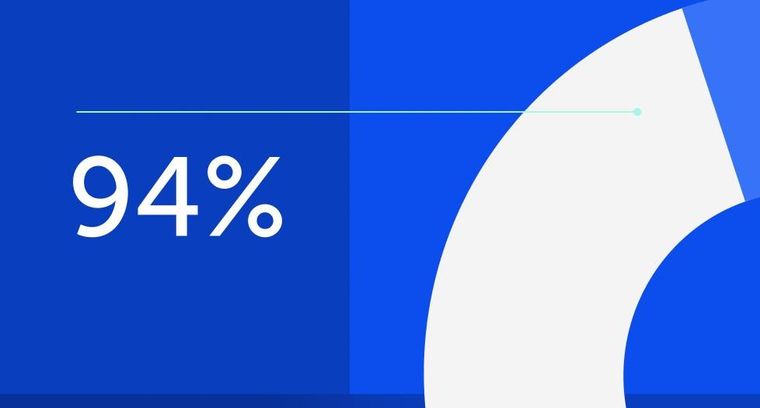
94% of researchers rate our articles as excellent or good
Learn more about the work of our research integrity team to safeguard the quality of each article we publish.
Find out more
ORIGINAL RESEARCH article
Front. Aging Neurosci., 07 May 2020
Sec. Cellular and Molecular Mechanisms of Brain-aging
Volume 12 - 2020 | https://doi.org/10.3389/fnagi.2020.00122
This article is part of the Research TopicPotential Neurotoxic Effects of Perioperative FactorsView all 25 articles
Previous studies have shown multiple mechanisms and pathophysiological changes after anesthesia, and genome-wide studies have been implemented in the studies of brain aging and neurodegenerative diseases. However, the genome-wide gene expression patterns and modulation networks after general anesthesia remains to be elucidated. Therefore, whole transcriptome microarray analysis was used to explore the coding gene expression patterns in the hippocampus of aged rats after sevoflurane anesthesia. Six hundred and thirty one upregulated and 183 downregulated genes were screened out, then 44 enriched terms of biological process, 16 of molecular function and 18 of the cellular components were identified by Gene Ontology (GO) and KEGG analysis. Among them, oxidative stress, metabolism, aging, and neurodegeneration were the most enriched biological processes and changed functions. Thus, involved genes of these processes were selected for qPCR verification and a good consistency was confirmed. The potential signaling pathways were further constructed including mitochondrion and oxidative stress-related Hifs-Prkcd-Akt-Nfe2l2-Sod1 signaling, multiple metabolism signaling (Scd2, Scap-Hmgcs2, Aldh18a1-Glul and Igf1r), as well as aging and neurodegeneration related signaling (Spidr-Ercc4-Cdkn1a-Pmaip1 and Map1lc3b). These results provide potential therapeutic gene targets for brain function modulation and memory formation process after inhaled anesthesia in the elderly, which could be valuable for preventing postoperative brain disorders and diseases, such as perioperative neurocognitive disorders (PND), from the genetic level in the future.
Neurodegenerations, including Alzheimer’s disease (AD), represent important causes for the brain’s aging processes and related cognitive dysfunction and dementia (Wyss-Coray, 2016). For the pathogenesis of stroke, conventional risk factors explain only a small proportion of causes, and evidence from twins and family history study suggests that genetic predisposition is important (Dichgans, 2007). In common with many other complex diseases, in which environmental risk factors are thought to interact with multiple genes, the identification of the underlying molecular mechanisms and genes contributing to degenerated brain diseases is valuable and challenging. Candidate gene studies have produced few replicable associations (Dichgans and Markus, 2005). More recently, genome-wide association studies, using microarray platforms, have allowed a deeper understanding of the molecular factors involved in the pathophysiology of degenerated brain disease. These studies identified multiple susceptibility loci for neurodegeneration (Harold et al., 2009; Lambert et al., 2009; Seshadri et al., 2010; Hollingworth et al., 2011), and these genes were clustered into pathways including inflammation and immune response, lipid metabolism, endocytosis/intracellular trafficking (Kunkle et al., 2019). Studies also found that oxidative stress is associated with neurodegenerative disorders (Coyle and Puttfarcken, 1993). And oxidative stress (Chamorro et al., 2016), lipid metabolism, blood circulation (Ji et al., 2017), multi-organism process, protein catabolic metabolism (Cui et al., 2018), and autophagy (Menzies et al., 2015) are the major shared genetic etiologies for stroke. Nevertheless, the are few studies about the gene network and pathophysiology for brain function modulation during the perioperative context.
Sixty-six million patients over 65 years of old worldwide undergo surgeries each year, including 8.5 million AD patients (Xie and Xu, 2013). Up to 40% of these patients suffer from perioperative neurocognitive disorders (PND), which includes postoperative cognitive dysfunction, postoperative delirium, et cetera (Monk et al., 2008; Evered et al., 2018). Anesthesia, surgical trauma, aging, as well as preoperative cognitive impairment propose to the onset of PND (Monk et al., 2008; Schenning et al., 2016; Racine et al., 2018). Meanwhile, neuroinflammation, mitochondrial dysfunction and oxidative stress (Fischer and Maier, 2015), DNA damage and apoptosis (Madabhushi et al., 2014), synaptic plasticity dysfunction (Li X. M. et al., 2014), amyloid plaques and neurofibrillary tangles (Ni et al., 2013) could be the contributing pathological factors. Ours and related researches have indicated that inhaled general anesthesia plays a major role in the PND (Xu et al., 2014; Ni et al., 2015), however, the gene expression patterns and modulation networks during general anesthesia remains to be elucidated. Therefore, we used the genome-wide screen to explore the gene expression patterns in the hippocampus of aged rats after sevoflurane anesthesia. And we established functional annotation of differentially expressed genes, modulation networks, as well as potential signaling pathways during the process, to provide insights into the monolithic mechanisms for inhaled anesthesia and related brain function modulation and memory formation.
Male Sprague–Dawley rats, 18-month old, weighing 550–600 g, were used in the studies. Before sevoflurane exposure, the rats were maintained on a standard housing condition with food and water ad libitum for 2 weeks.
The animal protocol was approved by the Peking University biomedical ethics committee experimental animal ethics branch (No. LA2018085). The rats were randomly assigned to control and sevoflurane groups. Minimum alveolar concentration (MAC) of sevoflurane for aged rats has been reported as 2.4–2.7% (Li X. Q. et al., 2014). In the present study, rats in the sevoflurane group received 2.5% sevoflurane in 100% oxygen for 4 h in the anesthetizing chamber, whereas the control group received 100% oxygen for 4 h in an identical chamber. The rats breathed spontaneously, and the anesthetic and oxygen concentrations were monitored continuously (Datex, Tewksbury, MA, USA). Temperature of the anesthetizing chamber was controlled to maintain the rectal temperature of the animals at 37 ± 0.5°C. Four hours sevoflurane anesthesia has been shown not to significantly alter values of blood pressure and blood gas in our preliminary experiment. After the termination of sevoflurane anesthesia, rats were placed in a chamber containing 100% oxygen until the regain of consciousness 20 min later. The rats were sacrificed by decapitation at the end of the experiments. The brain tissues were removed, and the hippocampus was dissected out and frozen in liquid nitrogen for the subsequent experiments.
Total RNAs were isolated from the hippocampus using trizol reagent (Invitrogen, Carlsbad, CA, USA), then digested with RNase-Free DNase to remove residual DNAs. The RNA concentrations were analyzed using the Nanodrop2000 (Thermo Fisher Scientific), then total RNA (2 μg) was reverse-transcribed using the GoScriptTM ReverseTranscription System (Promega, Madison, WI, USA).
Whole transcriptome microarray analysis was performed using GeneChip™ Rat Transcriptome Array 1.0 (Affymetrix, Santa Clara, CA, USA), and the result data were deposited in NCBI with the GEO accession code GSE141242. Briefly, isolated RNA (100 ng) was mixed with 1.5 μl of Poly-A RNA control solution and subjected to reverse transcription. The obtained cDNA was used for in vitro transcription to prepare antisense RNA (aRNA) by incubation at 40°C for 16 h. Then, the aRNA was applied for the second round of sense cDNA synthesis using the WT Expression kit (Ambion, Austin, TX, USA). The obtained cDNA was used for biotin labeling and fragmentation by Affymetrix GeneChip® WT Terminal Labeling and Hybridization. Biotin-labeled fragments of cDNA (5.5 μg) were hybridized to the Affymetrix® Rat Transcriptome Array Strip (45°C/24 h), and up to 25 unique probe sequences were hybridized to a single transcript. Following hybridization, each array strip was washed and stained using the Fluidics Station of GeneChip® Scanner 3000 7G system (Affymetrix, Santa Clara, CA, USA). The array strips were scanned using the Imaging Station of the GeneChip® Scanner 3000 7G system. Gene Ontology (GO) functional annotation and Kyoto Encyclopedia of Genes and Genomes (KEGG) pathway enrichment analyses were performed for DEGs using Database for Annotation, Visualization, and Integrated Discovery (DAVID1). GO enrichment analysis contains three categories: biological process, molecular function, and cellular component.
