- 1Department of Bio-Medical Sciences, Center for Chronic Disorders of Aging, Philadelphia College of Osteopathic Medicine, Philadelphia, PA, United States
- 2Department of Biochemistry, Immunology and Microbiology, Wayne State University School of Medicine, Detroit, MI, United States
The disease known as late-onset Alzheimer’s disease is a neurodegenerative condition recognized as the single most commonform of senile dementia. The condition is sporadic and has been attributed to neuronal damage and loss, both of which have been linked to the accumulation of protein deposits in the brain. Significant progress has been made over the past two decades regarding our overall understanding of the apparently pathogenic entities that arise in the affected brain, both for early-onset disease, which constitutes approximately 5% of all cases, as well as late-onset disease, which constitutes the remainder of cases. Observable neuropathology includes: neurofibrillary tangles, neuropil threads, neuritic senile plaques and often deposits of amyloid around the cerebrovasculature. Although many studies have provided a relatively detailed knowledge of these putatively pathogenic entities, understanding of the events that initiate and support the biological processes generating them and the subsequent observable neuropathology and neurodegeneration remain limited. This is especially true in the case of late-onset disease. Although early-onset Alzheimer’s disease has been shown conclusively to have genetic roots, the detailed etiologic initiation of late-onset disease without such genetic origins has remained elusive. Over the last 15 years, current and ongoing work has implicated infection in the etiology and pathogenesis of late-onset dementia. Infectious agents reported to be associated with disease initiation are various, including several viruses and pathogenic bacterial species. We have reported extensively regarding an association between late-onset disease and infection with the intracellular bacterial pathogen Chlamydia pneumoniae. In this article, we review previously published data and recent results that support involvement of this unusual respiratory pathogen in disease induction and development. We further suggest several areas for future research that should elucidate details relating to those processes, and we argue for a change in the designation of the disease based on increased understanding of its clinical attributes.
Introduction
A longstanding idea in the medical literature is that a wide variety of chronic diseases could be caused or exacerbated by a microbial infection. For example, in the early 20th century rheumatoid arthritis was considered to be an infectious disease, an explanation that was more or less abandoned by mid-century but which has re-emerged (Lansbury, 1950; Ford, 1963; Albert, 2000; Carty et al., 2004). Multiple sclerosis long has been attributed at least in part to involvement of an infectious component, although the identity of the specific agent(s) remain(s) to be firmly established (Swanborg et al., 2003). Similar arguments have been made in regard to other chronic diseases of an idiopathic nature, but in most cases, an infectious etiology and/or involvement of microbes in disease exacerbation have been difficult to establish. The pattern is paralleled in cancer etiology, in which infectious causation of some cancers has been demonstrated (for review see de Martel et al., 2012); however, most cancers are not thought to have an infectious etiology.
Instead of viral, bacterial, or mycological involvement in disease causation, alternative mechanisms that might explain chronic disease genesis have been pursued. Among the most prominent explanations has been the potential genetic basis of chronic clinical entities, including what is termed late-onset Alzheimer’s disease. Most of these studies have indicated that development of disease is not easily attributable to one or even a few mutations or gene polymorphisms. Rather, such studies have indicated that disease genesis is multifactorial, resulting from as yet unknown environmental and host genetic factors (Harney and Wordsworth, 2002; O’Connor et al., 2006).
With regard to Alzheimer’s disease, the single idea that has predominated for almost three decades in studies of the neuropathology underlying this clinical entity is the “amyloid cascade” hypothesis. The hypothesis proposes that the deposition of the amyloid-β (Aβ) peptide is the critical event underlying neuronal degeneration, and thus cognitive dysfunction (Schellenberg, 1995). This idea is appropriately applicable to the early form of dementia, familial Alzheimer’s disease, since it is well established that this type of dementia is caused by genetic mutations resulting in an increase in amyloid formation and deposition (Tanzi, 2012). However, many studies have determined that causation of late-onset disease is not attributable to a small number of identical, similar, or other genetic defects leading to Aβ deposition, thereby undermining the contention that Aβ is the universal etiologic factor eliciting the dementia. Importantly, in both familial Alzheimer’s disease and late-onset disease, the neuropathology is essentially identical; this clearly indicates that factors other than the genetic lesions which underlie familial disease must exist to explain the neuropathology of late-onset disease.
Late-onset dementia typically is observed in older age; indeed, age is the primary risk factor for its development. Other factors also may increase risk of late-onset disease. These include: atherosclerosis (de la Torre, 2006), Type 2 diabetes (Revill et al., 2006), neurotrauma (Szczygielski et al., 2005) and infection (Miklossy, 1993; Itzhaki et al., 1997; Balin et al., 1998). Intriguingly, many of the same risk factors could promote systemic inflammation that may also influence disease pathogenesis. Furthermore, chronic, persistent, or latent infections in the brain, all of which may be an outcome following infection with a variety of organisms (see Itzhaki et al., 2004), could reactivate to initiate and/or exacerbate late-onset disease. These possibilities implicate both systemic and neuroinflammation in late-onset dementia. Thus, a likely scenario for development of late-onset dementia centers on poorly understood interactions between genetic risk, as exemplified in part by possession of the Apolipoprotein E (APOEε)4 allele, and environmental factor(s), including infection. For these and other reasons, we have argued that late-onset disease is distinguishable from the genetically-based early-onset disease. On that basis we suggested the designation “late-onset dementia of the Alzheimer’s type” for the late-onset clinical entity, since it is more consistent with our current knowledge of the clinical and neuropathological underpinnings of the disease (Balin et al., 2017); in this article, we employ that designation, or simply late-onset dementia, throughout.
Many attempts have been made to identify infectious agents responsible for late-onset dementia of the Alzheimer’s type (see e.g., Emery et al., 2017). However, none of the agents studied has been unequivocally accepted to be either etiologic, or indeed exacerbating, for dementia-related neuropathology. These include many viral pathogens such as measles virus, lentiviruses, adenovirus and others (Pogo et al., 1987; Friedland et al., 1990). Importantly, studies of herpes simplex virus type 1 (HSV-1) infection in late-onset disease identified this virus as a risk factor for people expressing APOEε4 (Itzhaki et al., 1997, 2001). Further, bacterial pathogens, including Chlamydia trachomatis, Coxiella burnettii and others have been investigated, but no clear relationship with Alzheimer’s pathogenesis has been demonstrated to date (Renvoize et al., 1987). In contrast, we discovered an association between the genesis of late-onset disease and infection with the intracellular respiratory bacterial pathogen Chlamydia pneumoniae. (Balin et al., 1998; Gérard et al., 2006). We summarize that work in this review, along with recently published studies from our group and those of others’ that implicate involvement of this unusual and ubiquitous pathogen in the genesis of senile cognitive dysfunction reflective of late-onset disease.
Chlamydia Pneumoniae
C. pneumoniae, an obligate intracellular bacterium, is a pathogen of the respiratory tract, infecting mucosal surfaces, specifically the lung/pulmonary and nasal mucosa (Grayston et al., 1990; Campbell and Kuo, 2002; Hahn et al., 2002). It is ubiquitous in all societal cultures and geographic regions studied to date (Leinonen, 1993). Many reports have demonstrated that the organism is responsible for a significant proportion of community-acquired pneumonia, and it has been associated with numerous other pulmonary diseases (Grayston et al., 1990; Clementsen et al., 2002). Interestingly, C. pneumoniae infections also have been linked with an array of non-respiratory diseases, including atherosclerosis, inflammatory arthritis, multiple sclerosis and others (Sriram et al., 1998; Schumacher et al., 1999; Wagner et al., 2000; Belland et al., 2004). While some such associations are certainly controversial, credence has been gained for the role of this organism in atherogenesis during the last 20 years (Grayston, 2000; Rosenfeld et al., 2000; Belland et al., 2004).
Similar to other chlamydial species, C. pneumoniae displays a biphasic developmental cycle. The first phase involves the infectious extracellular form of the organism, the elementary body (EB). EB typically infect epithelial cells, but many cell types can be infected, including cells in the nervous system such as astroglia, microglia and neurons (Hatch, 1999; Dreses-Werringloer et al., 2006; Gérard et al., 2006). Following endocytosis into a cytoplasmic inclusion, EB reorganize into a reticulate body (RB), which is the metabolically active form of the organism. RB undergo multiple cycles of cell division followed by a “dedevelopmental” process yielding the infectious EB form again. These are released via eukaryotic cell lysis or exocytosis, which spreads the infection (Hatch, 1999).
Systemic dissemination of the organism from the respiratory tract has been well-documented (Gieffers et al., 2004), and several studies have indicated that this occurs via monocytes (Moazed et al., 1998). Importantly, C. pneumoniae, like other chlamydial species, undergoes long-term infection in a biological state termed “persistence” (Hogan et al., 2004). In persistence, the organism, as with other chlamydial species, is viable and metabolically active but does not complete its normal developmental cycle. Further, persistent chlamydial infections have been shown to be refractory to antibiotic treatment (e.g., Deniset and Pierce, 2010; Phillips-Campbell et al., 2014).
Initial Studies of C. Pneumoniae and Late-Onset Disease
Our initial studies of possible involvement of C. pneumoniae in late-onset disease identified DNA of the organism in 90% of postmortem brain samples from patients diagnosed with late-onset dementia of the Alzheimer’s type; this study employed highly specific polymerase chain reaction (PCR) assays (Balin et al., 1998; Schumacher et al., 1999). Only 5% of control non-demented brain samples contained C. pneumoniae DNA. Positive DNA results were obtained from brain tissues from areas that normally display characteristic neuropathology (e.g., hippocampus) and from those less often demonstrating characteristic pathology (e.g., cerebellum). In nearly 90% of affected brain samples, positive PCR signals were obtained from at least one area showing neuropathology, and from the cerebellum in four cases. In these four cases, neuropathology existed within the cerebella as well as other areas. In contrast, the two relevant affected brain samples that failed to give a positive PCR signal for C. pneumoniae DNA exhibited only mild pathology (Balin et al., 1998). We further analyzed frozen brain samples for intact bacterial RNA using reverse transcriptase-PCR (RT-PCR). mRNA species encoding the KDO transferase and a ~376 kDa protein specific to C. pneumoniae were successfully targeted.
In these initial studies, immunohistochemistry and electron microscopy also were used to analyze samples from PCR-positive brains. Samples from individuals with clear late-onset disease contained C. pneumoniae antigens, specifically in cortical regions such as the temporal, parietal and pre-frontal cortices as well as the hippocampus. In these regions, organism immuno-positivity was observed in various cell types including perivascular macrophages, microglia and astroglia. Ultrastructural analysis of the positive brain samples revealed chlamydial inclusions containing both EB and RB. Immuno-electron microscopy using a gold-conjugated monoclonal antibody specific to an outer membrane protein demonstrated gold particles labeling the organism (Balin et al., 1998; Arking et al., 1999). Immuno-electron microscopy was negative in comparable PCR-negative control brain sections.
