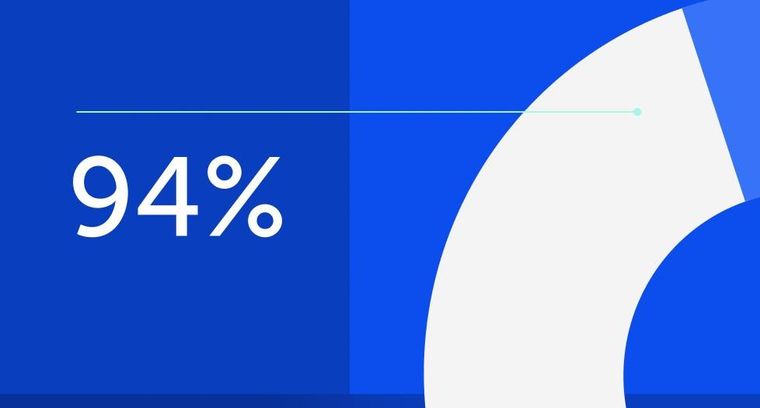
94% of researchers rate our articles as excellent or good
Learn more about the work of our research integrity team to safeguard the quality of each article we publish.
Find out more
BRIEF RESEARCH REPORT article
Front. Aging Neurosci., 28 September 2021
Sec. Neuroinflammation and Neuropathy
Volume 13 - 2021 | https://doi.org/10.3389/fnagi.2021.711784
This article is part of the Research TopicBlood Biomarkers of Neurodegenerative DiseasesView all 26 articles
Background: Cerebral small vessel disease (SVD) is associated with increased risk of stroke and dementia. Progressive damage to the cerebral microvasculature may also trigger angiogenic processes to promote vessel repair. Elevated levels of circulating endothelial progenitor cells (EPCs) and pro-angiogenic signaling proteins are observed in response to vascular injury. We aimed to examine circulating levels of EPCs and proangiogenic proteins in older adults with evidence of SVD.
Methods: Older adults (ages 55–90) free of dementia or stroke underwent venipuncture and brain magnetic resonance imaging (MRI). Flow cytometry quantified circulating EPCs as the number of cells in the lymphocyte gate positively expressing EPC surface markers (CD34+CD133+CD309+). Plasma was assayed for proangiogenic factors (VEGF-A, VEGF-C, VEGF-D, Tie-2, and Flt-1). Total SVD burden score was determined based on MRI markers, including white matter hyperintensities, cerebral microbleeds and lacunes.
Results: Sixty-four older adults were included. Linear regression revealed that older adults with higher circulating EPC levels exhibited greater total SVD burden [β = 1.0 × 105, 95% CI (0.2, 1.9), p = 0.019], after accounting for age and sex. Similarly, a positive relationship between circulating VEGF-D and total SVD score was observed, controlling for age and sex [β = 0.001, 95% CI (0.000, 0.001), p = 0.048].
Conclusion: These findings suggest that elevated levels of circulating EPCs and VEGF-D correspond with greater cerebral SVD burden in older adults. Additional studies are warranted to determine whether activation of systemic angiogenic growth factors and EPCs represents an early attempt to rescue the vascular endothelium and repair damage in SVD.
Cerebral small vessel disease (SVD) occurs commonly with advancing age and is associated with increased risk of cognitive impairment, vascular dementia and Alzheimer’s disease, contributing to up to 45% of all cases of dementia (Gorelick et al., 2011; Lee et al., 2016). Small vessel changes often remain asymptomatic, but are evident on brain magnetic resonance imaging (MRI) (Wardlaw et al., 2019) and believed to result from pathological changes in the perforating cerebral arteries, penetrating arterioles, capillaries, and venules (Pantoni, 2010). Microvascular function is crucial to addressing cerebral metabolic demands, clearing waste products and maintaining the blood-brain barrier (Zlokovic, 2008; Chabriat et al., 2009; Wardlaw et al., 2013a, 2019; Pettersen et al., 2017; Nation et al., 2019). Reduced blood flow and hypoxia due to degradation of small blood vessels may trigger angiogenesis—the process of blood vessel growth—as a compensatory mechanism (Quick et al., 2021). While it is still debated whether the process of angiogenesis is beneficial or detrimental in the context of vascular brain injury, animal models and studies of large vessel disease show increased angiogenesis in response to vascular injury (Adamczak and Hoehn, 2015).
