- 1Hoglund Brain Imaging Center, University of Kansas Medical Center, Kansas City, KS, USA
- 2Department of Anatomy and Cell Biology, University of Kansas Medical Center, Kansas City, KS, USA
- 3Department of Neurology, University of Kansas Medical Center, Kansas City, KS, USA
- 4Department of Molecular and Integrative Physiology, University of Kansas Medical Center, Kansas City, KS, USA
Following a brain injury, the mobilization of reactive astrocytes is part of a complex neuroinflammatory response that may have both harmful and beneficial effects. There is also evidence that astrocytes progressively accumulate in the normal aging brain, increasing in both number and size. These astrocyte changes in normal brain aging may, in the event of an injury, contribute to the exacerbated injury response and poorer outcomes observed in older traumatic brain injury (TBI) survivors. Here we present our view that proton magnetic resonance spectroscopy (1H-MRS), a neuroimaging approach that probes brain metabolism within a defined region of interest, is a promising technique that may provide insight into astrocyte metabolic changes in the injured and aging brain in vivo. Although 1H-MRS does not specifically differentiate between cell types, it quantifies certain metabolites that are highly enriched in astrocytes (e.g., Myo-inositol, mlns), or that are involved in metabolic shuttling between astrocytes and neurons (e.g., glutamate and glutamine). Here we focus on metabolites detectable by 1H-MRS that may serve as markers of astrocyte metabolic status. We review the physiological roles of these metabolites, discuss recent 1H-MRS findings in the injured and aging brain, and describe how an astrocyte metabolite profile approach might be useful in clinical medicine and clinical trials.
Introduction
Once thought to be merely part of the “glue” that holds together the neurons in the central nervous system (CNS), astrocytes are now known to serve a variety of complex and essential roles in CNS function. Astrocytes are intimately involved in regulating neurotransmission and local cerebral blood flow, and in maintaining the osmotic, pH, and neurotransmitter homeostasis of the extracellular environment in a manner that is essential for normal synaptic function. They influence neuronal structure and function by releasing growth factors and cytokines, and they play a critical role in brain energy metabolism, taking up glucose from the blood and furnishing energy metabolites to neurons (Sofroniew and Vinters, 2010; Allaman et al., 2011).
After a brain injury, molecular signals induce astrocyte activation and migration to the site of injury. These reactive astrocytes play two somewhat conflicting roles. One is protective, by controling extracellular ionic and neurotransmitter balance, providing essential metabolic substrates to neurons, scavenging oxygen free radicals, and contributing to the formation of an astroglial scar which protects the adjacent, relatively uninjured tissue surrounding the brain lesion (Chen and Swanson, 2003). The other may exacerbate injury pathology by playing a key role in the neuroinflammatory response.
Astrocytes also progressively accumulate in the brain during normal aging, increasing in both number and size (Cotrina and Nedergaard, 2002). These aging-related astrocyte changes might, in the event of an injury, contribute to the exacerbated injury response and poorer outcomes observed in older traumatic brain injury (TBI) patients (Popa-Wagner et al., 2011).
Given these critical roles of astrocytes in the function of the normal and injured brain, the ability to monitor key aspects of astrocyte metabolic status in the intact, living organism would be of great value. We propose that proton magnetic resonance spectroscopy (1H-MRS) provides this capability. 1H-MRS is a non-invasive imaging technique that quantifies specific brain metabolites within a defined brain region of interest (ROI; Figure 1). Such metabolites can serve as biomarkers of specific molecular and cellular mechanisms, which can be used to monitor brain pathologies and effectiveness of treatment. 1H-MRS can be carried out on most magnetic resonance imaging (MRI) scanners, and can quantify from 3 to 20 or more metabolites in vivo depending on the magnetic field strength and the selected pulse sequences. These metabolites reflect various physiological processes including bioenergetics, oxidative stress, neurotransmission, and neuroinflammation. The high reproducibility of 1H-MRS data acquired in vivo has been demonstrated in humans and rodents (Brooks et al., 1999; Harris et al., 2012).
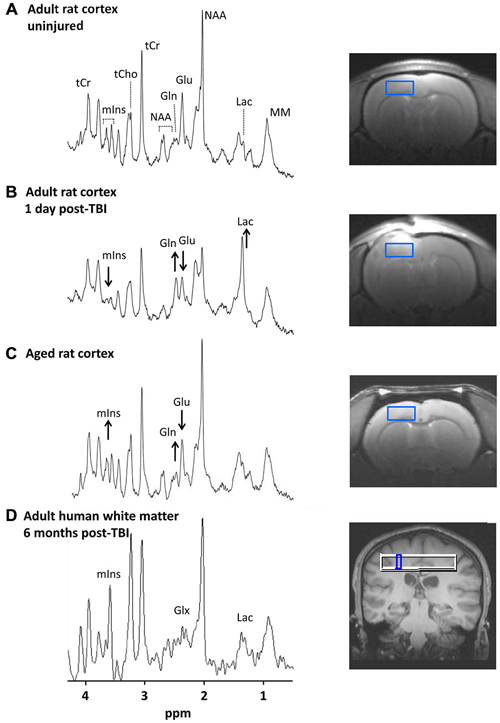
Figure 1. 1H-magnetic resonance spectra in the injured and aging brain. (A–C) show spectra from rat cortex (2.7 × 1.3 × 2.7 mm3 ROI). Images to the right show the location of each ROI. (A) Spectrum from an un-injured adult male rat (3 months old). Major metabolite peaks visible at 9.4 T are labeled. (B) Spectrum from the same animal 1 day after a moderate severity controlled cortical impact TBI. Acute post-injury changes are visible including lower mIns and Glu and higher Gln and Lac. (C) Spectrum from an un-injured aged rat (22 months old). More subtle metabolic changes in the aging rat brain compared with younger controls include lower Glu and higher mIns and Gln. The complex resonance pattern of GSH is not immediately visible even at high magnetic field strengths but is detectable through digital signal processing. (D) Spectrum from human white matter (5 × 5 × 15 mm3 ROI) of an adult male (20 years old) at 6 months post-TBI. The mIns, Lac, and Glx peaks visible at 3 T are indicated. Human studies have reported elevated mIns and Glx in TBI survivors from sub-acute to chronic time points (~1 week to 6 months post-injury; Brooks et al., 2000; Ashwal et al., 2004a,b; Kierans et al., 2014). Abbreviations: ROI, region of interest; TBI, traumatic brain injury; mIns, myo-inositol; Glu, glutamate; Gln, glutamine; Lac, lactate, Glx, Glutamate + Glutamine; NAA, N = acetylaspartate; tCr, total creatine; tCho, total choline; MM, macromolecules. Figure reproduced in part from Harris et al. (2012) and Harris et al. (2014).