PCR and RT-PCR positive sample homogenates were prepared and incubated with human THP-1 monocytes in culture. Viable bacteria were successfully recovered from two brains positive for the organism. Culture of homogenates from two control brains prepared in a similar fashion generated negative results (Balin et al., 1998). Thus, DNA and antigens of C. pneumoniae were found in areas of neuropathology in brain samples from late-onset disease individuals, and samples from frozen brain tissues from these subjects yielded viable organisms. Genetic analyses revealed that 11 of the 17 PCR-positive samples had at least one allele for the APOEε4 isoform (64%), consistent with that allele type being a risk factor for development of late-onset dementia (Roses, 1996). Importantly, in a separate study, it was shown that in patients with reactive arthritis who had DNA from C. pneumoniae in synovial tissues, 2/3 had one or more copies of the APOEε4 allele (Gérard et al., 1999). Together, these and other observations discussed below clearly implicate a relationship between the APOEε4 allelic genotype and infection by C. pneumoniae; they suggest that both factors confer an increased risk for chronic disease genesis (Balin et al., 1998; Gérard et al., 1999).
Not surprisingly, these initial studies and their implications suggesting that an infection with a common bacterium is involved in the origin of late-onset dementia instigated several other groups to attempt confirmation of the organism in brain tissues from other affected and control patient cases. Those studies yielded mixed results, with some reports providing positive identification (e.g., Mahony et al., 2000; Ossewaarde et al., 2000; Di Pietro et al., 2013a), and others failing to find DNA or antigens from the organism in relevant samples (e.g., Nochlin et al., 1999; Gieffers et al., 2000; Ring and Lyons, 2000; Taylor et al., 2002). Importantly and as reviewed by us previously in detail, numerous and various different techniques were used in the confirmation studies, with none utilizing methodology identical to our own. For example, one study failed to find C. pneumoniae in paraffin-embedded brain tissues from confirmed late-onset dementia patients via PCR or immunohistochemical (IHC) analyses (Nochlin et al., 1999); similarly, the organism was not found in several relevant paraffin-embedded brain samples using either PCR or IHC (Gieffers et al., 2000). The recovery of reasonable quality template for PCR from paraffin-embedded tissue can be unreliable, which might explain some negative results. We used exclusively frozen brain-tissue samples were in our studies. One report indicated success in identifying C. pneumoniae by in situ hybridization in many relevant brain samples (Ossewaarde et al., 2000); controls were negative, including those from individuals with other neurological diseases. In our studies, in negative IHC studies, and in the positive study, the same mAb targeting the C. pneumoniae MOMP or lipopolysaccharide (LPS) were employed, suggesting differences in technique in obtaining positive results. Another report explains the positive and negative PCR data from some studies (Mahony et al., 2000). This study employed replicate PCR assays and probit regression analyses to show that DNA from most frozen brain samples analyzed from relevant patients was PCR-positive for C. pneumoniae if enough replicates were performed; multiple assayed controls were always PCR-negative. Clearly, discrepancies in analytical methods used among the different laboratories severely constrain the results obtained (Campbell and Kuo, 2004).
Confirmatory Studies
In subsequent research to confirm and extend our initial studies, we analyzed new tissue samples from late-onset dementia and control brains (Gérard et al., 2006). PCR analyses targeting two C. pneumoniae genes showed that samples from 20/25 late-onset brains, but only 3/27 control brains, were PCR-positive (Gérard et al., 2006). Viable organisms were cultured from the former brain samples, and metabolic activity of the cultured bacteria was demonstrated via identification of several intact chlamydial transcripts. Immunohistochemistry of relevant brain samples identified that astroglia, microglia and ~1/5 of neurons were infected with C. pneumoniae. As in our initial study, infected cells were located in the brain in proximity to both senile plaques and nerve cells containing neurofibrillary tangles (Balin et al., 1998). Together, these observations suggested that C. pneumoniae infection had a direct effect on neuronal cell injury/death. Further, the potential for the organism to act as initiator of granulovacuolar degeneration, another previously acknowledged pathology in late-onset disease, was suggested (Funk et al., 2011).
Continued analyses demonstrated that immunolabeling was positive for C. pneumoniae in the entorhinal and frontal cortices, and in the hippocampal formation of all relevant affected brains (Hammond et al., 2010). These areas exhibited both amyloid deposition and Chlamydia immunoreactivity in apposition to one another when stained with Thioflavin S and labeled with anti-C. pneumoniae antibodies on the same sections, thus revealing fibrillary amyloid and chlamydial immunoreactivity, respectively. Two extracellular patterns of chlamydial immunoreactivity were observed: a punctate pattern and a pattern with amorphous foci. These may represent extrusion of whole organism (punctate) or secreted chlamydial products, e.g., LPS (amorphous foci), into the tissues (Stuart et al., 1994; Hybiske and Stephens, 2007). Amyloid is known to have anti-microbial properties, perhaps allowing it to act as an anionic defensin (Bishop and Robinson, 2004; Kammerman et al., 2006; Soscia et al., 2010; Kumar et al., 2016). As reflected by the deposition of amyloid in the same region as C. pneumoniae, tropism of this organism to the central nervous system (CNS) may be a precursor or trigger for development of damage (Hammond et al., 2010).
The entorhinal cortex and the hippocampal formation, both olfactory structures, are known to be regions demonstrating the earliest damage in the late-onset brain (Mann et al., 1988; Christen-Zaech et al., 2003). Relevant to this, C. pneumoniae has been identified in both human and animal olfactory bulbs in experimental systems (Balin et al., 1998; Little et al., 2004, 2005, 2014). In animals, the organism appeared to spread centrifugally from the olfactory bulbs into the brain proper (Itzhaki et al., 2004; Little et al., 2004, 2005; Little et al., 2014). Thus, these and other observations outlined below support the idea that infection by this pathogen is an early event in the triggering of neuropathogenesis and not a consequence of prior damage providing access of infection to the CNS.
As indicated, C. pneumoniae is a respiratory pathogen, and both olfactory and lung routes for infection of the CNS are supported by DNA sequencing studies in which the organism isolated from a late-onset brain sample was shown to be more closely related to respiratory than to atherosclerotic strains (Roulis et al., 2015). We prepared two cultures of C. pneumoniae from infected brain tissues and evaluated genetic and cell biological features for both. As with most respiratory isolates, both of these isolates were genetically diverse (i.e., not clonal), and single nucleotide polymorphism (SNP) analysis indicated a number of differences even from standard respiratory isolates and strains (Dreses-Werringloer et al., 2009). However, we could not identify any genetic attributes that would indicate neuro-tropism. The recent full genome sequencing of one of the brain isolates in another laboratory confirmed this initial observation (Roulis et al., 2015). Cell biological studies of both isolates demonstrated that they showed standard inclusion morphology and typical chlamydial morphology upon culture in human epithelial cells (HEp-2), astrocytes (U-87 MG) and microglial cells (CHME-5), as in a prior publication (Dreses-Werringloer et al., 2006).
C. Pneumoniae and Apoeε4
apoE was initially identified as a protein component of very low density lipoprotein (VLDL) complexes (Shore and Shore, 1973). The gene encoding APOE is found on human chromosome 19 and is extensively expressed in many tissues. This locus has five allele types: ε2, ε3 and ε4 alleles are the most common in most groups studied, and some data indicate that the ε4 allele type is ancestral. Many studies have demonstrated that apoE is involved in cholesterol homeostasis and metabolism through the direction of the metabolic handling of triglycerides and cholesterol (Mahley and Rall, 2000). The ε4 allele product, apoEε4, is associated with increased risk for several diseases, including Alzheimer’s disease, atherosclerosis and others (e.g., Swanborg et al., 2003; Yu et al., 2014).
Importantly, in situ hybridization analyses targeting apoE showed that brain regions of ε4-bearing individuals with late-onset dementia contained a higher number of C. pneumoniae-infected cells as compared to congruent brain regions from individuals lacking the allele (Gérard et al., 2005). Real time PCR analyses of brain tissues targeting DNA sequences from the organism showed that although the bacterial load in samples lacking the ε4 allele varied, the samples from ε4-bearing individuals had higher loads than did comparable samples from those lacking the allele (Gérard et al., 2005). These observations are consistent with a role for the ε4 gene product in enhancement of dissemination of the organism from the pulmonary system, although we are unaware of studies specifically targeting this issue; this seems an area of interest for future research. Unlike apoE2 and apoE3, apoE4 appears to enhance attachment of C. pneumoniae EB to astroglia and microglia by several-fold over levels observed in the absence of that allelic product (Gérard et al., 2008). When adherent to the chlamydial EB, apoE4 retains its ability to attach to its receptor, the LDL receptor and other members of this receptor family, on the surface of host eukaryotic cells. Thus, while much remains to be elucidated regarding the relationship between apoE4 and enhanced C. pneumoniae uptake into individuals with this phenotype, the link between infection, apoE4, and diseases associated with both, including late-onset dementia of the Alzheimer’s type, is strengthened by our observations.
Neuroinflammation
Infection with all chlamydial species promotes secretion of proinflammatory cytokines in response to outer membrane proteins, heat shock proteins, and the chlamydial LPS, all of which engender prominent inflammatory responses (Rasmussen et al., 1997). Indeed, LPS alone may account for several aspects of relevant neuropathology in late-onset dementia of the Alzheimer’s type. For example, E. coli LPS injected at low dose into the brains of rats elicits an inflammatory response characterized by increased production of cytokines as well as activation of microglial cells (Hauss-Wegrzyniak et al., 1998); this inflammation is comparable to that currently attributed to Aβ deposition in the late-onset brain (Lue et al., 1996). Interestingly, trials investigating the effects of NSAID treatment implicate inflammation as a pathologic factor in the disease, although they did not demonstrate NSAID treatment to be an effective therapeutic approach following disease onset (Breitner, 1996). Importantly, for a number of reasons it was long thought that chlamydiae did not produce peptididoglycan, although genome analyses have demonstrated genes encoding it, and recent studies from several groups have shown that it is, in fact, produced (e.g., Liechti et al., 2014). Clearly this molecule can and probably does act to induce inflammation in the nervous system and elsewhere.