One feature of angiogenesis is the mobilization of cells that can repair the vasculature. Endothelial progenitor cells (EPCs) represent a heterogeneous cell population known to be mobilized in response to vascular-endothelial damage to promote vessel repair and regenesis (Medina et al., 2017). Increased levels of circulating EPCs at day 7 post-intracerebral hemorrhage predict improved functional outcome at 12 months (Pías-Peleteiro et al., 2016) and mobilization of EPCs has been observed in response to cerebral large vessel disease (Sobrino et al., 2007). Animal mouse models of ischemic stroke suggest that transplantation of in vitro cultured EPCs attenuates blood–brain barrier leakage, tight junction protein degradation, and neurological deficits (Geng et al., 2017; Kong et al., 2018).
Similarly, damage to the cerebral vasculature can trigger the release of signaling proteins that are involved in angiogenesis. The vascular endothelial growth factor (VEGF) family proteins, as well as other angiogenic factors, are also known to induce endothelial and neuronal remodeling after stroke (Hayakawa et al., 2017). Levels of these proteins are elevated after ischemic insult in brain tissue and serum in rodent models and in humans (Issa et al., 1999; Zhang et al., 2000; Prodjohardjono et al., 2020). However, few previous studies have evaluated whether changes in circulating angiogenic cells and proteins also occur in response to progressive and insidious cerebral SVD changes which commonly occur with advancing age.
The current study evaluated whether circulating levels of EPCs and pro-angiogenic proteins are associated with SVD burden in older adults who were otherwise neurologically healthy, with no history of clinical stroke or dementia. Consistent with prior studies, we hypothesized that higher levels of circulating angiogenic proteins and EPCs would be associated with greater cerebral SVD burden.
Participants were recruited from the community and included if they were 55 years of age or older, independently living with no history of clinical stroke, dementia or other systemic or neurological illness that may impact central nervous system function. History of vascular risk factors, including hypertension, body mass index, dyslipidemia, diabetes, as well as history of other medical illnesses, was determined by clinical interview. This study was approved by the local Institutional Review Board; all participants gave informed consent and underwent blood draw and brain MRI.
Venipuncture was performed after an overnight fast. EPCs were quantified with flow cytometry. Peripheral blood mononuclear cells (PBMCs) were isolated by density gradient centrifugation and washed twice with DPBS + 2% FBS at 120 × g for 10 min and 300 × g for 8 min at room temperature. Fluorescently labeled antibodies to EPC surface antigens were utilized (Nation et al., 2018). 1 million cells were transferred to an unstained tube blank, and the remainder was transferred to the stained tube, where 5 μL of Human BD Fc Block (BD Biosciences) was added and incubated for 10– 15 min. An additional 1 μL of each of the following antibodies were then added: (Gorelick et al., 2011) CD34-PE-Vio770 (clone: AC136, Miltenyi Biotec), (Lee et al., 2016) CD133-VioBright FITC (clone: AC133, Miltenyi Biotec), (Wardlaw et al., 2019) PerCP/Cy5.5-CD309 (clone: 7D4-6, BioLegend). Tubes were incubated in the dark for 30 min at 4°C. Samples were washed with 3 mL PBS, centrifuged at 300 × g for 8 min, and fixed with 2% formaldehyde in PBS until analysis. Compensation controls were conducted using AbC Total Antibody Compensation Bead Kit (Thermo Fisher Scientific). Samples were acquired on a BD LSR II flow cytometer and analyzed on FlowJo software. EPCs were defined as CD34+CD133+CD309+ cells (Medina et al., 2017). Circulating EPCs were quantified by flow cytometry as the number of cells in the lymphocyte gate positively expressing EPC surface markers (CD34+CD133+CD309+). CD34+CD133+CD309+ cell concentrations were calculated as a percentage of lymphocytes.
