- 1Department of Microbiology and Immunology, Drexel University College of Medicine, Philadelphia, PA, United States
- 2Center for Molecular Virology and Translational Neuroscience, Institute for Molecular Medicine & Infectious Disease, Drexel University College of Medicine, Philadelphia, PA, United States
- 3Sidney Kimmel Cancer Center, Thomas Jefferson University, Philadelphia, PA, United States
Human immunodeficiency virus type 1 (HIV-1)- associated neurocognitive disorders (HAND) is a disease of neurologic impairment that involves mechanisms of damage similar to other degenerative neurologic diseases such as Alzheimer’s disease (AD). In the current era of antiretroviral therapy (ART), HIV-1 replication is well-suppressed, and yet, HIV-1-infected patients still have high levels of chronic inflammation, indicating that factors other than viral replication are contributing to the development of neurocognitive impairment in these patients. The underlying mechanisms of HAND are still unknown, but the HIV-1 protein, Tat, has been highlighted as a potential viral product that contributes to the development of cognitive impairment. In AD, the presence of senescent cells in the CNS has been discussed as a contributing factor to the progression of cognitive decline and may be a mechanism also involved in the development of HAND. This mini-review discusses the viral protein HIV-1 Tat, and its potential to induce senescence in the CNS, contributing to the development of HAND.
Introduction
Human immunodeficiency virus type 1 (HIV-1)-associated neurocognitive disorders (HAND) represents a range of neurologic impairments observed in HIV-1-infected patients who are well-suppressed on antiretroviral therapy (ART) (Dickens et al., 2017; Williams et al., 2020). HAND is diagnosed by neurocognitive testing and is further classified based on the level of impairment that interferes with activities of daily living, where the most common clinical characteristics are subcortical dementia with deficits in memory, attention, and concentration (Nightingale et al., 2014; Eggers et al., 2017). Although implementation of ART has decreased the severity of HAND, it still remains prevalent, with over 50% of HIV-1-infected patients exhibiting mild symptoms of HAND (Chai et al., 2017). Because HAND remains prevalent even in the ART era, it has been suggested that viral replication is not a major factor involved in mediating cognitive decline (Zeitler et al., 2015; Berman et al., 2016; Dickens et al., 2017; Cohen et al., 2018). The viral protein HIV-1 Tat therefore, has been highlighted as a potential viral product that may contribute to the development of HAND (Chen et al., 1997; Eugenin et al., 2003). Tat is an early gene product of HIV-1, and is continually secreted from latently infected cells (Mele et al., 2018; Link et al., 2019; Spector et al., 2019), where extracellular Tat has been detected in 36.8% of patient CSF samples at concentrations ranging from 200 pg/ml to 6.5 ng/ml (Henderson et al., 2019). Extracellular Tat is biologically active and can cause induction of apoptosis, oxidative stress, and release of pro-inflammatory cytokines and neurotransmitters, all of which may contribute to the development of HAND (Table 1; Henderson et al., 2019; Ajasin and Eugenin, 2020). Additionally, the presence of Tat alone in the CNS of animal models is sufficient to replicate HAND pathologic and behavioral changes (Zeitler et al., 2015; Berman et al., 2016; Dickens et al., 2017; Cohen et al., 2018).
A major mechanism of HIV neuropathogenesis involves infiltration of immune cells, such as lymphocytes and macrophages, across the blood-brain barrier (BBB), and into the CNS (Rao et al., 2014; Eggers et al., 2017). These cells can then produce chemokines and cytokines, such as MCP-1 and TNF-α, to recruit more cells from the periphery into the CNS, while also creating a pro-inflammatory environment within the CNS (Rao et al., 2014; Eggers et al., 2017). Infected immune cells within the CNS can then infect CNS resident microglia and possibly astrocytes, and newly infected cells will continually secrete viral proteins and contribute to inflammation (Andras et al., 2005).
Although neurons cannot become infected by HIV-1, they can become damaged by viral proteins, such as Tat, inflammatory cytokines, such as TNF-α and IL-1β, and small metabolites, such as reactive oxygen species (ROS) and nitric oxide (NO) (Rao et al., 2014). The resulting neuronal damage can lead to apoptosis and loss of dendrite processes where these changes have been shown to contribute to HAND development (Rao et al., 2014).
Hand and Alzheimer’s Disease
A potentially significant contributor of HAND pathology is beta-amyloid (Aβ), where Aβ deposition is accelerated in HIV-1 patients as compared to uninfected, age-matched controls (Clifford et al., 2009; Chai et al., 2017; Eggers et al., 2017). Accumulation of amyloid plaques is associated with neurodegenerative conditions such as Alzheimer’s Disease (AD) and amyloid measurements in the CSF of HAND patients has been reported to be similar to those in Alzheimer’s type dementia (Clifford et al., 2009; Chai et al., 2017). However, there are distinct differences in Aβ deposition in AD as compared to HAND, where HAND patients have diffuse amyloid plaques, while AD patients have senile plaques and neurofibrillary tangles (Clifford et al., 2009; Canet et al., 2018). Chai et al. (2017) HIV-1 Tat can also mediate amyloid dysfunction as Tat can inhibit neprolysin, which is an endonuclease necessary for Aβ breakdown (Clifford et al., 2009). Tat can also compete with amyloid precursor protein (APP) and apolipoproteins E (an Aβ chaperone), for binding to the low-density lipoprotein receptor related protein (LRP), and this will result in a blockage of LRP-mediated clearance of Aβ from the brain (Clifford et al., 2009). Similar to AD, patients with HAND also have significantly decreased levels of Aβ42, which is the cleavage product of APP, while tau protein is increased, suggesting that HAND may be associated with AD-like processes (Clifford et al., 2009; Eggers et al., 2017).