In this article, we advance the view that 1H-MRS is a promising technique for non-invasive monitoring of astrocyte metabolism in vivo. Although 1H-MRS does not specifically differentiate between cell types, it can quantify metabolites that are enriched in astrocytes or are related to astrocyte-neuron metabolic shuttling. We identify five 1H-MRS-detectable metabolites related to astrocyte metabolic status (Table 1), review the known physiological roles of these compounds, discuss recent 1H-MRS findings in the injured and aging brain and describe how an astrocyte metabolite profile approach might be useful in clinical medicine and clinical trials.
Components of the Astrocyte Metabolite Profile
Myo-inositol
Myo-inositol (mIns) is the most abundant isomer of inositol in animal tissues. mIns is a component of membrane phospholipids, plays a role in intracellular second messenger cascades, and is an important organic osmolyte. Because the concentration of mIns is higher in astrocytes than in neurons (Brand et al., 1993), mIns has widely been considered an astroglial marker. Due to its high concentration in brain ranging from 4–10 μmol/g, the mIns peak is readily detectable with in vivo 1H-MRS in both laboratory animals (≥3 Tesla, T) and human subjects (≥1.5 T; Kreis et al., 1992; Pfeuffer et al., 1999; Blüml, 2013).
mIns plays an important role in osmotic control of astrocyte cell volume. Decreased mIns is observed early after TBI (Schuhmann et al., 2003; Xu et al., 2011; Harris et al., 2012; Figure 1B), which may reflect mIns efflux from astrocytes as a volume-regulatory strategy under conditions of edema, or alternatively may reflect cell lysis and death (Zhao et al., 2003). By contrast, pathologically activated astrocytes with larger cell volumes tend to have increased mIns (Chang et al., 2013). Thus, later (beginning at several days to weeks) in the progression of the injury, increased mIns suggesting neuroinflammation and reactive astrogliosis has been observed (Bitsch et al., 1999; Schuhmann et al., 2003; Ashwal et al., 2004b; Filibian et al., 2012; Harris et al., 2012; Kierans et al., 2014).
mIns levels also increase with normal brain aging in both humans and animal models (Chang et al., 1996; Reyngoudt et al., 2012; Zhang et al., 2013; Harris et al., 2014; Figure 1C), suggesting an aging-related increase in activated astrocytes. Therefore 1H-MRS might be useful to monitor the process of “inflamm-aging”, the low-grade, chronic up-regulation of pro-inflammatory signals in the normal aging brain (Franceschi et al., 2007).
A link between increased mIns and astrocyte activation is supported by invasive studies in animal models. In rats in status epilepticus, hippocampal mIns was strongly correlated with staining for two astrocyte markers, glial fibrillary acidic protein (GFAP) and S100b (Filibian et al., 2012). In models of Alzheimer’s disease and TBI, increased mIns corresponded with increased GFAP staining within the same ROI (Chen et al., 2009) and following the same time course (Schuhmann et al., 2003). Moreover, an experimental anti-inflammatory treatment in a mouse model of Alzheimer’s disease attenuated both the increase in 1H-MRS-detectable mIns and the increase in GFAP-positive astrocytes (Chen et al., 2012).
Data from humans are more limited, but also support a link between mIns and astrocyte activation. In patients with multiple sclerosis, GFAP-positive biopsies of demyelinating lesions corresponded with elevated mIns measured in the same location (Bitsch et al., 1999). In patients with schizophrenia, mIns concentrations in the amygdala correlated with serum levels of S100b (Rothermundt et al., 2007). A group of high-likelihood Alzheimer’s disease patients had higher mIns and higher postmortem GFAP staining than low-likelihood controls, although in the combined sample GFAP and mIns levels did not reach a statistical correlation (Murray et al., 2014).
In sum, the evidence from 1H-MRS suggests that the mIns changes observed in brain injury and aging reflect physiological changes in astrocytes. As others have recently suggested (Rae, 2014), mIns is unlikely to be a marker for astrocyte cell density alone since it is evident that pathological conditions such as osmotic stress can alter intracellular concentrations of mIns (Strange et al., 1994). Rather, we propose that early decreases after a brain injury might reflect edema and/or cell lysis. Increases seen in aging or at later intervals after a brain injury likely reflect astrocyte activation and proliferation during neuroinflammation.