In the late-onset brain, both activated astrocytes and microglia have been identified around amyloid plaques (Wood, 1998). In our studies, we found infected microglia, astroglia, perivascular macrophages and neurons in areas of amyloid deposition. Identification of C. pneumoniae infection in microglia and astroglia in late-onset disease suggests that inflammation initiated by infection might be involved in neuropathogenesis (Balin et al., 1998; Gérard et al., 2006). Both of these cell types respond to insult by producing proinflammatory cytokines and reactive oxygen species. Further activation of microglia and astroglia in response to infected, activated monocytes entering the brain also likely would result in increased production of a variety of cytokines and chemokines (Simpson et al., 1998; Hu and Van Eldik, 1999). Proinflammatory molecules also have been shown to be significantly elevated in supernatant fluids of murine microglial cells infected with C. pneumoniae compared with controls, and infected murine astrocytes also showed elevated levels of cytokines, in particular MCP-1 and IL-6, when compared to controls (Boelen et al., 2007b). Conditioned supernatants from infected murine microglial cells increased neuronal cell death when compared to mock-infected supernatants; upon addition of neutralizing antibodies to IL-6 and TNFα to the conditioned supernatant, neuronal cell death was reduced by ~50% (Boelen et al., 2007b).
Observations from our cell culture studies indicate that although transcription of genes encoding inflammatory mediators in C. pneumoniae-infected monocytes changes by 48 h post-infection, infected cells maintain pro-inflammatory cytokine secretion of IL-1β, IL-6 and IL-8 over 5 days (Lim et al., 2014). Others have found similar proinflammatory cytokines secreted by monocytes in late-onset disease (Fiala et al., 2005, 2007; Feng et al., 2011; Lim et al., 2014; Saresella et al., 2014). High levels of IL-1β are correlated with neuroinflammation in the late-onset brain (Griffin et al., 1994; Sheng et al., 1996; Serou et al., 1999; Mrak and Griffin, 2001). This cytokine activates nitric oxide synthase, which has been implicated in hippocampal neuronal cell death (Cacabelos et al., 1991; Blum-Degen et al., 1995). Additional evidence has implicated IL-1 cytokines in promotion of the neuronal synthesis of the β-amyloid precursor protein (Griffin et al., 1994). These observations provide a rationale for triggering events by which Aβ production would be a result of neuropathogenesis, rather than an initializing event.
Transcription Studies
Our ongoing studies are investigating infection of human astrocytes with C. pneumoniae strain AR39 and its affects on the expression of numerous genes related to APP processing, including ADAM10, BACE1, PSEN1 and the associated subunits of the γ-secretase complex. Transcriptional changes for these genes have been observed in late-onset brains in conjunction with inflammation (reviewed in Agostinho et al., 2015). In this regard, we suggest that C. pneumoniae infection could serve as a pro-inflammatory stimulus to initiate and promote relevant amyloid neuropathogenesis. Interestingly, previous studies demonstrated an interrelationship of C. pneumoniae infection and the altered expression of genes for lipid-homeostasis (APOE, ABCA1, LPL, LRP1), as well as for cytoskeletal organization (MAPT; Alvesalo et al., 2008; Di Pietro et al., 2013b; El Yazouli et al., 2017). These gene changes assure that the pathogen receives sufficient host-derived lipids for the energy-demanding processes of growth and replication. In the process of commandeering the expression of these and other related genes, including those for APP processing, C. pneumoniae infection promotes pathogenesis through amyloid generating pathways (Little et al., 2004, 2014). Because astrocytes are known to propagate a pro-inflammatory state in the late-onset brain (Zhao et al., 2011), we suspect that the transcriptional changes observed following infection with C. pneumoniae will support the role of infection in promoting a pro-inflammatory response.
Related Studies
We have begun to investigate whether a direct link exists between this respiratory pathogen and the chronic, pathologic neurodegenerative pathology characteristic of late-onset disease. Utilizing an in vitro glial model of C. pneumoniae infection, we are asking whether a specific mechanism for the production of relevant neuropathogenesis is identifiable. Additional studies will be required to address whether in vitro results can be replicated in an in vivo setting.
The seminal studies from our group investigating C. pneumoniae’s presence in the late-onset brain demonstrated the involvement of microglial and neuronal cells in addition to astrocytes, indicating that the coordinated response of each cell type may contribute cooperatively to the development of pathology. This coordinated response is especially important to define within the framework of neuroinflammation, since microglial cells and neurons may interact differently through recognition and release of pro-inflammatory molecules based on their heterogenous expression of Nod-like receptors, scavenger receptors, toll-like receptors and complement receptors (Shastri et al., 2013).
Downstream of neuroinflammation, the interdependance of microglia, neurons and astrocytes in producing neuropathology may also manifest from the paracrine signaling of post-cleavage APP byproducts. For example, in recently published studies from others, sAPPα released post-ADAM10 ectodomain shedding of APP was shown to serve neuroprotective roles in other cell types by inhibiting tau phosphorylation by GSK3β (Deng et al., 2015) and BACE1 activation (Peters-Libeu et al., 2015). Such diverse interactions between cell types may complicate an isolated glial cell model of C. pneumoniae-induced neuropathology, but this elucidation is essential for full understanding of the pathophysiology of C. pneumoniae infection in the CNS.
Expression of Other Relevant Gene Transcripts Resulting From C. pneumoniae Infection
In our study of monocyte infection, a number of genes encoding host defense products against bacteria were significantly up-regulated 48 h after C. pneumoniae infection (Lim et al., 2014). One of these genes, DEFB4, encodes a defensin protein with anti-microbial activity linking the innate and adaptive immune responses (Hollox et al., 2003). A second transcript, from DMBT1, is typically up-regulated in response to bacterial activation of NOD2, the intracellular pattern recognition molecule, which activates the NFκB transcription factor (Rosenstiel et al., 2007). The gene product of DMBT1 acts to hinder bacterial invasion and the LPS—induced activation of the toll-like receptor 4 on the surface of cells.
The transcript encoding MCP1/CCL2, a key chemokine for recruiting monocytes and macrophages (Ubogu et al., 2006), was increased up to 1,000-fold following infection of monocytes with C. pneumoniae (Lim et al., 2014). This gene product appears to be an important contributor to the neuroinflammatory process observed in the late-onset brain and is increased in both cerebrospinal fluid and plasma from patients with mild cognitive impairment and dementia compared with controls (Fiala et al., 1998; Galimberti et al., 2006). CCL2 may alter the blood brain barrier to allow increased monocyte migration into brain tissues, as well as affecting production and clearance of Aβ from the brain, and possibly allowing Aβ found in the blood access to the brain (Fiala et al., 1998; Yamamoto et al., 2005; Galimberti et al., 2006). Thus, our studies demonstrating increased CCL2 production during C. pneumoniae infection of monocytes has implications for late-onset disease since C. pneumoniae has been found in cells resident in the brain as well as in perivascular monocytes and macrophages found around the blood vessels in late-onset disease (Balin et al., 1998; MacIntyre et al., 2003; Gérard et al., 2006; Hammond et al., 2010).
Another set of gene product comprise the inflammasome complex that is associated with toll-like receptors and which mediate the response to both extracellular and intracellular pathogens (Schroder and Tschopp, 2010). We determined that the NLRC4 inflammasome transcript was significantly up-regulated following infection. Others have shown previously that activation of this particular inflammasome is responsible for activating caspase-1 and IL-1β secretion in response to bacterial infection (Franchi et al., 2009; Pereira et al., 2011). Intriguingly, this same inflammasome complex can be activated by type III secretion systems characteristic of C. pneumoniae and other gram-negative bacteria (Miao and Warren, 2010). This secretion system acts to transfer effector proteins from bacteria into the cytosol of the host cell, resulting in the generation of reactive oxygen species. These latter are thought to be involved in assemblage of another inflammasome complex, NLRP3 (Abdul-Sater et al., 2009a,b), which coincidentally has also been shown to be activated by chlamydial infections (Abdul-Sater et al., 2010; He et al., 2010). Further, we observed up-regulation of the AIM2 inflammasome transcript, which would result in activation of an additional inflammasome complex as a result of detecting double-stranded DNA from the bacteria in the cytoplasm (Fernandes-Alnemri et al., 2009; Hornung et al., 2009).
Antibiotic Treatment
As C. pneumoniae may be involved in induction of late-onset dementia, antimicrobial treatment might constitute a therapeutic approach to eliminate the organism from the brain. One clinical trial used a combination approach with doxycycline and rifampin for such treatment and evaluated the change in the Standardized Alzheimer’s Disease Assessment Scale cognitive subscale (SADAScog) at 6 months as the primary outcome (Loeb et al., 2004); changes in that score at 12 months was a secondary outcome measure. Overall, results indicated less decline in SADAScog score at 6 months in the antibiotic group compared with those receiving placebo, although the 12-month score for both groups was not significantly different. Importantly, less dysfunctional behavior was observed in the antibiotic group at 3 months, and for that group reduced decline in mini-mental status scores was observed at 12 months. No correlations were made to changes in C. pneumoniae infection as determined by serum antibody titer analysis and following PCR of blood samples. Interestingly, doxycycline has been found to be correlated with the lowering of neuroinflammation in APP/PS1 transgenic mice, suggesting that it may be acting as an anti-inflammatory agent as well as having antibiotic effects (Balducci et al., 2018). This anti-inflammatory activity well could be responsible for the attenuated decline in mini-mental status scores observed at 12 months in the Loeb et al. (2004) trial. However, similar to antibiotic trials assessing efficacy in obviating atherogenesis and cardiovascular disease in which C. pneumoniae has been implicated (Campbell and Rosenfeld, 2014), no meaningful efficacy in amelioration of relevant pathogenesis was demonstrated as an outcome of the late-onset-related antibiotic trial. These failures clearly suggest that an antibiotic treatment regimen for complex disease entities once manifested is not a viable strategy. As with NSAIDS following onset of late-onset dementia (Breitner, 1996), use of antibiotics following disease diagnosis probably is too late to provide meaningful efficacy. Individuals demonstrating evidence of C. pneumoniae infection prior to disease onset, or at the mild cognitive impairment stage, may respond differently, and perhaps better, to antibiotic therapy. However, this approach has yet to be tried in a controlled clinical trial setting.
Approaches other than, or in addition to, antibiotic therapy may be helpful in treating late-onset disease. C. pneumoniae is well known to persist in various contexts, and a persistent form is implicated in chronic diseases. One possible approach to therapy is to manipulate an immune response that can eliminate intracellular infections. To address this issue, we used a synthetic peptide, acALY18 derived from an 18-mer sequence of the transient receptor channel protein 1 (TRPC1; Thacker et al., 2009). This peptide activates, in part, the NLRP3 inflammasome to combat C. pneumoniae infections of monocytes in vitro (Thacker et al., 2012). Using a low dose of acALY18, only 12% of the cells remained infected at 24 h post-treatment, compared to 90% of cells left untreated (Thacker et al., 2012). At 48 h post-infection, analysis of the infected cells revealed that 26 innate and adaptive immune gene transcripts were up regulated in the treated cells compared to infected/untreated cells. These transcripts occurred in four functional groups: (1) cytokines, chemokines, receptors and signaling molecules; (2) host defense; (3) anti-bacterial response; and (4) modulators of the tissue response to inflammation. These specific up-regulations appear to be effective in clearing infection from a large percentage of C. pneumoniae-infected monocytes. Future studies will address more specific transcript up-regulation and specific protein expression leading to eradication of C. pneumoniae infection.
