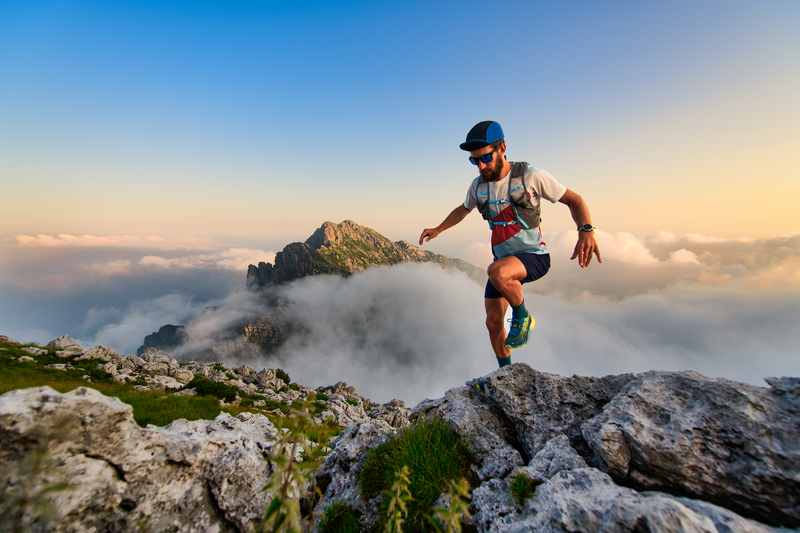
95% of researchers rate our articles as excellent or good
Learn more about the work of our research integrity team to safeguard the quality of each article we publish.
Find out more
REVIEW article
Front. Aging Neurosci. , 08 July 2021
Sec. Neuroinflammation and Neuropathy
Volume 13 - 2021 | https://doi.org/10.3389/fnagi.2021.689098
This article is part of the Research Topic Cerebral Edema Formation in Stroke: From Blood-Brain Barriers to Glymphatic System View all 9 articles
The glymphatic system (GS) is a novel defined brain-wide perivascular transit network between cerebrospinal fluid (CSF) and interstitial solutes that facilitates the clearance of brain metabolic wastes. The complicated network of the GS consists of the periarterial CSF influx pathway, astrocytes-mediated convective transport of fluid and solutes supported by AQP4 water channels, and perivenous efflux pathway. Recent researches indicate that the GS dysfunction is associated with various neurological disorders, including traumatic brain injury, hydrocephalus, epilepsy, migraine, and Alzheimer’s disease (AD). Meanwhile, the GS also plays a pivotal role in the pathophysiological process of stroke, including brain edema, blood–brain barrier (BBB) disruption, immune cell infiltration, neuroinflammation, and neuronal apoptosis. In this review, we illustrated the key anatomical structures of the GS, the relationship between the GS and the meningeal lymphatic system, the interaction between the GS and the BBB, and the crosstalk between astrocytes and other GS cellular components. In addition, we contributed to the current knowledge about the role of the GS in the pathology of stroke and the role of AQP4 in stroke. We further discussed the potential use of the GS in early risk assessment, diagnostics, prognostics, and therapeutics of stroke.
Clearing the metabolic wastes and maintaining the fluid homeostasis are important for brain function. In most organs, the lymphatic network is responsible for the wastes clearance and fluid drainage (Ikomi et al., 2012). However, a hallmark of the brain is the absence of typical lymphatic structures. Due to the presence of blood–brain barrier (BBB), the movement of solutes and ions in the brain is strictly restricted. Cerebrospinal fluid (CSF) has been considered to be important for the exchange of water-soluble metabolites; however, its mechanisms remain largely unknown. Iliff et al. (2012) reported the existence of the glymphatic system (GS) in the central nervous system (CNS), which is an alternative clearance system located in the perivascular space and aquaporin-4 (AQP4) dependent (Iliff et al., 2012). Emerging evidence from human studies and rodent models suggests that the GS is crucial for maintaining brain health, and dysfunction of GS is closely associated with various neurological disorders, including aging, neurodegeneration, and acute brain injury (de Leon et al., 2017; Ringstad et al., 2017). In parallel, the meningeal lymphatic vessels were discovered and demonstrated to participate in solutes transport and in immune surveillance (Aspelund et al., 2015; Louveau et al., 2015, 2016; Antila and Karaman, 2017).
Stroke, a major cause of death and disability, affects over 800,000 individuals annually (Coutts, 2017). It has been well-recognized that the GS plays a crucial role in the pathophysiology of stroke, including brain edema, blood–brain barrier (BBB) disruption, immune cell infiltration, neuroinflammation, and neuronal apoptosis (Ji et al., 2021). Targeting the GS, therefore, has provided potential for the early risk assessment, diagnosis, prognosis, and therapeutic of stroke. In this review, we summarize the latest research progress in the GS, including the anatomy and function, the interaction with the meningeal lymphatic systems and the BBB, and the communication between astrocytes and other GS cellular components. We emphasize the role of the GS in pathophysiology of different stroke subtypes, especially the role of AQP4 in the pathophysiology of stroke. In the end, we summarize the concerns and give some perspectives for future research.
The anatomy of the GS and its precise roles in fluid movement and drainage in the brain are complex. The GS is a glial-dependent fluid exchange and drainage system that comprises the entire perivascular space (PVS) network surrounding arteries, arterioles, capillaries, venules, and veins within the brain parenchyma. The PVS is constructed as a coaxial system where the inner cylinder is the cerebral vascular wall and the outer cylinder is the glial limitans that ensheathes the penetrating arterioles or perivascular astrocytic end-feet around the capillaries (Mathiisen et al., 2010). The GS mainly consists of periarterial CSF-inflow channel, perivenous ISF-outflow channel, and astrocytes-mediated convective transport of fluid and solutes supported by AQP4 water channels polarized on astrocytic end-feet (Benveniste et al., 2019). The patterns of fluid movement in the GS are similar to the classical Starling principle (Figure 1A), CSF from the subarachnoid space is propelled into the brain parenchyma via the PVS of penetrating arteries, also called Virchow–Robin space (Hannocks et al., 2018; Pizzo et al., 2018). CSF exchange with ISF then occurs through the glial basement membrane and astrocytic end-feet (Kress et al., 2014). From the interstitium, interstitial solutes and brain metabolic wastes flow into perivenous space and ultimately drain into lymph vessels existing in the meninges and perineural sheaths of cranial and spinal nerves to transport out of the CNS (Ma et al., 2017; Klarica et al., 2019). Currently, the glymphatic mechanism of solutes transport and wastes clearance is still unclear. The convective solutes transport and parenchymal diffusion transport are accepted by the majority of researchers (Smith et al., 2017; Smith and Verkman, 2018). What is particularly noteworthy is that the gap between astrocytic end-feet (gap width, 20–30 nm) traps larger molecular weight (MW) solutes and wastes during parenchymal CSF transport and drainage (Iliff et al., 2012). In addition, the GS is regulated by multiple factors, including arterial pulsations, respiratory pulsations, body position, and level of consciousness (Xie et al., 2013; Lee et al., 2015; Kiviniemi et al., 2016; Bedussi et al., 2018; Plog et al., 2019). However, there are limited investigations focusing on molecular mechanism that drives glymphatic fluid flow; more further studies are needed.
Figure 1. The anatomy and function of the GS in physiological and pathological conditions. (A) The GS mainly consists of periarterial CSF-inflow channel, perivenous ISF-outflow channel, and astrocytes-mediated convective transport of fluid and solutes. AQP4 polarized on astrocytic end-feet facilitates fluid and solutes exchange between the CSF and the brain interstitium. In physiological condition, CSF from the subarachnoid space is propelled into the brain parenchyma via the PVS of penetrating arteries. Then, CSF exchange with ISF in the extracellular space. Afterward, ISF and solutes move toward the perivenous space, ultimately drain out of the CNS via meningeal lymphatics. (B) After SAH, blood components invade the PVS rapidly, resulting in PVS occlusion and reduced CSF influx and ISF clearance. Furthermore, the perivascular polarity of AQP4 decreases after SAH, which resulted in accumulation of proinflammatory cytokines and neurotoxic solutes. In addition, AQP4 in the influx routes is upregulated markedly, while that in the efflux routes changes slightly. (C) In models of ICH, PVS is enlarged and responsible for brain edema. EPVS is also an independent risk factor for ICH recurrence. Moreover, glymphatic clearance rate is reduced, which contributes to the accumulation of proinflammatory cytokines and neurotoxic solutes. (D) In models of ischemic stroke, the ischemic spreading depolarizations along with subsequent vasoconstriction result in EPVS and doubled glymphatic inflow speeds. The increased influx of CSF in the GS contributes to poststroke edema. Additionally, the GS dysfunction after ischemic stroke impedes the clearance of neurotoxic solutes, proinflammation cytokines, and tau, which results in tissue damage and PSD. GS, glymphatic system; CSF, cerebrospinal fluid; ISF, interstitial fluid; PVS, perivascular space; CNS, central nervous system; SAH, subarachnoid hemorrhage; ICH, intracerebral hemorrhage; PSD, poststroke dementia.
Furthermore, there is evidence for the crucial role of AQP4 in the GS. AQP4 is located in chromosome 18 (18q11.2–q12.1), and there is growing consensus that AQP4 polarized at the perivascular astrocytic end-feet facilitates glymphatic fluid transport and amyloid-β (Aβ) export in rodents. Iliff et al. (2012) indicated that glymphatic clearance was significantly reduced in AQP4 knockout mice compared with that in normal littermates. Several independent groups have replicated the initial finding of AQP4-dependent glymphatic clearance and the crucial role of AQP4 in facilitating Aβ clearance (Xia et al., 2017; Mestre and Hablitz, 2018). Recent study confirmed that altered localization of AQP4 in aged rodent brain resulted in significantly increased parenchymal retention of adeno-associated viruses (AAV) vectors, which supported the importance of AQP4 in facilitating efficient glymphatic transport and clearance (Murlidharan et al., 2016). However, Smith et al. (2017) observed glymphatic clearance in AQP4 knockout rodent brain parenchyma and questioned the importance of AQP4 in the GS. Currently, except AQP4, no other astrocytic ion channels have been reported to be involved in the functional regulation of GS.
Another controversial topic is the drainage routes out of the CNS. Previous studies have found that perineural sheaths of the olfactory nerve passing through the cribriform plate and ultimately flowing into the cervical lymphatic vessels are primary solutes and wastes egress routes in both rodents and humans (Ma et al., 2017). Previous studies found that surgical blockade of the perineural cribriform pathway contributed to a rapid and sustained increase in intracranial pressure (Mollanji et al., 2002). Over the past few years, emerging studies have shown that the meningeal lymphatic systems are involved in the drainage of glymphatic fluid, and the anatomy and function of this drainage pathway are being defined (Aspelund et al., 2015; Louveau et al., 2015; Antila and Karaman, 2017; Da Mesquita et al., 2018; Ahn et al., 2019; Esposito et al., 2019; Song et al., 2020). Therefore, we will discuss its association with the GS in the following sections.
The meninges are composed of three layers: pia, arachnoid, and dura. Initially, similar to brain parenchyma, the meninges were long suggested to be devoid of lymphatic vessels. Aspelund et al. (2015) first described the structural and functional features of meningeal lymphatics and demonstrated that meningeal lymphatic vessels were mainly in the dura mater and well developed around the venous sinuses. Over the past decade, emerging evidence suggests that the meningeal lymphatics play a crucial role in macromolecular clearance, immune surveillance, and export of CSF/ISF from the CNS (Ahn et al., 2019; Alvesx de Lima et al., 2020; Song et al., 2020; Wojciechowski et al., 2020; Ding et al., 2021; Graham and Mellinghoff, 2021). Malfunction of meningeal lymphatics may cause the accumulation of toxic Aβ, cellular debris, inflammatory mediators, and immune cells, eventually resulting in neurological dysfunction and impacting neurological disease progression such as AD, traumatic brain injury, and subarachnoid hemorrhage (Da Mesquita et al., 2018; Bolte and Dutta, 2020; Chen and Wang, 2020; Mentis et al., 2021).