The significances of genes changes were calculated by −log10 (p-value), and higher −log10 (p-value) indicated that the gene was with more significant changes. We selected the top differentially expressed genes for qPCR verification.
qPCR was performed on the CFX96 Real-Time PCR Detection System (Bio-Rad, Hercules, CA, USA). Amplification mixture consisted of PowerUpTM SYBR® Green master mix (Thermo Fisher Scientific), 10 μM forward and reverse primers (Invitrogen, Carlsbad, CA, USA) and approximately 1.5 μl of cDNA template. Primer sequences were obtained from the literature and checked for their specificity through in silico PCR. The forward and reverse primers are shown in Table 1. Amplification was carried out with an initial denaturation step at 95°C for 2 min followed by 45 cycles of 95°C for 10 s, 55°C for 30 s and 60°C for 30 s, then 65°C for 2 min in 10 μl reaction volume. All reactions were run in duplicate and the results were averaged from six independent studies. qPCR was quantified in two steps, first, β-actin levels were used to normalize target gene levels [ΔCycle threshold (ΔCt) = Cttarget gene—Ctβ-actin, target gene level = 2-ΔCt]. Second, the target gene levels of the sevoflurane group were presented as the percentage of those of the control group, and 100% of the target gene levels referred to the control levels.
Immunofluorescence was performed to determine the expression of typical genes, as described in our previous studies (Ni et al., 2015). The hippocampus was fixed with 4% paraformaldehyde for 24 h, cryoprotected with 30% sucrose for 48 h, and sectioned using a cryostat (Cryotome E, Thermo Fisher, Waltham, MA, USA). Coronal sections (10 μm thickness) were incubated with HIF3α antibody (1:200 dilution; Abcam, Cambridge, UK), HMGCS2 antibody (1:100 dilution; Abcam, Cambridge, UK) or p21 antibody (1:100 dilution; Abcam, Cambridge, UK) overnight at 4°C, followed by incubation with a goat anti-rabbit FITC conjugated antibody (1:400 dilution; Servicebio, Wuhan, China) for 50 min at room temperature. Nuclei were subsequently counterstained with DAPI (1:5,000 dilution; Servicebio, Wuhan, China) for 10 min at room temperature. Images were captured using a Nikon Eclipse Ti confocal microscope. Hippocampal subregions CA1 and DG serve important roles in memory formation and other functions, these two regions were analyzed for HIF3α, HMGCS2, and p21 expressions.
The FCT (Xeye CPP, Beijing MacroAmbition S&T Development, Beijing, China) was used to assess the cognitive function of rats after sevoflurane anesthesia as described in previous studies (Dong and Li, 2014; Cheng et al., 2015) with modification. FCT consisted of a training process at 3 h after the sevoflurane anesthesia and the evaluations at 2 and 7 days after anesthesia. In the training process, rats were placed in the context chamber to acclimate for 180 s, then they received a 2 Hz pulsating tone (80 dB, 3,600 Hz) for 60 s co-terminated with a mild foot shock (0.8 mA, a 0.5 s). In the evaluations, the hippocampal-dependent memory was assessed by the freezing time during exposure to a novel context test (the test was performed in the same chamber but with no cues or shock), while the hippocampal independent memory was assessed by the freezing time during exposure to the tone stimulus (the test was performed in an alternative context and with no shock; Chowdhury et al., 2005).
Statistical analysis was performed with Graphpad Prism 7.0 software. Quantitative data are presented as the mean ± SD. Non-paired two-tailed Student’s t-test was used to determine significant differences between the two groups. One-way ANOVA with Bonferroni’s multiple comparison test was used to analyze significant differences between multiple groups. p < 0.05 was considered significant. The microarray analysis was performed by Expression Console and Transcriptome Analysis Console Software. One-way ANOVA was applied. The p-value was adjusted with the FDR method (Benjamini Hochberg procedure). RNAs were screened with p < 0.05. The significance of GO and KEGG enrichment was calculated by the hypergeometric distribution and Fisher exact test, and a lower p-value indicated that the specific term was more significantly enriched. Two-way repeated-measures analysis of variance followed by a post hoc Bonferroni test was performed to analyze the results of behavioral studies. Values of p < 0.05 were considered to be significant.
Aged rats were assigned to control and sevoflurane groups. The vital signs and arterial blood gas analysis results during anesthesia were within the normal range. Due to previous studies from ours and other groups, multiple pathophysiological changes in the hippocampus emerged 3–12 h after anesthesia, and for oxidative stress, even immediately after anesthesia (Zhang et al., 2012; Li et al., 2013). And inhaled anesthetics could affect the hippocampus related behavioral function from 3 h after anesthesia (Zhang et al., 2012), so the hippocampus was dissected and tested 3 h after anesthesia in the present study.
The whole transcriptome gene expressions in the hippocampus of aged rats were detected by whole transcriptome microarray analysis (GeneChip™ Rat Transcriptome Array, n = 3). The microarray analysis was performed by Expression Console and Transcriptome Analysis Console Software. One-way ANOVA was applied and the p-value was adjusted with the FDR method. And the genome-wide map of all autosomal and heterosomal coding and complex genes was represented as a circular ideogram, composed of concentric circles depicting the entire autosome complement, with chromosomal location annotated in a clockwise manner and statistical significance indicated by radial arrangements and color codes. The black innermost ring (with vertical lines) represents autosome ideograms (annotated is the chromosomal number), with the pter-qter orientation in a clockwise direction. Small red lines represent the centromeres within each chromosome. Red dots outside the ideograms mark genes with expression (p < 0.05), while green dots inside mark genes with decreased expression (p < 0.05). The dot position marks the location of the Illumina 450K probe distribution along the genome. The second innermost black circle represents baseline (zero) and the β-value difference between sevoflurane and control groups. Red lines signify increased gene expression regions and green lines signify decreased gene expression regions, with the length of each line representing the difference level (fold change). The names of DEGs match the Ensembl gene database were listed in the two outermost circles, the first outermost circle listed DEGs with increased expression (Red), and the second outermost circle listed DEGs with decreased expression (green, Figure 1).
Figure 1. Circos plot of genome-wide coding gene expression differences of rat hippocampi in sevoflurane group vs. control condition (n = 3). The black innermost ring (with vertical lines) represents autosome ideograms (annotated is the chromosomal number), with the pter-qter orientation in a clockwise direction. Small red lines represent the centromeres within each chromosome. Dots outside the ideograms mark gene expression increase (red dots denote significantly increased mRNA signal), while dots inside mark gene expression decrease (green dots denote significantly decreased mRNA signal). The dot position marks the location of the Illumina 450K probe distribution along the genome. The second outermost black circle represents baseline (zero) and the β-value difference between isoflurane anesthesia and control condition. Red lines signify increased gene expression regions and green lines signify decreased gene expression regions, with the length of each line representing the difference level (p < 0.05). The last two circles show the RefSeq genes associated with different signal intensity (p < 0.05, and within the Ensembl database). Outer circle (red) shows genes with increased signal, and inner circle (green) shows genes with decreased signal.
The scatter plot indicated the variation in hippocampal gene expressions between the sevoflurane group and control condition. The values corresponding to the X- and Y-axes in the scatter plot are the normalized signal values of the control and sevoflurane groups (log2 scaled). Expression values are represented in different colors, indicating expression levels above and below the median expression level across all samples. The red dots indicate increased-expressed genes, while the green dots indicate decreased-expressed genes of the sevoflurane group compared with the control condition (p < 0.05, Figure 2A). Hierarchical cluster analysis showed differentially expressed genes in the sevoflurane group compared to the control condition. The non-paired t-test was used to determine the differences between the two groups. We identified 814 differentially expressed genes (DEGs, p < 0.05), 631 of which were down-regulated and 183 were up-regulated (Figure 2B).
Figure 2. Differential expression of mRNAs in the hippocampus (n = 6). (A) The scatter plot indicated the variation in hippocampal gene expressions between the sevoflurane group and control condition. The values corresponding to the X- and Y-axes in the scatter plot are the normalized signal values of the control and sevoflurane groups (log2 scaled). (B) Hierarchical cluster analysis showed differentially expressed genes between groups from microarray analysis (p < 0.05).