Animal Models for C. Pneumoniae Infection of the CNS
The typical animal models of late-onset disease have utilized humanized transgenic mice that over-express mutants of presenilin and the Aβ precursor protein (Guénette and Tanzi, 1999). In these models, the over-expression of amyloid leads to the development of amyloid plaques in the brain, thus paralleling the pathology typically observed in familial Alzheimer’s disease. Interestingly, recent studies have demonstrated that infecting two different models of humanized transgenic animals separately with different organisms (Bordetella pertussis, McManus et al., 2014) and (Helicobacter pylori, Wang et al., 2014) both result in increased Aβ-amyloid, although through dissimilar mechanisms. What these models do not address, however, are the initiating or triggering events of late-onset disease wherein mutations of the Aβ precursor protein and presenilin proteins are not evident.
We developed non-transgenic animal models to study the means by which infection might impact the pathogenesis of late-onset disease, independent of predisposing genetic factors (Little et al., 2004, 2005). We utilized the C. pneumoniae isolate from a late-onset brain (see above) to infect naïve BALB/c mice to assess if infection would promote brain damage similar to that identified in human disease. BALB/c mice previously had been found to be susceptible to respiratory infection with C. pneumoniae (strain AR-39), which would produce a persistent infection (Laitinen et al., 1996). Thus, we tested the hypothesis that C. pneumoniae infection by a natural route of inoculation in BALB/c mice might trigger processes resulting in development of relevant brain neuropathology (Little et al., 2004). Following intranasal infection, identification of C. pneumoniae in the olfactory neuroepithelia (chlamydial antigens) and the olfactory bulbs (chlamydial antigens and chlamydial bodies) was confirmed by both light and electron microscopy (Little et al., 2004). Analysis of the brain revealed pathological Aβ1–42 deposits that reflected amyloid plaques seen in human disease. Activated astrocytes in addition to reactive astrocytes co-localized with amyloid deposits, suggesting that a cellular inflammatory response had been initiated. This response may be due to C. pneumoniae and/or be directed against amyloid deposits or soluble amyloid induced by C. pneumoniae infection. These observations suggest that the infectious insult results in Aβ generation and lend support to the hypothesis that Aβ can act as a “bioflocculant” (Robinson and Bishop, 2002). Induction of amyloid deposits in the non-transgenic BALB/c mouse brain supports the hypothesis that C. pneumoniae infection can accelerate or induce relevant neuropathology, and that it can trigger late-onset disease pathogenesis without the early-onset genetic mutations.
Studies evaluating treatment following intranasal infection used antibiotics to determine if this approach could limit the pathology induced by infection in the CNS (Hammond et al., 2006). Following intranasal infection with C. pneumoniae (strain AR-39), mice were treated with Avelox (moxifloxacin hydrochloride) for 7–21, 28–42, 56–70, or 84–98 days post-infection; sacrifice was at 6 months post-infection, with brains analyzed for C. pneumoniae, Aβ1–42 deposition (plaques) and astrocyte (GFAP) cellular reactivity. Immunohistochemistry revealed that the organism (or its antigens) was still present at 6 months post-infection in olfactory tissues and in the brain. At 7–21 days post antibiotic treatment, the number of Aβ1–42-reactive amyloid plaques were equal to the level seen in uninfected mice. In infected mice given antibiotic treatment delayed until 56 days post-infection, the amyloid plaque number was 8–9-fold higher than baseline, comparable to the plaque load in the brains of infected animals that received no antibiotics. These data validate the need for early antibiotic intervention prior to disease manifestation, and they support our contention that antibiotic treatment of late-onset disease may not be effective following disease diagnosis. Further, they suggest that early antibiotic intervention post-infection is effective in limiting the amyloid plaque formation that arises because of infection, even though complete eradication of the organism or antigens arising there from may not be achieved.
Additional Animal Model Studies
Our latest animal studies employed the AR-39 laboratory strain for infection rather than C. pneumoniae isolates from the human brain. Brains were analyzed at 1–4 months post-infection by immunohistochemistry using Chlamydia-specific antibodies and antibodies specific for Aβ1–42 (Little et al., 2014). As in our previous report using our human brain isolate of C. pneumoniae, no substantial amyloid deposits were found at 1 month post-infection, and only limited relevant amyloid pathology was apparent at 2 months post-infection. In contrast to the original study, however, at 4 months post-infection amyloid pathology was diminished; brains resembled those from mock-infected mice, suggesting that pathology actually had decreased during the 2–4 months post-infection. Interestingly, our analysis indicated that peak chlamydial burden preceded peak amyloid deposition by 1 month, suggesting that C. pneumoniae infection can serve as a primary stimulus for the production of Aβ-amyloid and subsequent deposition in animal brain tissues. These observations strongly suggest that the human brain isolates elicit a level of neuropathology that is different from the standard AR-39 respiratory strain of C. pneumoniae (see below).
Precedents for infection in the exacerbation of relevant amyloid pathology have been reported for other pathogens in other relevant animal models (McManus et al., 2014; Wang et al., 2014). Once the infection is brought under control though, levels of soluble amyloid apparently decrease, resulting in fewer deposits at 3–4 months post-infection (Hawkes et al., 2012). In mice infected with the brain isolate in our earlier study, amyloid deposits were found as early as 2 months post-infection, with the greatest number identified at 3 months post-infection (Little et al., 2004). Relevant neuropathology developed progressively as both the size and number of amyloid deposits increased from 1–3 months post-infection. Animal models that reflect late-onset disease, however, have been hampered by lack of understanding of the initial factors that promote the early deposition of Aβ-amyloid. Models utilizing direct injection of microbial products have shown induction of transient amyloid production and deposition, suggesting that bacterial products can induce this production (Erickson et al., 2012; Krstic et al., 2012). Interestingly, one previous study did not identify substantial neuropathology in the mouse brain following infection with a respiratory isolate/laboratory strain of C. pneumoniae (Boelen et al., 2007a). The authors of that report noted that discrepancies could have been the result of use of the laboratory strain of C. pneumoniae, suggesting a difference in virulence properties than that of the human brain isolate used in our previous study. Given that our findings with laboratory isolate AR-39 of C. pneumoniae also resulted in less pathology than when using a human brain isolate, we concur with the interpretation of differences in virulence properties between these strains (Little et al., 2014).
Our observations further indicate that C. pneumoniae infections differ in their ability to establish persistence and promote progressive neuropathology as a function of age and dosing. A critical issue in development of late-onset disease is age, and by extension, the age at which C. pneumoniae infection occurs. An earlier study from our group suggested that brain infection in older animals is readily established following exposure to C. pneumoniae (Little et al., 2005). In other studies, aged C57BL/6 mice as compared to young counterparts had a greater propensity to develop chronic and/or progressive respiratory infections following intranasal infection with C. pneumoniae (Eddens et al., 2012). A heptavalent CTL epitope minigene vaccine conferred equal protection in the lungs of both young and older mice. However, although the vaccine partially protected against infection spread to the cardiovascular system of young animals, it failed to provide protection in aged animals (Eddens et al., 2012). These data suggest that vaccine strategies targeting the C. pneumoniae-specific CTL response are protective for respiratory infection in both young and old animals; however, the vaccine used was ineffective in preventing dissemination to the cardiovascular system in aged mice, or in controlling replication of organism in these tissues (Eddens et al., 2012).
In a new study, we will address the issue of whether induction of Alzheimers-like neuropathology is a feature exclusive to infection with C. pneumoniae, or whether this pathology can be induced following exposure to other chlamydial species. Non-transgenic BALB/c mice will be inoculated intranasally with Chlamydia muridarum, a mouse-adapted respiratory isolate of Chlamydia trachomatis. Mouse brain tissues will be examined for Chlamydia-specific labeling and amyloid pathology. Based on data collected from the previous mouse model with C. pneumoniae, we hypothesize that intranasal infection with C. muridarum can also induce relevant pathology in the brains of BALB/c mice. Thus, these experiments will address whether or not this organism will actually enter the brain and promote Alzheimers-like pathology as readily as previously observed following infections with C. pneumoniae. Additional considerations will include the sex of the animals in our studies. To date we, like most others, have used only female animals in our work, partly because human females appear to be at higher risk for the development of late-onset dementia compared to males (Barron and Pike, 2012). Female transgenic mice also have been shown to accumulate more amyloid as compared to males, and they have significant spatial memory deficits (Gallagher et al., 2013; Sierksma et al., 2013). Whether C. pneumoniae infections differ with regard to pathology generated in the brains of non-transgenic female mice compared to those of males remains to be determined.
Conclusion
Clearly, much remains to be elucidated regarding the fundamental biochemical, cellular and molecular genetic underpinnings supporting the initiation and development of neuropathology of late-onset dementia of the Alzheimer’s type, and the possible involvement of C. pneumoniae in those processes (see Figure 1. Infection relationship to late-onset dementia). We suggest that the difference between progressive and non-progressive neuropathology may be due to uncharacterized differences between/among C. pneumoniae strains and host genetic backgrounds. This implies that different virulence factors exist, including those specifying tissue tropism among C. pneumoniae isolates and strains. Thus, the ability of the organism to enter and persist in the CNS, and to potentiate a chronic inflammatory response, is crucial to its role in the initiation and maintenance of neuropathogenesis. We emphasize that, as developed in detail in an earlier article, disease definitions should be reconsidered in light of new observations from our group and many other sources. The most obvious neuropathologic aspects, and the disease phenotype, of late-onset dementia are similar to, indeed are functionally identical to, those of early-onset disease. However, all studies to date consistently demonstrate that late-onset disease does not result from lesions in any of the three genes associated with the latter, and extensive research from many groups over the last 30 years has failed to identify any convincing mechanism by which the plaques and tangles are produced specifically in late-onset patients.
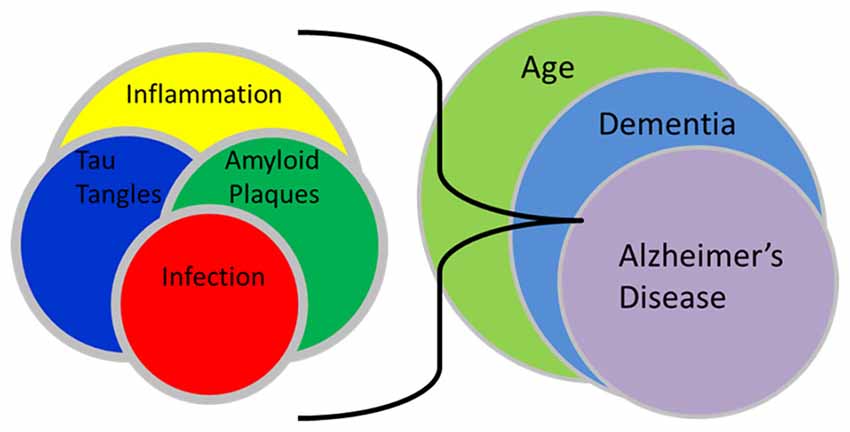
Figure 1. Infection relationship to late-onset dementia. This schematic illustration demonstrates the interactions between infection, amyloid plaques, tau tangles and inflammation with the processes of aging, dementia and Alzheimer’s disease. The interplay of an infection and resultant inflammation can lead to the formation of amyloid plaques and tau tangles which following their accumulation, may promote even more inflammation. These interactions as related to aging may eventually result in a final imbalance that may lead to recognized late-onset dementia of the Alzheimer’s type.
