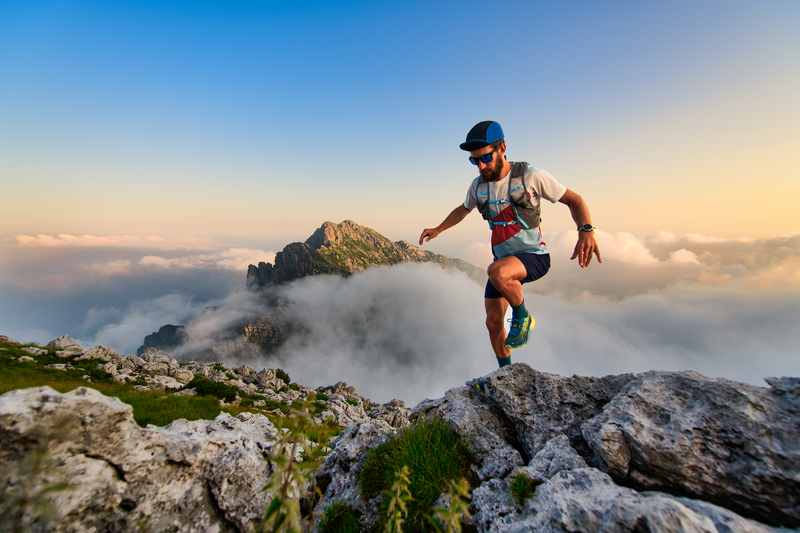
95% of researchers rate our articles as excellent or good
Learn more about the work of our research integrity team to safeguard the quality of each article we publish.
Find out more
PERSPECTIVE article
Adv. Opt. Technol. , 11 July 2023
Sec. Optical Manufacturing and Design
Volume 12 - 2023 | https://doi.org/10.3389/aot.2023.1237524
Ultrafast lasers are now unanimously recognized as processing tools capable of providing utmost precision. This becomes key in the context of material processing as precise feature scales can render a range of new characteristics to the processed materials. These features redesign their properties optically, mechanically, electrically, or from a chemical point of view. Precision is often accompanied by an increase in resolution. The advances in optical beam engineering and irradiation strategies, alongside with controlled material responses, have put in sight the opportunity to reach record small feature sizes, below 100 nm. Is there an intrinsic limit to optical fabrication? What are the new opportunities provided by laser processing on these scales? How one can make light adapt to matter and at the same time control the matter’s response under light on the smallest scales? In this article I intend to provide a brief overview into the latest developments in ultrafast laser volume nanostructuring, fundamentals and applications alike, stressing out the prospective roadmap and the new potential emerging from super-resolved ultrafast smart laser processing technologies.
Structured materials are ubiquitous, as structuring boosts their functional behavior. Among the present structuring technologies, laser structuring aims to reshape materials properties by changing their topography or their morphology on surfaces and within their volumes upon remote interaction with intense light. Light determines material transformation and, as such, the material characteristics evolve. The resulting property tuning is scale-dependent. Consequently, according to the feature scale, a range of phenomena can be triggered in the structured material that either enhances its natural properties or defines a completely new type of characteristics “by design.” At this point a new class of lasers intervenes, the ultrashort pulsed lasers, with an enhanced capacity to render precision to the process. If micromachining shows already substantial maturity that favors the uptake in industrial processes, smaller scales are highly desirable. They pertain particularly to precision applications ranging from cutting and polishing to shaping and structuring. In this context the sub-100 nm scale becomes a generic keystone for engineered materials, subject to an increasing number of studies (Cheng and Sugioka, 2013; Stoian and Bonse, 2023), as this scale is compatible with a range of transport phenomena and the associated mean free path in thermal, contact, or optical processes. In other words, a material showing densely packed structured features in the sub-100 nm range will be able to acquire a capacity to modify its thermal, electrical, or optical characteristics, quasi-resonantly filtering and guiding energy transport. Depending on scale, shape, and arrangement, individual and collective effects can be induced by these structured features. They trigger phenomena at the border between quantum and mesoscopic physics, unleashing new potential. The enhanced engineered properties on the nanoscale pave the way towards metamaterials engineering by light. Harnessing the properties of ultrafast light, the material design can occur in a variety of geometries and spatial arrangements. The ability of ultrashort pulses to cross interfaces and to localize energy within volumes by exploiting excitation nonlinearities associated to high intensity puts forward a clear perspective for single step volume three dimensional material engineering that has currently no viable alternative. In this article, highlighting the major milestones in laser volume nanostructuring, I aim to underline a unique capability of ultrashort laser pulses to generate structural features in 3D, way beyond the standard scale of optical beams, suggesting at the same time the relevant physical drivers as well as the potential applications.
