- 1Department of Neurosciences, University of Florida, Gainesville, FL, United States
- 2Department of Physiology, Pharmacology and Neuroscience, University of South Carolina School of Medicine, Columbia, SC, United States
- 3Institute on Aging, University of Florida, Gainesville, FL, United States
As the number of individuals living beyond the age of 65 is rapidly increasing, so is the need to develop strategies to combat the age-related cognitive decline that may threaten independent living. Although the link between altered neuronal signaling and age-related cognitive impairments is not completely understood, it is evident that declining cognitive abilities are at least partially due to synaptic dysfunction. Aging is accompanied by well-documented changes in both excitatory and inhibitory synaptic signaling across species. Age-related synaptic alterations are not uniform across the brain, however, with different regions showing unique patterns of vulnerability in advanced age. In the hippocampus, increased activity within the CA3 subregion has been observed across species, and this can be reversed with anti-epileptic medication. In contrast to CA3, the dentate gyrus shows reduced activity with age and declining metabolic activity. Ketogenic diets have been shown to decrease seizure incidence and severity in epilepsy, improve metabolic function in diabetes type II, and improve cognitive function in aged rats. This link between neuronal activity and metabolism suggests that metabolic interventions may be able to ameliorate synaptic signaling deficits accompanying advanced age. We therefore investigated the ability of a dietary regimen capable of inducing nutritional ketosis and improving cognition to alter synapse-related gene expression across the dentate gyrus, CA3 and CA1 subregions of the hippocampus. Following 12 weeks of a ketogenic or calorie-matched standard diet, RTq-PCR was used to quantify expression levels of excitatory and inhibitory synaptic signaling genes within CA1, CA3 and dentate gyrus. While there were no age or diet-related changes in CA1 gene expression, expression levels were significantly altered within CA3 by age and within the dentate gyrus by diet for several genes involved in presynaptic glutamate regulation and postsynaptic excitation and plasticity. These data demonstrate subregion-specific alterations in synaptic signaling with age and the potential for a ketogenic diet to alter these processes in dissociable ways across different brain structures that are uniquely vulnerable in older animals.
Introduction
Although declining cognitive function is common among older adults, across species, significant neuron loss is not observed in most areas of the brain, including the hippocampus (Rapp and Gallagher, 1996; Merrill et al., 2001; Mohammed and Santer, 2001; Rapp et al., 2002; Keuker et al., 2004; Pannese, 2011). While cell number in the hippocampus remains stable throughout the lifespan, relative to younger animals, aged primates and rodents show well-documented changes in synaptic structure and function within this brain region that relate to behavioral impairments (Barnes, 2003; Rosenzweig and Barnes, 2003; Pannese, 2011; Morrison and Baxter, 2012). These findings point to hippocampal synapses as key players in age-related cognitive deficits, suggesting that therapies designed to improve synaptic function in this structure could mitigate age-related behavioral decline.
While the entire hippocampus undergoes alterations in synaptic structure, function and plasticity with advancing age, these changes are not uniform between the different subregions (for review, see Rosenzweig and Barnes, 2003; Burke and Barnes, 2006). Synaptophysin, a presynaptic marker, is significantly reduced in old compared to young rats in both CA3 and the dentate gyrus, but remains stable in aged CA1 (Smith et al., 2000). In the dentate gyrus, there is also a significant age-related decrease in synaptic number within the molecular layer (Geinisman et al., 1992) that correlates with impaired spatial memory (Geinisman et al., 1986). This reduction in dentate gyrus synapses is likely related to loss of perforant path fibers from the entorhinal cortex that terminate in the outer and middle molecular layers (Barnes and McNaughton, 1980; Foster et al., 1991; Yassa et al., 2010, 2011). In the CA3 subregion, in addition to the loss of synapses in older animals, pyramidal cells show higher firing rates (Wilson, 2005; Thomé et al., 2016). Moreover, there is an increase in intrinsic excitability (Simkin et al., 2015; Villanueva-Castillo et al., 2017), and interneurons lose their phenotypic expression profiles (Cadacio et al., 2003; Spiegel et al., 2013; Koh et al., 2014; Thomé et al., 2016). Although the Schaffer collateral to CA1 pyramidal cell synapse does not exhibit an age-related decrease in total number (Geinisman et al., 2004), perforated synapses have reduced postsynaptic densities that correlate with cognitive impairment (Nicholson et al., 2004). These morphological data and the associated age-related decrease in the excitatory post-synaptic field potential that is evoked from Schaffer collateral stimulation (Landfield et al., 1986; Barnes et al., 1992) suggest that a portion of CA1 synapses may become non-functional or silent in advanced age (Rosenzweig and Barnes, 2003).
At the level of gene expression, there is also evidence that advanced age leads to alterations that are hippocampal subregion dependent. Overall gene expression profiles obtained from microarray analysis of individual hippocampal subregions show that while many genes are differentially regulated in old compared to young rats, a higher number of genes are altered with age in CA3 relative to CA1 and the dentate gyrus indicating that different cell signaling properties are uniquely vulnerable in old age between CA1, CA3 and the dentate gyrus (Haberman et al., 2011). Moreover, age-related changes in gene expression in CA3 account for more variability in spatial memory performance (Haberman et al., 2013). Another study using next-generation RNA sequencing, however, identified the dentate as the subregion with the greatest number of age-related changes in gene expression (Ianov et al., 2016). While CA1 appears to have fewer age-associated changes in synaptic function and gene expression relative to the other subregions, there is evidence that metabolism and plasticity-related gene expression in this subregion is altered between young and older rats, and that these changes correlate with impaired cognitive performance (Blalock et al., 2003).
The importance of synaptic activity for behavior is highlighted by the brain’s disproportionate use of metabolic resources for this process. A wide body of literature supports age-related impairments in metabolism within the hippocampus (Gage et al., 1984; Rasgon et al., 2005; Goyal et al., 2017; Hernandez et al., 2018b). Because synaptic transmission is so metabolically costly (Attwell and Laughlin, 2001; Howarth et al., 2012), it is possible that age-related impairments in cerebral glucose metabolism contribute to age-related synaptic dysfunction. Although cerebral glucose metabolism declines with age, the ability to utilize ketone bodies for energy production does not (Lying-Tunell et al., 1981; Ogawa et al., 1996; Castellano et al., 2015; Cunnane et al., 2016). Therefore, the potential to bypass impaired glycolysis through the elevation of ketone body synthesis and promotion of fat oxidation may alter the expression of hippocampal synaptic signaling-related genes (Hernandez et al., 2018b) to ultimately improve cognitive function (Hernandez et al., 2018a).
Ketogenic diets are high in fat and low in carbohydrates, switching the body’s primary fuel source from glucose to ketone bodies. Ketogenic diets have been utilized to alter neuronal signaling patterns in patients with epilepsy for almost a century (Freeman et al., 1998). More recent evidence indicates that it can alter both excitatory and inhibitory signaling-related proteins within young and aged brains (Hernandez et al., 2018a, b). These alterations in protein expression have been shown to be both age- and brain region-specific, with more pronounced effects in the hippocampus than the prefrontal cortex. Quantification of proteins, however, does not easily afford the ability to delineate between hippocampal subregions, as is possible by examining gene expression at the level of RNA. This distinction is critical as previous work has suggested that ketone bodies may differentially affect the CA1 and dentate gyrus subregions. Specifically, the addition of medium chain triglyceride oil to rodent chow, which moderately increases ketone body levels in aged rats, was reported to increase the number of synaptic contacts and improve mitochondrial functioning within the dentate gyrus, but reduce synaptic contacts in CA1 (Balietti et al., 2008). Relatedly, caloric restriction, which can induce fat oxidation and elevate ketone body levels, has also been shown to have a greater impact on gene expression in CA3 and the dentate gyrus compared to CA1 (Zeier et al., 2011). Thus, there is a clear link between synaptic function, age and metabolism that differs across hippocampal subregions. However, the extent to which switching neurometabolism from glycolysis to fat oxidation impacts the expression of genes related to synaptic signaling has not been empirically examined in young and aged animals.
To investigate the role of a ketogenic dietary intervention on synaptic signaling-related gene expression within hippocampal subregions, young and aged rats were placed on an isocaloric ketogenic or control standard diet for 12 weeks. Excitatory and inhibitory synaptic signaling-related gene expression was quantified within the dentate gyrus, CA3 and CA1 regions of the hippocampus with RTq-PCR. While there were no significant age- or diet-dependent changes within CA1, there were several age-related changes in gene expression within CA3 and several diet-related changes observed within the dentate gyrus. These data add to a growing body of work establishing the CA3 hippocampal subregion as being particularly vulnerable to dysfunction with age and provides new insights into how promoting fat oxidation over glycolysis leads to dissociable effects between the different hippocampal subregions. To investigate changes in synapse-related expression at the level of proteins, Rho-associated coiled-coil containing protein kinases (ROCKs), which regulate the actin cytoskeleton at these synapses, was quantified within entire hippocampal homogenates from an additional set of rats on the same dietary regimen. While aged rats on a standard diet demonstrated significantly elevated ROCK2 levels, which may be indicative of excitatory synapse loss, aged rats fed a ketogenic diet have levels comparable to that of young rats.
Materials and Methods
Subjects and Handling
Young (4 months; n = 15) and aged (20 months: n = 16) male Fischer 344 × Brown Norway F1 Hybrid rats from the NIA colony at Charles River were used for the current study. One week after arrival to the colony, 7–8 rats per age group were placed on either the ketogenic or standard diet. Rats were housed individually and maintained on a 12-h reverse light/dark cycle. Feeding and all data collection, including tissue harvesting, were conducted during the rats’ dark cycle. All experimental procedures were performed in accordance with National Institutes of Health guidelines and were approved by Institutional Animal Care and Use Committees at the University of Florida.
Diet
Prior to diet administration, rats were randomly assigned to either a high-fat, low-carbohydrate ketogenic diet (KD; 75.85% fat, 20.12% protein, 3.85% carbohydrate; Lab Supply; 5722, Fort Worth, TX) mixed with medium chain triglyceride (MCT) oil (Neobee 895, Stephan, Northfield, IL) or a calorically and micronutrient matched standard diet (SD; 16.35% fat, 18.76% protein, 64.89% carbohydrate; Lab Supply; 1810727, Fort Worth, TX; for details on diet, see Hernandez et al., 2018b). Rats were weighed daily and fed equivalent calories (∼51 kcal/day) at the same time each day for 12 weeks. When rats are meal-fed consistently, ≥90% of their food is consumed within 3 h (unpublished observations). This caloric quantity, when fed once daily, is sufficient for healthy weight maintenance on both a KD and SD (Hernandez et al., 2018b), and the young rats in this study gained weight consuming this amount of kcal, though aged, obese animals lost weight (Supplementary Figure S1). In fact, young animals raised on this diet for greater than 1 year continue to gain weight as they age (unpublished data). Importantly, since all age and diet groups were calorically matched, energy intake could not account for group differences in the data. Access to water was ad libitum and all groups consumed comparable amounts of water (Hernandez et al., 2018b).
Confirmation of Nutritional Ketosis
To confirm rats were in nutritional ketosis, blood glucose and ketone body (β-hydroxybutyrate; BHB) levels were quantified on the day of sacrifice and tissue collection. For each measurement, one drop of blood was taken directly from the trunk immediately following decapitation for each test. Blood was collected directly onto the appropriate test strip (Abbott Diabetes Care, Inc., Alameda, CA; glucose SKU#: 9972865 and ketone SKU#: 7074565), which was placed into a Precision Xtra blood monitoring system (Abbott Diabetes Care Inc., Alameda, CA; SKU#: 9881465).
Tissue Collection
On the day of sacrifice, rats were fed their normal meal. After 1 h with access to the food, rats were placed into a bell jar containing isoflurane-saturated cotton (Abbott Laboratories, Chicago, IL, United States), separated from the animal by a wire mesh shield. Animals lost righting reflex within 30 s of being placed within the jar and were then immediately euthanized by rapid decapitation. Left and right hippocampi were dissected away from surrounding tissues on an ice-cold plate. The left hippocampus was frozen on dry ice and the right hippocampus was sub-dissected into dentate gyrus (DG; including the hilus), CA3 (including CA2) and CA1 regions from 1 mm-thick transverse sections made through the entire septal–temporal axis using a tissue chopper. Each slice was transferred to a chilled, back-lit glass stage and viewed through a dissecting scope to enable the visualization of cellular layers within the hippocampus. Dissecting filaments were used to separate the CA1 subregion from dentate gyrus at the hippocampal fissure. The boundary between CA3 and CA1 was demarcated by the transition from the thicker, more diffuse pyramidal cell layer of the CA3 to the thinner, more densely packed pyramidal cell layer of the CA1. The CA3 was separated from DG and hilus by a cut that extended between the distal edges of the superior and inferior blades of the DG, which were readily visualized as densely packed layers of granule neurons. Subregions were pooled across slices, frozen on dry ice and stored at −80°C until prepared for molecular and biochemical analyses. This sub-dissection strategy has been previously used to identify regional differences in protein function and mRNA levels of young and aging rats (Haberman et al., 2011; McQuail et al., 2013). The whole, left hippocampus was used for Western blotting and the CA1, CA3 and DG subregions dissected from the right hippocampus were used to measure mRNA by PCR.
