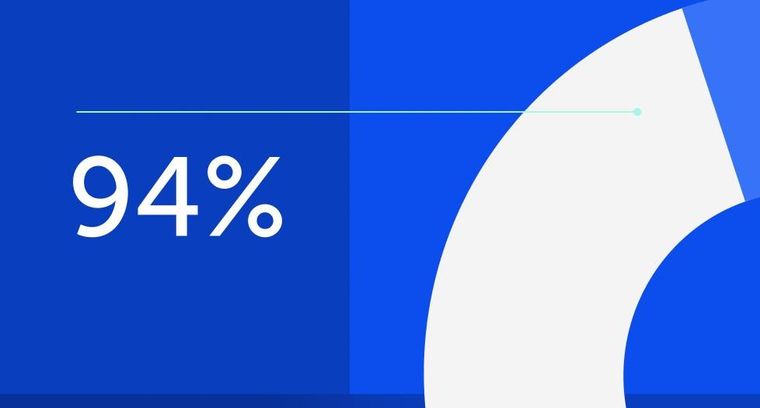
94% of researchers rate our articles as excellent or good
Learn more about the work of our research integrity team to safeguard the quality of each article we publish.
Find out more
REVIEW article
Front. Aging Neurosci., 16 November 2021
Sec. Cellular and Molecular Mechanisms of Brain-aging
Volume 13 - 2021 | https://doi.org/10.3389/fnagi.2021.768156
This article is part of the Research TopicRole of Glial Cells of the Central and Peripheral Nervous System in the Pathogenesis of Neurodegenerative DisordersView all 16 articles
Parkinson’s disease (PD) is one of the prevalent neurodegenerative diseases associated with preferential loss of dopaminergic (DA) neurons in the substantia nigra compacta (SNc) and accumulation of α-synuclein in DA neurons. Even though the precise pathogenesis of PD is not clear, a large number of studies have shown that microglia-mediated neuroinflammation plays a vital role in the process of PD development. G protein-coupled receptors (GPCRs) are widely expressed in microglia and several of them act as regulators of microglial activation upon corresponding ligands stimulations. Upon α-synuclein insults, microglia would become excessively activated through some innate immune receptors. Presently, as lack of ideal drugs for treating PD, certain GPCR which is highly expressed in microglia of PD brain and mediates neuroinflammation effectively could be a prospective source for PD therapeutic intervention. Here, six kinds of GPCRs and two types of innate immune receptors were introduced, containing adenosine receptors, purinergic receptors, metabotropic glutamate receptors, adrenergic receptors, cannabinoid receptors, and melatonin receptors and their roles in neuroinflammation; we highlighted the relationship between these six GPCRs and microglial activation in PD. Based on the existing findings, we tried to expound the implication of microglial GPCRs-regulated neuroinflammation to the pathophysiology of PD and their potential to become a new expectation for clinical therapeutics.
Microglia are the main resident macrophages in the central nervous system (CNS), which originate from the yolk sac and merge into CNS at the early stage development (McGeer et al., 1988; Colonna and Butovsky, 2017; Prinz et al., 2019). Under physiological conditions, microglia make great contributions to the CNS homeostasis; however, in face of inflammatory stimuli, microglia rapidly transform their status into activated (Colonna and Butovsky, 2017; Wolf et al., 2017; Li and Barres, 2018). The link between microglia and PD was first reported 33 years ago and they found a mass of reactive microglia in PD brain (McGeer et al., 1988). Whereafter, a number of studies indicated that microglia-regulated neuroinflammation plays vital roles in PD pathogenesis (Jankovic, 2008; De Virgilio et al., 2016). Reactive microglia could be simply classified into two categories, including M1 phenotype and M2 phenotype (Ransohoff, 2016; Tang and Le, 2016), although this classification is not precise. M1 phenotype makes microglia detrimental with exaggerated pro-inflammatory factors, while M2 phenotype is neuroprotective and produces anti-inflammatory mediators like Arg1, IL-10, and CD206 (Jha et al., 2016; Tang and Le, 2016). In PD brain, excessive pro-inflammatory like interleukin-1β (IL-1β) and tumor necrosis factor-α (TNF-α) lead to severe damage to DA neurons (Sawada et al., 2006). Damaged DA neurons in turn stimulate microglial activation, resulting in a positive feedback loop of microglial activation and DA neuronal death (Glass et al., 2010). Although it is still undetermined whether neuroinflammation is the cause of PD pathogenesis or a downstream response of neuronal damage, some studies reported that inhibiting microglial action or clear activated microglia is neuroprotective and could relieve some motor symptoms in PD animal models (Gu et al., 2018), suggesting targeting neuroinflammation might show new lights on the research of PD pathogenesis.
Mammalian β-adrenergic receptor, as the first G protein-coupled receptor (GPCR), was identified in 1986 (Dixon et al., 1986). Nearly 800 members GPCRs are found and become the largest superfamily of cell surface receptors in mammals (Fredriksson et al., 2003; Nieto Gutierrez and McDonald, 2018). Almost 90% of the GPCRs can be bound by a wide range of neurotransmitters and neuromodulators, like norepinephrine, dopamine, and serotonin in brain (Doze and Perez, 2012). It is well documented that some microglial innate immune receptors, including Toll-like receptors (TLRs) and NOD-like receptors (NLRs) regulate microglial activation through the recognition of their ligands (Lan et al., 2017; Salvi et al., 2017; Ifuku et al., 2020; Ma et al., 2020). Recently, it has been reported that microglial TLR4 could mediate neuron-released α-synuclein clearance through selective autophagy (Choi et al., 2020). Interestingly, in the PD animal model, α-synuclein accumulation in microglia has been demonstrated to induce its activation by binding with several microglial innate immune receptors, such as CXCR4 (Li Y. et al., 2019). Numbers of endogenous or exogenous stimuli and perturbation could act on GPCRs, changing a wide variety of cell signaling, affecting the pathogenesis of many neurodegenerative disorders, including PD (Guixa-Gonzalez et al., 2012; Gandía et al., 2013; Azam et al., 2020). Moreover, G protein-coupled receptor kinases (GRKs) can act as the regulators of dopamine receptor functions, further influencing PD development (Gurevich et al., 2016). Currently, GPCRs have already been the targets of 34% Food and Drug Administration (FDA)-approved drugs available and some GPCRs have potential for clinical treatment in neurodegenerative disorders (Lütjens and Rocher, 2017; Hauser et al., 2018). Whether GPCRs also play roles in the regulation of microglia activation is worth further study.
In this review, we attempted to assess the relationship between GPCRs and neuroinflammation and the mechanisms underlying this phenomenon, as well as its importance in PD. We would like to discuss the role of GPCRs in microglial activation and we will focus on adenosine receptors, purinergic receptors, metabotropic glutamate receptors, adrenergic receptors, cannabinoid receptors, and melatonin receptors. We intended to focus on the therapeutic perspective of GPCRs as emerging drug targets for the development of novel therapeutic agents to PD treatment.
Adenosine, as a neurotransmitter, is widely expressed in various cell types of CNS, including glial cells and neurons (Liu et al., 2019). Adenosine exerts physiological effects through four subtypes of adenosine receptors: A1, A2A, A2B, and A3 receptors, which belong to the purinergic GPCR family (Peleli et al., 2017). It is well known that adenosine acts as a vital role in neuronal excitability and synaptic transmission (Boison and Aronica, 2015); however, the adenosine-mediated role in glial function remains unclear. The dysfunction of adenosine receptors is reported to be closely related to PD pathogenesis (Cunha, 2016). Caffeine, as an adenosine antagonist, is neuroprotective against PD (Brothers et al., 2010; Bagga et al., 2016). In the MPTP-induced mouse model of PD, adenosine deaminase inhibition remarkably ameliorated DA neuronal death and improved motor disabilities (Huang et al., 2019).
A1 receptors are highly expressed in the cortex and hippocampus (Soliman et al., 2018). Even though there is no significant change of A1 receptors in the early stages of PD detected by 11C-MPDX PET (Mishina et al., 2017), an obvious increase of A1 receptors in the cerebellum was detected in virus-infected encephalitis rat model (Paul et al., 2014), suggesting the possible role of A1 receptors in some inflammatory-mediated pathological conditions. A1 receptors activation in microglia could suppress glioblastoma growth acting (Synowitz et al., 2006). Stimulation of A1 receptors inhibits microglial morphological activation through suppressing ATP-induced Ca2+ influx (Luongo et al., 2014), indicating the possible beneficial role of A1 receptors in microglia-mediated neuroinflammation. One study showed that paeoniflorin inhibits neuroinflammation via activating microglial A1 receptors in the MPTP mouse model (Liu et al., 2006), implying the protective role of A1 receptors in PD. Unlike A1 receptors, more research based on the relationship between A2A receptors and microglial activation was reported. In MPTP-treated mice, there is a significant increase in A2A receptor expression both in the substantia nigra (SN) and in the striatum (Gyoneva et al., 2014). Moreover, In lipopolysaccharide (LPS)-stimulated primary microglia, increased A2A receptors expression was also detected (Orr et al., 2009). Also, A2A receptors inhibition could suppress microglia activation in different neurodegenerative disorders (Gyoneva et al., 2014; Madeira et al., 2016), while A2A receptor activation could counteract the anti-inflammatory effects mediated by dopamine and further strengthen neuroinflammation (Meng et al., 2019). The combination of adenosine A1A receptor agonist and adenosine A2A receptor antagonist has a stronger inhibitory effect on neuroinflammation; among them, A2A antagonists have been used in clinical evaluation for the treatment of PD (Marucci et al., 2021). Interestingly, CD73-derived adenosine was reported to regulate inflammation and neurodegeneration (Meng et al., 2019).