The prospect of high resolution triggered an extraordinary quest to further gain in precision and structuring accuracy, deep into the nanoscale. Super-resolution became a mainstream objective and pertains to processes on surfaces, in the bulk, or in additive manufacturing. Notwithstanding, the discussion here focuses mainly on processing bulk monolithic materials. Being the result of directed optical energy beams, precise laser processing poses two main challenges; the strong spatial confinement of the irradiation zone and the restraint of the diffusion of the deposited energy. Properly controlled, the two of them can render a highly local character to the laser interaction process, keeping the transformation region to a minimal extent. How small this can be and where may one anticipate the resolution limits? How light and matter can synergistically concur to achieve the highest precision? In the resolution quest, a major control knob is the pulse duration, with two essential levers. On one side, the high intensity takes advantage of the nonlinearity of interaction. That restricts the volume of excitation around the intensity peak, squeezing in space the absorption profile. The process initiates excitation mechanisms involving the simultaneous absorption of multiple photons, restricting the interaction regions both on surfaces and in the bulk. Secondly, the short interaction time enables a fast process, consuming the energy before being diffused away in thermal and mechanical forms. The fast process can be ablative, i.e., involving a swift, strongly nucleated transition to the gas phase, or be mechanical, shock-based, explosion-like. The neighboring zones are minimally affected by residual diffusion, as much of the energy was already consumed. This observation pertains to surface as well as, or even more, to volume interactions. In the latter case, in addition, the challenge of the light wavepacket propagation should be considered, pushing up innovative concepts of space-time engineering of light (Stoian et al., 2010; He et al., 2014). These developments allow to restrict the process, linearly or nonlinearly, to its intrinsic optical limits, However, in case of optical interactions, resolution limits may apply, and a significant effort is put into bypassing them. With a far-field optical interaction being intrinsically limited by diffraction to roughly half of the wavelength, going beyond diffraction limit requires a more elaborate approach. In most of the cases this implies a closer and local interaction between the optical field and the material. Using external sharp objects and apertures, it was early recognized (Korte et al., 1999) that a near-field interaction can drastically reduce the size of the interaction region. Not being subject of far-field diffraction limitations, the evanescent components can squeeze the interaction region below the wavelength. Nonetheless, generating near-fields by external means may seem laborious and limited to surfaces or to specific environments. Therefore new approaches emerge exploiting near-field components induced by intense laser fields themselves (Li et al., 2020; Lei et al., 2021) which can be in principle applied at surfaces and below. This comes with two specific advantages. The first relates to the confinement of light on extreme scales without intrinsic limitations. This extreme confinement will initiate material transformation on similar or even smaller scales. Feature sizes well below 100 nm were demonstrated (Lei et al., 2021). The second, based on an internal mechanism of scattering, implies a dependence on the direction of the optical field that allows for directional control during direct laser writing. The interaction zone can be spatially guided, squeezed depending on the local field interaction, with electrical field adding as well to the energy transport (Li et al., 2020; Lei et al., 2021). This particular aspect on localizing light within a material (Gamaly and Rode, 2016) could be at the origin of complex laser processing strategies driving local anisotropies by light and heat coupling (Yang et al., 2008).
