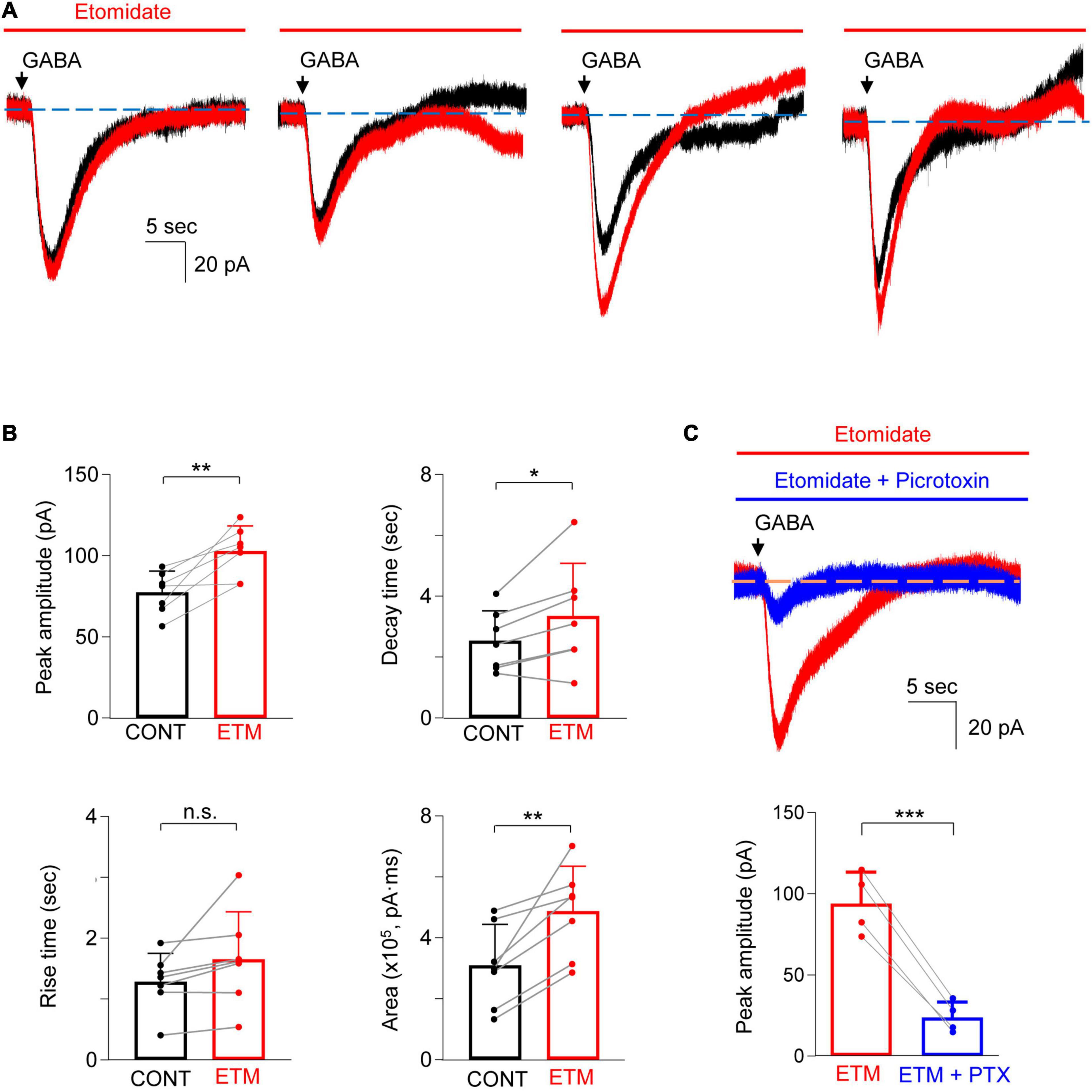
Figure 3. Etomidate potentiated GABA-evoked current in astrocytes. (A) Representative traces show the GABA-evoked responses before and during etomidate (ETM) treatment from four different astrocytes. Note the different shapes of the late phase of the current decay in both the absence and the presence of etomidate. (B) Summarized data for peak amplitude, rise time, decay time, and charge transfer (area). n = 7, *P < 0.05, **P < 0.01, n.s.: not significant, paired Student’s t-test. CONT: control. (C) Representative traces (top) and summarized data (bottom) show that GABA-evoked current in the presence of etomidate (ETM) is inhibited by picrotoxin (PTX, 0.5 mM, 5–10 min). n = 4, ***P = 0.001, paired Student’s t-test. Data are presented as mean ± SD.
Etomidate increased the amplitude of the current by 35.0 ± 24.4% (Figure 3B; control: 77.5 ± 13.0 pA, vs. etomidate: 102.9 ± 15.4 pA, n = 7; P = 0.006, paired t-test). Etomidate did not alter the current rise time (control: 1.3 ± 0.5 s vs. etomidate: 1.7 ± 0.8 s, n = 7; P = 0.10, paired t-test) but prolonged the decay time by 27.2 ± 24.3% (control: 2.6 ± 1.0 s vs. etomidate: 3.4 ± 1.7 s, n = 7; P = 0.037, paired t-test). The total charge transfer was also increased by 71.2 ± 45.9% [control: 3.1 ± 1.4 (×105) pA⋅ms vs. etomidate: 4.9 ± 1.5 (×105) pA⋅ms, n = 7; P = 0.006, paired t-test]. We next confirmed that GABA-evoked responses in the presence of etomidate were primarily generated by GABAA receptors. The co-application of etomidate and picrotoxin (0.5 mM) to the bath solution showed that the peak current was reduced by 75.3 ± 5.5% (Figure 3C; pre-picrotoxin: 94.0 ± 19.4 pA vs. picrotoxin: 23.8 ± 9.6 pA, n = 4; P = 0.001, paired t-test).