To explore the pathophysiologic mechanism of sevoflurane anesthesia-related brain dysfunction, enrichment analysis was carried out. The significance of GO and KEGG enrichment was calculated by the hypergeometric distribution and Fisher exact test, and a lower p-value [higher −log10 (p-value)] indicated that the specific term was more significantly enriched. The significance of GO and KEGG enrichment was calculated by the hypergeometric distribution and Fisher exact test, and a lower p-value indicated that the specific term was more significantly enriched. The results of DAVID for GO and KEGG analysis revealed that 44 terms of biological process, 16 terms of molecular function, and 18 terms of the cellular component were significantly enriched after sevoflurane anesthesia (p < 0.05), respectively. Among them, oxidative stress, metabolism, aging, and neurodegeneration were most enriched biological processes and changed functions after sevoflurane. Six GO terms of oxidative stress, 28 GO and 7 KEGG terms of metabolism, 12 GO terms of aging and neurodegeneration, and 20 terms of the cellular component were significantly enriched.
The typical terms were displayed and ranked according to the value of −log10 (Enrichment p-value, Figure 3). Previous studies have found that oxidative stress is involved in the development of AD, Parkinson’s disease and other neurodegenerations (Giasson et al., 2000). And we focused firstly on the enriched GO terms related to oxidative stress. The terms include mitochondrion, response to hypoxia, cellular response to hypoxia, cellular response to oxidative stress, positive regulation of superoxide anion generation and cellular response to mechanical stimulus, and the −log10 (p-value) of these terms were 5.32, 4.6, 2.79, 2.21, 1.37 and 1.13, respectively (Figure 3A). Our previous results also indicate that elevated reactive oxygen species (ROS) and related DNA damage are involved in anesthesia-related pathophysiological changes (Ni et al., 2017).
Figure 3. Gene Ontology (GO) and KEGG enrichment analysis of differentially expressed genes using the DAVID database. The typical terms were displayed and ranked according to the value of −log10 (p-value), including six GO terms related to oxidative stress (A), 28 GO terms and seven KEGG terms related to metabolism (B,C), 12 GO terms related to aging and neurodegeneration (D), and 20 GO terms of cellular component (E).
Enriched metabolic terms including energy, carbohydrate, lipid, nucleotides and amino acid metabolism, were associated with the most potential target genes (Figures 3B,C). Glucose transport, long-chain fatty acid metabolic process, and protein binding were top significant enriched GO terms in carbohydrate, lipid, and amino acid-related metabolic process, and the −log10 (p-value) were 2.26, 1.75 and 4.18, respectively (Figure 3B). Then KEGG pathway analysis was employed to reveal involved molecular interaction, reaction and relation networks after sevoflurane anesthesia. Figure 3C highlighted seven significantly enriched signaling pathways in our annotation with –log10 (p-value) > 1, including certain signaling pathways such as adipocytokine signaling pathway, insulin resistance, metabolic pathways, lysine degradation, inositol phosphate metabolism, and biosynthesis of amino acids. Previous studies show that apolipoprotein E plays a central role in the clearance of β-amyloid (Aβ) from the brain (Cramer et al., 2012) and insulin pathway is involved in resistance to oxidative stress and aging (Byrne et al., 2014). The present results indicate that the sevoflurane anesthesia cloud also alters multiple metabolic pathways and processes.
Enriched GO terms related to aging and neurodegeneration include aging, regulation of cell cycle, negative regulation of cell growth, negative regulation of neuron apoptotic process, intrinsic apoptotic signaling pathway in response to DNA damage by p53 class mediator, positive regulation of extrinsic apoptotic signaling pathway via death domain receptors, apoptotic process, negative regulation of apoptotic process and positive regulation of MAPK cascade, and −log10 (p-value) of these terms were 2.84, 2.68, 2.14, 1.87, 1.20, 1.14, 1.06, 1.04 and 1.01, respectively (Figure 3D). Apoptosis and related pathway contribute to the Aβ neurotoxicity in AD, neurodegeneration and dementia (Gervais et al., 1999), and DNA damage also involves in the processes of aging (Lu et al., 2004). And the present results indicate that apoptosis, DNA damage, and other aging neurodegenerative mechanisms are activated, and related gene expressions have been changed in the aged brain after inhaled anesthesia stimulation.
Enriched GO terms of cellular components were ranked according to the location of cellular organelles (from cell membrane to nucleus, Figure 3E). Cytosol, extracellular exosome, membrane, and mitochondrial inner membrane were top significant enriched terms, −log10 (p-value) of which was 3.9, 3.09, 2.45 and 4.18, respectively. These cellular components could play important roles in sevoflurane-induced pathophysiologic changes. Among which, the mitochondrion is a critical regulator for cell death and mitochondrial dysfunction occurs early and acts causally in multiple disease pathogenesis. Mutations in mitochondrial DNA and oxidative stress both contribute to the aging process, which is the greatest risk factor for neurodegenerative diseases (Lin and Beal, 2006).
Based on GO and KEGG functional annotation and enrichment analysis, involved mechanisms and genes of oxidative stress, metabolism, aging, and neurodegeneration were selected for qPCR verification (n = 6 in each group). These included eight DEGs involved in oxidative stress (Hif2a, Hif3a, Prkcd, Akt, Nfe2l2, Sod1, Scap, and Scd2), six DEGs involved in metabolism (Scap, Hmgcs2, Scd2, Aldh18a1, Glul, and Igf1r), and eight DEGs (Spdir, Ercc4, Cdkn1a, Hipk2, Mal, Pmaip1, Bmpr1b, and Map1lc3b) involved in aging and neurodegeneration processes. A good consistency between the qPCR and microarray results was confirmed in 17 genes. The non-paired t-test was used to determine significant differences between the two groups. However, qPCR validation did not show significant changes for Hipk2 (103.70 ± 9.061 vs. 100.00 ± 22.04, p = 0.8790), Mal (115.7 ± 20.74 vs. 100.00 ± 21.49, p = 0.6101) and Bmpr1b (97.88 ± 17.84 vs. 100 ± 15.52, p = 0.9300) after sevoflurane anesthesia. As data quality parameters such as array p values and fold change may exert influence on the consistency of the two methods, we assume PCR validations across different experimental conditions are more reliable according to previous studies (Morey et al., 2006). Furthermore, Hifαs, Hmgcss, and Cdkn1a are involved in multiple signaling pathways in oxidative stress, metabolism, and aging/neurodegeneration processes respectively, and were selected for immunofluorescence analysis for their expression levels and regions.
Since close monitoring excluded hypoxia during anesthesia, we assume that sevoflurane may induce perioperative oxidative stress in the brain, and activate related mechanisms and genes. Figure 4 shows oxidative stress-related signaling pathways involved in the aged hippocampus after sevoflurane anesthesia, and differently transcribed genes are shown in red. Sevoflurane activated hypoxia-inducible factors (HIFs) directly, or through Prkcd and Akt-mTOR-signaling, and DEGs include Hif2a and Hif3a. Although the increase of Hif1a expression was not significant in the present microarray, our previous studies have shown that the expression of HIF-1α increased significantly after inhaled anesthesia (Cao et al., 2018a,b). Activated HIFs involved in Scap/SREBP1 and Scd2 expression increase, and resulted in oxidative stress, then more ROS were generated. It has been reported that the balance of oxygen supply and demand relies on HIFs (Huang, 2013), and Prkcd could also control HIFs translation via AKT-mTOR signaling under hypoxic conditions (Kim et al., 2016). On another aspect, ROS products activated the Nfe2l2-antioxidant response element pathway and increased Sod1 expression. And Nfe2l2 mediated Sod1 increase could attenuate oxidative stress and protect DNA from related damage (Bordoni et al., 2019). Finally, qPCR validation for the mRNA levels of DEGs related to oxidative stress showed significant up-regulation of Hif2a (151.29 ± 28.40 vs. 100.00 ± 39.26, p = 0.027), Hif3a (242.68 ± 76.62 vs. 100.00 ± 30.69, p = 0.0017), Prkcd (175.87 ± 47.79 vs. 100.00 ± 47.95, p = 0.021), Akt (134.46 ± 21.08 vs. 100.00 ± 29.77, p = 0.043), Scap (166.98 ± 47.91 vs. 100.00 ± 53.02, p = 0.044), Scd2 (129.10 ± 20.24 vs. 100.00 ± 19.69, p = 0.030), Nfe2l2 (170.28 ± 59.04 vs. 100.00 ± 37.35, p = 0.034) and Sod1 (8.045 ± 2.39 vs. 5.34 ± 1.689, p = 0.0471) after sevoflurane anesthesia compared with control condition. As immunofluorescence shows both the presence and location of protein expression, it was selected for further protein expression verification. Due to previous studies, the hippocampal CA1 region is the substrate for long-lasting potentiation and encoding of synaptic memory (Volianskis and Jensen, 2003), dentate gyrus (DG) serves an important role in engram maintenance and remote memory generalization (Guo et al., 2018). Thus, these regions were selected as the target regions in the present study, and the results showed that the protein expression of HIF-3α in both regions increased after sevoflurane anesthesia (Figure 5).