Apolipoprotein E (APOE) genotyping was conducted on the blood cell pellet fraction obtained from plasma separation. DNA was isolated from the pellet fraction using the PureLink Genomic DNA Mini Kit (Thermo Fisher Scientific). Genotyping was conducted on isolated DNA using the TaqMan SNP Genotyping Assay (Thermo Fisher Scientific) on an Applied Biosystems 7300 Real Time PCR System. APOE gene SNPs were assessed for dbSNP IDs rs429358 and rs7412. Allelic discrimination was conducted using the included qPCR software. The APOE-ε4 allele was designated as rs429358-C + rs7412-C.
Levels of angiogenic proteins in plasma were determined using the Meso Scale Discovery V- PLEX Human Biomarker 40-Plex Kit Angiogenesis Panel 1 (VEGF-A, VEGF-C, VEGF-D, Tie-2, Flt-1, PIGF, and bFGF), following manufacturer’s protocol without modification. Briefly, we utilized the MSD Multi-Spot 96-well 7-spot plate pre-coated with capture antibodies. Plates were washed three times with at least 150 μl/well of wash buffer. 50 μl of diluted plasma samples per well were added for the angiogenesis panel and incubated at room temperature with shaking for 2 h. Plates were then washed 3 times with at least 150 μl/well of washing buffer. 25 μl of detection antibody solution was added to each well and incubated at room temperature with shaking for 2 h. Plates were then washed three times again. 150 μl of 2X Read Buffer T was added to each well. Plates were then analyzed on an MSD instrument. Values below the lower limit of detection (LLOD) were replaced with zero.
All participants underwent a comprehensive neuroimaging protocol as previously described (Nation et al., 2018). The following sequences were examined for the current analysis: 3D T1-weighted anatomical scan for qualitative assessment of brain structures and abnormalities, T2-weighted scan for identification and differentiation of lacunes, fluid-attenuated inversion recovery (T2-FLAIR) for the evaluation of white matter hyperintensities, and T2∗-weighted imaging for assessment of cerebral microbleeds (Pantoni, 2010; Gorelick et al., 2011; Lee et al., 2016; Wardlaw et al., 2019). MRI markers were identified and scored in accordance with established neuroimaging standards for SVD (Wardlaw et al., 2013b). To determine total MRI SVD burden, all imaging markers were combined using a SVD score (amended version) recently developed by Amin Al Olama et al. (2020), which ranges from 0 to 7 and includes grading of white matter hyperintensities (0–3; using the Fazekas scale), number of lacunes (0–3; 0 = no lacunes, 1 = 1–2, 2 = 3–5, 3 = > 5) and presence of microbleeds (0–1; 0 = absent, 1 = present).
All analyses were performed using IBM SPSS Statistics 27 and R Version 3.6.1. Data were initially screened for extreme values (outliers) defined as values greater than 5 standard deviations from the mean and values that were unduly affecting regression parameter estimates based on measures of distance or influence. Two participants had extreme values for CD34+CD133+CD309+ cell counts, two participants had extreme values for VEGF-C and one participant had an extreme value for VEGF-D and were excluded from analyses. We examined the relationship between circulating EPCs as well as angiogenic proteins (independent predictors) and SVD score (dependent outcome), independently and after adjusting for age and sex. In addition, we examined any significant effects of APOE4 carrier status on the relationship between angiogenic circulating cells as well as proteins and SVD burden. Significance threshold was set at p < 0.05 for all analyses.
A total of 64 participants were included in the analysis. Age of study participants ranged from 55 to 90 years [Mean (M) = 69.8, standard deviation (SD) = 7.3], education ranged from 6 to 20 years [M = 15.8, SD = 2.8] and 40.6% were male (Table 1). Levels of circulating angiogenic proteins can be found in Table 2.
Small vessel disease markers were evident (SVD score > 1) in 29 (45.3%) participants (Figure 1). SVD scores ranged from 0 to 4 (M = 1.6, SD = 1.0). Microbleeds were identified in 5 (7.8%) participants, and small lacunes in 8 (12.5%) participants. White matter hyperintensities were identified in majority of participants; 36 (56.3%) displayed mild white matter hyperintensity burden (Fazekas 1), 18 (28.1%) displayed moderate burden (Fazekas 2) and 6 (9.4%) showed severe burden (Fazekas 3).
