- Graduate Program in Acoustics, The Pennsylvania State University, University Park, PA, United States
Sonogenetics has emerged as a tool of therapeutic ultrasound which is revolutionizing the ability to non-invasively modulate the activity of neurons and other excitatory cells. This technology utilizes bioengineering methods to confer or amplify ultrasound sensitivity in target cells using engineered or modified protein mediators. The neuromodulation community has shown a growing interest in sonogenetics due to ultrasound’s ability to penetrate the skull and reach deep brain tissue, enabling non-invasive modulation of neurons. Novel methods of sonogenetics aim to enhance cellular control in humans by leveraging mechanosensitive and thermosensitive cellular mechanisms activated by ultrasound to address cellular dysfunction and degeneration. This mini review summarizes the progress of sonogenetic mediators proposed for neuromodulation and looks at new therapeutic applications of sonogenetics for cancer treatment and vision restoration.
1 Introduction
Focused ultrasound (FUS) has emerged in the biomedical field as a highly effective and widely applicable, non-invasive therapeutic tool. As ultrasound waves pass through the body, energy delivered causes a wide range of bio-effects evoked by different low or high pressure, frequency, and duration parameters. With the advent of FUS guided by magnetic resonance imaging (MRI), it is possible to precisely direct an ultrasound’s beam to a desired treatment location with high spatiotemporal resolution (Hokland et al., 2006). This has offered precision targetability of small volumes of cells in deep tissue environments. Clinical applications of FUS have improved patient quality of care by providing non-invasive treatments, such as ablation of cancerous tumors, improved spatial control of drug delivery, and modulation of the activity of excitatory cells such as neurons.
The field of bioengineering has also made vast strides in improving the treatment of diseases and cancers (Tamura and Toda, 2020). Advancements in genomics and gene editing have enabled researchers to rapidly test and identify genes which may be associated with specific disease pathology or biomolecular function (Moraes and Góes, 2016). Genetic engineering and transfection techniques allow for the modification and cross-species transfer of specific genes to improve or alter genetic function. Bioengineering thus offers methods to finely hone cellular mechanisms sensitive to FUS in order to increase its efficacy, improve spatial resolution, or reduce unintended off-target effects (Ibsen et al., 2015). The pairing of these two technologies is known as sonogenetics, the non-invasive modulation of cellular function using bioengineering techniques to sensitize specific cells to ultrasound stimulation. Though only recently introduced, two mechanisms of sonogenetics have already yielded promising results: The insertion of a gene or protein mediator using genetic engineering to sensitize a cell to ultrasound (Ibsen et al., 2015), and the use of genetically encoded proteins or acoustic biomolecules to amplify the mechanical effects of ultrasound on targeted cells (Huang et al., 2019; Hou et al., 2021).
The inception and development of sonogenetics have been primarily influenced by the neuromodulation community. FUS has opened the doorway to access and treat the most complicated organ in the human body; the brain. Neurodegenerative diseases are becoming more prevalent around the world with expanding population and life expectancy (Van Schependom and D’haeseleer, 2023). However, there is currently no effective way to address the underlying pathology causing neurodegeneration (Wareham et al., 2022). Accessing the brain to image, diagnose, and treat neurodegeneration is naturally limited by the skull and blood brain barrier. Symptoms are generally managed through drug administration, but continual systemic delivery of such drugs can cause severe side effects to organs throughout the body (Achar et al., 2021). Techniques to modulate neural activity using light, electrical, or chemical stimulus have been effective in alleviating degeneration symptoms, but require invasive surgical procedures or implants, risking additional brain tissue damage and post surgical complications (Lewis et al., 2016). FUS has therefore offered an advantage over other neuromodulation techniques due to its non-invasive nature and has been approved by the FDA to treat Parkinson’s dyskinesia and tremor via thermal ablation. Sonogenetics, on the other hand, shows promising potential to improve ultrasound neuromodulation, incurring minimal cell destruction by specifying and amplifying neural response to FUS through bioengineering.
2 Cellular effects of ultrasound neuromodulation
FUS transducers deliver mechanical and thermal energy via mechanical waves which penetrate through bone and tissue non-invasively, with a scattering effect much less than that of light (Kim et al., 2021). As depicted in Figure 1A, it may also induce stable or inertial intramembrane cavitation of microbubbles if they are present within the lipid bilayer (Plaksin et al., 2014). Mechanisms of ultrasound have been investigated at the cellular level using a range of frequency, intensity, and pulse parameters. While high intensity focused ultrasound (HIFU) is commonly used to perform thermal ablation of tissue (Bachu et al., 2021), low intensity focused ultrasound (LIFU) can be applied thermally or non-thermally and can stimulate or suppress cellular activity (Tyler et al., 2008). This effect was observed in hippocampal neurons where low frequency LIFU was used to stimulate electrical activity in cells by activating membrane bound proteins, triggering increased synaptic transmission (Tyler et al., 2008).