Sevoflurane Increases the Function of γ-Aminobutyric Acid Type A Receptors in Astrocytes
In the next set of studies, sevoflurane (532 μM) was added to the bath solution and the changes in the peak amplitude and time course of GABA-evoked currents were investigated (Figure 4A). Sevoflurane did not increase the peak current (Figure 4B; control: 75.3 ± 10.4 pA vs. sevoflurane: 71.9 ± 10.4 pA, n = 7; P = 0.21, paired t-test) nor did it increase the current rise time (Figure 4B; control: 1.4 ± 0.4 s vs. sevoflurane: 1.5 ± 0.4 s, n = 7; P = 0.33, paired t-test). However, sevoflurane prolonged the current decay by 28.3 ± 23.1% (Figure 4B; control: 2.2 ± 0.6 s vs. sevoflurane: 2.8 ± 0.9 s, n = 7; P = 0.030, paired t-test) and increased the total charge transfer by 51.8 ± 48.9% [Figure 4B; control: 2.86 ± 0.64 (×105) pA⋅ms vs. sevoflurane: 4.22 ± 1.10 (×105) pA⋅ms, n = 7; P = 0.029, paired t-test]. Thus, sevoflurane increased the GABA-evoked currents. GABA-evoked currents in the presence of sevoflurane were also inhibited by 64.4 ± 12.5% when picrotoxin (0.5 mM) was added to the bath solution (no picrotoxin: 76.9 ± 7.1 pA vs. picrotoxin: 27.4 ± 10.0 pA, n = 4; P = 0.003, paired t-test), confirming that the currents were mainly GABAA receptor-dependent (Figure 4C).
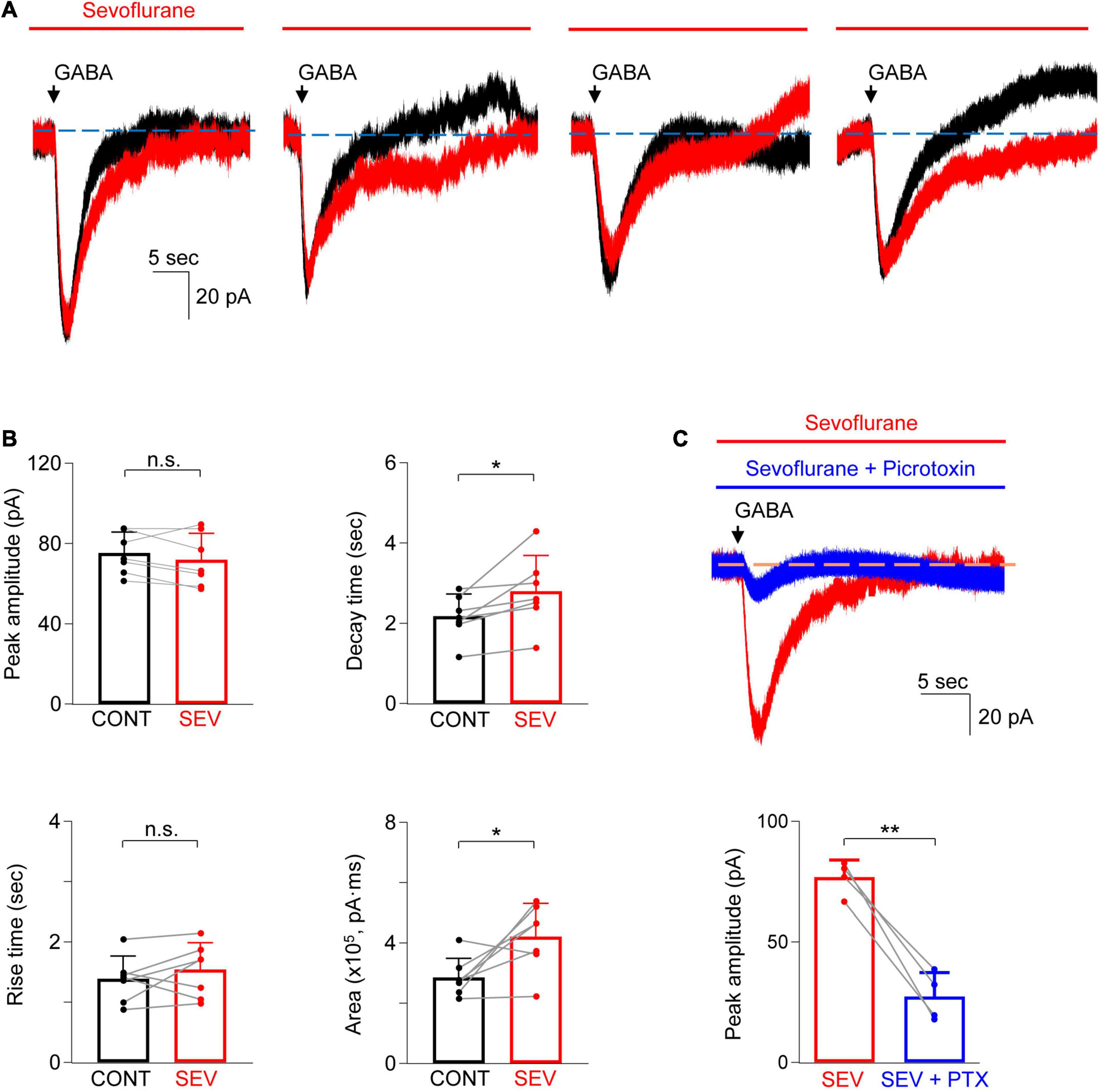
Figure 4. Sevoflurane potentiated GABA-evoked current in astrocytes. (A) Representative traces show the GABA-evoked responses before and during sevoflurane (SEV) treatment from four different astrocytes. Note the different shapes of the late phase of the current decay in both the absence and presence of sevoflurane. (B) Summarized data for peak amplitude, rise time, decay time, and charge transfer (area). n = 7, *P < 0.05, n.s.: not significant, paired Student’s t-test. CONT: control. (C) Representative traces (top) and summarized data (bottom) show that GABA-evoked current in the presence of sevoflurane (SEV) is inhibited by picrotoxin (PTX, 0.5 mM, 5–10 min). n = 4, **P = 0.003, paired Student’s t-test. Data are presented as mean ± SD.
