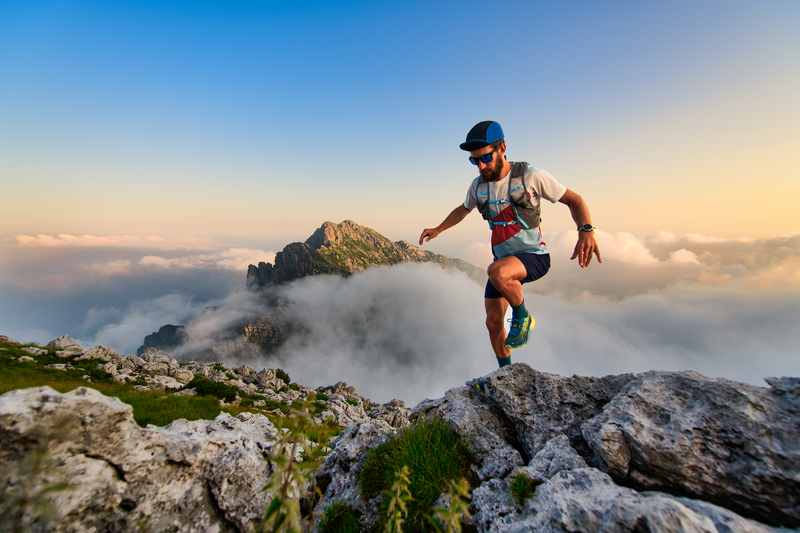
95% of researchers rate our articles as excellent or good
Learn more about the work of our research integrity team to safeguard the quality of each article we publish.
Find out more
MINI REVIEW article
Front. Acoust. , 02 October 2023
Sec. Acoustofluidics
Volume 1 - 2023 | https://doi.org/10.3389/facou.2023.1261027
The propagation of acoustic waves in fluids and solids produces fascinating phenomena that have been studied since the late 1700s and through to today, where it is finding broad application in manipulating fluids and particles at the micro to nano-scale. Due to the recent and rapid increase in application frequencies and reduction in the scale of devices to serve this new need, discrepancies between theory and reality have driven new discoveries in physics that are underpinning the burgeoning discipline. While many researchers are continuing to explore the use of acoustic waves in microfluidics, some are exploring vastly smaller scales, to nanofluidics and beyond. Because many of the applications incorporate biological material—organelles, cells, tissue, and organs—substantial effort is also being invested in understanding how ultrasound interacts with these materials. Surprisingly, there is ample evidence that ultrasound can be used to directly drive cellular responses, producing a new research direction beyond the established efforts in patterning and agglomerating cells to produce tissue. We consider all these aspects in this mini-review after a brief introduction to acoustofluidics as an emerging research discipline.
Acoustofluidics is the transport and manipulation of fluid and suspended solid objects through the attenuation, compression and non-linear interactions of a passing acoustic wave. The discipline has flourished in the past 15 years after it was found to solve several important problems at small scales commensurate with many current and anticipated applications, from medical diagnostics to spray generation, improving recharging rates in batteries, and the formation of tissues. Acoustofluidics phenomena first served to confound scientists since some of the earliest published works, from helping to drive a disagreement between E.F.F. Chladni (Chladni, 1787) and Félix Savart in the early 19th century over spurious particle patterns atop vibrating plates (Bell, 1991) to demonstrations by Michael Faraday 30 years later (Faraday, 1831), the pioneering analyses by John William Strutt (later Lord Rayleigh) 30 years after that (Strutt, 1883), and onwards to mysterious “quartz” wind blowing in the faces of materials scientists working with quartz oscillators (Eckart, 1948) and the unique presentations and demonstrations of fluid jetting from surface acoustic wave (SAW) devices by Showko Shiokawa in the 1990s (Shiokawa et al., 1989).
If not for an extraordinary need for new methods to manipulate fluids and suspended cells, particles, and molecules, acoustofluidics might have remained merely a curiosity just as it had for nearly two hundred years. Pressure, capillary, electromotive, magnetic, and acceleration-driven forces were too weak or too cumbersome to use at scales below a few millimeters in many potential applications in healthcare and chemistry (Laser and Santiago, 2004). The concept of microfluidics as a means to offer “lab-on-a-chip” became less a revolution and more an evolution with the tiny fluidic chips taking up residence in labs filled with large pieces of equipment capable of delivering the pressures and controlled flows necessary to operate them: “chips-in-a-lab.” It took the two previous decades to today for microfluidics researchers to begin to integrate acoustofluidics and produce solutions to the problems. Today, acoustofluidics is a flourishing discipline, with research being pursued from better understanding the fundamentals of how acoustic waves interact with materials to commercial and clinical application of the phenomena to address immediate unmet needs. We briefly review these topics, highlighting a few remarkable works among a growing body of quality work, hinting at the directions the discipline is progressing into the future.