To elucidate the connection between the meningeal lymphatics and the GS, Louveau et al. (2015) administered fluorescent tracers into brain parenchyma and ventricles and observed tracers primarily along the meningeal lymphatic vessels that ultimately flowed into deep cervical lymph nodes (dcLNs). Furthermore, surgical ligation of the cervical lymphatic vessels enhanced tracers accumulation into the meningeal lymphatic network and prevented tracers from retention in dcLNs. These findings are supported by multiple studies using MRI and diffusion of radiolabeled tracers (Eide and Vatnehol, 2018; Alvesx de Lima et al., 2020; Zhou et al., 2020; Wu et al., 2021). These observations further indicate that meningeal lymphatics are the major efflux route for macromolecules, immune cells, and CSF/ISF. However, the exact proportions of different downstream routes of the GS, including meningeal lymphatics, perineural cribriform, and rostral migratory stream, are unknown (Goldmann et al., 2006; Kaminski et al., 2012; Ratnam et al., 2019). Hence, further investigation is required to elucidate the contribution and function of the different paths under both physiological and pathological statuses and to eventually understand how the GS links to downstream routes to maintain cerebral homeostasis.
In brain parenchyma clearance systems, besides the GS, other clearance mechanisms also have been observed, such as endocytosis and phagocytosis by pericytes, and transvascular clearance modulated by low-density lipoprotein receptor-related protein 1 (LRP1) (Kanekiyo and Bu, 2014). Indeed, studies have reported that BBB-associated pericytes could acquire a microglial phenotype following ischemic stroke (Özen et al., 2014). Ischemic brain injury increased the expression of microglial markers: IBA1, CD11b, and CD68 enhanced phagocytosis ability of pericytes to clear compromised cells (Özen et al., 2014). Pericytes also facilitate the clearance of soluble Aβ mediated by LRP1 (Ma et al., 2018). Moreover, it has been confirmed that brain endothelium enhanced the clearance of soluble brain Aβ in an LRP1-dependent manner (Storck et al., 2016). It is essential for these clearance systems to work in symbiosis to ensure the proper transport of solutes and brain homeostasis. In the future, further investigations should address the precise role of each clearance system in physiological and pathophysiological conditions and focus on the association of clearance systems with the meningeal lymphatics.
The GS is anatomically and functionally interconnected with the BBB, and together, they regulate the transport and exchange of fluid and solutes throughout the brain, thus maintaining brain homeostasis (Braun and Iliff, 2020).
The BBB is a multicellular vascular structure that insulates the neural tissue from the peripheral blood circulation. The BBB is composed of capillary endothelial cells, tight junctions between the adjacent endothelial cells, basement membrane, mural cells including pericytes and smooth muscle cells (SMCs), and astrocytes (Figure 2; Abbott et al., 2010). As mentioned above, the GS is a coaxial system that comprises the entire PVS network surrounding cerebral vessels. The inner cylinder of the GS is the cerebral vascular wall, while the outer cylinder is the glial limitans that ensheathes the penetrating arterioles or perivascular astrocytic end-feet around capillaries (Figure 2). Hence, there is some overlap in anatomical structures between the BBB and the GS. It is worth noting that BBB is a lateral physiological structure and places more emphasis on the selective permeability of substances, while the GS is a longitudinal physiological structure and places more emphasis on influx and efflux of CSF (Troili et al., 2020). Additionally, astrocytes play an essential role in both the BBB and the GS. Astrocytes take part in BBB maturation and maintenance by providing additional support such as perivascular astrocytic end-feet around capillaries and glial limitans around penetrating arterioles (Sweeney et al., 2019). In addition, astrocytes strengthen the tight junctions by regulating gene expression in endothelial cells via astrocyte–endothelial SHH pathway (Abbott et al., 2006). Astrocytes also enhance the basement membrane by producing laminin and stabilize pericytes via apolipoprotein E (ApoE) and low-density lipoprotein receptor-related protein 1 (LRP1) pathway (Bell et al., 2012). In the GS, astrocytes comprise the outer cylinder by astrocytic end-feet and glial limitans. Furthermore, AQP4-polarized astrocytes are necessary for the CSF/ISF exchange.
Figure 2. Interaction between the GS and the BBB. The GS is anatomically and functionally interconnected with the BBB. There is some overlap in anatomical structures between the BBB and the GS. At the arterial level (left inset), endothelial cells form the inner layer of the vascular wall. The basement membrane separates endothelium from SMCs. The basement membrane and SMCs are enveloped by the pia. The PVS is between the pia and the glia limitans formed by astrocytic end-feet. At the capillary level (right inset), pericytes and endothelial cells share a basement membrane. The PVS is between the basement membrane and astrocytic end-feet. The BBB regulates the exchange of molecules between the blood and the brain tissue via multiple transport systems. The GS regulates the exchange of fluid and solutes between the CSF and the ISF. GS, glymphatic system; BBB, blood–brain barrier; PVS, perivascular space; SMCs, smooth muscle cells.
The BBB regulates molecules in blood transported into and out of the brain tissue and prevents blood cells, neurotoxic plasma components, and pathogens from entering the brain tissue. Currently, several kinds of transport system presented in the BBB have been identified, such as carbohydrate transporters, amino acid transporters, monocarboxylate transporters, hormone transporters, fatty acid transporters, nucleotide transporters, and organic anion and cation transporters (Sweeney et al., 2019). These transport systems are regulated mainly based on brain endothelial cells and vascular pericytes. The GS also regulates the exchange of solutes, but this mainly occurs between CSF and brain tissue. The transport and exchange of molecules in the GS are mainly dependent on the gap between astrocytic end-feet and AQP4 polarized on astrocytic end-feet (Iliff et al., 2012). Meanwhile, both the BBB and the GS are critical for the clearance of metabolic wastes in the brain (Ueno et al., 2019). Metabolic wastes or neurotoxic substances in the brain parenchyma could be eliminated by flowing into the blood through efflux transporters at the BBB to the blood or ISF bulk flow clearance of the GS through PVS to the cervical lymph nodes (Ueno et al., 2019). The BBB and the GS may cooperatively play a significant role in the maintenance of cerebral homeostasis (Braun and Iliff, 2020). BBB breakdown will cause alteration of cell polarity and change in transport mechanisms, ultimately aggravating the GS dysfunction. Disruption in the GS will lead to the obstruction of drainage space, metabolite imbalance, and accumulation of toxic substances, which, in turn, deteriorates the BBB structurally and functionally. One recent study enrolled 109 participants with cerebral small vessel disease (cSVD) and explored the association of the GS dysfunction with BBB integrity (Li et al., 2019). The results demonstrated that high-grade enlarged PVS (EPVS) and the GS dysfunction were associated with a higher BBB leakage rate, supporting the hypothesis that the GS dysfunction is part of the pathological processes of compromised BBB integrity. Collectively, further studies are needed to verify the causal relationship between the increased BBB permeability and the GS dysfunction.
The major cellular components of the GS are astrocytes but also include other cells, such as neurons, microglia, and pericytes. Astrocytes constitute the physical barrier of the GS with their end-feet processes in the PVS (Filosa et al., 2016) and regulate the exchange and clearance of solutes between CSF and ISF through AQP4 localized on its end-feet. Up to 50% of the brain AQP4 is expressed on the astrocytic end-feet, which supported the key role of astrocytes in the GS (Hubbard et al., 2018; Plog and Nedergaard, 2018; Yin et al., 2018). Through communicating with neurons, microglia, and pericytes, astrocytes regulate synaptic transmission, modulate microglial phenotypes, and control fluid and ion homeostasis in physiological and pathological conditions of the CNS.
Astrocytes communicate closely with neurons. Previously, astrocytes were considered simply as a supportive function for neurons. Astrocytes protect neurons by releasing neurotrophic factors and antioxidants and by eliminating neuronal wastes (Wang et al., 2006). Astrocytes play a crucial role in synaptic transmission. It is estimated that a single astrocyte interacts with over 100,000 synapses, suggesting the close association between astrocytes and neurons (Bunney et al., 2017). Astrocytes also modulate neurotransmitter homeostasis through wrapping around presynapses and postsynapses to form a tripartite synapse (Allen and Barres, 2009; Sultan et al., 2015). Astrocytes also promote the neurogenesis via secreting D-serine (Sultan et al., 2013; MacKay et al., 2019), lactate (Zhou et al., 2019), interleukin-6 (IL-6) (Erta et al., 2015), interleukin-1 beta (IL-1β) (Jones et al., 2018), and growth factors, such as brain-derived neurotrophic factor (BDNF), fibroblast growth factor 2 (FGF-2), glial cell line-derived neurotrophic factor (GDNF), and vascular endothelial growth factor (VEGF) (Araki and Ikegaya, 2020). A recent research from rodent middle cerebral artery occlusion (MCAO) models demonstrates that, although astrocytes are not as mobile as microglia, they are able to polarize their distal processes without migration of cell body and phagocytose apoptotic bodies derived from dendrites of dying neurons in the infarct core (Damisah and Hill, 2020). What is more, a recent study demonstrated that decreased expression of astrocytic AQP4 accelerated deposition of α-synuclein and aggravated the loss of dopamine neurons via impairment of the GS (Cui et al., 2021). The decreased expression of astrocytic AQP4 is also associated with reduced dendritic spine density of cortical neurons (Venkat et al., 2017). Moreover, it has been proven that the depolarization of astrocytic AQP4 contributed to motor neuron degeneration (Dai et al., 2017).
In turn, astrocytes respond to the stimulation from neurons. Neurons, as a pacemaker of the neurovascular unit, detect very slight variations in nutrients and oxygen and then translate these changes into electrical signals and chemical messages to adjacent astrocytes (Figley and Stroman, 2011). It has been reported that astrocytic end-feet covered more than the 99% surface of cerebral blood vessels (Filosa et al., 2016). Neurons communicate with vessels via astrocytes, thus impacting the vascular tone and adjusting regional cerebral blood flow to provide proper supply of oxygen and nutrients (Muoio et al., 2014). Recent researches demonstrated that a high prevalence of pathological mitochondria in neurons increased the degree of astrogliosis and reduced the perivascular expression of AQP4 (Hasan-Olive et al., 2019). In general, further investigations that report the effect of neuron damage on the polarization of astrocytic AQP4 and the role of astrocytes in the GS are necessary.
Microglia comprise 5–10% of the brain cells and act as resident immune cells in the CNS (Frost and Schafer, 2016). There are two phenotypes being identified for microglia: M1 phenotype characterized by producing inflammatory mediators, including tumor necrosis factor (TNF), IL-1β, and reactive oxygen species (ROS), and M2 phenotype characterized by secreting anti-inflammatory mediators, including interleukin IL-10, transforming growth factor beta (TGFβ), and glucocorticoids (Orihuela et al., 2016). It has been reported that the interaction between activated microglia and reactive astrocytes plays an important role in neuroinflammation following stroke (Magaki et al., 2018). Microglia are activated via responding to pathogens and secreting cytokines, such as interleukin-1 alpha (IL-1α), TNF-α, and the complement component subunit 1q (C1q), thus triggering astrocyte reactions (Liddelow et al., 2017; Yun et al., 2018). In the intracerebral hemorrhage (ICH) mice model, microglial activation in the perihematomal region is found within 1 h after ICH was induced. Molecular signals produced by M1 phenotype, such as IL-1β, TNF, and IL-6, and inducible nitric oxide synthase (iNOS) are amplified both in human and rodent perihematomal brain tissues (Clausen et al., 2008; Wu et al., 2010; Liesz et al., 2011; Takaoka et al., 2016). Similarly, in the MCAO mice model, microglia are activated and invade the infarct core, simultaneously secreting cytokines IL-1β, TNF, and IL-1 receptor antagonist (IL-1Ra) (Clausen et al., 2008, 2016; Michelucci et al., 2009). In addition, microglia enhance astrocytes responses via Toll-like receptor 4 (TLR4) activation under insults, injury, or inflammation in stroke (Holm et al., 2012). Notably, although reactive astrocytes triggered by microglia activation are harmful to their function in the GS, an enhanced uptake and phagocytosis of astrocyte solutes and microglia may offset the impairment of glymphatic clearance (Feng et al., 2020).