Discussion
The goal of this study was to determine whether GABAA receptors in astrocytes are targets for etomidate and sevoflurane. We first recorded GABAA receptor–mediated current from astrocytes in the stratum radiatum of the CA1 region of hippocampal slices from mice and then showed that both etomidate and sevoflurane increased the GABA-evoked responses. More specifically, etomidate increased the peak amplitude of the current and prolonged its decay, whereas sevoflurane prolonged current decay but had no effect on the peak. Overall, both etomidate and sevoflurane increased the total charge transfer of the GABA-evoked responses. To the best of our knowledge, these results provide the first direct evidence that commonly used general anesthetic drugs increase the function of GABAA receptors in astrocytes.
As noted by others, it is technically challenging to perform voltage-clamp recording of GABA-evoked responses in astrocytes (Ma et al., 2014). While patch-clamp recording techniques have been widely used by us and others to study anesthetic modulation of GABAA receptors in neurons (Orser et al., 1994; Bai et al., 1999; Caraiscos et al., 2004; Schools et al., 2006; Zhou et al., 2021), astrocytes have a low membrane resistance because of passive K+ conductances and are extensively coupled into a syncytium through gap junctions (Ma et al., 2016), which makes patch-clamp recording difficult. Such unfavorable patch-clamp recording conditions cause the voltage change to occur primarily at the tip of the recording electrode, rather than across the cell membrane (Ma et al., 2014). Poor voltage-clamp conditions also cause instability of baseline currents and prevent accurate measurement of current responses during long-term whole-cell recordings. To minimize the impact of such current instability, we recorded from slices obtained from young mice and used a Picospritzer perfusion system to focally and rapidly deliver a transient puff (<150 ms) of GABA. Using this combined approach, we were able to record relatively stable GABA-evoked responses that were generated by GABAA receptors.
Our results provide convincing evidence that the function of astrocytic GABAA receptor is increased by commonly used general anesthetic drugs. These results are consistent with findings from several earlier studies that date back to the 1980s and 1990s of pentobarbital and benzodiazepines (Backus et al., 1988; Bormann and Kettenmann, 1988; Macvicar et al., 1989; Muller et al., 1994; Fraser et al., 1995). For instance, pentobarbital but not diazepam increased GABA-evoked responses in Bergmann glial cells of cerebellar slices from young animals (postnatal days 5–12) (Muller et al., 1994). However, these studies were undertaken at a development stage when glial cells are still immature (Zhou et al., 2006). In another study, using hippocampal slices, to which kainic acid had been applied to reduce the number of neurons, GABA-evoked responses in CA3 astrocytes increased after pentobarbital and flunitrazepam treatment (Macvicar et al., 1989). Although kainic acid helps in isolating astrocytes, it also modifies the surviving cells into a pathological state (Kim et al., 2007). The same limitation remains for acutely isolated astrocytes obtained through enzymatic digestion (Fraser et al., 1995). Surprisingly, since those earlier studies, little progress has been made regarding anesthetic modulation of astrocytic GABAA receptors, probably because of the technical challenges outlined above. We overcame these limitations by recording currents in mature astrocytes in brain slices from young mice.
Interestingly, we observed that etomidate, but not sevoflurane, increased the peak amplitude of GABA responses in astrocytes. At least three factors could contribute to this difference including drug bioavailability, the effects of the drugs on GABAA receptor kinetics, and the subunit composition of the underlying receptors. For instance, it is unlikely that “MAC equivalent” concentrations of etomidate and sevoflurane were present at the tip of the recording electrode as the physical properties of the drugs differ. Etomidate is stable in aqueous solution but requires a considerable time to penetrate the brain slices. Indeed, it can take as long as 1–2 h to reach an equilibrium in brain slices (Benkwitz et al., 2007). In contrast, sevoflurane readily diffuses into brain tissues, but rapidly evaporates from the perfusion solution (Nishikawa and MacIver, 2001; Sebel et al., 2006). Another reason could be differences in drug action on GABAA receptor kinetics. Anesthetic drugs generally increase the potency of GABA, increase the rate of receptor activation, and slow the rate of receptor deactivation (Orser et al., 1994; Yang and Uchida, 1996; Belelli et al., 1997; Bai et al., 1999; Benkwitz et al., 2004). However, etomidate, similar to other intravenous anesthetics including propofol, reduced desensitization, whereas volatile anesthetics increased receptor desensitization (Wu et al., 1996; Liu et al., 2015). Since the peak current reflects the summed effects of receptor activation, deactivation and desensitization, differences in the peak current could result from differences in drug action on receptor kinetics. Finally, the effects of anesthetic drugs on GABAA receptor kinetics are highly dependent on the subunit composition of the receptors. Thus, the subunit composition of the heterogeneous GABAA receptors influences the response to anesthetic drugs (Uchida et al., 1995; Krasowski et al., 1998; Jenkins et al., 2001; Nishikawa and Harrison, 2003; Benkwitz et al., 2004; Zhong et al., 2008; Hoft et al., 2014; Woll et al., 2018; Liao et al., 2019).