Even though there is no definitive research of A2B and A3 receptors in PD pathogenesis, in LPS-treated microglia, A2B receptors activation has been reported to aggravate pro-inflammatory factors production through phosphorylation of cAMP response element binding (CREB) and promoting the p38 mitogen-activated protein kinase (MAPK) pathway (Koscsó et al., 2012; Merighi et al., 2017). The role of A3 receptor in microglia has also been reported. Activation of the microglial A3 receptors by adenosine or Cl-IB-MECA, a selective adenosine A3 receptor agonist, is capable of inhibiting TNF-α production through suppressing Akt and NF-κB activation in BV-2 microglia cells, while MRS1523, a selective A3 receptor antagonist, reverses this neuroprotective effect (Hammarberg et al., 2003; Lee et al., 2006).
Purinergic receptors are categorized into two kinds of receptor families, containing ATP-gated ion channels (P2X) and GPCRs (P2Y) (Burnstock, 2017). P2X receptors are further subdivided into seven subtypes: P2X1, P2X2, P2X3, P2X4, P2X5, P2X6, and P2X7 receptors. GPCRs (P2Y) receptors are further subdivided into eight subtypes, including five Gq-coupled receptors: P2Y1, P2Y2, P2Y4, P2Y6, and P2Y11 and three Gi-coupled receptors: P2Y12, P2Y13, and P2Y14 (Burnstock, 2017). It is well reported that purinergic signaling is involved in the pathophysiology of CNS, including PD (Puchałowicz et al., 2014; Tóth et al., 2019). A recent study showed that purinergic receptor modulation could reverse aberrant Ca2+ signaling, might appear novel therapeutic potential for HD and PD (Glaser et al., 2020). P2X7 receptors are preferentially expressed on microglia compared to that on astrocytes and oligodendrocytes (Illes, 2020). P2X7 receptors are the amplifier of CNS damage in neurodegenerative diseases (Illes, 2020). In LPS-primed microglia, extracellular ATP generated from damaged tissue and astrocytes could bind to P2X7 receptors, leading to the inflammasome assembly and the releases of IL-1β and IL-18, which further make neurons damage (Heneka et al., 2018). In addition, P2X7 receptors in DA neurons are responsible for α-synuclein-induced oxidative stress and mitophagy impairment (Wilkaniec et al., 2020), implying its damage exacerbation in PD. P2X7 receptor antagonist can inhibit neuroinflammation and may be a potential drug target for the treatment of movement disorders (Fonteles et al., 2020), such as PD. Besides P2X7, P2X4 in the P2X receptor family is reported to regulate microglial function (Suurväli et al., 2017). In the experimental autoimmune encephalomyelitis (EAE) model, P2X4 receptors signaling inhibition or P2X4 knockout increases pro-inflammatory gene expression in microglia (Zabala et al., 2018). Moreover, P2X4 receptor protein is increased in the SN of the PD rat model and P2X4 receptor activation is involved in DA neuronal autophagy inhibition (Zhang X. et al., 2021), indicating that P2X4 receptor might serve as a potential way for the treatment of PD.
P2Y receptors, as G-coupled receptors are also well reported to tend to regulate microglial function (Von Kügelgen and Hoffmann, 2016). One study indicated that P2Y1 receptors might be the modulator of microglia–astrocyte interaction. It showed that microglia-derived cytokines could act on astrocytes, resulting in the downregulation of P2Y1 receptor and exerting neuroprotective effects (Shinozaki et al., 2017). Exogenous ATP triggered P2Y1 receptors activation, resulting in Ca2+ release from intracellular stores, making microglia to be pro-inflammatory (Orellana et al., 2013). In addition, both P2Y1 and P2Y12 are involved in ADP-induced migration of microglia mediated by transforming growth factor (TGF-β) (De Simone et al., 2010). There are very few studies about P2Y(2/4) receptors in neuroinflammation. Both P2Y2 and P2Y4 receptors are linked to amyloid beta (Aβ)-induced self-uptake by microglial pinocytosis, showing their more important role in AD (Kim et al., 2012; Li et al., 2013).
It seems that P2Y6 receptors play a more significant role than other P2Y receptors in PD.
P2Y6 receptors were markedly upregulated both in LPS-induced microglia and in the peripheral blood mononuclear cells (PBMCs) of PD patients (Yang et al., 2017). UDP, as the ligand of P2Y6, could promote microglial activation via the ERK1/2 pathway (Yang et al., 2017). Blocking UDP/P2Y6 receptor signaling could reverse these PD pathological processes (Yang et al., 2017; Anwar et al., 2020). It is also reported that a combination of P2Y6 and P2X7 receptor antagonists can be more protective in the 6-OHDA-induced PD rat model (Oliveira-Giacomelli et al., 2019). Moreover, P2Y6 receptors contribute to MPTP-induced neuronal SHSY5Y cell death (Qian et al., 2018). Therefore, P2Y6 receptor might be a potential clinical biomarker of PD. The role of P2Y12 and P2Y13 mediating microglial function has also been reported (Haynes et al., 2006; Zeng et al., 2014; Tatsumi et al., 2015). During neuropathic pain, P2Y12 receptor-dependent GTP-RhoA/ROCK2 signaling pathway upregulate excitatory synaptic transmission and microglial activation (Yu et al., 2019). A very recent study indicated that IL-1β release is increased remarkably in the P2Y13 knockout microglia while release evoked by LPS and ATP was not affected (Kyrargyri et al., 2020).
It has been well reported that there is abundant glutamate in the CNS. Glutamate is an excitatory neurotransmitter maintaining communication and balance between glial cells and neurons (Ribeiro et al., 2017). Glutamate receptors are categorized into two types, namely, metabotropic glutamate receptors (mGluRs) and ionotropic glutamate receptors. mGluRs are further divided into three subgroups: Group I including mGlu1 and mGlu5 receptors; Group II including mGlu2 and mGlu3 receptors; and Group III consists of mGlu4, mGlu6, mGlu7, and mGlu8 receptors on the basis of sequence homology (Perfilova and Tyurenkov, 2016). mGluRs have been reported to be the therapeutic targets in PD (Litim et al., 2017). Among these mGluRs, Group III mGluRs activation could suppress glutamate release from presynaptic terminals of microglia, preventing neurons from excitotoxicity (Williams and Dexter, 2014). mGlu5 receptor seems to be closely linked to neuroinflammation. mGlu5 receptor is a potential regulator of microglial function and was first found in glial cells in 1999 (Biber et al., 1999). One study reported that microvesicles (MVs) released from microglia BV2 cells contribute to the communication between microglia and neurons and this interaction was mediated by mGlu5 receptor, resulting in the increased rotenone-induced neurotoxicity (Beneventano et al., 2017). This finding is really interesting. In the inflammatory-induced PD mouse model, the traditional Chinese medicine triptolide inhibits microglial activation via increasing mGlu5 receptor expression (Huang et al., 2018). Moreover, in the recent study of this group, they found that mGlu5 agonists inhibit α-synuclein-mediated neuroinflammation by regulating the binding of mGlu5 to α-synuclein (Zhang Y.N. et al., 2021), further indicating the significant role of mGlu5 in PD. Importantly, in mGlu5 receptor knockout (mGluR5–/–) mice, the increasing number of both microglia and astrocytes was found (Carvalho et al., 2019), implying that mGlu5 is involved in neuroinflammation.
Adrenergic receptors are divided into two groups, including α and β adrenergic receptors, and further subdivided into several kinds of subtypes, containing α1, α2 (subtypes α2A, α2B, and α2C), β1, β2, and β3 (Strosberg, 1993). These adrenergic receptors exert physiological effects mainly through their ligand binding named noradrenaline (Strosberg, 1993). Dysfunction of the locus coeruleus noradrenergic system has been reported to be closely related to PD progression (O’Neill and Harkin, 2018; Sommerauer et al., 2018). Noradrenergic impairment contributes to the high prevalence of the non-motor symptoms in PD (O’Neill and Harkin, 2018). There are a few studies on the relationship between α-adrenergic receptors and microglial function. One study reported that dexmedetomidine, as a selective α2-adrenoceptor agonist, suppresses LPS-induced release of pro-inflammatory cytokines from hippocampal microglia specifically through α2-adrenergic receptor activation (Yamanaka et al., 2017). In another research, dexmedetomidine could regulate microglial polarization induced by 6-OHDA. Upon the pretreatment of dexmedetomidine, the inhibitory effects of 6-OHDA on IL-4-mediated induction of the anti-inflammatory factors, like IL-10 and IL-13, were remarkably alleviated, while the release of pro-inflammatory factors, such as IL-6 and IL-1β, were inhibited (Zhang et al., 2017).
Rather than α-adrenergic receptors, β-adrenergic receptors, especially β2-adrenergic receptors seem to be more closely linked to neuroinflammation in PD pathogenesis. The relationship between microglia and β-adrenergic receptors was first reported in 1988 (Fujita et al., 1998). They found that a β2-selective agonist terbutaline but not a β1-selective agonist restrains the microglial proliferation via enhancing intracellular cAMP level (Fujita et al., 1998). A number of studies reported that β2-adrenergic receptors inhibit microglia-mediated neuroinflammation via multiple signaling pathways to protect DA neurons from damage (Qian et al., 2009, 2011; Peterson et al., 2014; O’Neill et al., 2020). One of the studies showed that transformation from an M1- to M2-like phenotype in LPS-activated microglia by β2-adrenergic receptors agonists involves activation of the classical cAMP/PKA/CREB as well as the PI3K and p38 MAPK signaling pathways (Sharma et al., 2019). Another study reported that low doses of salmeterol inhibit microglial activation through a β2AR/β-arrestin2-dependent but cAMP/protein kinase A-independent pathway (Qian et al., 2011). A recent study indicated that β2-adrenergic receptors activation using different agonists dramatically alleviates the progression of dopaminergic neurodegeneration via inhibiting microglial activation in the LPS-challenged inflammatory PD mouse model (O’Neill et al., 2020).