Thus, we reiterate here that in our view the age-related dementia referred to as late-onset Alzheimer’s disease should be redefined as late-onset dementia of the Alzheimer’s type. It is functionally unrelated to the familial early-onset, genetically-determined form of dementia properly designated (familial) Alzheimer’s disease. We contend that the etiologies of early-onset dementia and late-onset dementia of the Alzheimer’s type are fundamentally different. Progress in prevention and treatment of increasingly prevalent late-onset disease will be promoted by observing and acting upon this distinction.
Importantly, we suggest that, in concert with other articles in this issue, pathogens in addition to C. pneumoniae well may be involved in elicitation of the age-related neuropathology that underlies late-onset disease. As argued in previous publications from this group, complex and largely idiopathic diseases of many types, including rheumatoid arthritis and multiple sclerosis, may be the result of complex and yet poorly understood interactions between various aspects of host genetic background and infectious or other environmental agent(s) (Swanborg et al., 2003; Stanich et al., 2009; Balin et al., 2017). Such complex and multi-faceted interactions will be difficult to elucidate in detail. Indeed, details of those interactions may not be fully congruent among/between the diseases. However, it is abundantly clear at this point that many important diseases under intense current study do not conform to the simplicity of Koch’s postulates, and thus they must be scrutinized with non-traditional modern investigational approaches.
Author Contributions
All authors contributed to this article, reviewed and approved the final text. BB and AH were the laboratory heads for the initial studies summarized here, but all other authors contributed to the research results to those given in the text as well.
Funding
The authors thank the many funding agencies that have supported the great body of this work. These include: Adolph and Rose Levis Foundation (BB and DA), Alzheimer’s Association IIRG 04-1016 (BB), Center for Chronic Disorders of Aging (endowed by the Osteopathic Heritage Foundation; BB) at PCOM, Foundation for Research Into Diseases of Aging (FRIDA; BB and CL), National Foundation for Infectious Diseases (DA), and PHS/National Institutes of Health (NIH) grants AG-10160 (BB), AI-44055 (AH), AI-44493 (JW-H) and AR-48331 (JW-H).
Conflict of Interest Statement
The authors declare that the research was conducted in the absence of any commercial or financial relationships that could be construed as a potential conflict of interest.
Acknowledgments
We are grateful for the magnanimous donations of brain tissues from numerous families to further our research into the etiology of Alzheimer’s disease.
References
Abdul-Sater, A. A., Koo, E., Häcker, G., and Ojcius, D. M. (2009a). Inflammasome-dependent caspase-1 activation in cervical epithelial cells stimulates growth of the intracellular pathogen Chlamydia trachomatis. J. Biol. Chem. 284, 26789–26796. doi: 10.1074/jbc.M109.026823
Abdul-Sater, A. A., Saïd-Sadier, N., Ojcius, D. M., Yilmaz, O., and Kelly, K. A. (2009b). Inflammasomes bridge signaling between pathogen identification and the immune response. Drugs Today 45, 105–112.
Abdul-Sater, A., Said-Sadier, N., Padilla, E. V., and Ojcius, D. M. (2010). Chlamydial infection of monocytes stimulates IL-1β secretion through activation of the NLRP3 inflammasome. Microbes Infect. 12, 652–661. doi: 10.1016/j.micinf.2010.04.008
Agostinho, P., Pliássova, A., Oliveira, C. R., and Cunha, R. A. (2015). Localization and trafficking of amyloid-β protein precursor and secretases: impact on Alzheimer’s disease. J. Alzheimers Dis. 45, 329–347. doi: 10.3233/JAD-142730
Albert, N. M. (2000). Inflammation and infection in acute coronary syndrome. J. Cardiovasc. Nurs. 15, 13–26. doi: 10.1097/00005082-200010000-00003
Alvesalo, J., Greco, D., Leinonen, M., Raitila, T., Vuorela, P., and Auvinen, P. (2008). Microarray analysis of a Chlamydia pneumoniae-infected human epithelial cell line by use of gene ontology hierarchy. J. Infect. Dis. 197, 156–162. doi: 10.1086/524142
Arking, E. A., Appelt, D. M., Abrams, J. T., Kolbe, S., Hudson, A. P., and Balin, B. J. (1999). Ultrastructural analysis of Chlamydia pneumoniae in the Alzheimer’s brain. Pathogenesis 1, 201–211.
Balducci, C., Santamaria, G., La Vitola, P., Brandi, E., Grandi, F., Viscomi, A. R., et al. (2018). Doxycycline counteracts neuroinflammation restoring memory in Alzheimer’s disease mouse models. Neurobiol. Aging 70, 128–139. doi: 10.1016/j.neurobiolaging.2018.06.002
Balin, B. J., Hammond, C. J., Little, C. S., Hingley, S. T., Appelt, D. M., Whittum-Hudson, J., et al. (2017). “Chlamydia pneumoniae as an etiologic agent for late-onset Alzheimer’s disease,” in Handbook of Infection and Alzheimer’s Disease (Vol. 5), ed. J. Miklossy (Amsterdam: IOS Press), 41–54.
Balin, B. J., Gerard, H. C., Arking, E. J., Appelt, D. M., Branigan, P. J., Abrams, J. T., et al. (1998). Identification and localization of Chlamydia pneumoniae in the Alzheimer’s brain. Med. Microbiol. Immunol. 187, 23–42. doi: 10.1007/s004300050071
Barron, A. M. I., and Pike, C. J. (2012). Sex hormones, aging and Alzheimer’s disease. Front. Biosci. 4, 976–997.
Belland, R. J., Ouellette, S. P., Gieffers, J., and Byrne, G. I. (2004). Chlamydia pneumoniae and atherosclerosis. Cell. Microbiol. 6, 117–127. doi: 10.1046/j.1462-5822.2003.00352.x
Bishop, G. M., and Robinson, S. R. (2004). Physiological roles of amyloid-β and implications for its removal in Alzheimer’s disease. Drugs Aging 21, 621–630. doi: 10.2165/00002512-200421100-00001
Blum-Degen, D., Müller, T., Kuhn, W., Gerlach, M., Przuntek, H., and Riederer, P. (1995). Interleukin-1 β and interleukin-6 are elevated in the cerebrospinal fluid of Alzheimer’s and de novo Parkinson’s disease patients. Neuroscience Letters 202, 17–20. doi: 10.1016/0304-3940(95)12192-7
Boelen, E., Stassen, F. R., van der Ven, A. J., Lemmens, M. A., Steinbusch, H. P., Bruggeman, C. A., et al. (2007a). Detection of amyloid β aggregates in the brain of BALB/C mice after Chlamydia pneumoniae infection. Acta Neuropathol. 114, 255–261. doi: 10.1007/s00401-007-0252-3
Boelen, E., Steinbusch, H. W., van der Ven, A. J., Grauls, G., Bruggeman, C. A., and Stassen, F. R. (2007b). Chlamydia pneumoniae infection of brain cells: an in vitro study. Neurobiol. Aging 28, 524–532. doi: 10.1016/j.neurobiolaging.2006.02.014
Breitner, J. C. (1996). The role of anti-inflammatory drugs in the prevention and treatment of Alzheimer’s disease. Annu. Rev. Med. 47, 401–411. doi: 10.1146/annurev.med.47.1.401
Cacabelos, R., Barquero, M., García, P., Alvarez, X. A., and Varela de Seijas, E. (1991). Cerebrospinal fluid interleukin-1 β (IL-1 β) in Alzheimer’s disease and neurological disorders. Methods Find. Exp. Clin. Pharmacol. 13, 455–458.
Campbell, L. A., and Kuo, C. C. (2002). Chlamydia pneumoniae pathogenesis. J. Med. Microbiol. 51, 623–625. doi: 10.1099/0022-1317-51-8-623
Campbell, L. A., and Kuo, C. C. (2004). Chlamydia pneumoniae—an infectious risk factor for atherosclerosis? Nat. Rev. Microbiol. 2, 23–32. doi: 10.1038/nrmicro796
Campbell, L. A., and Rosenfeld, M. E. (2014). Persistent C. pneumoniae infection in atherosclerotic lesions: rethinking the clinical trials. Front. Cell. Infect. Microbiol. 4:34. doi: 10.3389/fcimb.2014.00034
Carty, S. M., Snowden, N., and Silman, A. J. (2004). Should infection still be considered as the most likely triggering factor for rheumatoid arthritis? Ann. Rheum. Dis. 63, ii46–ii49. doi: 10.1136/ard.2004.028241
Christen-Zaech, S., Kraftsik, R., Pillevuit, O., Kiraly, M., Martins, R., Khalili, K., et al. (2003). Early olfactory involvement in Alzheimer’s disease. Can. J. Neurol. Sci. 30, 20–25. doi: 10.1017/S0317167100002389
Clementsen, P., Permin, H., and Norn, S. (2002). Chlamydia pneumoniae infection and its role in asthma and chronic obstructive pulmonary disease. J. Investig. Allergol. Clin. Immunol. 12, 73–79.
de la Torre, J. C. (2006). How do heart disease and stroke become risk factors for Alzheimer’s disease? Neurol. Res. 28, 637–644. doi: 10.1179/016164106x130362
de Martel, C., Ferlay, J., Franceschi, S., Vignat, J., Bray, F., Forman, D., et al. (2012). Global burden of cancers attributable to infections in 2008: a review and synthetic analysis. Lancet Oncol. 13, 607–615. doi: 10.1016/S1470-2045(12)70137-7
Deng, J., Habib, A., Obregon, D. F., Barger, S. W., Giunta, B., Wang, Y. J., et al. (2015). Soluble amyloid precursor protein α inhibits tau phosphorylation through modulation of GSK3β signaling pathway. J. Neurochem. 135, 630–637. doi: 10.1111/jnc.13351
Deniset, J. F., and Pierce, G. N. (2010). Possibilities for therapeutic interventions in disrupting Chlamydophila pneumoniae involvement in atherosclerosis. Fundam. Clin. Pharmacol. 24, 607–617. doi: 10.1111/j.1472-8206.2010.00863.x
Di Pietro, M., Filardo, S., Cazzavillan, S., Segala, C., Bevilacqua, P., Bonoldi, E., et al. (2013a). Could past chlamydial vascular infection promote the dissemination of Chlamydia pneumoniae to the brain? J. Biol. Regul. Homeost. Agents 27, 155–164.