Figure 1. Small vessel disease as identified on magnetic resonance imaging (MRI) by white arrows. (A) Microbleed identified on T2*; (B) white matter hyperintensities identified on FLAIR; (C) lacune on FLAIR.
Endothelial progenitor cell count (CD34+CD133+CD309+ count per lymphocyte) and SVD score was available for 52 participants and 2 outliers were removed; 50 participants were therefore included in this analysis. Mean EPC count was 2.7 × 10–6 CD34+CD133+CD309+ cells/lymphocyte (SD = 3.4 × 10–6). Linear regression revealed that higher levels of circulating EPCs was associated with greater SVD burden, even after accounting for age and sex in multiple regression [β = 1.0 × 105, 95% CI (0.2, 1.9), p = 0.019]. The relationship remained significant even after adjusting for APOE4 carrier status and a significant positive effect of APOE4 carrier status was observed, with APOE4 carriers having greater SVD burden [N = 48; β = 0.57, 95% CI (0.01, 1.13), p = 0.046; Table 3].
We specifically examined the relationship of VEGF-A, VEGF-C, VEGF-D, Tie-2, and Flt-1 with SVD score. Values were available for all 64 participants, however, two participants had extreme values for VEGF-C and one participant had an extreme value for VEGF-D and were excluded from the current analyses. Simple linear regression revealed a positive relationship only between circulating VEGF-D and total SVD score, which remained significant in multiple regression controlling for age and sex [β = 0.001, 95% CI (0.000, 0.001), p = 0.048]. The relationship remained significant even after adjusting for APOE4 carrier status (Table 4).
Mobilization of EPCs and release of angiogenic growth factors has been observed in response to cerebral large vessel disease (Sobrino et al., 2007). Consistent with such studies, our findings indicate increased levels of circulating EPCs and angiogenic proteins in the presence of cerebral SVD. Specifically, circulating numbers of CD34+CD133+CD309+ cells and levels of VEGF-D protein correlated with greater cerebral SVD burden based on white matter hyperintensities, cerebral microbleeds and lacunes. SVD markers were evident on MRI in almost half our sample, yet participants did not have major cognitive dysfunction at the time of assessment, suggesting that the correlation between cerebral SVD and these angiogenic factors may be observed during the preclinical disease stage. While the current study was correlational, precluding causal inference, our findings are consistent with the hypothesis that EPCs and angiogenic signaling proteins may be increased in circulation in response to cerebral SVD (Nation et al., 2018).
Studies focused on clinically symptomatic patients with more severe white matter changes, cognitive impairment and dementia indicate a limited pool of EPCs may be exhausted with the emergence and progression of clinical symptomatology (Iadecola, 2013). Based on these data and the present study findings, we hypothesize that increased circulating EPC and VEGF-D levels in asymptomatic older adults may represent preclinical markers of early-stage cerebral small vessel injury, and that later exhaustion of the EPC pool may coincide with progressive cognitive decline (Lee et al., 2009). Elevation of VEGF in the presence of cognitive impairment is supported by prior research (Mahoney et al., 2021), although the literature suggests that EPC levels deplete in correspondence with the decline in cognitive function (Lee et al., 2009). If our hypothesized model is supported, the potential for circulating EPC and VEGF-D levels biomarkers for early-stage cerebral SVD changes warrants further research. Moreover, animal studies suggest that transplantation of EPCs may attenuate blood brain barrier breakdown (Geng et al., 2017), tight junction protein degradation, and neurological deficits (Geng et al., 2017; Kong et al., 2018), suggesting that EPCs may also offer potential therapeutic opportunities. Similarly, in vitro studies suggest that VEGF may support EPC differentiation and vascular repair (Li et al., 2017) but additional studies are needed.