Reverse Transcription and PCR Expression Assay
On the day of RNA extraction, tissue was transferred to QIAzol Lysis Reagent (PN: 79306, Qiagen, Frederick, MD, United States) and total RNA was isolated using the RNEasy Plus Mini kit according to the manufacturer’s protocol (PN: 73404, Qiagen). RNA concentration was determined with the use of a Qubit Fluorometer (Thermo Fisher Scientific, Waltham, MA, United States). The yield of RNA was consistent and reproduced across groups. The average RNA integrity number (RIN) was determined by TapeStation (Agilent Biosciences, Santa Clara, CA, United States) was 8.91 (±0.29 SD), and no sample had a RIN lower than 8.2.
From each sample, 600 ng of RNA was used to make cDNA using the RT2 First Strand cDNA Synthesis Kit (PN: 330411, Qiagen). Relative gene expression was measured using RT2 Profiler low-density PCR plates preloaded with qPCR primer assays for genes encoding GABA- and glutamate-related targets (PN: PARN-152ZA, Qiagen; see product page for details). Thermal cycling and data collection were accomplished using an ABI Real-Time PCR 7300. Raw gene expression data can be found at https://doi.org/10.6084/m9.figshare.9466577.v1.
Gene Exclusion Criteria and Statistical Analyses
Only RT-qPCR plates that passed the PCR array reproducibility, reverse transcription efficiency, and genomic DNA contamination quality control parameters set by Qiagen’s methods (RT2 Profiler PCR Array Data Analysis v3.5), as well as those reactions that produced the predicted peak by melting temperature (Tm) curve analysis were included in the final analyses. Genes were normalized to the house-keeping gene RPLP1. Any samples with cycle threshold (Ct) values for RPLP1 that were statistical outliers (as determined by the ROUT method with a false discovery rate (FDR) of q = 0.005) were excluded from downstream analyses. After necessary exclusions, the final group sizes across the different hippocampal subregions are shown in Table 1. Note, that for some genes the exclusion of outliers may have led to slightly smaller group sizes.
Each gene included in the RT-qPCR plates was cross-referenced with the Allen Brain Institute’s online in situ hybridization atlas1 and those not expressed in hippocampus were used to set the lowest Ct considered detectable. As such, the final number of genes tested per region was 60 (DG), 58 (CA3), and 55 (CA1). After normalization with RPLP1 and transformation using the 2(–ΔCt) method, values were standardized and expressed as z-scores. Two-factor ANOVAs (age × diet) were used to compare expression of gene transcripts between group samples in DG, CA3 and CA1 separately using the Benjamini–Hochberg method to correct for multiple comparisons with a FDR of q = 0.005 (Benjamini and Hochberg, 1995; Storey and Tibshirani, 2003).
Gene Functional Enrichment Analysis
The Database for Annotation, Visualization and Integrated Discovery (DAVID, v6.8) was used to determine any significantly enriched pathways associated with genes that met the FDR criteria (Huang et al., 2009a, b). Specifically, DAVID enabled the identification of the most robustly enriched Kyoto Encyclopedia of Genes and Genomes (KEGG) pathway per gene set. To simplify downstream analyses and interpretations, only the top FDR-ranked pathways per gene list were considered. Significantly enriched KEGG pathways were validated using a second enrichment analysis tool such as Enrichr (Chen et al., 2013; Kuleshov et al., 2016; Cowley et al., 2018).
Evaluation of ROCK Protein Levels
Whole hippocampal homogenates were used from 5 of these rats per age and diet group (n = 20 rats total) to quantify protein levels. Tissue from 1 aged SD-fed rat, however, was unrecoverable and not included in the analysis. The membrane and soluble fractions were isolated and quantified according to previously published procedures (McQuail et al., 2012; Hernandez et al., 2018a, b, c). ∼5 μg of protein per lane was separated on 4–15% TGX gels (Bio-Rad Laboratories, Hercules, CA, United States) at 200V for 40 min in Tris-glycine running buffer (Bio-Rad). Total protein was transferred to a 0.45 μm pore nitrocellulose membrane at 20 V for 7 min using iBlot Gel Transfer Nitrocellulose Stacks (NR13046-01, Invitrogen, Waltham, MA, United States) and an iBlot machine (Invitrogen, Waltham, MA, United States). All experiments were conducted in triplicate, and the loading order of samples was counterbalanced between gels and experiments to control for systematic variation in the electrophoresis and electroblotting procedures.
Immediately after transfer, membranes were stained for total protein using LiCor’s Revert total protein stain for 5 min (Li-Cor, 926-11011) and scanned using a 685 nm laser on an Odyssey IR Scanner (Li-Cor, Lincoln, NE, United States) to detect total protein levels. Membranes were then placed into Rockland blocking buffer (Rockland Blocking Buffer, Rockland, Gilbertsville, PA, United States) for 1 h at room temperature. After blocking, membranes were incubated at 4°C overnight with antibodies raised against ROCK2 (diluted 1:10,000, abcam #ab125025). Membranes were washed in Tris buffered saline before incubation in donkey anti-rabbit secondary antibodies conjugated to IRDye800 (diluted 1:15000; LI-COR). Blots were scanned using a 785 nm laser on an Odyssey IR Scanner. The target band signal to total protein signal ratio was calculated for each technical replicate and data from each independent biological sample were transformed to percent expression of the young, SD-fed group (that is, mean of this group is set to 100%) for statistical analysis.
Statistical Analysis
All data are expressed as the group median with interquartile ranges. Differences across age and diet groups were analyzed using a two-factor ANOVA with the between-subjects factors of age (2 levels: young and aged) and diet (2 levels: standard diet and ketogenic diet). For gene expression analyses, hippocampal subregions were analyzed independently. The null hypothesis was rejected at the level of p > 0.05 unless it was Bonferroni corrected for multiple comparisons. All analyses were performed with the Statistical Package for the Social Sciences v25 (IBM, Armonk, NY) or GraphPad Prism version 7.03 for Windows (GraphPad Software, La Jolla, CA, United States).
Results
Confirmation of Nutritional Ketosis
At the time of sacrifice and tissue collection, all rats on the ketogenic diet (KD) had significantly higher levels of β-hydroxybutyrate (BHB), the primary ketone body in the blood, relative to standard diet-fed (SD) rats (F[1,26] = 89.84; p < 0.0001; Figure 1A). Variance did not differ between young and aged KD-fed rats (F[1,13] = 0.67; p = 0.43; Levene’s test). Additionally, all rats on the ketogenic diet had significantly lower levels of circulating glucose relative to SD-fed rats (F[1,26] = 12.55; p = 0.002; Figure 1B). Neither BHB nor glucose significantly differed across age groups at this 12-week time point (F[1,26] = 1.41; p = 0.25 and F[1,26] = 0.54; p = 0.47, respectively). The ratio of glucose to ketone body levels can provide a single value quantification of inferred level of ketosis, which has been shown to correlate with cancer treatment efficacy (Meidenbauer et al., 2015). Therefore, the glucose ketone index (GKI) was also calculated as glucose (mmol/L)/BHB (mml/L). Thus, a lower GKI indicates higher levels of ketosis. The GKI was significantly lower in all KD-fed rats relative to SD-fed rats, regardless of age (F[1,26] = 59.91; p < 0.0001; Figure 1C). After 12 weeks on the diet, there was no significant effect of age on GKI (F[1,26] = 0.45; p = 0.51), and age and diet groups did not interact significantly for any of the three variables (p ≥ 0.40 for all).
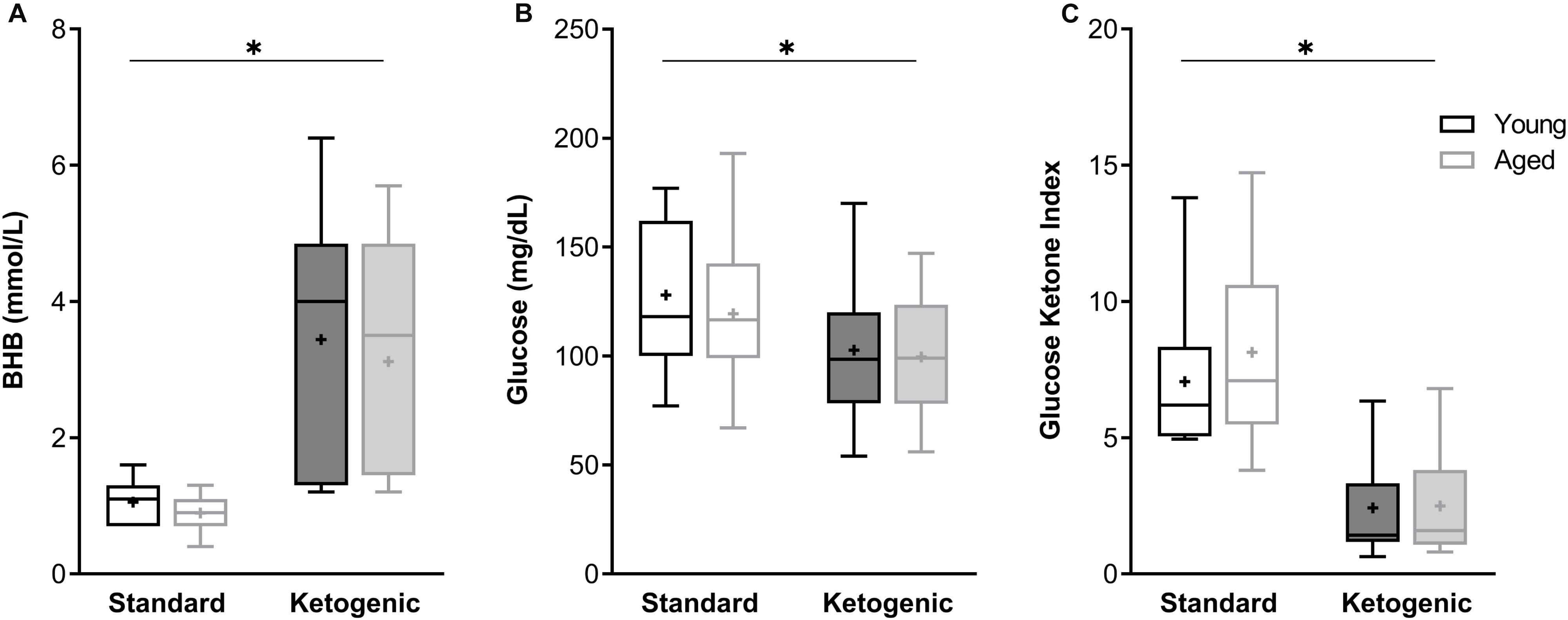
Figure 1. The ketogenic diet-fed rats had significantly higher (A) BHB, lower (B) glucose and lower (C) GKI relative to standard diet-fed rats, regardless of age group. Boxes represent the upper and lower quartiles around the median, the whiskers are the minimum and maximum observed values and + indicates the group mean. ∗ = main effect of diet.
Effects of Age and Diet on Hippocampal Subregion Gene Transcript Levels
A two-factor ANOVA (age × diet) confirmed that the house keeping gene RPLP1 did not differ by age or diet group across any region (largest mean difference was <0.425 Ct, Figure 2; main effects of age: F-values[1,20–23] = 0.891–1.762, p-values = 0.198–0.357; main effects of diet: F-values[1,20–23] = 0.014–1.927, p-values = 0.178–0.907; age × diet interactions: F-values[1,20–23] = 1.252–3.669, p-values = 0.069–0.275).
