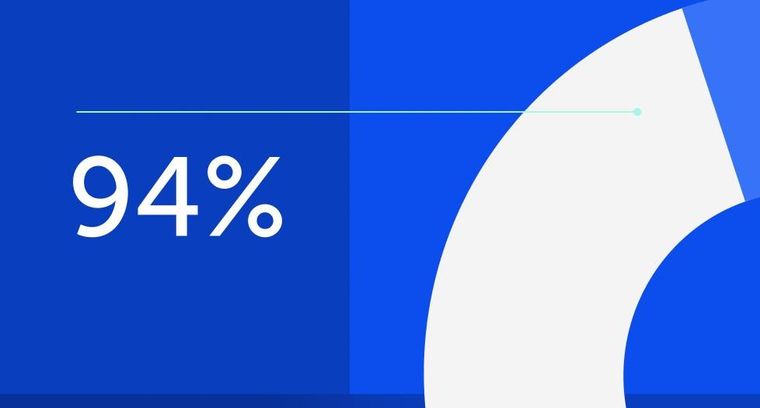
94% of researchers rate our articles as excellent or good
Learn more about the work of our research integrity team to safeguard the quality of each article we publish.
Find out more
MINI REVIEW article
Front. Aerosp. Eng., 09 September 2022
Sec. Aircraft Materials and Structures
Volume 1 - 2022 | https://doi.org/10.3389/fpace.2022.1002258
Structural energy storage composites, which combine energy storage capability with load-carrying function, are receiving increasing attention for potential use in portable electronics, electric vehicles, and aircraft structures to store electrical energy in replace of traditional electrochemical energy storage devices. The integration of energy storage ability into mechanically strong carbon fibre reinforced polymer composite is promising in reducing the weight and volume while providing additional functions, ultimately leading to energy-efficient systems. In this review, the key designs and strategies to reconcile the trade-off between mechanical properties and energy storage performances of structural dielectric capacitors, a typical type of structural energy storage composites, are highlighted. Opportunities and challenges are also discussed for the further development of structural energy storage composites for aviation applications.
The widespread usage of clean and sustainable energy ranging from built environment to transportation sectors underpins the global efforts to achieve carbon neutrality. In particular, the rapid development of high-performance lithium-ion (Li-ion) batteries in the past decade has spawned the fast adoption of electric vehicles (EVs), potentially replacing conventional fuel-burning cars as a greener transport solution. However, the range anxiety arising from the limited battery capacity becomes one of the most important barriers for the wide adoption of all-electric cars. Moreover, the bulky and heavy battery packs required to power the EVs also limit the range and payload. To extend the driving range of EVs, lightweight composite materials, especially carbon fibre reinforced polymer (CFRP) composites, have been used as the primary structural components owing to their high specific stiffness and strength. More importantly, the simultaneously high electrical conductivity and excellent mechanical properties of carbon fibres can be exploited as electrodes in an energy storage system, enabling the transformation of conventional CFRP composites into structural energy storage composites serving dual functionalities of structural reinforcement and energy storage. If the structural energy storage composites ultimately replace the batteries, a significant reduction in weight and volume can be achieved to improve the energy efficiency of the systems, potential for automotive and aviation applications (Carlstedt and Asp, 2020; Chan et al., 2020; Galos et al., 2020). However, the current state of the art has not yet satisfied the stringent requirements of structural performance, energy density, safety, and cost for aviation applications.