Increased levels of VEGF-D have been associated with atrial fibrillation, ischemic stroke and heart failure (Borné et al., 2018; Berntsson et al., 2019) and elevated levels are known to predict mortality in patients with coronary artery disease (Wada et al., 2020). VEGF-D is a secreted glycoprotein and one of five members of the VEGF family with high angiogenic and lymphangiogenic potential (Lohela et al., 2009). Recent animal and in vitro studies also suggest a role for VEGF-D in dendrite maintenance required for memory formation and other cognitive processes (Mauceri et al., 2011, 2020; Stacker and Achen, 2018). It remains unclear whether circulating EPCs may play related roles in central nervous system functions. In the present study, we demonstrate an association between circulating VEGF-D and SVD in older adults, suggesting that angiogenic and lymphangiogenic processes may be taking places. Prior studies have demonstrated a role for VEGF-A in EPC recruitment to stimulate vasculogenesis, however, no prior studies have examined the relationship between VEGF-D and EPCs, making it challenging to understand the relationship and directionality between the two based on current literature. Further studies are warranted to confirm these findings and uncover potential mechanisms.
Whether the mobilization of EPCs and angiogenic factors to promote angiogenesis is beneficial or detrimental in SVD warrants further attention. Angiogenic processes, including endothelial barrier function and cell connections that may be weakened during repair and remodeling, may lead to inflammation and increased blood–brain barrier permeability (Adamczak and Hoehn, 2015). The involvement and impact of angiogenic cells and signaling proteins in repairing cerebral SVD—often a progressive form of vascular damage which worsens with age—may be important in understanding cognitive decline in older adults.
Limitations of this study include the limited sample size and cross-sectional design. Further studies may elucidate the predictive value of these angiogenic biomarkers. Moreover, additional angiogenic markers warrant further attention. We utilized the MSD angiogenesis biomarker panel in this study; however, numerous other proteins play a significant role in mediating angiogenesis and advanced techniques could be further utilized to identify and examine additional markers of angiogenesis. Future studies could also examine the relationship between inflammation and EPCs, given that recent studies suggest that inflammation may influence EPC function (Naserian et al., 2020; Nouri Barkestani et al., 2021).
Another limitation is the lack of specificity and varying definitions of EPCs in current literature. Gating for flow cytometry and calculation of EPCs also remains inconsistent. We utilized the consensus statement and recent studies to define EPCs as CD34+CD133+CD309+ cells (Medina et al., 2017). However, inclusion of cell markers such as CD45 remains inconsistent (Medina et al., 2017) and commonly employed methods to quantify EPCs assume a high risk of cell loss. Additional studies are needed to better understand and quantify the EPC population. The findings of this study provide further insight into the potential role of angiogenesis in microvascular pathology of the aging brain.
The raw data supporting the conclusions of this article will be made available by the authors, upon reasonable request.
The studies involving human participants were reviewed and approved by the University of Southern California and University of California, Irvine. The patients/participants provided their written informed consent to participate in this study.
DN contributed to the study design. AK and DN contributed to analyzing the data and drafting the manuscript. AG, AM, IM, BY, JH, AEB, SD, IS, YL, JJ, AMB, and KR contributed to data collection. All authors contributed to manuscript revision, read, and approved the submitted version.
This research was supported by the National Institutes of Health grants (DN: R01AG064228, R01AG060049, P01AG052350, and P50AG016573), Alzheimer’s Association (DN: AARG-17-532905), National Science Foundation (SD: DGE1418060), and Canadian Institutes of Health Research (AK: DFD-170763).
IM was employed by company Chione GmbH.
The remaining authors declare that the research was conducted in the absence of any commercial or financial relationships that could be construed as a potential conflict of interest.
All claims expressed in this article are solely those of the authors and do not necessarily represent those of their affiliated organizations, or those of the publisher, the editors and the reviewers. Any product that may be evaluated in this article, or claim that may be made by its manufacturer, is not guaranteed or endorsed by the publisher.