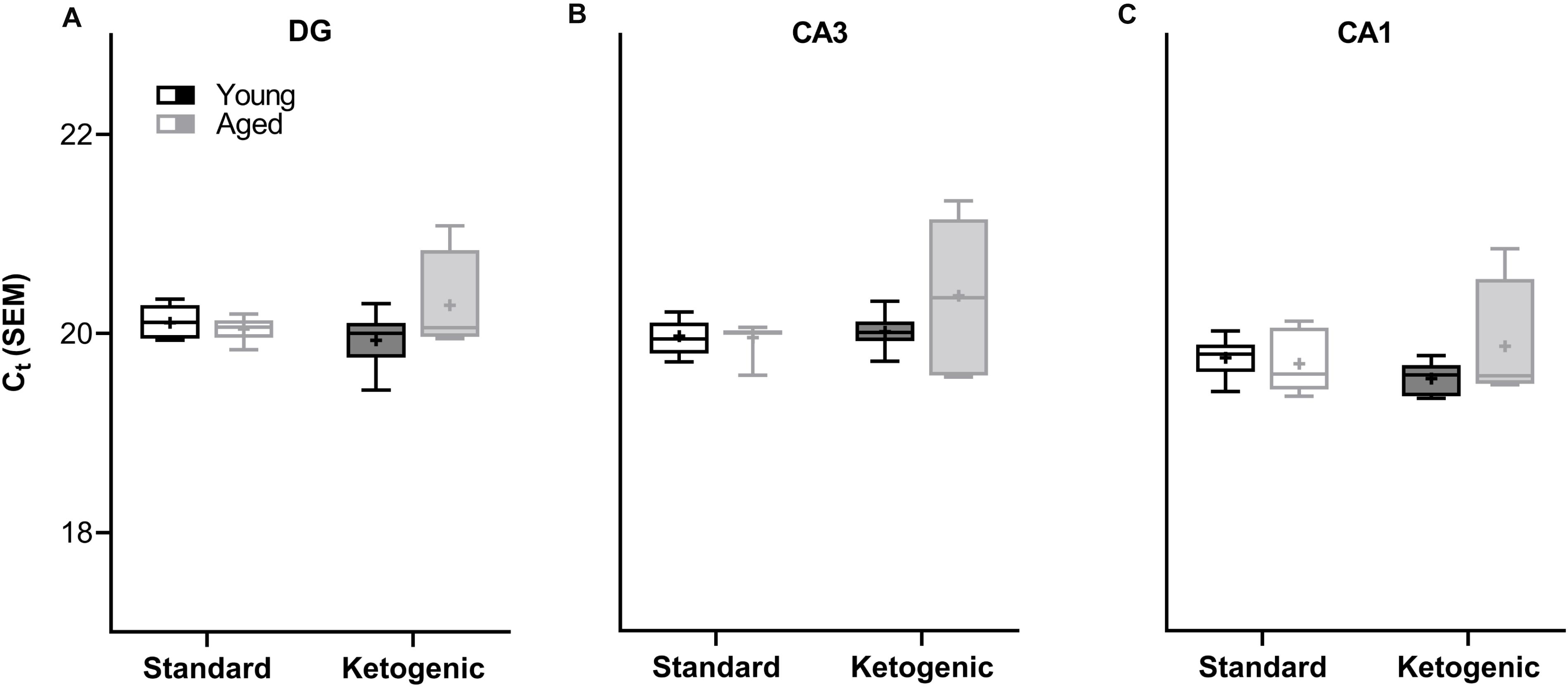
Figure 2. Cycle threshold (Ct) for housekeeping gene RPLP1 across age and diet groups in all hippocampal subregions examined. In the (A) dentate gyrus (DG), (B) CA3, and (C) CA1 subregions, the Ct did not vary across age and diet groups, nor was there a significant interaction. Thus, RPLP1 was utilized as a housekeeping gene to normalize the quantification of RNA levels for all other genes of interest. Boxes represent the upper and lower quartiles around the median, the whiskers are the minimum and maximum observed values and + indicates the group mean.
In the dentate gyrus (DG), a two-factor ANOVA (age × diet) detected that 40 gene transcripts were significantly reduced in the KD group compared to animals on the SD (F-values[1,21–22] = 11.418–22.921, p-values[FDR–adjusted] < 0.005; Figure 3). Figure 3 shows the relative expression levels of these 40 genes for the different age and diet groups. Interestingly, the main effects of age did not reach statistical significance for any of the genes examined (F-values[1,21–22] = 0.001–6.881, p-values[FDR–adjusted] > 0.005), nor were any significant age × diet interactions detected (F-values[1,21–22] = 0.010–3.934, p-values[FDR–adjusted] > 0.005). Analysis of the genes that were significantly affected by diet using the database for annotation, visualization and integrated discovery (DAVID; Huang et al., 2009a, b) identified that 21 of the genes affected by diet contributed to the top ranking and reliable enrichment of the glutamatergic synapse pathway (p(FDR–adjusted) = 6.632 × 10–25; Enrichr validation: p(FDR–adjusted) = 1.291 × 10–35; Figure 3). We further categorized the genes contributing to the enrichment of this pathway into presynaptic glutamate regulation, postsynaptic excitation, and post-synaptic plasticity. Figure 4 shows the z-scored values for these genes by category across the different diet and age groups. Supplementary Table S3 contains descriptive statistics and Supplementary Table S4 contains a statistical summary for all genes in the DG. Other pathways most significantly associated with genes affected by the KD in the DG are presented in Supplementary Table S1.
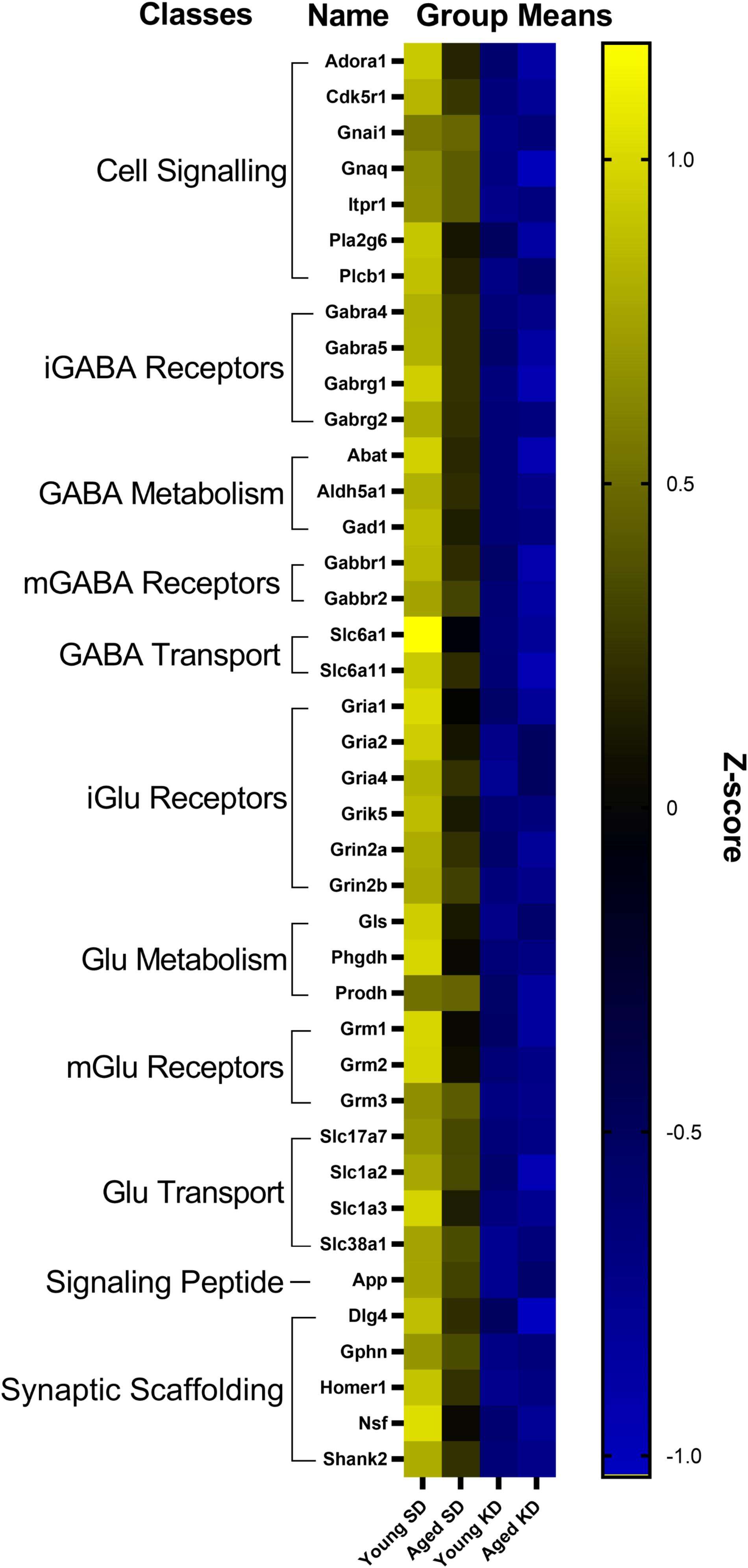
Figure 3. Genes affected by diet in the dentate gyrus. The heat map represents mean z-scores of all genes that were significantly reduced (FDR q = 0.005 threshold) as a function of diet. Genes were categorized into biologically relevant processes, and experimental groups were ordered to reflect the main effect of diet. Yellow represents greater gene expression, whereas blue represents lower gene expression. Black represents no change.
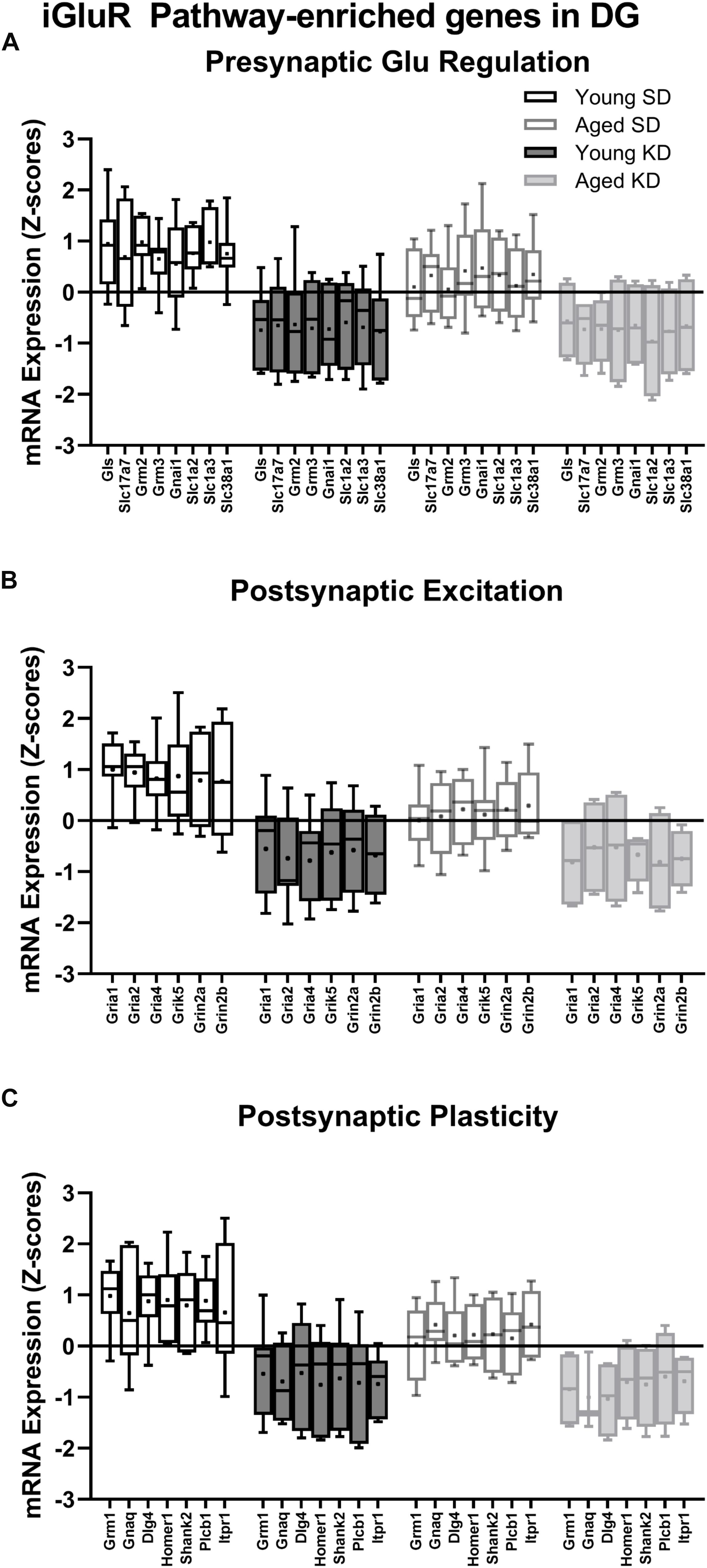
Figure 4. Genes within the ionotropic glutamate receptor (iGluR) pathway in the DG reliably changed with diet. (A) 8 genes associated with presynaptic glutamate regulation were reduced as a function of diet. (B) 6 genes associated with postsynaptic excitation were reduced as a function of diet. (C) 7 genes associated with postsynaptic plasticity were reduced as a function of diet. Black bars represent young; gray bars represent aged; open bars represent standard diet; and filled bars represent ketogenic diet. Boxes represent the upper and lower quartiles around the median, the whiskers are the minimum and maximum observed values and +indicates the group mean.
