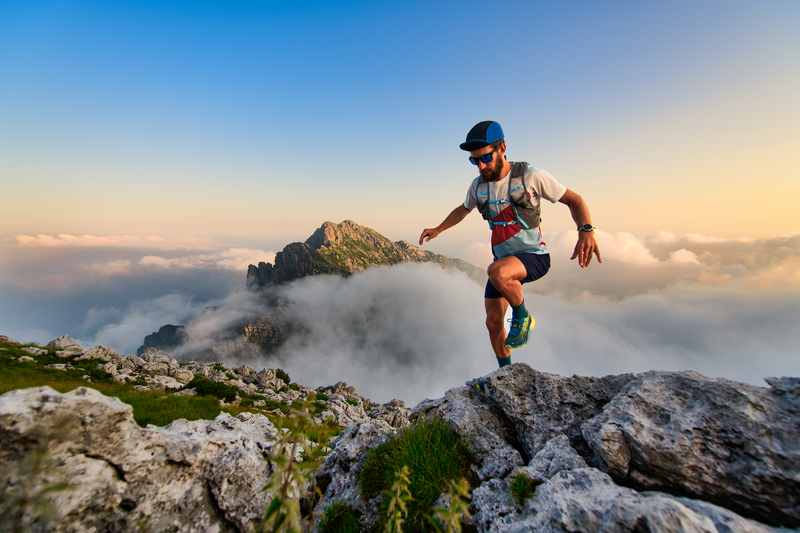
94% of researchers rate our articles as excellent or good
Learn more about the work of our research integrity team to safeguard the quality of each article we publish.
Find out more
REVIEW article
Front. Aging Neurosci. , 31 January 2020
Sec. Parkinson’s Disease and Aging-related Movement Disorders
Volume 12 - 2020 | https://doi.org/10.3389/fnagi.2020.00004
This article is part of the Research Topic Progress in Pharmacological Approaches and Drug Delivery Strategies Targeting Neurological Ailments View all 15 articles
Parkinson’s disease (PD) is the second-leading cause of dementia and is characterized by a progressive loss of dopaminergic neurons in the substantia nigra alongside the presence of intraneuronal α-synuclein-positive inclusions. Therapies to date have been directed to the restoration of the dopaminergic system, and the prevention of dopaminergic neuronal cell death in the midbrain. This review discusses the physiological mechanisms involved in PD as well as new and prospective therapies for the disease. The current data suggest that prevention or early treatment of PD may be the most effective therapeutic strategy. New advances in the understanding of the underlying mechanisms of PD predict the development of more personalized and integral therapies in the years to come. Thus, the development of more reliable biomarkers at asymptomatic stages of the disease, and the use of genetic profiling of patients will surely permit a more effective treatment of PD.
Due to the inability to find effective preventive or curative therapies (Fox et al., 2018), epidemiological predictions for the worldwide incidence of Parkinson’s disease (PD) are not optimistic (Lees et al., 2009; AlDakheel et al., 2014; Savica et al., 2016). For example, it is projected that by the year 2040, neurodegenerative diseases will surpass cancer as the leading cause of disease-related death (Gammon, 2014). Accordingly, there is an urgent need to identify effective treatments that transcend a reduction of symptoms.
PD leads to an array of symptoms and is characterized by a progressive loss of motor functions with bradykinesia, gate alterations, and posture instability (Gelb et al., 1999; Dexter and Jenner, 2013). Other symptoms include anxiety, depression, cognitive dysfunction, hallucinations, hypophonia, micrographia, and dysphagia as well as sialorrhea, dysphagia, hyposmia, impaired color vision, bladder hyperreflexia, and abnormalities of nociception and sleep (Voon et al., 2011; Hemmerle et al., 2012; Lindqvist et al., 2012; Schapira et al., 2017b). The pathological hallmark of the disease is the presence of Lewy bodies containing increased levels of α-synuclein, neurofilaments, and ubiquitin in neuronal and glial cells across an array of brain regions (Braak et al., 1998; Kotzbauer et al., 2001; Martin et al., 2012).
While PD was first described almost 200 years ago (Parkinson, 2002), it took nearly 150 years to determine that deficiencies of the dopamine (DA) system play a leading role in the pathology’s etiology (Lees et al., 2009). In the mid-20th century, Arvid Carlsson, who was later awarded the Nobel Prize in Physiology and Medicine for these discoveries in 2000, found that a decrease in DA levels in the brain led to PD-like symptoms (Carlsson et al., 1957). Then, Alexander et al. (1986) described parallel afferent pathways originating from the dorsal striatum. It has since been argued that the death of DA producing neurons leads to imbalanced communication from the dopaminergic midbrain system. Inasmuch, it is believed that motor dysfunction results from altered signaling of both direct and indirect pathways to the Globus pallidus internal (GPi)/Substantia nigra pars reticulata (SNpr) regions, which significantly disrupts thalamic connectivity with the motor cortex (Joyce, 2001; Gerfen and Surmeier, 2011; Calabresi et al., 2014). Concerning the cognitive and emotional deficits, it has been shown disruption of the output from dopaminergic neurons in the ventral tegmental area (VTA; Blonder and Slevin, 2011).
Despite these discoveries, the fundamental mechanisms inducing dopaminergic cell death are still unknown (Olanow and Tatton, 1999; Han et al., 2015, 2016). Among various pools of neurons that produce DA in the central nervous system, midbrain dopaminergic neuronal lesions alone can lead to PD-like symptoms in animal models. However, there appears to be some resilience associated with DA cell death, as humans must lose 48–68% of the dopaminergic neurons of the substantia nigra pars compacta (SNc) and about 70–80% of the DA content of the striatum to experience symptomatic PD (Bernheimer et al., 1973; Fearnley and Lees, 1991).
Given that PD genesis is multifactorial and depends mainly on the age of disease onset (Marsden, 1990; Cheng et al., 2010; Okun, 2017), the prognosis is highly variable, and personalized treatment regimens are theoretically possible. While early diagnosis of PD is difficult, some display an extended period (up to 5 years) of asymptomatic development (Fearnley and Lees, 1991). This latent period of disease progression opens up broad opportunities for therapeutic intervention. Accordingly, the early detection of degenerating dopaminergic neurons opens the possibility of halting PD progression at asymptomatic stages. In this review article, we review the pathological changes associated with the functional disorganization of the frontostriatal circuit in PD and overview current efforts to develop therapeutic approaches for treating PD.
The complexity of the dopaminergic system seems to coincide with evolutionary development given that the number, size, and distribution, as well as receptor subtypes of dopaminergic neurons in the brain, increases alongside phylogenetic complexity (Callier et al., 2003; Yamamoto and Vernier, 2011; Yamamoto et al., 2013). For example, dopaminergic terminal fields arising from midbrain clusters are more prominent and less segregated in the neocortex of primates than in rodents (Joel and Weiner, 2000; Björklund and Dunnett, 2007).
Dopaminergic neurons in the midbrain are mainly located in the SNc and VTA, although some smaller clusters have been found elsewhere, for instance, the dorsal and median raphe nuclei (Ochi and Shimizu, 1978). In a classic article by Dahlstroem and Fuxe (1964), SNc and VTA DA neurons were characterized based on their organization and projection patterns, which, in rat, can be found discrete clusters (A8, A9, and A10 see Figure 1). SNc neurons (cluster A9) innervate the dorsal and lateral striatum, thus forming a nigrostriatal pathway (Andén et al., 1964), and are necessary for the initiation and control of motor movements. Accordingly, the degeneration of this pathway is considered to be responsible for much of the motor dysfunction associated with PD. The VTA (A10) innervates the ventral striatum, nucleus accumbens, and limbic and cortical areas, and this way forms the mesolimbic and mesocortical pathways (Willner, 1991; Schott et al., 2008).
Figure 1. Diagram depicting the dopaminergic system of the midbrain. The dotted lines indicate the dopaminergic regions of the midbrain. GP, globus pallidus; SNc, substantia nigra pars compacta; VTA, ventral tegmental area; RRF, retrorubral field; A8, A9, and A10—clusters of dopaminergic neurons in the midbrain (RRF, SNc, and VTA clusters, respectively).
It has been documented that VTA neurons are involved in the regulation of motivation and reward as well as emotion-related behavior. Accordingly, degeneration of dopaminergic VTA neurons may underlie the development of depression and anhedonia in patients with PD (Thobois et al., 2010; Blonder and Slevin, 2011; Drui et al., 2014). Finally, the A8 cluster projects from the retrorubral field (RRF), and functionally appears related to the A10 cluster.
While the contributions from Dahlstroem and Fuxe (1964) were monumental, recent research calls this oversimplified view into question. As referred to above, organizational complexity is more significant in primates than in rats. Furthermore, the projection patterns from SNc and VTA neurons are not so refined, even within rodents. For examples, in addition to the targets listed above: (i) DA neurons of the SNc also innervate cortical and limbic regions; (ii) dopaminergic VTA neurons project to both the ventral striatum and the ventral-medial aspects of the head of the caudate-putamen; and (iii) the A8 cell group, which forms the dorsal and caudal expansion of the A9 cell group, contains cells that project into striatal, limbic, and cortical regions (Bentivoglio and Morelli, 2005; Björklund and Dunnett, 2007).
In general, midbrain DA neurons could be divided into two classes: the DA neurons located in the more dorsal tier of VTA and SNc and A8 cluster cells that are typically fusiform in appearance, with 2–5 dendrites emanating from the poles of each neuron (Björklund and Dunnett, 2007). These A8 cells are characteristically calbindin-positive and express relatively low levels of the DA transporter (DAT). In contrast, more ventrally located DA neurons are both multipolar in shape, are packed more densely, are calbindin-negative, and express higher levels of DAT. The ventral tier neurons extend (probably exclusively) to the striatum where they innervate, above all, the striosome compartment. Furthermore, many of these cells also possess prominent dendritic arborizations that spread within the zona reticulata (Björklund and Dunnett, 2007).
DA neurons of midbrain clusters have several unique morphological characteristics that may contribute to their specialized functions. For example, DA neurons appear capable of storing and releasing DA from their dendrites, permitting self-regulatory control of neurotransmitter release from nigral afferent fibers as well as to influence the activity of non-dopaminergic nigral cells (Cheramy et al., 1981). SN midbrain neurons typically have long unmyelinated axons and massive dendrites that branch into SN reticulata; the somas of these DA neurons make up much less than 1% of the total cell volume. A relatively small number of neurons provide the massive dopaminergic innervation of the striatum. It is estimated that each SN neuron can have as many as 150,000 presynaptic terminals in the striatum (Sulzer, 2007). In that regard, much is required for the normal functioning of a neuron with such a morphology. For example, each DA neuron must contain highly active axonal transport through microtubules to support metabolic and repair processes, synaptogenesis, removal of cell waste, and communication with other brain cells (Prots et al., 2013, 2018; Lu et al., 2014). In turn, each of these processes requires constant mitochondrial production of adenosine 5’-triphosphate (ATP) to assist the motor proteins dynein, kinesin, myosin, and actin (Course and Wang, 2016; Course et al., 2017; Vanhauwaert et al., 2019). Altogether, this makes the DA neurons in SN especially susceptible to mitochondrial dysfunction, and the resultant energy deficits could contribute mightily to DA-related impairments, such as those occurring in PD (Horowitz et al., 2011; Venkateshappa et al., 2012; Burbulla et al., 2017; Prots et al., 2018).
Mitochondria are complex cytosolic organelles of eukaryotic cells whose primary function is the generation of cellular energy in the form of ATP by oxidative phosphorylation. Mammalian mitochondria contain between 2 and 10 mitochondrial DNA (mtDNA) molecules encoding 22 transfer RNAs, two ribosomal RNAs, and 13 polypeptides, each of which is part of the respiratory chain and the oxidative phosphorylation system (Schapira, 1994). The mitochondrial respiratory chain contains four protein complexes that form the site of oxidative phosphorylation. This site is responsible for NADH and FADH2 oxidation, co-occurring with the movement of protons from the matrix into the intermembrane space. This movement produces an electrochemical gradient denoted as mitochondrial membrane potential (ΔΨm). This gradient stimulates the ATP synthase to reduce molecular oxygen and synthesize ATP. This step is fundamental in aerobic metabolism and constitutes the primary provider of ATP at the final stage of cellular respiration (Schapira, 1994; Videira and Castro-Caldas, 2018). Nevertheless, the biological function of mitochondria goes far beyond energy production and includes the metabolism of lipids and amino acids and the support of intermediate metabolic pathways, such as the Krebs cycle.
Mitochondria also play numerous regulatory actions outside of metabolism, which underscores the importance of maintaining optimal mitochondrial function. For example, mitochondrial cells regulate calcium homeostasis, remove free radicals, and control programmed neuron death (Keane et al., 2011; Franco-Iborra et al., 2016). In that regard, mitochondrial dysregulation or dysfunction can lead to an array of cellular and neuronal circuit perturbations. One common source of such disruptions is oxidative stress and, cyclically, neuroinflammation. Oxidative stress occurs as a result of high levels of unstable radicals, also called reactive oxygen and nitrogen species (RONS). These radicals can, rapidly and somewhat indiscriminately, alter proximal molecular structures through chained reduction-oxidation (redox) reactions (Betteridge, 2000). Proper mitochondrial-dependent cellular function is particularly threatened by oxidative stress due to multiple unique features of mitochondria: (i) electron leakage from the transfer chain can react with oxygen-generating RONS, thus perpetuating and amplifying proximal; (ii) mtDNA is particularly susceptible to damage due to its proximity to the electron transfer chain, and (iii) mitochondria lack effective mechanisms for mtDNA repair and protection.
Under physiological conditions, RONS production is neutralized by endogenous antioxidant factors such as Manganese superoxide dismutase and glutathione. However, many factors can disrupt this balance, such as diet, injury, illness, and age. For example, the “free radical theory of aging” (Harman, 1956), now commonly referred to as the “oxidative damage theory” (Gladyshev, 2014), posits that aging itself is the result of an oxidative stress-favoring imbalance of a tripartite relationship between RONS generation, antioxidant defenses, and repair from oxidative damage (Beckman and Ames, 1998). Indeed, many have shown that in aging, the vulnerability of mtDNA to damage increases due to reductions of antioxidant defense mechanisms (Chakrabarti et al., 2011; Kubben et al., 2016). It is noteworthy that this assertion has been under continued scrutiny (Beckman and Ames, 1998; Payne and Chinnery, 2015), likely due to multiple potential avenues of mtDNA dysfunction, for example, age-related mutation of mtDNA (Bandy and Davison, 1990; Arnheim and Cortopassi, 1992). That said, such alterations frequently coincide with increased levels of free radicals, which can perpetuate the aforementioned imbalance and result in elevations of oxidative stress. Almost a decade ago, a therapeutic approach for PD targeting the mitochondrial dysfunction was reported. This study consisted of a double-blind, placebo-controlled study to assess the effect of the antioxidant MitoQ in PD pathology progression, and it was the first clinical trial of a mitochondria-targeted antioxidant (Snow et al., 2010; Chaturvedi and Beal, 2013).
Increased RONS production and/or decreased neutralization can also cause neuronal death through lipid peroxidation and oxidation and nitration of proteins (Keane et al., 2011; Videira and Castro-Caldas, 2018). Together, these processes can then trigger apoptotic signaling leading to mitochondrial dysfunction. Indeed, RONS-initiated mitochondrial dysfunction accelerates the damage and death of dopaminergic neurons (Keane et al., 2011; Bose and Beal, 2016). Thus, energy and mitochondrial dysfunction is the earliest modifiable defect in the aging brain, and treatment with agents that improve mitochondrial function or enhance antioxidant activity may be beneficial in neurodegenerative diseases (Beal, 2005). A recent study investigating the role of telomerase in neuronal degeneration reported a new mechanism of mitochondrial dysfunction. Kim H. et al. (2017) used CRISP9/Cas9 technology to eliminate telomere repeats in the Neuroblastoma cells SH-SY5Y. Telomere removal resulted in mitochondrial dysfunction that adversely affected mitochondrial respiration and cell viability. Telomere removal also altered the levels of various PD-associated proteins, including PTEN-induced putative kinase 1, peroxisome proliferator-activated receptor gamma coactivator 1-alpha, nuclear respiratory factor 1, parkin, and aminoacyl tRNA synthetase complex interacting multifunctional protein 2. Finally, telomere removal enhanced α-synuclein protein aggregation, suggesting that this mechanism may be one of the links between aging and PD (Kim H. et al., 2017).
As reviewed above, it is generally accepted that dysfunction in PD stems from the degeneration of SNc neurons (i.e., nigrostriatal pathway), which leads to motor dysfunction and the loss of VTA neurons (i.e., mesolimbic and mesocortical pathways), which leads to behavioral dysregulation, including demotivation, anhedonia, and depression within PD (Thobois et al., 2010; Blonder and Slevin, 2011; Drui et al., 2014). While both pathways have been studied extensively across an array of conditions and pathologies, the modulatory mechanisms of the nigrostriatal pathway neurons have been fairly well described while the varied mechanisms and roles of VTA efferents continue to be elucidated. Within the nigrostriatal pathway, GABAergic medium spiny neurons (MSN) of the dorsal/lateral striatum receive excitatory glutamatergic signals that can be modulated via dopaminergic inputs originating from the SNc. MSNs are moderately sized cells with large, multi-structured dendritic arbors that constitute a staggering 95% of all postsynaptic nigrostriatal neurons (Kemp and Powell, 1971). Local circuit interneurons of the dorsal striatum are also actively involved in regulating MSN activity (Gittis and Kreitzer, 2012) and can be subdivided into cholinergic interneurons (1–2% of all striatal cells) and aspiny GABAergic interneurons known as low-threshold, fast-spiking neurons (Lim et al., 2014). Striatal cholinergic and MSNs express several neurotransmitter receptors including the γ-aminobutyric acid (GABA), glutamate, DA, adenosine, serotonin, opioids, and substance P (NK1) receptors (Lee et al., 1997; Tzaferis and McGinty, 2001; Solbrig et al., 2002; Lim et al., 2014).