An alternative strategy for extreme processing involves a material response under light relying on sharp transitions of the excited matter, the so-called “optics at critical intensity”, in the range of the breakdown threshold (Joglekar et al., 2004). Close to the breakdown threshold, electronic excitation determines swift variations of the optical properties, tuning the absorption in shape and depth. A critical amount of energy is deposited in a confined region, enough to generate local sub-wavelength ablation of the excited material. This excitation, via electronic relaxation, can simply imply rapidly heated matter relaxing under pressure (Juodkazis et al., 2006), or a more complex situation where screened molecular potentials will enable a fast non-thermally-driven decomposition of matter (Jeschke et al., 2001). The process illustrates a capability of a material destabilized by light to show a reaction on scales smaller than the extent of the illumination zone. The gradient of light energy becomes in this case the critical parameter as it determines the material evolution. An evolution under strong gradients of energy can be reduced to a spatially limited source that develops under thermomechanical constraints, being brought down to scales disconnected from the incident wavelength. A first example pointing in this direction was given by the concept of micro-explosion (Glezer and Mazur, 1997), one of the first direct demonstration of bulk processing with ultrashort laser pulses. Disconnecting the size of modification from the optical dimension represents thus a genuine change of paradigm. Either relying on thresholding (Joglekar et al., 2004) or on generating nanocavitation (Bhuyan et al., 2017), this approach implies a restricted movement of matter under thermomechanical drives in a range several times smaller than the exciting wavelength, rapidly quenched by diffusion (Grady, 1988). The required energy gradients can be obtained by engineering the beam in space and time (Stoian et al., 2010; He et al., 2014; Salter and Booth, 2019), sometimes with tradeoffs between far and near field. One particularly efficient example has newly come into attention; high cone-angle non-diffractive beams for extreme nanostructuring with high aspect ratio (Courvoisier et al., 2009). Several examples were recently reviewed in Xu et al. (2021) and Stoian and Bonse (2023). As the final result is an extremely reduced processing feature, it is expectable that, in order to trigger nanoscale effects, both optical and material evolutions are strongly interrelated. Single sub-wavelength features illustrated above are already a convincing proof of the synergistic interaction between field confinement and material reactions. This interrelation is illustrated in Figure 1. The relation can acquire even more complex forms. A powerful example showing this intrication relates to the process of self-organisation under light and the quasi-spontaneous emergence of periodic patterns with subwavelength periodicity (Shimotsuma et al., 2003).
FIGURE 1. Intrication of light and matter, combining field confinement and matter evolution. Scanning electron microscopy image, courtesy of G. Zhang, Northwestern Polytechnical University, Xi’An.
This case, that equally combines light and matter reactions, has an underlying physics which is different from the direct structuring approaches mentioned above, based on single spot irradiation events or small number of accumulated pulses. Starting from a collection of stochastically-arranged scattering centers that diffuse light, corrugated matter determines first distributed light patterns through a coherent superposition of near-field and far-field scattered components (Rudenko et al., 2021). The patterns push the excited matter to rearrange. We are talking about self-organisation, where, as in a self-action, the process correlates self-consistently external light stimuli and material transport mechanisms as well as thermodynamic evolutions. It thus determines, with every incoming pulse, a gradual evolution towards order in the arrangement of the structures. The tendency towards order does not stop at the mesoscopic scale but acts on a variety of scales. These are related to local interference patterns and fluid flow. Furthermore, dissipative self-organisation effects (Prigogine, 1978) mixing field and heat transport can push the scale to the nm level. The transition to order can also be revealed at molecular scales as localized phase transitions can generate nanoscale crystallites in previously amorphous glassy materials either by precipitation, by gradual cooling of liquid phases (Fan et al., 2012; Sun et al., 2022), or by initiating dense structural phases by extreme thermomechanical shock-like conditions (Vailionis et al., 2011). The combination of light-induced bond-breaking and shock defines nm-scale ranges of structurally dense matter embedded in material matrices. The behavior exposed by the mixture of near-field evanescent optics and thermomechanical material reactions show that in principle no intrinsic spatial limits can restrict the process except the size of the energy deposition range. This can eventually be reduced in three dimensions to the skin depth, i.e., a few nm (Joglekar et al., 2004). The observed feature scales are related to practical limitations, for example, the strength of confinement of the evanescent field in the presence of dephasing electronic excitation or the quenching time freezing the material evolution. Besides single feature dimensions, in many cases, the process resolution and the close-packing of the structures on the same scale relate to intrinsic artifacts associated to thermomechanics in the form of mechanically affected regions around the features of interest (Zhang et al., 2021). Part of energy diffuses away from the interaction region as pressure or heat fronts and creates material constraints that pose limitation to generating new structures in the affected regions. A judicious choice of pulse parameters will minimize the effect confining it to a minimal extent of few 100 nm. Structural modifications within or outside the interaction region can boost the nanoscale. In particular cases, the addition of chemical etching will give further spatial selectivity in revealing volume nanoscale structures (Ròdenas et al., 2019). If in principle similar phenomena rule surface and volume interaction which gives a generic character to the description above, I will focus below on specific emerging fields related to volume 3D interactions.