Adamczak, J., and Hoehn, M. (2015). Poststroke Angiogenesis, Con. Stroke 2015:46. doi: 10.1161/STROKEAHA.114.007642
Amin Al Olama, A., Wason, J. M. S., Tuladhar, A. M., van Leijsen, E. M. C., Koini, M., Hofer, E., et al. (2020). Simple MRI score aids prediction of dementia in cerebral small vessel disease. Neurology 94, e1294–e1302. doi: 10.1212/WNL.0000000000009141
Berntsson, J., Smith, J. G., Johnson, L. S. B., Söderholm, M., Borné, Y., Melander, O., et al. (2019). Increased vascular endothelial growth factor D is associated with atrial fibrillation and ischaemic stroke. Heart 105, 553–558. doi: 10.1136/heartjnl-2018-313684
Borné, Y., Gränsbo, K., Nilsson, J., Melander, O., Orho-Melander, M., Smith, J. G., et al. (2018). Vascular Endothelial Growth Factor D, Pulmonary Congestion, and Incidence of Heart Failure. J. Am. Coll. Cardiol. 71, 580–582. doi: 10.1016/j.jacc.2017.11.058
Chabriat, H., Joutel, A., Dichgans, M., Tournier-Lasserve, E., and Bousser, M.-G. (2009). CADASIL. Lancet Neurol. 2009, 643–653. doi: 10.1016/S1474-4422(09)70127-9
Geng, J., Wang, L., Qu, M., Song, Y., Lin, X., Chen, Y., et al. (2017). Endothelial progenitor cells transplantation attenuated blood-brain barrier damage after ischemia in diabetic mice via HIF- 1α. Stem Cell Res. Ther. 8:163. doi: 10.1186/s13287-017-0605-3
Gorelick, P. B., Scuteri, A., Black, S. E., Decarli, C., Greenberg, S. M., Iadecola, C., et al. (2011). Vascular contributions to cognitive impairment and dementia: A statement for healthcare professionals from the American Heart Association/American Stroke Association. Stroke 42, 2672–2713. doi: 10.1161/STR.0b013e3182299496
Hayakawa, K., Seo, J. H., Miyamoto, N., Pham, L.-D. D., Navaratna, D., Lo, E. H., et al. (2017). Brain Angiogenesis After Stroke. In: Biochemical Basis and Therapeutic Implications of Angiogenesis. Cham: Springer International Publishing, 473–494. doi: 10.1007/978-3-319-61115-0_21
Iadecola, C. (2013). The pathobiology of vascular dementia. Neuron 2013, 844–866. doi: 10.1016/j.neuron.2013.10.008
Issa, R., Krupinski, J., Bujny, T., Kumar, S., Kaluza, J., and Kumar, P. (1999). Vascular endothelial growth factor and its receptor, KDR, in human brain tissue after ischemic stroke. Lab. Invest. 79, 417–425.
Kong, Z., Hong, Y., Zhu, J., Cheng, X., and Liu, Y. (2018). Endothelial progenitor cells improve functional recovery in focal cerebral ischemia of rat by promoting angiogenesis via VEGF. J. Clin. Neurosci. 55, 116–121. doi: 10.1016/j.jocn.2018.07.011
Lee, S., Viqar, F., Zimmerman, M. E., Narkhede, A., Tosto, G., Benzinger, T. L. S., et al. (2016). White matter hyperintensities are a core feature of Alzheimer’s disease: Evidence from the dominantly inherited Alzheimer network. Ann. Neurol. 79, 929–939. doi: 10.1002/ana.24647
Lee, S.-T., Chu, K., Jung, K.-H., Park, H.-K., Kim, D.-H., Bahn, J.-J., et al. (2009). Reduced circulating angiogenic cells in Alzheimer disease. Neurology 72, 1858–1863. doi: 10.1212/WNL.0b013e3181a711f4
Li, L., Liu, H., Xu, C., Deng, M., Song, M., Yu, X., et al. (2017). VEGF promotes endothelial progenitor cell differentiation and vascular repair through connexin 43. Stem Cell Res. Ther. 8:237. doi: 10.1186/s13287-017-0684-1
Lohela, M., Bry, M., Tammela, T., and Alitalo, K. (2009). VEGFs and receptors involved in angiogenesis versus lymphangiogenesis. Curr. Opin. Cell Biol. 21, 154–165. doi: 10.1016/j.ceb.2008.12.012