Present on MSNs is multiple functional receptors capable of binding DA. All DA receptors are G-protein coupled and are generally classified into two subgroups according to structure, function, and pharmacokinetic properties (Watts and Neve, 1997; Gerfen and Surmeier, 2011). The first often termed “D1-like receptors,” are comprised of D1 and D5 receptors subtypes and, upon DA binding, drive adenylyl cyclase and thus cyclic adenosine monophosphate (cAMP) activity. On the other hand, “D2-like receptors,” which comprise D2, D3, and D4 receptor subtypes, suppress cAMP activity, thereby producing an inhibitory effect upon DA binding. While there is some evidence that D1- and D2-like receptors can colocalize in 4–6% of dorsal and 17–30% of ventral striatal MSNs (Matamales et al., 2009; Gangarossa et al., 2013; Perreault et al., 2015), there appears to be little, if any, functional competition between receptor subtypes within the same neuron (Biezonski et al., 2015; Frederick et al., 2015). This, therefore, yields two distinct MSN subtypes that exist in approximately equal quantities (Gerfen and Surmeier, 2011). These two types of MSNs can be further organized based on their differential projection patterns. D1-like containing MSNs monosynaptically innervate SNpr and are thusly termed the “direct” pathway while D2-like containing MSNs of the “indirect” pathway project to the GPi which, in turn, innervates various interface nuclei of the basal ganglia (Gerfen and Surmeier, 2011; Leisman et al., 2012, 2014; Leisman and Melillo, 2013; Rangel-Barajas et al., 2015). MSNs of the direct pathway also synthesize dynorphin and substance P as co-transmitters. MSNs of the indirect pathway co-transmit encephalin. While some have argued, particularly following electrophysiological studies, that the complexity of DA system physiology may be due to the coexpression patterns of D1- and D2-like receptors described above on direct and indirect pathway neurons, work using bacterial artificial chromosome (BAC) transgenic mice confirms that the “murkiness” of this system is instead a consequence of the complexity of striatal circuitry (Gerfen and Surmeier, 2011).
The influence of dopaminergic cells on the brain and behavior is impressive, considering that they account for less than 1% of the total number of neurons in the brain. This is achieved due to the numerous input signals by a small number of dopaminergic neurons in the midbrain within the striatum (Nagy et al., 2006; Reig and Silberberg, 2014). Indeed, MSNs integrate, and DA modulates signals from the cortex, thalamus, hippocampus, midbrain, brain stem, and various limbic structures (Plenz and Wickens, 2016).
While each integrated signal within the striatum plays its critical role, inputs from the frontal cortex are particularly crucial in goal-directed movements. For example, while motoric information from the premotor cortex (PrC) converges with situational reward information from the dopaminergic system in MSNs, all three of these systems are predominantly orchestrated via input from the prefrontal cortex (PFC; Figure 2; Deutch, 1993; Vogelsang and D’Esposito, 2018). With a panoply of roles in the top-down regulation of emotion, cognition, and goal-directed planning, various subregions of the PFC participate in the cognitive control and planning of movements. By projecting to the PrC, the PFC organizes, and the PrC then sequences voluntary bodily actions. The PrC then projects to the primary motor cortex, which is responsible for executing the associated movements. That having been said, movements initiated by this pathway are further refined by sub-second, situational updates that loop back to the PFC, PrC, and primary motor cortex via several subcortical pathways. Simultaneous glutamatergic projections from the PFC and PrC to the striatum are subjected to conditional modulation via SNc DA, which in turn projects via the direct and indirect pathways that loop back to the PrC and primary motor cortices by way of interface nuclei of the basal ganglia and, finally, the thalamus. These pathways are jointly referred to as the frontostriatal circuit or motor loops. The schematic diagram in Figure 2 describes the basal ganglia circuitry involved in voluntary motor control affected in PD (Ray and Strafella, 2010).
Figure 2. Diagram describing the frontostriatal motor loop controlling motor function under physiological and parkinsonian states. The prefrontal cortex (PFC) participates in cognitive control and planning of movements. The premotor cortex organizes sequences of body actions, and the primary motor cortex is responsible for executing them. Excitatory signals, which are initiated by cortical glutamatergic neurons, project from the PFC to the premotor cortex, and then to the motor cortex through several subcortical structures. Then, the resultant signals received by the pyramidal cells of the motor cortex go to the motor neurons of the spinal cord. Together, this is called the frontostriatal motor loop. Midbrain dopaminergic neurons play an essential role in modulating the signals that go along the frontostriatal motor loop. Changes in the direct inhibitory (initiated by D2 receptors) and indirect (D1 receptors) pathways under parkinsonian states due to the loss of dopaminergic neurons in the SNc are indicated. GABA, γ-aminobutyric acid; SNc, substantia nigra pars compacta; GPe, globus pallidus external; GPi, globus pallidus internal; STN, subthalamic nucleus; PPN, peripeduncular nucleus.
As alluded to above, the dorsal striatum controls and modulates signals passing from the PFC and PrC areas toward the motor cortex through the striatal-motor loop. The complexity of this process is underscored by the integration and signal modulation of more than a dozen neurotransmitter systems and their receptors (Figure 3). Accordingly, dopaminergic cell death in PD is associated with massive disruptions in the flow of information coming from the midbrain system and leading to an imbalance in the action of the direct and indirect pathways (Joyce, 2001; Alexander, 2004). However, the integrated use of these various other neurotransmitter systems provides an opportunity for targeted treatments in PD.
Figure 3. Diagram depicting the striatal neurotransmitter systems modulating the responses of the striatal neurons to the premotor cortex and SN afferent signals. The direct and indirect pathways, as well as stimulating and inhibitory neurotransmitter receptors, are outlined. MSNs, medium spiny neurons; GABA, γ-aminobutyric acid; SNc, substantia nigra pars compacta; ACh, acetylcholine; Enk, enkephalin; Dyn, dynorphin; GABA (PLTS and FS), aspiny GABAergic interneurons, low-threshold spiking (PLTS) and fast-spiking (FS) neurons.
Despite the fact that 200 years passed since the discovery of PD, it was not until later in the 20th century that progress in the treatment of PD was achieved, predominantly due to the limited understanding of PD pathophysiology. Given Carlsson’s discoveries of DA’s involvement in the 1950s, it became clear that PD development involved dopaminergic cell death and a decrease of DA in the striatum and other structures of the forebrain. The first steps towards treatment were made by Carlsson (2001), who proposed targeting this DA deficiency to facilitate symptom reduction.
Based on Carlsson’s discoveries, Hornykiewicz and colleagues developed the treatment of PD with the DA precursor, L-DOPA (Lees et al., 2015). This approach compensates for decreased DA by promoting DA synthesis in midbrain DA neurons. As evidenced in several pop-culture pieces, such as the award-winning motion picture Awakenings starring Robin Williams and Robert De Niro and based on the novel of the same name written by Oliver Sacks, the success of this approach in patients with PD was dramatic and often quite rapid (Birkmayer and Hornykiewicz, 1961). Despite these dramatic effects, it was reported that L-DOPA’s effects were often inconsistent, even within the same patients, and often eventually induced profound and intolerable side effects such as dyskinesia, motor fluctuations, and various emotional disturbances and psychiatric problems (Allan, 2007; Voon and Fox, 2007; Fox and Lang, 2008; Salat and Tolosa, 2013). Furthermore, all the clinical benefits of the treatment are eventually reverted with a continuation of dopaminergic neuronal death, as L-DOPA administration does not halt disease progression (Castrioto et al., 2013). However, despite these limitations, the improvement seen in some patients is so pronounced that these downsides do not prevent its use. Indeed, after almost 60 years, L-DOPA remains the gold-standard medication for PD (Tan, 2001; Salat and Tolosa, 2013).
In the late 1990s, the theory of the dual organization of the striatum and its outputs to other parts of the basal ganglia (Albin et al., 1989; DeLong, 1990; Figure 3) led to a renaissance of neurosurgical procedures for the treatment of PD. Based on this theory, a new surgical treatment to reduce the symptoms of PD called deep brain stimulation (DBS) was established (Lozano and Snyder, 2008; Lozano et al., 2010). Despite its highly invasive nature, DBS led to significant improvements in the quality of life of patients with advanced PD and consists of the direct electrostimulation of the subthalamic nucleus (STN) or the GPi that are hyperactivated due to the decrease in midbrain dopaminergic neurons.
In general, among PD patients, before surgery, women with PD use lower doses of dopaminergic medication and experienced more dyskinesias, mobility limitations, and sensory symptoms than men (Hariz et al., 2013). Nonetheless, after DBS, both sexes show a similar functional improvement (Hariz et al., 2013). Although it is effective at motor symptom alleviation, DBS can also induce adverse side effects such as an aggravation of freezing of gait and worsening of verbal fluency (Carlson et al., 2014; Foley et al., 2017; Højlund et al., 2017). Yet another downside of DBS is that PD-induced speech disruption is much less responsive to DBS than any other motor dysfunctions (Limousin and Martinez-Torres, 2008; Moro et al., 2010). The mechanism underlying the beneficial effect of DBS is not completely understood; however, the actual evidence suggests that vascular changes may be involved in its therapeutic effects (Pienaar et al., 2015). The overexpression of the vascular endothelial growth factor (VEGF) and the downregulation of neuroinflammatory factors are considered to be key molecular mechanisms involved in DBS-induced microvascular changes (Pienaar et al., 2015; Sharma et al., 2016; Lozano et al., 2018).
L-DOPA and DBS treatments are frequently applied together to potentiate their beneficial effects (Pienaar et al., 2015; Sharma et al., 2016; Lozano et al., 2018). This said, although these treatments can efficaciously reduce PD symptomology, they are ineffective at halting PD progression. Still, L-DOPA and DBS have been widely used for some decades now, thus improving the lives of patients with PD where few other options are available (Timpka et al., 2016).
Another promising therapeutic strategy for PD is cell replacement therapy to restore dopaminergic neurons (Kupsch et al., 1995; Stoker et al., 2017). This strategy, supported by reports in the late 1970s and early 1980s, showed that dopaminergic neurons derived from the developing embryonic midbrain were able to survive when implanted in adult brains and, moreover, that the transplanted neurons formed axons that extended through surrounding scar tissue and thus restored innervation in the host brain (Björklund et al., 1976; Stenevi et al., 1976). More recent investigations in animal models have focused on the survival and differentiation of implanted cells. This includes fetal dopaminergic neurons (Thompson and Parish, 2013) as well as embryonic, adult, neural, mesenchymal, and induced pluripotent stem cells (Freed et al., 2003; Hedlund and Perlmann, 2009; Thompson and Parish, 2013; Barker et al., 2016; Xu et al., 2016; Venkatesh and Sen, 2017; Zhang et al., 2017). These efforts were accompanied by the development of biomaterial scaffolds to provide support materials for cell adhesion and growth (Moriarty et al., 2019).
The first clinical trials were conducted in the late 1980s to investigate the transplantation of embryonic mesencephalic tissues into the striatum but resulted in only minimal clinical improvements (Drucker-Colín et al., 1988; Iriarte et al., 1988; Madrazo et al., 1988a,b). However, after refining the technique, subsequent clinical studies showed significant clinical improvements following implantation of fetal DA neurons into the brains of young PD patients (Freed et al., 2001). Unfortunately, this approach resulted in high morbidity and mortality rate in elderly patients (Madrazo et al., 1988b; Goetz et al., 1989; Brundin et al., 2010). However, postmortem studies showed a sustained survival of the transplanted cells (Barrow, 2015; Björklund and Lindvall, 2017). These studies revealed that cell replacement is a promising approach for the treatment of PD, but with many problems that remain unresolved. A significant issue has been the absence of significant and consistent therapeutic effects in patients with PD. This failure results in a 10-year moratorium for this kind of surgery, which was imposed in 2003 (Han et al., 2015). Also, other ethical and logistical problems exist, including the absence of sufficient source material for tissue transplantation for a large number of patients (Barker et al., 2016), inconvenient side effects such as graft-induced dyskinesia (Lindvall and Björklund, 2004; Lindvall, 2015, 2016), and the enhanced risk of tumor formation (Wolff et al., 2015). Nonetheless, the biology of stem cells has advanced significantly over the past decade, as has the obtention of dopaminergic neurons from neural and induced pluripotent stem cells (Björklund and Lindvall, 2017). These advances aid in resolving problems of availability as well as the rejection of transplanted cells while circumventing ethical issues associated with obtaining stem cells from embryos.
While promising, another problem with this approach resides in the complicated architecture of the midbrain dopaminergic system. As mentioned above, there are two prominent populations of dopaminergic neurons in the midbrain (i.e., SNc vs. VTA). While the loss of dopaminergic neurons in the SNc is primarily associated with motor impairment, the loss in the VTA may induce psychological and emotional disturbances (Thobois et al., 2010; Blonder and Slevin, 2011; Drui et al., 2014). In most clinical studies, stem cells were grafted directly in the striatum or the lateral ventricle. Importantly, dopaminergic neurons have complex relationships with glial and other neural cells that support their survival and activity including the formation of tripartite synapses with astrocytes in the midbrain (Hennigan et al., 2015; Xin et al., 2019). An ongoing clinical trial (NCT01898390) named The Transeuro Transplant study consists in grafting fetal tissue into the brain of patients with PD. The last update informed that 11 PD patients were subjected to cell transplantation in the UK and Sweden. The study will finish the clinical evaluation of all these patients in 2021.
These studies raise important questions in need of addressing: (i) can grafted stem cells retain their functions outside SNc and VTA; and (ii) can the new cells correctly replace the damaged ones by integrating into the existing information networks of the brain? New answers for these questions continue to appear. A more detailed description of more than 30 years of neuronal transplantation studies in PD, can be found in excellent reviews (Björklund and Lindvall, 2000; Lindvall and Björklund, 2004; Lindvall, 2015; Lindstrom, 1997; Stoker et al., 2017). Despite the challenges associated with this therapeutic approach, the considerable progress in the field predicts essential advances in the years to come.
Unfortunately, although some therapies for PD produce a period of recovery for about 5 years, there is a sharp decrease in the beneficial effects of treatments thereafter (Castrioto et al., 2013). Indeed, the best approach would be to understand the relevant triggers of the disease in order to target the physiopathological mechanisms causing the death of dopaminergic neurons. Epidemiological studies have shown that less than 10% of PD cases have a strict familial etiology, while most of them are sporadic and appear to be caused by other factors associated with susceptibility genes (Thomas and Beal, 2007; Videira and Castro-Caldas, 2018). Although these factors are not fully understood, there is a consensus that PD is induced by a combination of age, gender, genetic background, and environmental factors. However, neither of these has, alone, been identified as a leading cause of PD (Allam et al., 2005; Thomas and Beal, 2007; Wirdefeldt et al., 2011). While the cellular and neurochemical mechanisms underlying PD have remained incompletely understood, what data have been collected point to heavily to mitochondrial dysfunction, oxidative stress, inflammation, and excitotoxicity in the pathogenesis of both familial and sporadic cases of PD (Ouchi et al., 2009).
Despite low heritability rates, as discussed above, some rare familial forms of PD give important clues regarding the molecular mechanisms of the sporadic form of PD pathology. Currently, 28 chromosomal regions have been linked to PD; from these, six contain genes in which a single mutation causes monogenic forms of PD (3–5% of all cases; Klein and Westenberger, 2012; Videira and Castro-Caldas, 2018). These mutations affect genes responsible for autosomal dominant forms of PD including genes such as α-synuclein, Park 1/4 (SNCA), Park 8 (the Leucine-Rich Repeat Kinase 2, LRRK2), and genes exhibiting an autosomal recessive mode of inheritance such as Parkin, PINK1, DJ-1, and ATP13A2. The remaining cases of PD seem to be the result of complex gene-environmental interactions influencing the development of the disease (Klein and Westenberger, 2012).
The α-synuclein gene encodes a small protein present in nerve terminals. The physiological function and role of α-synuclein in the etiology of PD are still unclear. However, evidence suggests that this protein plays a significant role in the pathogenesis of the disease (Schapira and Jenner, 2011). Because of its structure, α-synuclein can interact with anionic lipids, which results in conformational changes favoring aggregation into toxic complexes. Also, these aggregate-prone forms of α-synuclein can interfere with lysosomal and mitochondrial functions, autophagy, vesicular homeostasis, and microtubule transport (Keane et al., 2011; Rocha et al., 2018). For example, the accumulation of mutant forms of α-synuclein in the inner mitochondrial membrane impairs the complex I, increasing RONS production and promoting neuronal death (Devi et al., 2008).
Interestingly, all familial forms of PD are associated with mutations in genes that directly or indirectly cause mitochondrial dysfunction. Often these mutations have multiple pathological effects on mitochondria. For example, mutations in the genes SNCA, Parkin, DJ-1 inhibit the activity of the complexes I, II, and III and affect mechanisms regulating the morphology and dynamics of mitochondria (Beal, 2005; Moon and Paek, 2015; Ryan et al., 2015; Bose and Beal, 2016). These disturbances in the mitochondria or direct inhibition of its complexes in both sporadic and familial PD affects mitochondrial integrity and induce negative bioenergetic effects, such as a dysregulation of glucose metabolism, impaired pentose phosphate pathway, and a decrease in ATP production (Dunn et al., 2014). The complex I of damaged mitochondria vigorously produces RONS, and when the production exceeds the cell antioxidant capacity, the oxidant species damage mitochondrial proteins, lipids, and mtDNA. Damage to mtDNA leads to mutations that inhibit the respiratory chain and reduce the mitochondrial membrane potential (Ryan et al., 2016). Ultimately, these self-reinforcing processes lead to proliferative cell death.