As the application of acoustics at high power and small scales is pursued, the fundamental theories, models, and approaches to analysis in acoustofluidics needs revisiting and revision. Some of this work has been underway for a number of years (Friend and Yeo, 2011; Connacher et al., 2018), but many efforts are still underway today to explain phenomena both old and new.
Among the earliest evidence of a discrepancy in theory relevant to acoustofluidics was the careful exposition by Sir James Lighthill (Lighthill, 1978), pointing out that past theories devised to explain acoustic streaming in the boundary layer (Schlichting, 1932) and bulk (Strutt, 1883; Nyborg, 1965) had several key problems, most especially the choice to ignore inertial effects in bulk streaming. Even so, much of the acoustofluidics literature even to today—and even popular computational software like COMSOL—makes use of the classical approach espoused by Nyborg (1965), and the consequences have been to produce analytical results that only roughly approximate reality. Lighthill (1978) pointed out that the creep flow or slow streaming approximation inherent in these models was invalid for frequencies beyond about 1 MHz and powers of more than a few tens of milliwatts.
More recent efforts to recast acoustic streaming have produced useful closed-form representations of the phenomena valid even for high frequencies and high powers, so-called fast streaming, but with dauntingly complex intermediate steps (Orosco and Friend, 2022). Ample opportunity remains in further describing acoustic streaming with analysis more appropriate to modern uses of the phenomena. Work is still underway to improve these modern approaches and overcome the flaws of past models in acoustic streaming, but researchers are also working on new forms of acoustic streaming in hopes of overcoming one of its key flaws: an inability to pump fluids against a significant pressure head. Acoustic streaming drives flow only against weak pressure gradients of less than approximately 1 kPa. However, by enlisting the finite amplitude deformation of the boundary with the propagation of the acoustic wave through the fluid bulk, a new form of acoustic streaming in confined media—acoustogeometric streaming—has been discovered in which the non-linear coupling between the motion of the boundary and the acoustic wave drives rapid fluid flow in a channel and against adverse pressures exceeding 1 MPa (Zhang et al., 2021a), far superior to classic streaming. Though reminiscent of peristaltic pumping and the Liebau pump mechanism (Davtyan and Sarvazyan, 2021), in fact the mechanism relies on acoustic wave propagation in the fluid, unlike these schemes. It is also sufficient to drive rapid fluid flows in nanochannels and to transport, split, combine, and even mix 20 to 200 fL droplets within (Zhang et al., 2021b). A notable use of acoustic streaming in confined flows is in rechargeable batteries, where it has been used to rapidly recharge previously unrechargeable lithium metal batteries (Huang et al., 2020) and to make 10-min charging possible in lithium ion batteries for up to 2,000 cycles (Huang et al., 2022). If driven continuously, large amplitude acoustic waves streaming can lead to significant heating in milli-scale and larger frozen and fluid samples (Horesh et al., 2023), but because of the dominance of surface area-driven effects together with the rapid flow, at micro-scale and smaller, the heating is mitigated by rapid conduction into the surrounding materials.
There is also work proceeding on understanding the nature of acoustic streaming around obstacles and through complex media. Zhang and Rallabandi (2022) demonstrated with careful work that the one and two-dimensional representations of acoustic streaming (Lighthill, 1978; Dentry et al., 2014) may be inadequate to fully describe even the qualitative behavior of confined flows. Instead, because the acoustic wave propagation is made more complex by the confinement, fully three-dimensional representations may be necessary to accurately describe the flow, even in slow streaming conditions. By introducing complex forms of the acoustic wave that, for example, contains helical propagation components that add in an angular momentum flux in addition to the axially propagating component pioneered by Hefner and Marston (1999) and analyzed in careful detail by Zhang and Marston (2011), it becomes possible to selectively manipulate particles in fluid samples (Jiang et al., 2016; Tian et al., 2019; Gong and Baudoin, 2021), an aspect described later. It is also possible to produce particle concentration in sample droplets suitable for biological samples (Zhang et al., 2021c) with acoustic streaming from spirally propagating acoustic waves, though it does require the use of a different cut of the piezoelectric substrate (Zhang N. et al., 2020), an aspect not often studied in modern acoustofluidics and one in which significant progress could be made into the future. Likewise, in many of these devices the electrodes used to generate the acoustic waves within and upon the piezoelectric substrate are typically cursory or directly derived from device designs used for telecommunications in the 1970s or even the original interdigital electrode design (White and Voltmer, 1965). However, recent work on electrode design tailored to acoustofluidics appears to offer distinct improvements in performance and capabilities, such as the remarkable multielectrode structure used to selectively trap particles described later (Gong and Baudoin, 2021), an “unapodization” approach to produce a laterally uniform acoustic wave (Zhang et al., 2021d), or even to completely remove the electrode from the substrate and using a flexible substrate simply pressed into contact with it instead (Dumčius et al., 2023).