Both AD and HAND pathologies result in neurodegeneration and cognitive impairment that progresses over time. As discussed previously, HAND is characterized by immune cell infiltrate into the CNS and production of pro-inflammatory cytokines (Rao et al., 2014; Eggers et al., 2017). Similar changes have also been detected in AD, where studies have reported chronic CNS inflammation (increased IL-1β and IL-6), as well as increased immune cell infiltrate in the CNS (Eugenin et al., 2011; Bhat et al., 2012; Atluri et al., 2015; Chen et al., 2017; Dickens et al., 2017; Baker and Petersen, 2018; Madeira et al., 2018). The mechanisms involved in neurodegenerative diseases such as AD and HAND, although not identical, are similar and have some overlapping features. Because some similarities in underlying mechanisms of AD and HAND have already been identified, it is possible that there are additional similarities, yet to be reported. One such pathway is senescence, which has been discussed in AD as a mechanism that both contributes to and results from cell stress and inflammation. Due to the similarities between HAND and AD, we propose a possible HIV-1-Tat-induced mechanism of senescence in the CNS of HIV patients that exhibit HAND.
Senescence
Senescence is a cell mechanism usually involved in aging that is driven by telomere shortening or oxidative stress and results in a permanent exit of the cell cycle, and resistance to apoptosis (Childs et al., 2018). When senescence occurs prematurely, it is associated with neurodegenerative disorders such as AD, and it is possible that premature CNS senescence could also be an underlying mechanism in the development of HAND (Cohen et al., 2018). Premature senescence can occur in human astrocytes when exposed to high levels of oxidative stress, and involves increased p16ink4a and p21, decreased telomere length, and increased secretion of IL-6, CCL5, IL-8 and intercellular adhesion molecule 1 (I-CAM-1) (Bhat et al., 2012; Baker and Petersen, 2018). Astrocytes also undergo senescence in response to ROS, and in astrocytes derived from brains of AD patients, there was increased expression of the cell cycle regulator p16, as well as increased matrix metallopeptidase-1 (MMP-1) and IL-6, all of which have also been shown to be upregulated in senescence (Baker and Petersen, 2018).
As previously discussed, Tat can mediate oxidative stress and inflammation, both of which are driving factors in the development of premature senescence (Table 1; Capone et al., 2013; Baker and Petersen, 2018; Jeon et al., 2018). Although Tat can be produced and secreted by infected CNS cells, peripherally produced Tat can also cross the blood-brain barrier (BBB), and interact with and damage cells of the barrier (Buscemi et al., 2007; Debaisieux et al., 2012; Ma et al., 2016). The BBB is composed of brain microvascular endothelial cells (BMECs) and astrocytes and acts as a semi-permeable barrier that restricts entry into the CNS, a function that is essential for proper brain function (Kanmogne et al., 2007; Eugenin et al., 2011; Strazza et al., 2011; Williams et al., 2012; Atluri et al., 2015; Yamazaki et al., 2016). Endothelial cells at the BBB are surrounded by pericytes that help promote endothelial cell survival and contribute to stabilization of capillaries (Nakagawa et al., 2012). Dysregulation of the BBB, caused by HIV-1 can exacerbate neurodegenerative disorders by allowing aberrant influx of cells into the CNS, leading to increased inflammation and neurotoxicity (Rao et al., 2014; Yamazaki et al., 2016; Baker and Petersen, 2018).
In mesenchymal stem cells (MSCs), a precursor to astrocytes, extracellular Tat reduced population doublings and increase p21 expression, both indicators of senescence (Beaupere et al., 2015). Furthermore, senescent astrocytes lose their neuroprotective function, and can negatively impact surrounding cells and tissues primarily through production of a pro-inflammatory cytokines (Table 1; Kanmogne et al., 2007; Liu et al., 2011; Williams et al., 2012; Yamazaki et al., 2016; Cohen et al., 2018; Herranz and Gil, 2018; Jeon et al., 2018). This is important because astrocytes exposed to Tat produce pro-inflammatory cytokines, and this may indicate the ability of Tat exposure to induce senescence in astrocytes.
Although BMECs at the BBB cannot be infected by HIV-1, they are significantly affected by the indirect action of HIV-1, such as through the activity of viral proteins like Tat, where BMECs may undergo senescence in response to external stressors (Johnston et al., 2001; Liu et al., 2011; Williams et al., 2012; Rahimian and He, 2016; Cohen et al., 2018; Herranz and Gil, 2018; Jeon et al., 2018). In BMECs treated with Tat at 500 ng/ml for 24 h, there was increased senescence and production of IL-6 and TNF-α (Table 1; Hijmans et al., 2018). Senescence can also affect TJPs, and in a co-culture system of senescent murine BBB cells, the TJP expression level was unchanged, however, structurally, the TJP were disorganized and diffuse, and resulted in increased BBB permeability as measured by Evans Blue (Table 1; Yamazaki et al., 2016). Decreased BBB integrity occurs in HIV-1 infection, where the loss of BBB integrity, potentially due to exposure to Tat and altered glutamate levels may induce the onset of senescence in astrocytes and BMECs, the major cells of the BBB, culminating in the generation of neurocognitive disorders such as HAND (Beaupere et al., 2015; Yamazaki et al., 2016; Hijmans et al., 2018).
Glutamate in CNS
Glutamate is the major excitatory neurotransmitter in the CNS, and is tightly regulated where high levels of glutamate will lead to excitotoxicity and neuronal death (Andras et al., 2007; Madeira et al., 2018). Na+-dependent excitatory amino acid transporters (EAATs) are responsible for the removal of extracellular glutamate in the CNS, and regulate extracellular concentrations of glutamate (Magi et al., 2019). The glial glutamate transporter, EAAT-2 is located on astrocytes and is the major transporter responsible for glutamate uptake (Erdo et al., 2017). Tat can negatively affect EAAT-2 functioning, and in a study of primary cultured mouse astrocytes treated with recombinant Tat for 48 h there was decreased EAAT-2 protein and mRNA expression, resulting in high levels of extracellular glutamate (Ye et al., 2017). Additionally, in neuroblastoma cells treated with extracellular subtype B Tat, there was significantly increased glutamate as compared to subtype C Tat-treated cells (Williams et al., 2020). This observation is important because subtype B Tat has been linked to increased neurotoxicity as compared to subtype C Tat, indicating that altered glutamate levels may be a major factor responsible for neurotoxicity (Williams et al., 2020). Furthermore, astrocytes exposed to subtype B Tat had a reduced capacity for buffering glutamate, as well as impaired glutamate intake as compared to subtype C Tat, resulting in higher concentrations of extracellular glutamate with subtype B Tat (Zhou et al., 2004; Zou et al., 2011).