Research efforts in structural energy storage composites have been focused on the development of multifunctional energy storage composites, which serve as both load-carrying component and energy storage device simultaneously. They include structural dielectric capacitors (SDCs), structural supercapacitors (SSCs) and structural batteries (SBs) (Asp and Greenhalgh, 2014; Chan et al., 2018a; Danzi et al., 2021). Nevertheless, there is always a trade-off between load-bearing capability and energy storage performance, requiring more fundamental studies to optimize their overall performances. Although SBs have the highest energy density compared to SDCs and SSCs, their mechanical stability might not be good enough for aviation applications because of the potential formation of internal stress due to the volume expansion of carbon fibres during lithiation and delithiation (Jacques et al., 2013). In addition, the electrochemically active species in batteries can be depleted after only ∼10,000 cycles, necessitating replacement and thus increasing the long-term maintenance cost. Conventional SSCs require the use of liquid electrolytes for ion transport between two electrodes, not suitable for structural applications. Research efforts have been devoted to developing new solid polymer electrolytes that can fulfil both mechanical and electrical requirements (Shirshova et al., 2013). Nevertheless, such complicated structural material designs restrict the mass production of SSCs for commercialization. In comparison, SDCs are expected to have much better mechanical properties than SBs and SSCs owing to the absence of electrolytes despite a lower energy density. Furthermore, SDCs exhibit a high power density which are essential for emerging transportation systems such as EVs, drones, and electric vertical take-off and landing (eVTOL) aircraft requiring high power for acceleration (Chung, 2018). Existing reviews on multifunctional energy storage composites are mainly focused on SBs and SSCs (Danzi et al., 2021; Xu et al., 2021; Zhou et al., 2021; Zhou et al., 2022), while little attention has been paid to SDCs. Herein, we highlight the recent advances in the design and applications of SDCs, focusing on the key design strategies to reconcile the trade-off between mechanical properties and energy storage performances.
SDCs store the electrical energy in the form of electrostatic charges at electrically conductive CFRP electrodes separated by an insulating dielectric layer, as shown in Figure 1. It should be noted that the lateral size of dielectric layer should be at least 5 mm larger than CFRP electrodes to avoid the edge effects and contact between two carbon fabrics which can cause electric short-circuit (Carlson et al., 2010). Usually, SDCs are fabricated using vacuum assisted resin infiltration or hand layup process followed by curing under vacuum condition to remove the low-dielectric-constant air bubbles from the devices. Because no chemical conversion and ion transport are involved, SDCs exhibit high-power density, potential for accelerating EVs and eVTOL aircraft. To achieve high energy storage performance, a dielectric layer with high dielectric constant and breakdown strength is required (Figure 1A). In addition, CFRP electrodes need to be highly conductive to reduce the equivalent series resistance (ESR) for high energy storage efficiency (Figure 1B). Moreover, the dielectric layer should be fully conformable on the wavy surfaces of carbon fabrics in the CFRP electrodes to avoid air gaps at the interfaces (Figure 1C). In terms of structural applications, the intrinsic mechanical properties of dielectric layers as well as their interfacial interaction with CFRP electrodes are important design considerations to retain the high mechanical stability of SDCs. All in all, the electrical energy storage performance and mechanical properties of SDCs are determined by the design and properties of 1) the dielectric layer, 2) the CFRP electrodes, and 3) the interface between the two. These design parameters are discussed in detail below.
FIGURE 1. Schematics of the components of SDCs, and the keys to achieving high energy storage performance: (A) high dielectric polarization within the dielectric layer, (B) high electrical conductivity of CFRP electrodes, and (C) high conformation between electrodes and dielectrics to minimize the presence of air or polymer resin.
The dielectric layer is an electrically insulating film between conductive electrodes to store electrical energy through dielectric polarization (Figure 1A). The capacitance,
where
Dielectric constant. A high dielectric constant allows easy polarization under external electric fields, beneficial to a high capacitance of SDCs. Polymers usually exhibit relatively low dielectric constants of 2–10, leading to low specific capacitance per unit area (0.21—1.86 μF m−2) and low energy density (0.034—0.089 J g−1) of the resulting SDCs (Carlson et al., 2010). One effective strategy to improve the dielectric constants is the use of high-dielectric nano- and micro-fillers such as BaTiO3 and carbon-based fillers in polymer-based films by providing abundant interfaces for interfacial polarization, forming numerous micro-capacitor networks (Mao et al., 2010; Rahman et al., 2013). In addition, GO films were also used as dielectric layers in SDCs exhibiting much higher specific capacitance of 205.0 μF m−2 (Chan et al., 2018b; Chan et al., 2018c; Chan et al., 2021) compared to their polymer-based counterparts (0.21—1.86 μF m−2) (Carlson et al., 2010). This is because the high electronegativity of oxygen-containing functional groups enhanced the dipolar polarization, resulting in high dielectric constants of 900—2075 for GO films.