Much of what has come to define acoustofluidics has depended on the interaction between acoustic waves and small objects suspended in fluids, dating from particle propulsion (King, 1934) and patterning (Kundt, 1866) that still produce valuable research questions today (Tan et al., 2007a; Dorrestijn et al., 2007; Zhou et al., 2016). Though Lord Rayleigh (Strutt, 1883) was one of the first researchers to explain the effects of acoustically-driven forces on particles—acoustophoresis—a more thorough treatment was produced by King (1934), though he assumed incompressible fluids and particles in a manner incompatible with most of today’s work. Today’s research on acoustofluidics typically directly or indirectly relies upon a classic publication by Gor’kov (2014), where a potential function was defined that streamlined the computational effort to determine the forces that appear on particles. Gor’kov’s method permits the determination of the forces on small or Rayleigh particles from an acoustic field around them. For many years, particle manipulation using acoustic waves relied on standing waves in a fluid, with numerous publications reporting various perturbations of this basic arrangement.
Roughly coincident with the advent of surface acoustic wave devices to access higher frequencies in a format compatible with the needs of microfluidics (Stone et al., 2004), traveling waves or a combination of standing and traveling waves were found to be useful in producing particle patterning and manipulation in droplets (Tan et al., 2007b; Li et al., 2008), plain channels (Tan et al., 2009a), channels with wall patterning (Behrens et al., 2015) to prevent reflections and standing waves, focusing electrodes (Destgeer et al., 2013) to accomplish the same aim, changing frequencies with split electrodes (Tan et al., 2009b), tilted electrodes (Collins et al., 2016) for precise particle separations across the width of a fluidic channel, in polymer devices (Moiseyenko and Bruus, 2019), and multiple devices in series (Ng and Neild, 2021). The analysis of particle behavior in fluids also rapidly progressed in this time, with the elimination of assumptions made in King’s work by Yosioka and Kawasima (1955), though with a complex presentation by Doinikov (1996) and Doinikov (2006) in later years, made more cogent by Bruus’ efforts even later on (Settnes and Bruus, 2012), though it is important to note that there is an error in that paper: their predictions for the viscous force for the traveling wave case has a factor of two error, explained in a pair of works by Marston and Zhang (Marston, 2013; Marston and Zhang, 2016). These efforts (Doinikov, 1996; Doinikov, 2006; Settnes and Bruus, 2012) notably go through the difficult process of including viscous and thermal effects in particle manipulation from the acoustic waves, which are important in many modern acoustofluidics devices. Related to this effort was the discovery of Saito et al. (2001) and Shi et al. (2009) and thorough analysis (O et al., 2018) of acoustic tweezing—capture of one or a small number (Gong and Baudoin, 2019) of particles by acoustic waves, and the elucidation of acoustic streaming’s effects on propelling particles (Nadal and Lauga, 2014).
In recent years, researchers have reported more thorough approaches to understanding how particles are being manipulated. Guo et al. (2016), report the ability to precisely control particle positioning in microfluidic devices fully in three dimensions. Kang et al. (2018), report the formation of cellular cylindroids using acoustic waves as a precursor to functional tissue in understanding inadequate blood supply. Acoustic tweezers, too, have continued to be refined and improved, particularly with spiral electrode structures (Baudoin et al., 2019; Tian et al., 2019; Baudoin et al., 2020; Gong and Baudoin, 2021) that accommodate dynamic rearrangement of the particle patterns. However, the most innovative recent discovery regarding multiple particle manipulation is perhaps the adoption of holography to produce complex patterns in two (Melde et al., 2016) and three dimensions (Melde et al., 2023).