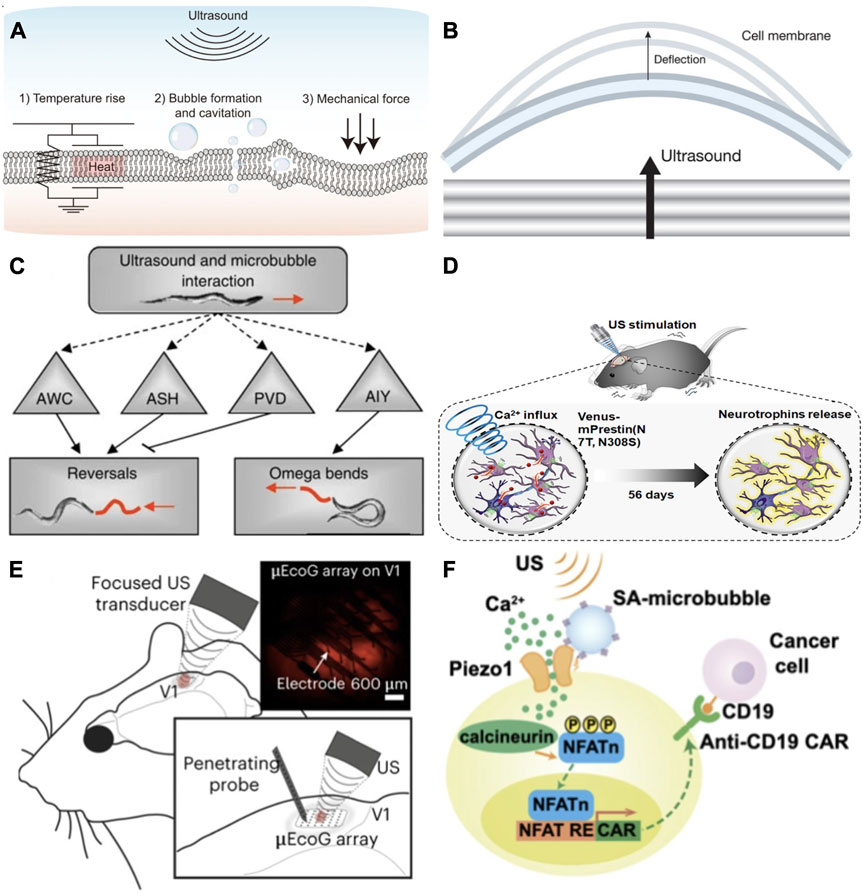
FIGURE 1. (A) Low intensity focused ultrasound mechanisms induce bioeffects within the cellular membrane including perturbation of the cellular membrane, local thermal rise and cavitation of microbubbles [reproduced from Yoo et al. (2022), licensed CC-BY-4.0]. (B) Mechanical waves perturb the cellular membrane, deflecting the lipid bilayer leaflets in differing amounts due to cytoskeletal components. The increase in membrane area due to stretching of the leaflets triggers transmembrane electrical response [reproduced from Vasan et al. (2022), licensed CC-BY-4.0]. (C) TRP-4 misexpressed in ASH and AWC neurons in the nematode C. elegans increased the animal’s large reversals due to ultrasound stimulation. In PWC neurons, misexpressed TRP-4 suppressed large reversals, and in AIY neurons stimulated omega bend behavior [reproduced from Ibsen et al. (2015), licensed CC-BY-4.0]. (D) Modified prestin protein inserted into mouse dopaminergic neurons increased calcium influx and output of neurotrophins due to ultrasound stimulus, ameliorating dopaminergic neurodegeneration 10-fold and mitigating PD symptoms by 4-fold. Reprinted (adapted) with permission from Fan et al. (2021). Copyright 2021, American Chemical Society. (E) Using a high frequency transducer targetting the primary visual cortex (V1) in vivo, retinal and cortical neurons transfected with red fluorescent MscL-G22s were activated with millisecond temporal precision to generate a visual cortex behavior associated with light perception. A micro-electrocorticography (μ EcoG) electrode array and penetrating probe were placed on V1 and used to measure US evoked responses [reproduced from Cadoni et al. (2023), licensed CC-BY-4.0]. (F) Depiction of a synthetic genetic circuit for sonogenetic transcription activation of chimeric antigen receptor t (CAR-t) cells. Microbubbles couple to the surface of the cell, where mechanosensitive Piezo 1 channels are expressed and activated by mechanical stimulation from ultrasound waves. Released calcium triggers downstream pathways activating calcineurin, NFAT dephosphorylation, and translocation to the nucleus. Translocated NFAT binds to upstream response elements to initiate gene expression through genetic transduction modules [reproduced from Pan et al. (2018), published under the PNAS license].
As ultrasound waves pass though tissue, cellular membranes deflect and oscillate (Figure 1B), which drives biophysical transduction mechanisms (Lee et al., 2020). Visualization of cellular membrane dynamics in neurons using high speed digital holographic microscopy shows membrane deflections occur up to 150 nm under 7 MHz ultrasound stimulation (Vasan et al., 2022). Prolonged oscillation of the membrane creates an accumulation of action potential, occurring in phase with the membrane’s deflection (Heimburg and Jackson, 2005). The change in capacitance due to the elastic change in membrane area, while maintaining constant volume, induces transmembrane voltage changes and subsequent depolarization (Vasan et al., 2022). Mechanical stimuli transmitted by ultrasound transducers are then directly translated into electrical and chemical signals by membrane bound mechanosensitive receptor proteins that form gated ion channels within the lipid bilayer (Chu et al., 2022). These mechanosensitive ion channels are found to function either by force from lipids which creates conformational membrane deformation, or deformation caused by forces applied by the cytoskeleton or extracellular matrix (Chu et al., 2022). Thermosensitive channels increase ion discharge frequency in response to a range of potentially noxious heating or cooling (Lamas et al., 2019). FUS, used at low-intensity pulsed parameters at a threshold of 42°C to limit cell death, delivers thermal energy with minimal mechanical deformation, thereby activating receptors sensitive to temperature increase.