This evidence suggests that preventing mitochondrial dysfunction can be a key therapeutic goal to achieve as stand-alone or adjunctive therapy against PD.
The explosive development of new genetic editing technologies, although still under investigation for clinical use, open the possibility to correct mutated genes and regulatory DNA in the monogenic forms of PD (Nadim et al., 2017; Singh and Sen, 2017; Deverman et al., 2018; Kabra et al., 2018; Lu et al., 2018). With these ideas in mind, several methods of gene delivery, including viral vectors and CRISPR, have been developed (Lino et al., 2018). Outstandingly, new reports for clinical trials have shown success in CRISPR technology use against diseases induced by single mutations such as β-thalassemia and sickle cell anemia. However, because Cas9 induces a double-strand DNA break, potential detrimental side effects of CRISPR-Cas9 technology are possible, such as non-specific CRISPR-induced mutation due to deletions in non-intended regions of the genome (Ihry et al., 2018). A description of PD linkage studies using CRISPR technology as well as the process of genome editing in PD patients’ inducible progenitor stem cells (iPSCs) has been reviewed and reported (Safari et al., 2019).
Active research efforts are currently underway to overcome these limitations. Recently one of the latest advances in CRISP technology was reported by Rees et al. (2019) from Harvard University (Anzalone et al., 2019). In this new approach, Cas9 hybridizes to the target DNA site using a guide engineered RNA containing a complementary spacer. To transfer the latest information from these guide RNAs, the genomic DNA is nicked at only one location (Anzalone et al., 2019). This method reduces the risk of undesired DNA mutations, and it may very well revolutionize the therapy of PD and other pathologies linked to single-gene mutations.
Another promising approach lies in gene therapy using non-replicating viral vectors such as gene delivery forms of adeno-associated virus (AAVs), retro and lentiviruses (Lundberg et al., 2008), and glycoprotein-deleted rabies virus (Chen et al., 2019; Wang and Huang, 2019; Wang F. et al., 2019; Wang X. et al., 2019; Wang Y. et al., 2019). Gene delivery using AAVs has the advantage in that these viruses do not integrate into host chromosomes yet persist as episomic chromosomes that do not provoke insertional mutations and permit stable gene expression in neuronal and glial cells (Penaud-Budloo et al., 2008). Furthermore, AAVs do not induce immunoreactions in humans and, as a result, are regarded as one of the best viral gene delivery systems for use in preclinical biomedical research and clinical trials (Naso et al., 2017). The main limitation of AAVs is that they can only deliver up to 5.2 kb of genetic material (Wu et al., 2010). Lentiviruses, however, can deliver genetic sequences of up to 9 kb to dividing and non-dividing cells. After transduction, the lentiviral RNA is reverse transcribed to DNA and randomly integrated into the host chromosomes (Rodríguez et al., 2019). This disadvantage limits its clinical application though lentiviruses are frequently used in preclinical research (Maes et al., 2019).
The recent approval of human AAV vector use in Europe and the USA has led to an array of gene therapy attempts in various clinical trials (Piguet et al., 2017; Axelsen and Woldbye, 2018; Hitti et al., 2019). The genetic approaches taken for PD treatment are largely neuro-regenerative in nature, and they are directed to halt neuronal cell death. For example, some strategies include inducing the overexpression of neurotrophic factors in the substantia nigra or the increasing repair genes to disrupt the formation and accumulation of aggregated and neurotoxic forms of neuronal proteins such as a-synuclein. More than a decade ago, a pioneering phase 1 study assessing the safety of human aromatic L-amino acid decarboxylase (hAADC) gene therapy for PD tested the effect of bilateral AAV2-induced AADC expression in the putamen of subjects with advanced PD (Eberling et al., 2008). Although the authors reported no adverse effects of AAV-mediated AADC overexpression in humans, they found no significant clinical recovery as tested using the Unified PD Rating Scale (UPDRS; Eberling et al., 2008). Follow-up clinical studies reported positive effects, such as reduction of symptoms and improvement of UPDRS scores, as well as lowered L-DOPA dosage required for treatment. Other recent studies include the Phase 1 trial and current Phase II trial for Voyager’s AAV2-hAADC transplantation, a year-long clinical trial in PD patients that also investigates changes in overnight time free of dyskinesia (McFarthing et al., 2019). Furthermore, gene therapy has been used with the intent to prevent mitochondrial dysfunction in the brain of patients with PD, by increasing the expression of synaptic proteins, neurotrophic factors (NTFs), antioxidants, and anti-inflammatory proteins.
The overexpression of neurotrophic factors (NTF) is a powerful strategy to prevent the neurodegeneration of dopaminergic neurons in PD brains. The delivery of these factors, including the neurotrophic factor (NF), glial cell line-derived neurotrophic factor (GDNF), neurturin (NRTN), cerebral dopamine neurotrophic factor (CDNF) and growth/differentiation factor 5 (GDF5) is a challenging task. An alternative approach is the use of recombinant viral vectors to enable long-term expression of these factors in brain cells without the risk of hemorrhages induced by the catheter placement into the brain. From them, genetic therapy directed to increase the expression of GDNF and NRTN alone or combined with other NFs have shown promising results.
The study of GDNF both in vitro and in vivo using rodent (Rosenblad et al., 1998; Sullivan et al., 1998; Georgievska et al., 2002) and monkey models of PD (Gash et al., 1996; Miyoshi et al., 1997; Palfi et al., 2002; Eberling et al., 2009; Su et al., 2009; Redmond et al., 2013), revealed potential therapeutic effects that encouraged its clinical investigation. These benefits included behavioral improvements and protective effect on the dopaminergic nigrostriatal neurons (Eslamboli et al., 2005; Sun et al., 2005; Sajadi et al., 2006; Eberling et al., 2009) However, GDNF therapy was not initially successful due predominantly to the low efficiency of delivery methods (Lang et al., 2006). Accordingly, subsequent efforts were refocused on improving delivery and expression methods, including; infusion, cannula design, and insertion zones to optimize the delivery of AAV vectors expressing GDNF to the brain (Lang et al., 2006; Richardson et al., 2011).
Other study tested AAV2-delivered NRTN, under the name CERE-120 (Ceregene Incorporated; Marks et al., 2010). While animal models of PD and an open-label, phase I clinical trial suggested tolerability and a favorable safety profile as well as reductions of UPDRS scores and dyskinesias, a follow-up phase II trial with bilateral intraputaminal injections resulted in nearly a third of CERE-120-treated patients reporting serious adverse events, including surgery-related complaints in many and tumor formation in three of the CERE-120-treated patients, and in two control subjects. Furthermore, treatment with CERE-120 for a year did not improve the UPDRS scores of the PD patients when compared to the control sham-operated group. However, CERE-120-treated patients did show significant improvements in off-medication UPDRS scores when assessed after 18 months, suggesting a delayed neurotrophic effect (Marks et al., 2010).
Another open-label trial assessed the safety and efficacy of targeting both the SNpc and striatum. Patients received nigral and putaminal doses of CERE-120 and were monitored for 2 years. This trial reported no treatment-induced adverse medical events during the entire study (Bartus et al., 2013). In the follow-up, placebo-controlled, double-blind phase 2b study, the data did not show significant improvements in motor-off scores. However, significant motor-off improvements were observed in patients that had been diagnosed less than 5 years before treatments when compared with those who were diagnosed more than 10 years before gene therapy interventions began. This indicates that gene therapy can be useful as a preventative approach in PD (Bartus et al., 2014).
A more recent study reported the results of a 6-month double-blind, randomized trial assessing the clinical effects of bilateral brain delivery of the glutamic acid decarboxylase (GAD) gene into the subthalamic nuclei (STN) of advanced PD patients (LeWitt et al., 2011). The results showed significant improvements in the UPDRS scores in the AAV2-GAD group compared with the sham group at 6 and 12 months. Also, the levodopa-induced dyskinesia significantly diminished in duration in the AAV2-GAD group, but not in the control group that remained constant. On the other hand, functional network connectivity analysis showed an increase in the metabolism of the network after a year from baseline, as investigated by PET imaging. Specifically, enhanced metabolic activity was observed in the premotor cortex, motor cortex, and supramarginal gyrus. Reduced metabolic activity is observed in the putamen, caudate, globus pallidus, inferior frontal gyrus, medial dorsal thalamus, and ventral anterior thalamus. The beneficial effects persisted when investigated 12 months after interventions (Niethammer et al., 2017).
Recently, another study reported positive effects using a lentiviral vector named AXO-Lenti-PD, which encodes three enzymes essential for DA synthesis (aromatic L-amino acid decarboxylase; cyclohydrolase 1; and tyrosine hydroxylase). Three months into the ongoing phase II trial of AXO-Lenti-PD, initial reports show a 25-point reduction in UPDRS scores. The study (NCT03720418) consists of two parts. In the first part, two patients with advanced PD who received a one-time administration of the lowest dose of AXO-Lenti-PD, which was reportedly well-tolerated and safe. A new cohort with up to six patients will receive three times the initial dose. The Initial data from this cohort is expected by the end of 2019.
Additional gene therapy approaches can be directed to knockdown the expression of PD-related genes using small interfering RNA (siRNA) or microRNA (miRNA). Various groups have focused on suppressing α-synuclein expression in animal models using RNAi (Zharikov et al., 2015; Kim Y.-C. et al., 2017. These groups have shown that reducing α-synuclein expression leads to reductions in dopaminergic neuron loss alongside fewer motor deficits. Thought, it is crucial to consider that blocking the expression of α-synuclein may interfere with the normal function of this protein (Surguchev and Surguchov, 2017; Burré et al., 2018; Sorrentino et al., 2019; Taguchi et al., 2019).
Green tea is prepared from the leaves of the Camellia Sinensis plant and contains phenolic compounds such as (-)-Epigallocatechin-3-gallate a potent antioxidant and neuroprotective compound. Preclinical clinical and self-report studies suggest that green tea may prevent PD (Kandinov et al., 2009; Bitu Pinto et al., 2015). However, the therapeutic mechanism of green tea’s potential protective actions in PD is unclear. It is feasible that green tea’s phenolic compounds are modulating critical neuroprotective signaling pathways in the brain (Jurado-Coronel et al., 2016b). On the other hand, green tea could exert its effects via caffeine-induced inactivation of the adenosine receptor.
Almost two decades ago, a clinical study investigating the relationship between coffee consumption and the risk of developing PD found that coffee intake negatively correlated with PD in a dose-dependent manner (Ross et al., 2000). PD incidence declined from 10.4 per 10,000 person-years in male subjects who consume no coffee to 1.9 per 10,000 people/year in men who drink at least 28 oz)approximately three cups) of coffee a day. The authors concluded that higher coffee and caffeine intake are associated with a significantly lower risk of developing PD (Ross et al., 2000). Numerous studies have since confirmed these findings and point to adenosine A2A receptor antagonists as a putative treatment for PD (Schwarzschild et al., 2002; Kalda et al., 2006; Postuma et al., 2012, 2017). However, a recent study concluded that in PD patients, consumption of 200 mg coffee a day for 6 months did not improve the motor symptoms. Still, other clinical studies using the A2A receptor antagonist, istradefylline, are encouraging given that istradefylline significantly improved motor manifestations of PD and reduced nighttime urinary frequency (Jankovic, 2008; Matsuura and Tomimoto, 2015; Kitta et al., 2018).
The cholinergic system plays a broad role in controlling neurotransmitter release, reducing neuroinflammation, and promoting neuronal survival and synaptic plasticity in the brain. The binding of acetylcholine (ACh) to nicotinic ACh receptors (nAChRs) occurs throughout the brain, including within striatum and other constituents of the mesolimbic, mesocortical, nigrostriatal, and frontostriatal loops. nAChRs are pentameric ligand-gated ion channels composed of α-subunits (α2-α7) or containing α and β-subunits (β2–β4; Quik et al., 2007). Presynaptic nAChRs mediate neurotransmitter release and postsynaptic receptors increase neuronal firing rates and thus facilitate long-term potentiation.
As discussed above, the striatum contains large aspiny cholinergic interneurons (ChIs) that interact with DA inputs. While ChIs is the primary source for ACh in the striatum, cholinergic projections also arrive from the pedunculopontine nucleus (PPN) and the laterodorsal tegmental nuclei (Tanimura et al., 2018). DA depletion in the striatum causes increased the excitability of ChIs as a consequence of the loss of the inhibitory dopaminergic modulation via presynaptic D2-like receptors on ChIs. Deficits in ChI function is involved in various basal ganglia-related movement disorders such as dystonia, PD, and Tourette’s syndrome (Pisani et al., 2007; Deffains and Bergman, 2015; Tanimura et al., 2018). Striatal ChIs appear to support synaptic plasticity and cognitive functions, mediated by the dorsal striatum such as attention, and motivation (Bohnen and Albin, 2011; Deffains and Bergman, 2015; Aarsland, 2016; Aarsland et al., 2017; Schapira et al., 2017a). ChIs and DA work together to regulate motor function and represent good targets to alleviate PD symptoms (Ztaou and Amalric, 2019). In the striatum, ChIs express the muscarinic acetylcholine receptors (mAChRs; M1/M5) as well as various subtypes of nAChRs, composed mainly of α4, α6, α7, β2, and β3 subunits, with the primary expression of the α4β2 and α6β2 receptors (Quik and Wonnacott, 2011). Striatal nAChRs are expressed in dopaminergic and glutamatergic neurons, as well as ChIs and GABAergic interneurons (English et al., 2011; Nelson et al., 2014). On the other hand, nAChRs are absent from MSNs (Quik et al., 2007).
Several studies have investigated changes in the expression of muscarinic and nAChRs in PD (James and Nordberg, 1995; Lindstrom, 1997; Quik and Jeyarasasingam, 2000; Forgacs and Bodis-Wollner, 2004; Picciotto and Zoli, 2008; Shimohama, 2009; Kawamata et al., 2012; Jurado-Coronel et al., 2016a; Zhao et al., 2016). Various studies showed that α4β2 and α7nAChRs were reduced in the cortical and subcortical regions of the brain, including the frontal and temporal cortices, hippocampus, caudate nucleus, and the pons of patients with PD when compared to healthy controls (Lange et al., 1993; Perry et al., 1995; Banerjee et al., 2000). It has also been reported an inverse correlation between the level of dementia and nAChRs expression in the hippocampus and temporal cortex of the PD patients with a loss or down-regulation of these receptors preceding the loss of dopaminergic neurons (Meyer et al., 2009). A significant decrease in nicotine binding (65–75%) has also been reported in the SNc of the midbrain, as well as a significant pathological change of cholinergic neurons of the pedunculopontine region (Perry et al., 1995). Other studies showed severe losses in α6β2 receptor expression and a minor decline in the α4β2 subtypes in PD brains. The decrease in α6β2, but not α7 receptors, paralleled a reduction in markers of nigrostriatal degeneration (Bohr et al., 2005). Within the putamen, there was no change in the expression of the α2-α7, β2, and β3 nicotinic subunits and the authors suggested that the observed binding deficits may be the result of a change in the assembly of the receptors’ subunits likely induced by α-synuclein instead of a change in the expression of the nicotinic subunits in the striatum (Martin-Ruiz et al., 2002). Brain imaging studies using positron emission tomography, and the α4β2 receptor-specific radioligand 2-18F-FA-85380 or (123I)5IA and single-photon emission computed tomography revealed a decrease in the number of nAChRs in the amygdala of patients with PD (Quirion, 1993; Pimlott et al., 2004; Fujita et al., 2006; Schmaljohann et al., 2006; Oishi et al., 2007; Meyer et al., 2009, 2014). This is similarly true in frontal and parietal cortices, the striatum, and substantia nigra in the PD brain (Kas et al., 2009). Nevertheless, a compensatory increase in the expression of the nAChRs has been identified during the early stages of the pathology (Isaias et al., 2014).
Epidemiological studies have shown lower rates of PD development in people consuming tobacco products, which suggests that the nicotinic receptors may play an essential role in preventing PD and that one or more tobacco-derived compounds may be neuroprotective (Fratiglioni and Wang, 2000; Parain et al., 2003; Hong et al., 2009). Various studies using cellular models have shown a neuroprotective effect of nicotine that diminished dopaminergic neuronal damage (Riveles et al., 2008; Toulorge et al., 2011; Getachew et al., 2019). Other reports have shown that both nicotine and its main derivative, cotinine, have a neuroprotective effect against 6-hydroxydopamine (6-OHDA)-induced toxicity in cultured differentiated SH-SY5Y neuroblastoma cells expressing nAChRs (Pogocki et al., 2007; Riveles et al., 2008).