Breakdown strength. The energy density of SDCs is directly proportional to the square of dielectric breakdown strength according to Eq. 2. Therefore, increasing the breakdown strength of dielectric layer could be effective in improving the energy density of SDCs significantly. Although the introduction of nano- and micro-fillers enhanced the dielectric constants of nanocomposites, the breakdown strength might even decrease because of the low breakdown strength of fillers and the formation of conductive networks. To mitigate the breakdown strength reduction due to nanofillers, one possible solution is to introduce a buffer layer on the surface of conductive fillers (Yang et al., 2014). In addition to polymer composite films, the GO film exhibited an excellent dielectric breakdown strength due to the presence of oxygen-containing functional groups that hinder the charge transport in the sp2 network, making GO an excellent insulator to supress the leakage current and achieve good breakdown strength (Wu et al., 2013).
Mechanical properties. The intrinsic mechanical properties of dielectric layers are important for the overall strengths and stiffnesses of SDCs. GO films are good candidates for dielectric layers because of their excellent intrinsic mechanical properties. Incorporating a GO film dielectric into CFRP electrodes to build an SDC only marginally reduced the tensile modulus and strength of the CFRP by 0.4 and 6.3%, respectively (Chan et al., 2018c). However, delamination cracks within the GO film were found under interlaminar shear loads. Further improving the interlayer strengths between GO sheets was obtained by chemically crosslinking GO using polyallylamine (PAA) (Chan et al., 2020). The PAA-modified GO imparted a 27% higher interlaminar shear strength of the SDC made therefrom against that of an unmodified sample, significantly reducing the possibility of shear-induced delamination. On the other hand, the chemical crosslinking restricted the orientation of polar functional groups, reducing the capacitance and energy density of the SDC. By contrast, a significant reduction of 35% in the tensile modulus was observed when a PET film with thickness of 125 µm was used as the dielectric layer (Carlson and Asp, 2013). This was because the PET film was much softer than the CFRP composite. Nano- and micro-fillers for improving the dielectric constant could potentially improve the mechanical properties of the resultant nanocomposites because of the strong and stiff fillers (Li et al., 2019; Ahmed et al., 2021). However, the dispersion and agglomeration issues always led to inferior properties when a large amount of fillers were used (Zare, 2016). Therefore, it is rather challenging to achieve both dielectric and mechanical properties in the same dielectric layer while maintaining good interfacial adhesion between dielectric and CFRP layers, necessitating the development of novel strategies.
Achieving simultaneous dielectric and mechanical properties. The foregoing discussion indicates that it is rather challenging to achieve both dielectric and mechanical properties in the same dielectric layer while maintaining good interfacial adhesion between dielectric and CFRP layers. To improve the overall performance of SDCs, novel strategies have been developed by tailoring the chemical compositions of dielectric layers. A recent effort was made to investigate the effects of chemical composition of GO on enhancing both mechanical and dielectric properties of the resulting film for SDC applications (Chan et al., 2021). The mechanical and dielectric properties of GO films were closely related to the carbon-to-oxygen atomic ratio. It is well known that the high dielectric constant of GO arises from the abundant oxygen-containing functional groups because of the extensive dipolar polarization. Interestingly, the partial elimination of functional groups through a mild chemical reduction created vacancy defects in GO, leading to the local redistribution of positive and negative charges and forming extra dipole moments to further increase the dielectric constant. Nevertheless, the electrical conductivity of the GO film increased significantly after excessive reduction, making them unsuitable for dielectric layers in SDCs. Furthermore, the removal of functional groups restored the sp2 carbon bonds in GO, leading to improved reduced elastic modulus and hardness of the film. The work demonstrated that both mechanical and dielectric properties of GO films were enhanced simultaneously through a mild reduction whilst retaining the electrical insulating nature of GO, resulting in improved overall performance of SDCs.