Calcium signaling is shown to act as a primary initiator and indicator of response to FUS stimulation in cortical neurons (Yoo et al., 2022). As calcium ions accumulate, they trigger the opening of calcium-gated cation channels. This leads to depolarization of the cellular membrane and the further opening of voltage-gated sodium and potassium channels (Kubanek et al., 2016). Calcium imaging is simple yet robust, involving transfection of a fluorescence tracer to view and record intracellular calcium levels through time-lapse imaging (Chu et al., 2022). Through these mechanisms, ultrasound neuromodulation has shown its capability to non-invasively stimulate deep-brain regions affected by neurodegeneration. The limitation of ultrasound neuromodulation lies in its inability to target specific cell types within the focal region. The answer to this limitation has been found through sonogenetics.
3 Direct neuromodulatory sonogenetic mediators
Sonogenetics was initially proposed as a method to improve targeted neuromodulation based on the idea of optogenetics, a technique in which neurons are sensitized to light stimulus by artificially overexpressing photosensitive ion channels (Duebel et al., 2015). The term sonogenetics was first dubbed by Chalasani’s team at the Salk Institute for Biological Studies for the genetic sensitization of neurons to low pressure ultrasound in the nematode C. elegans (Ibsen et al., 2015). This species, having a compact and fully characterized nervous system with highly sensitive thermosensory and mechanosensory neurons and a short life cycle, has proven useful in quickly testing individual genes and proteins for thermal or mechanical sensitivity through gene editing knockdowns. Firstly, Ibsen et al. (Ibsen et al., 2015) demonstrated that the animal was unresponsive to low pressure ultrasound stimulation on its own, requiring microbubble mediation to enhance ultrasound wave transduction and elicit any response. Secondly, they identified TRP-4, a stretch-sensitive mechanotransduction cation channel naturally found in four C. elegans neurons, as being sensitive to low pressure ultrasound stimulation. After genetically induced misexpression of the TRP-4 protein in other neuron types, C. elegans showed behavioral locomotion differences under stimulation, indicating that the expression of TRP-4 sensitized these neurons to ultrasound (Figure 1C). Further study of the biophysical mechanisms behind TRP-4’s ultrasound sensitivity show that it functions along with MEC-4, a DEG/ENaC/ASIC ion channel required in sensing touch which is insensitive to changes in membrane voltage or capacitance (Kubanek et al., 2018). In C. Elegans, co-expression of these two channels is required in other neurons as they act together to affect the reversal behavior exhibited by C elegans and may point to other downstream mechanisms involved in ultrasound sensitivity (Magaram et al., 2022). Although this first example of sonogenetics showed that such genetically targeted neuronal response is possible with ultrasound, these specific channels are not genetic homologs which directly translate to mammals.
Neuromodulatory sonogenetic mediators are genes which can be modified and inserted into cells to express mechanosensitive or thermosensitive protein, affect membrane elasticity, or confer sensitivity to ultrasound by some other mechanisms. Identifying sonogenetic mediators which are compatible with the human genome is the most essential element for the success of sonogenetics and its advancement to human clinical trials. To be usable in humans, genes identified as potential sonogenetic mediators must be naturally occurring in humans or have a homolog capable of being modified through genetic engineering and inserted into human DNA. While many transmembrane protein ion channel families have now been identified as being mechano- or thermosensitive, only a few have been validated as potential sonogenetic mediators. Table 1 provides a list of the ion channels and other protein mediators highlighted in this review.
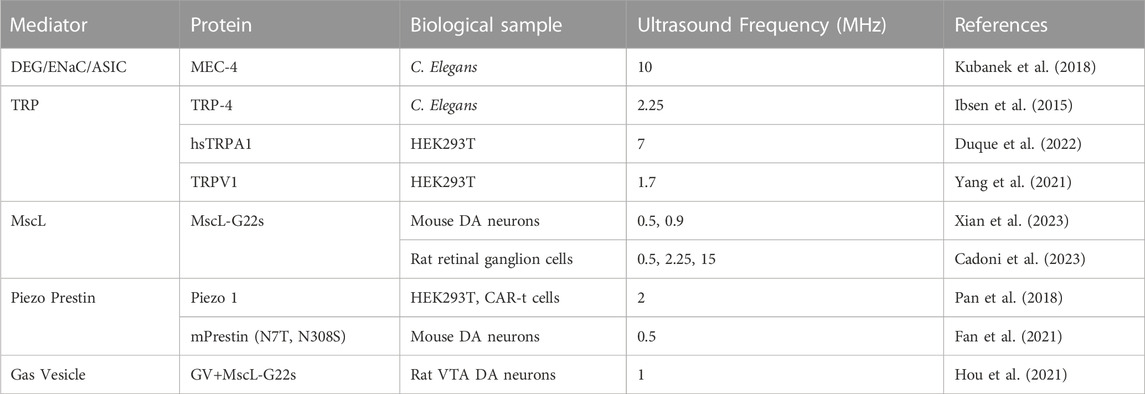
TABLE 1. Sonogenetic mediators used to confer ultrasound sensitivity to neurons and other cells in order to activate specific cellular responses.