Tat may also simultaneously increase extracellular levels of glutamate in the CNS by binding to Connexin 43 (Ye et al., 2017). Connexin 43 is a gap junction protein that connects the cytoplasmic compartments of coupled cells, which allows the cells to directly interact with one another (Berman et al., 2016). Connexin 43 controls the release of glutamate from astrocytes into the extracellular space, where astrocytes are a major cell responsible for maintaining glutamate homeostasis (He et al., 2020). Importantly, Tat is the only HIV-1 protein capable of interacting with and significantly increasing the expression of Connexin 43 on astrocytes (Berman et al., 2016). Efflux of glutamate from astrocytes due to Connexin 43 activation leads to increased glutamate in the extracellular space, which can result in neuronal excitotoxicity and death (He et al., 2020). Importantly, Connexin 43 has a key role in learning and memory and when normal functions of Connexin 43 were interrupted it led to impaired hippocampal spatial memory (He et al., 2020). Increased expression of Connexin 43 has also been detected in AD, where Connexin 43 was specifically increased at amyloid plaques (He et al., 2020). The development of AD symptoms has also been linked to increased glutamate resulting from activation of Connexin 43 on astrocytes (He et al., 2020). Increased Connexin 43 in astrocytes will result in increased calcium levels, which leads to the development of ER stress and can culminate in neuronal damage and cognitive decline, in both HAND and AD (He et al., 2020). The ability of Tat to alter the regulatory mechanisms of glutamate results in increased concentrations of extracellular glutamate and can have significant consequences on normal CNS signaling pathways (Magi et al., 2019).
Changes in Signaling Pathways
In normal signaling pathways in the CNS, extracellular glutamate will interact with and activate synaptic N-methyl-D-aspartate receptors (NMDARs), resulting in neuroprotection (Andras et al., 2007; Zhou and Sheng, 2013; Magi et al., 2019). NMDARs are a subclass of postsynaptic neuronal glutamate receptors that mediate cellular calcium entry and are involved in cell signaling (Zhou and Sheng, 2013; Madeira et al., 2018). Activation of NMDARs requires simultaneous binding of both glutamate and glycine/D-serine to the GluN2 and GluN1 subunits of NMDAR (Vieira et al., 2020). In a normal physiologic system, glutamate is removed from the synaptic cleft and converted into glutamine by glutamine synthetase, where glutamine is then transported back and converted to glutamate by glutaminase (Madeira et al., 2018). However, excitotoxicity caused by excessive glutamate release from the presynaptic terminal can lead to NMDAR hyperactivation and result in excitotoxicity, which contributes to neurological degeneration (Vieira et al., 2020). Excessive extracellular glutamate can bind to NMDARs located in the extra-synaptic regions of neurons and lead to neurotoxicity (Zhou and Sheng, 2013). It has also been shown that Aβ can stimulate extra-synaptic NMDARs to increase the neuronal production of Aβ to further contribute to CNS dysfunction (Zhou and Sheng, 2013).
Tat is able to stimulate NMDARs, and Tat-mediated stimulation can lead to glutamate-dependent excitotoxicity and apoptosis in neurons (Haughey et al., 2001; Sharp et al., 2003; Hogan-Cann and Anderson, 2016; Ye et al., 2017). Additionally, excitotoxicity resulting from Tat exposure is enhanced by the addition of glutamate, which results in calcium flux from neurons where excessive calcium may be involved in neuroinflammation (Haughey et al., 2001; Hogan-Cann and Anderson, 2016). Extracellular Tat can also potentiate excitotoxicity to lead to neuronal cell death by increasing the phosphorylation of NMDAR subunits in rat cortical neurons (Haughey et al., 2001). Additionally, over-activation of NMDARs has been previously linked to the development of AD, where there are increased levels of both glutamate and glutamine in the CSF, as compared to healthy controls, and patients with higher glutamate and glutamine levels also had lower neurocognitive testing scores (Madeira et al., 2018).
Glutamate excitotoxicity can also lead to a cascade of inflammation in the CNS, where glutamate can increase the levels of nitric oxide synthase (NOS), which has been linked to CNS dysfunction (Madeira et al., 2018; Williams et al., 2020). At the BBB, exposure of BMECs to high concentrations of glutamate resulted in the formation of ROS leading to oxidative stress, as well as decreased trans-endothelial electrical resistance (TEER) and re-localization of occludin, a major tight junction protein (TJP) at the BBB, suggesting that high concentrations of extracellular glutamate can negatively affect BBB integrity (Capone et al., 2013).
Although cytokines and ROS each have roles in CNS damage during HIV-1 infection, alterations of TJPs has been shown to be the most predominant mechanism underlying BBB disruption (Almutairi et al., 2016). In astrocytes, Tat exposure led to increased MMP-9 which disrupted the BBB by cleaving occludin to inactivate it (Johnston et al., 2001; Rao et al., 2014; Atluri et al., 2015). Similarly, BMECs exposed to Tat had decreased occludin and claudin-5 TJP mRNA (Andras et al., 2005; Atluri et al., 2015). Redistribution and inhibition of TJPs due to Tat exposure resulting in increased glutamate concentrations will result in impaired BBB integrity, which can allow cells and protein to more easily cross the BBB to enter into the CNS to lead to damage and inflammation.
Tat exposure has also been shown to lead to oxidative stress and can induce transcription pathways like NF-κB and AP-1, to produce the pro-inflammatory cytokines IL-6 and IL-8 in BMECs (Li et al., 2009; Rizzo and Leaver, 2010; Labus et al., 2014; Youn et al., 2017). Astrocytes exposed to Tat had increased NF-κB, resulting in the production of MCP-1, IL-1β, IL-6, IL-8, chemokine ligand 5 (CCL5/RANTES), interferon-γ-induced protein-10 (IP-10/CXCL10), and tumor necrosis factor alpha (TNF-α) (Table 1; Boven et al., 1999; Weiss et al., 1999; Nookala and Kumar, 2014; Almutairi et al., 2016; Fan and He, 2016). Alteration of signaling pathways in the CNS, either by direct Tat interactions, or indirectly, through Tat-altered glutamate signaling, can result in increased cell stress and damage, and together these changes will culminate in the onset of senescence in the CNS.
Discussion
Based on the literature, Tat-induced senescence represents a possible mechanism underlying the development of HAND (Figure 1). Here we link data from multiple studies to discuss a potential mechanism of Tat-induced senescence.