In contrast to DG, a two-factor ANOVA (age × diet) did not detect a significant main effect of diet on the expression of synapse-related genes in CA3 (F-values[1,20–23] = 1.259–15.734, p-values(FDR–adjusted) > 0.005). Age group, however, did significantly affect 24 gene transcripts, with aged rats having significantly lower expression levels compared to young animals (F-values[1,20–23] = 12.683–28.988, p-values[FDR–adjusted] < 0.005; Figure 5). The interaction effect of diet and age group did not reach statistical significance for any of the genes examined in CA3 (F-values[1,20–23] = 0.001–4.028, p-values(FDR–adjusted) > 0.005). Analysis of genes affected by age using DAVID identified that 12 of these genes contributed to the top ranking and reliable enrichment of the glutamatergic synapse pathway (p(FDR–adjusted) = 2.975 × 10–12; Enrichr validation: p(FDR–adjusted) = 5.055 × 10–26; Figure 5). Genes contributing to the enrichment of this pathway were categorized into presynaptic glutamate regulation, postsynaptic excitation, and post-synaptic plasticity (Figure 6). Supplementary Table S5 contains descriptive statistics and Supplementary Table S6 contains a statistical summary for all genes in CA3. Other pathways most significantly associated with these genes are presented in Supplementary Table S2.
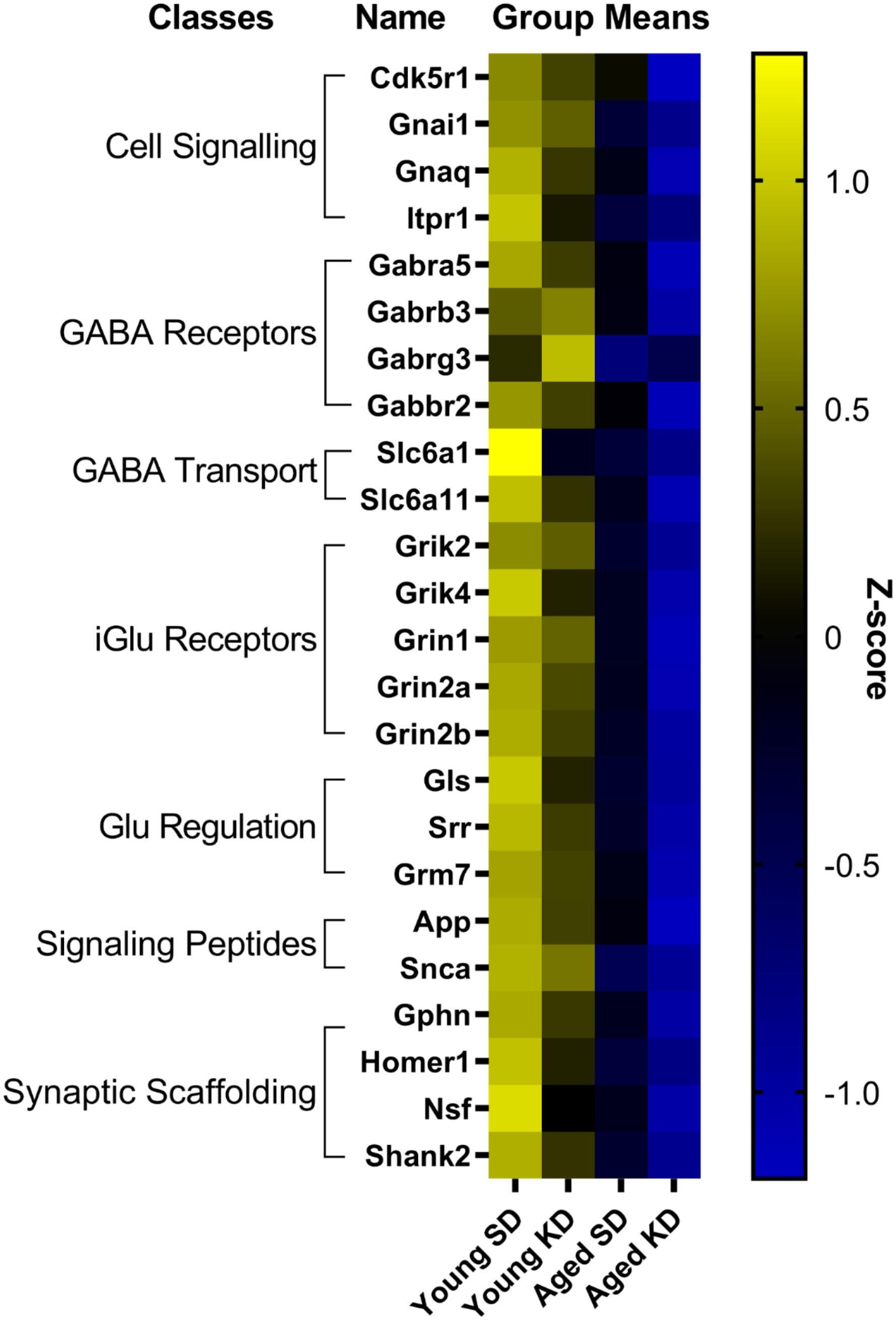
Figure 5. Genes affected by age in CA3. The heat map represents mean z-scores of all genes that were significantly reduced (FDR q = 0.005 threshold) as a function of age. Genes were categorized into biologically relevant processes, and experimental groups were ordered to reflect the main effect of age. Yellow represents greater gene expression, whereas blue represents lower gene expression. Black represents no change.
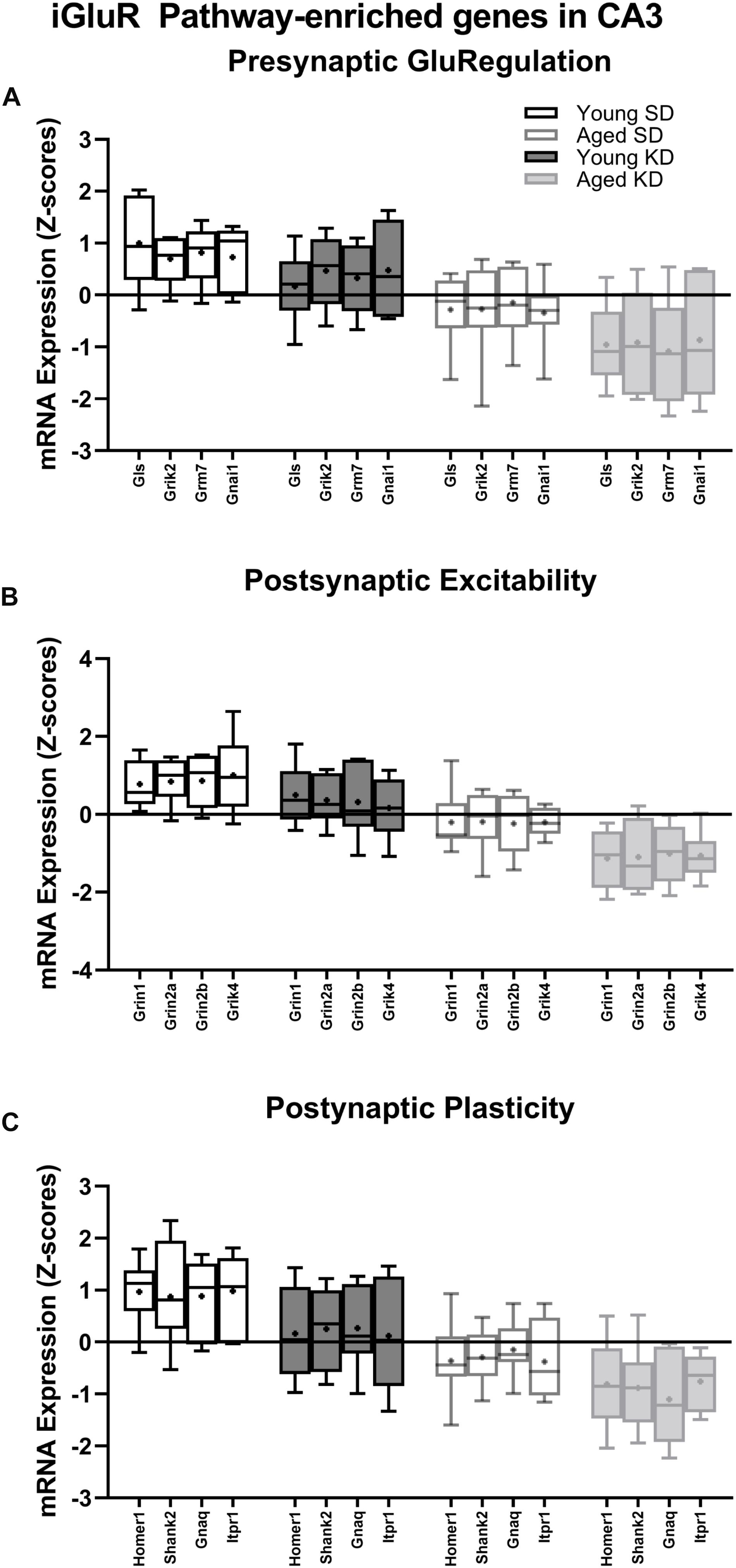
Figure 6. Genes within the ionotropic glutamate receptor (iGluR) pathway reliably changed with age within CA3. (A) 4 genes associated with presynaptic glutamate regulation were reduced as a function of age. (B) 4 genes associated with postsynaptic excitation were reduced as a function of age. (C) 4 genes associated with postsynaptic plasticity were reduced as a function of age. Black bars represent young; gray bars represent aged; open bars represent standard diet; and filled bars represent ketogenic diet. Boxes represent the upper and lower quartiles around the median, the whiskers are the minimum and maximum observed values and +indicates the group mean.
Finally, within CA1, there were no significant effects of age or diet on gene transcript levels, nor were there any significant age × diet interactions after adjusting for a FDR of q = 0.005 (two-factor, age × diet ANOVA: F-values[1,18–20] = 0.000–8.629, ps(FDR–adjusted) > 0.005). Supplementary Table S7 contains descriptive statistics and Supplementary Table S8 contains a statistical summary for all genes in CA1.
The Ketogenic Diet Restores Age-Related Increases in Hippocampal ROCK2 Expression
The reduction in gene transcripts associated with glutamatergic signaling in the DG suggests that long-term nutritional ketosis could decrease excitatory synaptic transmission. Previous work examining protein levels of the vesicular glutamate transporter 1 in whole hippocampal homogenates, however, showed that the KD remediated age-related loss of this essential synaptic protein without otherwise influencing expression in young rats (Hernandez et al., 2018b). This previous work suggests that there is not an overall loss of excitatory synapses within the hippocampus following the KD, and that there may even be a restoration of synapse number in aged rats. In the hippocampus, most excitatory synapses are on dendritic spines (McKinney, 2010). Rho-associated coiled-coil containing protein kinases (ROCKs) regulate the actin cytoskeleton at these synapses, and inhibition of these molecules increases spine density (Swanger et al., 2016). These data suggest that elevated ROCK levels in the hippocampus could potentially be related to excitatory synapse loss. Furthermore, inhibition of ROCK improves spatial working memory in aged rats suggesting that decreasing ROCK may be beneficial for the treatment of cognitive aging or neurodegenerative disorders resulting in dendritic spine alterations (Kubo et al., 2008; Henderson et al., 2016). Therefore, ROCK2 protein levels within the soluble fraction of hippocampal homogenates were quantified via Western Blotting. Within the hippocampus, there was a significant age-dependent increase in ROCK2 expression in aged rats on the SD relative to young animals on the SD (F[1,7] = 11.61; p = 0.01; Figure 7). Because of the main effect of age across SD-fed subjects, the possibility that a KD could restore these levels was explored using a multivariate ANOVA. While there were no main effects of either age (F[1,15] = 2.21; p = 0.16) or diet (F[1,15] = 4.36; p = 0.054), there was a significant interaction between age and diet (F[1,15] = 11.74; p = 0.004). Furthermore, when young and aged rats on KD were directly compared, there was no longer an effect of age (F[1,8] = 1.97; p = 0.20). These data show that the KD was able to reduce levels of ROCK2 in the hippocampus of aged animals to levels comparable to those observed in young rats. This observation provides indirect evidence that the KD does not lead to an overall reduction of excitatory synapse number. Thus, the diet related changes within the dentate gyrus are likely due to altered regulation of glutamatergic synapses without a reduction in excitatory synapses. Functional assessment of hippocampal neurophysiology will be necessary to determine the impact of long-term nutritional ketosis on the aged hippocampus.