The initial success by Ibsen et al. has led to extensive investigation of the transient receptor potential (TRP) family of cationic channels for potential sonogenetic mediators (Duque et al., 2022; Yoo et al., 2022). These integral membrane proteins show mostly conserved sequence homology from nematodes to humans and are involved in sensory physiological processes (Samanta et al., 2018). TRP channels act as signal transducers by altering membrane potential via intercellular calcium (Ca2+)concentration (Samanta et al., 2018) and activate at very low stress levels for ultrasound frequencies below 3 MHz (Chu et al., 2022). Various TRP channels have been tested for ultrasound sensitivity using chemical and CRISPR knockdown methods (Yoo et al., 2022). Results suggested that the TRPP1/2 complex and TRPC1 mechanoreceptors are responsive to ultrasound. This was promising as TRPC subfamily are store operated calcium channels broadly expressed in mammalian tissues (Samanta et al., 2018) and decreased expression of TRPC1 has been found in Parkinson’s disease patients (Dietrich et al., 2014). TRPM4, a non-selective cation channel highly expressed in neurons and cells in the prostate and intestine, is activated by intracellular Ca2+ and was strongly implicated as a downstream amplifier in the ultrasound response pathway (Yoo et al., 2022). A screening performed of 191 candidate protein channels thought to have mechanosensitive properties and their homologs identified TRPA1, a unique non-selective cation channel expressed in primary neurons and other cells such as epithelial cells, as robustly responsive to a wide range of ultrasound frequency in vitro (Duque et al., 2022). In vivo expression of the human homolog hsTRPA1 via adeno-associated viral vector transmission in mouse motor cortical neurons successfully elicited limb responses to 7 MHz ultrasound stimulation (Duebel et al., 2015). This cross-species success highlights hsTRPA1 as a likely candidate for sonogenetic mediation of neuron activity in humans to treat motor dysfunction and tremor dominant neurodegeneration.
The TRPV subfamily contains thermosensitive channels expressed in neurons that react to temperature increase inducible by FUS. TRPV1 is a ligand-gated nonselective cation channel expressed in sensory neurons and skin. In tissues exposed to dynamic temperature change, it activates by noxious heat above 42°C as an ambient temperature sensor. In sensory neurons it is an initiator of pain response, modulates neurotransmitter release, synaptic efficiency and affects plasticity (Meza et al., 2022). It was identified as ultrasound sensitive when response was evoked due to FUS heating of TRPV1 virally expressed in mouse neurons (Yang et al., 2021), opening the door for another class of potential sonogenetic mediators controllable through bioengineering.
The mechanosensitive (MS) channel family consists of ion channels which translate physical forces applied to the membrane into electrophysical functions. Small conductance (MscS) and Large conductance mechanosensitive ion channels (MscL) are stretch-activated osmotic release valves which act as electromechanical switches, sensing the state of lipid bilayer membranes and activating independent of other proteins or ligands (Teng et al., 2015). When opened, these channels are able to pass ions, water and small proteins. The MscL gene was originally extracted from Escherichia Coli and has been thoroughly analyzed. Its small genetic structure makes it a versatile model for genetic engineering (Ye et al., 2018). MscL channels expressed in vitro in rat hippocampal neurons have shown inherent response to ultrasound stimulation (Ye et al., 2018). Gain-of-function mutants such as the sonic response mutation MscL-192L show increased sensitivity to ultrasound without the need for addition of microbubbles to amplify stimulation (Ye et al., 2018). A notable mutant MscL-G22s has been much more effective than wild-type MscL in conferring ultrasound sensitivity as it has a lower threshold for gating without showing spontaneous activity (Qiu et al., 2020). Further work by the same group expressed MscL-G22s in vivo in the dorsal striatum, subthalamic nucleus, and ventral tegmental area of mouse brains in order to alleviate Parkinsonian motor disfunction and stimulate dopamine release (Xian et al., 2023).
Piezo 1 is a mechanosensitive ion channel involved in touch, support of the auto-immune system and skeletal structure. These channels naturally occur in many human tissues including sensory neurons, blood vessels, lung, heart, bladder, cartilage, and bone cells. Low intensity pulsed ultrasound was shown to activate Piezo 1 in dental stem cells (Gao et al., 2017), indicating potential as a sonogenetic mediator. Ultrasound activation of Piezo 1 in mouse primary cortical neurons-initiated calcium influx and nuclear c-Fos expression dependant on acoustic pressure (Qiu et al., 2019).