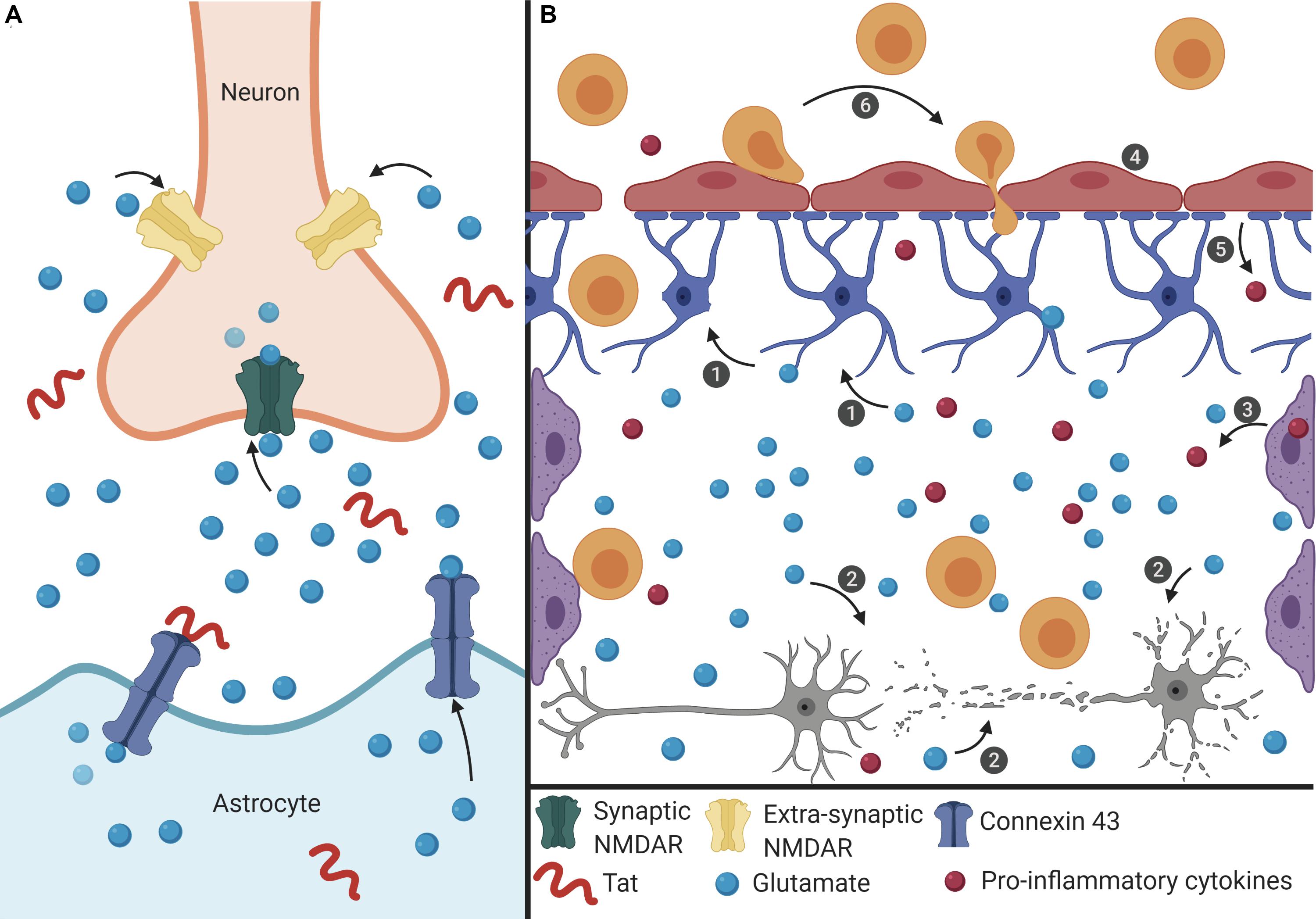
Figure 1. Proposed mechanism of Tat-induced senescence. (A) Extracellular Tat binds to Connexin 43 and decreases EAAT-2 expression on astrocytes to result in increased extracellular glutamate concentrations. Under normal conditions, glutamate binds to synaptic NMDARs (green) to promote neuroprotective pathways. However, high concentrations of extracellular glutamate will allow glutamate to exit the synaptic cleft and bind to extra-synaptic NMDARs (pink), which promotes excitotoxicity. (B) The interaction depicted in panel (A) has widespread effects on other cells of the CNS. (1) Extracellular glutamate causes cell stress and damage in astrocytes (blue), depicted as loss of foot process, and (2) neurons (gray), depicted as synaptodendritic damage, and (3) causes stress in perivascular macrophages (purple), depicted as an increase in proinflammatory cytokines. Extracellular glutamate also affects (4) endothelial cells (red), causing increased permeability, (5) and release of ROS and pro-inflammatory cytokines (red), which results in (6) recruitment of immune cells (orange) across the BBB and into the CNS, creating a feedback loop of chronic inflammation, and damage. This inflammatory microenvironment will force cells to become senescent, and this will lead to further decreased BBB integrity and production of pro-inflammatory cytokines.
Extracellular Tat can interact with Connexin 43 on astrocytes, which is involved in glutamate regulation. Tat binding to Connexin 43 opens the channel, resulting in efflux of glutamate from the astrocytes and into the extracellular environment (Berman et al., 2016; Figure 1A). At the same time, Tat can also decrease the expression of EAAT-2 on astrocytes, which in turn decreases glutamate uptake (Ye et al., 2017). Together, these two effects on astrocytes will lead to high concentrations of extracellular glutamate in the CNS. As glutamate is a highly regulated neurotransmitter in the CNS, cells are sensitive to alterations in the concentration of glutamate (Madeira et al., 2018). The ability of Tat to alter two of the major mechanisms used to regulate the level of glutamate will have significant effects on other cells of the CNS.