Glutathione
The reduced form of glutathione (GSH) and ascorbate (Asc, or vitamin C) are the two most prominent endogenous antioxidants in the CNS, protecting cells from damaging reactive oxygen species and preserving essential cellular functions. While Asc predominates in neurons, GSH concentrations are considerably higher in astrocytes (Rice and Russo-Menna, 1998; Sun et al., 2006). Therefore, GSH measured with 1H-MRS may provide an in vivo marker of astrocyte antioxidant status. Additional cellular functions of GSH include amino acid transport, acting as a storage form of cysteine and a cofactor for redox reactions, and protecting neuronal signal transduction (Brown, 1994; Rae, 2014). GSH is routinely quantified in animal studies using 1H-MRS at high magnetic fields, but is somewhat more challenging to measure in humans, requiring specialized acquisition strategies (Trabesinger and Boesiger, 2001; Terpstra et al., 2003; Choi et al., 2011).
Brain GSH levels fall rapidly after TBI (Ansari et al., 2008a,b; Harris et al., 2012), consistent with an early post-injury increase in reactive oxygen species that depletes brain antioxidant reserves (Kontos and Povlishock, 1986; Hall et al., 2010). Moreover, the depletion of GSH is related to the severity of brain damage (Harris et al., 2012; Di Pietro et al., 2014). In contrast, GSH levels may increase in pathologies where astrocytes are chronically activated and recruited. In a rat model of epilepsy, Filibian et al. (2012) showed that elevated GSH concentrations in the hippocampus were highly correlated with quantitative GFAP staining, supporting the use of GSH as an in vivo marker of astrocyte activation.
Recent evidence from animal models and humans points to lower GSH concentrations in the aging brain (Maher, 2005; Emir et al., 2011). Our group has found that the regional pattern of GSH depletion in the aging brain differs somewhat from that of Asc, suggesting that local populations of astrocytes and neurons might be differentially sensitive to oxidative stress during aging (Harris et al., 2014). In any case, lower antioxidant levels suggest that the brain’s ability to combat oxidative stress may be impaired in aging. Lower GSH levels could contribute to an age-related decline in cellular function and increase the brain’s susceptibility to insult (Maher, 2005). This notion is supported by studies of brain injury in aged rats, which show more severe oxidative damage after TBI compared with young adult animals (Shao et al., 2006; Gilmer et al., 2010). Since antioxidant therapies are currently under investigation for both brain trauma and aging, GSH offers a potential in vivo marker to evaluate therapeutic target engagement.
Glutamate and glutamine
Glutamate (Glu) serves as the major excitatory neurotransmitter and is a precursor of γ-aminobutyric acid (GABA), the major inhibitory neurotransmitter in the CNS. Glu is also closely associated with glutamine (Gln) via the Glu-Gln cycle between neurons and astrocytes. After its release from neuronal synapses, Glu is taken up by nearby astrocytes, converted to Gln, then transported back to neurons. Overall, brain Glu concentrations range from 6–13 μmol/g and Gln from 2–4 μmol/g (Michaelis et al., 1993; Petroff et al., 1995; Hurd et al., 2004). Although Glu is found in all cells, glutamatergic neurons contain the highest levels of Glu compared with other neuronal and glial cell types. In contrast, because Gln synthetase is exclusive to astrocytes, Gln levels are typically much higher in astrocytes. Therefore, 1H-MRS measures of the relative concentrations of Gln and Glu may be useful to infer changes in astrocyte populations or metabolic status.
Studies by our group and others in animal models point to rapidly increased Gln concurrent with decreased Glu after brain injury (Lei et al., 2009; Xu et al., 2011; Harris et al., 2012; Figure 1B), a finding consistent with injury-induced Glu release from neurons followed by rapid uptake and conversion to Gln by astrocytes i.e., the Glu-Gln cycle (Bartnik-Olson et al., 2013). This likely reflects a critical role of astrocytes in helping to limit the effects of Glu excitotoxicity in the acute post-injury period.
In human and animal studies of the normal aging brain, the Gln/Glu ratio increases progressively (Kaiser et al., 2005; Duarte et al., 2014; Harris et al., 2014; Figure 1C), suggesting a potential aging-related shift in the Glu-Gln cycle. This is consistent with the finding of ~30% lower flux through the Glu-Gln cycle in healthy elderly humans compared with younger adults (Boumezbeur et al., 2010). Whether such changes in neuronal-astrocyte metabolic shuttling are responsible for age-related declines in cognitive function remains an open question. However, this could be addressed by studies that link 1H-MRS measures of Glu and Gln with cognitive function in aging.
Lactate
According to the lactate shuttle hypothesis, glycolysis in astrocytes generates lactate (Lac), which is then released from the astrocytes to serve as a preferred fuel for neurons (Pellerin and Magistretti, 1994). Lac is present in brain at approximately 0.5 μmol/g, which is approaching the lower threshold for detection in animals and humans with standard 1H-MRS techniques. However, in pathological conditions that alter bioenergetics and result in increased Lac accumulation, the Lac peak is easily detectable.
After a brain injury, an acutely increased energy demand results in increased glycolysis, which along with impaired respiratory function of mitochondria results in rapid Lac accumulation. Elevated brain Lac after injury has been well documented with spectroscopic methods (Ross et al., 1998; Ashwal et al., 2000; Schuhmann et al., 2003; Lescot et al., 2010; Harris et al., 2012; Figure 1B) and microdialysis (Nilsson et al., 1990; Yokobori et al., 2011). Since Lac is produced by astrocytes in areas of glutamatergic neuronal activity, astrocytes are likely a major source of this Lac spike early after TBI. In support of this view, Schuhmann et al. (2003) reported an increase in Lac up to 24 h after TBI in animals which corresponded to an increase in glutamate dehydrogenase (a Glu metabolizing enzyme preferentially localized in astrocytes) in the ROI used for 1H-MRS. Since Lac has been shown to strongly predict poor outcomes after TBI in humans (Ashwal et al., 2000; Glenn et al., 2003), the ability to track Lac accumulation in vivo could have important clinical applications.