In summary, polymer composite and GO films are two commonly used dielectric layers to improve both the mechanical and dielectric properties of SDCs. Generally, nano- and micro-fillers can act as reinforcement in polymer composites to enhance the structural performance when added in small amounts. The introduction of high-dielectric-constant fillers such as BaTiO3, graphene, and MWCNTs into polymer films can induce interfacial polarization and formation of micro-capacitors, improving the capacitive performance of SDCs. Nevertheless, the high capacitance could lead to deteriorated dielectric breakdown strength. Conventional two-phrase composites might not be able to achieve both high dielectric constant and breakdown strength simultaneously. Novel three-phase composites (Shen et al., 2013; Yang et al., 2014) need to be developed as new dielectric materials to optimize the overall performance of SDCs. Alternatively, tuning the chemical composition of GO film by controlling the reduction degree led to both high dielectric and mechanical properties (Chan et al., 2021), making it an ideal dielectric layer for SDCs.
In addition to the dielectric layer, the properties of CFRP electrodes should also be optimized to improve the electrical conductivity without sacrificing the excellent mechanical properties of CFRP. A high electrical conductivity reduces the ESR, which is the major bottleneck limiting the power density and capacity of energy storage devices. Due to the presence of insulating epoxy matrix (10–12 S m−1) between fibres (Figure 1B), the electrical conductivity of CFRP (∼700 S m−1) is much lower compared to metal-based electrodes (Jia et al., 2014; Chan et al., 2019). To achieve high electrical conductivity of CFRP composite, conductive polymers such as polyaniline can be used instead of epoxy (Salinas-Torres et al., 2013; Hirano et al., 2016). However, the mechanical properties of SDCs might not be as good as those using epoxy as the matrix. To simultaneously improve the electrical conductivity and mechanical properties of CFRP electrodes, one common strategy was incorporating conductive nanoparticles, such as metal and carbon nanofillers, in the epoxy matrix, so that conduction paths were created between fibres (Moisala et al., 2006; Chan et al., 2019). It should be noted that the highly conductive resins can only be used to prepare CFRP prepregs after removing the sizing on the surfaces of carbon fibres, while insulating resins have to be used to bind different capacitive layers together to prevent any electric short-circuit.
The interfacial properties between the CFRP electrodes and dielectric layer also play an important role in determining the mechanical and dielectric properties of SDCs. Delamination between CFRP electrodes and dielectric layers is the most common failure mode for SDCs under mechanical loadings, especially shear and tension (Carlson et al., 2010; Chan et al., 2018c). The interfacial interactions between dielectric layers and CFRP electrodes were enhanced by forming strong covalent bonds through creating functional groups on the surface of dielectric layers, significantly improving the interlaminar shear strength of the SDCs (Carlson et al., 2010). In terms of dielectric properties, common CFRP electrodes were made from carbon woven fabrics containing wavy carbon tows, giving rise to a larger total surface area than that of the unidirectional fibre mat having straight fibres. The presence of micro-sized pores between fibres due to the waviness improved the capacitance by forming a conformable interface with the dielectric layer (Figure 1C). In view of the above, an excellent flexibility of the dielectric layer is essential to ensure their good conformation in the interface region without increasing the separation between the two electrodes (Chung, 2018).