Di Pietro, M., Filardo, S., De Santis, F., and Sessa, R. (2013b). Chlamydia pneumoniae infection in atherosclerotic lesion development through oxidative stress: a brief overview. Int. J. Mol. Sci. 14, 15105–15120. doi: 10.3390/ijms140715105
Dreses-Werringloer, U., Gérard, H. C., Whittum-Hudson, J., and Hudson, A. P. (2006). Chlamydophila (Chlamydia) pneumoniae infection of human astrocytes and microglia in culture displays an active, rather than a persistent, phenotype. Am. J. Med. Sci. 332, 168–174. doi: 10.1097/00000441-200610000-00003
Dreses-Werringloer, U., Bhuiyan, M., Zhao, Y., Gérard, H. C., Whittum-Hudson, J., and Hudson, A. P. (2009). Initial characterization of Chlamydophila (Chlamydia) pneumoniae cultured from the late-onset Alzheimer brain. Int. J. Med. Microbiol. 299, 187–201. doi: 10.1016/j.ijmm.2008.07.002
Eddens, T., Beaudoin, S., Steinberger, A., Little, C. S., Shell, D., Wizel, B., et al. (2012). Effect of age and vaccination on extent and spread of chlamydia pneumoniae infection in C57BL/6 mice. Immun. Ageing 9:11. doi: 10.1186/1742-4933-9-11
El Yazouli, L., Criscuolo, A., Hejaji, H., Bouaaza, M., Elmdaghri, N., Alami, A. A., et al. (2017). Molecular characterization of Chlamydia pneumoniae associated to atherosclerosis. Pathog. Dis. 75:4. doi: 10.1093/femspd/ftx039
Emery, D. C., Shoemark, D. K., Batstone, T. E., Waterfall, C. M., Coghill, J. A., Cerajewska, T. L., et al. (2017). 16S rRNA next generation sequencing analysis shows bacteria in Alzheimer’s post-mortem brain. Front. Aging Neurosci. 9:195. doi: 10.3389/fnagi.2017.00195
Erickson, M. A., Hartvigson, P. E., Morofuji, Y., Owen, J. B., Butterfield, D. A., and Banks, W. A. (2012). Lipopolysaccharide impairs amyloid β efflux from brain: altered vascular sequestration, cerebrospinal fluid reabsorption, peripheral clearance and transporter function at the blood-brain barrier. J. Neuroinflammation 9:150. doi: 10.1186/1742-2094-9-150
Feng, Y., Li, L., and Sun, X. H. (2011). Monocytes and Alzheimer’s disease. Neurosci. Bull. 27, 115–122. doi: 10.1007/s12264-011-1205-3
Fernandes-Alnemri, T., Yu, J. W., Datta, P., Wu, J., and Alnemri, E. S. (2009). AIM2 activates the inflammasome and cell death in response to cytoplasmic DNA. Nature 458, 509–513. doi: 10.1038/nature07710
Fiala, M., Cribbs, D. H., Rosenthal, M., and Bernard, G. (2007). Phagocytosis of amyloid-β and inflammation: two faces of innate immunity in Alzheimer’s disease. J. Alzheimers Dis. 11, 457–463. doi: 10.3233/jad-2007-11406
Fiala, M., Lin, J., Ringman, J., Kermani-Arab, V., Tsao, G., Patel, A., et al. (2005). Ineffective phagocytosis of amyloid-β by macrophages of Alzheimer’s disease patients. J. Alzheimers Dis. 7, 221–232; discussion 255–262. doi: 10.3233/jad-2005-7304
Fiala, M., Zhang, L., Gan, X., Sherry, B., Taub, D., Graves, M. C., et al. (1998). Amyloid-β induces chemokine secretion and monocyte migration across a human blood—brain barrier model. Mol. Med. 4, 480–489. doi: 10.1007/bf03401753
Ford, D. K. (1963). Rheumatoid arthritis—an infection? Arthritis Rheum. 6, 159–165. doi: 10.1002/art.1780060207
Franchi, L., Eigenbrod, T., Muñoz-Planillo, R., and Nuñez, G. (2009). The inflammasome: a caspase-1-activation platform that regulates immune responses and disease pathogenesis. Nat. Immunol. 10, 241–247. doi: 10.1038/ni.1703
Friedland, R. P., May, C., and Dahlberg, J. (1990). The viral hypothesis of Alzheimer’s disease. Absence of antibodies to lentiviruses. Arch. Neurol. 47, 177–178. doi: 10.1001/archneur.1990.00530020083019
Funk, K. E., Mrak, R. E., and Kuret, J. (2011). Granulovacuolar degeneration (GVD) bodies of Alzheimer’s disease (AD) resemble late-stage autophagic organelles. Neuropathol. Appl. Neurobiol. 37, 295–306. doi: 10.1111/j.1365-2990.2010.01135.x
Galimberti, D., Schoonenboom, N., Scheltens, P., Fenoglio, C., Bouwman, F., Venturelli, E., et al. (2006). Intrathecal chemokine synthesis in mild cognitive impairment and Alzheimer disease. Arch. Neurol. 63, 538–543. doi: 10.1001/archneur.63.4.538
Gallagher, J. J., Minogue, A. M., and Lynch, M. A. (2013). Impaired performance of female APP/PS1 mice in the Morris water maze is coupled with increased Aβ accumulation and microglial activation. Neurodegener. Dis. 11, 33–41. doi: 10.1159/000337458
Gérard, H. C., Dreses-Werringloer, U., Wildt, K. S., Deka, S., Oszust, C., Balin, B. J., et al. (2006). Chlamydia (Chlamydophila) pneumoniae in the Alzheimer’s brain. FEMS Immunol. Med. Microbiol. 48, 355–366. doi: 10.1111/j.1574-695X.2006.00154.x
Gérard, H. C., Fomicheva, E., Whittum-Hudson, J., and Hudson, A. P. (2008). Apolipoprotein E4 enhances attachment of Chlamydophila (Chlamydia) pneumoniae elementary bodies to host cells. Microb. Pathog. 44, 279–285. doi: 10.1016/j.micpath.2007.10.002
Gérard, H. C., Wang, G. F., Balin, B. J., Schumacher, H. R., and Hudson, A. P. (1999). Frequency of apolipoprotein E (APOE) allele types in patients with Chlamydia-associated arthritis and other arthritides. Microb. Pathog. 26, 35–43. doi: 10.1006/mpat.1998.0242
Gérard, H. C., Wildt, K. L., Whittum-Hudson, J., Lai, Z., Ager, J., and Hudson, A. P. (2005). The load of Chlamydia pneumoniae in the Alzheimer’s brain varies with APOE genotype. Microbial Pathogenesis 39, 19–26. doi: 10.1016/j.micpath.2005.05.002
Gieffers, J., Reusche, E., Solbach, W., and Maass, M. (2000). Failure to detect Chlamydia pneumoniae in brain sections of Alzheimer’s disease patients. J. Clin. Microbiol. 38, 881–882.
Gieffers, J., van Zandbergen, G., Rupp, J., Sayk, F., Kruger, S., Ehlers, S., et al. (2004). Phagocytes transmit Chlamydia pneumoniae from the lungs to the vasculature. Eur. Respir. J. 23, 506–510. doi: 10.1183/09031936.04.00093304
Grayston, J. T. (2000). What is needed to prove that chlamydia pneumoniae does, or does not, play an etiologic role in atherosclerosis? J. Infect. Dis. 181, S585–S586. doi: 10.1086/315595
Grayston, J. T., Campbell, L. A., Kuo, C. C., Mordhorst, C. H., Saikku, P., Thom, D. H., et al. (1990). A new respiratory tract pathogen: chlamydia pneumoniae strain TWAR. J. Infect. Dis. 161, 618–625. doi: 10.1093/infdis/161.4.618
Griffin, W. S., Sheng, J. G., Gentleman, S. M., Graham, D. I., Mrak, R. E., and Roberts, G. W. (1994). Microglial interleukin-1 α expression in human head injury: correlations with neuronal and neuritic β-amyloid precursor protein expression. Neurosci. Lett. 176, 133–136. doi: 10.1016/0304-3940(94)90066-3
Guénette, S. Y., and Tanzi, R. E. (1999). Progress toward valid transgenic mouse models for Alzheimer’s disease. Neurobiol. Aging 20, 201–211. doi: 10.1016/s0197-4580(99)00042-1
Hahn, D. L., Azenabor, A. A., Beatty, W. L., and Byrne, G. I. (2002). Chlamydia pneumoniae as a respiratory pathogen. Front. Biosci. 7, e66–e76. doi: 10.2741/hahn
Hammond, C. J., Hallock, L. R., Howanski, R. J., Appelt, D. M., Little, C. S., and Balin, B. J. (2010). Immunohistological detection of Chlamydia pneumoniae in the Alzheimer’s disease brain. BMC Neurosci. 11:121. doi: 10.1186/1471-2202-11-121
Hammond, C. J., Little, C. S., Longo, N., Procacci, C., Appelt, D. M., and Balin, B. J. (2006). “Antibiotic alters inflammation in the mouse brain during persistent chlamydia pnemoniae infection,” in Alzheimer’s Disease: New Advances, eds K. Igbal, B. Winblat and J. Avila (Bologna, Italy: Medimond), 295–299.
Harney, S., and Wordsworth, B. P. (2002). Genetic epidemiology of rheumatoid arthritis. Tissue Antigens 60, 465–473. doi: 10.1034/j.1399-0039.2002.600601.x
Hatch, T. P. (1999). “Developmental biology,” in Chlamydia: Intracellular Biology, Pathogenesis, and Immunity, ed. R. S. Stephens (Washington DC: ASM Press), 29–67.