Cannabinoid (CB) receptors as classical G-protein coupled receptors are widely expressed throughout CNS, as well as certain parts of the body. Cannabinoid receptors mainly contain two subtypes, namely, CB1 and CB2 receptors (Zou and Kumar, 2018). A recent study showed that GPR55, an orphan receptor that can be activated by many classical cannabinoids, has been added as a third cannabinoid receptor (Moriconi et al., 2010). It is well documented that endocannabinoid as the endogenous ligand of cannabinoid receptors mediates large numbers of physiological effects through ligand-receptor binding (Zou and Kumar, 2018). Both Gi and Gs proteins were regulated by cannabinoid receptors activation (Howlett et al., 1986; Glass and Felder, 1997). A mass of studies regard cannabinoid receptors as the potential therapeutic target for PD (Baul et al., 2019; Cristino et al., 2020). CB receptors are equipped to play protective roles in different cellular stress in PD. Here, we focused on the roles of microglial CB1 and CB2 receptors, as well as GPR55 in neuroinflammation.
In MPTP-induced PD mouse model, pretreatment with non-selective CB receptors agonist WIN55212-2 or HU210 inhibited NADPH oxidase reactive oxygen species (ROS) production and reduced pro-inflammatory cytokines production from activated microglia, leading to increased DA neurons survival in the SN and improved the mouse motor function (Chung et al., 2011). The anti-inflammatory and neuroprotective effects were reversed upon treatment with CB1 receptor selective antagonist AM251 or SR14716A, confirming the importance of the CB1 receptor (Chung et al., 2011). It has been reported that the treatment of selective CB1 receptor antagonist SR141716A significantly increases pro-inflammatory factors (TNF-α, IL-1β, and IL-6) and chemokines (MCP-1 and CX3CL1) production in BV2 microglial cells through upregulating TLR4 and NF-κB/p65 expressions, further accelerating the clinical onset and development of EAE (Lou et al., 2018). However, one study showed that the anti-inflammatory effects of CB1 receptor might not be such important. In CB1 receptor knockout (Cnr1–/–) mice, less noradrenergic neurons in the locus coeruleus were found compared to their age-matched wild-type controls. Nevertheless, there was no enhanced pro-inflammatory profile in Cnr1–/– mice even the density of microglia was increased (Gargano et al., 2020). Very interestingly, CB1 receptor was found to be localized on neuronal mitochondria, the G protein-coupled mitochondrial CB1 (mtCB1) receptors modulate memory processes through regulating mitochondrial energy metabolism (Hebert-Chatelain et al., 2016). This effect was mediated by mtCB1/mtGαi/PKA-dependent phosphorylation of complex I subunit NDUFS2 (Hebert-Chatelain et al., 2016). In their recent research, mtCB1 was also found in astrocytes, regulating glucose metabolism via phosphorylation of the mitochondrial complex I subunit NDUFS4 (Jimenez-Blasco et al., 2020). These two studies are indeed striking, as regulation of mitochondrial energy metabolism on microglial activation is currently a research hotspot. Whether microglial mtCB1 exists, if exists, will microglial mtCB1 modulate microglial activation through regulating mitochondrial energy metabolism mediated by certain molecules? This is really worthy of further study.
More research about CB2 receptors has been reported than CB1 receptors on microglia. One study reported that CB1 receptor gene expression was unchanged, while CB2Ar (A isoform, CB2Ar) gene expression was significantly increased (fourfold) in the SN of patients with PD (Navarrete et al., 2018). Another study also found the upregulation of CB2 receptors in microglia of SN in postmortem tissues of PD patients (Gómez-Gálvez et al., 2016). Moreover, in the rotenone or 6-OHDA-induced PD rat model, obvious upregulation of CB2 receptors expression was detected (Concannon et al., 2015, 2016). Interestingly, in the inflammatory rat PD model induced by Poly (I:C) or LPS, a more remarkable CB2 receptors increase was found (Concannon et al., 2015, 2016), implying that CB2 receptors were more influenced by inflammatory stimulation. These studies indicated targeting the CB2 receptor might represent a viable way for neuroinflammation modification in PD. CB2 receptor activation has been reported to inhibit neurotoxin-mediated neuroinflammation through regulating multiple molecules, including NRF2, ERK1/2, cPLA2, and NF-κB (Ribeiro et al., 2013; Galán-Ganga et al., 2020). Importantly, a much more intense deterioration of tyrosine hydroxylase (TH)-containing nigral neurons in CB2 receptor-deficient mice compared to wild-type animals (Gómez-Gálvez et al., 2016), indicating a potential neuroprotective role for CB2 receptor. The role of microglial GPR55 has also been reported recently. They found that KIT77, as an inverse agonist on GPR55 independent of the endocannabinoid system, significantly inhibited the release of PGE2 in primary microglia partially relying on the reduction of protein synthesis of mPGES-1 and COX-2 (Saliba et al., 2018). Recently, as the crystal structure of CB1 and CB2 receptors was analyzed (Krishna Kumar et al., 2019; Li X. et al., 2019), it becomes the guiding light for further research on CB receptors and really contributes to the drug discovery targeting CB receptors in PD.
Melatonin is an amine hormone secreted mainly through the pineal gland in mammals (Hardeland et al., 2011). Circadian rhythm modulation and anti-oxidation effects have been thought to be the central physiological functions of melatonin (Reiter et al., 2000; Sanchez et al., 2015). G-protein coupled receptors (GPCR), namely, MT1 (encoded by MTNR1A) and MT2 (MTNR1B) regulate the majority of the biological functions of melatonin (Zlotos et al., 2014). Both MT1 and MT2 receptors are widely expressed in brain and play important roles in neurodegenerative diseases (Liu et al., 2016). It has been reported that MT1 receptors are reduced in AD patients (Wu et al., 2007). Silence of MTNR1A increases the amyloidogenic processing of amyloid precursor protein (APP) and exacerbates mutant huntingtin-mediated toxicity (Wang et al., 2011; Sulkava et al., 2018), indicating that MT1 plays a protective role in neurons. MT2 receptor is also closed related to AD pathogenesis. There was impaired hippocampal MT2 receptor signaling and MT2 activation ameliorated dendritic abnormalities through the cAMP-C/EBPα/miR-125b/GluN2A signaling pathway in AD (Tang et al., 2019). However, the role of MT receptors in PD pathogenesis.
It is well known that the relationship between melatonin and PD is mainly dependent on the anti-inflammatory and antioxidant effects. The majority of these neuroprotective effects on DA neurons in PD is receptor-independent and relies on antioxidant properties of the melatonin drug structure (Reiter et al., 2000, 2016; Pandi-Perumal et al., 2013). The role of MT1 and MT2 receptor in regulating PD pathogenesis, especially neuroinflammation remains unclear. Importantly, one study reported that there is a significant reduction of both MT1 and MT2 receptors in amygdaloid nucleus and SN of PD patients (Adi et al., 2010), possibly implying the significance of MT receptor in PD progression. Sleep disorder caused by circadian rhythm dysfunction is regarded as one of the vital non-motor symptoms of PD and often occurs in the early stage of PD progression (Fifel, 2017). Some researchers agree that dysfunction of circadian rhythm might be involved in PD pathogenesis (Lauretti et al., 2017). Both MT1 and MT2 receptors play vital roles in the regulation of circadian rhythm (Pandi-Perumal et al., 2007; Comai and Gobbi, 2014). Dysfunction of MT1 or MT2 might have the potential to participate in PD progression. The role in microglial MT1 on neuroinflammation is poorly understood. Our latest study indicated that microglial MT1 regulates microglial activation through PDHA1-mediated enhancement of oxidative phosphorylation (Gu et al., 2021). Others also reported that non-selective agonists such as ramelteon or agomelatine exert powerful anti-inflammatory effects (Molteni et al., 2013; Wang et al., 2019). Agomelatine, as a novel antidepressant, inhibits LPS-induced upregulation of pro-inflammatory factors via suppressing NF-κB nuclear translocation (Molteni et al., 2013). Ramelteon, which is used for treating insomnia clinically, protects neuronal degeneration in traumatic brain injury (TBI) through promoting nuclear factor erythroid 2-related factor 2 (Nrf2) nuclear accumulation, leading to the increasing of downstream factors of NRF2, including SOD-1, heme oxygenase-1, and NQO1 (Wang et al., 2019). It is very interesting that MT1 receptor is also located on neuronal mitochondria, like CB1 receptor. They found that melatonin is also synthesized in the mitochondrial matrix and released by the organelle to activate the mitochondrial MT1 signal-transduction pathway, thus inhibiting stress-mediated cytochrome c release and caspase activation, protecting neurons from death (Suofu et al., 2017). As microglial MT1 is closely linked to mitochondrial metabolic programming, there is the possibility that microglial mitochondrial MT1 exists and regulates mitochondrial-mediated microglial activation.