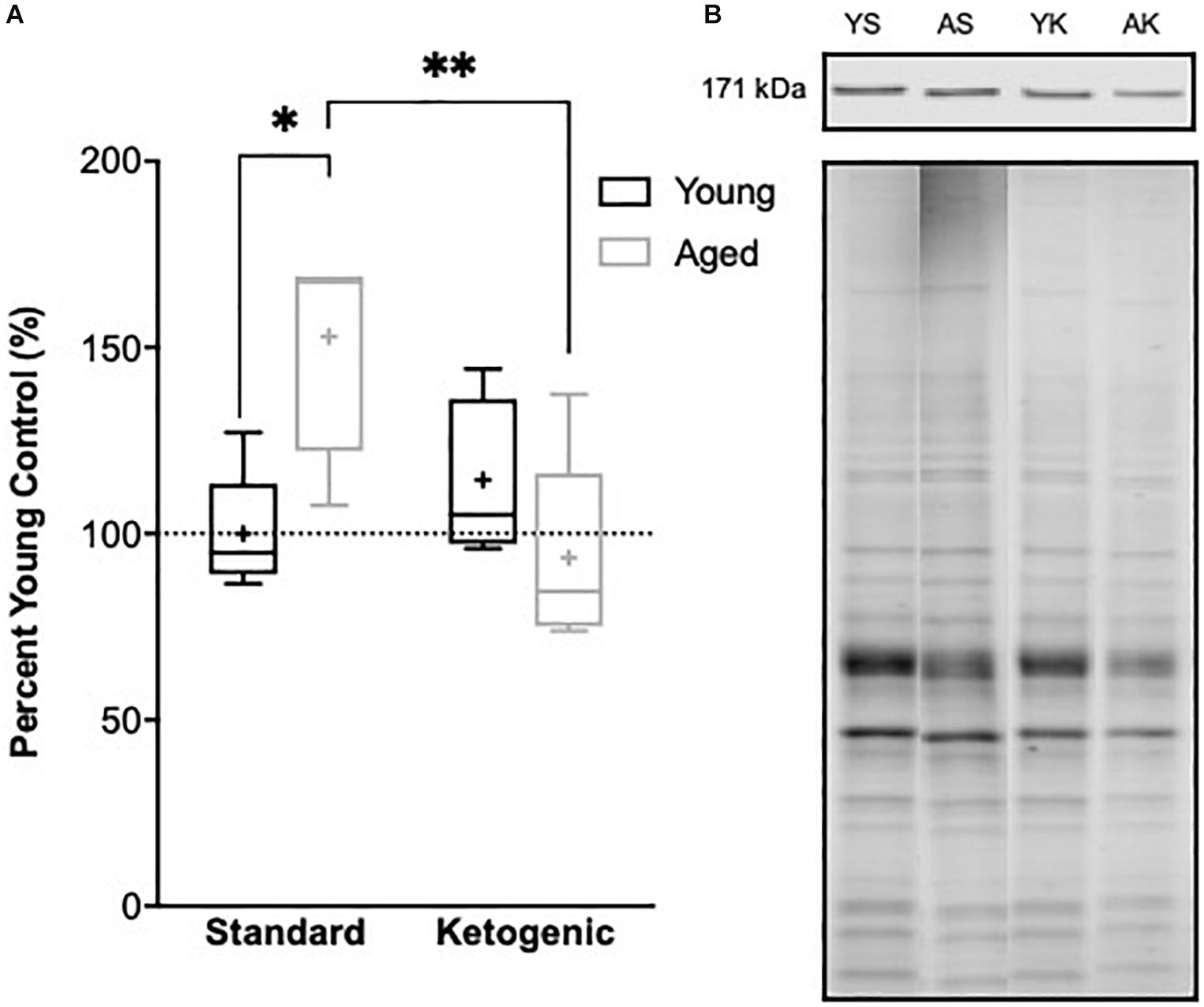
Figure 7. Hippocampal ROCK2 protein expression. (A) Aged rats demonstrated significantly higher levels of ROCK2 expression in the hippocampus relative to young standard-fed (SD) rats, but levels were restored to that of the young SD-fed rats in ketogenic-fed (KD) aged subjects. (B) Representative ROCK2 bands (top), which were then normalized to total protein stains (bottom) to account for variability in total protein loading. Note total protein was less in aged compared to young animals. Boxes represent the upper and lower quartiles around the median, the whiskers are the minimum and maximum observed values and + indicates the group mean. All values are expressed as percent of young SD-fed controls (dotted line). ∗ indicates a significant effect of age across standard-fed subjects and ∗∗ indicates a significant effect of diet across aged subjects.
Discussion
The goal of this study was to investigate the effects of age and diet on glutamatergic and GABAergic signaling-related gene expression from cell body-enriched tissue samples across subregions of the hippocampus. There was a significant reduction in transcript levels of several genes associated with pre- and post-synaptic signaling mechanisms within the dentate gyrus (DG) of ketogenic diet (KD)-fed rats relative to standard diet (SD)-fed rats irrespective of age. Notably, several gene targets reduced as a function of diet in the DG were also reduced in CA3 of aged rats relative to young rats irrespective of diet. Consistent with previous work, there were no effects of age on transcript levels detected within CA1 (Haberman et al., 2011; Tran et al., 2018; but see Zeier et al., 2011). The current findings build on this to show that CA1 synapse-related transcript levels were also not significantly altered by long-term dietary ketosis. Interestingly, a pathway enrichment analysis highlighted the age-related vulnerability of CA3 glutamatergic synapses in addition to a potential diet-dependent compensation within DG glutamatergic synapses (see Figure 8).
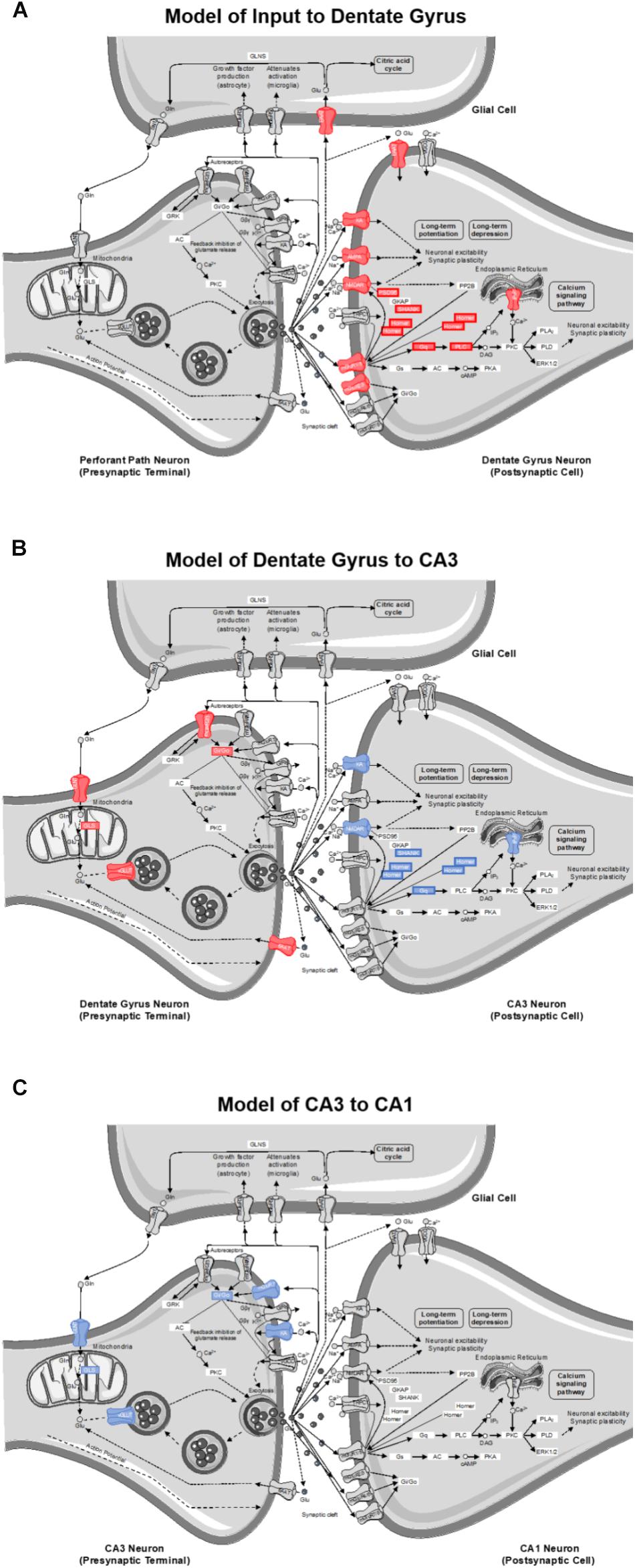
Figure 8. Model of gene contributions to significant ionotropic glutamate receptor (glutamatergic synapse; KEGG) pathway enrichment as determined by DAVID for (A) inputs to the DG, (B) projections from DG to CA3, and (C) projections from CA3 to CA1. Significant reductions in receptors/genes by diet (red) and age (blue) are indicated by color.
Reduction of Gene Transcript Levels by Ketogenic Diet Is Specific to Dentate Gyrus
Although the DG receives input from several cortical and subcortical regions, its primary excitatory input is from the perforant pathway emanating from layer II neurons of the medial and lateral entorhinal cortices and synapsing in the outer two-thirds of the molecular layer (Witter and Amaral, 2004). The inner molecular layer receives commissural and other non-entorhinal cortical inputs, including inhibitory basket cell projections (Sik et al., 1997). The tissue isolated for quantitative RNA analysis in the current study, however, primarily consisted of DG neuron cell bodies with a smaller contribution from hilar and non-neuronal cells. Thus, changes in gene expression largely reflect alterations within the DG granule cells densely packed into the principal cell layer, but may also be influenced to some extent by glutamatergic mossy cells (Amaral et al., 2007). Moreover, changes in synapse-related gene transcripts cannot be localized to a specific input layer of the dendritic field that will have variable distributions of inhibitory, excitatory and neuromodulatory input. That being said, transcript reductions in animals on the KD point to potential alterations in granule cell dendritic input (post-synaptic signaling), as well as altered granule cell axonal output from the mossy fibers (presynaptic transmission). Therefore, a pathway enrichment analysis was used to facilitate modeling the most reliable changes to DG dendritic input and DG axonal transmission. According to DAVID’s functional pathway enrichment analyses, the glutamatergic synapse pathway was the most reliably enriched. However, it should be noted that entire hippocampal subregions were utilized, and the influence of neuron subtype could not be delineated.
Of the 40 genes significantly affected by the KD in the DG, 21 genes contributed to this pathway enrichment. Among these genes, 13 had primarily postsynaptic functions (see Figure 8A), and therefore reflect changes on dendrites receiving input from perforant path axons or other afferents. Specifically, a number of contributors to this pathway enrichment included gene transcripts of postsynaptic proteins integral to neuronal excitability (e.g., ionotropic glutamate receptors), synaptic plasticity (e.g., scaffolding proteins), and second messenger signaling cascades (e.g., metabotropic glutamate receptors and downstream effectors). Together these data suggest that a KD may reduce glutamatergic signaling in granule cell dendrites. This has interesting implications for both aging and epilepsy. In advanced age, the excitatory postsynaptic field potential for a given amplitude of the presynaptic perforant path fiber potential is larger relative to young animals (Barnes and McNaughton, 1980), suggesting that excitatory synapses in aged rats are more powerful. Similarly, intracellular recording methods measuring unitary excitatory postsynaptic potential amplitude in young and aged rats, show that the depolarization resulting from stimulation of a single fiber is increased in old compared to young granule cells (Foster et al., 1991). Thus, it is conceivable that nutritional ketosis may reverse this age-related increase in synaptic strength. Relatedly, the KD is known to be effective at reducing seizure activity (Lefevre and Aronson, 2000; Baranano and Hartman, 2008). Epileptiform activity in medial temporal lobe epilepsy is generated by dentate gyrus granule cells (Bumanglag and Sloviter, 2018). The ketogenic diet may reduce excitatory drive onto granule cells to reset the balance between excitation and inhibition thereby conferring seizure projection. Consistent with the current data showing that the DG is particularly sensitive to a KD, a previous study observed an increased number and size of mitochondria within the DG region of the hippocampus in KD-fed rats (Bough et al., 2006). In this study, the authors hypothesized that increasing the quantity of key metabolites involved in oxidative phosphorylation like glutamate and phosphocreatine can enhance ATP production to fuel pumps on the cell membrane. These changes could therefore stabilize the membrane potential and increase resistance to metabolic stress. Using a gene microarray approach, this study also reported an increase in the transcription of genes involved in mitochondrial biogenesis, which the authors concluded might confer anticonvulsant properties within the hippocampus (Bough et al., 2006).
The KD reduced transcript levels of 8 genes that contribute to pathway enrichment of presynaptic proteins integral to glutamate metabolism (e.g., synthesis and packaging) and synaptic glutamate regulation (e.g., autoreceptors and transport) in DG. This observation has implications for how nutritional ketosis may alter mossy fiber axonal transmission to the stratum lucidum of CA3 (see Figure 8B). With age, there are neurophysiological alterations at the mossy fiber-CA3 synapse that include reduced inhibition (Villanueva-Castillo et al., 2017). It is conceivable that when consuming a KD, altered presynaptic glutamate metabolism may decrease excitatory drive to CA3 to ameliorate age-related hyperexcitability (Wilson, 2005; Thomé et al., 2015), but this idea needs to be empirically tested with functional measurements.