This scale between 10 and 100 nm can now be seen as an enabling scale, helping to construct functions in various fields, from photonics to nanofluidics, from nanomechanics to biology and material science. Photonic systems fabricated by ultrafast laser refractive index engineering in monolithic optical materials have gained in the last years a significant interest. The prospect of the nanoscale will further boost their functional design. This interest resulted from an intrinsic phase stability and from three-dimensional geometries with high collection efficiencies and low cross-talk. They offer the opportunity to transport and transform light in embedded optical circuits of arbitrary forms. To this, nanoscale features can add a set of new functionalities, both local and non-locally defined. They can engineer the dielectric function on local domains and its distribution in space, generating functional design resulting from light interaction with the nanoscale. Single features can be placed in 1D to 3D arrays with complex arrangements that can enable optical resonances and sudden phase jumps. A single nanoscale feature with a size much smaller than the wavelength can almost unperturbatively scatter light according to its size and shape. Grouping spatially individual features, resonant effects may be induced, exploiting the coherence of optical signals. Besides Bragg resonances (Ams et al., 2017), these features enable sampling optical signals in optical circuits by fabricating nanoscale scattering centers along the circuit lines (typically waveguides and optical routers) but also extracting the embedded information, acting as antennas (Callejo et al., 2022). Guiding, interference, and scattering, i.e., spectroscopy and imaging, can be combined (le Coarer et al., 2007). Periodic patterns triggered by coherent self-organisation under field can spontaneously build highly packed planar arrays. Anisotropies in shape and orientation can further determine local birefringence and dichroism, with the ability to manipulate light wavefronts (Poumellec et al., 2016). These combined characteristics led to one prominent example of engineered materials for high-density data storage using voxels produced by sub-wavelength periodic patterns (Zhang et al., 2014). This field of optical storage takes thus full advantage from combining nanophotonics to nanostructuring (Gu et al., 2014). A substantial prospect for developing photonic elements that can sense, store, transport, and manipulate information on the nanoscale is then to be noted. As well, the potential for developing capabilities for wavefront shaping, sensing, or extreme imaging and microscopy can be of significant importance. The scale significantly smaller than the wavelength, the potential for high density packaging, and the 3D capability are key conditions for the design of innovative metamaterials. The challenge remains now to predict and design the engineered material, capable to code, decode, and record information, suited at the same time for super-resolved ultrafast laser processing. The task becomes highly multidisciplinary, combining inverse design, metrology, and computational methods that will define how light will shape matter and how matter will reshape light. Multiscale approaches will equally determine how engineered materials can be integrated in more complex optical systems, leading to compactness and enhanced performances. Tackling the design and fabrication complexity of volume nanoscale systems with periodic and aperiodic forms, the combination of fabrication and computational power can generate new degrees of freedom (Gerke and Piestun, 2010). The design process started to benefit from the latest developments in prediction and optimization algorithms. Similar predictive techniques can be used to assist laser processing towards the highest resolution, with recent developments guided by artificial intelligence in developing accurate self-organization models (Brandao et al., 2022) but also in the in situ visualisation of nanoscale structures (Maire et al., 2009; Ishikawa and Hayasaki, 2018).