Hauss-Wegrzyniak, B., Lukovic, L., Bigaud, M., and Stoeckel, M. E. (1998). Brain inflammatory response induced by intracerebroventricular infusion of lipopolysaccharide: an immunohistochemical study. Brain Res. 794, 211–224. doi: 10.1016/s0006-8993(98)00227-3
Hawkes, C. A., Deng, L., Fenili, D., Nitz, M., and McLaurin, J. (2012). In vivo uptake of β-amyloid by non-plaque associated microglia. Curr. Alzheimer Res. 9, 890–901. doi: 10.2174/156720512803251084
He, X., Mekasha, S., Mavrogiorgos, N., Fitzgerald, K. A., Lien, E., and Ingalls, R. R. (2010). Inflammation and fibrosis during Chlamydia pneumoniae infection is regulated by IL-1 and the NLRP3/ASC inflammasome. J. Immunol. 184, 5743–5754. doi: 10.4049/jimmunol.0903937
Hogan, R. J., Mathews, S. A., Mukhopadhyay, S., Summersgill, J. T., and Timms, P. (2004). Chlamydial persistence: beyond the biphasic paradigm. Infect. Immun. 72, 1843–1855. doi: 10.1128/iai.72.4.1843-1855.2004
Hollox, E. J., Armour, J. A., and Barber, J. C. (2003). Extensive normal copy number variation of a β-defensin antimicrobial-gene cluster. Am. J. Hum. Genet. 73, 591–600. doi: 10.1086/378157
Hornung, V., Ablasser, A., Charrel-Dennis, M., Bauernfeind, F., Horvath, G., Caffrey, D. R., et al. (2009). AIM2 recognizes cytosolic dsDNA and forms a caspase-1-activating inflammasome with ASC. Nature 458, 514–518. doi: 10.1038/nature07725
Hu, J., and Van Eldik, L. (1999). Glial-derived proteins activate cultured astrocytes and enhance β amyloid-induced glial activation. Brain Res. 842, 46–54. doi: 10.1016/s0006-8993(99)01804-1
Hybiske, K., and Stephens, R. S. (2007). Mechanisms of host cell exit by the intracellular bacterium chlamydia. Proc. Natl. Acad. Sci. U S A 104, 11430–11435. doi: 10.1073/pnas.0703218104
Itzhaki, R. F., Dobson, C. B., Lin, W. R., and Wozniak, M. A. (2001). Association of HSV1 and apolipoprotein E-ε4 in Alzheimer’s disease. J. Neurovirol. 7, 570–571. doi: 10.1080/135502801753248169
Itzhaki, R. F., Lin, W. R., Shang, D., Wilcock, G. K., Faragher, B., and Jamieson, G. A. (1997). Herpes simplex virus type 1 in brain and risk of Alzheimer’s disease. Lancet 349, 241–244. doi: 10.1016/S0140-6736(96)10149-5
Itzhaki, R. F., Wozniak, M. A., Appelt, D. M., and Balin, B. J. (2004). Infiltration of the brain by pathogens causes Alzheimer’s disease. Neurobiol. Aging 25, 619–627. doi: 10.1016/j.neurobiolaging.2003.12.021
Kammerman, E. M., Neumann, D. M., Ball, M. J., Lukiw, W., and Hill, J. M. (2006). Senile plaques in Alzheimer’s diseased brains: possible association of β-amyloid with herpes simplex virus type 1 (HSV-1) L-particles. Med. Hypotheses 66, 294–299. doi: 10.1016/j.mehy.2005.07.033
Krstic, D., Madhusudan, A., Doehner, J., Vogel, P., Notter, T., Imhof, C., et al. (2012). Systemic immune challenges trigger and drive Alzheimer-like neuropathology in mice. J. Neuroinflammation 9:151. doi: 10.1186/1742-2094-9-151
Kumar, D. K., Choi, S. H., Washicosky, K. J., Eimer, W. A., Tucker, S., Ghofrani, J., et al. (2016). Amyloid-β peptide protects against microbial infection in mouse and worm models of Alzheimer’s disease. Sci. Transl. Med. 8:340ra72. doi: 10.1126/scitranslmed.aaf1059
Laitinen, K., Laurila, A. L., Leinonen, M., and Saikku, P. (1996). Reactivation of chlamydia pneumoniae infection in mice by cortisone treatment. Infect. Immun. 64, 1488–1490.
Leinonen, M. (1993). Pathogenetic mechanisms and epidemiology of Chlamydia pneumoniae. Eur. Heart J. 14, 57–61.
Liechti, G. W., Kuru, E., Hall, E., Kalinda, A., Brun, Y. V., VanNieuwenhze, M., et al. (2014). A new metabolic cell-wall labelling method reveals peptidoglycan in Chlamydia trachomatis. Nature 506, 507–510. doi: 10.1038/nature12892
Lim, C., Hammond, C. J., Hingley, S. T., and Balin, B. J. (2014). Chlamydia pneumoniae infection of monocytes in vitro stimulates innate and adaptive immune responses relevant to those in Alzheimer’s disease. J. Neuroinflammation 11:217. doi: 10.1186/s12974-014-0217-0
Little, C. S., Bowe, A., Lin, R., Litsky, J., Fogel, R. M., Balin, B. J., et al. (2005). Age alterations in extent and severity of experimental intranasal infection with Chlamydophila pneumoniae in BALB/C mice. Infect. Immun. 73, 1723–1734. doi: 10.1128/iai.73.3.1723-1734.2005
Little, C. S., Hammond, C. J., MacIntyre, A., Balin, B. J., and Appelt, D. M. (2004). Chlamydia pneumoniae induces alzheimer-like amyloid plaques in brains of BALB/C mice. Neurobiol. Aging 25, 419–429. doi: 10.1016/s0197-4580(03)00127-1
Little, C. S., Joyce, T. A., Hammond, C. J., Matta, H., Cahn, D., Appelt, D. M., et al. (2014). Detection of bacterial antigens and Alzheimer’s disease-like pathology in the central nervous system of BALB/C mice following intranasal infection with a laboratory isolate of chlamydia pneumoniae. Front. Aging Neurosci. 6:304. doi: 10.3389/fnagi.2014.00304
Loeb, M. B., Molloy, D., Smieja, M., Standish, T., Goldsmith, C. H., Mahony, J., et al. (2004). A randomized, controlled trial of doxycycline and rifampin for patients with Alzheimer’s disease. J. Am. Geriatr. Soc. 52, 381–387. doi: 10.1111/j.1532-5415.2004.52109.x
Lue, L. F., Brachova, L., Civin, W. H., and Rogers, J. (1996). Inflammation, a β deposition and neurofibrillary tangle formation as correlates of Alzheimer’s disease neurodegeneration. J. Neuropathol. Exp. Neurol. 55, 1083–1088. doi: 10.1097/00005072-199655100-00008
MacIntyre, A., Abramov, R., Hammond, C. J., Hudson, A. P., Arking, E. J., Little, C. S., et al. (2003). Chlamydia pneumoniae infection promotes the transmigration of monocytes through human brain endothelial cells. J. Neurosci. Res. 71, 740–750. doi: 10.1002/jnr.10519
Mahley, R. W., and Rall, C. R. (2000). Apolipoprotein E: far more than a lipid transport protein. Annu. Rev. Genomics Hum. Genet. 1, 507–537. doi: 10.1146/annurev.genom.1.1.507
Mahony, J., Woulfe, J., Munoz, D., Chong, S., Browning, D., and Smieja, M. (2000). “Chlamydia pneumoniae in the Alzheimer’s brain—is DNA detection hampered by low copy number,” in Proceedings of the Fourth Meeting of the European Society for Chlamydia Research editor Pekka Saiku (University of Helsinki), 275.
Mann, D. M., Tucker, C. M., and Yates, P. O. (1988). Alzheimer’s disease: an olfactory connection? Mech. Ageing Dev. 42, 1–15. doi: 10.1016/0047-6374(88)90058-9
McManus, R. M., Higgins, S. C., Mills, K. H., and Lynch, M. A. (2014). Respiratory infection promotes T cell infiltration and amyloid-β deposition in APP/PS1 mice. Neurobiol. Aging 35, 109–121. doi: 10.1016/j.neurobiolaging.2013.07.025
Miao, E. A., and Warren, S. E. (2010). Innate immune detection of bacterial virulence factors via the NLRC4 inflammasome. J. Clin. Immunol. 30, 502–506. doi: 10.1007/s10875-010-9386-5
Miklossy, J. (1993). Alzheimer’s disease—a spirochetosis? Neuroreport 4, 841–848. doi: 10.1097/00001756-199307000-00002
Moazed, T. C., Kuo, C. C., Grayston, J. T., and Campbell, L. A. (1998). Evidence of systemic dissemination of Chlamydia pneumoniae via macrophages in the mouse. J. Infect. Dis. 177, 1322–1325. doi: 10.1086/515280
Mrak, R. E., and Griffin, W. S. (2001). Interleukin-1, neuroinflammation and Alzheimer’s disease. Neurobiol. Aging 22, 903–908. doi: 10.1016/s0197-4580(01)00287-1
Nochlin, D., Shaw, C. M., Campbell, L. A., and Kuo, C. C. (1999). Failure to detect Chlamydia pneumoniae in brain tissues of Alzheimer’s disease. Neurology 53:1888. doi: 10.1212/wnl.53.8.1888-a
O’Connor, S. M., Taylor, C. E., and Hughes, J. M. (2006). Emerging infectious determinants of chronic diseases. Emerg. Infect. Dis. 12, 1051–1057. doi: 10.3201/eid1207.060037
Ossewaarde, J. M., Gielis-Proper, S., Meijer, A., and Roholl, P. J. M. (2000). “Chlamydia pneumoniae antigens are present in the brains of Alzheimer patients, but not in the brains of patients with other dementias,” in Proceedings of the Fourth Meeting of the European Society for Chlamydia Research editor Pekka Saiku (Helsinki, Finland: University of Helsinki), 20–23.
Pereira, M. S., Morgantetti, G. F., Massis, L. M., Horta, C. V., Hori, J. I., and Zamboni, D. S. (2011). Activation of NLRC4 by flagellated bacteria triggers caspase-1-dependent and -independent responses to restrict legionella pneumophila replication in macrophages and in vivo. J. Immunol. 187, 6447–6455. doi: 10.4049/jimmunol.1003784
Peters-Libeu, C., Campagna, J., Mitsumori, M., Poksay, K. S., Spilman, P., Sabogal, A., et al. (2015). sAβPPα is a potent endogenous inhibitor of BACE1. J. Alzheimers Dis. 47, 545–555. doi: 10.3233/JAD-150282
Phillips-Campbell, R., Kintner, J., and Schoborg, R. V. (2014). Induction of the Chlamydia muridarum stress/persistence response increases azithromycin treatment failure in a murine model of infection. Antimicrob. Agents and Chemother. 58, 1782–1784. doi: 10.1128/aac.02097-13
Pogo, B. G., Casals, J., and Elizan, T. S. (1987). A study of viral genomes and antigens in brains of patients with Alzheimer’s disease. Brain 110, 907–915. doi: 10.1093/brain/110.4.907
Rasmussen, S. J., Eckmann, L., Quayle, A. J., Shen, L., Zhang, Y. X., Anderson, D. J., et al. (1997). Secretion of proinflammatory cytokines by epithelial cells in response to chlamydia infection suggests a central role for epithelial cells in chlamydial pathogenesis. J. Clin. Invest. 99, 77–87. doi: 10.1172/jci119136
Renvoize, E. B., Awad, I. O., and Hambling, M. H. (1987). A sero-epidemiological study of conventional infectious agents in Alzheimer’s disease. Age Ageing 16, 311–314. doi: 10.1093/ageing/16.5.311
Revill, P., Moral, M. A., and Prous, J. R. (2006). Impaired insulin signaling and the pathogenesis of Alzheimer’s disease. Drugs Today 42, 785–790. doi: 10.1358/dot.2006.42.12.1032059
Ring, R. H., and Lyons, J. M. (2000). Failure to detect chlamydia pneumoniae in the late-onset Alzheimer’s brain. J. Clin. Microbiol. 38, 2591–2594.