Beyond particles, substantial work has also been conducted in examining the effects of acoustic waves on bubbles, both in terms of manipulating them (Bertin et al., 2015) and inducing acoustic streaming around them (Rallabandi et al., 2014). Pioneering work on the forces produced upon the bubbles from acoustic waves was provided by Eller (1968), particularly for bubbles smaller than the size required for resonance, Asaki and Marston (1994) for bubbles larger than the size required for resonance, and Lee and Wang (1993). Submicron gas vesicles formed internally by bacteria and algae to resist sedimentation and to cause them to float towards the surface of water—where there may be abundant light—has been found to be a durable alternative to microbubbles used in acoustic contrast imaging and, potentially, targeted delivery of drugs or serving as a nucleation site for cavitation. Researchers have shown an understanding of the underlying resonance behavior of these vesicles, showing they resonate at over 1 GHz (Zhang S. et al., 2020), but vastly more important has been the demonstration of their ability to selectively bind to specific cells for manipulation (Wu et al., 2023).
Moreover, active particles—motile cells—have been explored as interesting measurement devices for describing the pattern of acoustic waves in devices (Kim et al., 2021a) and to determine the amplitude of the acoustic forces in two (Kim et al., 2021b) and three dimensions (Cui et al., 2023). Motile particles have been shown to strongly affect the rheology of fluids via experiments enabled by acoustically-driven jetting (McDonnell et al., 2015), in agreement with analytical predictions (Saintillan, 2010). Researchers have also made progress on understanding the discrepancies present between analysis and experiments, with the discovery of the importance of matching, or at a minimum, predicting acoustic impedances (Baek et al., 2021). There also has been work on replacing soft microfluidics materials with bonded glass or silicon to improve acoustic transmission and reduce losses for improved efficiency (Langelier et al., 2012).
Acoustofluidics also serves in shaping the fluid interface at the boundary between water, oil, or air. The phenomenon of jetting was a principal driver of the start of modern acoustofluidics, with Shiokawa et al. (1989) presenting the curious jetting of droplets of water from the surface of SAW devices in the 1990s, followed by early demonstrations of atomization (Kurosawa et al., 1995). Over the years, there have been many studies of jetting and atomization, with various attempts at trying to both better understand the phenomena and to utilize it.
Jetting via SAW devices has produced useful models for acoustic streaming that take into account both the viscosity and the compressibility of the fluid (Tan et al., 2009a); both are important in properly predicting the formation of the jet. Such jets were generated from vertical ejection against gravity with symmetrically induced acoustic waves at high intensity for a short period. This format produced a means to rapidly form a thin fluid filament across a narrow gap: an extensional rheometer. The filament narrows over time due to drainage of the fluid axially along the filament. This, in turn is controlled by the viscosity of the fluid, such that the diameter of the fluid filament reduces over time in a manner dependent upon the extensional viscosity of the fluid and the presence of large molecules (Bhattacharjee et al., 2011) or even active particles (McDonnell et al., 2015) that alters the stability of the fluid filament. It has been known, however, that the jet could be ejected at an angle since Shiokawa’s time, dependent in a complex way on the relative amplitude of SAW coming into the parent droplet responsible for generating the jet. This was finally explained in a recent work where jetting angles exceeding the Rayleigh angle were shown to occur due to surface tension-mediated effects (Connacher et al., 2020). Ample work remains, however, as illustrated by a recent fascinating work in which individual droplets were extracted from a fluid interface using acoustic waves (Lirette et al., 2019) that produce an unprecedented pulling force (Fan et al., 2021) via vortices that likewise can be generated even in inhomogeneous materials (Fan et al., 2019).
With atomization, early work mainly attempted to produce basic characterization of the phenomena through either imaging (Barreras et al., 2002) or physical analogy (James et al., 2003; Qi et al., 2008), though the challenge has been to produce a physical understanding of the phenomena that stands up to greater scrutiny. Recent work has indicated the reason for the challenge is the fact the destabilization of the fluid interface necessary for the atomization occurs via non-linear coupling of the standing wave generated in the parent fluid. As the fluid interface deforms, the standing wave pattern shifts, changing the acoustic pressure distribution on the fluid interface, changing its shape. At low amplitudes, this deformation transitions from a static configuration (Manor et al., 2011) to a linear, harmonic (Tan et al., 2010) oscillation. As the oscillation increases in amplitude, however, it quickly becomes non-linear (Zhang et al., 2023), exhibiting strong turbulence (Orosco and Friend, 2023), making linear stability theory, approximate solution methods, and similar traditionally useful approaches ineffective. Nonetheless, many applications have been explored by researchers in recent years, from generation of nanoparticles (Alvarez et al., 2008) to the generation of intercellular matrix atomization to identify molecule concentrations in mass spectroscopy (Bllaci et al., 2013), and delivery of gene therapeutics-based vaccines via the lungs (Rajapaksa et al., 2014). Many aspects of atomization require further study, from the fundamental physics underpinning the phenomena to better designs (Collignon et al., 2018) for reliably and continuously producing droplets.