Switching from the optical perspective, I note that extreme volume nanostructuring is equally associated with changes in specific volumes and thus in local thermal and mechanical properties. A straightforward development leads to mechanical systems for actuating nanoscale movement (Vlugter et al., 2019). Opto-mechanical systems can be directly carved using techniques of stealth dicing (Meyer et al., 2019) or selective laser etching (Marcinkevičius et al., 2001), relevant for 3D digital processing, where local properties can be engineered via nanoscale self-organized domains. Extrapolating to the thermal and electrical domains, bulk-engineered materials on the nanoscale can be designed for controlling thermal transport as the structuring scale becomes similar to the phonon or electron mean free path (Fei and Yang, 2014). Engineered thermal and electrical barriers may be created as well energy convertors. Contact functions depend on nanoscale porosity, and nanoscale contact functions can be engineered for fluidics. All these briefly recalled opportunities can be combined, opening up the perspective of integrating a multitude of functions on the same substrate, enabled by the nanoscale.
To conclude, one can anticipate strong developments of laser and material engineering approaches towards the achievement of extreme resolutions using remote optical tools. The 10 nm scale (Gan et al., 2013; Yan et al., 2021) within monolithic materials is in sight and the processing limits are continuously being pushed back. Investigating ultrafast and extreme spatial scales enabled by light will generate a solid body of knowledge concerning mesoscopic behavior of matter in non-equilibrium conditions. As such, the present achievements lead to the believe that precise and robust nanostructuring techniques converging towards the 10 nm scale can be conceived, combining precise field and material effects. The vectorial character as well as the phase information carried by the beam will play an increasing role. Complex fields coherently combining multiple frequencies can nowadays be designed in a programmable manner, which, alongside with rapid in situ observation means, will generate advanced control loops for volume nanostructuring. All light characteristics, beyond the most obvious such as wavelength, energy and pulse duration, can be harnessed to define an extremely versatile process; spatial and spectral phase, field orientation, wavevector, Poynting vector, etc. The application range follows up swiftly and it concerns both the development of ultrafast reconfigurable sources and 3D engineered materials (Flamm et al., 2021). A roadmap starts to emerge concerning applications empowered by fast nanoscale prototyping of volumes beyond the optical limits and lasre fabrication of metamaterials. These developments will increasingly exploit synergies between light and matter at mesoscopic scales with light that adapts to structure and to material. They represent a far reaching effort to develop smart and reconfigurable processing tools, capable of addressing the nanoscale challenges in prediction, fabrication, visualisation and exploitation. Harnessing the behavior of light at the nanoscale and, at the same time, controlling the material mesoscopic behavior in degrees of freedom and dimensions is then key in pushing the limits of the process towards unprecedented laser structuring resolutions.
The author confirms being the sole contributor of this work and has approved it for publication.
The author declares that the research was conducted in the absence of any commercial or financial relationships that could be construed as a potential conflict of interest.
All claims expressed in this article are solely those of the authors and do not necessarily represent those of their affiliated organizations, or those of the publisher, the editors and the reviewers. Any product that may be evaluated in this article, or claim that may be made by its manufacturer, is not guaranteed or endorsed by the publisher.
This manuscript was transferred from Walter De Gruyter GmbH to Frontiers Media SA. The peer review for this paper was conducted in full by Walter De Gruyter GmbH. In accordance with their peer review, editor names and reviewer names have not been published. For any queries regarding the peer review of this manuscript, please contact YWR2YW5jZWRvcHRpY3MuZWRpdG9yaWFsLm9mZmljZUBmcm9udGllcnNpbi5vcmc=.