While our previous work has shown the diet can restore hippocampal levels of the vesicular glutamate transporter 1 (vGluT1; Hernandez et al., 2018b), the level of transcript encoding vGluT1 (Slc17a7) in the DG was lower in KD-fed relative to SD-fed rats. One possible explanation for the opposing directional changes between protein and transcript levels is that reduced vGluT1 protein was detected from whole-hippocampal homogenates and could have been driven by changes in other subregions. Another possible explanation reconciling contrasting changes between protein and transcript levels is that the KD increased the stability of vGlut1 protein, resulting in a reduced need for gene transcription. Indeed, reductions in levels of a gene transcript are not always translated to a loss of its protein product (Gygi et al., 1999; Greenbaum et al., 2003; Maier et al., 2009). Additional functional assessments of synaptic integrity will be necessary to differentiate between these contrasting possibilities. Importantly, our ROCK2 protein findings suggest a diet-induced increase in synaptic stability, and as such, KD-related reduced transcript levels in the DG may reflect synaptic stability and improved cognitive performance (Hernandez et al., 2018a).
Notably, the results presented here agree with a hippocampal cDNA microarray study on KD-fed rats showing diet-induced reductions in several genes associated with neuronal excitability that overlap with the current study (Noh et al., 2004). Although the null age effects in the DG are consistent with previous work showing CA3-specific changes in gene expression not observed in DG (Haberman et al., 2011), it must be noted that the SD-fed rats in the current study were fed under a meal-feeding schedule. This feeding regimen may have improved peripheral metabolism relative to ad libitum eating (Mitchell et al., 2016). While rodent consumption was not reduced to levels comparable to caloric restriction studies, meal feeding prevented overconsumption of food relative to ab libitum consumption. Previous studies showing age-related changes in the DG were conducted in ad libitum fed rats (Ianov et al., 2016), that may have been metabolically compromised due to excessive caloric intake (Martin et al., 2010). The DG is the subregion that is most vulnerable to glucose dysregulation. In fact, individuals with diabetes have reduced basal cerebral blood volume, an indirect measure of oxygen metabolism, in the DG compared to normal study participants. The magnitude of the decline is related to blood glucose levels. In contrast, basal cerebral blood volume in CA1 and CA3 is not affected by diabetes (Wu et al., 2008). It is likely that metabolic changes disproportionately affect the DG due to the high energy demands of adult neurogenesis (Beckervordersandforth, 2017). This cost is further driven by how densely packed the principal cell layer is and the sheer number of neurons within the DG (Amaral et al., 2007).
Reduction of Gene Expression With Advanced Age Is Specific to CA3
As the CA3 subregion of the hippocampus receives input from the DG, any functional changes within DG likely have direct downstream consequences on CA3. Similar to the DG, the RNA extracted from CA3 was from cell body-enriched samples, and therefore, transcript level changes observed within this subregion predict alterations in dendritic input primarily from DG (post-synaptic signaling) in addition to CA3 axonal output via Schafer collaterals projecting to CA1 (presynaptic transmission). Of the 24 genes that showed a significant reduction in transcript levels in aged compared to young rats, 12 of these genes contributed to glutamatergic synapse pathway enrichment. Specifically, four contribute to presynaptic glutamate regulation, four contribute to postsynaptic signaling transmission, and four contribute to synaptic plasticity (see Figure 8B). Age-related vulnerability of CA3 neurons is a well-documented phenomenon. The data presented here are consistent with alterations in CA3 excitability observed in humans (Yassa et al., 2010, 2011), monkeys (Thomé et al., 2015), and rats (Wilson, 2005; Robitsek et al., 2015; Maurer et al., 2017). Additionally, changes in gene expression specifically within CA3, and not DG, correlate with cognitive outcomes in aged rats (Haberman et al., 2011).
Interestingly, there were no diet-induced changes in gene expression within CA3 that survived the false discovery rate threshold. Prior work has shown that in whole hippocampal homogenates nutritional ketosis leads to increased levels of protein for the vesicular GABA transporter (vGAT; Hernandez et al., 2018a, b). This led to the hypothesis that the KD’s effectiveness at preventing epileptiform activity (Gasior et al., 2006; Hartman et al., 2007), reducing anxiety-like behavior and improving cognitive function (Hernandez et al., 2018a) was through increasing synaptic levels of GABA. Although the gene encoding vGaT (Slc32a1) was not quantified in the present study, diet-induced reductions in transcript levels of genes associated with glutamate synthesis, packaging, and synaptic clearance on presynaptic DG afferents (Figure 8B) suggest that the KD may reduce excitatory afferent drive to CA3, which could confer resilience in aging and epilepsy. This hypothesis needs to be tested with functional studies that directly measure the neurophysiological interactions between the DG and CA3. The complexity of age- and metabolism-related changes demonstrate the need for such studies and require a multifaceted approach beyond the scope of the excitatory and inhibitory gene panel used here.
Age-Related Decrease in Synaptic Stability Is Restored in Ketogenic Diet-Fed Aged Rats
In addition to gene expression, whole hippocampal homogenates were used to investigate protein expression of rho-associated coiled-coil containing protein kinase 2 (ROCK2). ROCK2 is an essential regulator of cytoskeletal elements with the ability to modify dendritic spine stability and number, and this protein has been shown to accumulate in the brains of patients with Alzheimer’s disease (Bobo-Jiménez et al., 2017). Excessive ROCK proteins lead to dendritic spine instability, and inhibition of ROCKs restore microtubules at the dendrites, leading to improved dendritic arborization (Li et al., 2000; Nakayama et al., 2000; Chen and Firestein, 2007; Bobo-Jiménez et al., 2017). Furthermore, ROCK inhibition in aged subjects improves cognitive performance on the hippocampal-dependent Morris water maze (Huentelman et al., 2009). We found aged rats fed the SD had significantly increased levels of ROCK2, suggesting decreased stability in axospinous synapses within the hippocampus of aged subjects. Aged rats fed a KD, however, had ROCK2 levels similar to young rats on both diets, demonstrating a restored ability to appropriately regulate dendritic morphology. These data demonstrate a KD, in conjunction with meal-feeding, exerts changes in neuronal signaling at the levels of both gene and protein expression.
Conclusion
Although there were no significant changes within CA1, there were alterations in genes encoding presynaptic proteins at CA3 Schafer collateral terminals synapsing onto CA1. Along these lines, the null diet effects on gene expression within CA3 does not mean that this subregion is functionally unaltered. Although the KD did not rescue an age-related decrease in CA3 transcript levels, the KD altered functionally related genes in the DG, which is upstream and sensitive to metabolic perturbations. Consequently, diet-induced decreases in DG transcript levels may be a compensatory mechanism leading to positive change that helps confer resilience in the face of age-related dysfunction in network dynamics between subregions of the hippocampus. This hypothesis needs to be directly tested with in vivo approaches to measure DG-CA3 interactions in aging.
Our study also did not detect age-related calcium dyshomeostasis within CA1, as previously described (Campbell et al., 1996; Bodhinathan et al., 2010; Zeier et al., 2011; Oh et al., 2013). We did not see an increase in ITPR1 gene expression, which is translated into the IP3 receptor protein. Notably, however, the transciption of other genes encoding proteins related to calcium regulation that are known to be affected by age were not evaluated in this panel, including voltage-gated L-type calcium channels and ryanodine receptors (Thibault and Landfield, 1996). Alternatively, others have documented age-related shifts in intracellular signaling cascades and their propensity to recruit IP3 receptor-gated calcium pools despite preserved expression of constituent signaling proteins (e.g., Nicolle et al., 1999; Kumar and Foster, 2007, 2014; McQuail et al., 2013).
Finally, differences between this study and previous reports could be due to implementing meal-feeding and prevention of overeating, which improves peripheral metabolism (Golbidi et al., 2017; Manoogian and Panda, 2017; Anton et al., 2018), potentially altering neurometabolism in aged rats regardless of diet. The majority of studies utilizing aged rodents have provided access to food ad libitum throughout the lifespan, resulting in metabolically impaired subjects (Martin et al., 2010). Thus, free feeding may exacerbate cognitive and neurobiological deficits, increasing the probability of detecting age effects. In fact, it is well documented that excessive energy consumption can lead to cognitive impairments (Stranahan et al., 2008; Kanoski and Davidson, 2011; Bocarsly et al., 2015). Therefore, it is imperative that investigations into the effects of cognitive interventions account for this often overlooked, yet vital component of animal maintenance through the implementation of proper feeding strategies, or at the very least consider rodent metabolic health as a variable in aging studies providing food ad libitum.
Data Availability
The datasets generated for this study can be found in the https://doi.org/10.6084/m9.figshare.9466577.v1.
Ethics Statement
The animal study was reviewed and approved by University of Florida Institutional Animal Care and Use Committee.
Author Contributions
AH and CH designed the study, contributed to data acquisition, analysis and interpretation, and prepared the manuscript. LT and KC contributed to data acquisition and analysis. JM, JB, and SB designed the study, contributed to data analysis and interpretation, and prepared the manuscript.
Funding
This research was funded by NIA R01AG060977 (SB), NIA F31AG058455 (AH), NIA K01AG061263 (JM), Pat Tillman Foundation (CH), McKnight Brain Research Foundation (AH, CH, JM, JB, SB), Claude D. Pepper Older Americans Independence Center Scholar Award P30 AG028740 (JM, SB), University of Florida University Scholars Program (LT, KC).
Conflict of Interest Statement
The authors declare that the research was conducted in the absence of any commercial or financial relationships that could be construed as a potential conflict of interest.