Mahoney, E. R., Dumitrescu, L., Moore, A. M., Cambronero, F. E., De Jager, P. L., Koran, M. E. I., et al. (2021). Brain expression of the vascular endothelial growths factor gene family in cognitive aging and alzheimer’s disease. Mol Psychiatry 26, 888–896. doi: 10.1038/s41380-019-0458-5
Mauceri, D., Buchthal, B., Hemstedt, T. J., Weiss, U., Klein, C. D., and Bading, H. (2020). Nasally delivered VEGFD mimetics mitigate stroke-induced dendrite loss and brain damage. Proc. Natl. Acad. Sci. 117, 8616–8623. doi: 10.1073/pnas.2001563117
Mauceri, D., Freitag, H. E., Oliveira, A. M. M., Bengtson, C. P., and Bading, H. (2011). Nuclear Calcium-VEGFD Signaling Controls Maintenance of Dendrite Arborization Necessary for Memory Formation. Neuron 71, 117–130. doi: 10.1016/j.neuron.2011.04.022
Medina, R. J., Barber, C. L., Sabatier, F., Dignat-George, F., Melero-Martin, J. M., Khosrotehrani, K., et al. (2017). Endothelial Progenitors: A Consensus Statement on Nomenclature. Stem Cells Transl. Med. 6, 1316–1320. doi: 10.1002/sctm.16-0360
Naserian, S., Abdelgawad, M. E., Afshar Bakshloo, M., Ha, G., Arouche, N., Cohen, J. L., et al. (2020). The TNF/TNFR2 signaling pathway is a key regulatory factor in endothelial progenitor cell immunosuppressive effect. Cell Commun. Signal. 18:94. doi: 10.1186/s12964-020-00564-3
Nation, D. A., Sweeney, M. D., Montagne, A., Sagare, A. P., D’Orazio, L. M., Pachicano, M., et al. (2019). Blood- brain barrier breakdown is an early biomarker of human cognitive dysfunction. Nat. Med. 25, 270–276. doi: 10.1038/s41591-018-0297-y
Nation, D. A., Tan, A., Dutt, S., McIntosh, E. C., Yew, B., Ho, J. K., et al. (2018). Circulating Progenitor Cells Correlate with Memory, Posterior Cortical Thickness, and Hippocampal Perfusion. J. Alzheimers Dis. 61, 91–101. doi: 10.3233/JAD-170587
Nouri Barkestani, M., Shamdani, S., Afshar Bakshloo, M., Arouche, N., Bambai, B., Uzan, G., et al. (2021). TNFα priming through its interaction with TNFR2 enhances endothelial progenitor cell immunosuppressive effect: new hope for their widespread clinical application. Cell Commun. Signal. 19:1. doi: 10.1186/s12964-020-00683-x
Pantoni, L. (2010). Cerebral small vessel disease: from pathogenesis and clinical characteristics to therapeutic challenges. Lancet Neurol. 9, 689–701. doi: 10.1016/S1474-4422(10)70104-6
Pettersen, J. A., Keith, J., Gao, F., Spence, J. D., and Black, S. E. (2017). CADASIL accelerated by acute hypotension: Arterial and venous contribution to leukoaraiosis. Neurology 88, 1077–1080. doi: 10.1212/WNL.0000000000003717
Pías-Peleteiro, J., Pérez-Mato, M., López-Arias, E., Rodríguez-Yáñez, M., Blanco, M., Campos, F., et al. (2016). Increased Endothelial Progenitor Cell Levels are Associated with Good Outcome in Intracerebral Hemorrhage. Sci. Rep. 6:28724. doi: 10.1038/srep28724
Prodjohardjono, A., Vidyanti, A. N., Susianti, N. A., Sudarmanta, S. S., and Setyopranoto, I. (2020). Higher level of acute serum VEGF and larger infarct volume are more frequently associated with post-stroke cognitive impairment. PLoS One 15:e0239370. doi: 10.1371/journal.pone.0239370
Quick, S., Moss, J., Rajani, R. M., and Williams, A. A. (2021). Vessel for Change: Endothelial Dysfunction in Cerebral Small Vessel Disease. Trends Neurosci. 44, 289–305. doi: 10.1016/j.tins.2020.11.003