Figure 4. Hypothetical pathway related with oxidative stress identified with altered mRNA expression in sevoflurane group vs. control condition, Regular triangle represents genes up-regulated; inverted triangle represents genes down-regulated; Graphs show the difference in expression of Hif2a, Hif3a, Akt, Nfe2l2, Prkcd, Sod1 and Scap for sevoflurane anesthesia group and controls (*p < 0.05; **p < 0.01).
Figure 5. Immunofluorescent staining of hippocampal hif3a in the CA1 region and DG region. Immunofluorescence images show RelA (FITC, green) and DAPI (blue) counterstain. In the control condition, RelA was primarily distributed in the cytosol of the pyramidal cell layer, CA1 region and the cytosol of the granular cell layer, DG region. Six hours after exposure, RelA was distributed in both nucleus and cytosol. Arrows point to the typical RelA distribution, which are provided as high magnification images. Magnification 400×, scale bar 100 and 25 μm.
Figure 6 shows metabolism-related signaling pathways involved in the aged hippocampus after sevoflurane anesthesia with DEGs. Sevoflurane activated Scap/SREBP signaling and Hmgcs2 expression. Hmgcs2 expression is both sufficient and necessary to the control of fatty acid oxidation in cells (Vila-Brau et al., 2011), and Scap/SREBP signaling also involves the process. Sevoflurane induced Scd2 expression increases and mitochondrial dysfunction related genes. Scd2 knockdown increases whole-body energy expenditure (de Moura et al., 2016), and the increased expression of Scd2 could result in energy metabolism decrease. Sevoflurane also increased Aldh18a1, Glul and Igf1r expression. Hypoxia activated proline biosynthesis via upregulation of Aldh18a1 (Tang et al., 2018), Glul is an enzyme that converts glutamate and ammonia to glutamine (Eelen et al., 2018), and Igf1r plays a central role in glucose metabolism and regulates lifespan and resistance to oxidative stress as well (Holzenberger et al., 2003). Thus, sevoflurane also affected the metabolism of protein and glucose. Then, qPCR validation for the DEGs related to metabolism showed significant changes for Scap (167.13 ± 48.00 vs. 100.00 ± 53.06 p = 0.0444), Hmgcs2 (156.52 ± 32.07 vs. 100.00 ± 27.43, p = 0.0083), Scd2 (129.10 ± 20.24 vs. 100.00 ± 19.69, p = 0.030), Glul (150.55 ± 42.23 vs. 100.00 ± 30.84, p = 0.039), Aldh18a1 (200.42 ± 90.37 vs. 100.00 ± 59.11, p = 0.046) and Igf1r (160.26 ± 44.83 vs. 4.678 ± 2.097, p = 0.047) after sevoflurane anesthesia compared with control condition. And as shown in Figure 7, the protein expression levels of HMGCS2 increased significantly in the CA1 region and DG of the hippocampus after sevoflurane anesthesia.
Figure 6. Hypothetical pathway related to metabolism identified with altered DNA expression in the sevoflurane anesthesia group in comparison with the control group. The regular triangle represents genes up-regulated; inverted triangle represents genes down-regulated; Graphs show the difference in expression of Aldh18a1, Glul, Hmgcs2, Lgf1r, Scap, and Scd2 were for sevoflurane anesthesia group and controls (*p < 0.05; **p < 0.01).
Figure 7. Immunofluorescent staining of hippocampal Hmgcs2 in the CA1 region and DG region. Immunofluorescence images show RelA (FITC, green) and DAPI (blue) counterstain. In the control condition, the staining intensity of RelA in the cytosol of the pyramidal cell layer, CA1 region and the cytosol of the granular cell layer, DG region are weak. Six hours after exposure, the staining intensity of RelA in the cytosol of cells in both the CA1 region and the DG region is obviously increased. Arrows point to the typical RelA distribution, which are provided as high magnification images. Magnification 400×, scale bar 100 and 25 μm.
Figure 8 shows aging/neurodegeneration related signaling pathways involved in the aged hippocampus after sevoflurane anesthesia with DEGs. Besides oxidative stress and metabolism-related signaling, sevoflurane affected Spidr and Ercc4 expression. Spidr involved in DNA repair, and its depletion leads to genome instability and causes hypersensitivity to DNA damaging agents (Wan et al., 2013). Ercc4 is one of the components of structure-specific endonucleases, which mediate cleavage of DNA structures formed during the repair of collapsed replication forks and double-strand breaks (Svendsen et al., 2009). These changes indicate that sevoflurane could induce DNA damage. DNA damage is a unifying mechanism in neurodegeneration (Ross and Truant, 2017), and could also involve in brain function alteration after anesthesia. Sevoflurane increased Cdkn1a and Pmaip1 expression. Activation of the tumor suppressor p53 by DNA damage induces either cell cycle arrest or apoptotic cell death, and the cytostatic effect of p53 is mediated by transcriptional activation of the cyclin-dependent kinase inhibitor p21 (coded by Cdkn1a, Seoane et al., 2002). Cdkn1a was also associated with aberrant cell-cycle and apoptosis (Khan et al., 2018), and Pmaip1 was associated with apoptosis (Zhao et al., 2014). Sevoflurane induced Map1lc3b expression, which plays an important role in autophagy (Samdal et al., 2018). Then, qPCR validation for the DEGs related to aging/neurodegeneration showed significant changes for Spidr (158.56 ± 53.87 vs. 100.00 ± 33.15, p = 0.047), Ercc4 (70.43 ± 12.22 vs. 100.00 ± 29.81, p = 0.049), Cdkn1a (206.99 ± 51.71 vs. 100.00 ± 30.31, p = 0.0014), Pmaip1 (232.86 ± 106.46 vs. 100.00 ± 44.78, p = 0.018) and Map1lc3b (141.64 ± 35.05 vs. 100.00 ± 22.76, p = 0.034) after sevoflurane anesthesia compared with control condition. The protein expression levels of p21 correlated with mRNA results and increased significantly in both the CA1 region and DG of the hippocampus after sevoflurane anesthesia (Figure 9).
Figure 8. Hypothetical pathway related to metabolism identified with altered DNA expression in the sevoflurane anesthesia group in comparison with the control group. Regular triangle represents genes up-regulated; inverted triangle represents genes down-regulated; graphs show the difference in expression of Spdir, Pmaip1, Ercc4, Map1lc3b, and Cdkn1a were for sevoflurane anesthesia group and controls (*p < 0.05; **p < 0.01).
Figure 9. Immunofluorescent staining of hippocampal Cdkn1a in the CA1 region and DG region. Immunofluorescence images show RelA (FITC, green) and DAPI (blue) counterstain. In the control condition, the staining intensity of RelA in the cytosol of the pyramidal cell layer, CA1 region and the cytosol of the granular cell layer, DG region are weak. Six hours after exposure, the staining intensity of RelA in the cytosol of cells in both the CA1 region and the DG region is obviously increased. Arrows point to the typical RelA distribution, which are provided as high magnification images. Magnification 400×, scale bar 100 and 25 μm.
To assess the relationship between sevoflurane anesthesia and hippocampus-dependent behavioral variations, a subgroup of aged rats was subjected to the FCT, consisted of a training process at 3 h after anesthesia (the same time of genomic expression analysis in the present study), and evaluations at 2 days and 7 days after anesthesia. The results showed that the freezing time decreased significantly at 7 days (21.75 ± 11.32 vs. 36.29 ± 13.50, p = 0.0091, Figure 10C), but not 2 days (34.71 ± 19.77 vs. 46.59 ± 20.33, p = 0.1609, Figure 10A) after anesthesia in the context test (reflected hippocampus-dependent memory), which suggested that sevoflurane related hippocampal-dependent cognitive dysfunction persisted for a relatively long period. During the tone test, which is related to amygdala function (Li X. Q. et al., 2014), the freezing time did not decrease significantly at 2 days (61.7 ± 31.05 vs. 59.87 ± 26.55, P = 0.8777, Figure 10B) or 7 days (45.49 ± 20.75 vs. 42.3 ± 18.19, P = 0.6933, Figure 10D) after anesthesia, which suggested that the amygdala function was not grossly impaired.
Figure 10. Fear conditioning test (FCT) consisted of a training process at 3 h after anesthesia and evaluations at 2 days and 7 days after anesthesia. The freezing time decreased significantly at 7 days (B), but not 2 days (A) after anesthesia in the context test. During the tone test, the freezing time did not decrease significantly at 2 days (C) or 7 days (D) after anesthesia. **p < 0.01 and N.S.: Not significant.