Ams, M., Dekker, P., Gross, S., and Withford, M. J. (2017). Fabricating waveguide Bragg gratings (WBGs) in bulk materials using ultrashort laser pulses. Nanophotonics 6, 743. doi:10.1515/nanoph-2016-0119
Bhuyan, M. K., Somayaji, M., Mermillod-Blondin, A., Bourquard, F., Colombier, J. P., and Stoian, R. (2017). Ultrafast laser nanostructuring in bulk silica, a “slow” microexplosion. Optica 4, 951. doi:10.1364/optica.4.000951
Brandao, E., Colombier, J.-P., Duffner, S., Emonet, R., Garrelie, F., Habrard, A., et al. (2022). Learning PDE to model self-organization of matter. Entropy 24, 1096. doi:10.3390/e24081096
Callejo, M., Bonduelle, M., Morand, A., Zhang, G., Lv, J., Cheng, G., et al. (2022). Waveguide scattering antennas made by direct laser writing in bulk glass for spectrometry applications in the short-wave IR. Appl. Opt. 61, 7173. doi:10.1364/ao.464017
Cheng, Y., and Sugioka, K. (Editors) (2013). Ultrafast laser processing; from micro-to nanoscale (United States: Jenny Stanford Publishing).
Courvoisier, F., Lacourt, P.-A., Jacquot, M., Bhuyan, M. K., Furfaro, L., and Dudley, J. M. (2009). Surface nanoprocessing with nondiffracting femtosecond Bessel beams. Opt. Lett. 34, 3163. doi:10.1364/ol.34.003163
Fan, C., Poumellec, B., Lancry, M., He, X., Zeng, H., Erraji-Chahid, A., et al. (2012). Three-dimensional photoprecipitation of oriented LiNbO_3-like crystals in silica-based glass with femtosecond laser irradiation. Opt. Lett. 37 (14), 2955. doi:10.1364/ol.37.002955
Fei, R., and Yang, L. (2014). Strain-engineering the anisotropic electrical conductance of few-layer black phosphorus. Nanolett 14, 2884–2889. doi:10.1021/nl500935z
Flamm, D., Grossmann, D. G., Sailer, M., Kaiser, M., Zimmermann, F., Chen, K., et al. (2021). Structured light for ultrafast laser micro- and nanoprocessing. Opt. Eng. 60, 025105. doi:10.1117/1.OE.60.2.025105
Gamaly, E. G., and Rode, A. V. (2016). Coupling of polarisation of high frequency electric field and electronic heat conduction in laser created plasma. Opt. Laser Technol. 82, 69–71. doi:10.1016/j.optlastec.2016.02.011
Gan, Z., Cao, Y., Evans, R. A., and Gu, M. (2013). Three-dimensional deep sub-diffraction optical beam lithography with 9 nm feature size. Nat. Commun. 4, 2061. doi:10.1038/ncomms3061
Gerke, T. D., and Piestun, R. (2010). Aperiodic volume optics. Nat. Photonics 4, 188–193. doi:10.1038/nphoton.2009.290
Glezer, E. N., and Mazur, E. (1997). Ultrafast-laser driven micro-explosions in transparent materials. Appl. Phys. Lett. 71, 882–884. doi:10.1063/1.119677
Grady, D. (1988). The spall strength of condensed matter. J. Mech. Phys. Solids 36, 353–384. doi:10.1016/0022-5096(88)90015-4
Gu, M., Li, X., and Cao, Y. (2014). Optical storage arrays: A perspective for future big data storage. Light Sci. Appl. 3, e177. doi:10.1038/lsa.2014.58
He, F., Zeng, B., Chu, W., Ni, J., Sugioka, K., Cheng, Y., et al. (2014). Characterization and control of peak intensity distribution at the focus of a spatiotemporally focused femtosecond laser beam. Opt. Express 22, 9734. doi:10.1364/oe.22.009734
Ishikawa, S., and Hayasaki, Y. (2018). Nanostructure identification using interference microscope with database. Opt. Rev. 25, 571–577. doi:10.1007/s10043-018-0449-9
Jeschke, H. O., Garcia, M. E., and Bennemann, K. H. (2001). Theory for the ultrafast ablation of graphite films. Phys. Rev. Lett. 87, 015003. doi:10.1103/physrevlett.87.015003