High levels of extracellular glutamate will allow it to bind to extra-synaptic NMDARs, rather than the normal synaptic NMDARs, and this extra-synaptic activation will potentiate excitotoxicity (Zhou and Sheng, 2013; Madeira et al., 2018). In other cells of the CNS, such as perivascular macrophages and BMECs, glutamate can cause cell stress, leading to the release of pro-inflammatory cytokines and ROS (He et al., 2020; Williams et al., 2020). These effects culminate in a chronic feedback loop where the pro-inflammatory environment creates additional cell stress and oxidative damage, and this leads to immune infiltrate across the BBB and into the CNS (Figure 1B). As a result, there is chronic inflammation and damage in the CNS which can cause neuronal death, and in response to the inflammatory extracellular environment, cells of the CNS, such as astrocytes and BMECs, become senescent in order to avoid further cell stress and damage that would lead to apoptosis. Additionally, in HAND there is a loss of pericytes at the BBB, which are important to promote endothelial cell survival (Niu et al., 2015). Onset of senescence further reinforces a pro-inflammatory feedback loop to promote an inflammatory microenvironment that can further induce adjacent cells to also become senescent. As cells at the BBB become senescent, there is redistribution of tight junction proteins and changes in cell morphology that affects the integrity of the barrier, thus enabling more cells to cross into the CNS (Yamazaki et al., 2016). Establishment of this inflammatory feedback mechanism will cause multiple cells of the CNS to become senescent which can result in neurocognitive impairment and lead to cognitive decline.
Tat is a small HIV-1 protein that is capable of causing large scale damage due to its ability to alter multiple cellular pathways, including glutamate regulation in the CNS. Senescence of CNS cells has been identified as an underlying mechanism responsible for cognitive impairment in AD, a condition that shares many mechanistic similarities to other neurodegenerative disorders, such as HAND (Table 1). Further studies elucidating the underlying mechanisms of Tat-induced dysregulation will be important to understand how these interactions may influence the development of HAND. Additionally, determining the impact of Tat-induced senescence in the CNS is important to understand the contribution of senescence to the pathogenesis of HAND (Yamazaki et al., 2016; Baker and Petersen, 2018).
Although this mini-review has focused on Tat and its impact on glutamate levels and induction of senescence, there are other HIV-1 proteins that are relevant to the development of CNS dysfunction. The viral proteins Nef and Gag are released from HIV-1-infected cells via exosomes and Nef can cause activation-induced cell death and cause neuronal toxicity (Hu et al., 2016; Dickens et al., 2017). Apoptosis has been observed in BMECs at the BBB exposed to gp120, Vpr, or Nef which has indicated the ability of these viral proteins to alter BBB integrity (Toborek et al., 2005). Additionally, exposure of astrocytes to extracellular Vpr led to increased concentrations of IL-6, IL-8, and MCP-1 as well as increased production of ROS due to an increase in the level of oxidized glutathione (GSSG), and these effects may result in senescence onset (Ferrucci et al., 2012, 2013). Finally, a study examining Tat and Nef showed that exposure of human mesenchymal stem cells to either protein had increased senescence after 20 days, indicating the ability of other HIV-1 proteins to induce senescence in non-CNS cell types (Beaupere et al., 2015). While multiple HIV-1 proteins can mediate effects similar to Tat in the CNS, it has been suggested that examining combination effects is warranted. However, it is important to keep in mind that Tat seems to be the one protein still measurable in the CNS during ART.
Author Contributions
JM and MN conceived and designed the study. All authors prepared and designed the figures, drafted the manuscript, and read and approved the final manuscript.
Funding
The authors were funded in part by the Public Health Service, National Institutes of Health, through grants from the National Institute of Neurological Disorders and Stroke (NINDS) R01 NS089435 (PI, MN), the NIMH Comprehensive NeuroAIDS Center (CNAC) P30 MH092177 (Kamel Khalili, PI; BW, PI of the Drexel subcontract involving the Clinical and Translational Research Support Core) and under the Ruth L. Kirschstein National Research Service Award T32 MH079785 (PI, Kamel Khalili and Tricia Burdo; with BW serving as the PI of the Drexel University College of Medicine component and Olimpia Meucci as Co-Director). The contents of the manuscript are solely the responsibility of the authors and do not necessarily represent the official views of the NIH.
Conflict of Interest
The authors declare that the research was conducted in the absence of any commercial or financial relationships that could be construed as a potential conflict of interest.
Acknowledgments
The authors would like to acknowledge BioRender. The software was used to help create Figure 1 using the standard academic license held by MN.
References
Ajasin, D., and Eugenin, E. A. (2020). HIV-1 Tat: role in bystander toxicity. Front. Cell. Infect. Microbiol. 10:61. doi: 10.3389/fcimb.2020.00061
Almutairi, M. M., Gong, C., Xu, Y. G., Chang, Y., and Shi, H. (2016). Factors controlling permeability of the blood-brain barrier. Cell. Mol. Life Sci. 73, 57–77. doi: 10.1007/s00018-015-2050-8
Andras, I. E., Deli, M. A., Veszelka, S., Hayashi, K., Hennig, B., and Toborek, M. (2007). The NMDA and AMPA/KA receptors are involved in glutamate-induced alterations of occludin expression and phosphorylation in brain endothelial cells. J. Cereb. Blood Flow Metab. 27, 1431–1443. doi: 10.1038/sj.jcbfm.9600445
Andras, I. E., Pu, H., Tian, J., Deli, M. A., Nath, A., Hennig, B., et al. (2005). Signaling mechanisms of HIV-1 Tat-induced alterations of claudin-5 expression in brain endothelial cells. J. Cereb. Blood Flow Metab. 25, 1159–1170. doi: 10.1038/sj.jcbfm.9600115
Atluri, V. S., Hidalgo, M., Samikkannu, T., Kurapati, K. R., Jayant, R. D., Sagar, V., et al. (2015). Effect of human immunodeficiency virus on blood-brain barrier integrity and function: an update. Front. Cell. Neurosci. 9:212. doi: 10.3389/fncel.2015.00212
Baker, D. J., and Petersen, R. C. (2018). Cellular senescence in brain aging and neurodegenerative diseases: evidence and perspectives. J. Clin. Invest. 128, 1208–1216. doi: 10.1172/JCI95145
Beaupere, C., Garcia, M., Larghero, J., Feve, B., Capeau, J., and Lagathu, C. (2015). The HIV proteins Tat and Nef promote human bone marrow mesenchymal stem cell senescence and alter osteoblastic differentiation. Aging Cell 14, 534–546. doi: 10.1111/acel.12308
Berman, J. W., Carvallo, L., Buckner, C. M., Luers, A., Prevedel, L., Bennett, M. V., et al. (2016). HIV-tat alters Connexin43 expression and trafficking in human astrocytes: role in NeuroAIDS. J. Neuroinflammation 13:54. doi: 10.1186/s12974-016-0510-1
Bhat, R., Crowe, E. P., Bitto, A., Moh, M., Katsetos, C. D., Garcia, F. U., et al. (2012). Astrocyte senescence as a component of Alzheimer’s disease. PLoS One 7:e45069. doi: 10.1371/journal.pone.0045069
Boven, L. A., Gomes, L., Hery, C., Gray, F., Verhoef, J., Portegies, P., et al. (1999). Increased peroxynitrite activity in AIDS dementia complex: implications for the neuropathogenesis of HIV-1 infection. J. Immunol. 162, 4319–4327.