Pericytes are the main mural cells that maintain the BBB integrity at the capillary level (Jeske et al., 2020; Zhang et al., 2020e). In the brain, astrocytes–pericytes interactions play a key role in regulating BBB permeability and cerebral blood flow and helping to facilitate the clearance of toxic substances. Astrocytes are the main cells expressing ApoE in the brain (Zhao et al., 2017). Previous studies demonstrated that ApoE4 astrocytes reduced Aβ clearance and increased cholesterol accumulation compared with ApoE3 astrocytes (Lin et al., 2018; Prasad and Rao, 2018). In addition, ApoE4 astrocytes aggravate pericytes degeneration and BBB breakdown via activating the cyclophylin A–nuclear factor kappa B (NF-κB)–matrix metalloproteinase 9 pathway in pericytes (Halliday et al., 2016; Sweeney et al., 2016). Furthermore, astrocytes promote cell contraction by increasing intracellular Ca2+ or K+ transients in pericytes (Mishra et al., 2016; Sweeney et al., 2016). Astrocytes have been reported to secrete prostaglandin E2 (PGE2) and activate PGE2 receptor 4 in pericytes to induce pericytes relaxation (MacVicar and Newman, 2015). Astrocytes regulate cell differentiation and maintain BBB integrity via secreting laminin α-2 chain (Lamα2) and interact with integrin α-2 receptor in pericytes (Yao et al., 2014). Conversely, pericytes regulate the polarization of astrocytic AQP4 (Gautam et al., 2020). In pathological conditions, pericyte deficiency reduces the expression of α-syntrophin, the component of dystrophin complex that regulates AQP4 anchoring, resulting in a redistribution of AQP4 on astrocytic end-feet where AQP4 moves away and is expressed onsite without vessel contact (Anderova et al., 2014; Gundersen et al., 2014).
The GS dysfunction has been demonstrated to be involved in the pathophysiology of brain edema, BBB disruption, neuroinflammation, and neuronal cell death after stroke. In this section, we summarize the new discoveries about function of the GS in pathophysiological process of hemorrhagic and ischemic stroke (Table 1).
Subarachnoid hemorrhage (SAH) is a devastating form of stroke often with permanent brain impairment. Currently, there is no effective intervention for preventing secondary neuropathological damage and improving the prognosis of SAH patients. Impaired CSF circulation along periarterial influx routes has been indicated in rodent models and gyrencephalic non-human primate model of SAH and has shed new light on translational therapeutic strategies (Gaberel et al., 2014; Goulay et al., 2017; Golanov et al., 2018; Liu et al., 2020a,b; Figure 1B). Following SAH, blood components particularly fibrin and fibrinogen deposit in PVS, which led to occlusion and dysfunction of the GS, ultimately worsening cerebral ischemia and edema (Goulay et al., 2017). Further studies confirmed that subarachnoid blood invaded the PVS within 5 min after SAH induction, gradually penetrating into the brain parenchyma in the following hours (Luo et al., 2016). They further demonstrated that the GS dysfunction following SAH resulted in vasculitis, widespread microinfarction, and neuroinflammation (Luo et al., 2016). In a more recent study, Pu et al. (2019) injected fluorescent tracers into the cisterna magna of SAH mice and found that the tracers that flowed into the brain parenchyma and drained to the dcLNs were significantly reduced after SAH induction. A further study found that the CSF influx into the brain and the ISF egress from the brain both significantly decreased after SAH (Liu et al., 2020a). In non-human primates, Goulay et al. (2017) injected gadolinium chelate into the cisterna magna and evaluated the parenchymal CSF circulation in healthy or SAH induction status using living MRI. They found a gradual active distribution of CSF from cerebral ventricles to the superficial part of the brain and then to deeper structures of the brain in normal physiological status, whereas SAH induction significantly impaired parenchymal CSF circulation (Goulay et al., 2017). Collectively, these observations support the evidence that GS dysfunction is an important pathophysiological feature and associated with secondary brain injury and neurological deficits following SAH. Interestingly, it has been reported that clearance of the PVS with tissue-type plasminogen activator could alleviate histological injury and improve behavioral deficits of SAH (Luo et al., 2016; Bosche and Mergenthaler, 2020). Therefore, targeting the GS potentially serves as a novel strategy for the treatment of SAH. However, further studies are necessary to investigate the underlying molecular mechanisms governing the link between SAH and the GS.
Intracerebral hemorrhage (ICH) is another severe hemorrhagic stroke subtype with high risk of death and disability (Charidimou et al., 2017). The research related to the association of the GS with ICH is limited. Several studies reported the role of EPVS in the pathology of ICH. As mentioned above, PVS is a key anatomical structure of the GS. Normally, the diameter cutoff of PVS is set as 3 mm (Zhu et al., 2011; Wardlaw et al., 2013, 2020; Dubost et al., 2019). Previous studies demonstrated that EPVS was linked to impaired glymphatic clearance (Mestre et al., 2017; Boespflug et al., 2018; Opel et al., 2019). The GS dysfunction contributes to the accumulation of metabolic wastes and neurotoxic substances in PVS, ultimately resulting in EPVS (Figure 1C). Therefore, EPVS is a marker of the GS dysfunction. EPVS has been indicated to be associated with perforator arteriopathy and emerged as a marker of small vessel disease (SVD) (Charidimou et al., 2017; Brown et al., 2018; Jokinen et al., 2020). Recent studies provide new evidence that EPVS is linked to an increased risk of ICH. One study followed 1,678 participants for 10 years and found that increasing global EPVS burden was associated with a higher risk of incident ICH (Duperron et al., 2019). In another study, researchers included 1,386 patients with atrial fibrillation receiving oral anticoagulants (OAC) after a recent transient ischemic attack (TIA) or ischemic stroke and followed subjects for a mean of 2.3 years while assessing EPVS with MRI and found that EPVS was an independent risk factor for symptomatic ICH in patients receiving OAC (Best et al., 2020). However, the underlying mechanisms leading to the transformation from EPVS to symptomatic ICH remain unclear. Recent research has further demonstrated that EPVS was associated with ICH recurrence (Raposo and Viswanathan, 2020). During the recovery process of ICH, one study examined the connection between EPVS and Aβ deposition through MRI and 18F-florbetapir PET, respectively, and demonstrated that EPVS that appears in the centrum semiovale was the marker of vascular Aβ burden and enhanced the risk of poor prognosis (Raposo et al., 2019). Therefore, there is no doubt that EPVS is of great clinical significance in the pathology of ICH, and further investigations need to illustrate the molecular mechanism of EPVS aggravating the pathological process of ICH.
Findings from the current studies indicate that the GS is involved in the pathology of ischemic stroke (Figure 1D). First, the GS dysfunction is a predictor of incidence of ischemic stroke. According to accumulating brain MRI and pathological studies, EPVS has been identified as the risk of stroke (Zhang et al., 2020d; Bu et al., 2021).
Second, CSF circulation is impaired after ischemic stroke, although there is still lack of consensus on the dynamic change in CSF flow in the GS. In murine models of acute ischemic stroke (AIS), CSF inflow in the ipsilateral cortex has been verified to be impaired at 3 h after AIS induction via MRI and histological examination (Gaberel et al., 2014). Consistently, researchers found that the influx of CSF was slow even 7 days after ischemic stroke (Lin et al., 2020). However, a recent study found a conflicting conclusion; researchers discovered that the influx of CSF in the GS increased rapidly within minutes of an ischemic insult (Mestre and Du, 2020). The ischemic spreading depolarizations along with subsequent vasoconstriction might be responsible for EPVS and doubled glymphatic inflow speeds (Mestre and Du, 2020). Meanwhile, the increased CSF flow in the GS was considered to be the primary cause of acute ischemic tissue swelling (Mestre and Du, 2020). These findings revised our understanding of poststroke edema.
Notably, the reduced glymphatic clearance after ischemic stroke is defined. Researchers injected fluorescent tracers into the infarction area of a rodent MCAO stereotactically to evaluate the clearance of solutes, and the results showed that tracers were trapped in the infarct core (Zbesko et al., 2018). Further results from Yang et al. (2015) are consistent with this finding (Lin et al., 2020). They used contrast-enhanced MRI by injecting Gd-DTPA into the cisterna magna to assess the GS function in the acute and subacute phases of ischemic stroke induced by MCAO. They evaluated the time course of the signal-to-noise ratio (SNR) in the substantia nigra (SNe) and ventral thalamic nucleus (VTN) and found that the SNR time-to-peak on the ipsilateral side was longer in SN both in the acute phase and in the subacute phase than in the contralateral phase (Lin et al., 2020). In addition, the GS dysfunction contributes to the accumulation of toxic solutes and proinflammatory cytokines within the core infarction area. It is well known that damaged brain tissue after AIS goes through the stage of liquefactive necrosis, which produces poisonous extracellular fluid. One study demonstrated that extracellular fluid present in areas of liquefactive necrosis following ischemic stroke was injurious to primary cultured cortical and hippocampal neurons even after 7 weeks following stroke (Zbesko et al., 2018). Interestingly, a recent study found that overexpression of slit2 alleviated neuronal excitotoxicity and improved cognition via accelerating glymphatic clearance after ischemic stroke (He et al., 2020). This study provided a new evidence for potential therapeutic role of the GS in ischemic stroke.
Furthermore, the GS is beneficial to the restoration phase of ischemic stroke. The GS dysfunction is related to poststroke dementia (PSD), which is one of the most common and severe consequences of stroke (Pantoni, 2017). Deposition and hyperphosphorylation of tau have been implicated as the main pathophysiology underlying PSD (Zhao et al., 2014; Qiu et al., 2016). Recently, a study demonstrated that the parenchymal infiltration of CSF tracers injected into the cisterna magna was attenuated in the PSD model and that tau clearance was obstructed, suggesting that GS malfunction was a risk factor for the incidence of PSD (Back et al., 2020). Therapeutic strategies to improve the clearance of brain metabolic wastes, including tau, may be a promising approach to prevent PSD after stroke.
Collectively, the GS plays an essential role in the pathology of ischemic stroke; further studies should investigate the role of the GS in different phases of ischemic stroke so as to develop alternative treatment strategies for ischemic stroke.
Aquaporin-4, the most important molecular component in the GS, mediates the influx of CSF into the brain parenchyma and the efflux of ISF into the subarachnoid space. AQP4 is involved in multiple biological processes, including the regulation of brain edema, promotion of migration of astrocytes, calcium signal transduction, and synaptic plasticity (Chu et al., 2016). In this section, we review the expression of AQP4 in different subtypes of stroke and then examine the signaling pathways that regulate AQP4 expression and discuss therapeutic opportunities that target AQP4 (Figure 3).
Figure 3. The change in AQP4 expression after stroke. AQP4 mislocalization and an increase in total AQP4 expression appear in all subtypes of stroke; however, the perivascular polarity of AQP4 decreased. The matrix constituent agrin and α-syntrophin play an important role in anchoring of AQP4 to astrocytic end-feet. After stroke, multiple factors increase the expression of AQP4, including glutamate VEGF, G-CSF, EPO, RSG5, Nrf2, lncRNA MALAT1, and Cav1. Moreover, activation of MAPK pathway is responsible for AQP4 upregulation. AQP4 mislocalization and expression change are involved in the GS dysfunction, BBB disruption, brain edema, and neuronal apoptosis induced by stroke.
Previous studies have revealed that the expression level of AQP4 messenger RNA (mRNA) and protein increased at day 1 and peaked at day 3 after SAH (Long et al., 2019). One study indicated that AQP4 maintained high level even at 7 days after SAH (Luo et al., 2016).
The role of AQP4 in the pathophysiological process of SAH is complex. Several recent studies validated that AQP4 was responsible for brain edema following SAH. Zhang et al. (2020a) demonstrated that glutamate might be responsible for the elevation of AQP4 following SAH and that metabotropic glutamate receptor 1 (mGluR1) negative allosteric modulator could reduce BBB damage and cerebral edema via inhibition of AQP4. Another study found that treatment with pituitary adenylate cyclase-activating polypeptide (PACAP) inhibited the expression of AQP4 and attenuated brain edema (Fang et al., 2020). Additionally, salvinorin A and baicalin were confirmed to reduce SAH-induced brain edema via AQP4 inhibition (Sun et al., 2018; Zhang et al., 2020c). Furthermore, AQP4 inhibition preserved the function of the BBB and the GS and provided neurological benefits (Fang et al., 2020).
Some other studies reported the neuroprotective role of AQP4 in early brain injury (EBI) following SAH. Given that AQP4 facilitated ISF transport in the brain parenchyma to eliminate the toxic factors, AQP4 knockout has been shown to aggravate EBI following SAH through impairment of the GS (Liu et al., 2020a,b). Pu et al. (2019) also demonstrated that the perivascular polarity of AQP4 decreased after SAH, which resulted in accumulations of tau proteins and CD3+, CD4+, and CD8+ cells, and led to a series of pathological changes, including microvascular spasm, activation of glial cells, neuroinflammation, and neuronal apoptosis (El Amki et al., 2018). Meanwhile, the length density of AQP4-positive capillaries in the hippocampus was significantly reduced following SAH (Anzabi et al., 2018). One potential explanation for the controversial role of AQP4 in SAH is that the expression of AQP4 in the influx and efflux routes of the GS is different. After SAH, astrocytes surrounding the arteries were activated to increase the expression of AQP4; however, the expression of AQP4 around the veins changed slightly (Liu et al., 2020b). Therefore, the inflow of CSF from periarterial space (PAS) increased highly due to the enhanced level of AQP4, and the ISF volume expanded owing to the unchanged expression of AQP4 around the veins.