Sobrino, T., Hurtado, O., Moro, M. A., Rodríguez-Yáñez, M., Castellanos, M., Brea, D., et al. (2007). The increase of circulating endothelial progenitor cells after acute ischemic stroke is associated with good outcome. Stroke 38, 2759–2764. doi: 10.1161/STROKEAHA.107.484386
Stacker, S., and Achen, M. (2018). Emerging Roles for VEGF-D in Human Disease. Biomolecules 8:1. doi: 10.3390/biom8010001
Wada, H., Suzuki, M., Matsuda, M., Ajiro, Y., Shinozaki, T., Sakagami, S., et al. (2020). Distinct Characteristics of VEGF-D and VEGF-C to Predict Mortality in Patients With Suspected or Known Coronary Artery Disease. J. Am. Heart Assoc. 9:9. doi: 10.1161/JAHA.119.015761
Wardlaw, J. M., Doubal, F. N., Valdes-Hernandez, M., Wang, X., Chappell, F. M., Shuler, K., et al. (2013a). Blood-brain barrier permeability and long-term clinical and imaging outcomes in cerebral small vessel disease. Stroke 2013, 525–527. doi: 10.1161/STROKEAHA.112.669994
Wardlaw, J. M., Smith, C., and Dichgans, M. (2019). Small vessel disease: mechanisms and clinical implications. Lancet Neurol. 18, 684–696. doi: 10.1016/S1474-4422(19)30079-1
Wardlaw, J. M., Smith, E. E., Biessels, G. J., Cordonnier, C., Fazekas, F., Frayne, R., et al. (2013b). Neuroimaging standards for research into small vessel disease and its contribution to ageing and neurodegeneration. Lancet Neurol. 12, 822–838. doi: 10.1016/S1474-4422(13)70124-8
Zhang, Z. G., Zhang, L., Jiang, Q., Zhang, R., Davies, K., Powers, C., et al. (2000). VEGF enhances angiogenesis and promotes blood-brain barrier leakage in the ischemic brain. J. Clin. Invest. 106, 829–838. doi: 10.1172/JCI9369
Keywords: vascular endothelial growth factor, endothelial progenitor cells, cerebral microvascular pathology, cerebral small vessel disease, aging, vascular dementia, dementia
Citation: Kapoor A, Gaubert A, Marshall A, Meier IB, Yew B, Ho JK, Blanken AE, Dutt S, Sible IJ, Li Y, Jang JY, Brickman AM, Rodgers K and Nation DA (2021) Increased Levels of Circulating Angiogenic Cells and Signaling Proteins in Older Adults With Cerebral Small Vessel Disease. Front. Aging Neurosci. 13:711784. doi: 10.3389/fnagi.2021.711784
Received: 19 May 2021; Accepted: 14 July 2021;
Published: 28 September 2021.
Edited by:
Thomas K. Karikari, University of Gothenburg, SwedenReviewed by:
Sina Naserian, INSERM UMR-S-MD 1197, Hôpital Paul Brousse, FranceCopyright © 2021 Kapoor, Gaubert, Marshall, Meier, Yew, Ho, Blanken, Dutt, Sible, Li, Jang, Brickman, Rodgers and Nation. This is an open-access article distributed under the terms of the Creative Commons Attribution License (CC BY). The use, distribution or reproduction in other forums is permitted, provided the original author(s) and the copyright owner(s) are credited and that the original publication in this journal is cited, in accordance with accepted academic practice. No use, distribution or reproduction is permitted which does not comply with these terms.
*Correspondence: Daniel A. Nation, ZG5hdGlvbkB1Y2kuZWR1
Disclaimer: All claims expressed in this article are solely those of the authors and do not necessarily represent those of their affiliated organizations, or those of the publisher, the editors and the reviewers. Any product that may be evaluated in this article or claim that may be made by its manufacturer is not guaranteed or endorsed by the publisher.
Research integrity at Frontiers
Learn more about the work of our research integrity team to safeguard the quality of each article we publish.