Buscemi, L., Ramonet, D., and Geiger, J. D. (2007). Human immunodeficiency virus type-1 protein Tat induces tumor necrosis factor-alpha-mediated neurotoxicity. Neurobiol. Dis. 26, 661–670. doi: 10.1016/j.nbd.2007.03.004
Canet, G., Dias, C., Gabelle, A., Simonin, Y., Gosselet, F., Marchi, N., et al. (2018). HIV Neuroinfection and Alzheimer’s Disease: similarities and Potential Links? Front. Cell. Neurosci. 12:307. doi: 10.3389/fncel.2018.00307
Capone, C., Cervelli, M., Angelucci, E., Colasanti, M., Macone, A., Mariottini, P., et al. (2013). A role for spermine oxidase as a mediator of reactive oxygen species production in HIV-Tat-induced neuronal toxicity. Free Radic. Biol. Med. 63, 99–107. doi: 10.1016/j.freeradbiomed.2013.05.007
Chai, Q., Jovasevic, V., Malikov, V., Sabo, Y., Morham, S., Walsh, D., et al. (2017). HIV-1 counteracts an innate restriction by amyloid precursor protein resulting in neurodegeneration. Nat. Commun. 8:1522. doi: 10.1038/s41467-017-01795-8
Chen, N. C., Partridge, A. T., Sell, C., Torres, C., and Martin-Garcia, J. (2017). Fate of microglia during HIV-1 infection: from activation to senescence? Glia 65, 431–446. doi: 10.1002/glia.23081
Chen, P., Mayne, M., Power, C., and Nath, A. (1997). The Tat protein of HIV-1 induces tumor necrosis factor-alpha production. Implications for HIV-1-associated neurological diseases. J. Biol. Chem. 272, 22385–22388.
Childs, B. G., Li, H., and van Deursen, J. M. (2018). Senescent cells: a therapeutic target for cardiovascular disease. J. Clin. Invest. 128, 1217–1228. doi: 10.1172/JCI95146
Clifford, D. B., Fagan, A. M., Holtzman, D. M., Morris, J. C., Teshome, M., Shah, A. R., et al. (2009). CSF biomarkers of Alzheimer disease in HIV-associated neurologic disease. Neurology 73, 1982–1987. doi: 10.1212/WNL.0b013e3181c5b445
Cohen, J., D’Agostino, L., Tuzer, F., and Torres, C. (2018). HIV antiretroviral therapy drugs induce premature senescence and altered physiology in HUVECs. Mech. Ageing Dev. 175, 74–82. doi: 10.1016/j.mad.2018.07.008
Debaisieux, S., Rayne, F., Yezid, H., and Beaumelle, B. (2012). The ins and outs of HIV-1 Tat. Traffic 13, 355–363. doi: 10.1111/j.1600-0854.2011.01286.x
Dickens, A. M., Yoo, S. W., Chin, A. C., Xu, J., Johnson, T. P., Trout, A. L., et al. (2017). Chronic low-level expression of HIV-1 Tat promotes a neurodegenerative phenotype with aging. Sci. Rep. 7:7748. doi: 10.1038/s41598-017-07570-5
Eggers, C., Arendt, G., Hahn, K., Husstedt, I. W., Maschke, M., Neuen-Jacob, E., et al. (2017). HIV-1-associated neurocognitive disorder: epidemiology, pathogenesis, diagnosis, and treatment. J. Neurol. 264, 1715–1727. doi: 10.1007/s00415-017-8503-2
Erdo, F., Denes, L., and de Lange, E. (2017). Age-associated physiological and pathological changes at the blood-brain barrier: a review. J. Cereb. Blood Flow Metab. 37, 4–24. doi: 10.1177/0271678X16679420
Eugenin, E. A., Clements, J. E., Zink, M. C., and Berman, J. W. (2011). Human immunodeficiency virus infection of human astrocytes disrupts blood-brain barrier integrity by a gap junction-dependent mechanism. J. Neurosci. 31, 9456–9465. doi: 10.1523/JNEUROSCI.1460-11.2011
Eugenin, E. A., D’Aversa, T. G., Lopez, L., Calderon, T. M., and Berman, J. W. (2003). MCP-1 (CCL2) protects human neurons and astrocytes from NMDA or HIV-tat-induced apoptosis. J. Neurochem. 85, 1299–1311.
Fan, Y., and He, J. J. (2016). HIV-1 Tat induces unfolded protein response and endoplasmic reticulum stress in astrocytes and causes neurotoxicity through Glial Fibrillary Acidic Protein (GFAP) activation and aggregation. J. Biol. Chem. 291, 22819–22829. doi: 10.1074/jbc.M116.731828
Ferrucci, A., Nonnemacher, M. R., Cohen, E. A., and Wigdahl, B. (2012). Extracellular human immunodeficiency virus type 1 viral protein R causes reductions in astrocytic ATP and glutathione levels compromising the antioxidant reservoir. Virus Res. 167, 358–369. doi: 10.1016/j.virusres.2012.06.002
Ferrucci, A., Nonnemacher, M. R., and Wigdahl, B. (2013). Extracellular HIV-1 viral protein R affects astrocytic glyceraldehyde 3-phosphate dehydrogenase activity and neuronal survival. J. Neurovirol. 19, 239–253. doi: 10.1007/s13365-013-0170-1
Haughey, N. J., Nath, A., Mattson, M. P., Slevin, J. T., and Geiger, J. D. (2001). HIV-1 Tat through phosphorylation of NMDA receptors potentiates glutamate excitotoxicity. J. Neurochem. 78, 457–467.