Within in vivo animal models of PD, nicotine also appears to produce beneficial effects (Linert et al., 1999; Salminen et al., 1999; Quik and Kulak, 2002; Quik et al., 2006; Huang et al., 2009). Studies using non-human primates show that nicotine reduced dyskinesia in PD (Bordia et al., 2008), but not all studies have found a positive effect of transdermal nicotine on the cognitive or motor symptoms of PD (Lemay et al., 2004). It is not clear whether nicotine effects are the result of the activation or desensitization of the nAChRs. Studies using antagonists, it has been discovered that both α7 and α4/β2 nAChRs contribute to the neuroprotective properties of nicotine, although the effects of the cholinergic modulators will vary according to the type of brain cells and the different combinations of nicotinic subunits that may predominate in specific brain regions. In addition to nicotine, the neuroprotective effects of other nAChR modulators have been investigated and yield promising results (Pogocki et al., 2007; Tiwari et al., 2015; Jurado-Coronel et al., 2019). Derivatives clinically tested include Isoproniclina (TC1734), (S)-N-metil-5-(5-pirimidinil)-4-penten-2-amina (TC1827), and RJR-2403 (transmetanicotina), which were shown to stimulate DA release and improve working memory in PD patients (Pogocki et al., 2007). Additionally, SIB-1508Y, an α4β2 agonist, has been investigated in various clinical trials for PD (Pogocki et al., 2007). The results obtained collectively support the view that nAChRs modulators are neuroprotective against the neurotoxic insults in the PD brain.
Research from our and other labs has shown that nicotine’s predominant metabolite, cotinine has unique pharmacokinetic and pharmacodynamic properties, acts as a weak agonist at nAChRs, and a positive allosteric modulator of α7nAChRs, and is safe and non-addictive (Grizzell and Echeverria, 2015). Cotinine protects astrocyte from the toxic effects of glucocorticoids and increases synaptogenesis in the PFC and hippocampus during stress in mice (Grizzell et al., 2014a,b; Alvarez-Ricartes et al., 2018). Cotinine also diminished the activation of macrophagic immune cells (Rehani et al., 2008) and showed neuroprotective effects in mice models of AD, reducing plaque deposition, tau phosphorylation, and cognitive impairments (Buccafusco and Terry, 2003; Buccafusco et al., 2007; Szymańska et al., 2007; Echeverria et al., 2011; Echeverria and Zeitlin, 2012; Gao et al., 2012, 2014; Moran, 2012; Patel et al., 2014; Li et al., 2015; Terry et al., 2015; Echeverria et al., 2017; Grizzell et al., 2017). Also, cotinine seems to control the number and stoichiometry of the nAChRs affecting their properties (Lester et al., 2009).
Despite their chemical similarities, cotinine and nicotine differ in their mechanisms of action, behavioral effects, and show distinct properties and toxicity profiles. Cotinine is a hundred times less toxic than nicotine and binds poorly α7nAChR in the orthostatic site (Grizzell and Echeverria, 2015). Based in its neuroprotective effects, and its ability to increase DA levels and positively modulate the α7nAChRs in the brain, we have postulated that cotinine might also delay the development of PD (Soto-Otero et al., 2002; O’Leary et al., 2008; Riveles et al., 2008; Barreto et al., 2014).
The failure of current therapies for PD may be due to the heterogeneity of syndromes collectively referred to as PD. While all converge in the massive loss of DA neurons in the midbrain alongside the appearance of Lewy bodies, different etiologies of PD have been found. Some of these etiologies specifically affect the DA neurons, while others may overlap with comorbid conditions such as AD and other synucleinopathies. Based on this idea, the “multiple hit” hypothesis was proposed, in which the basis for selective neuronal death is a combination of toxic stress, induced by DA oxidation or mitochondrial dysfunction, co-occurring with inhibition of neuroprotective responses, such as follows after the loss of parkin function (Sulzer, 2007).
This said, the bright side of PDs multifactorial etiology provides an opportunity for more personalized treatment regimens. Precision medicine is driven to improve specific molecular alterations and treat particular subtypes of PD (Okun, 2017). Personalized medicine is not a novel treatment approach outside of PD, and it is currently used in an array of conditions, such as oncology and cystic fibrosis (Schilsky, 2014). The slow development of PD gives a unique opportunity to study the patient’s genome and environmental factors to target the causes of the disease in each specific group of patients (Barouki et al., 2018).
The availability of biomarkers to assess the appearance and progression of PD is fundamental to perform an early therapeutic intervention as well as to monitor the clinical response. So far there are several leading biomarker candidates including α-SYN (Atik et al., 2016), image biomarkers (Saeed et al., 2017), LRRK2 (Taymans et al., 2017), and microRNAs (Quinlan et al., 2017; Khodadadian et al., 2018).
The discovery of additional molecular biomarkers in groups of patients with different etiologies may permit the classification of PD subtypes according to clinical symptoms and differential molecular profiles. Accordingly, better profiling of individual patients with PD will allow the development of more effective therapies for specific PD subtypes, thus increasing the effectiveness and saving valuable time and resources during treatment.
The development of effective preventive or curative therapies for PD has been extremely challenging. The causes may involve additive or impeding factors, including a limited understanding of the mechanisms of neurodegeneration in PD, the heterogeneity of the pathology, and lack of adequate animal models. Also, the clinical effectiveness of preventative therapies has been challenging to assess due to the limitations in trial designs and because of the absence of reliable biomarkers to diagnose the pathology at early stages before irreversible neuronal damage occurs. Despite the current restrictions, success in preventing or halting the development of PD should be possible due to the constant appearance of new diagnostic methods and the current significant advances in gene therapy and other therapeutic approaches in the field of neurology and neuroscience.
AI and VE drafted the manuscript. GB and JG participated in revisions for intellectual content.
This work was supported by Fondo Nacional de Desarrollo Científico, Tecnológico y de Innovación Tecnológica (FONDECYT) grant #1190264 (to VE and AI).
The authors declare that the research was conducted in the absence of any commercial or financial relationships that could be construed as a potential conflict of interest.
5-HT, serotonin; AADC, the aromatic L-amino acid decarboxylase; AD, Alzheimer’s disease; cAMP, cyclic adenosine monophosphate; DBS, deep brain stimulation; ESC, embryonic and adult stem cells; GPi, globus pallidus internal; iPSC, induced pluripotent stem cells; LRRK2, Leucine-Rich Repeat Kinase 2; MSCs, mesenchymal stem cells; MSN, medium spiny neurons; NK1, substance P; NSC, neural stem cells; NOS, nitric oxide synthase; PD, Parkinson’s disease; RONS, reactive oxygen/nitrogen species; rRNA, ribosomal RNAs; SNc, substantia nigra pars compacta; SNpr, substantia nigra pars reticulate; STN, subthalamic nucleus; tRNA, transfer RNAs; UPDRS, the unified PD rating scale; VEGF, vascular endothelial growth factor; VTA, ventral tegmental area.
Aarsland, D. (2016). Cognitive impairment in Parkinson’s disease and dementia with Lewy bodies. Parkinsonism Relat. Disord. 22, S144–S148. doi: 10.1016/j.parkreldis.2015.09.034
Aarsland, D., Creese, B., Politis, M., Chaudhuri, K. R., Ffytche, D. H., Weintraub, D., et al. (2017). Cognitive decline in Parkinson disease. Nat. Rev. Neurol. 13, 217–231. doi: 10.1038/nrneurol.2017.27
Albin, R. L., Young, A. B., and Penney, J. B. (1989). The functional anatomy of basal ganglia disorders. Trends Neurosci. 12, 366–375. doi: 10.1016/0166-2236(89)90074-x
AlDakheel, A., Kalia, L. V., and Lang, A. E. (2014). Pathogenesis-targeted, disease-modifying therapies in Parkinson disease. Neurotherapeutics 11, 6–23. doi: 10.1007/s13311-013-0218-1
Alexander, G. E. (2004). Biology of Parkinson’s disease: pathogenesis and pathophysiology of a multisystem neurodegenerative disorder. Dialogues Clin. Neurosci. 6, 259–280. doi: 10.1146/annurev.ne.09.030186.002041
Alexander, G. E., DeLong, M. R., and Strick, P. L. (1986). Parallel organization of functionally segregated circuits linking basal ganglia and cortex. Annu. Rev. Neurosci. 9, 357–381. doi: 10.1146/annurev.ne.09.030186.002041
Allam, M. F., Del Castillo, A. S., and Navajas, R. F. (2005). Parkinson’s disease risk factors: genetic, environmental, or both? Neurol. Res. 27, 206–208. doi: 10.1179/016164105X22057
Alvarez-Ricartes, N., Oliveros-Matus, P., Mendoza, C., Perez-Urrutia, N., Echeverria, F., Iarkov, A., et al. (2018). Intranasal cotinine plus krill oil facilitates fear extinction, decreases depressive-like behavior, and increases hippocampal calcineurin a levels in mice. Mol. Neurobiol. 55, 7949–7960. doi: 10.1007/s12035-018-0916-0
Andén, N.-E., Carlsson, A., Dahlström, A., Fuxe, K., Hillarp, N.-Å., and Larsson, K. (1964). Demonstration and mapping out of nigro-neostriatal dopamine neurons. Life Sci. 3, 523–530. doi: 10.1016/0024-3205(64)90161-4
Anzalone, A. V., Randolph, P. B., Davis, J. R., Sousa, A. A., Koblan, L. W., Levy, J. M., et al. (2019). Search-and-replace genome editing without double-strand breaks or donor DNA. Nature 576, 149–157. doi: 10.1038/s41586-019-1711-4
Arnheim, N., and Cortopassi, G. (1992). Deleterious mitochondrial DNA mutations accumulate in aging human tissues. Mutat. Res. 275, 157–167. doi: 10.1016/0921-8734(92)90020-p
Atik, A., Stewart, T., and Zhang, J. (2016). α-synuclein as a biomarker for Parkinson’s disease. Brain Pathol. 26, 410–418. doi: 10.1111/bpa.12370
Axelsen, T. M., and Woldbye, D. P. D. (2018). Gene therapy for Parkinson’s disease, an update. J. Parkinsons Dis. 8, 195–215. doi: 10.3233/JPD-181331
Bandy, B., and Davison, A. J. (1990). Mitochondrial mutations may increase oxidative stress: implications for carcinogenesis and aging? Free Radic. Biol. Med. 8, 523–539. doi: 10.1016/0891-5849(90)90152-9
Banerjee, C., Nyengaard, J. R., Wevers, A., de Vos, R. A., Jansen Steur, E. N., Lindstrom, J., et al. (2000). Cellular expression of α7 nicotinic acetylcholine receptor protein in the temporal cortex in Alzheimer’s and Parkinson’s disease—a stereological approach. Neurobiol. Dis. 7, 666–672. doi: 10.1006/nbdi.2000.0317
Barker, R. A., Parmar, M., Kirkeby, A., Björklund, A., Thompson, L., and Brundin, P. (2016). Are stem cell-based therapies for Parkinson’s disease ready for the clinic in 2016? J. Parkinsons Dis. 6, 57–63. doi: 10.3233/JPD-160798
Barouki, R., Audouze, K., Coumoul, X., Demenais, F., and Gauguier, D. (2018). Integration of the human exposome with the human genome to advance medicine. Biochimie 152, 155–158. doi: 10.1016/j.biochi.2018.06.023
Barreto, G. E., Iarkov, A., and Moran, V. E. (2014). Beneficial effects of nicotine, cotinine and its metabolites as potential agents for Parkinson’s disease. Front. Aging Neurosci. 6:340. doi: 10.3389/fnagi.2014.00340
Barrow, T. R. (2015). Cell replacement therapy in Parkinson’s disease. Biosci. Horiz. 8:hzv002. doi: 10.1093/biohorizons/hzv002
Bartus, R. T., Baumann, T. L., Siffert, J., Herzog, C. D., Alterman, R., Boulis, N., et al. (2013). Safety/feasibility of targeting the substantia nigra with AAV2-neurturin in Parkinson patients. Neurology 80, 1698–1701. doi: 10.1212/wnl.0b013e3182904faa
Bartus, R. T., Weinberg, M. S., and Samulski, R. J. (2014). Parkinson’s disease gene therapy: success by design meets failure by efficacy. Mol. Ther. 22, 487–497. doi: 10.1038/mt.2013.281
Beal, M. F. (2005). Mitochondria take center stage in aging and neurodegeneration. Ann. Neurol. 58, 495–505. doi: 10.1002/ana.20624
Beckman, K. B., and Ames, B. N. (1998). The free radical theory of aging matures. Physiol. Rev. 78, 547–581. doi: 10.1152/physrev.1998.78.2.547
Bentivoglio, M., and Morelli, M. (2005). “The organization and circuits of mesencephalic dopaminergic neurons and the distribution of dopamine receptors in the brain,” in Dopamine: Handbook of Chemical Neuroanatomy, eds S. B. Dunnett, M. Bentivoglio, A. Bjorklund and T. Hökfelt (Amsterdam: Elsevier), 1–107.
Bernheimer, H., Birkmayer, W., Hornykiewicz, O., Jellinger, K., and Seitelberger, F. (1973). Brain dopamine and the syndromes of Parkinson and Huntington. Clinical, morphological and neurochemical correlations. J. Neurol. Sci. 20, 415–455. doi: 10.1016/0022-510x(73)90175-5
Betteridge, D. J. (2000). What is oxidative stress? Metabolism 49, 3–8. doi: 10.1016/s0026-0495(00)80077-3
Biezonski, D. K., Trifilieff, P., Meszaros, J., Javitch, J. A., and Kellendonk, C. (2015). Evidence for limited D1 and D2 receptor coexpression and colocalization within the dorsal striatum of the neonatal mouse. J. Comp. Neurol. 523, 1175–1189. doi: 10.1002/cne.23730
Birkmayer, W., and Hornykiewicz, O. (1961). The L-3,4-dioxyphenylalanine (DOPA)-effect in Parkinson-akinesia. Wien. Klin. Wochenschr. 73, 787–788.
Bitu Pinto, N., da Silva Alexandre, B., Neves, K. R. T., Silva, A. H., Leal, L. K. A., and Viana, G. S. (2015). Neuroprotective properties of the standardized extract from Camellia sinensis (green tea) and its main bioactive components, epicatechin and epigallocatechin gallate, in the 6-OHDA model of Parkinson’s disease. Evid. Based Complement. Alternat. Med. 2015:161092. doi: 10.1155/2015/161092
Björklund, A., and Dunnett, S. B. (2007). Dopamine neuron systems in the brain: an update. Trends Neurosci. 30, 194–202. doi: 10.1016/j.tins.2007.03.006
Björklund, A., and Lindvall, O. (2000). Cell replacement therapies for central nervous system disorders. Nat. Neurosci. 3, 537–544. doi: 10.1038/75705
Björklund, A., and Lindvall, O. (2017). Replacing dopamine neurons in Parkinson’s disease: how did it happen? J. Parkinsons Dis. 7, S21–S31. doi: 10.3233/jpd-179002
Björklund, A., Stenevi, U., and Svendgaard, N. (1976). Growth of transplanted monoaminergic neurones into the adult hippocampus along the perforant path. Nature 262, 787–790. doi: 10.1038/262787a0
Blonder, L. X., and Slevin, J. T. (2011). Emotional dysfunction in Parkinson’s disease. Behav. Neurol. 24, 201–217. doi: 10.3233/BEN-2011-0329
Bohnen, N. I., and Albin, R. L. (2011). The cholinergic system and Parkinson disease. Behav. Brain Res. 221, 564–573. doi: 10.1016/j.bbr.2009.12.048
Bohr, I. J., Ray, M. A., McIntosh, J. M., Chalon, S., Guilloteau, D., McKeith, I. G., et al. (2005). Cholinergic nicotinic receptor involvement in movement disorders associated with Lewy body diseases. An autoradiography study using [125I]α-conotoxin MII in the striatum and thalamus. Exp. Neurol. 191, 292–300. doi: 10.1016/j.expneurol.2004.10.004
Bordia, T., Campos, C., Huang, L., and Quik, M. (2008). Continuous and intermittent nicotine treatment reduces L-3,4-dihydroxyphenylalanine (L-DOPA)-induced dyskinesias in a rat model of Parkinson’s disease. J. Pharmacol. Exp. Ther. 327, 239–247. doi: 10.1124/jpet.108.140897
Bose, A., and Beal, M. F. (2016). Mitochondrial dysfunction in Parkinson’s disease. J. Neurochem. 139, 216–231. doi: 10.1111/jnc.13731
Braak, H., de Vos, R. A., Jansen, E. N., Bratzke, H., and Braak, E. (1998). Neuropathological hallmarks of Alzheimer’s and Parkinson’s diseases. Prog. Brain Res. 117, 267–285. doi: 10.1016/s0079-6123(08)64021-2
Brundin, P., Barker, R. A., and Parmar, M. (2010). Neural grafting in Parkinson’s disease Problems and possibilities. Prog. Brain Res. 184, 265–294. doi: 10.1016/S0079-6123(10)84014-2
Buccafusco, J. J., Shuster, L. C., and Terry, A. V. Jr. (2007). Disconnection between activation and desensitization of autonomic nicotinic receptors by nicotine and cotinine. Neurosci. Lett. 413, 68–71. doi: 10.1016/j.neulet.2006.11.028
Buccafusco, J. J., and Terry, A. V. Jr. (2003). The potential role of cotinine in the cognitive and neuroprotective actions of nicotine. Life Sci. 72, 2931–2942. doi: 10.1016/s0024-3205(03)00226-1
Burbulla, L. F., Song, P., Mazzulli, J. R., Zampese, E., Wong, Y. C., Jeon, S., et al. (2017). Dopamine oxidation mediates mitochondrial and lysosomal dysfunction in Parkinson’s disease. Science 357, 1255–1261. doi: 10.1126/science.aam9080
Burré, J., Sharma, M., and Südhof, T. C. (2018). Cell biology and pathophysiology of α-synuclein. Cold Spring Harb. Perspect. Med. 8:a024091. doi: 10.1101/cshperspect.a024091
Calabresi, P., Picconi, B., Tozzi, A., Ghiglieri, V., and Di Filippo, M. (2014). Direct and indirect pathways of basal ganglia: a critical reappraisal. Nat. Neurosci. 17, 1022–1030. doi: 10.1038/nn.3743
Callier, S., Snapyan, M., Le Crom, S., Prou, D., Vincent, J. D., and Vernier, P. (2003). Evolution and cell biology of dopamine receptors in vertebrates. Biol. Cell 95, 489–502. doi: 10.1016/s0248-4900(03)00089-3
Carlson, J. D., Neumiller, J. J., Swain, L. D., Mark, J., McLeod, P., and Hirschauer, J. (2014). Postoperative delirium in Parkinson’s disease patients following deep brain stimulation surgery. J. Clin. Neurosci. 21, 1192–1195. doi: 10.1016/j.jocn.2013.12.007
Carlsson, A. (2001). A half-century of neurotransmitter research: impact on neurology and psychiatry. Nobel lecture. Biosci. Rep. 21, 691–710. doi: 10.1023/a:1015556204669
Carlsson, A., Lindqvist, M., and Magnusson, T. (1957). 3,4-Dihydroxyphenylalanine and 5-hydroxytryptophan as reserpine antagonists. Nature 180:1200. doi: 10.1038/1801200a0
Castrioto, A., Kistner, A., Klinger, H., Lhommée, E., Schmitt, E., Fraix, V., et al. (2013). Psychostimulant effect of levodopa: reversing sensitisation is possible. J. Neurol. Neurosurg. Psychiatry 84, 18–22. doi: 10.1136/jnnp-2012-302444
Chakrabarti, S., Munshi, S., Banerjee, K., Thakurta, I. G., Sinha, M., and Bagh, M. B. (2011). Mitochondrial dysfunction during brain aging: role of oxidative stress and modulation by antioxidant supplementation. Aging Dis. 2, 242–256.