In contrast, reports on Lac concentrations in normal brain aging are inconsistent, ranging from falls to increases (Urrila et al., 2004; Paban et al., 2010; Emir et al., 2011; Zhang et al., 2013; Duarte et al., 2014; Harris et al., 2014), perhaps reflecting a varying capacity for bioenergetic homeostasis in individual subjects or brain regions. Altered Lac levels in the aging brain compared with younger adults implies a mismatch between astrocytic glycolysis and neuronal oxidative metabolism. If metabolic coupling between neurons and astrocytes is indeed altered in aging, this could impair the ability of the brain to respond to insult and might contribute to the poor outcomes seen after TBI in older individuals.
Challenges for Magnetic Resonance Spectroscopy?
We envision a future in which 1H-MRS biomarkers will aid in prognostication and in selecting therapies to mitigate the cognitive impairment seen with TBI, or the cognitive decline seen with aging. Furthermore, these biomarkers will provide a non-invasive means to track therapeutic target engagement and efficacy. Although at present we can acquire signals from numerous 1H-MRS biomarkers in animals, there remains considerable opportunity for developing new acquisition approaches to expand the repertoire of metabolites routinely quantified in humans.
Using widely available spectroscopic acquisitions, Lac and mIns are easily quantified in animal models and in humans. However, GSH, Glu, and Gln are more challenging, largely due to overlapping signals. The issue of overlapping signals can be resolved at higher magnetic field strengths; indeed, there is already a proliferation of MRI scanners at 7 T and higher for research use and some are advocating the use of fields greater than 3 T for clinical investigation. An alternative approach is to develop more sophisticated acquisition sequences such as two-dimensional spectroscopy (Lin et al., 2015) and sequences aimed at individual brain metabolites such as double quantum acquisitions for GSH (Choi et al., 2011).
In addition to technical challenges of data acquisition, challenges of data validation remain to be addressed before 1H-MRS biomarkers can be used in clinical applications. The precise mechanisms underlying altered mIns, GSH, Gln/Glu, and Lac following brain injury or in aging are yet to be clarified. Thus, the use of these metabolites as biomarkers of astrocyte metabolic status needs to be further validated specifically in the context of brain injury and aging, by comparing their levels with other quantitative measures of astrocyte physiology. In addition, there is currently little information available about the possible contribution of other CNS cell types such as microglia to the 1H-MRS profiles. Non-invasive 1H-MRS approaches in animal models are well suited to addressing these questions, since they permit intra-subject validation of pathological mechanisms when in vivo scanning is followed by invasive histological and biochemical analyses of the same tissue. Once validated in animal models, 1H-MRS can form the basis for translational imaging studies in humans, where it can be combined with measures of cognitive function and other clinically relevant outcome measures.
How Would an Astrocyte Metabolite Profile be Useful?
We have identified five 1H-MRS-detectable metabolites related to astrocyte metabolic status. Although taken individually, each metabolite might provide valuable information on individual molecular or cellular mechanisms as discussed above, they can also be considered in combination—as an astrocyte metabolite profile. On the whole, the profiles of astrocyte-relevant metabolite changes in brain aging and injury look remarkably similar (Table 1) and are consistent with two possible pathological mechanisms associated with aging and injury. The first is bioenergetic disruption. Impaired mitochondrial respiration is known to increase Lac accumulation and may be related to increased Gln/Glu ratio in the aging and injured brain since Glu is derived from α-ketoglutarate, a TCA cycle intermediate. Moreover, bioenergetic deficit can also result in reduced production of endogenous anti-oxidants, including GSH. Disrupted mitochondrial respiration can also cause increased reactive oxidative species, which would further consume GSH. A second pathological mechanism is inflammation, associated with astrocyte activation and recruitment, which has also been reported in both aging and injured brain. An increased astrocyte pool would result in elevated mIns and could also yield elevated Gln/Glu.
Several lines of evidence suggest that a well-characterized metabolite profile is likely to be useful as an overall biomarker of injury severity and for prognostication, for stratifying patients into trials, and for treatment selection and management.
Larger metabolite changes are associated with greater injury severity and worse functional outcomes. For example, we have shown in an animal model of TBI that the magnitude of change in 1H-MRS-detectable metabolites is greater in tissue located closer to the primary lesion site (Harris et al., 2012). Further data from our laboratory show that Lac, GSH, and Glu, key components of the astrocyte metabolite profile, measured within a day of injury predicted the amount of subsequent tissue loss at 2 weeks (Sharrock et al., 2015). In human TBI survivors, Ashwal et al. found that greater Lac and mIns changes were associated with poorer Glasgow coma scores and also with poorer outcome (Ashwal et al., 1997, 2004b). Thus, the astrocyte metabolite profile shows promise as a prognostic indicator for brain injury.
The astrocyte metabolite profile might also be useful in understanding cognitive decline in aging. As shown in Table 1, the metabolite profile of the aging brain is similar to that of a younger brain following TBI. The TBI profile is known to be associated with poorer cognitive functioning (Ashwal et al., 1997, 2004b). Thus, we might speculate that if this profile is seen in aging, it could provide information related to declining cognitive function that could be used to identify individuals at risk for pathologies of aging or to stratify participants for trials.
Finally, astrocyte-associated 1H-MRS biomarkers might be used for treatment selection. To the extent that 1H-MRS-detected metabolites are associated with cellular and molecular mechanisms, changes observed in prognostic studies might identify targets for therapeutic intervention. For example, an elderly patient with cognitive impairment and lower GSH might benefit from anti-oxidant therapy and a TBI patient with high mIns might benefit from anti-inflammatory treatment. Finally, these biomarkers could be used as pharmacodynamic indicators to verify target engagement in the brain, and to help confirm treatment efficacy.