Our results raise some interesting questions that are worthy of future studies. It would be of interest to examine the concentration-dependence of anesthetic modulation of astrocytic GABAA receptors and whether the anesthetic sensitivity of astrocytic and neuronal GABAA receptors differ. Given technical challenges with standard whole-cell patch-clamp recordings from astrocytes, we investigated the effects of just one anesthetic concentration with one concentration of GABA. A previous study overcame some of these technical challenges by using a dual-patch technique that permitted simultaneous recordings of membrane currents and potentials in astrocytes (Ma et al., 2014). Future studies might also investigate GABAA receptors in astrocytes that are mechanically isolated from brain slices or macro patches that are excised from astrocytes. These techniques allow drugs and agonists to be rapidly applied to and then washed away from the recorded astrocytes. Drug concentrations can also be more accurately controlled to study the concentration-dependent effects of anesthetic drugs. Thus, the approaches allow anesthetic effects on GABAA receptor responses in astrocytes and neurons to be more effectively compared.
Another important issue that requires further study is whether astrocytic GABAA receptors play a role in PNDs in vivo. Given that exposure of astrocytes to anesthetic drugs triggers a sustained increase in cell-surface expression and function of GABAA receptors in neurons in vitro, it is possible the drugs act upon astrocytic GABAA receptors to cause similar changes in vivo. Indeed, general anesthetic drugs increase Ca2+ signaling in astrocytes by activating GABAA receptors in vivo (Meier et al., 2008; Thrane et al., 2012). This increase in cytosolic Ca2+ may trigger the release of soluble factor(s) that modify the function of neighboring neurons. Future studies will determine whether astrocytic GABAA receptors contribute to this crosstalk with neuronal GABAA receptors that may contribute to PNDs, as well as identify the soluble factor(s). Using genetic approaches, such as astrocyte-specific gene knockdown/knockout, and novel CRISPR-based technology to target anesthetic-sensitive astrocytic GABAA receptors in vitro and in vivo, may help answer these questions (Mori et al., 2006; Shinohara et al., 2016; Meneghini et al., 2021). Such studies may lead to the discovery of novel strategies to mitigate the cognitive dysfunction experienced by older patients with PNDs.
This study had some limitations. We were able to test only the early phase of current decay (90–40% decay time) induced by puff applications of GABA in astrocytes because the late phase is highly variable in both the absence and presence of anesthetic treatment. Such variability could be due to low membrane resistance of astrocytes and to changes in membrane resistance caused by secondary inhibition of the K+ channel after application of GABA (Ma et al., 2012, 2014). As noted above, performing dual-patch recordings in hippocampal astrocytes may reduce this variability and may help in further evaluating the effects of anesthetics on astrocytic GABAA receptors. In addition, this study focused on the effects of anesthetics in mature hippocampal astrocytes at a single age of animal. However, a distinct feature of astrocytes is their heterogeneity across different brain regions (Matias et al., 2019; Batiuk et al., 2020). Also, the subunit compositions and expression profiles of GABAA receptors are heterogeneous across brain regions and at different developmental stages (Backus et al., 1988; Macvicar et al., 1989; Muller et al., 1994; Fraser et al., 1995; Hoft et al., 2014; Zhang et al., 2016). Therefore, the anesthetic effects on GABAA receptors in astrocytes may differ in different brain regions and at different ages.
In summary, etomidate and sevoflurane, two modern general anesthetic drugs used in clinical settings, increased GABAA receptor function in hippocampal astrocytes. These results provide the foundation for future studies that will determine whether astrocytic GABAA receptors contribute to PNDs.
Data Availability Statement
The original contributions presented in the study are included in the article, further inquiries can be directed to the corresponding author.
Ethics Statement
The animal study was reviewed and approved by the Animal Care Committee of the University of Toronto (Toronto, Ontario, Canada).
Author Contributions
WC, D-SW, and BAO designed and developed the experiments and prepared manuscript. WC executed the experiments and analyzed the data. SK and AP helped in the design and development of experiments. All authors have approved the final manuscript.
Funding
This work was supported by the Canadian Institutes of Health Research (FDN-154312) to BAO, the Ontario Graduate Scholarship and Canada Graduate Scholarship-Doctoral Program from the Canadian Institutes of Health Research to SK, Kirk Weber Research Award in Anesthesia to SK and AP, and the National Research Foundation of Korea (NRF-2018R1C1B6003139 and NRF-2019M3E5D1A02068575) to WC.
Conflict of Interest
BAO serves on the Board of Trustees of the International Anesthesia Research Society (San Francisco, California, United States) and was a co-director of the Perioperative Brain Health Centre (Toronto, Ontario, Canada; http://www.perioperativebrainhealth.com). She was a named inventor on a Canadian patent (2,852,978) and two U.S. patents (9,517,265 and 10,981,954). The new methods identified in the patents aim to prevent and treat delirium and persistent neurocognitive deficits after anesthesia and surgery, as well as to treat mood disorders. BAO collaborates on clinical studies supported by in-kind software donations from Cogstate Ltd. (New Haven, Connecticut, United States).
The remaining authors declare that the research was conducted in the absence of any commercial or financial relationships that could be construed as a potential conflict of interest.
Publisher’s Note
All claims expressed in this article are solely those of the authors and do not necessarily represent those of their affiliated organizations, or those of the publisher, the editors and the reviewers. Any product that may be evaluated in this article, or claim that may be made by its manufacturer, is not guaranteed or endorsed by the publisher.