Chaturvedi, R. K., and Beal, M. F. (2013). Mitochondria targeted therapeutic approaches in Parkinson’s and Huntington’s diseases. Mol. Cell. Neurosci. 55, 101–114. doi: 10.1016/j.mcn.2012.11.011
Chen, S. H., Haam, J., Walker, M., Scappini, E., Naughton, J., and Martin, N. P. (2019). Recombinant viral vectors as neuroscience tools. Curr. Protoc. Neurosci. 87:e67. doi: 10.1002/cpns.67
Cheng, H. C., Ulane, C. M., and Burke, R. E. (2010). Clinical progression in Parkinson disease and the neurobiology of axons. Ann. Neurol. 67, 715–725. doi: 10.1002/ana.21995
Cheramy, A., Leviel, V., and Glowinski, J. (1981). Dendritic release of dopamine in the substantia nigra. Nature 289, 537–542. doi: 10.1038/289537a0
Course, M. M., Hsieh, C. H., Tsai, P. I., Codding-Bui, J. A., Shaltouki, A., and Wang, X. (2017). Live imaging mitochondrial transport in neurons. Neuromethods 123, 49–66. doi: 10.1007/978-1-4939-6890-9_3
Course, M. M., and Wang, X. (2016). Transporting mitochondria in neurons. F1000Res. 5:1735. doi: 10.12688/f1000research.7864.1
Dahlstroem, A., and Fuxe, K. (1964). Evidence for the existence of monoamine-containing neurons in the central nervous system. I. Demonstration of monoamines in the cell bodies of brain stem neurons. Acta Physiol. Scand. Suppl. 232, 231–255.
Deffains, M., and Bergman, H. (2015). Striatal cholinergic interneurons and cortico-striatal synaptic plasticity in health and disease. Mov. Disord. 30, 1014–1025. doi: 10.1002/mds.26300
DeLong, M. R. (1990). Primate models of movement disorders of basal ganglia origin. Trends Neurosci. 13, 281–285. doi: 10.1016/0166-2236(90)90110-v
Deutch, A. Y. (1993). Prefrontal cortical dopamine systems and the elaboration of functional corticostriatal circuits: implications for schizophrenia and Parkinson’s disease. J. Neural Transm. Gen. Sect. 91, 197–221. doi: 10.1007/bf01245232
Deverman, B. E., Ravina, B. M., Bankiewicz, K. S., Paul, S. M., and Sah, D. W. Y. (2018). Gene therapy for neurological disorders: progress and prospects. Nat. Rev. Drug Discov. 17, 641–659. doi: 10.1038/nrd.2018.110
Devi, L., Raghavendran, V., Prabhu, B. M., Avadhani, N. G., and Anandatheerthavarada, H. K. (2008). Mitochondrial import and accumulation of α-synuclein impair complex I in human dopaminergic neuronal cultures and Parkinson disease brain. J. Biol. Chem. 283, 9089–9100. doi: 10.1074/jbc.m710012200
Dexter, D. T., and Jenner, P. (2013). Parkinson disease: from pathology to molecular disease mechanisms. Free Radic. Biol. Med. 62, 132–144. doi: 10.1016/j.freeradbiomed.2013.01.018
Drucker-Colín, R., Madrazo, I., Ostrosky-Solís, F., Shkurovich, M., Franco, R., and Torres, C. (1988). Adrenal medullary tissue transplants in the caudate nucleus of Parkinson’s patients. Prog. Brain Res. 78, 567–574. doi: 10.1016/s0079-6123(08)60332-5
Drui, G., Carnicella, S., Carcenac, C., Favier, M., Bertrand, A., Boulet, S., et al. (2014). Loss of dopaminergic nigrostriatal neurons accounts for the motivational and affective deficits in Parkinson’s disease. Mol. Psychiatry 19, 358–367. doi: 10.1038/mp.2013.3
Dunn, L., Allen, G. F., Mamais, A., Ling, H., Li, A., Duberley, K. E., et al. (2014). Dysregulation of glucose metabolism is an early event in sporadic Parkinson’s disease. Neurobiol. Aging 35, 1111–1115. doi: 10.1016/j.neurobiolaging.2013.11.001
Eberling, J. L., Jagust, W. J., Christine, C. W., Starr, P., Larson, P., Bankiewicz, K. S., et al. (2008). Results from a phase I safety trial of hAADC gene therapy for Parkinson disease. Neurology 70, 1980–1983. doi: 10.1212/01.wnl.0000312381.29287.ff
Eberling, J. L., Kells, A. P., Pivirotto, P., Beyer, J., Bringas, J., Federoff, H. J., et al. (2009). Functional effects of AAV2-GDNF on the dopaminergic nigrostriatal pathway in parkinsonian rhesus monkeys. Hum. Gene Ther. 20, 511–518. doi: 10.1089/hum.2008.201
Echeverria, V., Barreto, G. E., Avila-Rodriguezc, M., Tarasov, V. V., and Aliev, G. (2017). Is VEGF a key target of cotinine and other potential therapies against Alzheimer disease? Curr. Alzheimer Res. 14, 1155–1163. doi: 10.2174/1567205014666170329113007
Echeverria, V., and Zeitlin, R. (2012). Cotinine: a potential new therapeutic agent against Alzheimer’s disease. CNS Neurosci. Ther. 18, 517–523. doi: 10.1111/j.1755-5949.2012.00317.x
Echeverria, V., Zeitlin, R., Burgess, S., Patel, S., Barman, A., Thakur, G., et al. (2011). Cotinine reduces amyloid-β aggregation and improves memory in Alzheimer’s disease mice. J. Alzheimers Dis. 24, 817–835. doi: 10.3233/JAD-2011-102136
English, D. F., Ibanez-Sandoval, O., Stark, E., Tecuapetla, F., Buzsaki, G., Deisseroth, K., et al. (2011). GABAergic circuits mediate the reinforcement-related signals of striatal cholinergic interneurons. Nat. Neurosci. 15, 123–130. doi: 10.1038/nn.2984
Eslamboli, A., Georgievska, B., Ridley, R. M., Baker, H. F., Muzyczka, N., Burger, C., et al. (2005). Continuous low-level glial cell line-derived neurotrophic factor delivery using recombinant adeno-associated viral vectors provides neuroprotection and induces behavioral recovery in a primate model of Parkinson’s disease. J. Neurosci. 25, 769–777. doi: 10.1523/JNEUROSCI.4421-04.2005
Fearnley, J. M., and Lees, A. J. (1991). Ageing and Parkinson’s disease: substantia nigra regional selectivity. Brain 114, 2283–2301. doi: 10.1093/brain/114.5.2283
Foley, J. A., Foltynie, T., Zrinzo, L., Hyam, J. A., Limousin, P., and Cipolotti, L. (2017). Apathy and reduced speed of processing underlie decline in verbal fluency following DBS. Behav. Neurol. 2017:7348101. doi: 10.1155/2017/7348101
Forgacs, P. B., and Bodis-Wollner, I. (2004). Nicotinic receptors and cognition in Parkinson’s disease: the importance of neuronal synchrony. J. Neural Transm. 111, 1317–1331. doi: 10.1007/s00702-004-0169-0
Fox, S. H., Katzenschlager, R., Lim, S. Y., Barton, B., de Bie, R. M., Seppi, K., et al. (2018). International Parkinson and movement disorder society evidence-based medicine review: update on treatments for the motor symptoms of Parkinson’s disease. Mov. Disord. 33, 1248–1266. doi: 10.1002/mds.27372
Fox, S. H., and Lang, A. E. (2008). Levodopa-related motor complications—phenomenology. Mov. Disord. 23, S509–S514. doi: 10.1002/mds.22021
Franco-Iborra, S., Vila, M., and Perier, C. (2016). The Parkinson disease mitochondrial hypothesis: where are we at? Neuroscientist 22, 266–277. doi: 10.1177/1073858415574600
Fratiglioni, L., and Wang, H. X. (2000). Smoking and Parkinson’s and Alzheimer’s disease: review of the epidemiological studies. Behav. Brain Res. 113, 117–120. doi: 10.1016/s0166-4328(00)00206-0
Frederick, A. L., Yano, H., Trifilieff, P., Vishwasrao, H. D., Biezonski, D., Mészáros, J., et al. (2015). Evidence against dopamine D1/D2 receptor heteromers. Mol. Psychiatry 20, 1373–1385. doi: 10.1038/mp.2014.166
Freed, C. R., Greene, P. E., Breeze, R. E., Tsai, W. Y., DuMouchel, W., Kao, R., et al. (2001). Transplantation of embryonic dopamine neurons for severe Parkinson’s disease. N. Engl. J. Med. 344, 710–719. doi: 10.1056/nejm200103083441002
Freed, C. R., Leehey, M. A., Zawada, M., Bjugstad, K., Thompson, L., and Breeze, R. E. (2003). Do patients with Parkinson’s disease benefit from embryonic dopamine cell transplantation? J. Neurol. 250, III44–III46. doi: 10.1007/s00415-003-1308-5
Fujita, M., Ichise, M., Zoghbi, S. S., Liow, J. S., Ghose, S., Vines, D. C., et al. (2006). Widespread decrease of nicotinic acetylcholine receptors in Parkinson’s disease. Ann. Neurol. 59, 174–177. doi: 10.1002/ana.20688
Gammon, K. (2014). Neurodegenerative disease: brain windfall. Nature 515, 299–300. doi: 10.1038/nj7526-299a
Gangarossa, G., Espallergues, J., de Kerchove d’Exaerde, A., El Mestikawy, S., Gerfen, C., Hervé, D., et al. (2013). Distribution and compartmental organization of GABAergic medium-sized spiny neurons in the mouse nucleus accumbens. Front. Neural Circuits 7:22. doi: 10.3389/fncir.2013.00022
Gao, J., Adam, B. L., and Terry, A. V. Jr. (2014). Evaluation of nicotine and cotinine analogs as potential neuroprotective agents for Alzheimer’s disease. Bioorg. Med. Chem. Lett. 24, 1472–1478. doi: 10.1016/j.bmcl.2014.02.008
Gao, J., La, B., Chapman, J. M., Bertrand, D., and Terry, A. V. (2012). “Neuroprotective effects of the nicotine metabolite, cotinine, and several structural analogs of cotinine,” in Paper Presented at the Society for Neuroscience (New Orleans, LA).
Gash, D. M., Zhang, Z., Ovadia, A., Cass, W. A., Yi, A., Simmerman, L., et al. (1996). Functional recovery in parkinsonian monkeys treated with GDNF. Nature 380, 252–255. doi: 10.1038/380252a0
Gelb, D. J., Oliver, E., and Gilman, S. (1999). Diagnostic criteria for Parkinson disease. Arch. Neurol. 56, 33–39. doi: 10.1001/archneur.56.1.33
Georgievska, B., Kirik, D., Rosenblad, C., Lundberg, C., and Björklund, A. (2002). Neuroprotection in the rat Parkinson model by intrastriatal GDNF gene transfer using a lentiviral vector. Neuroreport 13, 75–82. doi: 10.1097/00001756-200201210-00019
Gerfen, C. R., and Surmeier, D. J. (2011). Modulation of striatal projection systems by dopamine. Annu. Rev. Neurosci. 34, 441–466. doi: 10.1146/annurev-neuro-061010-113641
Getachew, B., Csoka, A. B., Aschner, M., and Tizabi, Y. (2019). Nicotine protects against manganese and iron-induced toxicity in SH-SY5Y cells: implication for Parkinson’s disease. Neurochem. Int. 124, 19–24. doi: 10.1016/j.neuint.2018.12.003
Gittis, A. H., and Kreitzer, A. C. (2012). Striatal microcircuitry and movement disorders. Trends Neurosci. 35, 557–564. doi: 10.1016/j.tins.2012.06.008
Gladyshev, V. N. (2014). The free radical theory of aging is dead. Long live the damage theory! Antioxid. Redox Signal. 20, 727–731. doi: 10.1089/ars.2013.5228
Goetz, C. G., Olanow, C. W., Koller, W. C., Penn, R. D., Cahill, D., Morantz, R., et al. (1989). Multicenter study of autologous adrenal medullary transplantation to the corpus striatum in patients with advanced Parkinson’s disease. N. Engl. J. Med. 320, 337–341. doi: 10.1056/nejm198902093200601
Grizzell, J. A., and Echeverria, V. (2015). New insights into the mechanisms of action of cotinine and its distinctive effects from nicotine. Neurochem. Res. 40, 2032–2046. doi: 10.1007/s11064-014-1359-2
Grizzell, J. A., Iarkov, A., Holmes, R., Mori, T., and Echeverria, V. (2014a). Cotinine reduces depressive-like behavior, working memory deficits, and synaptic loss associated with chronic stress in mice. Behav. Brain Res. 268, 55–65. doi: 10.1016/j.bbr.2014.03.047
Grizzell, J. A., Mullins, M., Iarkov, A., Rohani, A., Charry, L. C., and Echeverria, V. (2014b). Cotinine reduces depressive-like behavior and hippocampal vascular endothelial growth factor downregulation after forced swim stress in mice. Behav. Neurosci. 128, 713–721. doi: 10.1037/bne0000021
Grizzell, J. A., Patel, S., Barreto, G. E., and Echeverria, V. (2017). Cotinine improves visual recognition memory and decreases cortical Tau phosphorylation in the Tg6799 mice. Prog. Neuropsychopharmacol. Biol. Psychiatry 78, 75–81. doi: 10.1016/j.pnpbp.2017.05.010
Han, F., Grimes, D. A., Li, F., Wang, T., Yu, Z., Song, N., et al. (2016). Mutations in the glucocerebrosidase gene are common in patients with Parkinson’s disease from Eastern Canada. Int. J. Neurosci. 126, 415–421. doi: 10.3109/00207454.2015.1023436
Han, W., Liu, Y., Mi, Y., Zhao, J., Liu, D., and Tian, Q. (2015). α-synuclein (SNCA) polymorphisms and susceptibility to Parkinson’s disease: a meta-analysis. Am. J. Med. Genet. B Neuropsychiatr. Genet. 168B, 123–134. doi: 10.1002/ajmg.b.32288
Hariz, G. M., Limousin, P., Zrinzo, L., Tripoliti, E., Aviles-Olmos, I., Jahanshahi, M., et al. (2013). Gender differences in quality of life following subthalamic stimulation for Parkinson’s disease. Acta Neurol. Scand. 128, 281–285. doi: 10.1111/ane.12127
Harman, D. (1956). Aging: a theory based on free radical and radiation chemistry. J. Gerontol. 11, 290–300. doi: 10.1093/geronj/11.3.298
Hedlund, E., and Perlmann, T. (2009). Neuronal cell replacement in Parkinson’s disease. J. Intern. Med. 266, 358–371. doi: 10.1111/j.1365-2796.2009.02155.x
Hemmerle, A. M., Herman, J. P., and Seroogy, K. B. (2012). Stress, depression and Parkinson’s disease. Exp. Neurol. 233, 79–86. doi: 10.1016/j.expneurol.2011.09.035
Hennigan, K., D’Ardenne, K., and McClure, S. M. (2015). Distinct midbrain and habenula pathways are involved in processing aversive events in humans. J. Neurosci. 35, 198–208. doi: 10.1523/JNEUROSCI.0927-14.2015
Hitti, F. L., Yang, A. I., Gonzalez-Alegre, P., and Baltuch, G. H. (2019). Human gene therapy approaches for the treatment of Parkinson’s disease: an overview of current and completed clinical trials. Parkinsonism Relat. Disord. 66, 16–24. doi: 10.1016/j.parkreldis.2019.07.018
Højlund, A., Petersen, M. V., Sridharan, K. S., and Østergaard, K. (2017). Worsening of verbal fluency after deep brain stimulation in Parkinson’s disease: a focused review. Comput. Struct. Biotechnol. J. 15, 68–74. doi: 10.1016/j.csbj.2016.11.003
Hong, D. P., Fink, A. L., and Uversky, V. N. (2009). Smoking and Parkinson’s disease: does nicotine affect α-synuclein fibrillation? Biochim. Biophys. Acta 1794, 282–290. doi: 10.1016/j.bbapap.2008.09.026
Horowitz, M. P., Milanese, C., Di Maio, R., Hu, X., Montero, L. M., Sanders, L. H., et al. (2011). Single-cell redox imaging demonstrates a distinctive response of dopaminergic neurons to oxidative insults. Antioxid. Redox Signal. 15, 855–871. doi: 10.1089/ars.2010.3629
Huang, L. Z., Parameswaran, N., Bordia, T., Michael McIntosh, J., and Quik, M. (2009). Nicotine is neuroprotective when administered before but not after nigrostriatal damage in rats and monkeys. J. Neurochem. 109, 826–837. doi: 10.1111/j.1471-4159.2009.06011.x
Ihry, R. J., Worringer, K. A., Salick, M. R., Frias, E., Ho, D., Theriault, K., et al. (2018). p53 inhibits CRISPR-Cas9 engineering in human pluripotent stem cells. Nat. Med. 24, 939–946. doi: 10.1038/s41591-018-0050-6
Iriarte, L. M., Chacón, J., Madrazo, J., Chaparro, P., and Vadillo, J. (1988). Blink reflex in 57 parkinsonian patients with correlation between the clinical and electrophysiological parameters. Funct. Neurol. 3, 147–156.