It has been reported that AQP4 expression increased from 3 h after ICH and peaked between day 2 and 5 (Tang et al., 2010; Fang et al., 2015; Wang et al., 2015; Huang et al., 2020). Several studies demonstrated that VEGF, granulocyte-colony stimulating factor (G-CSF), and erythropoietin (EPO) contributed to the increase in AQP4 via the activation of the c-JUN N-terminal kinase (JNK) pathway, extracellular signal-regulated kinase (ERK) pathway, and mitogen-activated protein kinase (MAPK) pathway, respectively (Chu et al., 2013, 2014a,b).
Aquaporin-4 is involved in brain edema, BBB disruption, and neuronal apoptosis induced by ICH. It has been demonstrated that AQP4 is responsible for the explosive swelling of astrocytes and their dysfunction after ICH (Appelboom et al., 2015). Zhang et al. (2020b) observed that inhibition of the expression of AQP4 with glycyl-l-histidyl-l-lysine (GHK) could alleviate the injury of astrocytes and brain edema. Moreover, a different group also showed that AQP4 deletion reduced brain edema (Chen et al., 2020).
Several studies reported the opposite role of AQP4 in ICH. Tang et al. (2010) observed that AQP4 deletion aggravated brain edema. Meanwhile, AQP4 deletion resulted in swelling capillary endothelial cells and disruption of tight junctions (Chu et al., 2014a). Recently, in a rodent intracerebral hematoma expansion model, Chu et al. (2020) found that AQP4 deletion was associated with larger hematoma volume and more severe BBB disruption, which indicated that AQP4 reduced the hematoma volume and neurological deficits via the maintenance of BBB integrity. Furthermore, the presence of AQP4 alleviated neuronal apoptosis after ICH via inhibition of cytokines, especially TNF-α and IL-1β. These results indicate that AQP4 may have a protective effect on brain edema, BBB disruption, and neuronal apoptosis (Chu et al., 2014c). Therefore, it is difficult to draw definite conclusions about the effects of AQP4 in ICH. The mislocalization of astrocytic AQP4 may contribute to its controversial role in ICH (Qiu et al., 2015).
Currently, a series of studies reported AQP4 expression after ischemic stroke. The continuous and dynamic observation carried out in transient MCAO demonstrated that AQP4 mRNA and protein were upregulated at 30 min after ischemia and that this lasted at least 72 h and normalized after 28 days (Badaut et al., 2007). Another study using the transient MCAO model found that there were two peaks of AQP4 expression: 1 and 48 h (Ribeiro Mde et al., 2006). AQP4 expression was positively correlated to the regulator of G protein signaling 5 (RGS5), transcriptional factor Nrf2, long non-coding RNA (lncRNA) MALAT1, and caveolin-1 (Cav-1) after ischemic stroke (Özen et al., 2018; Liu et al., 2019; Filchenko et al., 2020; Wang et al., 2020). Furthermore, MAPK pathways have been proven to play a pivotal role in AQP4 upregulation, and the activation of protein kinase C (PKC) pathway may be responsible for AQP4 downregulation (Nito et al., 2012; Wei et al., 2015).
Aquaporin-4 plays a complex bimodal function in the pathology of ischemic stroke. Most of the studies found that AQP4 inhibition, including AQP4 knockout or AQP4 gene silence using small interfering RNA (siRNA), reduced brain edema in different cerebral ischemia models. Manley et al. (2000) first showed that brain edema was decreased in AQP4 knockout mice at 24 h after permanent MCAO. Then, several studies reveal similar results (Katada et al., 2014; He and Lu, 2015; Yang et al., 2015; Yao et al., 2015; Özen et al., 2018; Liu et al., 2019; Filchenko et al., 2020; Wang et al., 2020). One study found that treatment with AQP4 inhibitor TGN-020 in MCAO mice reduced brain edema and infarct volumes through blocking fluid flow toward the parenchyma in the perivascular drainage pathways (Pirici et al., 2017). Moreover, AQP4 knockout decreased mortality, increased motor recovery, and improved long-term outcome after transient MCAO (Hirt et al., 2017).
Several studies reported the opposite role of AQP4 in the pathology of ischemic stroke. One study reported that AQP4 knockout resulted in a striking hypertrophy of astrocytes and aggravated brain injury with enlarged infarct size and a serious loss of CA1 neurons (Zeng et al., 2012). Moreover, AQP4 knockout showed more severe neutrophil infiltration and microglial activation but less astrocyte proliferation in the brain after MCAO compared to wild-type mice (Shi et al., 2012). The dual role of AQP4 in ischemic stroke is mainly due to its spatial expression heterogeneity. Studies from cerebral infarction patients demonstrated that AQP4 expression increased only in white matter, while cortical astrocytes exhibited reduced perivascular AQP4 (Stokum et al., 2015). Back et al. (2017) also found that AQP4 distribution changed from perivessel to parenchyma. Moreover, a recent study showed that impaired perivascular AQP4 covering after ischemia was associated with altered reactive astrocyte morphology and enhanced brain edema. In summary, the effects of AQP4 on the pathophysiological process of ischemic stroke are very complex, and more studies are needed to further investigate the spatial and temporal differences of AQP4 expression and its role in different phase of ischemic stroke.
The CSF/ISF exchange and the transport systems for brain solutes and metabolic wastes have gained significant attention in recent years. Currently, the GS has become prominent in AD research by demonstrating its role in Aβ clearance. Several key proteins involved in neuroinflammation and cognitive decline, such as tau and cytokines, are also believed to be removed by the GS, supporting its potential role in the recovery stage of stroke. Information from human and rodent studies confirmed that the GS dysfunction caused a risk of stroke and was involved in the pathology of stroke. However, the molecular mechanisms of the interaction between the GS and stroke are not completely understood. First, we should clarify factors that impair or enhance the GS function in order to map the time course of pathological changes in the GS function during stroke. Second, we have already documented the existence of the GS through MRI and two-photon microscope, but novel neuroimaging modalities able to assess the function state of the GS have not yet been taken advantage of. Furthermore, high spatial resolution imaging and mathematical models are necessary to understand the CNS interstitial space. Third, it is not clear what kind of pathophysiological changes following stroke influence the redistribution of astrocytic AQP4. For instance, it is not known whether there are additional astrocytic ion channels, apart from AQP4, that facilitate the CSF/ISF exchange and metabolic wastes clearance. Finally, it has been established that glymphatic clearance is primarily active during sleep (Shokri-Kojori et al., 2018; Holth and Fritschi, 2019). Coincidentally, most stroke patients suffer from circadian rhythm disorders (Pérez-Carbonell and Bashir, 2020). Therefore, it is not clear whether improving the sleep quality of stroke patients is an effective approach to promoting recovery. In addition, some studies have shown that sleep-promoting states improved brain-wide drug distribution (Plog et al., 2018; Lilius et al., 2019). In the future, more studies should focus on the relevance of intrathecal delivery of drugs to the GS function. Anyway, a comprehensive understanding of GS will provide novel molecular markers for the prognosis and diagnosis of stroke, and promising therapeutic targets.
XZ and QH designed and conceptualized the review. TL drafted and revised the manuscript. BZ retrieved and screened the review. All authors contributed to the manuscript and approved the final version.
This work was supported by grants from the National Natural Science Foundation of China (Nos. 81971093 to XZ and 82071283 to QH).
The authors declare that the research was conducted in the absence of any commercial or financial relationships that could be construed as a potential conflict of interest.
Abbott, N. J., Patabendige, A. A., Dolman, D. E., Yusof, S. R., and Begley, D. J. (2010). Structure and function of the blood-brain barrier. Neurobiol. Dis. 37, 13–25. doi: 10.1016/j.nbd.2009.07.030
Abbott, N. J., Rönnbäck, L., and Hansson, E. (2006). Astrocyte-endothelial interactions at the blood-brain barrier. Nat. Rev. Neurosci. 7, 41–53. doi: 10.1038/nrn1824
Ahn, J. H., Cho, H., Kim, J. H., Kim, S. H., Ham, J. S., Park, I., et al. (2019). Meningeal lymphatic vessels at the skull base drain cerebrospinal fluid. Nature 572, 62–66. doi: 10.1038/s41586-019-1419-1415
Allen, N. J., and Barres, B. A. (2009). Neuroscience: Glia - more than just brain glue. Nature 457, 675–677. doi: 10.1038/457675a
Alves, de Lima, K., Rustenhoven, J., and Kipnis, J. (2020). Meningeal immunity and its function in maintenance of the central nervous system in health and disease. Annu. Rev. Immunol. 38, 597–620. doi: 10.1146/annurev-immunol-102319-103410
Anderova, M., Benesova, J., Mikesova, M., Dzamba, D., Honsa, P., Kriska, J., et al. (2014). Altered astrocytic swelling in the cortex of α-syntrophin-negative GFAP/EGFP mice. PLoS One 9:e113444. doi: 10.1371/journal.pone.0113444
Antila, S., and Karaman, S. (2017). Development and plasticity of meningeal lymphatic vessels. J. Exp. Med. 214, 3645–3667. doi: 10.1084/jem.20170391
Anzabi, M., Ardalan, M., Iversen, N. K., Rafati, A. H., Hansen, B., and Østergaard, L. (2018). Hippocampal atrophy following subarachnoid hemorrhage correlates with disruption of astrocyte morphology and capillary coverage by AQP4. Front. Cell Neurosci. 12:19. doi: 10.3389/fncel.2018.00019
Appelboom, G., Bruce, S., Duren, A., Piazza, M., Monahan, A., Christophe, B., et al. (2015). Aquaporin-4 gene variant independently associated with oedema after intracerebral haemorrhage. Neurol. Res. 37, 657–661. doi: 10.1179/1743132815y.0000000047
Araki, T., and Ikegaya, Y. (2020). The effects of microglia- and astrocyte-derived factors on neurogenesis in health and disease. Eur. J. Neurosci. doi: 10.1111/ejn.14969 Online ahead of print.