Supplementary Material
The Supplementary Material for this article can be found online at: https://www.frontiersin.org/articles/10.3389/fnagi.2019.00239/full#supplementary-material
Footnotes
References
Amaral, D. G., Scharfman, H. E., and Lavenex, P. (2007). The dentate gyrus: fundamental neuroanatomical organization (dentate gyrus for dummies). Prog. Brain Res. 163, 3–22. doi: 10.1016/S0079-6123(07)63001-5
Anton, S. D., Moehl, K., Donahoo, W. T., Marosi, K., Lee, S. A., Mainous, A. G., et al. (2018). Flipping the metabolic switch: understanding and applying the health benefits of fasting. Obesity 26, 254–268. doi: 10.1002/oby.22065
Attwell, D., and Laughlin, S. B. (2001). An energy budget for signaling in the grey matter of the brain. J. Cereb. Blood Flow Metab. 21, 1133–1145. doi: 10.1097/00004647-200110000-1
Balietti, M., Giorgetti, B., Fattoretti, P., Grossi, Y., Di Stefano, G., Casoli, T., et al. (2008). Ketogenic diets cause opposing changes in synaptic morphology in CA1 hippocampus and dentate gyrus of late-adult rats. Rejuvenation Res. 11, 631–640. doi: 10.1089/rej.2007.0650
Baranano, K., and Hartman, A. L. (2008). The ketogenic diet: uses in epilepsy and other neurologic diseases. Curr. Treat. Options Neurol. 10, 410–419. doi: 10.1007/s11940-008-0043-8
Barnes, C. A. (2003). Long-term potentiation and the ageing brain. Philos. Trans. R. Soc. Lond. B. Biol. Sci. 358, 765–772. doi: 10.1098/rstb.2002.1244
Barnes, C. A., and McNaughton, B. L. (1980). Physiological compensation for loss of afferent synapses in rat hippocampal granule cells during senescence. J. Physiol. 309, 473–485. doi: 10.1113/jphysiol.1980.sp013521
Barnes, C. A., Rao, G., Foster, T. C., and McNaughton, B. L. (1992). Region-specific age effects on AMPA sensitivity: electrophysiological evidence for loss of synaptic contacts in hippocampal field CA1. Hippocampus 2, 457–468. doi: 10.1002/hipo.450020413
Beckervordersandforth, R. (2017). Mitochondrial metabolism-mediated regulation of adult neurogenesis. Brain Plast. 3, 73–87. doi: 10.3233/BPL-170044
Benjamini, Y., and Hochberg, Y. (1995). Controlling the false discovery rate: a practical and powerful approach to multiple testing. J. R. Stat. Soc. Ser. B Methodol. 57, 289–300. doi: 10.1111/j.2517-6161.1995.tb02031.x
Blalock, E. M., Chen, K.-C., Sharrow, K., Herman, J. P., Porter, N. M., Foster, T. C., et al. (2003). Gene microarrays in hippocampal aging: statistical profiling identifies novel processes correlated with cognitive impairment. J. Neurosci. 23, 3807–3819. doi: 10.1523/jneurosci.23-09-03807.2003
Bobo-Jiménez, V., Delgado-Esteban, M., Angibaud, J., Sánchez-Morán, I., de la Fuente, A., Yajeya, J., et al. (2017). APC/C Cdh1 -Rock2 pathway controls dendritic integrity and memory. Proc. Natl. Acad. Sci. U.S.A. 114, 4513–4518. doi: 10.1073/pnas.1616024114
Bocarsly, M. E., Fasolino, M., Kane, G. A., LaMarca, E. A., Kirschen, G. W., Karatsoreos, I. N., et al. (2015). Obesity diminishes synaptic markers, alters microglial morphology, and impairs cognitive function. Proc. Natl. Acad. Sci. U.S.A. 112, 15731–15736. doi: 10.1073/pnas.1511593112
Bodhinathan, K., Kumar, A., and Foster, T. C. (2010). Redox sensitive calcium stores underlie enhanced after hyperpolarization of aged neurons: role for ryanodine receptor mediated calcium signaling. J. Neurophysiol. 104, 2586–2593. doi: 10.1152/jn.00577.2010
Bough, K. J., Wetherington, J., Hassel, B., Pare, J. F., Gawryluk, J. W., Greene, J. G., et al. (2006). Mitochondrial biogenesis in the anticonvulsant mechanism of the ketogenic diet. Ann. Neurol. 60, 223–235. doi: 10.1002/ana.20899
Bumanglag, A. V., and Sloviter, R. S. (2018). No latency to dentate granule cell epileptogenesis in experimental temporal lobe epilepsy with hippocampal sclerosis. Epilepsia 59, 2019–2034. doi: 10.1111/epi.14580
Burke, S. N., and Barnes, C. A. (2006). Neural plasticity in the ageing brain. Nat. Rev. Neurosci. 7, 30–40. doi: 10.1038/nrn1809
Cadacio, C. L., Milner, T. A., Gallagher, M., Pierce, J. P., and Cadiacio, C. L. (2003). Hilar neuropeptide Y interneuron loss in the aged rat hippocampal formation. Exp. Neurol. 183, 147–158. doi: 10.1016/s0014-4886(03)00126-2
Campbell, L. W., Hao, S.-Y., Thibault, O., Blalock, E. M., and Landfield, P. W. (1996). Aging changes in voltage-gated calcium currents in hippocampal CA1 neurons. J. Neurosci. 16, 6286–6295. doi: 10.1523/JNEUROSCI.16-19-06286.1996
Castellano, C.-A., Nugent, S., Paquet, N., Tremblay, S., Bocti, C., Lacombe, G., et al. (2015). Lower brain 18F-fluorodeoxyglucose uptake but normal 11C-acetoacetate metabolism in mild Alzheimer’s disease dementia. J. Alzheimers Dis. 43, 1343–1353. doi: 10.3233/JAD-141074
Chen, E. Y., Tan, C. M., Kou, Y., Duan, Q., Wang, Z., Meirelles, G. V., et al. (2013). Enrichr: interactive and collaborative HTML5 gene list enrichment analysis tool. BMC Bioinformatics 14:128. doi: 10.1186/1471-2105-14-128
Chen, H., and Firestein, B. L. (2007). RhoA regulates dendrite branching in hippocampal neurons by decreasing cypin protein levels. J. Neurosci. 27, 8378–8386. doi: 10.1523/JNEUROSCI.0872-07.2007
Cowley, M., Skaar, D. A., Jima, D. D., Maguire, R. L., Hudson, K. M., Park, S. S., et al. (2018). Effects of cadmium exposure on DNA methylation at imprinting control regions and genome-wide in mothers and newborn children. Environ. Health Perspect. 126:037003. doi: 10.1289/EHP2085
Cunnane, S. C., Courchesne-Loyer, A., St-Pierre, V., Vandenberghe, C., Pierotti, T., Fortier, M., et al. (2016). Can ketones compensate for deteriorating brain glucose uptake during aging? Implications for the risk and treatment of Alzheimer’s disease. Ann. N. Y. Acad. Sci. 1367, 12–20. doi: 10.1111/nyas.12999
Foster, T. C., Barnes, C. A., Rao, G., and McNaughton, B. L. (1991). Increase in perforant path quantal size in aged F-344 rats. Neurobiol. Aging 12, 441–448. doi: 10.1016/0197-4580(91)90071-Q
Freeman, J. M., Vining, E. P., Pillas, D. J., Pyzik, P. L., Casey, J. C., and Kelly, L. M. (1998). The efficacy of the ketogenic diet-1998: a prospective evaluation of intervention in 150 children. Pediatrics 102, 1358–1363. doi: 10.1542/peds.102.6.1358
Gage, F. H., Kelly, P. A., and Björklund, A. (1984). Regional changes in brain glucose metabolism reflect cognitive impairments in aged rats. J. Neurosci. 4, 2856–2865. doi: 10.1523/jneurosci.04-11-02856.1984
Gasior, M., Rogawski, M. A., and Hartman, A. L. (2006). Neuroprotective and disease-modifying effects of the ketogenic diet. Behav. Pharmacol. 17, 431–439. doi: 10.1097/00008877-200609000-00009
Geinisman, Y., de Toledo-Morrell, L., and Morrell, F. (1986). Loss of perforated synapses in the dentate gyrus: morphological substrate of memory deficit in aged rats. Proc. Natl. Acad. Sci. U.S.A. 83, 3027–3031. doi: 10.1073/pnas.83.9.3027
Geinisman, Y., de Toledo-Morrell, L., Morrell, F., Persina, I. S., and Rossi, M. (1992). Age-related loss of axospinous synapses formed by two afferent systems in the rat dentate gyrus as revealed by the unbiased stereological dissector technique. Hippocampus 2, 437–444. doi: 10.1002/hipo.450020411
Geinisman, Y., Ganeshina, O., Yoshida, R., Berry, R. W., Disterhoft, J. F., and Gallagher, M. (2004). Aging, spatial learning, and total synapse number in the rat CA1 stratum radiatum. Neurobiol. Aging 25, 407–416. doi: 10.1016/j.neurobiolaging.2003.12.001
Golbidi, S., Daiber, A., Korac, B., Li, H., Essop, M. F., and Laher, I. (2017). Health benefits of fasting and caloric restriction. Curr. Diab. Rep. 17:123. doi: 10.1007/s11892-017-0951-7
Goyal, M. S., Vlassenko, A. G., Blazey, T. M., Su, Y., Couture, L. E., Durbin, T. J., et al. (2017). Loss of brain aerobic glycolysis in normal human aging. Cell Metab. 26:353-360.e3. doi: 10.1016/j.cmet.2017.07.010
Greenbaum, D., Colangelo, C., Williams, K., and Gerstein, M. (2003). Comparing protein abundance and mRNA expression levels on a genomic scale. Genome Biol. 4:117. doi: 10.1186/gb-2003-4-9-117
Gygi, S. P., Rochon, Y., Franza, B. R., and Aebersold, R. (1999). Correlation between protein and mRNA abundance in yeast. Mol. Cell. Biol. 19, 1720–1730. doi: 10.1128/mcb.19.3.1720
Haberman, R. P., Colantuoni, C., Koh, M. T., and Gallagher, M. (2013). Behaviorally activated mrna expression profiles produce signatures of learning and enhanced inhibition in aged rats with preserved memory. PLoS One 8:e83674. doi: 10.1371/journal.pone.0083674
Haberman, R. P., Colantuoni, C., Stocker, A. M., Schmidt, A. C., Pedersen, J. T., and Gallagher, M. (2011). Prominent hippocampal CA3 gene expression profile in neurocognitive aging. Neurobiol. Aging 32, 1678–1692. doi: 10.1016/j.neurobiolaging.2009.10.005
Hartman, A. L., Gasior, M., Vining, E. P. G., and Rogawski, M. A. (2007). The neuropharmacology of the ketogenic diet. Pediatr. Neurol. 36, 281–292. doi: 10.1016/j.pediatrneurol.2007.02.008
Henderson, B. W., Gentry, E. G., Rush, T., Troncoso, J. C., Thambisetty, M., Montine, T. J., et al. (2016). Rho-associated protein kinase 1 (ROCK1) is increased in Alzheimer’s disease and ROCK1 depletion reduces amyloid-β levels in brain. J. Neurochem. 138, 525–531. doi: 10.1111/jnc.13688
Hernandez, A. R., Hernandez, C. M., Campos, K. T., Truckenbrod, L. M., Sakarya, Y., McQuail, J. A., et al. (2018b). The antiepileptic ketogenic diet alters hippocampal transporter levels and reduces adiposity in aged rats. J. Gerontol. Ser. A 73, 450–458. doi: 10.1093/gerona/glx193
Hernandez, A. R., Hernandez, C. M., Campos, K., Truckenbrod, L., Federico, Q., Moon, B., et al. (2018a). A ketogenic diet improves cognition and has biochemical effects in prefrontal cortex that are dissociable from hippocampus. Front. Aging Neurosci. 10:391. doi: 10.3389/fnagi.2018.00391
Hernandez, C. M., McQuail, J. A., Schwabe, M. R., Burke, S. N., Setlow, B., and Bizon, J. L. (2018c). Age-Related declines in prefrontal cortical expression of metabotropic glutamate receptors that support working memory. eNeuro 5:ENEURO.164-18. doi: 10.1523/ENEURO.0164-18.2018
Howarth, C., Gleeson, P., and Attwell, D. (2012). Updated energy budgets for neural computation in the neocortex and cerebellum. J. Cereb. Blood Flow Metab. 32, 1222–1232. doi: 10.1038/jcbfm.2012.35
Huang, D. W., Sherman, B. T., and Lempicki, R. A. (2009a). Bioinformatics enrichment tools: paths toward the comprehensive functional analysis of large gene lists. Nucleic Acids Res. 37, 1–13. doi: 10.1093/nar/gkn923
Huang, D. W., Sherman, B. T., and Lempicki, R. A. (2009b). Systematic and integrative analysis of large gene lists using DAVID bioinformatics resources. Nat. Protoc. 4, 44–57. doi: 10.1038/nprot.2008.211
Huentelman, M. J., Stephan, D. A., Talboom, J., Corneveaux, J. J., Reiman, D. M., Gerber, J. D., et al. (2009). Peripheral delivery of a ROCK inhibitor improves learning and working memory. Behav. Neurosci. 123, 218–223. doi: 10.1037/a0014260
Ianov, L., Rani, A., Beas, B. S., Kumar, A., and Foster, T. C. (2016). Transcription profile of aging and cognition-related genes in the medial prefrontal cortex. Front. Aging Neurosci. 8:113. doi: 10.3389/fnagi.2016.00113
Kanoski, S. E., and Davidson, T. L. (2011). Western diet consumption and cognitive impairment: links to hippocampal dysfunction and obesity. Physiol. Behav. 103, 59–68. doi: 10.1016/j.physbeh.2010.12.003
Keuker, J. I. H., de Biurrun, G., Luiten, P. G. M., and Fuchs, E. (2004). Preservation of hippocampal neuron numbers and hippocampal subfield volumes in behaviorally characterized aged tree shrews. J. Comp. Neurol. 468, 509–517. doi: 10.1002/cne.10996
Koh, M. T., Spiegel, A. M., and Gallagher, M. (2014). Age-associated changes in hippocampal-dependent cognition in diversity outbred mice. Hippocampus 24, 1300–1307. doi: 10.1002/hipo.22311
Kubo, T., Yamaguchi, A., Iwata, N., and Yamashita, T. (2008). The therapeutic effects of Rho-ROCK inhibitors on CNS disorders. Ther. Clin. Risk Manag. 4, 605–615. doi: 10.2147/tcrm.s2907
Kuleshov, M. V., Jones, M. R., Rouillard, A. D., Fernandez, N. F., Duan, Q., Wang, Z., et al. (2016). Enrichr: a comprehensive gene set enrichment analysis web server 2016 update. Nucleic Acids Res. 44, W90–W97. doi: 10.1093/nar/gkw377
Kumar, A., and Foster, T. C. (2007). “Neurophysiology of old neurons and synapses,” in Brain Aging: Models, Methods, and Mechanisms, ed. D. R. Riddle (Boca Raton (FL): CRC Press).
Kumar, A., and Foster, T. C. (2014). Interaction of DHPG-LTD and synaptic-LTD at senescent CA3-CA1 hippocampal synapses. Hippocampus 24, 466–475. doi: 10.1002/hipo.22240
Landfield, P. W., Pitler, T. A., and Applegate, M. D. (1986). The effects of high Mg2+-to-Ca2+ ratios on frequency potentiation in hippocampal slices of young and aged rats. J. Neurophysiol. 56, 797–811. doi: 10.1152/jn.1986.56.3.797
Lefevre, F., and Aronson, N. (2000). Ketogenic diet for the treatment of refractory epilepsy in children: a systematic review of efficacy. Pediatrics 105:E46.