Isaias, I. U., Spiegel, J., Brumberg, J., Cosgrove, K. P., Marotta, G., Oishi, N., et al. (2014). Nicotinic acetylcholine receptor density in cognitively intact subjects at an early stage of Parkinson’s disease. Front. Aging Neurosci. 6:213. doi: 10.3389/fnagi.2014.00213
James, J. R., and Nordberg, A. (1995). Genetic and environmental aspects of the role of nicotinic receptors in neurodegenerative disorders: emphasis on Alzheimer’s disease and Parkinson’s disease. Behav. Genet. 25, 149–159. doi: 10.1007/bf02196924
Jankovic, J. (2008). Are adenosine antagonists, such as istradefylline, caffeine, and chocolate, useful in the treatment of Parkinson’s disease? Ann. Neurol. 63, 267–269. doi: 10.1002/ana.21348
Joel, D., and Weiner, I. (2000). The connections of the dopaminergic system with the striatum in rats and primates: an analysis with respect to the functional and compartmental organization of the striatum. Neuroscience 96, 451–474. doi: 10.1016/s0306-4522(99)00575-8
Joyce, J. N. (2001). Dopamine D3 receptor as a therapeutic target for antipsychotic and antiparkinsonian drugs. Pharmacol. Ther. 90, 231–259. doi: 10.1016/s0163-7258(01)00139-5
Jurado-Coronel, J. C., Avila-Rodriguez, M., Capani, F., Gonzalez, J., Moran, V. E., and Barreto, G. E. (2016a). Targeting the nicotinic acetylcholine receptors (nAChRs) in astrocytes as a potential therapeutic target in Parkinson’s disease. Curr. Pharm. Des. 22, 1305–1311. doi: 10.2174/138161282210160304112133
Jurado-Coronel, J. C., Ávila-Rodriguez, M., Echeverria, V., Hidalgo, O. A., Gonzalez, J., Aliev, G., et al. (2016b). Implication of green tea as a possible therapeutic approach for Parkinson disease. CNS Neurol. Disord. Drug Targets 15, 292–300. doi: 10.2174/1871527315666160202125519
Jurado-Coronel, J. C., Loaiza, A. E., Diaz, J. E., Cabezas, R., Ashraf, G. M., Sahebkar, A., et al. (2019). (E)-nicotinaldehyde o-cinnamyloxime, a nicotine analog, attenuates neuronal cells death against rotenone-induced neurotoxicity. Mol. Neurobiol. 56, 1221–1232. doi: 10.1007/s12035-018-1163-0
Kabra, A., Sharma, R., Kabra, R., and Baghel, U. S. (2018). Emerging and alternative therapies for parkinson disease: an updated review. Curr. Pharm. Des. 24, 2573–2582. doi: 10.2174/1381612824666180820150150
Kalda, A., Yu, L., Oztas, E., and Chen, J. F. (2006). Novel neuroprotection by caffeine and adenosine A2A receptor antagonists in animal models of Parkinson’s disease. J. Neurol. Sci. 248, 9–15. doi: 10.1016/j.jns.2006.05.003
Kandinov, B., Giladi, N., and Korczyn, A. D. (2009). Smoking and tea consumption delay onset of Parkinson’s disease. Parkinsonism Relat. Disord. 15, 41–46. doi: 10.1016/j.parkreldis.2008.02.011
Kas, A., Bottlaender, M., Gallezot, J. D., Vidailhet, M., Villafane, G., Grégoire, M. C., et al. (2009). Decrease of nicotinic receptors in the nigrostriatal system in Parkinson’s disease. J. Cereb. Blood Flow Metab. 29, 1601–1608. doi: 10.1038/jcbfm.2009.74
Kawamata, J., Suzuki, S., and Shimohama, S. (2012). α7 nicotinic acetylcholine receptor mediated neuroprotection in Parkinson’s disease. Curr. Drug Targets 13, 623–630. doi: 10.2174/138945012800399026
Keane, P. C., Kurzawa, M., Blain, P. G., and Morris, C. M. (2011). Mitochondrial dysfunction in Parkinson’s disease. Parkinsons Dis. 2011:716871. doi: 10.4061/2011/716871
Kemp, J. M., and Powell, T. P. (1971). The termination of fibres from the cerebral cortex and thalamus upon dendritic spines in the caudate nucleus: a study with the Golgi method. Philos. Trans. R. Soc. Lond. B Biol. Sci. 262, 429–439. doi: 10.1098/rstb.1971.0105
Khodadadian, A., Hemmati-Dinarvand, M., Kalantary-Charvadeh, A., Ghobadi, A., and Mazaheri, M. (2018). Candidate biomarkers for Parkinson’s disease. Biomed. Pharmacother. 104, 699–704. doi: 10.1016/j.biopha.2018.05.026
Kim, H., Ham, S., Jo, M., Lee, G. H., Lee, Y. S., Shin, J. H., et al. (2017). CRISPR-Cas9 mediated telomere removal leads to mitochondrial stress and protein aggregation. Int. J. Mol. Sci. 18:E2093. doi: 10.3390/ijms18102093
Kim, Y.-C., Miller, A., Lins, L. C., Han, S. W., Keiser, M. S., Boudreau, R. L., et al. (2017). RNA interference of human α-synuclein in mouse. Front. Neurol. 8:13. doi: 10.3389/fneur.2017.00013
Kitta, T., Yabe, I., Kanno, Y., Higuchi, M., Ouchi, M., Togo, M., et al. (2018). Long-term outcome of adenosine A2A receptor antagonist on lower urinary tract symptoms in male Parkinson disease patients. Clin. Neuropharmacol. 41, 98–102. doi: 10.1097/WNF.0000000000000281
Klein, C., and Westenberger, A. (2012). Genetics of Parkinson’s disease. Cold Spring Harb. Perspect. Med. 2:a008888. doi: 10.1101/cshperspect.a008888
Kotzbauer, P. T., Trojanowsk, J. Q., and Lee, V. M. (2001). Lewy body pathology in Alzheimer’s disease. J. Mol. Neurosci. 17, 225–232. doi: 10.1385/jmn:17:2:225
Kubben, N., Zhang, W., Wang, L., Voss, T. C., Yang, J., Qu, J., et al. (2016). Repression of the antioxidant NRF2 pathway in premature aging. Cell 165, 1361–1374. doi: 10.1016/j.cell.2016.05.017
Kupsch, A., Oertel, W. H., Earl, C. D., and Sautter, J. (1995). Neuronal transplantation and neurotrophic factors in the treatment of Parkinson’s disease—update February 1995. J. Neural Transm. Suppl. 46, 193–207.
Lang, A. E., Gill, S., Patel, N. K., Lozano, A., Nutt, J. G., Penn, R., et al. (2006). Randomized controlled trial of intraputamenal glial cell line-derived neurotrophic factor infusion in Parkinson disease. Ann. Neurol. 59, 459–466. doi: 10.1002/ana.20737
Lange, K. W., Wells, F. R., Jenner, P., and Marsden, C. D. (1993). Altered muscarinic and nicotinic receptor densities in cortical and subcortical brain regions in Parkinson’s disease. J. Neurochem. 60, 197–203. doi: 10.1111/j.1471-4159.1993.tb05838.x
Lee, T., Kaneko, T., Shigemoto, R., Nomura, S., and Mizuno, N. (1997). Collateral projections from striatonigral neurons to substance P receptor-expressing intrinsic neurons in the striatum of the rat. J. Comp. Neurol. 388, 250–264. doi: 10.1002/(sici)1096-9861(19971117)388:2<250::aid-cne5>3.0.co;2-0
Lees, A. J., Hardy, J., and Revesz, T. (2009). Parkinson’s disease. Lancet 373, 2055–2066. doi: 10.1016/S0140-6736(09)60492-X
Lees, A. J., Tolosa, E., and Olanow, C. W. (2015). Four pioneers of L-dopa treatment: arvid carlsson, oleh hornykiewicz, george cotzias, and melvin yahr. Mov. Disord. 30, 19–36. doi: 10.1002/mds.26120
Leisman, G., Braun-Benjamin, O., and Melillo, R. (2014). Cognitive-motor interactions of the basal ganglia in development. Front. Syst. Neurosci. 8:16. doi: 10.3389/fnsys.2014.00016
Leisman, G., Machado, C., Melillo, R., and Mualem, R. (2012). Intentionality and “free-will” from a neurodevelopmental perspective. Front. Integr. Neurosci. 6:36. doi: 10.3389/fnint.2012.00036
Leisman, G., and Melillo, R. (2013). The basal ganglia: motor and cognitive relationships in a clinical neurobehavioral context. Rev. Neurosci. 24, 9–25. doi: 10.1515/revneuro-2012-0067
Lemay, S., Chouinard, S., Blanchet, P., Masson, H., Soland, V., Beuter, A., et al. (2004). Lack of efficacy of a nicotine transdermal treatment on motor and cognitive deficits in Parkinson’s disease. Prog. Neuropsychopharmacol. Biol. Psychiatry 28, 31–39. doi: 10.1016/s0278-5846(03)00172-6
Lester, H. A., Xiao, C., Srinivasan, R., Son, C. D., Miwa, J., Pantoja, R., et al. (2009). Nicotine is a selective pharmacological chaperone of acetylcholine receptor number and stoichiometry. Implications for drug discovery. AAPS J. 11, 167–177. doi: 10.1208/s12248-009-9090-7
LeWitt, P. A., Rezai, A. R., Leehey, M. A., Ojemann, S. G., Flaherty, A. W., Eskandar, E. N., et al. (2011). AAV2-GAD gene therapy for advanced Parkinson’s disease: a double-blind, sham-surgery controlled, randomised trial. Lancet Neurol. 10, 309–319. doi: 10.1016/S1474-4422(11)70039-4
Li, P., Beck, W. D., Callahan, P. M., Terry, A. V. Jr., and Bartlett, M. G. (2015). Pharmacokinetics of cotinine in rats: a potential therapeutic agent for disorders of cognitive function. Pharmacol. Rep. 67, 494–500. doi: 10.1016/j.pharep.2014.12.004
Lim, S. A., Kang, U. J., and McGehee, D. S. (2014). Striatal cholinergic interneuron regulation and circuit effects. Front. Synaptic Neurosci. 6:22. doi: 10.3389/fnsyn.2014.00022
Limousin, P., and Martinez-Torres, I. (2008). Deep brain stimulation for Parkinson’s disease. Neurotherapeutics 5, 309–319. doi: 10.1016/j.nurt.2008.01.006
Lindqvist, D., Kaufman, E., Brundin, L., Hall, S., Surova, Y., and Hansson, O. (2012). Non-motor symptoms in patients with Parkinson’s disease—correlations with inflammatory cytokines in serum. PLoS One 7:e47387. doi: 10.1371/journal.pone.0047387
Lindstrom, J. (1997). Nicotinic acetylcholine receptors in health and disease. Mol. Neurobiol. 15, 193–222. doi: 10.1007/BF02740634
Lindvall, O. (2015). Treatment of Parkinson’s disease using cell transplantation. Philos. Trans. R. Soc. Lond. B Biol. Sci. 370:20140370. doi: 10.1098/rstb.2014.0370
Lindvall, O. (2016). Clinical translation of stem cell transplantation in Parkinson’s disease. J. Intern. Med. 279, 30–40. doi: 10.1111/joim.12415
Lindvall, O., and Björklund, A. (2004). Cell therapy in Parkinson’s disease. NeuroRx 1, 382–393. doi: 10.1602/neurorx.1.4.382
Linert, W., Bridge, M. H., Huber, M., Bjugstad, K. B., Grossman, S., and Arendash, G. W. (1999). In vitro and in vivo studies investigating possible antioxidant actions of nicotine: relevance to Parkinson’s and Alzheimer’s diseases. Biochim. Biophys. Acta 1454, 143–152. doi: 10.1016/s0925-4439(99)00029-0
Lino, C. A., Harper, J. C., Carney, J. P., and Timlin, J. A. (2018). Delivering CRISPR: a review of the challenges and approaches. Drug Deliv. 25, 1234–1257. doi: 10.1080/10717544.2018.1474964
Lozano, A. M., and Snyder, B. J. (2008). Deep brain stimulation for parkinsonian gait disorders. J. Neurol. 255, 30–31. doi: 10.1007/s00415-008-4005-6
Lozano, A. M., Snyder, B. J., Hamani, C., Hutchison, W. D., and Dostrovsky, J. O. (2010). Basal ganglia physiology and deep brain stimulation. Mov. Disord. 25, S71–S75. doi: 10.1002/mds.22714
Lozano, C. S., Tam, J., and Lozano, A. M. (2018). The changing landscape of surgery for Parkinson’s disease. Mov. Disord. 33, 36–47. doi: 10.1002/mds.27228
Lu, X., Cui, Z., Liu, S., and Yin, F. (2018). MiRNAs participate in the diagnosis, pathogenesis and therapy of Parkinson’s disease. Histol. Histopathol. 33, 447–453. doi: 10.14670/HH-11-944
Lu, X., Kim-Han, J. S., Harmon, S., Sakiyama-Elbert, S. E., and O’Malley, K. L. (2014). The Parkinsonian mimetic, 6-OHDA, impairs axonal transport in dopaminergic axons. Mol. Neurodegener. 9:17. doi: 10.1186/1750-1326-9-17
Lundberg, C., Björklund, T., Carlsson, T., Jakobsson, J., Hantraye, P., Déglon, N., et al. (2008). Applications of lentiviral vectors for biology and gene therapy of neurological disorders. Curr. Gene Ther. 8, 461–473. doi: 10.2174/156652308786847996
Madrazo, I., Drucker-Colin, R., Madrazo, M., Zarate, A., Leon, V., Torres, C., et al. (1988a). Surgical technic of injecting autologous adrenal medullary tissue into the caudate nucleus for the treatment of Parkinson disease. Gac. Med. Mex. 124, 365–369.
Madrazo, I., León, V., Torres, C., Aguilera, M. C., Varela, G., Alvarez, F., et al. (1988b). Transplantation of fetal substantia nigra and adrenal medulla to the caudate nucleus in two patients with Parkinson’s disease. N. Engl. J. Med. 318:51. doi: 10.1056/nejm198801073180115
Maes, M. E., Colombo, G., Schulz, R., and Siegert, S. (2019). Targeting microglia with lentivirus and AAV: recent advances and remaining challenges. Neurosci. Lett. 707:134310. doi: 10.1016/j.neulet.2019.134310
Marks, W. J. Jr., Bartus, R. T., Siffert, J., Davis, C. S., Lozano, A., Boulis, N., et al. (2010). Gene delivery of AAV2-neurturin for Parkinson’s disease: a double-blind, randomised, controlled trial. Lancet Neurol. 9, 1164–1172. doi: 10.1016/S1474-4422(10)70254-4
Martin, Z. S., Neugebauer, V., Dineley, K. T., Kayed, R., Zhang, W., Reese, L. C., et al. (2012). α-Synuclein oligomers oppose long-term potentiation and impair memory through a calcineurin-dependent mechanism: relevance to human synucleopathic diseases. J. Neurochem. 120, 440–452. doi: 10.1111/j.1471-4159.2011.07576.x
Martin-Ruiz, C., Lawrence, S., Piggott, M., Kuryatov, A., Lindstrom, J., Gotti, C., et al. (2002). Nicotinic receptors in the putamen of patients with dementia with Lewy bodies and Parkinson’s disease: relation to changes in α-synuclein expression. Neurosci. Lett. 335, 134–138. doi: 10.1016/s0304-3940(02)01183-7
Matamales, M., Bertran-Gonzalez, J., Salomon, L., Degos, B., Deniau, J.-M., Valjent, E., et al. (2009). Striatal medium-sized spiny neurons: identification by nuclear staining and study of neuronal subpopulations in BAC transgenic mice. PLoS One 4:e4770. doi: 10.1371/journal.pone.0004770
Matsuura, K., and Tomimoto, H. (2015). Istradefylline is recommended for morning use: a report of 4 cases. Intern. Med. 54, 509–511. doi: 10.2169/internalmedicine.54.3522
McFarthing, K., Prakash, N., and Simuni, T. (2019). Clinical trial highlights: 1. gene therapy for Parkinson’s, 2. phase 3 study in focus—intec pharma’s accordion pill, 3. clinical trials resources. J. Parkinsons Dis. 9, 251–264. doi: 10.3233/jpd-199001
Meyer, P. M., Strecker, K., Kendziorra, K., Becker, G., Hesse, S., Woelpl, D., et al. (2009). Reduced α4β2*-nicotinic acetylcholine receptor binding and its relationship to mild cognitive and depressive symptoms in Parkinson disease. Arch. Gen. Psychiatry 66, 866–877. doi: 10.1001/archgenpsychiatry.2009.106
Meyer, P. M., Tiepolt, S., Barthel, H., Hesse, S., and Sabri, O. (2014). Radioligand imaging of α4β2* nicotinic acetylcholine receptors in Alzheimer’s disease and Parkinson’s disease. Q. J. Nucl. Med. Mol. Imaging 58, 376–386.