Because the principal use of microfluidics technologies is in the improvement of healthcare diagnostics and treatment, many of the applications of acoustofluidics have likewise been in this discipline (Rufo et al., 2022). The vast majority of particle manipulation work, for example, has ultimately been pursued due to a desire to pattern, sort (Franke et al., 2010; Zhang et al., 2021c), propel (Bo et al., 2009; Fujii et al., 2021), or agglomerate cells in wells (Kurashina et al., 2017) or open dishware (Mei et al., 2022). Exosome trapping has been an important topic of research via acoustofluidics (Habibi et al., 2020), as exosomes may be produced by cancer cells as they metastasize and may contain genetic information sufficient to identify their source. The release and transport of cells in cell culture flasks and media using acoustic streaming and pressure has helped to show that the use of trypsin is not always necessary to release cells (Kurashina et al., 2019) and that overall proliferation may be improved by acoustically-driven recirculation. The proliferation and viability of adherent cells has also been examined in conjunction with acoustic waves, using standing-wave ultrasound in a microfluidic chip (Hultström et al., 2007) and cells suspended in a droplet upon a SAW device (Li et al., 2009), demonstrating that cells survive and proliferate despite exposure to ultrasound above 1 MHz. Even better, it has recently been shown that exposing immotile sperm to standing wave SAW renders them motile, a potentially revolutionary result in treating impotence and in animal husbandry (Gai et al., 2022).
In the past few years, organoids—small three-dimensional tissue cultures derived from stem cells—have been found to be promising in identifying cancer treatments, how diseases develop, the communication between organs in homeostasis, and in understanding how the organs collectively respond to environmental stressors. Forming them has traditionally been tedious, but the adoption of acoustic manipulation methods has transformed organoid formation into a much more straightforward process using a well-based approach (Olofsson et al., 2018; Sandström et al., 2022). Also using acoustofluidics in a well-based approach, Ao et al. (2022) have shown via brain organoids that the aging of the human brain may principally be driven by monocytes. Traveling acoustic waves have also been used to rapidly develop blood vessels in three-dimensional tissue constructs that used human umbilical vein endothelial cells (Imashiro et al., 2021), an important part of tissue engineering that has eluded researchers for many years. Likewise, it has long been difficult to accurately position particles in the in vivo context, as tissue elasticity and potential uptake of particles in to the lymph or cardiovascular systems can quickly reposition such particles. Microbubbles were selectively manipulated within the vasculature of zebrafish embryos without disturbing the surrounding flow of blood (Jooss et al., 2022), showing a remarkable means to trap particles over a long period of time in live tissue. Durrer et al. (2022) introduced acoustic devices at the end of a robotics system, demonstrating the trapping, manipulation, pumping, and mixing of fluids, particles, and zebrafish embryos at will in a microfluidic system.
Drug release schemes have also been considered over the years, mainly through the use of small molecules through the skin or timed-release oral delivery, or even the use of microneedle patches that facilitate larger molecule delivery across the skin. Demonstrating the use of acoustically-driven microneedles, Xu et al. (2023) showed the ability to reverse anaphylaxis via a multiple-dose delivery of epinephrine across the skin, with results significantly improved over needle-based injectors for this application. Endovascular drug delivery via injectables, whether quick release or delayed release through encapsulated drugs has also long been studied, but an important missing tool is the ability to target specific tissues deep within the body and outside the range of optical techniques and without the cost of magnetic field systems (i.e., magnetic resonance imaging equipment). There have been attempts at encapsulating or decorating particles or bubbles with drugs so that ultrasound can be used to release the drugs at a particular location, such as a cancer tumor. A recent work reporting supercritical carbon dioxide-formed liposomes produces a much more efficient release of drugs upon exposure to ultrasound than past microparticle-based approaches (Orita et al., 2023).