References
Backus, K. H., Kettenmann, H., and Schachner, M. (1988). Effect of benzodiazepines and pentobarbital on the GABA-induced depolarization in cultured astrocytes. Glia 1, 132–140. doi: 10.1002/glia.440010205
Bai, D., Pennefather, P. S., Macdonald, J. F., and Orser, B. A. (1999). The general anesthetic propofol slows deactivation and desensitization of GABA(A) receptors. J. Neurosci. 19, 10635–10646. doi: 10.1523/JNEUROSCI.19-24-10635.1999
Batiuk, M. Y., Martirosyan, A., Wahis, J., De Vin, F., Marneffe, C., Kusserow, C., et al. (2020). Identification of region-specific astrocyte subtypes at single cell resolution. Nat. Commun. 11:1220. doi: 10.1038/s41467-019-14198-8
Belelli, D., Lambert, J. J., Peters, J. A., Wafford, K., and Whiting, P. J. (1997). The interaction of the general anesthetic etomidate with the gamma-aminobutyric acid type A receptor is influenced by a single amino acid. Proc. Natl. Acad. Sci. U.S.A. 94, 11031–11036. doi: 10.1073/pnas.94.20.11031
Benkwitz, C., Banks, M. I., and Pearce, R. A. (2004). Influence of GABAA receptor gamma2 splice variants on receptor kinetics and isoflurane modulation. Anesthesiology 101, 924–936. doi: 10.1097/00000542-200410000-00018
Benkwitz, C., Liao, M., Laster, M. J., Sonner, J. M., Eger, E. I. 2nd., and Pearce, R. A. (2007). Determination of the EC50 amnesic concentration of etomidate and its diffusion profile in brain tissue: implications for in vitro studies. Anesthesiology 106, 114–123. doi: 10.1097/00000542-200701000-00020
Berger, M., Schenning, K. J., Brown, C. H. T., Deiner, S. G., Whittington, R. A., Eckenhoff, R. G., et al. (2018). Best practices for postoperative brain health: recommendations from the fifth international perioperative neurotoxicity working group. Anesth. Analg. 127, 1406–1413. doi: 10.1213/ANE.0000000000003841
Boone, M. D., Sites, B., Von Recklinghausen, F. M., Mueller, A., Taenzer, A. H., and Shaefi, S. (2020). Economic burden of postoperative neurocognitive disorders among US medicare patients. JAMA Netw. Open 3:e208931. doi: 10.1001/jamanetworkopen.2020.8931
Bormann, J., and Kettenmann, H. (1988). Patch-clamp study of gamma-aminobutyric acid receptor Cl– channels in cultured astrocytes. Proc. Natl. Acad. Sci. U.S.A. 85, 9336–9340. doi: 10.1073/pnas.85.23.9336
Brioni, J. D., Varughese, S., Ahmed, R., and Bein, B. (2017). A clinical review of inhalation anesthesia with sevoflurane: from early research to emerging topics. J. Anesth. 31, 764–778. doi: 10.1007/s00540-017-2375-6
Caraiscos, V. B., Newell, J. G., You-Ten, K. E., Elliott, E. M., Rosahl, T. W., Wafford, K. A., et al. (2004). Selective enhancement of tonic GABAergic inhibition in murine hippocampal neurons by low concentrations of the volatile anesthetic isoflurane. J. Neurosci. 24, 8454–8458. doi: 10.1523/JNEUROSCI.2063-04.2004
De Majo, M., Koontz, M., Rowitch, D., and Ullian, E. M. (2020). An update on human astrocytes and their role in development and disease. Glia 68, 685–704. doi: 10.1002/glia.23771
Domínguez, S., Fernández De Sevilla, D., and Buño, W. (2016). Muscarinic long-term enhancement of tonic and phasic GABAA inhibition in Rat CA1 pyramidal neurons. Front. Cell. Neurosci. 10:244. doi: 10.3389/fncel.2016.00244
Du, Y., Kiyoshi, C. M., Wang, Q., Wang, W., Ma, B., Alford, C. C., et al. (2016). Genetic deletion of TREK-1 or TWIK-1/TREK-1 potassium channels does not alter the basic electrophysiological properties of mature hippocampal astrocytes in Situ. Front. Cell. Neurosci. 10:13. doi: 10.3389/fncel.2016.00013
Du, Y., Ma, B., Kiyoshi, C. M., Alford, C. C., Wang, W., and Zhou, M. (2015). Freshly dissociated mature hippocampal astrocytes exhibit passive membrane conductance and low membrane resistance similarly to syncytial coupled astrocytes. J. Neurophysiol. 113, 3744–3750. doi: 10.1152/jn.00206.2015
Egawa, K., Yamada, J., Furukawa, T., Yanagawa, Y., and Fukuda, A. (2013). Cl– homeodynamics in gap junction-coupled astrocytic networks on activation of GABAergic synapses. J. Physiol. 591, 3901–3917. doi: 10.1113/jphysiol.2013.257162
Evered, L., Silbert, B., Knopman, D. S., Scott, D. A., Dekosky, S. T., Rasmussen, L. S., et al. (2018). Recommendations for the Nomenclature of Cognitive Change Associated with Anaesthesia and Surgery-2018. Anesthesiology 129, 872–879.
Fraser, D. D., Duffy, S., Angelides, K. J., Perez-Velazquez, J. L., Kettenmann, H., and MacVicar, B. A. (1995). GABAA/benzodiazepine receptors in acutely isolated hippocampal astrocytes. J. Neurosci. 15, 2720–2732. doi: 10.1523/JNEUROSCI.15-04-02720.1995
Garcia, P. S., Kolesky, S. E., and Jenkins, A. (2010). General anesthetic actions on GABA(A) receptors. Curr. Neuropharmacol. 8, 2–9.