Miyoshi, Y., Zhang, Z., Ovadia, A., Lapchak, P. A., Collins, F., Hilt, D., et al. (1997). Glial cell line-derived neurotrophic factor-levodopa interactions and reduction of side effects in parkinsonian monkeys. Ann. Neurol. 42, 208–214. doi: 10.1002/ana.410420212
Moon, H. E., and Paek, S. H. (2015). Mitochondrial dysfunction in Parkinson’s disease. Exp. Neurobiol. 24, 103–116. doi: 10.5607/en.2015.24.2.103
Moran, V. E. (2012). Cotinine: beyond that expected, more than a biomarker of tobacco consumption. Front. Pharmacol. 3:173. doi: 10.3389/fphar.2012.00173
Moriarty, N., Parish, C. L., and Dowd, E. (2019). Primary tissue for cellular brain repair in Parkinson’s disease: promise, problems and the potential of biomaterials. Eur. J. Neurosci. 49, 472–486. doi: 10.1111/ejn.14051
Moro, E., Lozano, A. M., Pollak, P., Agid, Y., Rehncrona, S., Volkmann, J., et al. (2010). Long-term results of a multicenter study on subthalamic and pallidal stimulation in Parkinson’s disease. Mov. Disord. 25, 578–586. doi: 10.1002/mds.22735
Nadim, W. D., Simion, V., Benedetti, H., Pichon, C., Baril, P., and Morisset-Lopez, S. (2017). MicroRNAs in neurocognitive dysfunctions: new molecular targets for pharmacological treatments? Curr. Neuropharmacol. 15, 260–275. doi: 10.2174/1570159x14666160709001441
Nagy, A., Eördegh, G., Paróczy, Z., Márkus, Z., and Benedek, G. (2006). Multisensory integration in the basal ganglia. Eur. J. Neurosci. 24, 917–924. doi: 10.1111/j.1460-9568.2006.04942.x
Naso, M. F., Tomkowicz, B., Perry, W. L. III., and Strohl, W. R. (2017). Adeno-associated virus (AAV) as a vector for gene therapy. BioDrugs 31, 317–334. doi: 10.1007/s40259-017-0234-5
Nelson, A. B., Hammack, N., Yang, C. F., Shah, N. M., Seal, R. P., and Kreitzer, A. C. (2014). Striatal cholinergic interneurons Drive GABA release from dopamine terminals. Neuron 82, 63–70. doi: 10.1016/j.neuron.2014.01.023
Niethammer, M., Tang, C. C., LeWitt, P. A., Rezai, A. R., Leehey, M. A., Ojemann, S. G., et al. (2017). Long-term follow-up of a randomized AAV2-GAD gene therapy trial for Parkinson’s disease. JCI Insight 2:e90133. doi: 10.1172/jci.insight.90133
Ochi, J., and Shimizu, K. (1978). Occurrence of dopamine-containing neurons in the midbrain raphe nuclei of the rat. Neurosci. Lett. 8, 317–320. doi: 10.1016/0304-3940(78)90142-8
Oishi, N., Hashikawa, K., Yoshida, H., Ishizu, K., Ueda, M., Kawashima, H., et al. (2007). Quantification of nicotinic acetylcholine receptors in Parkinson’s disease with (123)I-5IA SPECT. J. Neurol. Sci. 256, 52–60. doi: 10.1016/j.jns.2007.02.014
Okun, M. S. (2017). Management of Parkinson disease in 2017: personalized approaches for patient-specific needs. JAMA 318, 791–792. doi: 10.1001/jama.2017.7914
Olanow, C. W., and Tatton, W. G. (1999). Etiology and pathogenesis of Parkinson’s disease. Annu. Rev. Neurosci. 22, 123–144. doi: 10.1146/annurev.neuro.22.1.123
O’Leary, K., Parameswaran, N., McIntosh, J. M., and Quik, M. (2008). Cotinine selectively activates a subpopulation of α3/α6β2 nicotinic receptors in monkey striatum. J. Pharmacol. Exp. Ther. 325, 646–654. doi: 10.1124/jpet.108.136838
Ouchi, Y., Yagi, S., Yokokura, M., and Sakamoto, M. (2009). Neuroinflammation in the living brain of Parkinson’s disease. Parkinsonism Relat. Disord. 15, S200–S204. doi: 10.1016/S1353-8020(09)70814-4
Palfi, S., Leventhal, L., Chu, Y., Ma, S. Y., Emborg, M., Bakay, R., et al. (2002). Lentivirally delivered glial cell line-derived neurotrophic factor increases the number of striatal dopaminergic neurons in primate models of nigrostriatal degeneration. J. Neurosci. 22, 4942–4954. doi: 10.1523/jneurosci.22-12-04942.2002
Parain, K., Hapdey, C., Rousselet, E., Marchand, V., Dumery, B., and Hirsch, E. C. (2003). Cigarette smoke and nicotine protect dopaminergic neurons against the 1-methyl-4-phenyl-1,2,3,6-tetrahydropyridine Parkinsonian toxin. Brain Res. 984, 224–232. doi: 10.1016/s0006-8993(03)03195-0
Parkinson, J. (2002). An essay on the shaking palsy. J. Neuropsychiatry Clin. Neurosci. 14, 223–236. doi: 10.1176/jnp.14.2.223
Patel, S., Grizzell, J. A., Holmes, R., Zeitlin, R., Solomon, R., Sutton, T. L., et al. (2014). Cotinine halts the advance of Alzheimer’s disease-like pathology and associated depressive-like behavior in Tg6799 mice. Front. Aging Neurosci. 6:162. doi: 10.3389/fnagi.2014.00162
Payne, B. A., and Chinnery, P. F. (2015). Mitochondrial dysfunction in aging: much progress but many unresolved questions. Biochim. Biophys. Acta 1847, 1347–1353. doi: 10.1016/j.bbabio.2015.05.022
Penaud-Budloo, M., Le Guiner, C., Nowrouzi, A., Toromanoff, A., Cherel, Y., Chenuaud, P., et al. (2008). Adeno-associated virus vector genomes persist as episomal chromatin in primate muscle. J. Virol. 82, 7875–7885. doi: 10.1128/JVI.00649-08
Perreault, M. L., Shen, M. Y., Fan, T., and George, S. (2015). Regulation of c-fos expression by the dopamine D1–D2 receptor heteromer. Neuroscience 285, 194–203. doi: 10.1016/j.neuroscience.2014.11.017
Perry, E. K., Morris, C. M., Court, J. A., Cheng, A., Fairbairn, A. F., McKeith, I. G., et al. (1995). Alteration in nicotine binding sites in Parkinson’s disease, Lewy body dementia and Alzheimer’s disease: possible index of early neuropathology. Neuroscience 64, 385–395. doi: 10.1016/0306-4522(94)00410-7
Picciotto, M. R., and Zoli, M. (2008). Neuroprotection via nAChRs: the role of nAChRs in neurodegenerative disorders such as Alzheimer’s and Parkinson’s disease. Front. Biosci. 13, 492–504. doi: 10.2741/2695
Pienaar, I. S., Lee, C. H., Elson, J. L., McGuinness, L., Gentleman, S. M., Kalaria, R. N., et al. (2015). Deep-brain stimulation associates with improved microvascular integrity in the subthalamic nucleus in Parkinson’s disease. Neurobiol. Dis. 74, 392–405. doi: 10.1016/j.nbd.2014.12.006
Piguet, F., Alves, S., and Cartier, N. (2017). Clinical gene therapy for neurodegenerative diseases: past, present, and future. Hum. Gene Ther. 28, 988–1003. doi: 10.1089/hum.2017.160
Pimlott, S. L., Piggott, M., Owens, J., Greally, E., Court, J. A., Jaros, E., et al. (2004). Nicotinic acetylcholine receptor distribution in Alzheimer’s disease, dementia with Lewy bodies, Parkinson’s disease, and vascular dementia: in vitro binding study using 5-[(125)i]-a-85380. Neuropsychopharmacology 29, 108–116. doi: 10.1038/sj.npp.1300302
Pisani, A., Bernardi, G., Ding, J., and Surmeier, D. J. (2007). Re-emergence of striatal cholinergic interneurons in movement disorders. Trends Neurosci. 30, 545–553. doi: 10.1016/j.tins.2007.07.008
Plenz, D., and Wickens, J. R. (2016). “The striatal skeleton: medium spiny projection neurons and their lateral connections,” in Handbook of Behavioral Neuroscience, eds H. Steiner and K. Y. Tseng. (Amsterdam: Elsevier BV), 121–136.
Pogocki, D., Ruman, T., Danilczuk, M., Danilczuk, M., Celuch, M., and Walajtys-Rode, E. (2007). Application of nicotine enantiomers, derivatives and analogues in therapy of neurodegenerative disorders. Eur. J. Pharmacol. 563, 18–39. doi: 10.1016/j.ejphar.2007.02.038
Postuma, R. B., Anang, J., Pelletier, A., Joseph, L., Moscovich, M., Grimes, D., et al. (2017). Caffeine as symptomatic treatment for Parkinson disease (Cafe-PD): a randomized trial. Neurology 89, 1795–1803. doi: 10.1212/wnl.0000000000004568
Postuma, R. B., Lang, A. E., Munhoz, R. P., Charland, K., Pelletier, A., Moscovich, M., et al. (2012). Caffeine for treatment of Parkinson disease: a randomized controlled trial. Neurology 79, 651–658. doi: 10.1212/WNL.0b013e318263570d
Prots, I., Grosch, J., Brazdis, R. M., Simmnacher, K., Veber, V., Havlicek, S., et al. (2018). α-Synuclein oligomers induce early axonal dysfunction in human iPSC-based models of synucleinopathies. Proc. Natl. Acad. Sci. U S A 115, 7813–7818. doi: 10.1073/pnas.1713129115
Prots, I., Veber, V., Brey, S., Campioni, S., Buder, K., Riek, R., et al. (2013). α-synuclein oligomers impair neuronal microtubule-kinesin interplay. J. Biol. Chem. 288, 21742–21754. doi: 10.1074/jbc.m113.451815
Quik, M., Bordia, T., and O’Leary, K. (2007). Nicotinic receptors as CNS targets for Parkinson’s disease. Biochem. Pharmacol. 74, 1224–1234. doi: 10.1016/j.bcp.2007.06.015
Quik, M., and Jeyarasasingam, G. (2000). Nicotinic receptors and Parkinson’s disease. Eur. J. Pharmacol. 393, 223–230. doi: 10.1016/s0014-2999(99)00888-2
Quik, M., and Kulak, J. M. (2002). Nicotine and nicotinic receptors; relevance to Parkinson’s disease. Neurotoxicology 23, 581–594. doi: 10.1016/s0161-813x(02)00036-0
Quik, M., Parameswaran, N., McCallum, S. E., Bordia, T., Bao, S., McCormack, A., et al. (2006). Chronic oral nicotine treatment protects against striatal degeneration in MPTP-treated primates. J. Neurochem. 98, 1866–1875. doi: 10.1111/j.1471-4159.2006.04078.x
Quik, M., and Wonnacott, S. (2011). α6β2* and α4β2* nicotinic acetylcholine receptors as drug targets for Parkinson’s disease. Pharmacol. Rev. 63, 938–966. doi: 10.1124/pr.110.003269
Quinlan, S., Kenny, A., Medina, M., Engel, T., and Jimenez-Mateos, E. M. (2017). MicroRNAs in neurodegenerative diseases. Int. Rev. Cell Mol. Biol. 334, 309–343. doi: 10.1016/bs.ircmb.2017.04.002
Quirion, R. (1993). Cholinergic markers in Alzheimer disease and the autoregulation of acetylcholine release. J. Psychiatry Neurosci. 18, 226–234.
Rangel-Barajas, C., Coronel, I., and Floran, B. (2015). Dopamine receptors and neurodegeneration. Aging Dis. 6, 349–368. doi: 10.14336/ad.2015.0330
Ray, N., and Strafella, A. P. (2010). Dopamine, reward, and frontostriatal circuitry in impulse control disorders in Parkinson’s disease: insights from functional imaging. Clin. EEG Neurosci. 41, 87–93. doi: 10.1177/155005941004100208
Redmond, D. E. Jr., McEntire, C. R., Kingsbery, J. P., Leranth, C., Elsworth, J. D., Bjugstad, K. B., et al. (2013). Comparison of fetal mesencephalic grafts, AAV-delivered GDNF and both combined in an MPTP-induced nonhuman primate Parkinson’s model. Mol. Ther. 21, 2160–2168. doi: 10.1038/mt.2013.180
Rees, H. A., Yeh, W.-H., and Liu, D. R. (2019). Development of hRad51-Cas9 nickase fusions that mediate HDR without double-stranded breaks. Nat. Commun. 10:2212. doi: 10.1038/s41467-019-09983-4
Rehani, K., Scott, D. A., Renaud, D., Hamza, H., Williams, L. R., Wang, H., et al. (2008). Cotinine-induced convergence of the cholinergic and PI3 kinase-dependent anti-inflammatory pathways in innate immune cells. Biochim. Biophys. Acta 1783, 375–382. doi: 10.1016/j.bbamcr.2007.12.003
Reig, R., and Silberberg, G. (2014). Multisensory integration in the mouse striatum. Neuron 83, 1200–1212. doi: 10.1016/j.neuron.2014.07.033
Richardson, R. M., Kells, A. P., Rosenbluth, K. H., Salegio, E. A., Fiandaca, M. S., Larson, P. S., et al. (2011). Interventional MRI-guided putaminal delivery of AAV2-GDNF for a planned clinical trial in Parkinson’s disease. Mol. Ther. 19, 1048–1057. doi: 10.1038/mt.2011.11
Riveles, K., Huang, L. Z., and Quik, M. (2008). Cigarette smoke, nicotine and cotinine protect against 6-hydroxydopamine-induced toxicity in SH-SY5Y cells. Neurotoxicology 29, 421–427. doi: 10.1016/j.neuro.2008.02.001
Rocha, E. M., De Miranda, B., and Sanders, L. H. (2018). α-synuclein: pathology, mitochondrial dysfunction and neuroinflammation in Parkinson’s disease. Neurobiol. Dis. 109, 249–257. doi: 10.1016/j.nbd.2017.04.004
Rodríguez, M. C., Ceaglio, N., Antuna, S., Tardivo, M. B., Etcheverrigaray, M., and Prieto, C. (2019). Production of therapeutic enzymes by lentivirus transgenesis. Adv. Exp. Med. Biol. 1148, 25–54. doi: 10.1007/978-981-13-7709-9_2
Rosenblad, C., Martinez-Serrano, A., and Björklund, A. (1998). Intrastriatal glial cell line-derived neurotrophic factor promotes sprouting of spared nigrostriatal dopaminergic afferents and induces recovery of function in a rat model of Parkinson’s disease. Neuroscience 82, 129–137. doi: 10.1016/s0306-4522(97)00269-8
Ross, G. W., Abbott, R. D., Petrovitch, H., Morens, D. M., Grandinetti, A., Tung, K. H., et al. (2000). Association of coffee and caffeine intake with the risk of Parkinson disease. JAMA 283, 2674–2679. doi: 10.1001/jama.283.20.2674
Ryan, Z. C., Craig, T. A., Folmes, C. D., Wang, X., Lanza, I. R., Schaible, N. S., et al. (2016). 1α,25-dihydroxyvitamin D3 regulates mitochondrial oxygen consumption and dynamics in human skeletal muscle cells. J. Biol. Chem. 291, 1514–1528. doi: 10.1074/jbc.m115.684399
Ryan, B. J., Hoek, S., Fon, E. A., and Wade-Martins, R. (2015). Mitochondrial dysfunction and mitophagy in Parkinson’s: from familial to sporadic disease. Trends Biochem. Sci. 40, 200–210. doi: 10.1016/j.tibs.2015.02.003
Saeed, U., Compagnone, J., Aviv, R. I., Strafella, A. P., Black, S. E., Lang, A. E., et al. (2017). Imaging biomarkers in Parkinson’s disease and Parkinsonian syndromes: current and emerging concepts. Transl. Neurodegener. 6:8. doi: 10.1186/s40035-017-0076-6
Safari, F., Hatam, G., Behbahani, A. B., Rezaei, V., Barekati-Mowahed, M., Petramfar, P., et al. (2019). CRISPR System: a high-throughput toolbox for research and treatment of Parkinson’s disease. Cell. Mol. Neurobiol. doi: 10.1007/s10571-019-00761-w [Epub ahead of print].