4 Secondary sonogenetic actuators
It must be noted that some of the proposed ion channels which show sensitivity to ultrasound must still be considered during sonogenetic stimulation. For example, TRP and ASIC channels are activated at very low stress levels, likely to spontaneously activate in response to environmental changes and may be unintentionally activated by ultrasound if expressed within the focal region (Chu et al., 2022). Sonogenetic methods to improve the control of ultrasound effects employ genes or biomolecules which control or amplify the sensitivity of ultrasound mechanisms, or allow lower intensity stimulation to reduce the possibility of off-target channel activation likely to trigger immune responses (Fan et al., 2021).
Prestin is a transmembrane electromotility protein found in ultrasound hearing mammals. This protein naturally occurs in hair follicle cells of ultrasound-hearing mammals and is involved in these animals’ high frequency auditory processing. Prestin responds to a limited bandwidth of ultrasound frequency near 0.5 MHz. Two mutations through amino acid substitution commonly found in echolocating species, mPrestin N7T, and N308S, have been shown to amplify sensitivity to ultrasound when transfected into mammalian cells (Huang et al., 2019). Prestin essentially acts as an electromechanical transducer activated by the movement of the membrane under ultrasound stimulation, and induces increased calcium release (Figure 1D), engaging calcium gated ion channels (Huang et al., 2019). These mutations expressed in mouse dopaminergic neurons and activated with 0.5 MHz ultrasound, promoted neurotrophic release in a Parkinson’s disease model, successfully ameliorating dopaminergic neuron degeneration after repeated ultrasound stimulation (Fan et al., 2021).
Gas-filled nanostructures have also been used as effective amplifiers of mechanical stimulation. Gas vesicles, genetically encodable hollow-shell protein structures extracted from cyanobacteria, function as force actuators similar to microbubbles (Hou et al., 2021). GVs amplify mechanical perturbation of the cellular membrane as they oscillate and buckle, allowing lower ultrasound intensity to avoid off-target channel activations. When engineered to bind with cell surface receptors, GVs enable targeted disruption of specific cells or inertial cavitation for release of molecular payloads (JO et al., 2019). While GVs have not been used to specifically target neurons, surface receptor-targeting peptides such as Arginyl-glycyl-aspartic acid (RGD) show promise in endowing GVs with a mechanism to target specific molecular markers in vivo (Lakshmanan et al., 2016). Hou et al. (Hou et al., 2021) showed that GVs injected in vitro into neurons over expressing MscL-G22s ion channel pathways, increased the calcium response. While GVs do not naturally occur in mammals, they merit further investigation as genetic engineering methods can be used to tune various characteristics affecting their oscillation and collapse in response to ultrasound as well as the way they scatter the ultrasound beam (Wu et al., 2023).
5 Discussion
While the early stages of sonogenetic research were primarily centered around mechanosensitive channel mediators naturally occurring in neurons, there is a growing interest in discovering mechanosensitive channels that can be applied to other excitable cells found throughout the central and peripheral nervous system, as well as in the heart and other organs within the body. Many TRP channels are naturally expressed in the intestines, which may have implications for sonogenetic control of gut biome proliferation (Maresca et al., 2018). Ultrasound stimulation has also been shown to increase currents carried by two-pore domain (K2P) potassium channels and voltage-gated sodium channels (VGCs) expressed in Xenopus oocytes (Kubanek et al., 2018). K2P mechanosensitive ion channels are naturally expressed throughout the mammalian nervous system and are involved in neuroprotection, pain and depression (Wang et al., 2020). Activation of the TREK-1, TREK-2 and TRAAK K2P ion channels with ultrasound was shown in retinal cells as well as neurons (Kubanek et al., 2016). Interest in these channels has sparked investigation of sonogenetic methods designed to restore retinal function. In the same study, sodium channel Nav1.5, a voltage activated ion channel naturally found in cardiomyocytes, was also activated by ultrasound, sparking speculation of using sonogenetic methods to perform pace-maker activity in cardiac cells.
Sonogenetic activation of overexpressed mechanosensitive ion channels in retinal cells may help in developing new brain-machine interfaces for visual restoration (Cadoni et al., 2023). Retinal ganglion cells transfected with MscL-G22s in the rat visual cortex (Figure 1E) showed increased mechanosensitivity in response to ultrasound stimulation. By delivering millisecond patten presentations via ultrasound, cells transfected with MscL were induced to respond similarly to auditive cells, generating a motor behavior associated with light perception. Although still requiring a craniotomy, the incorporation of MscL greatly decreased the necessary ultrasound pressure applied, increasing safety and offering a less invasive method of high-resolution visual restoration at the cortical level. This study also postulated that similar sonogenetic methods may be useful in hearing restoration.
Sonogenetically mediated CAR-t immunotherapy has been explored (Wang et al., 2020; Wu et al., 2023) with exciting implications for targeted non-invasive cancer treatment in complex tissue environments. Piezo 1 shows promise in controlling CAR-t cells in cancer immunotherapy. Piezo 1 engineered into a genetic circuit (Figure 1F) was inserted into HEK293T cells as an actuator for CAR-t cell immunotherapy cancer treatment activated by focused ultrasound (Pan et al., 2018). The MscL mechanosensitive ion channel has also been employed for cancer treatment, producing targeted apoptosis effects in melanoma model mice (Sun and Wu, 2021). While many methods of sonogenetic directed systems for cancer immunotherapy are being proposed, further investigation is needed for validation (He et al., 2021).