In the present study, we screened out 814 coding and complex genes that were located across 21 pairs of chromosomes in the aged hippocampus at 3 h after sevoflurane anesthesia, among which, 631 genes were upregulated and 183 genes were downregulated, and the training process of FCT was conducted at the same time. GO and KEGG analysis revealed that 44 terms of biological process, 16 terms of molecular function, and 18 terms of the cellular component were enriched after anesthesia. Among them, oxidative stress (6 GO terms), metabolism (28 GO and 7 KEGG terms), aging and neurodegeneration (12 GO terms) were the most enriched biological processes and changed functions. Candidate genes of oxidative stress (Hif2a, Hif3a, Prkcd, Akt, Nfe2l2, Sod1, Scap, and Scd2), metabolism (Scap, Hmgcs2, Scd2, Aldh18a1, Glul, and Lgf1r), aging and neurodegeneration (Spdir, Ercc4, Cdkn1a, Hipk2, Mal, Pmaip1, Bmpr1b, and Map1lc3b) were selected for qPCR verification. A good consistency between the qPCR and microarray results was confirmed, and potential functional genes and signaling pathways were constructed in these biological processes including mitochondria and oxidative stress, metabolism, aging, and neurodegeneration. The FCT results showed that sevoflurane affected memory retrieval at 7 days after anesthesia.
The training process of FCT was performed at the same time of genomic expression analysis (3 h after sevoflurane anesthesia), and the fear conditioning memory retrievals were assessed at 2 and 7 days after the training process (or anesthesia), which both reflected the recent memory. The trend of hippocampus-dependent fear memory decrease was observed at 2 days after anesthesia, but the difference was not significant. While the significant difference of freezing time was observed at 7 days after anesthesia, which indicates that sevoflurane anesthesia could accelerate hippocampus-dependent memory decline. The effects of sevoflurane on memory formation and the hippocampal genomic expression changes during memory formation could be pivotal mechanisms for these effects. Furthermore, the similar phenomenon of contextual fear memory retrieval was observed in both perioperative period (Zhang et al., 2017) and isoproterenol treatment (Qi et al., 2008), and sevoflurane exposure has been reported to affect the level of noradrenaline in the brain (Anzawa et al., 2001).
Mitochondrion and oxidative stress were top enriched terms in cellular component and biological process in the hippocampus after sevoflurane anesthesia based on GO functional annotation, and both control and anesthesia groups received the same concentration of oxygen. Thus, the results indicate that elevated ROS is involved in anesthesia-related pathophysiological changes in the brain. Oxidative stress could be generated as a consequence of antioxidant molecules decrease or inactivation, an increase of ROS and other oxidant molecules, as well as an increase of endogenous metabolites capable of autoxidation (Lushchak, 2014). Oxidative stress is related to lipid droplet accumulation and lipid peroxidation process (Liu et al., 2015), which is involved in the development of neurodegeneration (Sultana et al., 2013). A previous study shows that the effect of intermittent hypoxia on serum triglycerides levels is mediated through HIFs, and HIF inhibitors have a neuroprotective effect in hippocampal apoptosis (Kunimi et al., 2019). HIF-1 impacts on posttranscriptional regulation of SREBP-1 by the increased level of SCAP (Pallottini et al., 2008), which could increase the lipid metabolism and lead to neurodegeneration (Hallett et al., 2019). HIFs also impacts on the of acid-synthesizing enzyme, stearoyl-CoA desaturase (SCD1 and SCD2), which transcription in the hippocampus has been implicated in neurodegeneration (Vozella et al., 2017). The aging retinal pigment epithelium (RPE) expressed higher levels of the Nrf2 (encoded by Nfe2l2) target genes compared with the RPE of younger mice under unstressed conditions, suggesting an age-related increase in basal oxidative stress and that Nrf2 signaling is a promising target for novel pharmacologic or genetic therapeutic strategies against aging (Sachdeva et al., 2014).
The results indicate that multiple metabolism-related signaling pathways involved in the process of the aged hippocampus after sevoflurane anesthesia, including energy, lipids, proteins, carbohydrates, etc. Energy metabolism in the aging brain is affected by numerous factors. SCD2 is the main δ9 desaturase expressed in the central nervous system, which has been found playing important role in controlling whole-body energy expenditure (de Moura et al., 2016) and maintaining normal biosynthesis of lipids during early skin and liver development (Miyazaki et al., 2005). SREBP1c is a transcription factor that induces an entire program of de novo lipogenesis primarily in response to increased insulin, and induction of de novo lipogenesis in adipocytes under excess carbohydrate intake is likely to be primarily mediated by SREBP1c as seen in the liver (Kim et al., 1998). SREBP1c is involved in the energy metabolic effects of phenelzine in rats fed a high-fat diet (Mercader et al., 2019). Mass spectrometry and purified protein analysis identified mitochondrial HMG-CoA synthase (HMGCS) as the primary autoantigens, which are ubiquitous and partition with mitochondria, and involved in energy metabolism and oxidative stress (Toivola et al., 2015). HMGCS2 is the regulatory enzyme of ketogenesis in liver mitochondria, which serves as an alternative fuel to reduce the use of glucose during the fasting period, especially in the brain (Nakamura et al., 2014). Ammonia is a toxic product of protein catabolism and involved in glutamate metabolism changes, one of the primary roles of astrocyte is to protect neurons against excitotoxicity by taking up excess ammonia and glutamate and converting it into glutamine via the enzyme glutamine synthetase (GLUL). Gene study has found that GLUL is associated with major depressive disorder, and loss of astroglial GLUL is reported in hippocampi of epileptic patients (Zhou et al., 2019). The study also showed that insulin/insulin-like growth factor 1 (IGF1) signaling inhibits age-dependent axon regeneration and involves in neurodegeneration, and growth hormone (GH)/IGF-1 pathway plays a key role in the modulation of the aging process (Byrne et al., 2014).
The sequence of the human genome represents our genetic blueprint, and accumulating evidence suggests that loss of genomic maintenance may causally contribute to aging, and brain aging and neurodegeneration show similarities at the molecular level (Maynard et al., 2015). The most studied molecular pathways involved in neurodegeneration are inflammation and oxidative stress (Fischer and Maier, 2015), metabolism (Citron et al., 2016) and DNA damage (Madabhushi et al., 2014), which are consistent with the pathophysiological process in the hippocampus after sevoflurane anesthesia. The brain consumes oxygen at a relatively high rate, leading to the high exposure of neurons to ROS products. If antioxidants are depleted in the brain, neurons become susceptible to ROS induced DNA damage (Nakae et al., 2000). DNA damage and mitochondrial dysfunction can adversely affect neuronal functions, thus increasing the risk of neurodegenerative disease (Madabhushi et al., 2014). Neurological dysfunction has been found in individuals and mouse models with genetic errors in DNA repair genes (Jeppesen et al., 2011). ERCC4 forms a complex with ERCC1 and is required for the 5′ incision during nucleotide excision repair. And ERCC4 illustrates a critical role in DNA interstrand crosslink repair, and pathogenic variants in this gene cause segmental progeroid syndromes (Mori et al., 2018). In the brains of AD patients and AD mouse models, Aβ plaque-associated Olig2- and NG2-expressing oligodendrocyte progenitor cells, exhibit a senescence-like phenotype through the upregulation of p21 (encoded by Cdkn1a) and p16 (Zhang et al., 2019). Autophagy is critical to the maintenance of organismal homeostasis in both physiological and pathological situations. Autophagy protects neurons against regulated cell death by preventing the accumulation of cytotoxic protein aggregates and preserving metabolic homeostasis (Menzies et al., 2015). mTOR inhibitor rapamycin activates autophagy, alleviates the accumulation of Aβ and ameliorates cognitive deficits in mice expressing mutant APP (Caccamo et al., 2010). Combined with the present results, autophagy could also be the therapeutic target for anesthesia-related brain function changes.
Previous studies have shown multiple mechanisms and pathophysiological changes after anesthesia (Ni et al., 2013, 2017; Xie and Xu, 2013; Xie et al., 2013), and genome-wide association studies have been implemented in the studies of brain aging and neurodegenerative diseases (Harold et al., 2009; Lambert et al., 2009; Seshadri et al., 2010). The present study explored sevoflurane anesthesia induced genome-wide changes in the hippocampus of aged rats. Based on functional annotation, mitochondrial dysfunction and oxidative stress, metabolism changes, aging and neurodegeneration, and multiple mechanisms were found to be involved in postoperative pathophysiological processes and function modulations in the hippocampus. Potential genetic regulatory network and involved signaling pathways were established, and genes (include Hifs, Prkcd, Nfe2l2, Hmgcs2, Glul, Ercc4, Cdkn1a, Map1lc3b, etc.) participate in this genetic regulatory network. These results provide the therapeutic gene targets for brain function modulation and memory formation process, which could be valuable for preventing postoperative brain disorders and diseases, such as PND, from the genetic level in the future.
The datasets generated for this study can be found in the GEO (submission number: GSE141242).