Conclusions and Future Directions
1H-MRS is one of the few techniques that can non-invasively assess the chemical concentrations and metabolic pathways of the brain in living individuals. We propose that 1H-MRS-detectable metabolites can serve as translational biomarkers of certain aspects of brain health and pathology. Specifically, certain metabolites seen on 1H-MRS promise insight into the metabolic status of astrocytes in vivo.
The studies we have discussed here have largely focused on 1H-MRS findings in normal brain aging or brain injury. Although it is clear that the risk of brain injury is elevated in older individuals and that outcomes in these patients are significantly worse (Thompson et al., 2006), there is a dearth of information on the combined effects of brain aging and brain injury. Thus, there is a critical need for further studies aimed at better understanding the effects of aging on TBI pathophysiology.
1H-MRS provides the ability to monitor specific aspects of brain metabolic status in animals and humans as well as across different biological conditions. Taken together, the evidence we have presented suggests that 1H-MRS provides a unique window onto astrocyte metabolic changes in vivo. Given the many essential roles of astrocytes in brain function, we believe that the ability to monitor astrocyte metabolism in vivo will provide important insights into pathological changes in the aged and injured brain—a critical step for developing and translating therapies to improve long-term outcomes in this vulnerable patient population.
Conflict of Interest Statement
The authors declare that the research was conducted in the absence of any commercial or financial relationships that could be construed as a potential conflict of interest.
Acknowledgments
This work was supported in part by the U.S. National Institutes of Health through the University of Kansas Alzheimer’s Center (P30 AG035982), Frontiers: The Heartland Institute for Clinical & Translational Research (UL1 TR000001), R21 NS091920, KUMC Lied Endowed Basic Science grants to Drs. Harris and Brooks, and the Hoglund Family Foundation. The contents are solely the responsibility of the authors and do not necessarily represent the official views of the National Institutes of Health.
References
Allaman, I., Bélanger, M., and Magistretti, P. J. (2011). Astrocyte-neuron metabolic relationships: for better and for worse. Trends Neurosci. 34, 76–87. doi: 10.1016/j.tins.2010.12.001
Ansari, M. A., Roberts, K. N., and Scheff, S. W. (2008a). Oxidative stress and modification of synaptic proteins in hippocampus after traumatic brain injury. Free Radic. Biol. Med. 45, 443–452. doi: 10.1016/j.freeradbiomed.2008.04.038
Ansari, M. A., Roberts, K. N., and Scheff, S. W. (2008b). A time course of contusion-induced oxidative stress and synaptic proteins in cortex in a rat model of TBI. J. Neurotrauma 25, 513–526. doi: 10.1089/neu.2007.0451
Ashwal, S., Holshouser, B., Tong, K., Serna, T., Osterdock, R., Gross, M., et al. (2004a). Proton MR spectroscopy detected glutamate/glutamine is increased in children with traumatic brain injury. J. Neurotrauma 21, 1539–1552. doi: 10.1089/0897715042441756
Ashwal, S., Holshouser, B., Tong, K., Serna, T., Osterdock, R., Gross, M., et al. (2004b). Proton spectroscopy detected myoinositol in children with traumatic brain injury. Pediatr. Res. 56, 630–638. doi: 10.1203/01.pdr.0000139928.60530.7d
Ashwal, S., Holshouser, B. A., Shu, S. K., Simmons, P. L., Perkin, R. M., Tomasi, L. G., et al. (2000). Predictive value of proton magnetic resonance spectroscopy in pediatric closed head injury. Pediatr. Neurol. 23, 114–125. doi: 10.1016/s0887-8994(00)00176-4
Ashwal, S., Holshouser, B. A., Tomasi, L. G., Shu, S., Perkin, R. M., Nystrom, G. A., et al. (1997). 1H-magnetic resonance spectroscopy-determined cerebral lactate and poor neurological outcomes in children with central nervous system disease. Ann. Neurol. 41, 470–481. doi: 10.1002/ana.410410410
Bartnik-Olson, B. L., Harris, N. G., Shijo, K., and Sutton, R. L. (2013). Insights into the metabolic response to traumatic brain injury as revealed by (13)C NMR spectroscopy. Front. Neuroenergetics 5:8. doi: 10.3389/fnene.2013.00008
Bitsch, A., Bruhn, H., Vougioukas, V., Stringaris, A., Lassmann, H., Frahm, J., et al. (1999). Inflammatory CNS demyelination: histopathologic correlation with in vivo quantitative proton MR spectroscopy. AJNR Am. J. Neuroradiol. 20, 1619–1627.
Blüml, S. (2013). “Magnetic resonance spectroscopy: basics,“ in MR Spectroscopy of Pediatric Brain Disorders, eds S. Blüml and A. Panigrahy (Heidelberg: Springer), 11–23. doi: 10.1007/978-1-4419-5864-8_2
Boumezbeur, F., Mason, G. F., de Graaf, R. A., Behar, K. L., Cline, G. W., Shulman, G. I., et al. (2010). Altered brain mitochondrial metabolism in healthy aging as assessed by in vivo magnetic resonance spectroscopy. J. Cereb. Blood Flow Metab. 30, 211–221. doi: 10.1038/jcbfm.2009.197
Brand, A., Richter-Landsberg, C., and Leibfritz, D. (1993). Multinuclear NMR studies on the energy metabolism of glial and neuronal cells. Dev. Neurosci. 15, 289–298. doi: 10.1159/000111347
Brooks, W. M., Friedman, S. D., and Stidley, C. A. (1999). Reproducibility of 1H-MRS in vivo. Magn. Reson. Med. 41, 193–197. doi: 10.1002/(SICI)1522-2594(199901)41:1193::AID-MRM273.0.CO;2-P
Brooks, W. M., Stidley, C. A., Petropoulos, H., Jung, R. E., Weers, D. C., Friedman, S. D., et al. (2000). Metabolic and cognitive response to human traumatic brain injury: a quantitative proton magnetic resonance study. J. Neurotrauma 17, 629–640. doi: 10.1089/089771500415382
Brown, L. A. (1994). Glutathione protects signal transduction in type II cells under oxidant stress. Am. J. Physiol. 266, L172–L177.