Except for the GPCRs mentioned above, innate immune receptors, also named pattern recognition receptors (PRRs), were widely expressed in innate immune cells, including microglia (De Nardo, 2015; Rodríguez-Gómez et al., 2020). It is well known that innate immune receptors are critical for modulating inflammatory responses (Rodríguez-Gómez et al., 2020). In regard to neurodegenerative diseases, TLRs and NOD-like receptors (NLRs), as best-characterized PRRs, play vital roles in neuroinflammation (Block et al., 2007; Kigerl et al., 2014; Kumar, 2019). Upon the binding of engaging ligands, microglial TLRs are activated, further causing internalization and conformational changes, leading to the recruitment of the adaptor proteins MyD88 or TRIF (TIR-domain-containing adaptor-inducing interferon-β) (De Nardo, 2015). Some transcription factors, including nuclear factor κB (NF-κB) are activated by downstream signaling cascades, resulting in subsequent production of pro-inflammatory cytokines (De Nardo, 2015). The relationship between TLRs and neuroinflammation is well clarified. For the pathogenesis of PD, TLRs are closely associated with α-synuclein. Misfolded or fibrillar α-synuclein released from neurons could be bound by the microglial TLR1/2 heterodimer, triggering NF-κB-mediated TNFα production (Kim et al., 2013). Another study also showed that fibrillar α-synuclein, but not oligomeric α-synuclein could promote IL-18 upregulation via a TLR1/2-dependent manner (Gustot et al., 2015). Microglial TLR4 also plays a significant role in α-synuclein-induced microglial activation, as α-synuclein could be uptake through TLR4, leading to pro-inflammatory cytokine release, and reactive oxygen species (ROS) production (Fellner et al., 2013). Furthermore, in the MPTP mouse PD model, TLR4 deficiency was neuroprotective (Noelker et al., 2013), indicating a detrimental role for TLR4 in PD pathogenesis.
Besides TLRs, NLRs mediate the process of the inactive IL-1β and IL-18 into active forms through inflammasomes activation, triggering pro-inflammatory responses (Latz et al., 2013). The NLR protein 3 (NLRP3) is the best-characterized inflammasome in PD, as in NLRP3-deficient mice, it was protective from MPTP-induced loss of DA neurons with decreased MPTP-induced caspase-1 activation and IL-1β release (Yan et al., 2015). There is extensive evidence that fibrillary α-synuclein, ATP, highmobility group box protein 1 (HMGB1), and lysophosphatidylcholine (LPC) could act as the activator of NLRs (Davalos et al., 2005; Freeman et al., 2017). Upon fibrillar α-synuclein insults, increased NLRP3 mRNA and protein expression were detected (Gustot et al., 2015; Zhou et al., 2016). This study suggested the key role of NLRP3 in PD pathogenesis.
In our present review, we mainly summarize six types of GPCRs and two types of innate immune receptors that are possibly related to disease progression of PD and their roles in mediating microglial action (Table 1). Some of these receptors, which consist of A1, A3, P2X4, P2Y13, Group III mGluRs, mGlu5, α2, β2, CB1, CB2, MT1, and MT2, act as beneficial roles and inhibit microglial activation, while A2A, A2B, P2X7, P2Y1, P2Y12, P2Y6, TLR1/2/4, and NLRP3 play unfavorable roles and are capable of inducing neuroinflammation (Figure 1). Drugs that target multiple receptors to inhibit microglial activation show considerable prospects in clinical therapeutic potential in PD. Very strikingly, CB1 and MT1 receptors were both found on mitochondrial outer membrane in neuron (Hebert-Chatelain et al., 2016; Suofu et al., 2017). Besides, CB1 receptor was also located on astrocytic mitochondria (Jimenez-Blasco et al., 2020). The possibility of microglial mitochondria is worthy to explore.
Figure 1. The summary of reported microglial GPCRs in microglial activation of PD. GPCRs expressed in microglia containing A2A, A2B, P2X7, P2Y1, P2Y12, P2Y6, TLR1/2/4, and NLRP3 act as detrimental roles, promoting microglial activation, damaging DA neurons, while microglial A1, A3, P2X4, P2Y13, mGlu5, α2, β2, CB1, CB2, MT1, and MT2 play protective roles in neuroinflammation.
In our opinion, as mitochondrial metabolic dysfunction is not only related to DA neuronal death directly but also closely involved in microglial activation, moreover, both crystal structures of MT1 and CB1 were analyzed recently, we believe that agonists that bind to both MT1 and CB1 receptors have great potential for the development of clinical treatment of PD drugs.
CG and YJC wrote the manuscript. YC, ZZ, C-FL, and MW edited and revised the manuscript. All authors read and approved the final manuscript.
This work was supported by the National Natural Science Foundation of China (81501181 and 82071420), Key Research and Development Program of Jiangsu Province (BE2018658 and BE2018668), and Jiangsu Provincial Medical Key Discipline Project (ZDXKB2016022).
The authors declare that the research was conducted in the absence of any commercial or financial relationships that could be construed as a potential conflict of interest.
All claims expressed in this article are solely those of the authors and do not necessarily represent those of their affiliated organizations, or those of the publisher, the editors and the reviewers. Any product that may be evaluated in this article, or claim that may be made by its manufacturer, is not guaranteed or endorsed by the publisher.
Adi, N., Mash, D. C., Ali, Y., Singer, C., Shehadeh, L., and Papapetropoulos, S. (2010). Melatonin MT1 and MT2 receptor expression in Parkinson’s disease. Med. Sci. Monit. 16, BR61–BR67.
Anwar, S., Pons, V., and Rivest, S. (2020). Microglia purinoceptor P2Y6: an emerging therapeutic target in CNS diseases. Cells 9:1595. doi: 10.3390/cells9071595
Azam, S., Haque, M. E., Jakaria, M., Jo, S.-H., Kim, I.-S., and Choi, D.-K. (2020). G-Protein-coupled receptors in CNS: a potential therapeutic target for intervention in neurodegenerative disorders and associated cognitive deficits. Cells 9:506. doi: 10.3390/cells9020506
Bagga, P., Chugani, A. N., and Patel, A. B. (2016). Neuroprotective effects of caffeine in MPTP model of Parkinson’s disease: a (13)C NMR study. Neurochem. Int. 92, 25–34. doi: 10.1016/j.neuint.2015.11.006
Baul, H. S., Manikandan, C., and Sen, D. (2019). Cannabinoid receptor as a potential therapeutic target for Parkinson’s Disease. Brain Res. Bull. 146, 244–252. doi: 10.1016/j.brainresbull.2019.01.016
Beneventano, M., Spampinato, S. F., Merlo, S., Chisari, M., Platania, P., Ragusa, M., et al. (2017). Shedding of microvesicles from microglia contributes to the effects induced by metabotropic glutamate receptor 5 activation on neuronal death. Front. Pharmacol. 8:812. doi: 10.3389/fphar.2017.00812
Biber, K., Laurie, D. J., Berthele, A., Sommer, B., Tölle, T. R., Gebicke-Härter, P. J., et al. (1999). Expression and signaling of group I metabotropic glutamate receptors in astrocytes and microglia. J. Neurochem. 72, 1671–1680.
Block, M. L., Zecca, L., and Hong, J.-S. (2007). Microglia-mediated neurotoxicity: uncovering the molecular mechanisms. Nat. Rev. Neurosci. 8, 57–69.
Boison, D., and Aronica, E. (2015). Comorbidities in neurology: is adenosine the common link? Neuropharmacology 97, 18–34. doi: 10.1016/j.neuropharm.2015.04.031
Brothers, H. M., Marchalant, Y., and Wenk, G. L. (2010). Caffeine attenuates lipopolysaccharide-induced neuroinflammation. Neurosci. Lett. 480, 97–100. doi: 10.1016/j.neulet.2010.06.013
Burnstock, G. (2017). Purinergic signalling and neurological diseases: an update. CNS Neurol. Disord. Drug Targets 16, 257–265. doi: 10.2174/1871527315666160922104848
Carvalho, T. G., Alves-Silva, J., De Souza, J. M., Real, A. L. C. V., Doria, J. G., Vieira, E. L. M., et al. (2019). Metabotropic glutamate receptor 5 ablation accelerates age-related neurodegeneration and neuroinflammation. Neurochem. Int. 126, 218–228. doi: 10.1016/j.neuint.2019.03.020
Choi, I., Zhang, Y., Seegobin, S. P., Pruvost, M., Wang, Q., Purtell, K., et al. (2020). Microglia clear neuron-released α-synuclein via selective autophagy and prevent neurodegeneration. Nat. Commun. 11:1386. doi: 10.1038/s41467-020-15119-w
Chung, Y. C., Bok, E., Huh, S. H., Park, J.-Y., Yoon, S.-H., Kim, S. R., et al. (2011). Cannabinoid receptor type 1 protects nigrostriatal dopaminergic neurons against MPTP neurotoxicity by inhibiting microglial activation. J. Immunol. 187, 6508–6517. doi: 10.4049/jimmunol.1102435
Colonna, M., and Butovsky, O. (2017). Microglia function in the central nervous system during health and neurodegeneration. Annu. Rev. Immunol. 35, 441–468. doi: 10.1146/annurev-immunol-051116-052358
Comai, S., and Gobbi, G. (2014). Unveiling the role of melatonin MT2 receptors in sleep, anxiety and other neuropsychiatric diseases: a novel target in psychopharmacology. J. Psychiatry Neurosci. 39, 6–21. doi: 10.1503/jpn.130009
Concannon, R. M., Okine, B. N., Finn, D. P., and Dowd, E. (2015). Differential upregulation of the cannabinoid CB2 receptor in neurotoxic and inflammation-driven rat models of Parkinson’s disease. Exp. Neurol. 269, 133–141. doi: 10.1016/j.expneurol.2015.04.007
Concannon, R. M., Okine, B. N., Finn, D. P., and Dowd, E. (2016). Upregulation of the cannabinoid CB2 receptor in environmental and viral inflammation-driven rat models of Parkinson’s disease. Exp. Neurol. 283, 204–212. doi: 10.1016/j.expneurol.2016.06.014
Cristino, L., Bisogno, T., and Di Marzo, V. (2020). Cannabinoids and the expanded endocannabinoid system in neurological disorders. Nat. Rev. Neurol. 16, 9–29. doi: 10.1038/s41582-019-0284-z
Cunha, R. A. (2016). How does adenosine control neuronal dysfunction and neurodegeneration? J. Neurochem. 139, 1019–1055. doi: 10.1111/jnc.13724
Davalos, D., Grutzendler, J., Yang, G., Kim, J. V., Zuo, Y., Jung, S., et al. (2005). ATP mediates rapid microglial response to local brain injury in vivo. Nat. Neurosci. 8, 752–758.