Hannam, J. A., Mitchell, S. J., Cumin, D., Frampton, C., Merry, A. F., Moore, M. R., et al. (2019). Haemodynamic profiles of etomidate vs propofol for induction of anaesthesia: a randomised controlled trial in patients undergoing cardiac surgery. Br. J. Anaesth. 122, 198–205. doi: 10.1016/j.bja.2018.09.027
Hoft, S., Griemsmann, S., Seifert, G., and Steinhauser, C. (2014). Heterogeneity in expression of functional ionotropic glutamate and GABA receptors in astrocytes across brain regions: insights from the thalamus. Philos. Trans. R. Soc. Lond. B. Biol. Sci. 369:20130602. doi: 10.1098/rstb.2013.0602
Jenkins, A., Greenblatt, E. P., Faulkner, H. J., Bertaccini, E., Light, A., Lin, A., et al. (2001). Evidence for a common binding cavity for three general anesthetics within the GABAA receptor. J. Neurosci. 21:Rc136. doi: 10.1523/JNEUROSCI.21-06-j0002.2001
Kim, D. W., Lee, J. H., Park, S. K., Yang, W. M., Jeon, G. S., Lee, Y. H., et al. (2007). Astrocytic expressions of phosphorylated Akt, GSK3beta and CREB following an excitotoxic lesion in the mouse hippocampus. Neurochem. Res. 32, 1460–1468. doi: 10.1007/s11064-007-9332-y
Krasowski, M. D., Koltchine, V. V., Rick, C. E., Ye, Q., Finn, S. E., and Harrison, N. L. (1998). Propofol and other intravenous anesthetics have sites of action on the gamma-aminobutyric acid type A receptor distinct from that for isoflurane. Mol. Pharmacol. 53, 530–538. doi: 10.1124/mol.53.3.530
Lecker, I., Yin, Y., Wang, D. S., and Orser, B. A. (2013). Potentiation of GABAA receptor activity by volatile anaesthetics is reduced by α5GABAA receptor-preferring inverse agonists. Br. J. Anaesth. 110(Suppl. 1), i73–i81. doi: 10.1093/bja/aet038
Li, D., Chen, M., Meng, T., and Fei, J. (2020). Hippocampal microglial activation triggers a neurotoxic-specific astrocyte response and mediates etomidate-induced long-term synaptic inhibition. J. Neuroinflamm. 17:109. doi: 10.1186/s12974-020-01799-0
Li, L., and Zhang, C. (2021). Venlafaxine attenuated the cognitive and memory deficit in mice exposed to isoflurane alone. Front. Neurol. 12:591223. doi: 10.3389/fneur.2021.591223
Liao, Y., Liu, X., Jounaidi, Y., Forman, S. A., and Feng, H. J. (2019). Etomidate effects on desensitization and deactivation of α4β3δ GABA(A) receptors inducibly expressed in HEK293 TetR cells. J. Pharmacol. Exp. Ther. 368, 100–105. doi: 10.1124/jpet.118.252403
Lipton, P., Aitken, P. G., Dudek, F. E., Eskessen, K., Espanol, M. T., Ferchmin, P. A., et al. (1995). Making the best of brain slices: comparing preparative methods. J. Neurosci. Methods 59, 151–156. doi: 10.1016/0165-0270(94)00205-u
Liu, K., Jounaidi, Y., Forman, S. A., and Feng, H. J. (2015). Etomidate uniquely modulates the desensitization of recombinant α1β3δ GABA(A) receptors. Neuroscience 300, 307–313.
Liu, Z., and Chopp, M. (2016). Astrocytes, therapeutic targets for neuroprotection and neurorestoration in ischemic stroke. Prog. Neurobiol. 144, 103–120. doi: 10.1016/j.pneurobio.2015.09.008
Ma, B., Buckalew, R., Du, Y., Kiyoshi, C. M., Alford, C. C., Wang, W., et al. (2016). Gap junction coupling confers isopotentiality on astrocyte syncytium. Glia 64, 214–226. doi: 10.1002/glia.22924
Ma, B., Xu, G., Wang, W., Enyeart, J. J., and Zhou, M. (2014). Dual patch voltage clamp study of low membrane resistance astrocytes in situ. Mol. Brain 7:18. doi: 10.1186/1756-6606-7-18
Ma, B. F., Xie, M. J., and Zhou, M. (2012). Bicarbonate efflux via GABA(A) receptors depolarizes membrane potential and inhibits two-pore domain potassium channels of astrocytes in rat hippocampal slices. Glia 60, 1761–1772. doi: 10.1002/glia.22395
Macvicar, B. A., Tse, F. W., Crichton, S. A., and Kettenmann, H. (1989). GABA-activated Cl– channels in astrocytes of hippocampal slices. J. Neurosci. 9, 3577–3583. doi: 10.1523/JNEUROSCI.09-10-03577.1989
Mahanna-Gabrielli, E., Schenning, K. J., Eriksson, L. I., Browndyke, J. N., Wright, C. B., Culley, D. J., et al. (2019). State of the clinical science of perioperative brain health: report from the American Society of Anesthesiologists Brain Health Initiative Summit 2018. Br. J. Anaesth. 123, 464–478.
Matias, I., Morgado, J., and Gomes, F. C. A. (2019). Astrocyte heterogeneity: impact to brain aging and disease. Front. Aging Neurosci. 11:59. doi: 10.3389/fnagi.2019.00059
Mederos, S., and Perea, G. (2019). GABAergic-astrocyte signaling: a refinement of inhibitory brain networks. Glia 67, 1842–1851. doi: 10.1002/glia.23644
Meier, S. D., Kafitz, K. W., and Rose, C. R. (2008). Developmental profile and mechanisms of GABA-induced calcium signaling in hippocampal astrocytes. Glia 56, 1127–1137. doi: 10.1002/glia.20684
Memtsoudis, S., Cozowicz, C., Zubizarreta, N., Weinstein, S. M., Liu, J., Kim, D. H., et al. (2019). Risk factors for postoperative delirium in patients undergoing lower extremity joint arthroplasty: a retrospective population-based cohort study. Reg. Anesth. Pain. Med. 44, 934–943.