Joglekar, A. P., Liu, H.-h., Meyhöfer, E., Mourou, G., and Hunt, A. J. (2004). Optics at critical intensity: Applications to nanomorphing. Proc. Natl. Acad. Sci. U.S.A. 101, 5856–5861. doi:10.1073/pnas.0307470101
Juodkazis, S., Nishimura, K., Tanaka, S., Misawa, H., Gamaly, E. G., Luther-Davies, B., et al. (2006). Laser-induced microexplosion confined in the bulk of a sapphire crystal: Evidence of multimegabar pressures. Phys. Rev. Lett. 96, 166101. doi:10.1103/physrevlett.96.166101
Korte, F., Nolte, S., Chichkov, B. N., Bauer, T., Kamlage, G., Wagner, T., et al. (1999). Far-field and near-field material processing with. femtosecond laser pulses. Appl. Phys. A 69, S7–S11. doi:10.1007/s003399900391
le Coarer, E., Blaize, S., Benech, P., Stefanon, I., Morand, A., Lérondel, G., et al. (2007). Wavelength-scale stationary-wave integrated Fourier-transform spectrometry. Nat. Phot. 1, 473–478. doi:10.1038/nphoton.2007.138
Lei, Y., Sakakura, M., Wang, L., Yu, Y., Wang, H., Shayeganrad, G., et al. (2021). High speed ultrafast laser anisotropic nanostructuring by energy deposition control via near-field enhancement. Optica 8, 1365. doi:10.1364/optica.433765
Li, Z.-Z., Wang, L., Fan, H., Yu, Y.-H., Chen, Q.-D., Juodkazis, S., et al. (2020). O-FIB: Far-field-induced near-field breakdown for direct nanowriting in an atmospheric environment. Light Sci. Appl. 9, 41. doi:10.1038/s41377-020-0275-2
Maire, G., Drsek, F., Girard, J., Giovannini, H., Talneau, A., Konan, D., et al. (2009). Experimental demonstration of quantitative imaging beyond abbe’s limit with optical diffraction tomography. Phys. Rev. Lett. 102, 213905–213914. doi:10.1103/physrevlett.102.213905
Marcinkevičius, A., Juodkazis, S., Watanabe, M., Miwa, M., Matsuo, S., Misawa, H., et al. (2001). Femtosecond laser-assisted three-dimensional microfabrication in silica. Opt. Lett. 26, 277. doi:10.1364/ol.26.000277
Meyer, R., Froehly, L., Giust, R., Del Hoyo, J., Furfaro, L., Billet, C., et al. (2019). Extremely high-aspect-ratio ultrafast Bessel beam generation and stealth dicing of multi-millimeter thick glass. Appl. Phys. Lett. 114, 201105. doi:10.1063/1.5096868
Poumellec, B., Lancry, M., Desmarchelier, R., Hervé, E., and Bourguignon, B. (2016). Light Sci. Appl. 5, e16178. doi:10.1038/lsa.2016.178
Prigogine, I. (1978). Time, structure, and fluctuations. Science 201, 777–785. doi:10.1126/science.201.4358.777
Ròdenas, A., Gu, M., Corrielli, G., Paiè, P., John, S., Kar, A. K., et al. (2019). Three-dimensional femtosecond laser nanolithography of crystals. Nat. Photonics 13, 105–109. doi:10.1038/s41566-018-0327-9
Rudenko, A., Colombier, J. P., Itina, T. E., and Stoian, R. (2021). Genesis of nanogratings in silica bulk via multipulse interplay of ultrafast photo-excitation and hydrodynamics. Adv. Opt. Mater. 9, 2100973. doi:10.1002/adom.202100973
Salter, P. S., and Booth, M. J. (2019). Adaptive optics in laser processing. Light Sci. Appl. 8, 110. doi:10.1038/s41377-019-0215-1
Shimotsuma, Y., Kazansky, P. G., Qiu, J., and Hirao, K. (2003). Self-organized nanogratings in glass irradiated by ultrashort light pulses. Phys. Rev. Lett. 91, 247405. doi:10.1103/physrevlett.91.247405
Stoian, R., and Bonse, J. (Editors) (2023). Ultrafast laser nanostructuring: The pursuit of extreme scales (Berlin, Germany: Springer Series in Optical Sciences Series), 239.