Aspelund, A., Antila, S., Proulx, S. T., Karlsen, T. V., Karaman, S., Detmar, M., et al. (2015). A dural lymphatic vascular system that drains brain interstitial fluid and macromolecules. J. Exp. Med. 212, 991–999. doi: 10.1084/jem.20142290
Back, D. B., Choi, B. R., and Han, J. S. (2020). Characterization of tauopathy in a rat model of post-stroke dementia combining acute infarct and chronic cerebral hypoperfusion. Int. J. Mol. Sci. 21:6929. doi: 10.3390/ijms21186929
Back, D. B., Kwon, K. J., Choi, D. H., Shin, C. Y., Lee, J., Han, S. H., et al. (2017). Chronic cerebral hypoperfusion induces post-stroke dementia following acute ischemic stroke in rats. J. Neuroinflamm. 14:216. doi: 10.1186/s12974-017-0992-995
Badaut, J., Ashwal, S., Tone, B., Regli, L., Tian, H. R., and Obenaus, A. (2007). Temporal and regional evolution of aquaporin-4 expression and magnetic resonance imaging in a rat pup model of neonatal stroke. Pediatr. Res. 62, 248–254. doi: 10.1203/PDR.0b013e3180db291b
Bedussi, B., Almasian, M., de Vos, J., VanBavel, E., and Bakker, E. N. (2018). Paravascular spaces at the brain surface: low resistance pathways for cerebrospinal fluid flow. J. Cereb. Blood Flow Metab. 38, 719–726. doi: 10.1177/0271678x17737984
Bell, R. D., Winkler, E. A., Singh, I., Sagare, A. P., Deane, R., Wu, Z., et al. (2012). Apolipoprotein E controls cerebrovascular integrity via cyclophilin a. Nature 485, 512–516. doi: 10.1038/nature11087
Benveniste, H., Liu, X., Koundal, S., Sanggaard, S., Lee, H., and Wardlaw, J. (2019). The glymphatic system and waste clearance with brain aging: a review. Gerontology 65, 106–119. doi: 10.1159/000490349
Best, J. G., Barbato, C., Ambler, G., and Du, H. (2020). Association of enlarged perivascular spaces and anticoagulant-related intracranial hemorrhage. Neurology 95, e2192–e2199. doi: 10.1212/wnl.0000000000010788
Boespflug, E. L., Simon, M. J., Leonard, E., Grafe, M., Woltjer, R., Silbert, L. C., et al. (2018). Targeted assessment of enlargement of the perivascular space in alzheimer’s disease and vascular dementia subtypes implicates astroglial involvement specific to Alzheimer’s disease. J. Alzheimers. Dis. 66, 1587–1597. doi: 10.3233/jad-180367
Bolte, A. C., and Dutta, A. B. (2020). Meningeal lymphatic dysfunction exacerbates traumatic brain injury pathogenesis. Nat. Commun. 11:4524. doi: 10.1038/s41467-020-18113-18114
Bosche, B., and Mergenthaler, P. (2020). Complex clearance mechanisms after intraventricular hemorrhage and rt-PA treatment-a review on clinical trials. Transl. Stroke Res. 11, 337–344. doi: 10.1007/s12975-019-00735-736
Braun, M., and Iliff, J. J. (2020). The impact of neurovascular, blood-brain barrier, and glymphatic dysfunction in neurodegenerative and metabolic diseases. Int. Rev. Neurobiol. 154, 413–436. doi: 10.1016/bs.irn.2020.02.006
Brown, R., Benveniste, H., Black, S. E., Charpak, S., Dichgans, M., Joutel, A., et al. (2018). Understanding the role of the perivascular space in cerebral small vessel disease. Cardiovasc. Res. 114, 1462–1473. doi: 10.1093/cvr/cvy113
Bu, N., Khlif, M. S., Lemmens, R., Wouters, A., Fiebach, J. B., Chamorro, A., et al. (2021). Imaging markers of brain frailty and outcome in patients with acute ischemic stroke. Stroke 52, 1004–1011. doi: 10.1161/strokeaha.120.029841
Bunney, P. E., Zink, A. N., Holm, A. A., Billington, C. J., and Kotz, C. M. (2017). Orexin activation counteracts decreases in nonexercise activity thermogenesis (NEAT) caused by high-fat diet. Physiol. Behav. 176, 139–148. doi: 10.1016/j.physbeh.2017.03.040
Charidimou, A., Imaizumi, T., Moulin, S., Biffi, A., Samarasekera, N., Yakushiji, Y., et al. (2017). Brain hemorrhage recurrence, small vessel disease type, and cerebral microbleeds: a meta-analysis. Neurology 89, 820–829. doi: 10.1212/wnl.0000000000004259
Chen, J., and Wang, L. (2020). Meningeal lymphatics clear erythrocytes that arise from subarachnoid hemorrhage. Nat. Commun. 11:3159. doi: 10.1038/s41467-020-16851-z
Chen, X., Deng, S., Lei, Q., He, Q., Ren, Y., Zhang, Y., et al. (2020). miR-7-5p affects brain edema after intracerebral hemorrhage and its possible mechanism. Front. Cell Dev. Biol. 8:598020. doi: 10.3389/fcell.2020.598020
Chu, H., Ding, H., Tang, Y., and Dong, Q. (2014a). Erythropoietin protects against hemorrhagic blood-brain barrier disruption through the effects of aquaporin-4. Lab. Invest. 94, 1042–1053. doi: 10.1038/labinvest.2014.84
Chu, H., Tang, Y., and Dong, Q. (2014b). Protection of granulocyte-colony stimulating factor to hemorrhagic brain injuries and its involved mechanisms: effects of vascular endothelial growth factor and aquaporin-4. Neuroscience 260, 59–72. doi: 10.1016/j.neuroscience.2013.12.017
Chu, H., Xiang, J., Wu, P., Su, J., Ding, H., Tang, Y., et al. (2014c). The role of aquaporin 4 in apoptosis after intracerebral hemorrhage. J. Neuroinflamm. 11:184. doi: 10.1186/s12974-014-0184-185
Chu, H., Gao, Z., Huang, C., Dong, J., Tang, Y., and Dong, Q. (2020). Relationship between hematoma expansion induced by hypertension and hyperglycemia and blood-brain barrier disruption in mice and its possible mechanism: role of Aquaporin-4 and Connexin43. Neurosci. Bull. 36, 1369–1380. doi: 10.1007/s12264-020-00540-544
Chu, H., Huang, C., Ding, H., Dong, J., Gao, Z., Yang, X., et al. (2016). Aquaporin-4 and cerebrovascular diseases. Int. J. Mol. Sci. 17:1249. doi: 10.3390/ijms17081249
Chu, H., Tang, Y., and Dong, Q. (2013). Protection of vascular endothelial growth factor to brain edema following intracerebral hemorrhage and its involved mechanisms: effect of Aquaporin-4. PLoS One 8:e66051. doi: 10.1371/journal.pone.0066051
Clausen, B. H., Lambertsen, K. L., Babcock, A. A., Holm, T. H., Dagnaes-Hansen, F., and Finsen, B. (2008). Interleukin-1beta and tumor necrosis factor-alpha are expressed by different subsets of microglia and macrophages after ischemic stroke in mice. J. Neuroinflamm. 5:46. doi: 10.1186/1742-2094-5-46
Clausen, B. H., Lambertsen, K. L., Dagnæs-Hansen, F., Babcock, A. A., von Linstow, C. U., Meldgaard, M., et al. (2016). Cell therapy centered on IL-1Ra is neuroprotective in experimental stroke. Acta Neuropathol. 131, 775–791. doi: 10.1007/s00401-016-1541-1545
Coutts, S. B. (2017). Diagnosis and management of transient ischemic attack. Continuum (Minneap Minn) 23, 82–92. doi: 10.1212/con.0000000000000424
Cui, H., Wang, W., Zheng, X., Xia, D., Liu, H., Qin, C., et al. (2021). Decreased AQP4 expression aggravates α-Synuclein pathology in parkinson’s disease mice, possibly via impaired glymphatic clearance. J. Mol. Neurosci. doi: 10.1007/s12031-021-01836-1834 Online ahead of print.
Da Mesquita, S., Louveau, A., Vaccari, A., Smirnov, I., Cornelison, R. C., Kingsmore, K. M., et al. (2018). Functional aspects of meningeal lymphatics in ageing and Alzheimer’s disease. Nature 560, 185–191. doi: 10.1038/s41586-018-0368-368
Dai, J., Lin, W., Zheng, M., Liu, Q., He, B., Luo, C., et al. (2017). Alterations in AQP4 expression and polarization in the course of motor neuron degeneration in SOD1G93A mice. Mol. Med. Rep. 16, 1739–1746. doi: 10.3892/mmr.2017.6786
Damisah, E. C., and Hill, R. A. (2020). Astrocytes and microglia play orchestrated roles and respect phagocytic territories during neuronal corpse removal in vivo. Sci. Adv. 6:eaba3239. doi: 10.1126/sciadv.aba3239
de Leon, M. J., Li, Y., Okamura, N., Tsui, W. H., Saint-Louis, L. A., Glodzik, L., et al. (2017). Cerebrospinal fluid clearance in alzheimer disease measured with dynamic PET. J. Nucl. Med. 58, 1471–1476. doi: 10.2967/jnumed.116.187211
Ding, X. B., Wang, X. X., and Xia, D. H. (2021). Impaired meningeal lymphatic drainage in patients with idiopathic Parkinson’s disease. Nat. Med. 27, 411–418. doi: 10.1038/s41591-020-01198-1191
Dubost, F., Adams, H., Bortsova, G., Ikram, M. A., Niessen, W., Vernooij, M., et al. (2019). 3D regression neural network for the quantification of enlarged perivascular spaces in brain MRI. Med. Image Anal. 51, 89–100. doi: 10.1016/j.media.2018.10.008
Duperron, M. G., Tzourio, C., Schilling, S., Zhu, Y. C., Soumaré, A., Mazoyer, B., et al. (2019). High dilated perivascular space burden: a new MRI marker for risk of intracerebral hemorrhage. Neurobiol. Aging 84, 158–165. doi: 10.1016/j.neurobiolaging.2019.08.031
Eide, P. K., and Vatnehol, S. A. S. (2018). Magnetic resonance imaging provides evidence of glymphatic drainage from human brain to cervical lymph nodes. Sci. Rep. 8:7194. doi: 10.1038/s41598-018-25666-25664
El Amki, M., Dubois, M., Lefevre-Scelles, A., Magne, N., Roussel, M., Clavier, T., et al. (2018). Long-Lasting cerebral vasospasm, microthrombosis, apoptosis and paravascular alterations associated with neurological deficits in a mouse model of subarachnoid hemorrhage. Mol. Neurobiol. 55, 2763–2779. doi: 10.1007/s12035-017-0514-516
Erta, M., Giralt, M., Esposito, F. L., Fernandez-Gayol, O., and Hidalgo, J. (2015). Astrocytic IL-6 mediates locomotor activity, exploration, anxiety, learning and social behavior. Horm. Behav. 73, 64–74. doi: 10.1016/j.yhbeh.2015.06.016
Esposito, E., Ahn, B. J., Shi, J., and Nakamura, Y. (2019). Brain-to-cervical lymph node signaling after stroke. Nat. Commun. 10:5306. doi: 10.1038/s41467-019-13324-w
Fang, J., Li, H., Li, G., and Wang, L. (2015). Effect of hyperbaric oxygen preconditioning on peri-hemorrhagic focal edema and aquaporin-4 expression. Exp. Ther. Med. 10, 699–704. doi: 10.3892/etm.2015.2539
Fang, Y., Shi, H., Ren, R., Huang, L., Okada, T., Lenahan, C., et al. (2020). Pituitary adenylate cyclase-activating polypeptide attenuates brain edema by protecting blood-brain barrier and glymphatic system after subarachnoid hemorrhage in rats. Neurotherapeutics 17, 1954–1972. doi: 10.1007/s13311-020-00925-923
Feng, W., Zhang, Y., Wang, Z., Xu, H., Wu, T., Marshall, C., et al. (2020). Microglia prevent beta-amyloid plaque formation in the early stage of an Alzheimer’s disease mouse model with suppression of glymphatic clearance. Alzheimers Res. Ther. 12:125. doi: 10.1186/s13195-020-00688-681
Figley, C. R., and Stroman, P. W. (2011). The role(s) of astrocytes and astrocyte activity in neurometabolism, neurovascular coupling, and the production of functional neuroimaging signals. Eur. J. Neurosci. 33, 577–588. doi: 10.1111/j.1460-9568.2010.07584.x
Filchenko, I., Blochet, C., Buscemi, L., Price, M., Badaut, J., and Hirt, L. (2020). Caveolin-1 regulates perivascular aquaporin-4 expression after cerebral ischemia. Front. Cell Dev. Biol. 8:371. doi: 10.3389/fcell.2020.00371