Li, Z., Van Aelst, L., and Cline, H. T. (2000). Rho GTPases regulate distinct aspects of dendritic arbor growth in Xenopus central neurons in vivo. Nat. Neurosci. 3, 217–225. doi: 10.1038/72920
Lying-Tunell, U., Lindblad, B. S., Malmlund, H. O., and Persson, B. (1981). Cerebral blood flow and metabolic rate of oxygen, glucose, lactate, pyruvate, ketone bodies and amino acids. Acta Neurol. Scand. 63, 337–350. doi: 10.1111/j.1600-0404.1981.tb00788.x
Maier, T., Güell, M., and Serrano, L. (2009). Correlation of mRNA and protein in complex biological samples. FEBS Lett. 583, 3966–3973. doi: 10.1016/j.febslet.2009.10.036
Manoogian, E. N. C., and Panda, S. (2017). Circadian rhythms, time-restricted feeding, and healthy aging. Ageing Res. Rev. 39, 59–67. doi: 10.1016/j.arr.2016.12.006
Martin, B., Ji, S., Maudsley, S., and Mattson, M. P. (2010). “Control” laboratory rodents are metabolically morbid: why it matters. Proc. Natl. Acad. Sci. U.S.A. 107, 6127–6133. doi: 10.1073/pnas.0912955107
Maurer, A. P., Johnson, S. A., Hernandez, A. R., Reasor, J., Cossio, D. M., Fertal, K. E., et al. (2017). Age-related changes in lateral entorhinal and CA3 neuron allocation predict poor performance on object discrimination. Front. Syst. Neurosci. 11:49. doi: 10.3389/fnsys.2017.00049
McKinney, R. A. (2010). Excitatory amino acid involvement in dendritic spine formation, maintenance and remodelling. J. Physiol. 588, 107–116. doi: 10.1113/jphysiol.2009.178905
McQuail, J. A., Bañuelos, C., LaSarge, C. L., Nicolle, M. M., and Bizon, J. L. (2012). GABAB receptor GTP-binding is decreased in the prefrontal cortex but not the hippocampus of aged rats. Neurobiol. Aging 33, 1124.e1–1124.e12. doi: 10.1016/j.neurobiolaging.2011.11.011
McQuail, J. A., Davis, K. N., Miller, F., Hampson, R. E., Deadwyler, S. A., Howlett, A. C., et al. (2013). Hippocampal Gαq/11 but not Gαo-coupled receptors are altered in aging. Neuropharmacology 70, 63–73. doi: 10.1016/j.neuropharm.2013.01.009
Meidenbauer, J. J., Mukherjee, P., and Seyfried, T. N. (2015). The glucose ketone index calculator: a simple tool to monitor therapeutic efficacy for metabolic management of brain cancer. Nutr. Metab. 12:12. doi: 10.1186/s12986-015-0009-2
Merrill, D. A., Chiba, A. A., and Tuszynski, M. H. (2001). Conservation of neuronal number and size in the entorhinal cortex of behaviorally characterized aged rats. J. Comp. Neurol. 438, 445–456. doi: 10.1002/cne.1327
Mitchell, S. J., Madrigal-Matute, J., Scheibye-Knudsen, M., Fang, E., Aon, M., González-Reyes, J. A., et al. (2016). Effects of sex, strain, and energy intake on hallmarks of aging in mice. Cell Metab. 23, 1093–1112. doi: 10.1016/j.cmet.2016.05.027
Mohammed, H. A., and Santer, R. M. (2001). Total neuronal numbers of rat lumbosacral primary afferent neurons do not change with age. Neurosci. Lett. 304, 149–152. doi: 10.1016/s0304-3940(01)01781-5
Morrison, J. H., and Baxter, M. G. (2012). The ageing cortical synapse: hallmarks and implications for cognitive decline. Nat. Rev. Neurosci. 13, 240–250. doi: 10.1038/nrn3200
Nakayama, A. Y., Harms, M. B., and Luo, L. (2000). Small GTPases rac and rho in the maintenance of dendritic spines and branches in hippocampal pyramidal neurons. J. Neurosci. 20, 5329–5338. doi: 10.1523/jneurosci.20-14-05329.2000
Nicholson, D. A., Yoshida, R., Berry, R. W., Gallagher, M., and Geinisman, Y. (2004). Reduction in size of perforated postsynaptic densities in hippocampal axospinous synapses and age-related spatial learning impairments. J. Neurosci. 24, 7648–7653. doi: 10.1523/JNEUROSCI.1725-04.2004
Nicolle, M. M., Colombo, P. J., Gallagher, M., and McKinney, M. (1999). Metabotropic glutamate receptor-mediated hippocampal phosphoinositide turnover is blunted in spatial learning-impaired aged rats. J. Neurosci. 19, 9604–9610. doi: 10.1523/JNEUROSCI.19-21-09604.1999
Noh, H. S., Lee, H. P., Kim, D. W., Kang, S. S., Cho, G. J., Rho, J. M., et al. (2004). A cDNA microarray analysis of gene expression profiles in rat hippocampus following a ketogenic diet. Brain Res. Mol. Brain Res. 129, 80–87. doi: 10.1016/j.molbrainres.2004.06.020
Ogawa, M., Fukuyama, H., Ouchi, Y., Yamauchi, H., and Kimura, J. (1996). Altered energy metabolism in Alzheimer’s disease. J. Neurol. Sci. 139, 78–82. doi: 10.1016/0022-510X(96)00033-0
Oh, M. M., Oliveira, F. A., Waters, J., and Disterhoft, J. F. (2013). Altered calcium metabolism in aging CA1 hippocampal pyramidal neurons. J. Neurosci. 33, 7905–7911. doi: 10.1523/JNEUROSCI.5457-12.2013
Pannese, E. (2011). Morphological changes in nerve cells during normal aging. Brain Struct. Funct. 216, 85–89. doi: 10.1007/s00429-011-0308-y
Rapp, P. R., Deroche, P. S., Mao, Y., and Burwell, R. D. (2002). Neuron number in the parahippocampal region is preserved in aged rats with spatial learning deficits. Cereb. Cortex 12, 1171–1179. doi: 10.1093/cercor/12.11.1171
Rapp, P. R., and Gallagher, M. (1996). Preserved neuron number in the hippocampus of aged rats with spatial learning deficits. Proc. Natl. Acad. Sci. U.S.A. 93, 9926–9930. doi: 10.1073/pnas.93.18.9926
Rasgon, N. L., Silverman, D., Siddarth, P., Miller, K., Ercoli, L. M., Elman, S., et al. (2005). Estrogen use and brain metabolic change in postmenopausal women. Neurobiol. Aging 26, 229–235. doi: 10.1016/j.neurobiolaging.2004.03.003
Robitsek, J., Ratner, M. H., Stewart, T., Eichenbaum, H., and Farb, D. H. (2015). Combined administration of levetiracetam and valproic acid attenuates age-related hyperactivity of CA3 place cells, reduces place field area, and increases spatial information content in aged rat hippocampus: improving hippocampal activity in aging. Hippocampus 25, 1541–1555. doi: 10.1002/hipo.22474
Rosenzweig, E. S., and Barnes, C. A. (2003). Impact of aging on hippocampal function: plasticity, network dynamics, and cognition. Prog. Neurobiol. 69, 143–179. doi: 10.1016/S0301-0082(02)00126-0
Sik, A., Penttonen, M., and Buzsáki, G. (1997). Interneurons in the hippocampal dentate gyrus: an in vivo intracellular study. Eur. J. Neurosci. 9, 573–588. doi: 10.1111/j.1460-9568.1997.tb01634.x
Simkin, D., Hattori, S., Ybarra, N., Musial, T. F., Buss, E. W., Richter, H., et al. (2015). Aging-related hyperexcitability in CA3 pyramidal neurons is mediated by enhanced A-type K+ channel function and expression. J. Neurosci. 35, 13206–13218. doi: 10.1523/JNEUROSCI.0193-15.2015
Smith, T. D., Adams, M. M., Gallagher, M., Morrison, J. H., and Rapp, P. R. (2000). Circuit-specific alterations in hippocampal synaptophysin immunoreactivity predict spatial learning impairment in aged rats. J. Neurosci. 20, 6587–6593. doi: 10.1523/jneurosci.20-17-06587.2000
Spiegel, A. M., Koh, M. T., Vogt, N. M., Rapp, P. R., and Gallagher, M. (2013). Hilar interneuron vulnerability distinguishes aged rats with memory impairment: hippocampal interneuron integrity in aging. J. Comp. Neurol. 521, 3508–3523. doi: 10.1002/cne.23367
Storey, J. D., and Tibshirani, R. (2003). Statistical significance for genomewide studies. Proc. Natl. Acad. Sci. U.S.A. 100, 9440–9445. doi: 10.1073/pnas.1530509100
Stranahan, A. M., Norman, E. D., Lee, K., Cutler, R. G., Telljohann, R. S., Egan, J. M., et al. (2008). Diet-induced insulin resistance impairs hippocampal synaptic plasticity and cognition in middle-aged rats. Hippocampus 18, 1085–1088. doi: 10.1002/hipo.20470
Swanger, S. A., Mattheyses, A. L., Gentry, E. G., and Herskowitz, J. H. (2016). ROCK1 and ROCK2 inhibition alters dendritic spine morphology in hippocampal neurons. Cell. Logist. 5:e1133266. doi: 10.1080/21592799.2015.1133266
Thibault, O., and Landfield, P. W. (1996). Increase in single L-type calcium channels in hippocampal neurons during aging. Science 272, 1017–1020. doi: 10.1126/science.272.5264.1017
Thomé, A., Gray, D. T., Erickson, C. A., Lipa, P., and Barnes, C. A. (2015). Memory impairment in aged primates is associated with region-specific network dysfunction. Mol. Psychiatry 21, 1257–1262. doi: 10.1038/mp.2015.160
Thomé, A., Gray, D. T., Erickson, C. A., Lipa, P., and Barnes, C. A. (2016). Memory impairment in aged primates is associated with region-specific network dysfunction. Mol. Psychiatry. 21, 1257–1262. doi: 10.1038/mp.2015.160
Tran, T., Gallagher, M., and Kirkwood, A. (2018). Enhanced postsynaptic inhibitory strength in hippocampal principal cells in high-performing aged rats. Neurobiol. Aging 70, 92–101. doi: 10.1016/j.neurobiolaging.2018.06.008
Villanueva-Castillo, C., Tecuatl, C., Herrera-López, G., and Galván, E. J. (2017). Aging-related impairments of hippocampal mossy fibers synapses on CA3 pyramidal cells. Neurobiol. Aging 49, 119–137. doi: 10.1016/j.neurobiolaging.2016.09.010
Wilson, I. A. (2005). Age-associated alterations of hippocampal place cells are subregion specific. J. Neurosci. 25, 6877–6886. doi: 10.1523/JNEUROSCI.1744-05.2005
Witter, M. P., and Amaral, D. G. (2004). “Chapter 21 - hippocampal formation,” in The Rat Nervous System (Third Edition), ed. G. Paxinos (Burlington: Academic Press), 635–704. doi: 10.1016/B978-012547638-6/50022-5
Wu, W., Brickman, A. M., Luchsinger, J., Ferrazzano, P., Pichiule, P., Yoshita, M., et al. (2008). The brain in the age of old: the hippocampal formation is targeted differentially by diseases of late life. Ann. Neurol. 64, 698–706. doi: 10.1002/ana.21557
Yassa, M. A., Mattfeld, A. T., Stark, S. M., and Stark, C. E. L. (2011). Age-related memory deficits linked to circuit-specific disruptions in the hippocampus. Proc. Natl. Acad. Sci. U.S.A. 108, 8873–8878. doi: 10.1073/pnas.1101567108
Yassa, M. A., Muftuler, L. T., and Stark, C. E. L. (2010). Ultrahigh-resolution microstructural diffusion tensor imaging reveals perforant path degradation in aged humans in vivo. Proc. Natl. Acad. Sci. U.S.A. 107, 12687–12691. doi: 10.1073/pnas.1002113107
Keywords: CA3, dentate gyrus, CA1, metabolism, glutamate, GABA, ROCK
Citation: Hernandez AR, Hernandez CM, Truckenbrod LM, Campos KT, McQuail JA, Bizon JL and Burke SN (2019) Age and Ketogenic Diet Have Dissociable Effects on Synapse-Related Gene Expression Between Hippocampal Subregions. Front. Aging Neurosci. 11:239. doi: 10.3389/fnagi.2019.00239
Received: 29 May 2019; Accepted: 19 August 2019;
Published: 13 September 2019.
Edited by:
Tao Ma, Wake Forest School of Medicine, United StatesReviewed by:
David Ruskin, Trinity College, United StatesChase Matthew Carver, The University of Texas Health Science Center at San Antonio, United States
Copyright © 2019 Hernandez, Hernandez, Truckenbrod, Campos, McQuail, Bizon and Burke. This is an open-access article distributed under the terms of the Creative Commons Attribution License (CC BY). The use, distribution or reproduction in other forums is permitted, provided the original author(s) and the copyright owner(s) are credited and that the original publication in this journal is cited, in accordance with accepted academic practice. No use, distribution or reproduction is permitted which does not comply with these terms.
*Correspondence: Sara N. Burke, QnVya2VzQHVmbC5lZHU=
†These authors have contributed equally to this work