He, J. T., Li, X. Y., Yang, L., and Zhao, X. (2020). Astroglial connexins and cognition: memory formation or deterioration? Biosci. Rep. 40:BSR20193510. doi: 10.1042/BSR20193510
Henderson, L. J., Johnson, T. P., Smith, B. R., Reoma, L. B., Santamaria, U. A., Bachani, M., et al. (2019). Presence of Tat and transactivation response element in spinal fluid despite antiretroviral therapy. AIDS 33(Suppl. 2), S145–S157. doi: 10.1097/QAD.0000000000002268
Herranz, N., and Gil, J. (2018). Mechanisms and functions of cellular senescence. J. Clin. Invest. 128, 1238–1246. doi: 10.1172/JCI95148
Hijmans, J. G., Stockleman, K., Reiakvam, W., Levy, M. V., Brewster, L. M., Bammert, T. D., et al. (2018). Effects of HIV-1 gp120 and tat on endothelial cell sensescence and senescence-associated microRNAs. Physiol. Rep. 6:e13647. doi: 10.14814/phy2.13647
Hogan-Cann, A. D., and Anderson, C. M. (2016). Physiological roles of non-neuronal NMDA receptors. Trends Pharmacol. Sci. 37, 750–767. doi: 10.1016/j.tips.2016.05.012
Hu, G., Yang, L., Cai, Y., Niu, F., Mezzacappa, F., Callen, S., et al. (2016). Emerging roles of extracellular vesicles in neurodegenerative disorders: focus on HIV-associated neurological complications. Cell Death Dis. 7:e2481. doi: 10.1038/cddis.2016.336
Jeon, O. H., David, N., Campisi, J., and Elisseeff, J. H. (2018). Senescent cells and osteoarthritis: a painful connection. J. Clin. Invest. 128, 1229–1237. doi: 10.1172/JCI95147
Johnston, J. B., Zhang, K., Silva, C., Shalinsky, D. R., Conant, K., Ni, W., et al. (2001). HIV-1 Tat neurotoxicity is prevented by matrix metalloproteinase inhibitors. Ann. Neurol. 49, 230–241.
Kanmogne, G. D., Schall, K., Leibhart, J., Knipe, B., Gendelman, H. E., and Persidsky, Y. (2007). HIV-1 gp120 compromises blood-brain barrier integrity and enhance monocyte migration across blood-brain barrier: implication for viral neuropathogenesis. Cereb. Blood Flow Metab. 27, 123–134.
Labus, J., Hackel, S., Lucka, L., and Danker, K. (2014). Interleukin-1beta induces an inflammatory response and the breakdown of the endothelial cell layer in an improved human THBMEC-based in vitro blood-brain barrier model. J. Neurosci. Methods 228, 35–45. doi: 10.1016/j.jneumeth.2014.03.002
Langford, D., Oh Kim, B., Zou, W., Fan, Y., Rahimain, P., Liu, Y., et al. (2018). Doxycycline-inducible and astrocyte-specific HIV-1 Tat transgenic mice (iTat) as an HIV/neuroAIDS model. J. Neurovirol. 24, 168–179. doi: 10.1007/s13365-017-0598-9
Li, W., Li, G., Steiner, J., and Nath, A. (2009). Role of Tat protein in HIV neuropathogenesis. Neurotox. Res. 16, 205–220. doi: 10.1007/s12640-009-9047-8
Link, R. W., Mele, A. R., Antell, G. C., Pirrone, V., Zhong, W., Kercher, K., et al. (2019). Investigating the distribution of HIV-1 Tat lengths present in the Drexel Medicine CARES cohort. Virus Res. 272:197727. doi: 10.1016/j.virusres.2019.197727
Liu, G., Hosomi, N., Hitomi, H., Pelisch, N., Fu, H., Masugata, H., et al. (2011). Angiotensin II induces human astrocyte senescence through reactive oxygen species production. Hypertens. Res. 34, 479–483. doi: 10.1038/hr.2010.269
Ma, R., Yang, L., Niu, F., and Buch, S. (2016). HIV Tat-mediated induction of human brain microvascular endothelial cell apoptosis involves endoplasmic reticulum stress and mitochondrial dysfunction. Mol. Neurobiol. 53, 132–142. doi: 10.1007/s12035-014-8991-3
Madeira, C., Vargas-Lopes, C., Brandao, C. O., Reis, T., Laks, J., Panizzutti, R., et al. (2018). Elevated glutamate and glutamine levels in the cerebrospinal fluid of patients with probable Alzheimer’s disease and depression. Front. Psychiatry 9:561. doi: 10.3389/fpsyt.2018.00561
Magi, S., Piccirillo, S., Amoroso, S., and Lariccia, V. (2019). Excitatory Amino Acid Transporters (EAATs): glutamate transport and beyond. Int. J. Mol. Sci. 20:E5674. doi: 10.3390/ijms20225674
Mele, A. R., Marino, J., Chen, K., Pirrone, V., Janetopoulos, C., Wigdahl, B., et al. (2018). Defining the molecular mechanisms of HIV-1 Tat secretion: PtdIns(4,5)P2 at the epicenter. Traffic. doi: 10.1111/tra.12578 [Epub ahead of print].
Nakagawa, S., Castro, V., and Toborek, M. (2012). Infection of human pericytes by HIV-1 disrupts the integrity of the blood-brain barrier. J. Cell. Mol. Med. 16, 2950–2957. doi: 10.1111/j.1582-4934.2012.01622.x
Nightingale, S., Winston, A., Letendre, S., Michael, B. D., McArthur, J. C., Khoo, S., et al. (2014). Controversies in HIV-associated neurocognitive disorders. Lancet Neurol. 13, 1139–1151. doi: 10.1016/S1474-4422(14)70137-1
Niu, F., Yao, H., Liao, K., and Buch, S. (2015). HIV Tat 101-mediated loss of pericytes at the blood-brain barrier involves PDGF-BB. Ther. Targets Neurol. Dis. 2:e471. doi: 10.14800/ttnd.471
Nookala, A. R., and Kumar, A. (2014). Molecular mechanisms involved in HIV-1 Tat-mediated induction of IL-6 and IL-8 in astrocytes. J. Neuroinflammation 11:214.