Robinson, S. R., and Bishop, G. M. (2002). Aβ as a bioflocculant: implications for the amyloid hypothesis of Alzheimer’s disease. Neurobiol. Aging 23, 1051–1072. doi: 10.1016/s0197-4580(01)00342-6
Rosenfeld, M. E., Blessing, E., Lin, T. M., Moazed, T. C., Campbell, L. A., and Kuo, C. (2000). Chlamydia, inflammation and atherogenesis. J. Infect. Dis. 181:7. doi: 10.1086/315618
Rosenstiel, P., Sina, C., End, C., Renner, M., Lyer, S., Till, A., et al. (2007). Regulation of DMBT1 Via NOD2 and TLR4 in intestinal epithelial cells modulates bacterial recognition and invasion. J. Immunol. 178, 8203–8211. doi: 10.4049/jimmunol.178.12.8203
Roses, A. D. (1996). Apolipoprotein E alleles as risk factors in Alzheimer’s disease. Annu. Rev. Med. 47, 387–400. doi: 10.1146/annurev.med.47.1.387
Roulis, E., Bachmann, N. L., Myers, G. S., Huston, W., Summersgill, J., Hudson, A., et al. (2015). Comparative genomic analysis of human Chlamydia pneumoniae isolates from respiratory, brain and cardiac tissues. Genomics 106, 373–383. doi: 10.1016/j.ygeno.2015.09.008
Saresella, M., Marventano, I., Calabrese, E., Piancone, F., Rainone, V., Gatti, A., et al. (2014). A complex proinflammatory role for peripheral monocytes in Alzheimer’s disease. J. Alzheimers Dis. 38, 403–413. doi: 10.3233/jad-131160
Schellenberg, G. D. (1995). Genetic dissection of Alzheimer disease, a heterogeneous disorder. Proc. Natl. Acad. Sci. U S A 92, 8552–8559. doi: 10.1073/pnas.92.19.8552
Schroder, K., and Tschopp, J. (2010). The inflammasomes. Cell 140, 821–832. doi: 10.1016/j.cell.2010.01.040
Schumacher, H. R. J., Gerard, H. C., Arayssi, T. K., Pando, J. A., Branigan, P. J., Saaibi, D. L., et al. (1999). Lower prevalence of Chlamydia pneumoniae DNA compared with Chlamydia trachomatis DNA in synovial tissue of arthritis patients. Arthritis Rheum. 42, 1889–1893. doi: 10.1002/1529-0131(199909)42:9<1889::aid-anr13>3.0.co;2-w
Serou, M. J., DeCoster, M. A., and Bazan, N. G. (1999). Interleukin-1 β activates expression of cyclooxygenase-2 and inducible nitric oxide synthase in primary hippocampal neuronal culture: platelet-activating factor as a preferential mediator of cyclooxygenase-2 expression. J. Neurosci. Res. 58, 593–598. doi: 10.1002/(sici)1097-4547(19991115)58:4<593::aid-jnr12>3.0.co;2-4
Shastri, A., Bonifati, D. M., and Kishore, U. (2013). Innate immunity and neuroinflammation. Mediators Inflamm. 2013:342931. doi: 10.1155/2013/342931
Sheng, J. G., Ito, K., Skinner, R. D., Mrak, R. E., Rovnaghi, C. R., Van Eldik, L. J., et al. (1996). In vivo and in vitro evidence supporting a role for the inflammatory cytokine interleukin-1 as a driving force in Alzheimer pathogenesis. Neurobiol. Aging 17, 761–766. doi: 10.1016/s0197-4580(96)00104-2
Shore, V. G., and Shore, B. (1973). Heterogeneity of human plasma very low density lipoproteins. Separation of species differing in protein components. Biochemistry 12, 502–507. doi: 10.1021/bi00727a022
Sierksma, A. S., Prickaerts, J., Chouliaras, L., Rostamian, S., Delbroek, L., Rutten, B. P., et al. (2013). Behavioral and neurobiological effects of prenatal stress exposure in male and female APPswe/PS1dE9 mice. Neurobiol. Aging 34, 319–337. doi: 10.1016/j.neurobiolaging.2012.05.012
Simpson, J. E., Newcombe, J., Cuzner, M., and Woodroofe, M. N. (1998). Expression of monocyte chemoattractant protein-1 and other β-chemokines by resident glia and inflammatory cells in multiple sclerosis lesions. J. Neuroimmunol. 84, 238–249. doi: 10.1016/S0165-5728(97)00208-7
Soscia, S. J., Kirby, J. E., Washicosky, K. J., Tucker, S. M., Ingelsson, M., Hyman, B., et al. (2010). The Alzheimer’s disease-associated amyloid β-protein is an antimicrobial peptide. PLoS One 5:e9505. doi: 10.1371/journal.pone.0009505
Sriram, S., Mitchell, W., and Stratton, C. (1998). Multiple sclerosis associated with chlamydia pneumoniae infection of the CNS. Neurology 50, 571–572. doi: 10.1212/wnl.50.2.571
Stanich, J. A., Carter, J. D., Whittum-Hudson, J., and Hudson, A. P. (2009). Rheumatoid arthritis: disease or syndrome? New Phytol. 1, 179–192. doi: 10.2147/OARRR.S7680
Stuart, E. S., Troidle, K. M., and MacDonald, A. B. (1994). Chlamydial glycolipid antigen: extracellular accumulaton, biological activity and antibody recognition. Curr. Microbiol. 28, 85–90. doi: 10.1007/bf01569052
Swanborg, R. H., Whittum-Hudson, J., and Hudson, A. P. (2003). Infectious agents and multiple sclerosis—are Chlamydia pneumoniae and human herpes virus 6 involved? J. Neuroimmunol. 136, 1–8. doi: 10.1016/s0165-5728(02)00465-4
Szczygielski, J., Mautes, A., Steudel, W. I., Falkai, P., Bayer, T. A., and Wirths, O. (2005). Traumatic brain injury: cause or risk of Alzheimer’s disease? A review of experimental studies. J. Neural Transm. 112, 1547–1564. doi: 10.1007/s00702-005-0326-0
Tanzi, R. E. (2012). The genetics of Alzheimer disease. Cold Spring Harb. Perspect. Med. 2:a006296. doi: 10.1101/cshperspect.a006296
Taylor, G. S., Vipond, I. B., Paul, I. D., Matthews, S., Wilcock, G. K., and Caul, E. O. (2002). Failure to correlate C. pneumoniae with late onset alzheimer’s disease. Neurology 59, 142–143. doi: 10.1212/wnl.59.1.142
Thacker, J. D., Balin, B. J., Appelt, D. M., Sassi-Gaha, S., Purohit, M., Rest, R. F., et al. (2012). NLRP3 inflammasome is a target for development of broad-spectrum anti-infective drugs. Antimicrob. Agents Chemother. 56, 1921–1930. doi: 10.1128/aac.06372-11
Thacker, J. D., Brown, M. A., Rest, R. F., Purohit, M., Sassi-Gaha, S., and Artlett, C. M. (2009). 1-peptidyl-2-arachidonoyl-3-stearoyl-Sn-glyceride: an immunologically active lipopeptide from goat serum (Capra hircus) is an endogenous damage-associated molecular pattern. J. Nat. Prod. 72, 1993–1999. doi: 10.1021/np900360m
Ubogu, E. E., Cossoy, M. B., and Ransohoff, R. M. (2006). The expression and function of chemokines involved in CNS inflammation. Trends Pharmacol. Sci. 27, 48–55. doi: 10.1016/j.tips.2005.11.002
Wagner, A. D., Gerard, H. C., Fresemann, T., Schmidt, W. A., Gromnica-Ihle, E., Hudson, A. P., et al. (2000). Detection of chlamydia pneumoniae in giant cell vasculitis and correlation with the topographic arrangement of tissue-infiltrating dendritic cells. Arthritis Rheum. 43, 1543–1551. doi: 10.1002/1529-0131(200007)43:7<1543::AID-ANR19>3.0.CO;2-8
Wang, X. L., Zeng, J., Feng, J., Tian, Y. T., Liu, Y. J., Qiu, M., et al. (2014). Helicobacter pylori filtrate impairs spatial learning and memory in rats and increases β-amyloid by enhancing expression of presenilin-2. Front. Aging Neurosci. 6:66. doi: 10.3389/fnagi.2014.00066
Wood, P. L. (1998). “Roles of CNS macrophages in neurodegeneration,” in Neuroinflammation. Contemporary Neuroscience, ed. P. L. Wood (Totowa, NJ: Humana Press), 1–59.
Yamamoto, M., Horiba, M., Buescher, J. L., Huang, D., Gendelman, H. E., Ransohoff, R. M., et al. (2005). Overexpression of monocyte chemotactic protein-1/CCL2 in β-amyloid precursor protein transgenic mice show accelerated diffuse α-amyloid deposition. Am. J. Pathol. 166, 1475–1485. doi: 10.1016/s0002-9440(10)62364-4
Yu, J. T., Tan, L., and Harley, J. (2014). Apolipoprotein E in Alzheimers disease: an update. Ann. Rev. Neurobiol. 37, 79–100. doi: 10.1146/annurev-neuro-071013-014300
Keywords: late-onset dementia, Alzheimer’s disease, amyloid, APOE, Chlamydia pneumoniae, etiology, infection, neuroinflammation
Citation: Balin BJ, Hammond CJ, Little CS, Hingley ST, Al-Atrache Z, Appelt DM, Whittum-Hudson JA and Hudson AP (2018) Chlamydia pneumoniae: An Etiologic Agent for Late-Onset Dementia. Front. Aging Neurosci. 10:302. doi: 10.3389/fnagi.2018.00302
Received: 19 March 2018; Accepted: 13 September 2018;
Published: 09 October 2018.
Edited by:
Judith Miklossy, Prevention Alzheimer International Foundation, SwitzerlandReviewed by:
Michael Lardelli, University of Adelaide, AustraliaRobert V. Schoborg, East Tennessee State University, United States
Copyright © 2018 Balin, Hammond, Little, Hingley, Al-Atrache, Appelt, Whittum-Hudson and Hudson. This is an open-access article distributed under the terms of the Creative Commons Attribution License (CC BY). The use, distribution or reproduction in other forums is permitted, provided the original author(s) and the copyright owner(s) are credited and that the original publication in this journal is cited, in accordance with accepted academic practice. No use, distribution or reproduction is permitted which does not comply with these terms.
*Correspondence: Alan P. Hudson, YWh1ZHNvbkBtZWQud2F5bmUuZWR1