The animal study was reviewed and approved by Peking University biomedical ethics committee experimental animal ethics branch.
YW performed the experiments, analyzed the data, and wrote the original draft of the manuscript. MQ performed the experiments, analyzed the data, and revised the manuscript. YQ and NY contributed to the experiments. KL, JY, and YZ contributed to the data analysis. XG and BM contributed to the manuscript revision. JZ contributed to the experiment design and manuscript revision. CN designed the project, supervised the experiments, drafted and revised the manuscript. All authors read and approved the final manuscript.
This work was supported by the National Natural Science Foundation of China (Grant Nos. 81771146, 81901095, 81971868 and 81400869).
The authors declare that the research was conducted in the absence of any commercial or financial relationships that could be construed as a potential conflict of interest.
Anzawa, N., Kushikata, T., Ohkawa, H., Yoshida, H., Kubota, T., and Matsuki, A. (2001). Increased noradrenaline release from rat preoptic area during and after sevoflurane and isoflurane anesthesia. Can. J. Anaesth. 48, 462–465. doi: 10.1007/bf03028309
Bordoni, M., Pansarasa, O., Dell’Orco, M., Crippa, V., Gagliardi, S., Sproviero, D., et al. (2019). Nuclear phospho-SOD1 protects DNA from oxidative stress damage in amyotrophic lateral sclerosis. J. Clin. Med. 8:E729. doi: 10.3390/jcm8050729
Byrne, A. B., Walradt, T., Gardner, K. E., Hubbert, A., Reinke, V., and Hammarlund, M. (2014). Insulin/IGF1 signaling inhibits age-dependent axon regeneration. Neuron 81, 561–573. doi: 10.1016/j.neuron.2013.11.019
Caccamo, A., Majumder, S., Richardson, A., Strong, R., and Oddo, S. (2010). Molecular interplay between mammalian target of rapamycin (mTOR), amyloid-β, and Tau: effects on cognitive impairments. J. Biol. Chem. 285, 13107–13120. doi: 10.1074/jbc.M110.100420
Cao, Y., Li, Z., Li, H., Ni, C., Li, L., Yang, N., et al. (2018a). Hypoxia-inducible factor-1α is involved in isoflurane-induced blood-brain barrier disruption in aged rats model of POCD. Behav. Brain Res. 339, 39–46. doi: 10.1016/j.bbr.2017.09.004
Cao, Y., Li, Z., Ma, L., Ni, C., Li, L., Yang, N., et al. (2018b). Isofluraneinduced postoperative cognitive dysfunction is mediated by hypoxiainducible factor1-α-dependent neuroinflammation in aged rats. Mol. Med. Rep. 17, 7730–7736. doi: 10.3892/mmr.2018.8850
Chamorro, Á., Dirnagl, U., Urra, X., and Planas, A. M. (2016). Neuroprotection in acute stroke: targeting excitotoxicity, oxidative and nitrosative stress, and inflammation. Lancet Neurol. 15, 869–881. doi: 10.1016/s1474-4422(16)00114-9
Cheng, B., Zhang, Y., Wang, A., Dong, Y., and Xie, Z. (2015). Vitamin C attenuates isoflurane-induced caspase-3 activation and cognitive impairment. Mol. Neurobiol. 52, 1580–1589. doi: 10.1007/s12035-014-8959-3
Chowdhury, N., Quinn, J. J., and Fanselow, M. S. (2005). Dorsal hippocampus involvement in trace fear conditioning with long, but not short, trace intervals in mice. Behav. Neurosci. 119, 1396–1402. doi: 10.1037/0735-7044.119.5.1396
Citron, B. A., Ameenuddin, S., Uchida, K., Suo, W. Z., SantaCruz, K., and Festoff, B. W. (2016). Membrane lipid peroxidation in neurodegeneration: role of thrombin and proteinase-activated receptor-1. Brain Res. 1643, 10–17. doi: 10.1016/j.brainres.2016.04.071
Coyle, J. T., and Puttfarcken, P. (1993). Oxidative stress, glutamate, and neurodegenerative disorders. Science 262, 689–695. doi: 10.1126/science.7901908
Cramer, P. E., Cirrito, J. R., Wesson, D. W., Lee, C. Y., Karlo, J. C., Zinn, A. E., et al. (2012). ApoE-directed therapeutics rapidly clear β-amyloid and reverse deficits in AD mouse models. Science 335, 1503–1506. doi: 10.1126/science.1217697
Cui, P., Ma, X., Li, H., Lang, W., and Hao, J. (2018). Shared biological pathways between Alzheimer’s disease and ischemic stroke. Front. Neurosci. 12:605. doi: 10.3389/fnins.2018.00605
de Moura, R. F., Nascimento, L. F., Ignacio-Souza, L. M., Morari, J., Razolli, D. S., Solon, C., et al. (2016). Hypothalamic stearoyl-CoA desaturase-2 (SCD2) controls whole-body energy expenditure. Int. J. Obes. 40, 471–478. doi: 10.1038/ijo.2015.188
Dichgans, M. (2007). Genetics of ischaemic stroke. Lancet Neurol. 6, 149–161. doi: 10.1016/S1474-4422(07)70028-5
Dichgans, M., and Markus, H. S. (2005). Genetic association studies in stroke: methodological issues and proposed standard criteria. Stroke 36, 2027–2031. doi: 10.1161/01.str.0000177498.21594.9e
Dong, X., and Li, Y. (2014). Peritraumatic startle response predicts the vulnerability to develop PTSD-like behaviors in rats: a model for peritraumatic dissociation. Front. Behav. Neurosci. 8:14. doi: 10.3389/fnbeh.2014.00014
Eelen, G., Dubois, C., Cantelmo, A. R., Goveia, J., Bruning, U., DeRan, M., et al. (2018). Role of glutamine synthetase in angiogenesis beyond glutamine synthesis. Nature 561, 63–69. doi: 10.1038/s41586-018-0466-7
Evered, L., Silbert, B., Knopman, D. S., Scott, D. A., DeKosky, S. T., Rasmussen, L. S., et al. (2018). Recommendations for the nomenclature of cognitive change associated with anaesthesia and surgery-2018. Anesthesiology 129, 872–879. doi: 10.1097/ALN.0000000000002334
Fischer, R., and Maier, O. (2015). Interrelation of oxidative stress and inflammation in neurodegenerative disease: role of TNF. Oxid. Med. Cell. Longev. 2015:610813. doi: 10.1155/2015/610813
Gervais, F. G., Xu, D., Robertson, G. S., Vaillancourt, J. P., Zhu, Y., Huang, J., et al. (1999). Involvement of caspases in proteolytic cleavage of Alzheimer’s amyloid-β precursor protein and amyloidogenic A β peptide formation. Cell 97, 395–406. doi: 10.1016/s0092-8674(00)80748-5
Giasson, B. I., Duda, J. E., Murray, I. V., Chen, Q., Souza, J. M., Hurtig, H. I., et al. (2000). Oxidative damage linked to neurodegeneration by selective α-synuclein nitration in synucleinopathy lesions. Science 290, 985–989. doi: 10.1126/science.290.5493.985
Guo, N., Soden, M. E., Herber, C., Kim, M. T., Besnard, A., Lin, P., et al. (2018). Dentate granule cell recruitment of feedforward inhibition governs engram maintenance and remote memory generalization. Nat. Med. 24, 438–449. doi: 10.1038/nm.4491
Hallett, P. J., Engelender, S., and Isacson, O. (2019). Lipid and immune abnormalities causing age-dependent neurodegeneration and Parkinson’s disease. J. Neuroinflammation 16:153. doi: 10.1186/s12974-019-1532-2
Harold, D., Abraham, R., Hollingworth, P., Sims, R., Gerrish, A., Hamshere, M. L., et al. (2009). Genome-wide association study identifies variants at CLU and PICALM associated with Alzheimer’s disease. Nat. Genet. 41, 1088–1093. doi: 10.1038/ng.440
Hollingworth, P., Harold, D., Sims, R., Gerrish, A., Lambert, J. C., Carrasquillo, M. M., et al. (2011). Common variants at ABCA7, MS4A6A/MS4A4E, EPHA1, CD33 and CD2AP are associated with Alzheimer’s disease. Nat. Genet. 43, 429–435. doi: 10.1038/ng.803
Holzenberger, M., Dupont, J., Ducos, B., Leneuve, P., Geloen, A., Even, P. C., et al. (2003). IGF-1 receptor regulates lifespan and resistance to oxidative stress in mice. Nature 421, 182–187. doi: 10.1038/nature01298