Among all structural energy storage composites, SDCs exhibit the best mechanical performance because of the absence of electrolyte. Although solid polymer electrolytes have been developed for SBs and SSCs, the presence of ionic liquids could affect the crystallinity of polymer matrix, reducing their mechanical properties (Demir et al., 2020). The use of ionic liquid also restricts the working voltage of SBs and SSCs to below 4 V, after which the decomposition of electrolyte might occur (Demir and Searles, 2020; Sharma and Kumar, 2020). The large-scale fabrication of SBs is also challenging because of the high reactivity of lithium, requiring a controlled environment such as glove box for the assembly of SBs (Johannisson et al., 2018). In addition, the service life of SBs is limited by the depletion of active species on the electrodes which takes much shorter time than the common service life of composite structures in aircraft, making them not economical for long-term applications. Despite the lower energy density of SDCs than SSCs and SBs, the broader working voltage allowed SDCs to be used in UAVs, more-electric-aircraft, and military aircraft, in which the most frequently applied voltages onboard ranged from 115 to 540 V (Wheeler and Bozhko, 2014; Alexander et al., 2018). In this case, there was no need to connect SDCs in series, resulting in a lower equivalent capacitance to efficiently provide sufficient voltage for onboard applications. Besides, the fast charge and discharge rates made SDCs superior in applications requiring high power density. SDCs can also be integrated with sustainable energy to supply power to UAV and aircraft avionics. For instance, a GO-based SDC was developed to store the electrostatic energy harvested from the friction between air and aircraft surfaces to power the navigation lights of the aircraft, reducing the total electrical load, as shown in Figure 2.
FIGURE 2. (A) Schematic showing SDCs to harvest and store the electrostatic energy from the environment directly in the body of aircraft. The generation of negative charges due to friction between the external surface of aircraft and air flow and formation of potential differences in aircraft caused electron flow. (B) Electric circuit used to demonstrate the GO-based SDC for powering navigation lights. (C) Voltage changes across the GO-based SDC (stage A→B: capacitor charging; stage B: Neon gas ionizes; stage B→C: capacitor discharging). (D) Images of neon light at different stages. Reproduced from ref (Chan et al., 2020). with permission. Copyright Elsevier 2020.
In summary, the unique combination of energy storage ability and load-carrying function in a single SDC shows great potential in developing next-generation energy-efficient systems. Research progress has been made to enhance both mechanical and energy storage performance of SDCs by using polymer nanocomposite and GO films as dielectric layers, and modifying the CFRP electrodes and interfacial properties. Although SDCs have great potential in automotive and aviation applications, more work should be done to demonstrate their usability beyond a few existing prototypes. The SDCs could eventually be integrated with renewable energy such as solar energy to provide totally green power for aviation and other industries, reducing the dependency on fossil fuels and thus leading to a sustainable future.
All authors involve the discussion on proposed ideas of this manuscript.
This project was financially supported by the Research Grants Council (GRF Projects: 16205517, 16200720) and start-up fund for new recruits of PolyU (P0038855, P0038858).
The authors declare that the research was conducted in the absence of any commercial or financial relationships that could be construed as a potential conflict of interest.
All claims expressed in this article are solely those of the authors and do not necessarily represent those of their affiliated organizations, or those of the publisher, the editors and the reviewers. Any product that may be evaluated in this article, or claim that may be made by its manufacturer, is not guaranteed or endorsed by the publisher.
Ahmed, R., Ibrahiem, A. A., El-Bayoumi, A. S., and Atta, M. M. (2021). Structural, mechanical, and dielectric properties of polyvinylchloride/graphene nano platelets composites. Int. J. Polym. Analysis Charact. 26 (1), 68–83. doi:10.1080/1023666x.2020.1845493
Alexander, R., Meyer, D., and Wang, J. “A comparison of electric vehicle power systems to predict architectures, voltage levels, power requirements, and load characteristics of the future all-electric aircraft,” in 2018 IEEE Transportation Electrification Conference and Expo (ITEC), Long Beach, CA, USA, 13-15 June 2018 (IEEE).