Filosa, J. A., Morrison, H. W., Iddings, J. A., Du, W., and Kim, K. J. (2016). Beyond neurovascular coupling, role of astrocytes in the regulation of vascular tone. Neuroscience 323, 96–109. doi: 10.1016/j.neuroscience.2015.03.064
Frost, J. L., and Schafer, D. P. (2016). Microglia: architects of the developing nervous system. Trends Cell Biol. 26, 587–597. doi: 10.1016/j.tcb.2016.02.006
Gaberel, T., Gakuba, C., Goulay, R., Martinez, De Lizarrondo, S., Hanouz, J. L., et al. (2014). Impaired glymphatic perfusion after strokes revealed by contrast-enhanced MRI: a new target for fibrinolysis? Stroke 45, 3092–3096. doi: 10.1161/strokeaha.114.006617
Garland, P., Morton, M., Zolnourian, A., Durnford, A., Gaastra, B., Toombs, J., et al. (2021). Neurofilament light predicts neurological outcome after subarachnoid haemorrhage. Brain 144, 761–768. doi: 10.1093/brain/awaa451
Gautam, J., Cao, Y., and Yao, Y. (2020). Pericytic laminin maintains blood-brain barrier integrity in an age-dependent manner. Transl. Stroke Res. 11, 228–242. doi: 10.1007/s12975-019-00709-708
Golanov, E. V., Bovshik, E. I., Wong, K. K., Pautler, R. G., Foster, C. H., Federley, R. G., et al. (2018). Subarachnoid hemorrhage - Induced block of cerebrospinal fluid flow: role of brain coagulation factor III (tissue factor). J. Cereb. Blood Flow Metab. 38, 793–808. doi: 10.1177/0271678x17701157
Goldmann, J., Kwidzinski, E., Brandt, C., Mahlo, J., Richter, D., and Bechmann, I. (2006). T cells traffic from brain to cervical lymph nodes via the cribroid plate and the nasal mucosa. J. Leukoc. Biol. 80, 797–801. doi: 10.1189/jlb.0306176
Goulay, R., Flament, J., Gauberti, M., Naveau, M., Pasquet, N., Gakuba, C., et al. (2017). Subarachnoid hemorrhage severely impairs brain parenchymal cerebrospinal fluid circulation in nonhuman primate. Stroke 48, 2301–2305. doi: 10.1161/strokeaha.117.017014
Graham, M. S., and Mellinghoff, I. K. (2021). Meningeal lymphatics prime tumor immunity in glioblastoma. Cancer Cell 39, 304–306. doi: 10.1016/j.ccell.2021.02.012
Gundersen, G. A., Vindedal, G. F., Skare, O., and Nagelhus, E. A. (2014). Evidence that pericytes regulate aquaporin-4 polarization in mouse cortical astrocytes. Brain Struct. Funct. 219, 2181–2186. doi: 10.1007/s00429-013-0629-620
Halliday, M. R., Rege, S. V., Ma, Q., Zhao, Z., Miller, C. A., Winkler, E. A., et al. (2016). Accelerated pericyte degeneration and blood-brain barrier breakdown in apolipoprotein E4 carriers with Alzheimer’s disease. J. Cereb. Blood Flow Metab. 36, 216–227. doi: 10.1038/jcbfm.2015.44
Hannocks, M. J., Pizzo, M. E., Huppert, J., Deshpande, T., Abbott, N. J., Thorne, R. G., et al. (2018). Molecular characterization of perivascular drainage pathways in the murine brain. J. Cereb. Blood Flow Metab. 38, 669–686. doi: 10.1177/0271678x17749689
Hasan-Olive, M. M., Enger, R., Hansson, H. A., Nagelhus, E. A., and Eide, P. K. (2019). Pathological mitochondria in neurons and perivascular astrocytic endfeet of idiopathic normal pressure hydrocephalus patients. Fluids Barriers CNS 16:39. doi: 10.1186/s12987-019-0160-167
He, X. F., Li, G., Li, L. L., Li, M. Y., Liang, F. Y., Chen, X., et al. (2020). Overexpression of Slit2 decreases neuronal excitotoxicity, accelerates glymphatic clearance, and improves cognition in a multiple microinfarcts model. Mol. Brain 13:135. doi: 10.1186/s13041-020-00659-655
He, Z. P., and Lu, H. (2015). Aquaporin-4 gene silencing protects injured neurons after early cerebral infarction. Neural. Regen. Res. 10, 1082–1087. doi: 10.4103/1673-5374.160099
Hirt, L., Fukuda, A. M., Ambadipudi, K., Rashid, F., Binder, D., Verkman, A., et al. (2017). Improved long-term outcome after transient cerebral ischemia in aquaporin-4 knockout mice. J. Cereb. Blood Flow Metab. 37, 277–290. doi: 10.1177/0271678x15623290
Holm, T. H., Draeby, D., and Owens, T. (2012). Microglia are required for astroglial Toll-like receptor 4 response and for optimal TLR2 and TLR3 response. Glia 60, 630–638. doi: 10.1002/glia.22296
Holth, J. K., and Fritschi, S. K. (2019). The sleep-wake cycle regulates brain interstitial fluid tau in mice and CSF tau in humans. Science 363, 880–884. doi: 10.1126/science.aav2546
Huang, P., He, X. Y., and Xu, M. (2020). Protease-activated receptor 1 inhibitor improves brain edema in rats with intracerebral hemorrhage. Bratisl. Lek. Listy 121, 600–604. doi: 10.4149/bll_2020_099
Hubbard, J. A., Szu, J. I., and Binder, D. K. (2018). The role of aquaporin-4 in synaptic plasticity, memory and disease. Brain Res. Bull. 136, 118–129. doi: 10.1016/j.brainresbull.2017.02.011
Ikomi, F., Kawai, Y., and Ohhashi, T. (2012). Recent advance in lymph dynamic analysis in lymphatics and lymph nodes. Ann. Vasc. Dis. 5, 258–268. doi: 10.3400/avd.ra.12.00046
Iliff, J. J., Wang, M., Liao, Y., Plogg, B. A., Peng, W., Gundersen, G. A., et al. (2012). A paravascular pathway facilitates CSF flow through the brain parenchyma and the clearance of interstitial solutes, including amyloid β. Sci. Transl. Med. 4:147ra111. doi: 10.1126/scitranslmed.3003748
Jeske, R., Albo, J., Marzano, M., Bejoy, J., and Li, Y. (2020). Engineering brain-specific pericytes from human pluripotent stem cells. Tissue Eng. Part B Rev. 26, 367–382. doi: 10.1089/ten.TEB.2020.0091
Ji, C., Yu, X., Xu, W., Lenahan, C., Tu, S., and Shao, A. (2021). The role of glymphatic system in the cerebral edema formation after ischemic stroke. Exp. Neurol. 340:113685. doi: 10.1016/j.expneurol.2021.113685
Jokinen, H., Koikkalainen, J., Laakso, H. M., Melkas, S., Nieminen, T., Brander, A., et al. (2020). Global burden of small vessel disease-related brain changes on MRI predicts cognitive and functional decline. Stroke 51, 170–178. doi: 10.1161/strokeaha.119.026170
Jones, M. E., Lebonville, C. L., Paniccia, J. E., Balentine, M. E., Reissner, K. J., and Lysle, D. T. (2018). Hippocampal interleukin-1 mediates stress-enhanced fear learning: a potential role for astrocyte-derived interleukin-1β. Brain Behav. Immun. 67, 355–363. doi: 10.1016/j.bbi.2017.09.016
Kaminski, M., Bechmann, I., Pohland, M., Kiwit, J., Nitsch, R., and Glumm, J. (2012). Migration of monocytes after intracerebral injection at entorhinal cortex lesion site. J. Leukoc. Biol. 92, 31–39. doi: 10.1189/jlb.0511241
Kanekiyo, T., and Bu, G. (2014). The low-density lipoprotein receptor-related protein 1 and amyloid-β clearance in Alzheimer’s disease. Front. Aging Neurosci. 6:93. doi: 10.3389/fnagi.2014.00093
Katada, R., Akdemir, G., Asavapanumas, N., Ratelade, J., Zhang, H., and Verkman, A. S. (2014). Greatly improved survival and neuroprotection in aquaporin-4-knockout mice following global cerebral ischemia. FASEB J. 28, 705–714. doi: 10.1096/fj.13-231274
Kiviniemi, V., Wang, X., Korhonen, V., Keinänen, T., Tuovinen, T., Autio, J., et al. (2016). Ultra-fast magnetic resonance encephalography of physiological brain activity - Glymphatic pulsation mechanisms? J. Cereb. Blood Flow Metab. 36, 1033–1045. doi: 10.1177/0271678x15622047
Klarica, M., Radoš, M., and Orešković, D. (2019). The movement of cerebrospinal fluid and its relationship with substances behavior in cerebrospinal and interstitial fluid. Neuroscience 414, 28–48. doi: 10.1016/j.neuroscience.2019.06.032
Kress, B. T., Iliff, J. J., Xia, M., Wang, M., Wei, H. S., Zeppenfeld, D., et al. (2014). Impairment of paravascular clearance pathways in the aging brain. Ann. Neurol. 76, 845–861. doi: 10.1002/ana.24271
Lee, H., Xie, L., Yu, M., Kang, H., Feng, T., Deane, R., et al. (2015). The effect of body posture on brain glymphatic transport. J. Neurosci. 35, 11034–11044. doi: 10.1523/jneurosci.1625-15.2015
Li, Y., Li, M., Yang, L., Qin, W., Yang, S., Yuan, J., et al. (2019). The relationship between blood-brain barrier permeability and enlarged perivascular spaces: a cross-sectional study. Clin. Interv. Aging 14, 871–878. doi: 10.2147/cia.s204269
Liddelow, S. A., Guttenplan, K. A., Clarke, L. E., Bennett, F. C., Bohlen, C. J., Schirmer, L., et al. (2017). Neurotoxic reactive astrocytes are induced by activated microglia. Nature 541, 481–487. doi: 10.1038/nature21029
Liesz, A., Middelhoff, M., Zhou, W., Karcher, S., Illanes, S., and Veltkamp, R. (2011). Comparison of humoral neuroinflammation and adhesion molecule expression in two models of experimental intracerebral hemorrhage. Exp. Transl. Stroke Med. 3:11. doi: 10.1186/2040-7378-3-11
Lilius, T. O., Blomqvist, K., Hauglund, N. L., Liu, G., Stæger, F. F., Bærentzen, S., et al. (2019). Dexmedetomidine enhances glymphatic brain delivery of intrathecally administered drugs. J. Control. Release 304, 29–38. doi: 10.1016/j.jconrel.2019.05.005
Lin, L., Hao, X., Li, C., Sun, C., Wang, X., Yin, L., et al. (2020). Impaired glymphatic system in secondary degeneration areas after ischemic stroke in rats. J. Stroke Cerebrovasc. Dis. 29:104828. doi: 10.1016/j.jstrokecerebrovasdis.2020.104828
Lin, Y. T., Seo, J., Gao, F., Feldman, H. M., Wen, H. L., Penney, J., et al. (2018). APOE4 causes widespread molecular and cellular alterations associated with Alzheimer’s disease phenotypes in human iPSC-Derived brain cell types. Neuron 98, 1141–1154.e7. doi: 10.1016/j.neuron.2018.05.008
Liu, E., Peng, X., Ma, H., Zhang, Y., Yang, X., Zhang, Y., et al. (2020a). The involvement of aquaporin-4 in the interstitial fluid drainage impairment following subarachnoid hemorrhage. Front. Aging Neurosci. 12:611494. doi: 10.3389/fnagi.2020.611494
Liu, E., Sun, L., Zhang, Y., Wang, A., and Yan, J. (2020b). Aquaporin4 knockout aggravates early brain injury following subarachnoid hemorrhage through impairment of the glymphatic system in rat brain. Acta Neurochir. Suppl. 127, 59–64. doi: 10.1007/978-3-030-04615-6_10
Liu, L., Kelly, M. G., Wierzbicki, E. L., Escober-Nario, I. C., Vollmer, M. K., and Doré, S. (2019). Nrf2 plays an essential role in long-term brain damage and neuroprotection of korean red ginseng in a permanent cerebral ischemia model. Antioxidants (Basel) 8:273. doi: 10.3390/antiox8080273
Long, C. Y., Huang, G. Q., Du, Q., Zhou, L. Q., and Zhou, J. H. (2019). The dynamic expression of aquaporins 1 and 4 in rats with hydrocephalus induced by subarachnoid haemorrhage. Folia Neuropathol. 57, 182–195. doi: 10.5114/fn.2019.86296
Louveau, A., Da Mesquita, S., and Kipnis, J. (2016). Lymphatics in neurological disorders: a neuro-lympho-vascular component of multiple sclerosis and alzheimer’s disease? Neuron 91, 957–973. doi: 10.1016/j.neuron.2016.08.027