Sonogenetics is the use of ultrasound together with genetic modification of cells and tissues to produce mechanosensitive proteins that respond to the ultrasound in situ (Maresca et al., 2018). There is substantial work underway to expand the library of ultrasound-responsive ion channels and to better understand the mechanisms underpinning cellular response to ultrasound, especially by naive cells present in the nervous system (Cotero et al., 2022) that may produce a useful method for treating diabetes. Among the many potential channels, transreceptor potential A1 has demonstrated a response to ultrasound at 6.91 MHz (Duque et al., 2022). The mechanism of action was thought to involve cavitation or some sort of small-scale streaming phenomenon, but it appears that membrane deformation and consequent stretching is entirely sufficient to produce the measured effects, including the threshold response that requires a minimum level of ultrasound for ion channel activation (Vasan et al., 2021; Vasan et al., 2022). This promises to produce a method to activate or silence small groups of neurons at will in a non-invasive manner.
The utility of acoustics spans many disciplines, from agricultural sprays to batteries, medical diagnostics, and drug delivery. Today, with the development of acoustofluidics, the utility has never been greater. A key to its rapid growth into the future is the better understanding of the principles that underpin it, especially in the context of high frequencies and exceedingly small scales that today’s applications demand. The discipline will need to adopt the broader range of acoustic waveforms and generation methods, from SAW to the many other forms of guided waves and beyond. Today, researchers trained not only in acoustofluidics but also in applications are making rapid advancements, using their knowledge of the needs together with the toolbox of acoustics to produce valuable solutions. More integration to produce complete technologies and devices to solve today’s problems will be needed, and work that spans engineering, physics, and medicine will be needed as this field quickly grows from its modest roots.
JF: Writing–original draft.
The author(s) declare financial support was received for the research, authorship, and/or publication of this article. This work is funded by the Office of Naval Research via grant 13423461, the National Institutes of Health via grant R01NS115591-01, and the National Science Foundation via grant 2224740.
The author declares that the research was conducted in the absence of any commercial or financial relationships that could be construed as a potential conflict of interest.
The author JF declared that they were an editorial board member of Frontiers at the time of submission. This had no impact on the peer review process and the final decision.
All claims expressed in this article are solely those of the authors and do not necessarily represent those of their affiliated organizations, or those of the publisher, the editors and the reviewers. Any product that may be evaluated in this article, or claim that may be made by its manufacturer, is not guaranteed or endorsed by the publisher.
Alvarez, M., Yeo, L., and Friend, J. (2008). Rapid generation of protein aerosols and nanoparticles via surface acoustic wave atomization. Nanotechnology 19, 455103. doi:10.1088/0957-4484/19/45/455103
Ao, Z., Song, S., Tian, C., Cai, H., Li, X., Miao, Y., et al. (2022). Understanding immune-driven brain aging by human brain organoid microphysiological analysis platform. Adv. Sci. 9, 2200475. doi:10.1002/advs.202200475
Asaki, T. J., and Marston, P. L. (1994). Acoustic radiation force on a bubble driven above resonance. J. Acoust. Soc. Am. 96, 3096–3099. doi:10.1121/1.411246
Baek, J., Kang, B., Rhyou, C., and Lee, H. (2021). Effect of the sound speed mismatch between fluid and channel on the particle alignment in a standing surface acoustic wave device. Sensors Actuators B Chem. 346, 130442. doi:10.1016/j.snb.2021.130442