Chang, L., Ernst, T., Poland, R. E., and Jenden, D. J. (1996). In vivo proton magnetic resonance spectroscopy of the normal aging human brain. Life Sci. 58, 2049–2056. doi: 10.1016/0024-3205(96)00197-x
Chang, L., Munsaka, S. M., Kraft-Terry, S., and Ernst, T. (2013). Magnetic resonance spectroscopy to assess neuroinflammation and neuropathic pain. J. Neuroimmune Pharmacol. 8, 576–593. doi: 10.1007/s11481-013-9460-x
Chen, S. Q., Cai, Q., Shen, Y. Y., Wang, P. J., Teng, G. J., Li, M. H., et al. (2012). (1)H-MRS evaluation of therapeutic effect of neural stem cell transplantation on Alzheimer’s disease in AβPP/PS1 double transgenic mice. J. Alzheimers Dis. 28, 71–80. doi: 10.3233/JAD-2010-110893
Chen, S. Q., Wang, P. J., Ten, G. J., Zhan, W., Li, M. H., and Zang, F. C. (2009). Role of myo-inositol by magnetic resonance spectroscopy in early diagnosis of Alzheimer’s disease in APP/PS1 transgenic mice. Dement. Geriatr. Cogn. Disord. 28, 558–566. doi: 10.1159/000261646
Chen, Y., and Swanson, R. A. (2003). Astrocytes and brain injury. J. Cereb. Blood Flow Metab. 23, 137–149. doi: 10.1097/01.WCB.0000044631.80210.3C
Choi, I. Y., Lee, S. P., Denney, D. R., and Lynch, S. G. (2011). Lower levels of glutathione in the brains of secondary progressive multiple sclerosis patients measured by 1H magnetic resonance chemical shift imaging at 3 T. Mult. Scler. 17, 289–296. doi: 10.1177/1352458510384010
Cotrina, M. L., and Nedergaard, M. (2002). Astrocytes in the aging brain. J. Neurosci. Res. 67, 1–10. doi: 10.1002/jnr.10121
Di Pietro, V., Lazzarino, G., Amorini, A. M., Tavazzi, B., D’urso, S., Longo, S., et al. (2014). Neuroglobin expression and oxidant/antioxidant balance after graded traumatic brain injury in the rat. Free Radic. Biol. Med. 69, 258–264. doi: 10.1016/j.freeradbiomed.2014.01.032
Duarte, J. M., Do, K. Q., and Gruetter, R. (2014). Longitudinal neurochemical modifications in the aging mouse brain measured in vivo by 1H magnetic resonance spectroscopy. Neurobiol. Aging 35, 1660–1668. doi: 10.1016/j.neurobiolaging.2014.01.135
Emir, U. E., Raatz, S., Mcpherson, S., Hodges, J. S., Torkelson, C., Tawfik, P., et al. (2011). Noninvasive quantification of ascorbate and glutathione concentration in the elderly human brain. NMR Biomed. 24, 888–894. doi: 10.1002/nbm.1646
Filibian, M., Frasca, A., Maggioni, D., Micotti, E., Vezzani, A., and Ravizza, T. (2012). In vivo imaging of glia activation using 1H-magnetic resonance spectroscopy to detect putative biomarkers of tissue epileptogenicity. Epilepsia 53, 1907–1916. doi: 10.1111/j.1528-1167.2012.03685.x
Franceschi, C., Capri, M., Monti, D., Giunta, S., Olivieri, F., Sevini, F., et al. (2007). Inflammaging and anti-inflammaging: a systemic perspective on aging and longevity emerged from studies in humans. Mech. Ageing Dev. 128, 92–105. doi: 10.1016/j.mad.2006.11.016
Gilmer, L. K., Ansari, M. A., Roberts, K. N., and Scheff, S. W. (2010). Age-related mitochondrial changes after traumatic brain injury. J. Neurotrauma 27, 939–950. doi: 10.1089/neu.2009.1181
Glenn, T. C., Kelly, D. F., Boscardin, W. J., Mcarthur, D. L., Vespa, P., Oertel, M., et al. (2003). Energy dysfunction as a predictor of outcome after moderate or severe head injury: indices of oxygen, glucose and lactate metabolism. J. Cereb. Blood Flow Metab. 23, 1239–1250. doi: 10.1097/01.wcb.0000089833.23606.7f
Hall, E. D., Vaishnav, R. A., and Mustafa, A. G. (2010). Antioxidant therapies for traumatic brain injury. Neurotherapeutics 7, 51–61. doi: 10.1016/j.nurt.2009.10.021
Harris, J. L., Yeh, H. W., Choi, I. Y., Lee, P., Berman, N. E., Swerdlow, R. H., et al. (2012). Altered neurochemical profile after traumatic brain injury: (1)H-MRS biomarkers of pathological mechanisms. J. Cereb. Blood Flow Metab. 32, 2122–2134. doi: 10.1038/jcbfm.2012.114
Harris, J. L., Yeh, H. W., Swerdlow, R. H., Choi, I. Y., Lee, P., and Brooks, W. M. (2014). High-field proton magnetic resonance spectroscopy reveals metabolic effects of normal brain aging. Neurobiol. Aging 35, 1686–1694. doi: 10.1016/j.neurobiolaging.2014.01.018
Hurd, R., Sailasuta, N., Srinivasan, R., Vigneron, D. B., Pelletier, D., and Nelson, S. J. (2004). Measurement of brain glutamate using TE-averaged PRESS at 3T. Magn. Reson Med. 51, 435–440. doi: 10.1002/mrm.20007
Kaiser, L. G., Schuff, N., Cashdollar, N., and Weiner, M. W. (2005). Age-related glutamate and glutamine concentration changes in normal human brain: 1H MR spectroscopy study at 4 T. Neurobiol. Aging 26, 665–672. doi: 10.1016/j.neurobiolaging.2004.07.001