Huang, L. E. (2013). Biochemistry. How HIF-1α handles stress. Science 339, 1285–1286. doi: 10.1126/science.1236966
Jeppesen, D. K., Bohr, V. A., and Stevnsner, T. (2011). DNA repair deficiency in neurodegeneration. Prog. Neurobiol. 94, 166–200. doi: 10.1016/j.pneurobio.2011.04.013
Ji, L. D., Hu, S. P., Li, J. Y., Yao, B. B., Shen, Q. J., and Xu, J. (2017). Shared genetic etiology of hypertension and stroke: evidence from bioinformatics analysis of genome-wide association studies. J. Hum. Hypertens. 32, 34–39. doi: 10.1038/s41371-017-0012-3
Khan, M. M., Xiao, J., Patel, D., and LeDoux, M. S. (2018). DNA damage and neurodegenerative phenotypes in aged Ciz1 null mice. Neurobiol. Aging 62, 180–190. doi: 10.1016/j.neurobiolaging.2017.10.014
Kim, H., Na, Y. R., Kim, S. Y., and Yang, E. G. (2016). Protein kinase C isoforms differentially regulate hypoxia-inducible factor-1α accumulation in cancer cells. J. Cell. Biochem. 117, 647–658. doi: 10.1002/jcb.25314
Kim, J. B., Sarraf, P., Wright, M., Yao, K. M., Mueller, E., Solanes, G., et al. (1998). Nutritional and insulin regulation of fatty acid synthetase and leptin gene expression through ADD1/SREBP1. J. Clin. Invest. 101, 1–9. doi: 10.1172/jci1411
Kunimi, H., Miwa, Y., Inoue, H., Tsubota, K., and Kurihara, T. (2019). A novel HIF inhibitor halofuginone prevents neurodegeneration in a murine model of retinal ischemia-reperfusion. Int. J. Mol. Sci. 20:E3171. doi: 10.3390/ijms20133171
Kunkle, B. W., Grenier-Boley, B., Sims, R., Bis, J. C., Damotte, V., Naj, A. C., et al. (2019). Genetic meta-analysis of diagnosed Alzheimer’s disease identifies new risk loci and implicates Aβ, tau, immunity and lipid processing. Nat. Genet. 51, 414–430. doi: 10.1038/s41588-019-0358-2
Lambert, J. C., Heath, S., Even, G., Campion, D., Sleegers, K., Hiltunen, M., et al. (2009). Genome-wide association study identifies variants at CLU and CR1 associated with Alzheimer’s disease. Nat. Genet. 41, 1094–1099. doi: 10.1038/ng.439
Li, X. Q., Cao, X. Z., Wang, J., Fang, B., Tan, W. F., and Ma, H. (2014). Sevoflurane preconditioning ameliorates neuronal deficits by inhibiting microglial MMP-9 expression after spinal cord ischemia/reperfusion in rats. Mol. Brain 7:69. doi: 10.1186/s13041-014-0069-7
Li, Z. Q., Rong, X. Y., Liu, Y. J., Ni, C., Tian, X. S., Mo, N., et al. (2013). Activation of the canonical nuclear factor-κB pathway is involved in isoflurane-induced hippocampal interleukin-1β elevation and the resultant cognitive deficits in aged rats. Biochem. Biophys. Res. Commun. 438, 628–634. doi: 10.1016/j.bbrc.2013.08.003
Li, X. M., Su, F., Ji, M. H., Zhang, G. F., Qiu, L. L., Jia, M., et al. (2014). Disruption of hippocampal neuregulin 1-ErbB4 signaling contributes to the hippocampus-dependent cognitive impairment induced by isoflurane in aged mice. Anesthesiology 121, 79–88. doi: 10.1097/aln.0000000000000191
Lin, M. T., and Beal, M. F. (2006). Mitochondrial dysfunction and oxidative stress in neurodegenerative diseases. Nature 443, 787–795. doi: 10.1038/nature05292
Liu, L., Zhang, K., Sandoval, H., Yamamoto, S., Jaiswal, M., Sanz, E., et al. (2015). Glial lipid droplets and ROS induced by mitochondrial defects promote neurodegeneration. Cell 160, 177–190. doi: 10.1016/j.cell.2014.12.019
Lu, T., Pan, Y., Kao, S. Y., Li, C., Kohane, I., Chan, J., et al. (2004). Gene regulation and DNA damage in the ageing human brain. Nature 429, 883–891. doi: 10.1038/nature02661
Lushchak, V. I. (2014). Free radicals, reactive oxygen species, oxidative stress and its classification. Chem. Biol. Interact. 224, 164–175. doi: 10.1016/j.cbi.2014.10.016
Madabhushi, R., Pan, L., and Tsai, L. H. (2014). DNA damage and its links to neurodegeneration. Neuron 83, 266–282. doi: 10.1016/j.neuron.2014.06.034
Maynard, S., Fang, E. F., Scheibye-Knudsen, M., Croteau, D. L., and Bohr, V. A. (2015). DNA damage, DNA repair, aging, and neurodegeneration. Cold Spring Harb. Perspect. Med. 5:a025130. doi: 10.1101/cshperspect.a025130
Menzies, F. M., Fleming, A., and Rubinsztein, D. C. (2015). Compromised autophagy and neurodegenerative diseases. Nat. Rev. Neurosci. 16, 345–357. doi: 10.1038/nrn3961
Mercader, J., Sabater, A., Le Gonidec, S., Decaunes, P., Chaplin, A., Gómez-Zorita, S., et al. (2019). Oral phenelzine treatment mitigates metabolic disturbances in mice fed a high-fat diet. J. Pharmacol. Exp. Ther. 371, 555–566. doi: 10.1124/jpet.119.259895
Miyazaki, M., Dobrzyn, A., Elias, P. M., and Ntambi, J. M. (2005). Stearoyl-CoA desaturase-2 gene expression is required for lipid synthesis during early skin and liver development. Proc. Natl. Acad. Sci. U S A 102, 12501–12506. doi: 10.1073/pnas.0503132102
Monk, T. G., Weldon, B. C., Garvan, C. W., Dede, D. E., van der Aa, M. T., Heilman, K. M., et al. (2008). Predictors of cognitive dysfunction after major noncardiac surgery. Anesthesiology 108, 18–30. doi: 10.1097/01.anes.0000296071.19434.1e
Morey, J. S., Ryan, J. C., and Van Dolah, F. M. (2006). Microarray validation: factors influencing correlation between oligonucleotide microarrays and real-time PCR. Biol. Proced. Online 8, 175–193. doi: 10.1251/bpo126
Mori, T., Yousefzadeh, M. J., Faridounnia, M., Chong, J. X., Hisama, F. M., Hudgins, L., et al. (2018). ERCC4 variants identified in a cohort of patients with segmental progeroid syndromes. Hum. Mutat. 39, 255–265. doi: 10.1002/humu.23367
Nakae, D., Akai, H., Kishida, H., Kusuoka, O., Tsutsumi, M., and Konishi, Y. (2000). Age and organ dependent spontaneous generation of nuclear 8-hydroxydeoxyguanosine in male Fischer 344 rats. Lab. Invest. 80, 249–261. doi: 10.1038/labinvest.3780028
Nakamura, M. T., Yudell, B. E., and Loor, J. J. (2014). Regulation of energy metabolism by long-chain fatty acids. Prog. Lipid Res. 53, 124–144. doi: 10.1016/j.plipres.2013.12.001
Ni, C., Li, C., Dong, Y., Guo, X., Zhang, Y., and Xie, Z. (2017). Anesthetic isoflurane induces DNA damage through oxidative stress and p53 pathway. Mol. Neurobiol. 54, 3591–3605. doi: 10.1007/s12035-016-9937-8
Ni, C., Li, Z., Qian, M., Zhou, Y., Wang, J., and Guo, X. (2015). Isoflurane induced cognitive impairment in aged rats through hippocampal calcineurin/NFAT signaling. Biochem. Biophys. Res. Commun. 460, 889–895. doi: 10.1016/j.bbrc.2015.03.083
Ni, C., Tan, G., Luo, A., Qian, M., Tang, Y., Zhou, Y., et al. (2013). Melatonin premedication attenuates isoflurane anesthesia-induced β-amyloid generation and cholinergic dysfunction in the hippocampus of aged rats. Int. J. Neurosci. 123, 213–220. doi: 10.3109/00207454.2012.742895
Pallottini, V., Guantario, B., Martini, C., Totta, P., Filippi, I., Carraro, F., et al. (2008). Regulation of HMG-CoA reductase expression by hypoxia. J. Cell. Biochem. 104, 701–709. doi: 10.1002/jcb.21757
Qi, X. L., Zhu, B., Zhang, X. H., and Li, B. M. (2008). Are β-adrenergic receptors in the hippocampal CA1 region required for retrieval of contextual fear memory? Biochem. Biophys. Res. Commun. 368, 186–191. doi: 10.1016/j.bbrc.2008.01.007
Racine, A. M., Fong, T. G., Gou, Y., Travison, T. G., Tommet, D., Erickson, K., et al. (2018). Clinical outcomes in older surgical patients with mild cognitive impairment. Alzheimers Dement. 14, 590–600. doi: 10.1016/j.jalz.2017.10.010