Rahimian, P., and He, J. J. (2016). Exosome-associated release, uptake, and neurotoxicity of HIV-1 Tat protein. J. Neurovirol. 22, 774–788. doi: 10.1007/s13365-016-0451-6
Rao, V. R., Ruiz, A. P., and Prasad, V. R. (2014). Viral and cellular factors underlying neuropathogenesis in HIV associated neurocognitive disorders (HAND). AIDS Res. Ther. 11:13. doi: 10.1186/1742-6405-11-13
Rizzo, M. T., and Leaver, H. A. (2010). Brain endothelial cell death: modes, signaling pathways, and relevance to neural development, homeostasis, and disease. Mol. Neurobiol. 42, 52–63. doi: 10.1007/s12035-010-8132-6
Sharp, C. D., Hines, I., Houghton, J., Warren, A., Jackson, T. H. T., Jawahar, A., et al. (2003). Glutamate causes a loss in human cerebral endothelial barrier integrity through activation of NMDA receptor. Am. J. Physiol. Heart Circ. Physiol. 285, H2592–H2598. doi: 10.1152/ajpheart.00520.2003
Spector, C., Mele, A. R., Wigdahl, B., and Nonnemacher, M. R. (2019). Genetic variation and function of the HIV-1 Tat protein. Med. Microbiol. Immunol. 208, 131–169. doi: 10.1007/s00430-019-00583-z
Strazza, M., Pirrone, V., Wigdahl, B., and Nonnemacher, M. R. (2011). Breaking down the barrier: the effects of HIV-1 on the blood-brain barrier. Brain Res. 1399, 96–115. doi: 10.1016/j.brainres.2011.05.015
Toborek, M., Lee, Y. W., Flora, G., Pu, H., Andras, I. E., Wylegala, E., et al. (2005). Mechanisms of the blood-brain barrier disruption in HIV-1 infection. Cell. Mol. Neurobiol. 25, 181–199.
Vieira, M., Yong, X. L. H., Roche, K. W., and Anggono, V. (2020). Regulation of NMDA glutamate receptor functions by the GluN2 subunits. J. Neurochem. e14970. doi: 10.1111/jnc.14970 [Epub ahead of print].
Weiss, J. M., Nath, A., Major, E. O., and Berman, J. W. (1999). HIV-1 Tat induces monocyte chemoattractant protein-1-mediated monocyte transmigration across a model of the human blood-brain barrier and up-regulates CCR5 expression on human monocytes. J. Immunol. 163, 2953–2959.
Williams, D. W., Eugenin, E. A., Calderon, T. M., and Berman, J. W. (2012). Monocyte maturation, HIV susceptibility, and transmigration across the blood brain barrier are critical in HIV neuropathogenesis. J. Leukoc. Biol. 91, 401–415. doi: 10.1189/jlb.0811394
Williams, M. E., Zulu, S. S., Stein, D. J., Joska, J. A., and Naudé, P. J. W. (2020). Signatures of HIV-1 subtype B and C Tat proteins and their effects in the neuropathogenesis of HIV-associated neurocognitive impairments. Neurobiol. Dis. 136:104701. doi: 10.1016/j.nbd.2019.104701
Yamazaki, Y., Baker, D. J., Tachibana, M., Liu, C. C., van Deursen, J. M., Brott, T. G., et al. (2016). Vascular cell senescence contributes to blood-brain barrier breakdown. Stroke 47, 1068–1077. doi: 10.1161/STROKEAHA.115.010835
Ye, X., Zhang, Y., Xu, Q., Zheng, H., Wu, X., Qiu, J., et al. (2017). HIV-1 Tat inhibits EAAT-2 through AEG-1 upregulation in models of HIV-associated neurocognitive disorder. Oncotarget 8, 39922–39934. doi: 10.18632/oncotarget.16485
Youn, G. S., Cho, H., Kim, D., Choi, S. Y., and Park, J. (2017). Crosstalk between HDAC6 and Nox2-based NADPH oxidase mediates HIV-1 Tat-induced pro-inflammatory responses in astrocytes. Redox Biol. 12, 978–986. doi: 10.1016/j.redox.2017.05.001
Zeitler, M., Steringer, J. P., Muller, H. M., Mayer, M. P., and Nickel, W. (2015). HIV-Tat protein forms phosphoinositide-dependent membrane pores implicated in unconventional protein secretion. J. Biol. Chem. 290, 21976–21984. doi: 10.1074/jbc.M115.667097
Zhou, B. Y., Liu, Y., Kim, B., Xiao, Y., and He, J. J. (2004). Astrocyte activation and dysfunction and neuron death by HIV-1 Tat expression in astrocytes. Mol. Cell. Neurosci. 27, 296–305. doi: 10.1016/j.mcn.2004.07.003
Zhou, Q., and Sheng, M. (2013). NMDA receptors in nervous system diseases. Neuropharmacology 74, 69–75. doi: 10.1016/j.neuropharm.2013.03.030
Keywords: HIV-1, Tat, HAND, Alzheimer’s, glutamate, CNS, senescence
Citation: Marino J, Wigdahl B and Nonnemacher MR (2020) Extracellular HIV-1 Tat Mediates Increased Glutamate in the CNS Leading to Onset of Senescence and Progression of HAND. Front. Aging Neurosci. 12:168. doi: 10.3389/fnagi.2020.00168
Received: 13 March 2020; Accepted: 14 May 2020;
Published: 09 June 2020.
Edited by:
Robert Petersen, Central Michigan University, United StatesReviewed by:
Jonathan David Geiger, University of North Dakota, United StatesTiantian Zhang, Ocean University of China, China
Copyright © 2020 Marino, Wigdahl and Nonnemacher. This is an open-access article distributed under the terms of the Creative Commons Attribution License (CC BY). The use, distribution or reproduction in other forums is permitted, provided the original author(s) and the copyright owner(s) are credited and that the original publication in this journal is cited, in accordance with accepted academic practice. No use, distribution or reproduction is permitted which does not comply with these terms.
*Correspondence: Michael R. Nonnemacher, mrn25@drexel.edu