Kierans, A. S., Kirov, I. I., Gonen, O., Haemer, G., Nisenbaum, E., Babb, J. S., et al. (2014). Myoinositol and glutamate complex neurometabolite abnormality after mild traumatic brain injury. Neurology 82, 521–528. doi: 10.1212/wnl.0000000000000105
Kontos, H. A., and Povlishock, J. T. (1986). Oxygen radicals in brain injury. Cent. Nerv. Syst. Trauma 3, 257–263. doi: 10.1007/978-3-642-73987-3_208
Kreis, R., Ross, B. D., Farrow, N. A., and Ackerman, Z. (1992). Metabolic disorders of the brain in chronic hepatic encephalopathy detected with H-1 MR spectroscopy. Radiology 182, 19–27. doi: 10.1148/radiology.182.1.1345760
Lei, H., Berthet, C., Hirt, L., and Gruetter, R. (2009). Evolution of the neurochemical profile after transient focal cerebral ischemia in the mouse brain. J. Cereb. Blood Flow Metab. 29, 811–819. doi: 10.1038/jcbfm.2009.8
Lescot, T., Fulla-Oller, L., Po, C., Chen, X. R., Puybasset, L., Gillet, B., et al. (2010). Temporal and regional changes after focal traumatic brain injury. J. Neurotrauma 27, 85–94. doi: 10.1089/neu.2009.0982
Lin, A. P., Ramadan, S., Stern, R. A., Box, H. C., Nowinski, C. J., Ross, B. D., et al. (2015). Changes in the neurochemistry of athletes with repetitive brain trauma: preliminary results using localized correlated spectroscopy. Alzheimers Res. Ther. 7:13. doi: 10.1186/s13195-015-0094-5
Maher, P. (2005). The effects of stress and aging on glutathione metabolism. Ageing Res. Rev. 4, 288–314. doi: 10.1016/j.arr.2005.02.005
Michaelis, T., Merboldt, K. D., Bruhn, H., Hänicke, W., and Frahm, J. (1993). Absolute concentrations of metabolites in the adult human brain in vivo: quantification of localized proton MR spectra. Radiology 187, 219–227. doi: 10.1148/radiology.187.1.8451417
Murray, M. E., Przybelski, S. A., Lesnick, T. G., Liesinger, A. M., Spychalla, A., Zhang, B., et al. (2014). Early Alzheimer’s disease neuropathology detected by proton MR spectroscopy. J. Neurosci. 34, 16247–16255. doi: 10.1523/jneurosci.2027-14.2014
Nilsson, P., Hillered, L., Ponten, U., and Ungerstedt, U. (1990). Changes in cortical extracellular levels of energy-related metabolites and amino acids following concussive brain injury in rats. J. Cereb. Blood Flow Metab. 10, 631–637. doi: 10.1038/jcbfm.1990.115
Paban, V., Fauvelle, F., and Alescio-Lautier, B. (2010). Age-related changes in metabolic profiles of rat hippocampus and cortices. Eur. J. Neurosci. 31, 1063–1073. doi: 10.1111/j.1460-9568.2010.07126.x
Pellerin, L., and Magistretti, P. J. (1994). Glutamate uptake into astrocytes stimulates aerobic glycolysis: a mechanism coupling neuronal activity to glucose utilization. Proc. Natl. Acad. Sci. U. S. A. 91, 10625–10629. doi: 10.1073/pnas.91.22.10625
Petroff, O. A., Pleban, L. A., and Spencer, D. D. (1995). Symbiosis between in vivo and in vitro NMR spectroscopy: the creatine, N-acetylaspartate, glutamate and GABA content of the epileptic human brain. Magn. Reson. Imaging 13, 1197–1211. doi: 10.1016/0730-725x(95)02033-p
Pfeuffer, J., Tkàc, I., Provencher, S. W., and Gruetter, R. (1999). Toward an in vivo neurochemical profile: quantification of 18 metabolites in short-echo-time (1)H NMR spectra of the rat brain. J. Magn. Reson. 141, 104–120. doi: 10.1006/jmre.1999.1895
Popa-Wagner, A., Buga, A. M., and Kokaia, Z. (2011). Perturbed cellular response to brain injury during aging. Ageing Res. Rev. 10, 71–79. doi: 10.1016/j.arr.2009.10.008
Rae, C. D. (2014). A guide to the metabolic pathways and function of metabolites observed in human brain 1H magnetic resonance spectra. Neurochem. Res. 39, 1–36. doi: 10.1007/s11064-013-1199-5
Reyngoudt, H., Claeys, T., Vlerick, L., Verleden, S., Acou, M., Deblaere, K., et al. (2012). Age-related differences in metabolites in the posterior cingulate cortex and hippocampus of normal ageing brain: a 1H-MRS study. Eur. J. Radiol. 81, e223–e231. doi: 10.1016/j.ejrad.2011.01.106
Rice, M. E., and Russo-Menna, I. (1998). Differential compartmentalization of brain ascorbate and glutathione between neurons and glia. Neuroscience 82, 1213–1223. doi: 10.1016/s0306-4522(97)00347-3
Ross, B. D., Ernst, T., Kreis, R., Haseler, L. J., Bayer, S., Danielsen, E., et al. (1998). 1H MRS in acute traumatic brain injury. J. Magn. Reson. Imaging 8, 829–840. doi: 10.1002/jmri.1880080412