De Nardo, D. (2015). Toll-like receptors: activation, signalling and transcriptional modulation. Cytokine 74, 181–189. doi: 10.1016/j.cyto.2015.02.025
De Simone, R., Niturad, C. E., De Nuccio, C., Ajmone-Cat, M. A., Visentin, S., and Minghetti, L. (2010). TGF-β and LPS modulate ADP-induced migration of microglial cells through P2Y1 and P2Y12 receptor expression. J. Neurochem. 115, 450–459. doi: 10.1111/j.1471-4159.2010.06937.x
De Virgilio, A., Greco, A., Fabbrini, G., Inghilleri, M., Rizzo, M. I., Gallo, A., et al. (2016). Parkinson’s disease: autoimmunity and neuroinflammation. Autoimmun. Rev. 15, 1005–1011. doi: 10.1016/j.autrev.2016.07.022
Dixon, R. A., Kobilka, B. K., Strader, D. J., Benovic, J. L., Dohlman, H. G., Frielle, T., et al. (1986). Cloning of the gene and cDNA for mammalian beta-adrenergic receptor and homology with rhodopsin. Nature 321, 75–79.
Doze, V. A., and Perez, D. M. (2012). G-protein-coupled receptors in adult neurogenesis. Pharmacol. Rev. 64, 645–675. doi: 10.1124/pr.111.004762
Fellner, L., Irschick, R., Schanda, K., Reindl, M., Klimaschewski, L., Poewe, W., et al. (2013). Toll-like receptor 4 is required for α-synuclein dependent activation of microglia and astroglia. Glia 61, 349–360. doi: 10.1002/glia.22437
Fifel, K. (2017). Alterations of the circadian system in Parkinson’s disease patients. Mov. Disord. 32, 682–692. doi: 10.1002/mds.26865
Fonteles, A. A., Neves, J. C. S., Menezes, A. P. F., Pereira, J. F., Silva, A. T. A., Cunha, R. A., et al. (2020). ATP signaling controlling dyskinesia through P2X7 receptors. Front. Mol. Neurosci. 13:111. doi: 10.3389/fnmol.2020.00111
Fredriksson, R., Lagerström, M. C., Lundin, L.-G., and Schiöth, H. B. (2003). The G-protein-coupled receptors in the human genome form five main families. Phylogenetic analysis, paralogon groups, and fingerprints. Mol. Pharmacol. 63, 1256–1272.
Freeman, L., Guo, H., David, C. N., Brickey, W. J., Jha, S., and Ting, J. P. Y. (2017). NLR members NLRC4 and NLRP3 mediate sterile inflammasome activation in microglia and astrocytes. J. Exp. Med. 214, 1351–1370. doi: 10.1084/jem.20150237
Fujita, H., Tanaka, J., Maeda, N., and Sakanaka, M. (1998). Adrenergic agonists suppress the proliferation of microglia through beta 2-adrenergic receptor. Neurosci. Lett. 242, 37–40.
Galán-Ganga, M., Del Río, R., Jiménez-Moreno, N., Díaz-Guerra, M., and Lastres-Becker, I. (2020). Cannabinoid CB Receptor Modulation by the Transcription Factor NRF2 is Specific in Microglial Cells. Cell. Mol. Neurobiol. 40, 167–177. doi: 10.1007/s10571-019-00719-y
Gandía, J., Fernández-Dueñas, V., Morató, X., Caltabiano, G., González-Muñiz, R., Pardo, L., et al. (2013). The Parkinson’s disease-associated GPR37 receptor-mediated cytotoxicity is controlled by its intracellular cysteine-rich domain. J. Neurochem. 125, 362–372. doi: 10.1111/jnc.12196
Gargano, A., Beins, E., Zimmer, A., and Bilkei-Gorzo, A. (2020). Lack of cannabinoid receptor type-1 leads to enhanced age-related neuronal loss in the locus coeruleus. Int. J. Mol. Sci. 22:5. doi: 10.3390/ijms22010005
Glaser, T., Andrejew, R., Oliveira-Giacomelli, Ribeiro, D. E., Bonfim Marques, L., Ye, Q., et al. (2020). Purinergic receptors in basal ganglia diseases: shared molecular mechanisms between huntington’s and Parkinson’s Disease. Neurosci. Bull. 36, 1299–1314. doi: 10.1007/s12264-020-00582-8
Glass, C. K., Saijo, K., Winner, B., Marchetto, M. C., and Gage, F. H. (2010). Mechanisms underlying inflammation in neurodegeneration. Cell 140, 918–934. doi: 10.1016/j.cell.2010.02.016
Glass, M., and Felder, C. C. (1997). Concurrent stimulation of cannabinoid CB1 and dopamine D2 receptors augments cAMP accumulation in striatal neurons: evidence for a Gs linkage to the CB1 receptor. J. Neurosci. 17, 5327–5333.
Gómez-Gálvez, Y., Palomo-Garo, C., Fernández-Ruiz, J., and García, C. (2016). Potential of the cannabinoid CB(2) receptor as a pharmacological target against inflammation in Parkinson’s disease. Prog. Neuropsychopharmacol. Biol. Psychiatry 64, 200–208. doi: 10.1016/j.pnpbp.2015.03.017
Gu, C., Hu, Q., Wu, J., Mu, C., Ren, H., Liu, C. F., et al. (2018). P7C3 inhibits LPS-induced microglial activation to protect dopaminergic neurons against inflammatory factor-induced cell death in vitro and in vivo. Front. Cell Neurosci. 12:400. doi: 10.3389/fncel.2018.00400
Gu, C., Wang, F., Zhang, Y.-T., Wei, S.-Z., Liu, J.-Y., Sun, H.-Y., et al. (2021). Microglial MT1 activation inhibits LPS-induced neuroinflammation via regulation of metabolic reprogramming. Aging Cell 20:e13375. doi: 10.1111/acel.13375
Guixa-Gonzalez, R., Bruno, A., Marti-Solano, M., and Selent, J. (2012). Crosstalk within GPCR heteromers in schizophrenia and Parkinson’s disease: physical or just functional? Curr. Med. Chem. 19, 1119–1134.
Gurevich, E. V., Gainetdinov, R. R., and Gurevich, V. V. (2016). G protein-coupled receptor kinases as regulators of dopamine receptor functions. Pharmacol. Res. 111, 1–6. doi: 10.1016/j.phrs.2016.05.010
Gustot, A., Gallea, J. I., Sarroukh, R., Celej, M. S., Ruysschaert, J.-M., and Raussens, V. (2015). Amyloid fibrils are the molecular trigger of inflammation in Parkinson’s disease. Biochem. J. 471, 323–333. doi: 10.1042/BJ20150617
Gyoneva, S., Shapiro, L., Lazo, C., Garnier-Amblard, E., Smith, Y., Miller, G. W., et al. (2014). Adenosine A2A receptor antagonism reverses inflammation-induced impairment of microglial process extension in a model of Parkinson’s disease. Neurobiol. Dis. 67, 191–202. doi: 10.1016/j.nbd.2014.03.004
Hammarberg, C., Schulte, G., and Fredholm, B. B. (2003). Evidence for functional adenosine A3 receptors in microglia cells. J. Neurochem. 86, 1051–1054.
Hardeland, R., Cardinali, D. P., Srinivasan, V., Spence, D. W., Brown, G. M., and Pandi-Perumal, S. R. (2011). Melatonin–a pleiotropic, orchestrating regulator molecule. Prog. Neurobiol. 93, 350–384. doi: 10.1016/j.pneurobio.2010.12.004
Hauser, A. S., Chavali, S., Masuho, I., Jahn, L. J., Martemyanov, K. A., Gloriam, D. E., et al. (2018). Pharmacogenomics of GPCR drug targets. Cell 172, 41–54.e19. doi: 10.1016/j.cell.2017.11.033
Haynes, S. E., Hollopeter, G., Yang, G., Kurpius, D., Dailey, M. E., Gan, W.-B., et al. (2006). The P2Y12 receptor regulates microglial activation by extracellular nucleotides. Nat. Neurosci. 9, 1512–1519.
Hebert-Chatelain, E., Desprez, T., Serrat, R., Bellocchio, L., Soria-Gomez, E., Busquets-Garcia, A., et al. (2016). A cannabinoid link between mitochondria and memory. Nature 539, 555–559. doi: 10.1038/nature20127
Heneka, M. T., Mcmanus, R. M., and Latz, E. (2018). Inflammasome signalling in brain function and neurodegenerative disease. Nat. Rev. Neurosci. 19, 610–621. doi: 10.1038/s41583-018-0055-7
Howlett, A. C., Qualy, J. M., and Khachatrian, L. L. (1986). Involvement of Gi in the inhibition of adenylate cyclase by cannabimimetic drugs. Mol. Pharmacol. 29, 307–313.