Sajadi, A., Bensadoun, J. C., Schneider, B. L., Lo Bianco, C., and Aebischer, P. (2006). Transient striatal delivery of GDNF via encapsulated cells leads to sustained behavioral improvement in a bilateral model of Parkinson disease. Neurobiol. Dis. 22, 119–129. doi: 10.1016/j.nbd.2005.10.006
Salat, D., and Tolosa, E. (2013). Levodopa in the treatment of Parkinson’s disease: current status and new developments. J. Parkinsons Dis. 3, 255–269. doi: 10.3233/jpd-130186
Salminen, O., Seppä, T., Gäddnäs, H., and Ahtee, L. (1999). The effects of acute nicotine on the metabolism of dopamine and the expression of Fos protein in striatal and limbic brain areas of rats during chronic nicotine infusion and its withdrawal. J. Neurosci. 19, 8145–8151. doi: 10.1523/jneurosci.19-18-08145.1999
Savica, R., Grossardt, B. R., Bower, J. H., Ahlskog, J. E., and Rocca, W. A. (2016). Time trends in the incidence of Parkinson disease. JAMA Neurol. 73, 981–989. doi: 10.1001/jamaneurol.2016.0947
Schapira, A. H. (1994). Evidence for mitochondrial dysfunction in Parkinson’s disease—a critical appraisal. Mov. Disord. 9, 125–138. doi: 10.1002/mds.870090202
Schapira, A. H. V., Chaudhuri, K. R., and Jenner, P. (2017a). Non-motor features of Parkinson disease. Nat. Rev. Neurosci. 18:509. doi: 10.1038/nrn.2017.91
Schapira, A. H. V., Fox, S. H., Hauser, R. A., Jankovic, J., Jost, W. H., Kenney, C., et al. (2017b). Assessment of safety and efficacy of safinamide as a levodopa adjunct in patients with Parkinson disease and motor fluctuations: a randomized clinical trial. JAMA Neurol. 74, 216–224. doi: 10.1001/jamaneurol.2016.4467
Schapira, A. H., and Jenner, P. (2011). Etiology and pathogenesis of Parkinson’s disease. Mov. Disord. 26, 1049–1055. doi: 10.1002/mds.23732
Schilsky, R. L. (2014). Implementing personalized cancer care. Nat. Rev. Clin. Oncol. 11, 432–438. doi: 10.1038/nrclinonc.2014.54
Schmaljohann, J., Gundisch, D., Minnerop, M., Bucerius, J., Joe, A., Reinhardt, M., et al. (2006). In vitro evaluation of nicotinic acetylcholine receptors with 2–[18F]F-A85380 in Parkinson’s disease. Nucl. Med. Biol. 33, 305–309. doi: 10.1016/j.nucmedbio.2005.12.012
Schott, B. H., Minuzzi, L., Krebs, R. M., Elmenhorst, D., Lang, M., Winz, O. H., et al. (2008). Mesolimbic functional magnetic resonance imaging activations during reward anticipation correlate with reward-related ventral striatal dopamine release. J. Neurosci. 28, 14311–14319. doi: 10.1523/JNEUROSCI.2058-08.2008
Schwarzschild, M. A., Chen, J. F., and Ascherio, A. (2002). Caffeinated clues and the promise of adenosine A(2A) antagonists in PD. Neurology 58, 1154–1160. doi: 10.1212/wnl.58.8.1154
Sharma, M., Naik, V., and Deogaonkar, M. (2016). Emerging applications of deep brain stimulation. J. Neurosurg. Sci. 60, 242–255.
Shimohama, S. (2009). Nicotinic receptor-mediated neuroprotection in neurodegenerative disease models. Biol. Pharm. Bull. 32, 332–336. doi: 10.1248/bpb.32.332
Singh, A., and Sen, D. (2017). MicroRNAs in Parkinson’s disease. Exp. Brain Res. 235, 2359–2374. doi: 10.1007/s00221-017-4989-1
Snow, B. J., Rolfe, F. L., Lockhart, M. M., Frampton, C. M., O’Sullivan, J. D., Fung, V., et al. (2010). A double-blind, placebo-controlled study to assess the mitochondria-targeted antioxidant MitoQ as a disease-modifying therapy in Parkinson’s disease. Mov. Disord. 25, 1670–1674. doi: 10.1002/mds.23148
Solbrig, M. V., Koob, G. F., and Lipkin, W. I. (2002). Key role for enkephalinergic tone in cortico-striatal-thalamic function. Eur. J. Neurosci. 16, 1819–1822. doi: 10.1046/j.1460-9568.2002.02240.x
Sorrentino, Z. A., Giasson, B. I., and Chakrabarty, P. (2019). α-synuclein and astrocytes: tracing the pathways from homeostasis to neurodegeneration in Lewy body disease. Acta Neuropathol. 138, 1–21. doi: 10.1007/s00401-019-01977-2
Soto-Otero, R., Méndez-Alvarez, E., Hermida-Ameijeiras, A., López-Real, A. M., and Labandeira-García, J. L. (2002). Effects of (−)-nicotine and (−)-cotinine on 6-hydroxydopamine-induced oxidative stress and neurotoxicity: relevance for Parkinson’s disease. Biochem. Pharmacol. 64, 125–135. doi: 10.1016/s0006-2952(02)01070-5
Stenevi, U., Björklund, A., and Svendgaard, N. A. (1976). Transplantation of central and peripheral monoamine neurons to the adult rat brain: techniques and conditions for survival. Brain Res. 114, 1–20. doi: 10.1016/0006-8993(76)91003-9
Stoker, T. B., Blair, N. F., and Barker, R. A. (2017). Neural grafting for Parkinson’s disease: challenges and prospects. Neural Regen. Res. 12, 389–392. doi: 10.4103/1673-5374.202935
Su, X., Kells, A. P., Huang, E. J., Lee, H. S., Hadaczek, P., Beyer, J., et al. (2009). Safety evaluation of AAV2-GDNF gene transfer into the dopaminergic nigrostriatal pathway in aged and parkinsonian rhesus monkeys. Hum. Gene Ther. 20, 1627–1640. doi: 10.1089/hum.2009.103
Sullivan, A. M., Pohl, J., and Blunt, S. B. (1998). Growth/differentiation factor 5 and glial cell line-derived neurotrophic factor enhance survival and function of dopaminergic grafts in a rat model of Parkinson’s disease. Eur. J. Neurosci. 10, 3681–3688. doi: 10.1046/j.1460-9568.1998.00378.x
Sulzer, D. (2007). Multiple hit hypotheses for dopamine neuron loss in Parkinson’s disease. Trends Neurosci. 30, 244–250. doi: 10.1016/j.tins.2007.03.009
Sun, M., Kong, L., Wang, X., Lu, X. G., Gao, Q., and Geller, A. I. (2005). Comparison of the capability of GDNF, BDNF, or both, to protect nigrostriatal neurons in a rat model of Parkinson’s disease. Brain Res. 1052, 119–129. doi: 10.1016/j.brainres.2005.05.072
Surguchev, A. A., and Surguchov, A. (2017). Synucleins and gene expression: ramblers in a crowd or cops regulating traffic? J. Environ. Manage. 10:224. doi: 10.3389/fnmol.2017.00224
Szymańska, I., Radecka, H., Radecki, J., and Kaliszan, R. (2007). Electrochemical impedance spectroscopy for study of amyloid β-peptide interactions with (−) nicotine ditartrate and (−) cotinine. Biosens. Bioelectron. 22, 1955–1960. doi: 10.1016/j.bios.2006.08.025
Taguchi, K., Watanabe, Y., Tsujimura, A., and Tanaka, M. (2019). Expression of α-synuclein is regulated in a neuronal cell type-dependent manner. Anat. Sci. Int. 94, 11–22. doi: 10.1007/s12565-018-0464-8
Tan, A. K. (2001). Current and emerging treatments in Parkinson’s disease. Ann. Acad. Med. Singapore 30, 128–133.
Tanimura, A., Pancani, T., Lim, S. A. O., Tubert, C., Melendez, A. E., Shen, W., et al. (2018). Striatal cholinergic interneurons and Parkinson’s disease. Eur. J. Neurosci. 47, 1148–1158. doi: 10.1111/ejn.13638
Taymans, J. M., Mutez, E., Drouyer, M., Sibran, W., and Chartier-Harlin, M. C. (2017). LRRK2 detection in human biofluids: potential use as a Parkinson’s disease biomarker? Biochem. Soc. Trans. 45, 207–212. doi: 10.1042/bst20160334
Terry, A. V. Jr., Callahan, P. M., and Bertrand, D. (2015). R-(+) and S-(−) isomers of cotinine augment cholinergic responses in vitro and in vivo. J. Pharmacol. Exp. Ther. 352, 405–418. doi: 10.1124/jpet.114.219881
Thobois, S., Ardouin, C., Schmitt, E., Lhommee, E., Klinger, H., Xie, J., et al. (2010). Behavioral disorders in Parkinson’s disease: from pathophysiology to the mastery of dopaminergic treatment. Rev. Neurol. 166, 816–821. doi: 10.1016/j.neurol.2010.07.006
Thomas, B., and Beal, M. F. (2007). Parkinson’s disease. Hum. Mol. Genet. 16, R183–R194. doi: 10.1093/hmg/ddm159
Thompson, L. H., and Parish, C. L. (2013). Transplantation of fetal midbrain dopamine progenitors into a rodent model of Parkinson’s disease. Methods Mol. Biol. 1059, 169–180. doi: 10.1007/978-1-62703-574-3_15
Timpka, J., Fox, T., Fox, K., Honig, H., Odin, P., Martinez-Martin, P., et al. (2016). Improvement of dyskinesias with L-dopa infusion in advanced Parkinson’s disease. Acta Neurol. Scand. 133, 451–458. doi: 10.1111/ane.12483
Tiwari, M. N., Agarwal, S., Bhatnagar, P., Singhal, N. K., Tiwari, S. K., Kumar, P., et al. (2015). Nicotine-encapsulated poly(lactic-co-glycolic) acid nanoparticles improve neuroprotective efficacy against MPTP-induced parkinsonism. Free Radic. Biol. Med. 65, 704–718. doi: 10.1016/j.freeradbiomed.2013.07.042
Toulorge, D., Guerreiro, S., Hild, A., Maskos, U., Hirsch, E. C., and Michel, P. P. (2011). Neuroprotection of midbrain dopamine neurons by nicotine is gated by cytoplasmic Ca2+. FASEB J. 25, 2563–2573. doi: 10.1096/fj.11-182824
Tzaferis, J. A., and McGinty, J. F. (2001). κ opioid receptor stimulation decreases amphetamine-induced behavior and neuropeptide mRNA expression in the striatum. Mol. Brain Res. 93, 27–35. doi: 10.1016/s0169-328x(01)00178-4
Vanhauwaert, R., Bharat, V., and Wang, X. (2019). Surveillance and transportation of mitochondria in neurons. Curr. Opin. Neurobiol. 57, 87–93. doi: 10.1016/j.conb.2019.01.015
Venkatesh, K., and Sen, D. (2017). Mesenchymal stem cells as a source of dopaminergic neurons: a potential cell based therapy for Parkinson’s disease. Curr. Stem Cell Res. Ther. 12, 326–347. doi: 10.2174/1574888x12666161114122059
Venkateshappa, C., Harish, G., Mythri, R. B., Mahadevan, A., Bharath, M. M., and Shankar, S. K. (2012). Increased oxidative damage and decreased antioxidant function in aging human substantia nigra compared to striatum: implications for Parkinson’s disease. Neurochem. Res. 37, 358–369. doi: 10.1007/s11064-011-0619-7
Videira, P. A. Q., and Castro-Caldas, M. (2018). Linking glycation and glycosylation with inflammation and mitochondrial dysfunction in Parkinson’s disease. Front. Neurosci. 12:381. doi: 10.3389/fnins.2018.00381
Vogelsang, D. A., and D’Esposito, M. (2018). Is there evidence for a rostral-caudal gradient in fronto-striatal loops and what role does dopamine play? Front. Neurosci. 12:242. doi: 10.3389/fnins.2018.00242
Voon, V., and Fox, S. H. (2007). Medication-related impulse control and repetitive behaviors in Parkinson disease. Arch. Neurol. 64, 1089–1096. doi: 10.1001/archneur.64.8.1089
Voon, V., Mehta, A. R., and Hallett, M. (2011). Impulse control disorders in Parkinson’s disease: recent advances. Curr. Opin. Neurol. 24, 324–330. doi: 10.1097/WCO.0b013e3283489687
Wang, S., and Huang, R. (2019). Non-viral nucleic acid delivery to the central nervous system and brain tumors. J. Gene Med. 21:e3091. doi: 10.1002/jgm.3091
Wang, Y., Li, S., Tian, Z., Sun, J., Liang, S., Zhang, B., et al. (2019). Generation of a caged lentiviral vector through an unnatural amino acid for photo-switchable transduction. Nucleic Acids Res. 47:e114. doi: 10.1093/nar/gkz659
Wang, F., Qin, Z., Lu, H., He, S., Luo, J., Jin, C., et al. (2019). Clinical translation of gene medicine. J. Gene Med. 21:e3108. doi: 10.1002/jgm.3108
Wang, X., Yuan, C., Huang, B., Fan, J., Feng, Y., Li, A. J., et al. (2019). Developing a versatile shotgun cloning strategy for single-vector-based multiplex expression of short interfering RNAs (siRNAs) in mammalian cells. ACS Synth. Biol. 8, 2092–2105. doi: 10.1021/acssynbio.9b00203
Watts, V. J., and Neve, K. A. (1997). Activation of type II adenylate cyclase by D2 and D4 but not D3 dopamine receptors. Mol. Pharmacol. 52, 181–186. doi: 10.1124/mol.52.2.181
Willner, P. (1991). Animal models as simulations of depression. Trends Pharmacol. Sci. 12, 131–136. doi: 10.1016/0165-6147(91)90529-2
Wirdefeldt, K., Adami, H. O., Cole, P., Trichopoulos, D., and Mandel, J. (2011). Epidemiology and etiology of Parkinson’s disease: a review of the evidence. Eur. J. Epidemiol. 26, S1–S58. doi: 10.1007/s10654-011-9581-6
Wolff, E. F., Mutlu, L., Massasa, E. E., Elsworth, J. D., Eugene Redmond, D. Jr., and Taylor, H. S. (2015). Endometrial stem cell transplantation in MPTP-exposed primates: an alternative cell source for treatment of Parkinson’s disease. J. Cell. Mol. Med. 19, 249–256. doi: 10.1111/jcmm.12433
Wu, Z., Yang, H., and Colosi, P. (2010). Effect of genome size on AAV vector packaging. Mol. Ther. 18, 80–86. doi: 10.1038/mt.2009.255
Xin, W., Schuebel, K. E., Jair, K. W., Cimbro, R., De Biase, L. M., Goldman, D., et al. (2019). Ventral midbrain astrocytes display unique physiological features and sensitivity to dopamine D2 receptor signaling. Neuropsychopharmacology 44, 344–355. doi: 10.1038/s41386-018-0151-4
Xu, X., Huang, J., Li, J., Liu, L., Han, C., Shen, Y., et al. (2016). Induced pluripotent stem cells and Parkinson’s disease: modelling and treatment. Cell Prolif. 49, 14–26. doi: 10.1111/cpr.12229
Yamamoto, K., Mirabeau, O., Bureau, C., Blin, M., Michon-Coudouel, S., Demarque, M., et al. (2013). Evolution of dopamine receptor genes of the D1 class in vertebrates. Mol. Biol. Evol. 30, 833–843. doi: 10.1093/molbev/mss268
Yamamoto, K., and Vernier, P. (2011). The evolution of dopamine systems in chordates. Front. Neuroanat. 5:21. doi: 10.3389/fnana.2011.00021
Zhang, Q., Chen, W., Tan, S., and Lin, T. (2017). Stem cells for modeling and therapy of Parkinson’s disease. Hum. Gene Ther. 28, 85–98. doi: 10.1089/hum.2016.116
Zhao, J., Zheng, Y., Xue, F., Chang, Y., Yang, H., and Zhang, J. (2016). Molecular basis of reactive oxygen species-induced inactivation of α4β2 nicotinic acetylcholine receptors. Free Radic. Biol. Med. 97, 520–530. doi: 10.1016/j.freeradbiomed.2016.07.012
Zharikov, A. D., Cannon, J. R., Tapias, V., Bai, Q., Horowitz, M. P., Shah, V., et al. (2015). shRNA targeting α-synuclein prevents neurodegeneration in a Parkinson’s disease model. J. Clin. Invest. 125, 2721–2735. doi: 10.1172/JCI64502
Keywords: gene therapy, prevention, cotinine, stem cells, precision medicine
Citation: Iarkov A, Barreto GE, Grizzell JA and Echeverria V (2020) Strategies for the Treatment of Parkinson’s Disease: Beyond Dopamine. Front. Aging Neurosci. 12:4. doi: 10.3389/fnagi.2020.00004
Received: 26 September 2019; Accepted: 09 January 2020;
Published: 31 January 2020.
Edited by:
Huaibin Cai, National Institute on Aging (NIA), United StatesReviewed by:
Guoxiang Liu, Northwestern University, United StatesCopyright © 2020 Iarkov, Barreto, Grizzell and Echeverria. This is an open-access article distributed under the terms of the Creative Commons Attribution License (CC BY). The use, distribution or reproduction in other forums is permitted, provided the original author(s) and the copyright owner(s) are credited and that the original publication in this journal is cited, in accordance with accepted academic practice. No use, distribution or reproduction is permitted which does not comply with these terms.
*Correspondence: Alexandre Iarkov, YWxleGFuZHJlLmlhcmtvdkB1c3MuY2w=; Valentina Echeverria, dmFsZW50aW5hLmVjaGV2ZXJyaWFAdXNzLmNs; dmFsZW50aW5hLmVjaGV2ZXJyaWEtbW9yYW5AdmEuZ292
Disclaimer: All claims expressed in this article are solely those of the authors and do not necessarily represent those of their affiliated organizations, or those of the publisher, the editors and the reviewers. Any product that may be evaluated in this article or claim that may be made by its manufacturer is not guaranteed or endorsed by the publisher.
Research integrity at Frontiers
Learn more about the work of our research integrity team to safeguard the quality of each article we publish.