Ross, C. A., and Truant, R. (2017). DNA repair: a unifying mechanism in neurodegeneration. Nature 541, 34–35. doi: 10.1038/nature21107
Sachdeva, M. M., Cano, M., and Handa, J. T. (2014). Nrf2 signaling is impaired in the aging RPE given an oxidative insult. Exp. Eye Res. 119, 111–114. doi: 10.1016/j.exer.2013.10.024
Samdal, H., Sandmoe, M. A., Olsen, L. C., Jarallah, E. A. H., Hoiem, T. S., Schonberg, S. A., et al. (2018). Basal level of autophagy and MAP1LC3B-II as potential biomarkers for DHA-induced cytotoxicity in colorectal cancer cells. FEBS J. 285, 2446–2467. doi: 10.1111/febs.14488
Schenning, K. J., Murchison, C. F., Mattek, N. C., Silbert, L. C., Kaye, J. A., and Quinn, J. F. (2016). Surgery is associated with ventricular enlargement as well as cognitive and functional decline. Alzheimers Dement. 12, 590–597. doi: 10.1016/j.jalz.2015.10.004
Seoane, J., Le, H. V., and Massagué, J. (2002). Myc suppression of the p21(Cip1) Cdk inhibitor influences the outcome of the p53 response to DNA damage. Nature 419, 729–734. doi: 10.1038/nature01119
Seshadri, S., Fitzpatrick, A. L., Ikram, M. A., DeStefano, A. L., Gudnason, V., Boada, M., et al. (2010). Genome-wide analysis of genetic loci associated with Alzheimer disease. JAMA 303, 1832–1840. doi: 10.1001/jama.2010.574
Sultana, R., Perluigi, M., and Butterfield, D. A. (2013). Lipid peroxidation triggers neurodegeneration: a redox proteomics view into the Alzheimer disease brain. Free Radic Biol. Med. 62, 157–169. doi: 10.1016/j.freeradbiomed.2012.09.027
Svendsen, J. M., Smogorzewska, A., Sowa, M. E., O’Connell, B. C., Gygi, S. P., Elledge, S. J., et al. (2009). Mammalian BTBD12/SLX4 assembles a Holliday junction resolvase and is required for DNA repair. Cell 138, 63–77. doi: 10.1016/j.cell.2009.06.030
Tang, L., Zeng, J., Geng, P., Fang, C., Wang, Y., Sun, M., et al. (2018). Global metabolic profiling identifies a pivotal role of proline and hydroxyproline metabolism in supporting hypoxic response in hepatocellular carcinoma. Clin. Cancer Res. 24, 474–485. doi: 10.1158/1078-0432.CCR-17-1707
Toivola, D. M., Habtezion, A., Misiorek, J. O., Zhang, L., Nystrom, J. H., Sharpe, O., et al. (2015). Absence of keratin 8 or 18 promotes antimitochondrial autoantibody formation in aging male mice. FASEB J. 29, 5081–5089. doi: 10.1096/fj.14-269795
Vila-Brau, A., De Sousa-Coelho, A. L., Mayordomo, C., Haro, D., and Marrero, P. F. (2011). Human HMGCS2 regulates mitochondrial fatty acid oxidation and FGF21 expression in HepG2 cell line. J. Biol. Chem. 286, 20423–20430. doi: 10.1074/jbc.m111.235044
Volianskis, A., and Jensen, M. S. (2003). Transient and sustained types of long-term potentiation in the CA1 area of the rat hippocampus. J. Physiol. 550, 459–492. doi: 10.1113/jphysiol.2003.044214
Vozella, V., Basit, A., Misto, A., and Piomelli, D. (2017). Age-dependent changes in nervonic acid-containing sphingolipids in mouse hippocampus. Biochim. Biophys. Acta Mol. Cell Biol. Lipids 1862, 1502–1511. doi: 10.1016/j.bbalip.2017.08.008
Wan, L., Han, J., Liu, T., Dong, S., Xie, F., Chen, H., et al. (2013). Scaffolding protein SPIDR/KIAA0146 connects the Bloom syndrome helicase with homologous recombination repair. Proc. Natl. Acad. Sci. U S A 110, 10646–10651. doi: 10.1073/pnas.1220921110
Wyss-Coray, T. (2016). Ageing, neurodegeneration and brain rejuvenation. Nature 539, 180–186. doi: 10.1038/nature20411
Xie, Z., McAuliffe, S., Swain, C. A., Ward, S. A., Crosby, C. A., Zheng, H., et al. (2013). Cerebrospinal fluid Aβ to tau ratio and postoperative cognitive change. Ann. Surg. 258, 364–369. doi: 10.1097/sla.0b013e318298b077
Xie, Z., and Xu, Z. (2013). General anesthetics and β-amyloid protein. Prog. Neuropsychopharmacol. Biol. Psychiatry 47, 140–146. doi: 10.1016/j.pnpbp.2012.08.002
Xu, Z., Dong, Y., Wang, H., Culley, D. J., Marcantonio, E. R., Crosby, G., et al. (2014). Age-dependent postoperative cognitive impairment and Alzheimer-related neuropathology in mice. Sci. Rep. 4:3766. doi: 10.1038/srep03766
Zhang, P., Kishimoto, Y., Grammatikakis, I., Gottimukkala, K., Cutler, R. G., Zhang, S., et al. (2019). Senolytic therapy alleviates Aβ-associated oligodendrocyte progenitor cell senescence and cognitive deficits in an Alzheimer’s disease model. Nat. Neurosci. 22, 719–728. doi: 10.1038/s41593-019-0372-9
Zhang, Y., Xu, Z., Wang, H., Dong, Y., Shi, H. N., Culley, D. J., et al. (2012). Anesthetics isoflurane and desflurane differently affect mitochondrial function, learning, and memory. Ann. Neurol. 71, 687–698. doi: 10.1002/ana.23536
Zhang, C., Zhang, Y., Shen, Y., Zhao, G., Xie, Z., and Dong, Y. (2017). Anesthesia/surgery induces cognitive impairment in female Alzheimer’s disease transgenic mice. J. Alzheimers Dis. 57, 505–518. doi: 10.3233/jad-161268
Zhao, X., Liu, X., and Su, L. (2014). Parthenolide induces apoptosis via TNFRSF10B and PMAIP1 pathways in human lung cancer cells. J. Exp. Clin. Cancer Res. 33:3. doi: 10.1186/1756-9966-33-3
Zhou, Y., Dhaher, R., Parent, M., Hu, Q. X., Hassel, B., Yee, S. P., et al. (2019). Selective deletion of glutamine synthetase in the mouse cerebral cortex induces glial dysfunction and vascular impairment that precede epilepsy and neurodegeneration. Neurochem. Int. 123, 22–33. doi: 10.1016/j.neuint.2018.07.009
Keywords: genome-wide screen, anesthesia, mitochondria, metabolism, aging
Citation: Wang Y, Qian M, Qu Y, Yang N, Mu B, Liu K, Yang J, Zhou Y, Ni C, Zhong J and Guo X (2020) Genome-Wide Screen of the Hippocampus in Aged Rats Identifies Mitochondria, Metabolism and Aging Processes Implicated in Sevoflurane Anesthesia. Front. Aging Neurosci. 12:122. doi: 10.3389/fnagi.2020.00122
Received: 17 December 2019; Accepted: 14 April 2020;
Published: 07 May 2020.
Edited by:
Zhongcong Xie, Massachusetts General Hospital and Harvard Medical School, United StatesReviewed by:
Jian-Jun Yang, Zhengzhou University, ChinaCopyright © 2020 Wang, Qian, Qu, Yang, Mu, Liu, Yang, Zhou, Ni, Zhong and Guo. This is an open-access article distributed under the terms of the Creative Commons Attribution License (CC BY). The use, distribution or reproduction in other forums is permitted, provided the original author(s) and the copyright owner(s) are credited and that the original publication in this journal is cited, in accordance with accepted academic practice. No use, distribution or reproduction is permitted which does not comply with these terms.
*Correspondence: Cheng Ni, bmljaGVuZ0BjaWNhbXMuYWMuY24=; Jing Zhong, anpob25nMTJAZnVkYW4uZWR1LmNu
† These authors share first authorship
Disclaimer: All claims expressed in this article are solely those of the authors and do not necessarily represent those of their affiliated organizations, or those of the publisher, the editors and the reviewers. Any product that may be evaluated in this article or claim that may be made by its manufacturer is not guaranteed or endorsed by the publisher.
Research integrity at Frontiers
Learn more about the work of our research integrity team to safeguard the quality of each article we publish.