Huang, W., Xu, Y., Zhang, Y., Zhang, P., Zhang, Q., Zhang, Z., et al. (2019). Metabolomics-driven identification of adenosine deaminase as therapeutic target in a mouse model of Parkinson’s disease. J. Neurochem. 150, 282–295. doi: 10.1111/jnc.14774
Huang, Y.-Y., Zhang, Q., Zhang, J.-N., Zhang, Y.-N., Gu, L., Yang, H.-M., et al. (2018). Triptolide up-regulates metabotropic glutamate receptor 5 to inhibit microglia activation in the lipopolysaccharide-induced model of Parkinson’s disease. Brain behav. Immun. 71, 93–107. doi: 10.1016/j.bbi.2018.04.006
Ifuku, M., Hinkelmann, L., Kuhrt, L. D., Efe, I. E., Kumbol, V., Buonfiglioli, A., et al. (2020). Activation of Toll-like receptor 5 in microglia modulates their function and triggers neuronal injury. Acta Neuropathol. Commun. 8:159. doi: 10.1186/s40478-020-01031-3
Illes, P. (2020). P2X7 receptors amplify CNS damage in neurodegenerative diseases. Int. J. Mol. Sci. 21:5996. doi: 10.3390/ijms21175996
Jankovic, J. (2008). Parkinson’s disease: clinical features and diagnosis. J. Neurol. Neurosurg. Psychiatry 79, 368–376. doi: 10.1136/jnnp.2007.131045
Jha, M. K., Lee, W.-H., and Suk, K. (2016). Functional polarization of neuroglia: implications in neuroinflammation and neurological disorders. Biochem. Pharmacol. 103, 1–16. doi: 10.1016/j.bcp.2015.11.003
Jimenez-Blasco, D., Busquets-Garcia, A., Hebert-Chatelain, E., Serrat, R., Vicente-Gutierrez, C., Ioannidou, C., et al. (2020). Glucose metabolism links astroglial mitochondria to cannabinoid effects. Nature 583, 603–608. doi: 10.1038/s41586-020-2470-y
Kigerl, K. A., De Rivero Vaccari, J. P., Dietrich, W. D., Popovich, P. G., and Keane, R. W. (2014). Pattern recognition receptors and central nervous system repair. Exp. Neurol. 258, 5–16. doi: 10.1016/j.expneurol.2014.01.001
Kim, C., Ho, D.-H., Suk, J.-E., You, S., Michael, S., Kang, J., et al. (2013). Neuron-released oligomeric α-synuclein is an endogenous agonist of TLR2 for paracrine activation of microglia. Nat. Commun. 4:1562. doi: 10.1038/ncomms2534
Kim, H. J., Ajit, D., Peterson, T. S., Wang, Y., Camden, J. M., Gibson Wood, W., et al. (2012). Nucleotides released from Aβ1–42 -treated microglial cells increase cell migration and Aβ1–42 uptake through P2Y2 receptor activation. J. Neurochem. 121, 228–238. doi: 10.1111/j.1471-4159.2012.07700.x
Koscsó, B., Csóka, B., Selmeczy, Z., Himer, L., Pacher, P., Virág, L., et al. (2012). Adenosine augments IL-10 production by microglial cells through an A2B adenosine receptor-mediated process. J. Immunol. 188, 445–453. doi: 10.4049/jimmunol.1101224
Krishna Kumar, K., Shalev-Benami, M., Robertson, M. J., Hu, H., Banister, S. D., Hollingsworth, S. A., et al. (2019). Structure of a signaling cannabinoid receptor 1-G protein complex. Cell 176, 448–458.e12. doi: 10.1016/j.cell.2018.11.040
Kumar, V. (2019). Toll-like receptors in the pathogenesis of neuroinflammation. J. Neuroimmunol. 332, 16–30. doi: 10.1016/j.jneuroim.2019.03.012
Kyrargyri, V., Madry, C., Rifat, A., Arancibia-Carcamo, I. L., Jones, S. P., Chan, V. T. T., et al. (2020). P2Y receptors regulate microglial morphology, surveillance, and resting levels of interleukin 1β release. Glia 68, 328–344. doi: 10.1002/glia.23719
Lan, X., Han, X., Li, Q., Li, Q., Gao, Y., Cheng, T., et al. (2017). Pinocembrin protects hemorrhagic brain primarily by inhibiting toll-like receptor 4 and reducing M1 phenotype microglia. Brain Behav. Immun. 61, 326–339. doi: 10.1016/j.bbi.2016.12.012
Latz, E., Xiao, T. S., and Stutz, A. (2013). Activation and regulation of the inflammasomes. Nat. Rev. Immunol. 13, 397–411. doi: 10.1038/nri3452
Lauretti, E., Di Meco, A., Merali, S., and Pratico, D. (2017). Circadian rhythm dysfunction: a novel environmental risk factor for Parkinson’s disease. Mol. Psychiatry 22, 280–286. doi: 10.1038/mp.2016.47
Lee, J. Y., Jhun, B. S., Oh, Y. T., Lee, J. H., Choe, W., Baik, H. H., et al. (2006). Activation of adenosine A3 receptor suppresses lipopolysaccharide-induced TNF-alpha production through inhibition of PI 3-kinase/Akt and NF-kappaB activation in murine BV2 microglial cells. Neurosci. Lett. 396, 1–6.
Li, H.-Q., Chen, C., Dou, Y., Wu, H.-J., Liu, Y.-J., Lou, H.-F., et al. (2013). P2Y4 receptor-mediated pinocytosis contributes to amyloid beta-induced self-uptake by microglia. Mol. Cell. Biol. 33, 4282–4293. doi: 10.1128/MCB.00544-13
Li, Q., and Barres, B. A. (2018). Microglia and macrophages in brain homeostasis and disease. Nat. Rev. Immunol. 18, 225–242. doi: 10.1038/nri.2017.125
Li, X., Hua, T., Vemuri, K., Ho, J.-H., Wu, Y., Wu, L., et al. (2019). Crystal Structure of the human cannabinoid receptor CB2. Cell 176, 459–467.e13. doi: 10.1016/j.cell.2018.12.011
Li, Y., Niu, M., Zhao, A., Kang, W., Chen, Z., Luo, N., et al. (2019). CXCL12 is involved in α-synuclein-triggered neuroinflammation of Parkinson’s disease. J. Neuroinflamm. 16:263. doi: 10.1186/s12974-019-1646-6
Litim, N., Morissette, M., and Di Paolo, T. (2017). Metabotropic glutamate receptors as therapeutic targets in Parkinson’s disease: an update from the last 5 years of research. Neuropharmacology 115, 166–179. doi: 10.1016/j.neuropharm.2016.03.036
Liu, H.-Q., Zhang, W.-Y., Luo, X.-T., Ye, Y., and Zhu, X.-Z. (2006). Paeoniflorin attenuates neuroinflammation and dopaminergic neurodegeneration in the MPTP model of Parkinson’s disease by activation of adenosine A1 receptor. Br. J. Pharmacol. 148, 314–325.
Liu, J., Clough, S. J., Hutchinson, A. J., Adamah-Biassi, E. B., Popovska-Gorevski, M., and Dubocovich, M. L. (2016). MT1 and MT2 melatonin receptors: a therapeutic perspective. Annu. Rev. Pharmacol. Toxicol. 56, 361–383. doi: 10.1146/annurev-pharmtox-010814-124742
Liu, Y.-J., Chen, J., Li, X., Zhou, X., Hu, Y.-M., Chu, S.-F., et al. (2019). Research progress on adenosine in central nervous system diseases. CNS Neurosci. Therap. 25, 899–910. doi: 10.1111/cns.13190
Lou, Z.-Y., Cheng, J., Wang, X.-R., Zhao, Y.-F., Gan, J., Zhou, G.-Y., et al. (2018). The inhibition of CB receptor accelerates the onset and development of EAE possibly by regulating microglia/macrophages polarization. J. Neuroimmunol. 317, 37–44. doi: 10.1016/j.jneuroim.2018.02.001
Luongo, L., Guida, F., Imperatore, R., Napolitano, F., Gatta, L., Cristino, L., et al. (2014). The A1 adenosine receptor as a new player in microglia physiology. Glia 62, 122–132. doi: 10.1002/glia.22592
Lütjens, R., and Rocher, J.-P. (2017). Recent advances in drug discovery of GPCR allosteric modulators for neurodegenerative disorders. Curr. Opin. Pharmacol. 32, 91–95. doi: 10.1016/j.coph.2017.01.001
Ma, K., Guo, J., Wang, G., Ni, Q., and Liu, X. (2020). Toll-Like receptor 2-mediated autophagy promotes microglial cell death by modulating the microglial M1/M2 phenotype. Inflammation 43, 701–711. doi: 10.1007/s10753-019-01152-5
Madeira, M. H., Boia, R., Elvas, F., Martins, T., Cunha, R. A., Ambrósio, A. F., et al. (2016). Selective A2A receptor antagonist prevents microglia-mediated neuroinflammation and protects retinal ganglion cells from high intraocular pressure-induced transient ischemic injury. Transl. Res. 169, 112–128. doi: 10.1016/j.trsl.2015.11.005
Marucci, G., Ben, D. D., Lambertucci, C., Navia, A. M., Spinaci, A., Volpini, R., et al. (2021). Combined therapy of AAR agonists and AAR antagonists in neuroinflammation. Molecules 26:1188. doi: 10.3390/molecules26041188
McGeer, P. L., Itagaki, S., Boyes, B. E., and Mcgeer, E. G. (1988). Reactive microglia are positive for HLA-DR in the substantia nigra of Parkinson’s and Alzheimer’s disease brains. Neurology 38, 1285–1291.
Meng, F., Guo, Z., Hu, Y., Mai, W., Zhang, Z., Zhang, B., et al. (2019). CD73-derived adenosine controls inflammation and neurodegeneration by modulating dopamine signalling. Brain 142, 700–718. doi: 10.1093/brain/awy351
Merighi, S., Bencivenni, S., Vincenzi, F., Varani, K., Borea, P. A., and Gessi, S. (2017). A adenosine receptors stimulate IL-6 production in primary murine microglia through p38 MAPK kinase pathway. Pharmacol. Res. 117, 9–19. doi: 10.1016/j.phrs.2016.11.024
Mishina, M., Ishii, K., Kimura, Y., Suzuki, M., Kitamura, S., Ishibashi, K., et al. (2017). Adenosine a receptors measured with C-MPDX PET in early Parkinson’s disease. Synapse 71:e21979. doi: 10.1002/syn.21979
Molteni, R., Macchi, F., Zecchillo, C., Dell’agli, M., Colombo, E., Calabrese, F., et al. (2013). Modulation of the inflammatory response in rats chronically treated with the antidepressant agomelatine. Eur. Neuropsychopharmacol. 23, 1645–1655. doi: 10.1016/j.euroneuro.2013.03.008
Moriconi, A., Cerbara, I., Maccarrone, M., and Topai, A. (2010). GPR55: current knowledge and future perspectives of a purported “Type-3” cannabinoid receptor. Curr. Med. Chem. 17, 1411–1429.