Asp, L. E., and Greenhalgh, E. S. (2014). Structural power composites. Compos. Sci. Technol. 101, 41–61. doi:10.1016/j.compscitech.2014.06.020
Bai, Y., Cheng, Z. Y., Bharti, V., Xu, H. S., and Zhang, Q. M. (2000). High-dielectric-constant ceramic-powder polymer composites. Appl. Phys. Lett. 76 (25), 3804–3806. doi:10.1063/1.126787
Carlson, T., and Asp, L. E. (2013). Structural carbon fibre composite/PET capacitors–Effects of dielectric separator thickness. Compos. Part B Eng. 49, 16–21. doi:10.1016/j.compositesb.2013.01.009
Carlson, T., Ordeus, D., Wysocki, M., and Asp, L. E. (2010). Structural capacitor materials made from carbon fibre epoxy composites. Compos. Sci. Technol. 70 (7), 1135–1140. doi:10.1016/j.compscitech.2010.02.028
Carlstedt, D., and Asp, L. E. (2020). Performance analysis framework for structural battery composites in electric vehicles. Compos. Part B Eng. 186, 107822. doi:10.1016/j.compositesb.2020.107822
Chan, K.-Y., Baktash, A., Demir, B., Mayes, E. L., Yang, D., Pham, D. Q., et al. (2021). Tailoring mechanical and electrical properties of graphene oxide film for structural dielectric capacitors. J. Power Sources 482, 229020. doi:10.1016/j.jpowsour.2020.229020
Chan, K.-Y., Jia, B., Lin, H., Hameed, N., Lee, J. H., and Lau, K. T. (2018). A critical review on multifunctional composites as structural capacitors for energy storage. Compos. Struct. 188, 126–142. doi:10.1016/j.compstruct.2017.12.072
Chan, K.-Y., Jia, B., Lin, H., Zhu, B., and Lau, K. T. (2018). Design of a structural power composite using graphene oxide as a dielectric material layer. Mater. Lett. 216, 162–165. doi:10.1016/j.matlet.2017.12.118
Chan, K.-Y., Lin, H., Qiao, K., Jia, B., and Lau, K. T. (2018). Multifunctional graphene oxide paper embodied structural dielectric capacitor based on carbon fibre reinforced composites. Compos. Sci. Technol. 163, 180–190. doi:10.1016/j.compscitech.2018.05.027
Chan, K.-Y., Pham, D. Q., Demir, B., Yang, D., Mayes, E. L., Mouritz, A. P., et al. (2020). Graphene oxide thin film structural dielectric capacitors for aviation static electricity harvesting and storage. Compos. Part B Eng. 201, 108375. doi:10.1016/j.compositesb.2020.108375
Chan, K.-Y., Yang, D., Demir, B., Mouritz, A. P., Lin, H., Jia, B., et al. (2019). Boosting the electrical and mechanical properties of structural dielectric capacitor composites via gold nanoparticle doping. Compos. Part B Eng. 178, 107480. doi:10.1016/j.compositesb.2019.107480
Chung, D. (2018). Development, design and applications of structural capacitors. Appl. Energy 231, 89–101. doi:10.1016/j.apenergy.2018.09.132
Danzi, F., Salgado, R. M., Oliveira, J. E., Arteiro, A., Camanho, P. P., and Braga, M. H. (2021). Structural batteries: A review. Molecules 26 (8), 2203. doi:10.3390/molecules26082203
Demir, B., and Searles, D. J. (2020). Investigation of the ionic liquid graphene electric double layer in supercapacitors using constant potential simulations. Nanomaterials 10 (11), 2181. doi:10.3390/nano10112181
Demir, B., Chan, K.-y., and Searles, D. J. (2020). Structural electrolytes based on epoxy resins and ionic liquids: A molecular-level investigation. Macromolecules 53 (18), 7635–7649. doi:10.1021/acs.macromol.0c00824