Barreras, F., Amaveda, H., and Lozano, A. (2002). Transient high-frequency ultrasonic water atomization. Exp. Fluids 33, 405–413. doi:10.1007/s00348-002-0456-1
Baudoin, M., Gerbedoen, J. C., Riaud, A., Matar, O. B., Smagin, N., and Thomas, J. L. (2019). Folding a focalized acoustical vortex on a flat holographic transducer: Miniaturized selective acoustical tweezers. Sci. Adv. 5, eaav1967–6. doi:10.1126/sciadv.aav1967
Baudoin, M., Thomas, J. L., Sahely, R. A., Gerbedoen, J. C., Gong, Z., Sivery, A., et al. (2020). Spatially selective manipulation of cells with single-beam acoustical tweezers. Nat. Commun. 11, 4244. doi:10.1038/s41467-020-18000-y
Behrens, J., Langelier, S., Rezk, A. R., Lindner, G., Yeo, L. Y., and Friend, J. R. (2015). Microscale anechoic architecture: Acoustic diffusers for ultra low power microparticle separation via traveling surface acoustic waves. Lab a Chip 15, 43–46. doi:10.1039/c4lc00704b
Bell, J. F. (1991). The late-twentieth century resolution of a mid-nineteenth century dilemma generated by the eighteenth-century experiments of Ernst Chladni on the dynamics of rods. Archive Hist. Exact Sci. 43, 251–273. doi:10.1007/bf00389434
Bertin, N., Spelman, T. A., Stephan, O., Gredy, L., Bouriau, M., Lauga, E., et al. (2015). Propulsion of bubble-based acoustic microswimmers. Phys. Rev. Appl. 4, 064012. doi:10.1103/PhysRevApplied.4.064012
Bhattacharjee, P. K., McDonnell, A., Prabhakar, R., Yeo, L., and Friend, J. (2011). Extensional flow of low viscosity fluids in capillary bridges formed by pulsed surface acoustic wave jetting. New J. Phys. 13, 023005–023014. doi:10.1088/1367-2630/13/2/023005
Bllaci, L., Kjellstrom, S., Eliasson, L., Friend, J. R., Yeo, L. Y., and Nilsson, S. (2013). Fast surface acoustic wave-matrix-assisted laser desorption ionization mass spectrometry of cell response from islets of Langerhans. Anal. Chem. 85, 2623–2629. doi:10.1021/ac3019125
Bok, M., Li, H., Yeo, L., and Friend, J. (2009). The dynamics of surface acoustic wave driven scaffold cell seeding. Biotechnol. Bioeng. 103, 387–401. doi:10.1002/bit.22243
Chladni, E. (1787). Entdeckungen uber die Theorie des Klanges. Leipzig, Germany: Weidmanns, Erben und Reich.
Collignon, S., Manor, O., and Friend, J. (2018). Improving and predicting fluid atomization via hysteresis-free thickness vibration of lithium niobate. Adv. Funct. Mater. 28. doi:10.1002/adfm.201704359
Collins, D. J., Devendran, C., Ma, Z., Ng, J. W., Neild, A., and Ai, Y. (2016). Acoustic tweezers via sub-time-of-flight regime surface acoustic waves. Sci. Adv. 2, e1600089. doi:10.1126/sciadv.1600089
Connacher, W., Orosco, J., and Friend, J. (2020). Droplet ejection at controlled angles via acoustofluidic jetting. Phys. Rev. Lett. 125, 184504. doi:10.1103/PhysRevLett.125.184504
Connacher, W., Zhang, N., Huang, A., Mei, J., Zhang, S., Gopesh, T., et al. (2018). Micro/nano acoustofluidics: Materials, phenomena, design, devices, and applications. Lab. Chip 18, 1952–1996. doi:10.1039/C8LC00112J
Cotero, V., Graf, J., Miwa, H., Hirschstein, Z., Qanud, K., Huerta, T. S., et al. (2022). Stimulation of the hepatoportal nerve plexus with focused ultrasound restores glucose homoeostasis in diabetic mice, rats and swine. Nat. Biomed. Eng. 6, 683–705. doi:10.1038/s41551-022-00870-w
Cui, M., Dutcher, S. K., Bayly, P. V., and Meacham, J. M. (2023). Robust acoustic trapping and perturbation of single-cell microswimmers illuminate three-dimensional swimming and ciliary coordination. Proc. Natl. Acad. Sci. 120, 22189511200–e2218951212. doi:10.1073/pnas.2218951120
Davtyan, R., and Sarvazyan, N. A. (2021). Output of a valveless liebau pump with biologically relevant vessel properties and compression frequencies. Sci. Rep. 11, 11505. doi:10.1038/s41598-021-90820-4