Navarrete, F., García-Gutiérrez, M. S., Aracil-Fernández, A., Lanciego, J. L., and Manzanares, J. (2018). Cannabinoid CB1 and CB2 receptors, and monoacylglycerol lipase gene expression alterations in the basal ganglia of patients with Parkinson’s disease. Neurotherapeutics 15, 459–469. doi: 10.1007/s13311-018-0603-x
Nieto Gutierrez, A., and McDonald, P. H. (2018). GPCRs: emerging anti-cancer drug targets. Cell. Signal. 41, 65–74. doi: 10.1016/j.cellsig.2017.09.005
Noelker, C., Morel, L., Lescot, T., Osterloh, A., Alvarez-Fischer, D., Breloer, M., et al. (2013). Toll like receptor 4 mediates cell death in a mouse MPTP model of Parkinson disease. Sci. Rep. 3:1393. doi: 10.1038/srep01393
Oliveira-Giacomelli, A., M Albino, C., De Souza, H. D. N., Corrêa-Velloso, J., De Jesus Santos, A. P., Baranova, J., et al. (2019). P2Y6 and P2X7 receptor antagonism exerts neuroprotective/neuroregenerative effects in an animal model of Parkinson’s Disease. Front. Cell. Neurosci. 13:476. doi: 10.3389/fncel.2019.00476
O’Neill, E., and Harkin, A. (2018). Targeting the noradrenergic system for anti-inflammatory and neuroprotective effects: implications for Parkinson’s disease. Neural Regen. Res. 13, 1332–1337. doi: 10.4103/1673-5374.235219
O’Neill, E., Yssel, J. D., Mcnamara, C., and Harkin, A. (2020). Pharmacological targeting of β -adrenoceptors is neuroprotective in the LPS inflammatory rat model of Parkinson’s disease. Br. J. Pharmacol. 177, 282–297. doi: 10.1111/bph.14862
Orellana, J. A., Montero, T. D., and Von Bernhardi, R. (2013). Astrocytes inhibit nitric oxide-dependent Ca(2+) dynamics in activated microglia: involvement of ATP released via pannexin 1 channels. Glia 61, 2023–2037. doi: 10.1002/glia.22573
Orr, A. G., Orr, A. L., Li, X.-J., Gross, R. E., and Traynelis, S. F. (2009). Adenosine A(2A) receptor mediates microglial process retraction. Nat. Neurosci. 12, 872–878. doi: 10.1038/nn.2341
Pandi-Perumal, S. R., Bahammam, A. S., Brown, G. M., Spence, D. W., Bharti, V. K., Kaur, C., et al. (2013). Melatonin antioxidative defense: therapeutical implications for aging and neurodegenerative processes. Neurotox Res. 23, 267–300. doi: 10.1007/s12640-012-9337-4
Pandi-Perumal, S. R., Srinivasan, V., Spence, D. W., and Cardinali, D. P. (2007). Role of the melatonin system in the control of sleep: therapeutic implications. CNS Drugs 21, 995–1018.
Paul, S., Khanapur, S., Boersma, W., Sijbesma, J. W., Ishiwata, K., Elsinga, P. H., et al. (2014). Cerebral adenosine A1 receptors are upregulated in rodent encephalitis. Neuroimage 92, 83–89. doi: 10.1016/j.neuroimage.2014.01.054
Peleli, M., Fredholm, B. B., Sobrevia, L., and Carlström, M. (2017). Pharmacological targeting of adenosine receptor signaling. Mol. Aspects Med. 55, 4–8. doi: 10.1016/j.mam.2016.12.002
Perfilova, V. N., and Tyurenkov, I. N. (2016). [Glutamate metabotropic receptors: structure, localisation, functions]. Usp. Fiziol. Nauk 47, 98–112.
Peterson, L., Ismond, K. P., Chapman, E., and Flood, P. (2014). Potential benefits of therapeutic use of β2-adrenergic receptor agonists in neuroprotection and Parkinsonμs disease. J. Immunol. Res. 2014:103780. doi: 10.1155/2014/103780
Prinz, M., Jung, S., and Priller, J. (2019). Microglia biology: one century of evolving concepts. Cell 179, 292–311. doi: 10.1016/j.cell.2019.08.053
Puchałowicz, K., Tarnowski, M., Baranowska-Bosiacka, I., Chlubek, D., and Dziedziejko, V. (2014). P2X and P2Y receptors—role in the pathophysiology of the nervous system. Int. J. Mol. Sci. 15, 23672–23704. doi: 10.3390/ijms151223672
Qian, L., Hu, X., Zhang, D., Snyder, A., Wu, H.-M., Li, Y., et al. (2009). Beta2 Adrenergic receptor activation induces microglial NADPH oxidase activation and dopaminergic neurotoxicity through an ERK-dependent/protein kinase a-independent pathway. Glia 57, 1600–1609. doi: 10.1002/glia.20873
Qian, L., Wu, H.-M., Chen, S.-H., Zhang, D., Ali, S. F., Peterson, L., et al. (2011). β2-adrenergic receptor activation prevents rodent dopaminergic neurotoxicity by inhibiting microglia via a novel signaling pathway. J. Immunol 186, 4443–4454. doi: 10.4049/jimmunol.1002449
Qian, Y., Xu, S., Yang, X., and Xiao, Q. (2018). Purinergic receptor P2Y6 contributes to 1-methyl-4-phenylpyridinium-induced oxidative stress and cell death in neuronal SH-SY5Y cells. J. Neurosci. Res. 96, 253–264. doi: 10.1002/jnr.24119
Ransohoff, R. M. (2016). A polarizing question: do M1 and M2 microglia exist? Nat. Neurosci. 19, 987–991. doi: 10.1038/nn.4338
Reiter, R. J., Calvo, J. R., Karbownik, M., Qi, W., and Tan, D. X. (2000). Melatonin and its relation to the immune system and inflammation. Ann. N. Y. Acad. Sci. 917, 376–386.
Reiter, R. J., Mayo, J. C., Tan, D. X., Sainz, R. M., Alatorre-Jimenez, M., and Qin, L. (2016). Melatonin as an antioxidant: under promises but over delivers. J. Pineal. Res. 61, 253–278. doi: 10.1111/jpi.12360
Ribeiro, F. M., Vieira, L. B., Pires, R. G. W., Olmo, R. P., and Ferguson, S. S. G. (2017). Metabotropic glutamate receptors and neurodegenerative diseases. Pharmacol. Res. 115, 179–191. doi: 10.1016/j.phrs.2016.11.013
Ribeiro, R., Wen, J., Li, S., and Zhang, Y. (2013). Involvement of ERK1/2, cPLA2 and NF-κB in microglia suppression by cannabinoid receptor agonists and antagonists. Prostaglandins Other Lipid Mediat. 100–101, 1–14. doi: 10.1016/j.prostaglandins.2012.11.003
Rodríguez-Gómez, J. A., Kavanagh, E., Engskog-Vlachos, P., Engskog, M. K. R., Herrera, A. J., Espinosa-Oliva, A. M., et al. (2020). Microglia: agents of the CNS Pro-Inflammatory Response. Cells 9:1717. doi: 10.3390/cells9071717
Saliba, S. W., Jauch, H., Gargouri, B., Keil, A., Hurrle, T., Volz, N., et al. (2018). Anti-neuroinflammatory effects of GPR55 antagonists in LPS-activated primary microglial cells. J. Neuroinflamm. 15:322. doi: 10.1186/s12974-018-1362-7
Salvi, V., Sozio, F., Sozzani, S., and Del Prete, A. (2017). Role of atypical chemokine receptors in microglial activation and polarization. Front. Aging Neurosci. 9:148. doi: 10.3389/fnagi.2017.00148
Sanchez, A., Calpena, A. C., and Clares, B. (2015). Evaluating the oxidative stress in inflammation: role of melatonin. Int. J. Mol. Sci. 16, 16981–17004. doi: 10.3390/ijms160816981
Sawada, M., Imamura, K., and Nagatsu, T. (2006). Role of cytokines in inflammatory process in Parkinson’s disease. J. Neural Transm. Suppl. 70, 373–381.
Sharma, M., Arbabzada, N., and Flood, P. M. (2019). Mechanism underlying β2-AR agonist-mediated phenotypic conversion of LPS-activated microglial cells. J. Neuroimmunol. 332, 37–48. doi: 10.1016/j.jneuroim.2019.03.017
Shinozaki, Y., Shibata, K., Yoshida, K., Shigetomi, E., Gachet, C., Ikenaka, K., et al. (2017). Transformation of astrocytes to a neuroprotective phenotype by microglia via P2Y receptor downregulation. Cell Rep. 19, 1151–1164. doi: 10.1016/j.celrep.2017.04.047
Soliman, A. M., Fathalla, A. M., and Moustafa, A. A. (2018). Adenosine role in brain functions: pathophysiological influence on Parkinson’s disease and other brain disorders. Pharmacol. Rep. 70, 661–667. doi: 10.1016/j.pharep.2018.02.003
Sommerauer, M., Fedorova, T. D., Hansen, A. K., Knudsen, K., Otto, M., Jeppesen, J., et al. (2018). Evaluation of the noradrenergic system in Parkinson’s disease: an 11C-MeNER PET and neuromelanin MRI study. Brain 141, 496–504. doi: 10.1093/brain/awx348
Strosberg, A. D. (1993). Structure, function, and regulation of adrenergic receptors. Protein sci. 2, 1198–1209.