Galos, J., Best, A., and Mouritz, A. (2020). Multifunctional sandwich composites containing embedded lithium-ion polymer batteries under bending loads. Mater. Des. 185, 108228. doi:10.1016/j.matdes.2019.108228
Guo, F., Shen, X., Zhou, J., Liu, D., Zheng, Q., Yang, J., et al. (2020). Highly thermally conductive dielectric nanocomposites with synergistic alignments of graphene and boron nitride nanosheets. Adv. Funct. Mat. 30 (19), 1910826. doi:10.1002/adfm.201910826
Hirano, Y., Yokozeki, T., Ishida, Y., Goto, T., Takahashi, T., Qian, D., et al. (2016). Lightning damage suppression in a carbon fiber-reinforced polymer with a polyaniline-based conductive thermoset matrix. Compos. Sci. Technol. 127, 1–7. doi:10.1016/j.compscitech.2016.02.022
Jacques, E., Hellqvist Kjell, M., Zenkert, D., Lindbergh, G., and Behm, M. (2013). Expansion of carbon fibres induced by lithium intercalation for structural electrode applications. Carbon 59, 246–254. doi:10.1016/j.carbon.2013.03.015
Jia, J., Sun, X., Lin, X., Shen, X., Mai, Y. W., and Kim, J. K. (2014). Exceptional electrical conductivity and fracture resistance of 3D interconnected graphene foam/epoxy composites. ACS Nano 8 (6), 5774–5783. doi:10.1021/nn500590g
Johannisson, W., Ihrner, N., Zenkert, D., Johansson, M., Carlstedt, D., Asp, L. E., et al. (2018). Multifunctional performance of a carbon fiber UD lamina electrode for structural batteries. Compos. Sci. Technol. 168, 81–87. doi:10.1016/j.compscitech.2018.08.044
Li, Y., Tian, X., Yang, W., Li, Q., Hou, L., Zhu, Z., et al. (2019). Dielectric composite reinforced by in-situ growth of carbon nanotubes on boron nitride nanosheets with high thermal conductivity and mechanical strength. Chem. Eng. J. 358, 718–724. doi:10.1016/j.cej.2018.10.004
Mao, Y., Mao, S. Y., Ye, Z. G., Xie, Z. X., and Zheng, L. S. (2010). Size-dependences of the dielectric and ferroelectric properties of BaTiO 3/polyvinylidene fluoride nanocomposites. J. Appl. Phys. 108 (1), 014102. doi:10.1063/1.3443582
Moisala, A., Li, Q., Kinloch, I., and Windle, A. (2006). Thermal and electrical conductivity of single-and multi-walled carbon nanotube-epoxy composites. Compos. Sci. Technol. 66 (10), 1285–1288. doi:10.1016/j.compscitech.2005.10.016
O'Brien, D., Baechle, D., and Wetzel, E. (2011). Design and performance of multifunctional structural composite capacitors. J. Compos. Mater. 45 (26), 2797–2809. doi:10.1177/0021998311412207
Rahman, M. A., Lee, B. C., Phan, D. T., and Chung, G. S. (2013). Fabrication and characterization of highly efficient flexible energy harvesters using PVDF–graphene nanocomposites. Smart Mat. Struct. 22 (8), 085017. doi:10.1088/0964-1726/22/8/085017
Salinas-Torres, D., Sieben, J., Lozano-Castello, D., Cazorla-Amoros, D., and Morallon, E. (2013). Asymmetric hybrid capacitors based on activated carbon and activated carbon fibre–PANI electrodes. Electrochimica Acta 89, 326–333. doi:10.1016/j.electacta.2012.11.039
Sharma, P., and Kumar, V. (2020). Current technology of supercapacitors: A review. J. Electron. Mat. 49 (6), 3520–3532. doi:10.1007/s11664-020-07992-4
Shen, Y., Guan, Y., Hu, Y., Lei, Y., Song, Y., Lin, Y., et al. (2013). Dielectric behavior of graphene/BaTiO3/polyvinylidene fluoride nanocomposite under high electric field. Appl. Phys. Lett. 103 (7), 072906. doi:10.1063/1.4818763