Louveau, A., Smirnov, I., Keyes, T. J., Eccles, J. D., Rouhani, S. J., Peske, J. D., et al. (2015). Structural and functional features of central nervous system lymphatic vessels. Nature 523, 337–341. doi: 10.1038/nature14432
Luo, C., Yao, X., Li, J., He, B., Liu, Q., Ren, H., et al. (2016). Paravascular pathways contribute to vasculitis and neuroinflammation after subarachnoid hemorrhage independently of glymphatic control. Cell Death Dis. 7:e2160. doi: 10.1038/cddis.2016.63
Ma, Q., Ineichen, B. V., and Detmar, M. (2017). Outflow of cerebrospinal fluid is predominantly through lymphatic vessels and is reduced in aged mice. Nat. Commun. 8:1434. doi: 10.1038/s41467-017-01484-1486
Ma, Q., Zhao, Z., Sagare, A. P., Wu, Y., Wang, M., Owens, N. C., et al. (2018). Blood-brain barrier-associated pericytes internalize and clear aggregated amyloid-β42 by LRP1-dependent apolipoprotein E isoform-specific mechanism. Mol. Neurodegener. 13:57. doi: 10.1186/s13024-018-0286-280
MacKay, M. B., Kravtsenyuk, M., Thomas, R., Mitchell, N. D., Dursun, S. M., and Baker, G. B. (2019). D-Serine: potential therapeutic agent and/or biomarker in schizophrenia and depression? Front. Psychiatry 10:25. doi: 10.3389/fpsyt.2019.00025
MacVicar, B. A., and Newman, E. A. (2015). Astrocyte regulation of blood flow in the brain. Cold Spring Harb. Perspect. Biol. 7:a020388. doi: 10.1101/cshperspect.a020388
Magaki, S. D., Williams, C. K., and Vinters, H. V. (2018). Glial function (and dysfunction) in the normal & ischemic brain. Neuropharmacology 134(Pt B), 218–225. doi: 10.1016/j.neuropharm.2017.11.009
Manley, G. T., Fujimura, M., Ma, T., Noshita, N., Filiz, F., Bollen, A. W., et al. (2000). Aquaporin-4 deletion in mice reduces brain edema after acute water intoxication and ischemic stroke. Nat. Med. 6, 159–163. doi: 10.1038/72256
Mathiisen, T. M., Lehre, K. P., Danbolt, N. C., and Ottersen, O. P. (2010). The perivascular astroglial sheath provides a complete covering of the brain microvessels: an electron microscopic 3D reconstruction. Glia 58, 1094–1103. doi: 10.1002/glia.20990
Mentis, A. A., Dardiotis, E., and Chrousos, G. P. (2021). Apolipoprotein E4 and meningeal lymphatics in Alzheimer disease: a conceptual framework. Mol. Psychiatry 26, 1075–1097. doi: 10.1038/s41380-020-0731-737
Mestre, H., and Du, T. (2020). Cerebrospinal fluid influx drives acute ischemic tissue swelling. Science 367:eaax7171. doi: 10.1126/science.aax7171
Mestre, H., and Hablitz, L. M. (2018). Aquaporin-4-dependent glymphatic solute transport in the rodent brain. eLife 7:e40070. doi: 10.7554/eLife.40070
Mestre, H., Kostrikov, S., Mehta, R. I., and Nedergaard, M. (2017). Perivascular spaces, glymphatic dysfunction, and small vessel disease. Clin. Sci. (Lond) 131, 2257–2274. doi: 10.1042/cs20160381
Michelucci, A., Heurtaux, T., Grandbarbe, L., Morga, E., and Heuschling, P. (2009). Characterization of the microglial phenotype under specific pro-inflammatory and anti-inflammatory conditions: effects of oligomeric and fibrillar amyloid-beta. J. Neuroimmunol. 210, 3–12. doi: 10.1016/j.jneuroim.2009.02.003
Mishra, A., Reynolds, J. P., Chen, Y., Gourine, A. V., and Rusakov, D. A. (2016). Astrocytes mediate neurovascular signaling to capillary pericytes but not to arterioles. Nat. Neurosci. 19, 1619–1627. doi: 10.1038/nn.4428
Mollanji, R., Bozanovic-Sosic, R., Zakharov, A., Makarian, L., and Johnston, M. G. (2002). Blocking cerebrospinal fluid absorption through the cribriform plate increases resting intracranial pressure. Am. J. Physiol. Regul. Integr. Comp. Physiol. 282, R1593–R1599. doi: 10.1152/ajpregu.00695.2001
Muoio, V., Persson, P. B., and Sendeski, M. M. (2014). The neurovascular unit - concept review. Acta Physiol. (Oxf) 210, 790–798. doi: 10.1111/apha.12250
Murlidharan, G., Crowther, A., Reardon, R. A., Song, J., and Asokan, A. (2016). Glymphatic fluid transport controls paravascular clearance of AAV vectors from the brain. JCI Insight 1:e88034. doi: 10.1172/jci.insight.88034
Nito, C., Kamada, H., Endo, H., Narasimhan, P., Lee, Y. S., and Chan, P. H. (2012). Involvement of mitogen-activated protein kinase pathways in expression of the water channel protein aquaporin-4 after ischemia in rat cortical astrocytes. J. Neurotrauma 29, 2404–2412. doi: 10.1089/neu.2012.2430
Opel, R. A., Christy, A., Boespflug, E. L., Weymann, K. B., Case, B., and Pollock, J. M. (2019). Effects of traumatic brain injury on sleep and enlarged perivascular spaces. J. Cereb. Blood Flow Metab. 39, 2258–2267. doi: 10.1177/0271678x18791632
Orihuela, R., McPherson, C. A., and Harry, G. J. (2016). Microglial M1/M2 polarization and metabolic states. Br. J. Pharmacol. 173, 649–665. doi: 10.1111/bph.13139
Özen, I., Deierborg, T., Miharada, K., Padel, T., Englund, E., Genové, G., et al. (2014). Brain pericytes acquire a microglial phenotype after stroke. Acta Neuropathol. 128, 381–396. doi: 10.1007/s00401-014-1295-x
Özen, I., Roth, M., Barbariga, M., Gaceb, A., Deierborg, T., Genové, G., et al. (2018). Loss of regulator of G-Protein signaling 5 leads to neurovascular protection in stroke. Stroke 49, 2182–2190. doi: 10.1161/strokeaha.118.020124
Pantoni, L. (2017). Have stroke neurologists entered the arena of stroke-related cognitive dysfunctions? not yet, but they should! Stroke 48, 1441–1442. doi: 10.1161/strokeaha.117.016869
Pérez-Carbonell, L., and Bashir, S. (2020). Narrative review of sleep and stroke. J. Thorac. Dis. 12, (Suppl. 2), S176–S190. doi: 10.21037/jtd-cus-2020-2022
Pirici, I., Balsanu, T. A., and Bogdan, C. (2017). Inhibition of Aquaporin-4 improves the outcome of ischaemic stroke and modulates brain paravascular drainage pathways. Int. J. Mol. Sci. 19:46. doi: 10.3390/ijms19010046
Pizzo, M. E., Wolak, D. J., Kumar, N. N., Brunette, E., Brunnquell, C. L., Hannocks, M. J., et al. (2018). Intrathecal antibody distribution in the rat brain: surface diffusion, perivascular transport and osmotic enhancement of delivery. J. Physiol. 596, 445–475. doi: 10.1113/jp275105
Plog, B. A., Lou, N., Pierre, C. A., Cove, A., Kenney, H. M., Hitomi, E., et al. (2019). When the air hits your brain: decreased arterial pulsatility after craniectomy leading to impaired glymphatic flow. J. Neurosurg. doi: 10.3171/2019.2.jns182675 Online ahead of print.
Plog, B. A., Mestre, H., Olveda, G. E., Sweeney, A. M., Kenney, H. M., Cove, A., et al. (2018). Transcranial optical imaging reveals a pathway for optimizing the delivery of immunotherapeutics to the brain. JCI Insight 3:e120922. doi: 10.1172/jci.insight.120922
Plog, B. A., and Nedergaard, M. (2018). The glymphatic system in central nervous system health and disease: past, present, and future. Annu. Rev. Pathol. 13, 379–394. doi: 10.1146/annurev-pathol-051217-111018
Prasad, H., and Rao, R. (2018). Amyloid clearance defect in ApoE4 astrocytes is reversed by epigenetic correction of endosomal pH. Proc. Natl. Acad. Sci. U S A. 115, E6640–E6649. doi: 10.1073/pnas.1801612115
Pu, T., Zou, W., Feng, W., Zhang, Y., Wang, L., Wang, H., et al. (2019). Persistent malfunction of glymphatic and meningeal lymphatic drainage in a mouse model of subarachnoid hemorrhage. Exp. Neurobiol. 28, 104–118. doi: 10.5607/en.2019.28.1.104
Qiu, G. P., Xu, J., Zhuo, F., Sun, S. Q., Liu, H., Yang, M., et al. (2015). Loss of AQP4 polarized localization with loss of β-dystroglycan immunoreactivity may induce brain edema following intracerebral hemorrhage. Neurosci. Lett. 588, 42–48. doi: 10.1016/j.neulet.2014.12.053
Qiu, L., Ng, G., Tan, E. K., Liao, P., Kandiah, N., and Zeng, L. (2016). Chronic cerebral hypoperfusion enhances Tau hyperphosphorylation and reduces autophagy in Alzheimer’s disease mice. Sci. Rep. 6:23964. doi: 10.1038/srep23964
Raposo, N., Planton, M., Payoux, P., Péran, P., Albucher, J. F., Calviere, L., et al. (2019). Enlarged perivascular spaces and florbetapir uptake in patients with intracerebral hemorrhage. Eur. J. Nucl. Med. Mol. Imaging 46, 2339–2347. doi: 10.1007/s00259-019-04441-4441
Raposo, N., and Viswanathan, A. (2020). MRI-visible enlarged perivascular spaces: beyond microbleeds to predict intracerebral hemorrhage. Neurology 95, 709–710. doi: 10.1212/wnl.0000000000010790
Ratnam, N. M., Gilbert, M. R., and Giles, A. J. (2019). Immunotherapy in CNS cancers: the role of immune cell trafficking. Neuro Oncol. 21, 37–46. doi: 10.1093/neuonc/noy084
Ribeiro Mde, C., Hirt, L., Bogousslavsky, J., Regli, L., and Badaut, J. (2006). Time course of aquaporin expression after transient focal cerebral ischemia in mice. J. Neurosci. Res. 83, 1231–1240. doi: 10.1002/jnr.20819
Ringstad, G., Vatnehol, S. A. S., and Eide, P. K. (2017). Glymphatic MRI in idiopathic normal pressure hydrocephalus. Brain 140, 2691–2705. doi: 10.1093/brain/awx191
Shi, W. Z., Zhao, C. Z., Zhao, B., Shi, Q. J., Zhang, L. H., Wang, Y. F., et al. (2012). Aggravated inflammation and increased expression of cysteinyl leukotriene receptors in the brain after focal cerebral ischemia in AQP4-deficient mice. Neurosci. Bull. 28, 680–692. doi: 10.1007/s12264-012-1281-z
Shokri-Kojori, E., Wang, G. J., Wiers, C. E., Demiral, S. B., Guo, M., Kim, S. W., et al. (2018). β-Amyloid accumulation in the human brain after one night of sleep deprivation. Proc. Natl. Acad. Sci. U S A. 115, 4483–4488. doi: 10.1073/pnas.1721694115
Smith, A. J., and Verkman, A. S. (2018). The “glymphatic” mechanism for solute clearance in Alzheimer’s disease: game changer or unproven speculation? Faseb J. 32, 543–551. doi: 10.1096/fj.201700999
Smith, A. J., Yao, X., Dix, J. A., Jin, B. J., and Verkman, A. S. (2017). Test of the ‘glymphatic’ hypothesis demonstrates diffusive and aquaporin-4-independent solute transport in rodent brain parenchyma. eLife 6:e27679. doi: 10.7554/eLife.27679
Song, E., Mao, T., Dong, H., Boisserand, L. S. B., Antila, S., Bosenberg, M., et al. (2020). VEGF-C-driven lymphatic drainage enables immunosurveillance of brain tumours. Nature 577, 689–694. doi: 10.1038/s41586-019-1912-x
Stokum, J. A., Mehta, R. I., Ivanova, S., Yu, E., Gerzanich, V., and Simard, J. M. (2015). Heterogeneity of aquaporin-4 localization and expression after focal cerebral ischemia underlies differences in white versus grey matter swelling. Acta Neuropathol. Commun. 3:61. doi: 10.1186/s40478-015-0239-236
Storck, S. E., Meister, S., Nahrath, J., Meißner, J. N., Schubert, N., Di Spiezio, A., et al. (2016). Endothelial LRP1 transports amyloid-β(1-42) across the blood-brain barrier. J. Clin. Invest. 126, 123–136. doi: 10.1172/jci81108
Sultan, S., Gebara, E. G., Moullec, K., and Toni, N. (2013). D-serine increases adult hippocampal neurogenesis. Front. Neurosci. 7:155. doi: 10.3389/fnins.2013.00155