Meneghini, V., Peviani, M., Luciani, M., Zambonini, G., and Gritti, A. (2021). Delivery platforms for CRISPR/Cas9 genome editing of glial cells in the central nervous system. Front. Genome Editing 3:644319. doi: 10.3389/fgeed.2021.644319
Mori, T., Tanaka, K., Buffo, A., Wurst, W., Kühn, R., and Götz, M. (2006). Inducible gene deletion in astroglia and radial glia—a valuable tool for functional and lineage analysis. Glia 54, 21–34. doi: 10.1002/glia.20350
Moskowitz, E. E., Overbey, D. M., Jones, T. S., Jones, E. L., Arcomano, T. R., Moore, J. T., et al. (2017). Post-operative delirium is associated with increased 5-year mortality. Am. J. Surg. 214, 1036–1038. doi: 10.1016/j.amjsurg.2017.08.034
Muller, T., Fritschy, J. M., Grosche, J., Pratt, G. D., Mohler, H., and Kettenmann, H. (1994). Developmental regulation of voltage-gated K+ channel and GABAA receptor expression in Bergmann glial cells. J. Neurosci. 14, 2503–2514. doi: 10.1523/JNEUROSCI.14-05-02503.1994
Nishikawa, K., and Harrison, N. L. (2003). The actions of sevoflurane and desflurane on the gamma-aminobutyric acid receptor type A: effects of TM2 mutations in the alpha and beta subunits. Anesthesiology 99, 678–684. doi: 10.1097/00000542-200309000-00024
Nishikawa, K., and MacIver, M. B. (2001). Agent-selective effects of volatile anesthetics on GABAA receptor-mediated synaptic inhibition in hippocampal interneurons. Anesthesiology 94, 340–347. doi: 10.1097/00000542-200102000-00025
Orser, B. A., Wang, L. Y., Pennefather, P. S., and Macdonald, J. F. (1994). Propofol modulates activation and desensitization of GABAA receptors in cultured murine hippocampal neurons. J. Neurosci. 14, 7747–7760. doi: 10.1523/JNEUROSCI.14-12-07747.1994
Santello, M., Toni, N., and Volterra, A. (2019). Astrocyte function from information processing to cognition and cognitive impairment. Nat. Neurosci. 22, 154–166. doi: 10.1038/s41593-018-0325-8
Schools, G. P., Zhou, M., and Kimelberg, H. K. (2006). Development of gap junctions in hippocampal astrocytes: evidence that whole cell electrophysiological phenotype is an intrinsic property of the individual cell. J. Neurophysiol. 96, 1383–1392. doi: 10.1152/jn.00449.2006
Sebel, L. E., Richardson, J. E., Singh, S. P., Bell, S. V., and Jenkins, A. (2006). Additive effects of sevoflurane and propofol on gamma-aminobutyric acid receptor function. Anesthesiology 104, 1176–1183. doi: 10.1097/00000542-200606000-00012
Shinohara, Y., Konno, A., Takahashi, N., Matsuzaki, Y., Kishi, S., and Hirai, H. (2016). Viral vector-based dissection of marmoset GFAP promoter in mouse and marmoset brains. PLoS One 11:e0162023. doi: 10.1371/journal.pone.0162023
Siracusa, R., Fusco, R., and Cuzzocrea, S. (2019). Astrocytes: role and functions in brain pathologies. Front. Pharmacol. 10:1114. doi: 10.3389/fphar.2019.01114
Sprung, J., Ogletree-Hughes, M. L., and Moravec, C. S. (2000). The effects of etomidate on the contractility of failing and nonfailing human heart muscle. Anesth. Analg. 91, 68–75. doi: 10.1097/00000539-200007000-00014
Sprung, J., Roberts, R. O., Weingarten, T. N., Nunes Cavalcante, A., Knopman, D. S., Petersen, R. C., et al. (2017). Postoperative delirium in elderly patients is associated with subsequent cognitive impairment. Br. J. Anaesth. 119, 316–323. doi: 10.1093/bja/aex130
Terrando, N., Gómez-Galán, M., Yang, T., Carlström, M., Gustavsson, D., Harding, R. E., et al. (2013). Aspirin-triggered resolvin D1 prevents surgery-induced cognitive decline. Faseb J. 27, 3564–3571. doi: 10.1096/fj.13-230276
Thrane, A. S., Rangroo Thrane, V., Zeppenfeld, D., Lou, N., Xu, Q., Nagelhus, E. A., et al. (2012). General anesthesia selectively disrupts astrocyte calcium signaling in the awake mouse cortex. Proc. Natl. Acad. Sci. U.S.A. 109, 18974–18979. doi: 10.1073/pnas.1209448109
Ting, J. T., Daigle, T. L., Chen, Q., and Feng, G. (2014). Acute brain slice methods for adult and aging animals: application of targeted patch clamp analysis and optogenetics. Methods Mol. Biol. 1183, 221–242. doi: 10.1007/978-1-4939-1096-0_14
Uchida, I., Kamatchi, G., Burt, D., and Yang, J. (1995). Etomidate potentiation of GABAA receptor gated current depends on the subunit composition. Neurosci. Lett. 185, 203–206. doi: 10.1016/0304-3940(95)11263-v
Verkhratsky, A., and Nedergaard, M. (2018). Physiology of astroglia. Physiol. Rev. 98, 239–389. doi: 10.1152/physrev.00042.2016
Wang, D. S., Kaneshwaran, K., Lei, G., Mostafa, F., Wang, J., Lecker, I., et al. (2018). Dexmedetomidine prevents excessive gamma-aminobutyric acid type A receptor function after anesthesia. Anesthesiology 129, 477–489. doi: 10.1097/ALN.0000000000002311