Sulkava, S., Muggalla, P., Sulkava, R., Ollila, H. M., Peuralinna, T., Myllykangas, L., et al. (2018). Melatonin receptor type 1A gene linked to Alzheimer’s disease in old age. Sleep 41:zsy103. doi: 10.1093/sleep/zsy103
Suofu, Y., Li, W., Jean-Alphonse, F. G., Jia, J., Khattar, N. K., Li, J., et al. (2017). Dual role of mitochondria in producing melatonin and driving GPCR signaling to block cytochrome c release. Proc. Natl. Acad. Sci. U.S.A. 114, E7997–E8006. doi: 10.1073/pnas.1705768114
Suurväli, J., Boudinot, P., Kanellopoulos, J., and Rüütel Boudinot, S. (2017). P2X4: a fast and sensitive purinergic receptor. Biomed. J. 40, 245–256. doi: 10.1016/j.bj.2017.06.010
Synowitz, M., Glass, R., Färber, K., Markovic, D., Kronenberg, G., Herrmann, K., et al. (2006). A1 adenosine receptors in microglia control glioblastoma-host interaction. Cancer Res. 66, 8550–8557.
Tang, H., Ma, M., Wu, Y., Deng, M.-F., Hu, F., Almansoub, H. A. M. M., et al. (2019). Activation of MT2 receptor ameliorates dendritic abnormalities in Alzheimer’s disease via C/EBPα/miR-125b pathway. Aging Cell 18:e12902. doi: 10.1111/acel.12902
Tang, Y., and Le, W. (2016). Differential roles of M1 and M2 microglia in neurodegenerative diseases. Mol. Neurobiol. 53, 1181–1194. doi: 10.1007/s12035-014-9070-5
Tatsumi, E., Yamanaka, H., Kobayashi, K., Yagi, H., Sakagami, M., and Noguchi, K. (2015). RhoA/ROCK pathway mediates p38 MAPK activation and morphological changes downstream of P2Y12/13 receptors in spinal microglia in neuropathic pain. Glia 63, 216–228. doi: 10.1002/glia.22745
Tóth, A., Antal, Z., Bereczki, D., and Sperlágh, B. (2019). Purinergic signalling in parkinson’s disease: a multi-target system to combat neurodegeneration. Neurochem. Res. 44, 2413–2422. doi: 10.1007/s11064-019-02798-1
Von Kügelgen, I., and Hoffmann, K. (2016). Pharmacology and structure of P2Y receptors. Neuropharmacology 104, 50–61. doi: 10.1016/j.neuropharm.2015.10.030
Wang, J., Jiang, C., Zhang, K., Lan, X., Chen, X., Zang, W., et al. (2019). Melatonin receptor activation provides cerebral protection after traumatic brain injury by mitigating oxidative stress and inflammation via the Nrf2 signaling pathway. Free Radic. Biol. Med. 131, 345–355. doi: 10.1016/j.freeradbiomed.2018.12.014
Wang, X., Sirianni, A., Pei, Z., Cormier, K., Smith, K., Jiang, J., et al. (2011). The melatonin MT1 receptor axis modulates mutant Huntingtin-mediated toxicity. J. Neurosci. 31, 14496–14507. doi: 10.1523/JNEUROSCI.3059-11.2011
Wilkaniec, A., Cieślik, M., Murawska, E., Babiec, L., Ga̧ssowska-Dobrowolska, M., Pałasz, E., et al. (2020). P2X7 receptor is involved in mitochondrial dysfunction induced by extracellular alpha synuclein in neuroblastoma SH-SY5Y cells. Int. J. Mol. Sci. 21:3959. doi: 10.3390/ijms21113959
Williams, C. J., and Dexter, D. T. (2014). Neuroprotective and symptomatic effects of targeting group III mGlu receptors in neurodegenerative disease. J. Neurochem. 129, 4–20. doi: 10.1111/jnc.12608
Wolf, S. A., Boddeke, H. W., and Kettenmann, H. (2017). Microglia in physiology and disease. Annu. Rev. Physiol. 79, 619–643. doi: 10.1146/annurev-physiol-022516-034406
Wu, Y. H., Zhou, J. N., Van Heerikhuize, J., Jockers, R., and Swaab, D. F. (2007). Decreased MT1 melatonin receptor expression in the suprachiasmatic nucleus in aging and Alzheimer’s disease. Neurobiol. Aging 28, 1239–1247. doi: 10.1016/j.neurobiolaging.2006.06.002
Yamanaka, D., Kawano, T., Nishigaki, A., Aoyama, B., Tateiwa, H., Shigematsu-Locatelli, M., et al. (2017). Preventive effects of dexmedetomidine on the development of cognitive dysfunction following systemic inflammation in aged rats. J. Anesth. 31, 25–35. doi: 10.1007/s00540-016-2264-4
Yan, Y., Jiang, W., Liu, L., Wang, X., Ding, C., Tian, Z., et al. (2015). Dopamine controls systemic inflammation through inhibition of NLRP3 inflammasome. Cell 160, 62–73. doi: 10.1016/j.cell.2014.11.047
Yang, X., Lou, Y., Liu, G., Wang, X., Qian, Y., Ding, J., et al. (2017). Microglia P2Y6 receptor is related to Parkinson’s disease through neuroinflammatory process. J. Neuroinflamm. 14:38. doi: 10.1186/s12974-017-0795-8
Yu, T., Zhang, X., Shi, H., Tian, J., Sun, L., Hu, X., et al. (2019). P2Y12 regulates microglia activation and excitatory synaptic transmission in spinal lamina II neurons during neuropathic pain in rodents. Cell Death Disease 10:165. doi: 10.1038/s41419-019-1425-4
Zabala, A., Vazquez-Villoldo, N., Rissiek, B., Gejo, J., Martin, A., Palomino, A., et al. (2018). P2X4 receptor controls microglia activation and favors remyelination in autoimmune encephalitis. EMBO Mol. Med. 10:e8743. doi: 10.15252/emmm.201708743
Zeng, J., Wang, G., Liu, X., Wang, C., Tian, H., Liu, A., et al. (2014). P2Y13 receptor-mediated rapid increase in intracellular calcium induced by ADP in cultured dorsal spinal cord microglia. Neurochem. Res. 39, 2240–2250. doi: 10.1007/s11064-014-1426-8
Zhang, P., Li, Y., Han, X., Xing, Q., and Zhao, L. (2017). Dexmedetomidine Regulates 6-hydroxydopamine-Induced microglial polarization. Neurochem. Res. 42, 1524–1532. doi: 10.1007/s11064-017-2209-9
Zhang, X., Wang, J., Gao, J.-Z., Zhang, X.-N., Dou, K.-X., Shi, W.-D., et al. (2021). P2X4 receptor participates in autophagy regulation in Parkinson’s disease. Neural Reg. Res. 16, 2505–2511. doi: 10.4103/1673-5374.313053
Zhang, Y.-N., Fan, J.-K., Gu, L., Yang, H.-M., Zhan, S.-Q., and Zhang, H. (2021). Metabotropic glutamate receptor 5 inhibits α-synuclein-induced microglia inflammation to protect from neurotoxicity in Parkinson’s disease. J. Neuroinflamm. 18:23. doi: 10.1186/s12974-021-02079-1
Zhou, Y., Lu, M., Du, R.-H., Qiao, C., Jiang, C.-Y., Zhang, K.-Z., et al. (2016). MicroRNA-7 targets Nod-like receptor protein 3 inflammasome to modulate neuroinflammation in the pathogenesis of Parkinson’s disease. Mol. Neurodegen. 11:28. doi: 10.1186/s13024-016-0094-3
Zlotos, D. P., Jockers, R., Cecon, E., Rivara, S., and Witt-Enderby, P. A. (2014). MT1 and MT2 melatonin receptors: ligands, models, oligomers, and therapeutic potential. J. Med. Chem. 57, 3161–3185. doi: 10.1021/jm401343c
Keywords: G protein-coupled receptor (GPCR), microglial activation, Parkinson’s disease, neuroinflammation, dopaminergic (DA) neuronal loss
Citation: Gu C, Chen Y, Chen Y, Liu C-F, Zhu Z and Wang M (2021) Role of G Protein-Coupled Receptors in Microglial Activation: Implication in Parkinson’s Disease. Front. Aging Neurosci. 13:768156. doi: 10.3389/fnagi.2021.768156
Received: 31 August 2021; Accepted: 23 October 2021;
Published: 16 November 2021.
Edited by:
Yong-Jing Gao, Nantong University, ChinaReviewed by:
Ning Song, Qingdao University, ChinaCopyright © 2021 Gu, Chen, Chen, Liu, Zhu and Wang. This is an open-access article distributed under the terms of the Creative Commons Attribution License (CC BY). The use, distribution or reproduction in other forums is permitted, provided the original author(s) and the copyright owner(s) are credited and that the original publication in this journal is cited, in accordance with accepted academic practice. No use, distribution or reproduction is permitted which does not comply with these terms.
*Correspondence: Chao Gu, OTkzNTExNzgwQHFxLmNvbQ==; Mei Wang, d2FuZ21laXNkZmV5QDE2My5jb20=
Disclaimer: All claims expressed in this article are solely those of the authors and do not necessarily represent those of their affiliated organizations, or those of the publisher, the editors and the reviewers. Any product that may be evaluated in this article or claim that may be made by its manufacturer is not guaranteed or endorsed by the publisher.
Research integrity at Frontiers
Learn more about the work of our research integrity team to safeguard the quality of each article we publish.