Stoian, R., Wollenhaupt, M., Baumert, T., and Hertel, I. V. (2010). “Temporal pulse tailoring in ultrafast laser manufacturing technologies,” in Laser precision microfabrication, ed. K. Sugioka, M. Meunier, and A. Piqué (Berlin, Heidelberg: Springer), 121.
Sun, K., Tan, D., Fang, X., Xia, X., Lin, D., Song, J., et al. (2022). Three-dimensional direct lithography of stable perovskite nanocrystals in glass. Science 375, 307–310. doi:10.1126/science.abj2691
Vailionis, A., Gamaly, E. G., Mizeikis, V., Yang, W., Rode, A. V., and Juodkazis, S. (2011). Evidence of superdense aluminium synthesized by ultrafast microexplosion. Nat. Commun. 2, 445. doi:10.1038/ncomms1449
Vlugter, P., Block, E., and Bellouard, Y. (2019). Local tuning of fused silica thermal expansion coefficient using femtosecond laser. Phys. Rev. Mater. 3, 053802. doi:10.1103/physrevmaterials.3.053802
Xu, J., Cheng, Y., and Sugioka, K. (2021). Handbook of laser micro- and nano-engineering. Editor K. Sugioka (Berlin, Germany: Springer), 527.
Yan, Z., Gao, J., Beresna, M., and Zhang, J. (2021). Near-field mediated 40 nm in-volume glass fabrication by femtosecond laser. Adv. Opt. Mater. 10, 2101676. doi:10.1002/adom.202101676
Yang, W., Kazansky, P., and Svirko, Y. (2008). Non-reciprocal ultrafast laser writing. Nat. Photon. 2, 99–104. doi:10.1038/nphoton.2007.276
Zhang, G., Stoian, R., Lou, R., Chen, T., Li, G., Wang, X., et al. (2021). Thermal and mechanical limitations to processing resolution in volume non-diffractive ultrafast laser structuring. Appl. Surf. Sci. 570, 151170. doi:10.1016/j.apsusc.2021.151170
Keywords: ultrafast laser processing, nanofabrication, super-resolution, 3D optical manufacturing, sub-wavelength optical design
Citation: Stoian R (2023) Ultrafast laser volume nanostructuring; a limitless perspective. Adv. Opt. Technol. 12:1237524. doi: 10.3389/aot.2023.1237524
Received: 09 June 2023; Accepted: 13 June 2023;
Published: 11 July 2023.
Copyright © 2023 Stoian. This is an open-access article distributed under the terms of the Creative Commons Attribution License (CC BY). The use, distribution or reproduction in other forums is permitted, provided the original author(s) and the copyright owner(s) are credited and that the original publication in this journal is cited, in accordance with accepted academic practice. No use, distribution or reproduction is permitted which does not comply with these terms.
*Correspondence: Razvan Stoian, cmF6dmFuLnN0b2lhbkB1bml2LXN0LWV0aWVubmUuZnI=
Disclaimer: All claims expressed in this article are solely those of the authors and do not necessarily represent those of their affiliated organizations, or those of the publisher, the editors and the reviewers. Any product that may be evaluated in this article or claim that may be made by its manufacturer is not guaranteed or endorsed by the publisher.
Research integrity at Frontiers
Learn more about the work of our research integrity team to safeguard the quality of each article we publish.