Mammalian heat shock promoters and bacterial thermal bioswitches have been used in the bioengineering community to transcribe control of biochemical responses in cells. This entails the production of slight heating by pulsed low intensity ultrasound to trigger the activation of therapeutic protein production in implanted cells (Maresca et al., 2018). Heat shock promoter HSP70 inserted into cells has been used to isolate specific gene expression, with rapid gene activation by holding the cells at 43°C for short amounts of time with tight repression in the off-state (Deckers et al., 2009). Control of gene expression non-invasively using thermal effects of ultrasound has broad implications for the use of sonogenetics outside the central nervous system. Challenges this method faces include cumulative expression due to leakage, thermal induction variation of cell types, unintended response induced by other stress stimuli (Deckers et al., 2009).
In conclusion, sonogenetics is still in an infant stage of research and much remains uncharted of its full effects or potential. Neuromodulatory sonogenetics, though promising, requires more in vivo verification of potential mediators and investigation into unintentional effects. Methods to compile how different ultrasound pulsing schemes influence ion channel dynamics and neural firing, such as the computational sonogenetics model proposed by Liu et al. (Liu et al., 2022) may be essential in finding new sonogenetic mediators. Computational prediction of the behavior of sonogenetic mediators and their influence on a wide range of ion channels, cell types, and tissues is also necessary. The emerging role of sonogenetics in medicine as a versatile therapeutic tool will transform the treatment of diseases and degeneration throughout the body.
Author contributions
KB: Writing–original draft. HH: Writing–review and editing. YJ: Supervision, Writing–review and editing.
Funding
The author(s) declare financial support was received for the research, authorship, and/or publication of this article. This work is supported by Dr. Jing’s Penn State startup funding.
Conflict of interest
The authors declare that the research was conducted in the absence of any commercial or financial relationships that could be construed as a potential conflict of interest.
The author(s) declared that they were an editorial board member of Frontiers, at the time of submission. This had no impact on the peer review process and the final decision.
Publisher’s note
All claims expressed in this article are solely those of the authors and do not necessarily represent those of their affiliated organizations, or those of the publisher, the editors and the reviewers. Any product that may be evaluated in this article, or claim that may be made by its manufacturer, is not guaranteed or endorsed by the publisher.
References
Achar, A., Myers, R., and Ghosh, C. (2021). Drug delivery challenges in brain disorders across the blood–brain barrier: Novel methods and future considerations for improved therapy. Biomedicines 9, 1834. doi:10.3390/biomedicines9121834
Bachu, V. S., Kedda, J., Suk, I., Green, J. J., and Tyler, B. (2021). High-intensity focused ultrasound: A review of mechanisms and clinical applications. Ann. Biomed. Eng. 49, 1975–1991. doi:10.1007/s10439-021-02833-9
Cadoni, S., Demené, C., Alcala, I., Provansal, M., Nguyen, D., Nelidova, D., et al. (2023). Ectopic expression of a mechanosensitive channel confers spatiotemporal resolution to ultrasound stimulations of neurons for visual restoration. Nat. Nanotechnol. 18, 667–676. doi:10.1038/s41565-023-01359-6
Chu, Y. C., Lim, J., Chien, A., Chen, C. C., and Wang, J. L. (2022). Activation of mechanosensitive ion channels by ultrasound, Ultrasound in medicine & biology.
Deckers, R., Quesson, B., Arsaut, J., Eimer, S., Couillaud, F., and Moonen, C. T. (2009). Image-guided, noninvasive, spatiotemporal control of gene expression. Proc. Natl. Acad. Sci. 106, 1175–1180. doi:10.1073/pnas.0806936106