Wang, Q., Jie, W., Liu, J. H., Yang, J. M., and Gao, T. M. (2017). An astroglial basis of major depressive disorder? An overview. Glia 65, 1227–1250. doi: 10.1002/glia.23143
Weinstein, S. M., Poultsides, L., Baaklini, L. R., Mörwald, E. E., Cozowicz, C., Saleh, J. N., et al. (2018). Postoperative delirium in total knee and hip arthroplasty patients: a study of perioperative modifiable risk factors. Br. J. Anaesth. 120, 999–1008. doi: 10.1016/j.bja.2017.12.046
Witlox, J., Eurelings, L. S., De Jonghe, J. F., Kalisvaart, K. J., Eikelenboom, P., and Van Gool, W. A. (2010). Delirium in elderly patients and the risk of postdischarge mortality, institutionalization, and dementia: a meta-analysis. JAMA 304, 443–451. doi: 10.1001/jama.2010.1013
Woll, K. A., Zhou, X., Bhanu, N. V., Garcia, B. A., Covarrubias, M., Miller, K. W., et al. (2018). Identification of binding sites contributing to volatile anesthetic effects on GABA type A receptors. Faseb J. 32, 4172–4189. doi: 10.1096/fj.201701347R
Wu, J., Harata, N., and Akaike, N. (1996). Potentiation by sevoflurane of the gamma-aminobutyric acid-induced chloride current in acutely dissociated CA1 pyramidal neurones from rat hippocampus. Br. J. Pharmacol. 119, 1013–1021. doi: 10.1111/j.1476-5381.1996.tb15772.x
Yang, J., and Uchida, I. (1996). Mechanisms of etomidate potentiation of GABAA receptor-gated currents in cultured postnatal hippocampal neurons. Neuroscience 73, 69–78. doi: 10.1016/0306-4522(96)00018-8
Zhang, X., Alnafisah, R. S., Hamoud, A. A., Shukla, R., Wen, Z., Mccullumsmith, R. E., et al. (2021). Role of astrocytes in major neuropsychiatric disorders. Neurochem. Res. 46, 2715–2730. doi: 10.1007/s11064-020-03212-x
Zhang, Y., Sloan, S. A., Clarke, L. E., Caneda, C., Plaza, C. A., Blumenthal, P. D., et al. (2016). Purification and characterization of progenitor and mature human astrocytes reveals transcriptional and functional differences with mouse. Neuron 89, 37–53. doi: 10.1016/j.neuron.2015.11.013
Zhong, H., Rüsch, D., and Forman, S. A. (2008). Photo-activated azi-etomidate, a general anesthetic photolabel, irreversibly enhances gating and desensitization of gamma-aminobutyric acid type A receptors. Anesthesiology 108, 103–112. doi: 10.1097/01.anes.0000296074.33999.52
Zhou, M., Du, Y., Aten, S., and Terman, D. (2021). On the electrical passivity of astrocyte potassium conductance. J. Neurophysiol. 126, 1403–1419. doi: 10.1152/jn.00330.2021
Zhou, M., Schools, G. P., and Kimelberg, H. K. (2006). Development of GLAST(+) astrocytes and NG2(+) glia in rat hippocampus CA1: mature astrocytes are electrophysiologically passive. J. Neurophysiol. 95, 134–143. doi: 10.1152/jn.00570.2005
Zhou, M., Xu, G., Xie, M., Zhang, X., Schools, G. P., Ma, L., et al. (2009). TWIK-1 and TREK-1 are potassium channels contributing significantly to astrocyte passive conductance in rat hippocampal slices. J. Neurosci. 29, 8551–8564. doi: 10.1523/JNEUROSCI.5784-08.2009
Zuo, W., Zhao, J., Zhang, J., Fang, Z., Deng, J., Fan, Z., et al. (2021). MD2 contributes to the pathogenesis of perioperative neurocognitive disorder via the regulation of α5GABA(A) receptors in aged mice. J. Neuroinflamm. 18:204. doi: 10.1186/s12974-021-02246-4
Keywords: astrocyte, GABAA receptors, general anesthesia, etomidate, sevoflurane, perioperative neurocognitive disorders, patch-clamp
Citation: Chung W, Wang D-S, Khodaei S, Pinguelo A and Orser BA (2022) GABAA Receptors in Astrocytes Are Targets for Commonly Used Intravenous and Inhalational General Anesthetic Drugs. Front. Aging Neurosci. 13:802582. doi: 10.3389/fnagi.2021.802582
Received: 26 October 2021; Accepted: 20 December 2021;
Published: 11 January 2022.
Edited by:
Zhongcong Xie, Massachusetts General Hospital and Harvard Medical School, United StatesReviewed by:
Yiying Zhang, Massachusetts General Hospital and Harvard Medical School, United StatesJian-Jun Yang, Zhengzhou University, China
Copyright © 2022 Chung, Wang, Khodaei, Pinguelo and Orser. This is an open-access article distributed under the terms of the Creative Commons Attribution License (CC BY). The use, distribution or reproduction in other forums is permitted, provided the original author(s) and the copyright owner(s) are credited and that the original publication in this journal is cited, in accordance with accepted academic practice. No use, distribution or reproduction is permitted which does not comply with these terms.
*Correspondence: Beverley A. Orser, YmV2ZXJsZXkub3JzZXJAdXRvcm9udG8uY2E=
†These authors have contributed equally to this work and share first authorship