Rothermundt, M., Ohrmann, P., Abel, S., Siegmund, A., Pedersen, A., Ponath, G., et al. (2007). Glial cell activation in a subgroup of patients with schizophrenia indicated by increased S100B serum concentrations and elevated myo-inositol. Prog. Neuropsychopharmacol. Biol. Psychiatry 31, 361–364. doi: 10.1016/j.pnpbp.2006.09.013
Schuhmann, M. U., Stiller, D., Skardelly, M., Bernarding, J., Klinge, P. M., Samii, A., et al. (2003). Metabolic changes in the vicinity of brain contusions: a proton magnetic resonance spectroscopy and histology study. J. Neurotrauma 20, 725–743. doi: 10.1089/089771503767869962
Shao, C., Roberts, K. N., Markesbery, W. R., Scheff, S. W., and Lovell, M. A. (2006). Oxidative stress in head trauma in aging. Free Radic. Biol. Med. 41, 77–85. doi: 10.1016/j.freeradbiomed.2006.03.007
Sharrock, M., Brooks, W. M., Yeh, H. W. and Harris, J. L. (2015). “Proton Magnetic Resonance Spectroscopy Predictors of Tissue Loss After Traumatic Brain Injury,” in National Neurotrauma Symposium, (New Rochelle, NY: Mary Ann Liebert, Inc.), A-1–A-152. doi: 10.1089/neu.2015.29000.abstracts
Sofroniew, M. V., and Vinters, H. V. (2010). Astrocytes: biology and pathology. Acta Neuropathol. 119, 7–35. doi: 10.1007/s00401-009-0619-8
Strange, K., Emma, F., Paredes, A., and Morrison, R. (1994). Osmoregulatory changes in myo-inositol content and Na+/myo-inositol cotransport in rat cortical astrocytes. Glia 12, 35–43. doi: 10.1002/glia.440120105
Sun, X., Shih, A. Y., Johannssen, H. C., Erb, H., Li, P., and Murphy, T. H. (2006). Two-photon imaging of glutathione levels in intact brain indicates enhanced redox buffering in developing neurons and cells at the cerebrospinal fluid and blood-brain interface. J. Biol. Chem. 281, 17420–17431. doi: 10.1074/jbc.m601567200
Terpstra, M., Henry, P. G., and Gruetter, R. (2003). Measurement of reduced glutathione (GSH) in human brain using LCModel analysis of difference-edited spectra. Magn. Reson. Med. 50, 19–23. doi: 10.1002/mrm.10499
Thompson, H. J., Mccormick, W. C., and Kagan, S. H. (2006). Traumatic brain injury in older adults: epidemiology, outcomes and future implications. J. Am. Geriatr. Soc. 54, 1590–1595. doi: 10.1111/j.1532-5415.2006.00894.x
Trabesinger, A. H., and Boesiger, P. (2001). Improved selectivity of double quantum coherence filtering for the detection of glutathione in the human brain in vivo. Magn. Reson. Med. 45, 708–710. doi: 10.1002/mrm.1094
Urrila, A. S., Hakkarainen, A., Heikkinen, S., Vuori, K., Stenberg, D., Hakkinen, A. M., et al. (2004). Stimulus-induced brain lactate: effects of aging and prolonged wakefulness. J. Sleep Res. 13, 111–119. doi: 10.1111/j.1365-2869.2004.00401.x
Xu, S., Zhuo, J., Racz, J., Shi, D., Roys, S., Fiskum, G., et al. (2011). Early microstructural and metabolic changes following controlled cortical impact injury in rat: a magnetic resonance imaging and spectroscopy study. J. Neurotrauma 28, 2091–2102. doi: 10.1089/neu.2010.1739
Yokobori, S., Watanabe, A., Matsumoto, G., Onda, H., Masuno, T., Fuse, A., et al. (2011). Time course of recovery from cerebral vulnerability after severe traumatic brain injury: a microdialysis study. J. Trauma 71, 1235–1240. doi: 10.1097/ta.0b013e3182140dd7
Zhang, X., Wu, J., Liu, H., and Zhang, X. (2013). Age- and gender-related metabonomic alterations in striatum and cerebellar cortex in rats. Brain Res. 1507, 28–34. doi: 10.1016/j.brainres.2013.02.033
Keywords: proton magnetic resonance spectroscopy, astrocytes, traumatic brain injury (TBI), aging, myo-inositol, glutathione, glutamic acid, lactic acid
Citation: Harris JL, Choi I-Y and Brooks WM (2015) Probing astrocyte metabolism in vivo: proton magnetic resonance spectroscopy in the injured and aging brain. Front. Aging Neurosci. 7:202. doi: 10.3389/fnagi.2015.00202
Received: 30 April 2015; Accepted: 07 October 2015;
Published: 28 October 2015.
Edited by:
George E. Barreto, Pontificia Universidad Javeriana, ColombiaReviewed by:
Catarina Oliveira, University of Coimbra, PortugalJ. Arturo García-Horsman, University of Helsinki, Finland
Copyright © 2015 Harris, Choi and Brooks. This is an open-access article distributed under the terms of the Creative Commons Attribution License (CC BY). The use, distribution and reproduction in other forums is permitted, provided the original author(s) or licensor are credited and that the original publication in this journal is cited, in accordance with accepted academic practice. No use, distribution or reproduction is permitted which does not comply with these terms.
*Correspondence: Janna L. Harris, jharris2@kumc.edu