Shirshova, N., Bismarck, A., Carreyette, S., Fontana, Q. P. V., Greenhalgh, E. S., Jacobsson, P., et al. (2013). Structural supercapacitor electrolytes based on bicontinuous ionic liquid–epoxy resin systems. J. Mat. Chem. A Mat. 1 (48), 15300–15309. doi:10.1039/c3ta13163g
Wheeler, P., and Bozhko, S. (2014). The more electric aircraft: Technology and challenges. IEEE Electrific. Mag. 2 (4), 6–12. doi:10.1109/mele.2014.2360720
Wu, C., Huang, X., Wu, X., Xie, L., Yang, K., and Jiang, P. (2013). Graphene oxide-encapsulated carbon nanotube hybrids for high dielectric performance nanocomposites with enhanced energy storage density. Nanoscale 5 (9), 3847–3855. doi:10.1039/c3nr00625e
Wu, Y., Wang, Z., Shen, X., Liu, X., Han, N. M., Zheng, Q., et al. (2018). Graphene/boron nitride–polyurethane microlaminates for exceptional dielectric properties and high energy densities. ACS Appl. Mat. Interfaces 10 (31), 26641–26652. doi:10.1021/acsami.8b08031
Xu, Y., Lu, W., Xu, G., and Chou, T. W. (2021). Structural supercapacitor composites: A review. Compos. Sci. Technol. 204, 108636. doi:10.1016/j.compscitech.2020.108636
Yang, L., Qiu, J., Ji, H., Zhu, K., and Wang, J. (2014). Enhanced dielectric and ferroelectric properties induced by TiO2@ MWCNTs nanoparticles in flexible poly (vinylidene fluoride) composites. Compos. Part A Appl. Sci. Manuf. 65, 125–134. doi:10.1016/j.compositesa.2014.06.006
Zare, Y. (2016). Study of nanoparticles aggregation/agglomeration in polymer particulate nanocomposites by mechanical properties. Compos. Part A Appl. Sci. Manuf. 84, 158–164. doi:10.1016/j.compositesa.2016.01.020
Zhou, H., Li, H., Li, L., Liu, T., Chen, G., Zhu, Y., et al. (2022). Structural composite energy storage devices—a review. Mater. Today Energy 24, 100924. doi:10.1016/j.mtener.2021.100924
Keywords: aviation and aerospace, smart structure, structural dielectric capacitors, carbon fibre reinforced polymers, multifunctional composites, renewable energy
Citation: Chan K-Y, Demir B, Lau K-T and Shen X (2022) Structural energy storage composites for aviation applications. Front. Aerosp. Eng. 1:1002258. doi: 10.3389/fpace.2022.1002258
Received: 24 July 2022; Accepted: 26 August 2022;
Published: 09 September 2022.
Edited by:
Rosario Pecora, University of Naples Federico II, ItalyReviewed by:
Yingjing Liang, Guangzhou University, ChinaCopyright © 2022 Chan, Demir, Lau and Shen. This is an open-access article distributed under the terms of the Creative Commons Attribution License (CC BY). The use, distribution or reproduction in other forums is permitted, provided the original author(s) and the copyright owner(s) are credited and that the original publication in this journal is cited, in accordance with accepted academic practice. No use, distribution or reproduction is permitted which does not comply with these terms.
*Correspondence: Xi Shen, eGkuc2hlbkBwb2x5dS5lZHUuaGs=
Disclaimer: All claims expressed in this article are solely those of the authors and do not necessarily represent those of their affiliated organizations, or those of the publisher, the editors and the reviewers. Any product that may be evaluated in this article or claim that may be made by its manufacturer is not guaranteed or endorsed by the publisher.
Research integrity at Frontiers
Learn more about the work of our research integrity team to safeguard the quality of each article we publish.