Dietrich, A., Fahlbusch, M., and Gudermann, T. (2014). Classical transient receptor potential 1 (trpc1): Channel or channel regulator? Cells 3, 939–962. doi:10.3390/cells3040939
Duebel, J., Marazova, K., and Sahel, J. A. (2015). Optogenetics. Curr. Opin. Ophthalmol. 26, 226–232. doi:10.1097/icu.0000000000000140
Duque, M., Lee-Kubli, C. A., Tufail, Y., Magaram, U., Patel, J., Chakraborty, A., et al. (2022). Sonogenetic control of mammalian cells using exogenous transient receptor potential a1 channels. Nat. Commun. 13, 600. doi:10.1038/s41467-022-28205-y
Fan, C. H., Wei, K. C., Chiu, N. H., Liao, E. C., Wang, H. C., Wu, R. Y., et al. (2021). Sonogenetic-based neuromodulation for the amelioration of Parkinson’s disease. Nano Lett. 21, 5967–5976. doi:10.1021/acs.nanolett.1c00886
Gao, Q., Cooper, P. R., Walmsley, A. D., and Scheven, B. A. (2017). Role of piezo channels in ultrasound-stimulated dental stem cells. J. Endod. 43, 1130–1136. doi:10.1016/j.joen.2017.02.022
He, T., Wang, H., Wang, T., Pang, G., Zhang, Y., Zhang, C., et al. (2021). Sonogenetic nanosystem activated mechanosensitive ion channel to induce cell apoptosis for cancer immunotherapy. Chem. Eng. J. 407, 127173. doi:10.1016/j.cej.2020.127173
Heimburg, T., and Jackson, A. D. (2005). On soliton propagation in biomembranes and nerves. Proc. Natl. Acad. Sci. 102, 9790–9795. doi:10.1073/pnas.0503823102
Hokland, S. L., Pedersen, M., Salomir, R., Quesson, B., Stodkilde-Jorgensen, H., and Moonen, C. T. (2006). Mri-guided focused ultrasound: Methodology and applications. IEEE Trans. Med. imaging 25, 723–731. doi:10.1109/tmi.2006.873296
Hou, X., Qiu, Z., Xian, Q., Kala, S., Jing, J., Wong, K. F., et al. (2021). Precise ultrasound neuromodulation in a deep brain region using nano gas vesicles as actuators. Adv. Sci. 8, 2101934. doi:10.1002/advs.202101934
Huang, Y. S., Fan, C. H., Hsu, N., Chiu, N. H., Wu, C. Y., Chang, C. Y., et al. (2019). Sonogenetic modulation of cellular activities using an engineered auditory-sensing protein. Nano Lett. 20, 1089–1100. doi:10.1021/acs.nanolett.9b04373
Ibsen, S., Tong, A., Schutt, C., Esener, S., and Chalasani, S. H. (2015). Sonogenetics is a non-invasive approach to activating neurons in caenorhabditis elegans. Nat. Commun. 6, 8264. doi:10.1038/ncomms9264
Jo, S., Bar-Zion, A., and Shapiro, M. G. (2019). Achieving spatial and molecular specificity with ultrasound-targeted biomolecular nanotherapeutics. Accounts Chem. Res. 52, 2427–2434. doi:10.1021/acs.accounts.9b00277
Kim, J., Shin, J., Kong, C., Lee, S. H., Chang, W. S., and Han, S. H. (2021). The synergistic effect of focused ultrasound and biophotonics to overcome the barrier of light transmittance in biological tissue. Photodiagnosis Photodyn. Ther. 33, 102173. doi:10.1016/j.pdpdt.2020.102173
Kubanek, J., Shi, J., Marsh, J., Chen, D., Deng, C., and Cui, J. (2016). Ultrasound modulates ion channel currents. Sci. Rep. 6, 24170. doi:10.1038/srep24170
Kubanek, J., Shukla, P., Das, A., Baccus, S. A., and Goodman, M. B. (2018). Ultrasound elicits behavioral responses through mechanical effects on neurons and ion channels in a simple nervous system. J. Neurosci. 38, 3081–3091. doi:10.1523/jneurosci.1458-17.2018
Lakshmanan, A., Farhadi, A., Nety, S. P., Lee-Gosselin, A., Bourdeau, R. W., Maresca, D., et al. (2016). Molecular engineering of acoustic protein nanostructures. ACS Nano 10, 7314–7322. doi:10.1021/acsnano.6b03364
Lamas, J. A., Rueda-Ruzafa, L., and Herrera-Pérez, S. (2019). Ion channels and thermosensitivity: Trp, trek, or both? Int. J. Mol. Sci. 20, 2371. doi:10.3390/ijms20102371
Lee, S. A., Kamimura, H. A., Burgess, M. T., and Konofagou, E. E. (2020). Displacement imaging for focused ultrasound peripheral nerve neuromodulation. IEEE Trans. Med. imaging 39, 3391–3402. doi:10.1109/tmi.2020.2992498
Lewis, P. M., Thomson, R. H., Rosenfeld, J. V., and Fitzgerald, P. B. (2016). Brain neuromodulation techniques: A review. Neurosci. 22, 406–421. doi:10.1177/1073858416646707
Liu, T., Choi, M. H., Zhu, J., Zhu, T., Yang, J., Li, N., et al. (2022). Sonogenetics: Recent advances and future directions. China: Brain Stimulation.