Sultan, S., Li, L., Moss, J., Petrelli, F., Cassé, F., Gebara, E., et al. (2015). Synaptic integration of adult-born hippocampal neurons is locally controlled by astrocytes. Neuron 88, 957–972. doi: 10.1016/j.neuron.2015.10.037
Sun, J., Zhang, Y., Lu, J., Zhang, W., Yan, J., Yang, L., et al. (2018). Salvinorin A ameliorates cerebral vasospasm through activation of endothelial nitric oxide synthase in a rat model of subarachnoid hemorrhage. Microcirculation 25:e12442. doi: 10.1111/micc.12442
Sweeney, M. D., Ayyadurai, S., and Zlokovic, B. V. (2016). Pericytes of the neurovascular unit: key functions and signaling pathways. Nat. Neurosci. 19, 771–783. doi: 10.1038/nn.4288
Sweeney, M. D., Zhao, Z., Montagne, A., Nelson, A. R., and Zlokovic, B. V. (2019). Blood-Brain barrier: from physiology to disease and back. Physiol. Rev. 99, 21–78. doi: 10.1152/physrev.00050.2017
Takaoka, Y., Takahashi, M., Kurauchi, Y., Hisatsune, A., Seki, T., Shudo, K., et al. (2016). Retinoic acid receptor agonist Am80 inhibits CXCL2 production from microglial BV-2 cells via attenuation of NF-κB signaling. Int. Immunopharmacol. 38, 367–376. doi: 10.1016/j.intimp.2016.06.025
Tang, Y., Wu, P., Su, J., Xiang, J., Cai, D., and Dong, Q. (2010). Effects of Aquaporin-4 on edema formation following intracerebral hemorrhage. Exp. Neurol. 223, 485–495. doi: 10.1016/j.expneurol.2010.01.015
Troili, F., Cipollini, V., Moci, M., Morena, E., Palotai, M., Rinaldi, V., et al. (2020). Perivascular unit: this must be the place. the anatomical crossroad between the immune, vascular and nervous system. Front. Neuroanat. 14:17. doi: 10.3389/fnana.2020.00017
Ueno, M., Chiba, Y., Murakami, R., Matsumoto, K., Fujihara, R., Uemura, N., et al. (2019). Disturbance of intracerebral fluid clearance and blood-brain barrier in vascular cognitive impairment. Int. J. Mol. Sci. 20:2600. doi: 10.3390/ijms20102600
Venkat, P., Chopp, M., Zacharek, A., Cui, C., Zhang, L., Li, Q., et al. (2017). White matter damage and glymphatic dysfunction in a model of vascular dementia in rats with no prior vascular pathologies. Neurobiol. Aging 50, 96–106. doi: 10.1016/j.neurobiolaging.2016.11.002
Wang, A. T., Lee, S. S., Sigman, M., and Dapretto, M. (2006). Developmental changes in the neural basis of interpreting communicative intent. Soc. Cogn. Affect. Neurosci. 1, 107–121. doi: 10.1093/scan/nsl018
Wang, B. F., Cui, Z. W., Zhong, Z. H., Sun, Y. H., Sun, Q. F., Yang, G. Y., et al. (2015). Curcumin attenuates brain edema in mice with intracerebral hemorrhage through inhibition of AQP4 and AQP9 expression. Acta Pharmacol. Sin. 36, 939–948. doi: 10.1038/aps.2015.47
Wang, H., Zheng, X., Jin, J., Zheng, L., Guan, T., Huo, Y., et al. (2020). LncRNA MALAT1 silencing protects against cerebral ischemia-reperfusion injury through miR-145 to regulate AQP4. J. Biomed. Sci. 27:40. doi: 10.1186/s12929-020-00635-630
Wang, M., Ding, F., Deng, S., Guo, X., Wang, W., Iliff, J. J., et al. (2017). Focal solute trapping and global glymphatic pathway impairment in a murine model of multiple microinfarcts. J. Neurosci. 37, 2870–2877. doi: 10.1523/jneurosci.2112-16.2017
Wardlaw, J. M., Benveniste, H., Nedergaard, M., and Zlokovic, B. V. (2020). Perivascular spaces in the brain: anatomy, physiology and pathology. Nat. Rev. Neurol. 16, 137–153. doi: 10.1038/s41582-020-0312-z
Wardlaw, J. M., Smith, E. E., Biessels, G. J., Cordonnier, C., Fazekas, F., Frayne, R., et al. (2013). Neuroimaging standards for research into small vessel disease and its contribution to ageing and neurodegeneration. Lancet Neurol. 12, 822–838. doi: 10.1016/s1474-4422(13)70124-70128
Wei, X., Zhang, B., Cheng, L., Chi, M., Deng, L., Pan, H., et al. (2015). Hydrogen sulfide induces neuroprotection against experimental stroke in rats by down-regulation of AQP4 via activating PKC. Brain Res. 1622, 292–299. doi: 10.1016/j.brainres.2015.07.001
Wojciechowski, S., Virenque, A., Vihma, M., Galbardi, B., Rooney, E. J., Keuters, M. H., et al. (2020). Developmental dysfunction of the central nervous system lymphatics modulates the adaptive neuro-immune response in the perilesional cortex in a mouse model of traumatic brain injury. Front. Immunol. 11:559810. doi: 10.3389/fimmu.2020.559810
Wu, C. H., Lirng, J. F., Ling, Y. H., Wang, Y. F., Wu, H. M., Fuh, J. L., et al. (2021). Noninvasive characterization of human glymphatics and meningeal lymphatics in an in vivo model of blood-brain barrier leakage. Ann. Neurol. 89, 111–124. doi: 10.1002/ana.25928
Wu, H., Zhang, Z., Hu, X., Zhao, R., Song, Y., Ban, X., et al. (2010). Dynamic changes of inflammatory markers in brain after hemorrhagic stroke in humans: a postmortem study. Brain Res. 1342, 111–117. doi: 10.1016/j.brainres.2010.04.033
Xia, M., Yang, L., Sun, G., Qi, S., and Li, B. (2017). Mechanism of depression as a risk factor in the development of Alzheimer’s disease: the function of AQP4 and the glymphatic system. Psychopharmacology (Berl) 234, 365–379. doi: 10.1007/s00213-016-4473-4479
Xie, L., Kang, H., Xu, Q., Chen, M. J., Liao, Y., Thiyagarajan, M., et al. (2013). Sleep drives metabolite clearance from the adult brain. Science 342, 373–377. doi: 10.1126/science.1241224
Yang, C., Liu, Z., Li, H., Zhai, F., Liu, J., and Bian, J. (2015). Aquaporin-4 knockdown ameliorates hypoxic-ischemic cerebral edema in newborn piglets. IUBMB Life 67, 182–190. doi: 10.1002/iub.1356
Yao, X., Derugin, N., Manley, G. T., and Verkman, A. S. (2015). Reduced brain edema and infarct volume in aquaporin-4 deficient mice after transient focal cerebral ischemia. Neurosci. Lett. 584, 368–372. doi: 10.1016/j.neulet.2014.10.040
Yao, Y., Chen, Z. L., Norris, E. H., and Strickland, S. (2014). Astrocytic laminin regulates pericyte differentiation and maintains blood brain barrier integrity. Nat. Commun. 5:3413. doi: 10.1038/ncomms4413
Yin, M., Pu, T., Wang, L., Marshall, C., Wu, T., and Xiao, M. (2018). Astroglial water channel aquaporin 4-mediated glymphatic clearance function: a determined factor for time-sensitive treatment of aerobic exercise in patients with Alzheimer’s disease. Med. Hypotheses 119, 18–21. doi: 10.1016/j.mehy.2018.07.016
Yun, S. P., Kam, T. I., Panicker, N., Kim, S., Oh, Y., Park, J. S., et al. (2018). Block of A1 astrocyte conversion by microglia is neuroprotective in models of Parkinson’s disease. Nat. Med. 24, 931–938. doi: 10.1038/s41591-018-0051-55
Zbesko, J. C., Nguyen, T. V., Yang, T., Frye, J. B., Hussain, O., Hayes, M., et al. (2018). Glial scars are permeable to the neurotoxic environment of chronic stroke infarcts. Neurobiol. Dis. 112, 63–78. doi: 10.1016/j.nbd.2018.01.007
Zeng, X. N., Xie, L. L., Liang, R., Sun, X. L., Fan, Y., and Hu, G. (2012). AQP4 knockout aggravates ischemia/reperfusion injury in mice. CNS Neurosci. Ther. 18, 388–394. doi: 10.1111/j.1755-5949.2012.00308.x
Zhang, C., Jiang, M., Wang, W. Q., Zhao, S. J., Yin, Y. X., Mi, Q. J., et al. (2020a). Selective mGluR1 negative allosteric modulator reduces blood-brain barrier permeability and cerebral edema after experimental subarachnoid hemorrhage. Transl. Stroke Res. 11, 799–811. doi: 10.1007/s12975-019-00758-z
Zhang, H., Wang, Y., Lian, L., Zhang, C., and He, Z. (2020b). Glycine-Histidine-Lysine (GHK) alleviates astrocytes injury of intracerebral hemorrhage via the Akt/miR-146a-3p/AQP4 pathway. Front. Neurosci. 14:576389. doi: 10.3389/fnins.2020.576389
Zhang, H. B., Tu, X. K., Song, S. W., Liang, R. S., and Shi, S. S. (2020c). Baicalin reduces early brain injury after subarachnoid hemorrhage in rats. Chin. J. Integr. Med. 26, 510–518. doi: 10.1007/s11655-020-3183-3187
Zhang, J., Han, F., Liang, X., Li, M., Zhang, D., Zhai, F., et al. (2020d). Lacune and large perivascular space: two kinds of cavities are of different risk factors and stroke risk. Cerebrovasc. Dis. 49, 522–530. doi: 10.1159/000508732
Zhang, Y., Zhang, X., Wei, Q., Leng, S., Li, C., Han, B., et al. (2020e). Activation of Sigma-1 receptor enhanced pericyte survival via the interplay between apoptosis and autophagy: implications for blood-brain barrier integrity in stroke. Transl. Stroke Res. 11, 267–287. doi: 10.1007/s12975-019-00711-710
Zhao, J., Davis, M. D., Martens, Y. A., Shinohara, M., Graff-Radford, N. R., Younkin, S. G., et al. (2017). APOE ε4/ε4 diminishes neurotrophic function of human iPSC-derived astrocytes. Hum. Mol. Genet. 26, 2690–2700. doi: 10.1093/hmg/ddx155
Zhao, Y., Gu, J. H., Dai, C. L., Liu, Q., Iqbal, K., Liu, F., et al. (2014). Chronic cerebral hypoperfusion causes decrease of O-GlcNAcylation, hyperphosphorylation of tau and behavioral deficits in mice. Front. Aging Neurosci. 6:10. doi: 10.3389/fnagi.2014.00010
Zhou, Y., Cai, J., Zhang, W., Gong, X., and Yan, S. (2020). Impairment of the glymphatic pathway and putative meningeal lymphatic vessels in the aging human. Ann. Neurol. 87, 357–369. doi: 10.1002/ana.25670
Zhou, Z., Ikegaya, Y., and Koyama, R. (2019). The astrocytic cAMP pathway in health and disease. Int. J. Mol. Sci. 20:779. doi: 10.3390/ijms20030779
Keywords: glymphatic system, AQP4, astrocytes, meningeal lymphatic system, stroke
Citation: Lv T, Zhao B, Hu Q and Zhang X (2021) The Glymphatic System: A Novel Therapeutic Target for Stroke Treatment. Front. Aging Neurosci. 13:689098. doi: 10.3389/fnagi.2021.689098
Received: 31 March 2021; Accepted: 07 June 2021;
Published: 08 July 2021.
Edited by:
Gang Chen, First Affiliated Hospital of Soochow University, ChinaCopyright © 2021 Lv, Zhao, Hu and Zhang. This is an open-access article distributed under the terms of the Creative Commons Attribution License (CC BY). The use, distribution or reproduction in other forums is permitted, provided the original author(s) and the copyright owner(s) are credited and that the original publication in this journal is cited, in accordance with accepted academic practice. No use, distribution or reproduction is permitted which does not comply with these terms.
*Correspondence: Qin Hu, aHVxaW5sZTIwMDEwNzA5QDEyNi5jb20=; Xiaohua Zhang, enhoMTk2OUBhbGl5dW4uY29t
Disclaimer: All claims expressed in this article are solely those of the authors and do not necessarily represent those of their affiliated organizations, or those of the publisher, the editors and the reviewers. Any product that may be evaluated in this article or claim that may be made by its manufacturer is not guaranteed or endorsed by the publisher.
Research integrity at Frontiers
Learn more about the work of our research integrity team to safeguard the quality of each article we publish.