Magaram, U., Weiss, C., Vasan, A., Reddy, K. C., Friend, J., and Chalasani, S. H. (2022). Two pathways are required for ultrasound-evoked behavioral changes in caenorhabditis elegans. PloS one 17, e0267698. doi:10.1371/journal.pone.0267698
Maresca, D., Lakshmanan, A., Abedi, M., Bar-Zion, A., Farhadi, A., Lu, G. J., et al. (2018). Biomolecular ultrasound and sonogenetics. Annu. Rev. Chem. Biomol. Eng. 9, 229–252. doi:10.1146/annurev-chembioeng-060817-084034
Meza, R. C., Ancatén-González, C., Chiu, C. Q., and Chávez, A. E. (2022). Transient receptor potential vanilloid 1 function at central synapses in health and disease. Front. Cell. Neurosci. 16, 864828. doi:10.3389/fncel.2022.864828
Moraes, F., and Góes, A. (2016). A decade of human genome project conclusion: Scientific diffusion about our genome knowledge. Biochem. Mol. Biol. Educ. 44, 215–223. doi:10.1002/bmb.20952
Pan, Y., Yoon, S., Sun, J., Huang, Z., Lee, C., Allen, M., et al. (2018). Mechanogenetics for the remote and noninvasive control of cancer immunotherapy. Proc. Natl. Acad. Sci. 115, 992–997. doi:10.1073/pnas.1714900115
Plaksin, M., Shoham, S., and Kimmel, E. (2014). Intramembrane cavitation as a predictive bio-piezoelectric mechanism for ultrasonic brain stimulation. Phys. Rev. X 4, 011004. doi:10.1103/physrevx.4.011004
Qiu, Z., Guo, J., Kala, S., Zhu, J., Xian, Q., Qiu, W., et al. (2019). The mechanosensitive ion channel piezo1 significantly mediates in vitro ultrasonic stimulation of neurons. IScience 21, 448–457. doi:10.1016/j.isci.2019.10.037
Qiu, Z., Kala, S., Guo, J., Xian, Q., Zhu, J., Zhu, T., et al. (2020). Targeted neurostimulation in mouse brains with non-invasive ultrasound. Cell. Rep. 32, 108033. doi:10.1016/j.celrep.2020.108033
Samanta, A., Hughes, T. E., and Moiseenkova-Bell, V. Y. (2018). Transient receptor potential (trp) channels. Membr. protein complexes Struct. Funct., 141–165.
Sun, S., and Wu, M. (2021). Sonodynamic therapy: Another “light” in tumor treatment by exogenous stimulus. Smart Mater. Med. 2, 145–149. doi:10.1016/j.smaim.2021.05.001
Tamura, R., and Toda, M. (2020). Historic overview of genetic engineering technologies for human gene therapy. Neurol. medico-chirurgica 60, 483–491. doi:10.2176/nmc.ra.2020-0049
Teng, J., Loukin, S., Anishkin, A., and Kung, C. (2015). The force-from-lipid (ffl) principle of mechanosensitivity, at large and in elements. Pflügers Archiv-European J. Physiology 467, 27–37. doi:10.1007/s00424-014-1530-2
Tyler, W. J., Tufail, Y., Finsterwald, M., Tauchmann, M. L., Olson, E. J., and Majestic, C. (2008). Remote excitation of neuronal circuits using low-intensity, low-frequency ultrasound. PloS one 3, e3511. doi:10.1371/journal.pone.0003511
Vasan, A., Orosco, J., Magaram, U., Duque, M., Weiss, C., Tufail, Y., et al. (2022). Ultrasound mediated cellular deflection results in cellular depolarization. Adv. Sci. 9, 2101950. doi:10.1002/advs.202101950
Wang, S., Meng, W., Ren, Z., Li, B., Zhu, T., Chen, H., et al. (2020). Ultrasonic neuromodulation and sonogenetics: A new era for neural modulation. Front. physiology 11, 787. doi:10.3389/fphys.2020.00787
Wareham, L. K., Liddelow, S. A., Temple, S., Benowitz, L. I., Di Polo, A., Wellington, C., et al. (2022). Solving neurodegeneration: Common mechanisms and strategies for new treatments. Mol. Neurodegener. 17, 23. doi:10.1186/s13024-022-00524-0
Wu, D., Baresch, D., Cook, C., Ma, Z., Duan, M., Malounda, D., et al. (2023). Biomolecular actuators for genetically selective acoustic manipulation of cells. Sci. Adv. 9, eadd9186. doi:10.1126/sciadv.add9186
Xian, Q., Qiu, Z., Murugappan, S., Kala, S., Wong, K. F., Li, D., et al. (2023). Modulation of deep neural circuits with sonogenetics. Proc. Natl. Acad. Sci. 120, e2220575120. doi:10.1073/pnas.2220575120
Yang, Y., Pacia, C. P., Ye, D., Zhu, L., Baek, H., Yue, Y., et al. (2021). Sonothermogenetics for noninvasive and cell-type specific deep brain neuromodulation. Brain Stimul. 14, 790–800. doi:10.1016/j.brs.2021.04.021
Ye, J., Tang, S., Meng, L., Li, X., Wen, X., Chen, S., et al. (2018). Ultrasonic control of neural activity through activation of the mechanosensitive channel mscl. Nano Lett. 18, 4148–4155. doi:10.1021/acs.nanolett.8b00935
Keywords: sonogenetics, focused ultrasound, thermosensitive, mechanosensitive, neuromodulation
Citation: Bell K, Heo H and Jing Y (2023) Sonogenetics: a mini review. Front. Acoust. 1:1269867. doi: 10.3389/facou.2023.1269867
Received: 31 July 2023; Accepted: 06 September 2023;
Published: 21 September 2023.
Edited by:
Chengzhi Shi, Georgia Institute of Technology, United StatesReviewed by:
Dingjie Suo, Beijing Institute of Technology, ChinaCopyright © 2023 Bell, Heo and Jing. This is an open-access article distributed under the terms of the Creative Commons Attribution License (CC BY). The use, distribution or reproduction in other forums is permitted, provided the original author(s) and the copyright owner(s) are credited and that the original publication in this journal is cited, in accordance with accepted academic practice. No use, distribution or reproduction is permitted which does not comply with these terms.
*Correspondence: Yun Jing, eXFqNTIwMUBwc3UuZWR1