Dentry, M. B., Yeo, L. Y., and Friend, J. R. (2014). Frequency effects on the scale and behavior of acoustic streaming. Phys. Rev. E 89, 013203. doi:10.1103/PhysRevE.89.013203
Destgeer, G., Lee, K. H., Jung, J. H., Alazzam, A., and Sung, H. J. (2013). Continuous separation of particles in a PDMS microfluidic channel via travelling surface acoustic waves (TSAW). Lab a Chip 13, 4210–4216. doi:10.1039/c3lc50451d
Doinikov, A. (2006). Acoustic radiation pressure on a compressible sphere in a viscous fluid. J. Fluid Mech. 267, 1–22. doi:10.1017/s0022112094001096
Doinikov, A. (1996). On the radiation pressure on small spheres. J. Acoust. Soc. Am. 100, 1231–1233. doi:10.1121/1.415969
Dorrestijn, M., Bietsch, A., Açıkalın, T., Raman, A., Hegner, M., Meyer, E., et al. (2007). Chladni figures revisited based on nanomechanics. Phys. Rev. Lett. 98, 026102. doi:10.1103/physrevlett.98.026102
Dumčius, P., Mikhaylov, R., Zhang, X., Bareford, M., Stringer, M., Errington, R., et al. (2023). Dual-wave acoustofluidic centrifuge for ultrafast concentration of nanoparticles and extracellular vesicles. Small 19, 1–13. doi:10.1002/smll.202300390
Duque, M., Lee-Kubli, C., Tufail, Y., Magaram, U., Lopez, J. M., Edsinger, E., et al. (2022). Sonogenetic control of mammalian cells using exogenous transient receptor potential a1 channels. Nat. Commun. 13, 600–617. doi:10.1038/s41467-022-28205-y
Durrer, J., Agrawal, P., Ozgul, A., Neuhauss, S. C. F., Nama, N., and Ahmed, D. (2022). A robot-assisted acoustofluidic end effector. Nat. Commun. 13, 6370. doi:10.1038/s41467-022-34167-y
Eckart, C. (1948). Vortices and streams caused by sound waves. Phys. Rev. 73, 68–76. doi:10.1103/physrev.73.68
Eller, A. (1968). Force on a bubble in a standing acoustic wave. J. Acoust. Soc. Am. 43, 170–171. doi:10.1121/1.1910755
Fan, X. D., and Zhang, L. (2021). Phase shift approach for engineering desired radiation force: Acoustic pulling force example. J. Acoust. Soc. Am. 150, 102–110. doi:10.1121/10.0005491
Fan, X. D., Zou, Z., and Zhang, L. (2019). Acoustic vortices in inhomogeneous media. Phys. Rev. Res. 1, 032014. doi:10.1103/PhysRevResearch.1.032014
Faraday, M. (1831). On a peculiar class of acoustical figures; and on certain forms assumed by groups of particles upon vibrating elastic surfaces. Philosophical Trans. R. Soc. Lond. 121, 299–340.
Franke, T., Braunmuller, S., Schmid, L., Wixforth, A., and Weitz, D. A. (2010). Surface acoustic wave actuated cell sorting (sawacs). Lab a Chip 10, 789–794. doi:10.1039/b915522h
Friend, J. R., and Yeo, L. Y. (2011). Microscale acoustofluidics: Microfluidics driven via acoustics and ultrasonics. Rev. Mod. Phys. 83, 647–704. doi:10.1103/revmodphys.83.647
Fujii, G., Kurashina, Y., Terao, Y., Azuma, T., Morikawa, A., Kodeki, K., et al. (2021). Suspension culture in a t-flask with acoustic flow induced by ultrasonic irradiation. Ultrason. Sonochemistry 73, 105488. doi:10.1016/j.ultsonch.2021.105488
Gai, J., Dervisevic, E., Devendran, C., Cadarso, V. J., O’Bryan, M. K., Nosrati, R., et al. (2022). High-frequency ultrasound boosts bull and human sperm motility. Adv. Sci. 9, 2104362. doi:10.1002/advs.202104362
Gong, Z., and Baudoin, M. (2019). Particle assembly with synchronized acoustic tweezers. Phys. Rev. Appl. 12, 024045. doi:10.1103/physrevapplied.12.024045
Gong, Z., and Baudoin, M. (2021). Three-dimensional trapping and dynamic axial manipulation with frequency-tuned spiraling acoustical tweezers: A theoretical study. Phys. Rev. Appl. 16, 024034. doi:10.1103/physrevapplied.16.024034
Gor’kov, L. P. (2014). Selected papers of lev P. Gor’kov 5 toh tuck link. Singapore: World Scientific Publishing Co.
Guo, F., Mao, Z., Chen, Y., Xie, Z., Lata, J. P., Li, P., et al. (2016). Three-dimensional manipulation of single cells using surface acoustic waves. Proc. Natl. Acad. Sci. 113, 1522–1527. doi:10.1073/pnas.1524813113
Habibi, R., He, V., Ghavamian, S., de Marco, A., Lee, T. H., Aguilar, M. I., et al. (2020). Exosome trapping and enrichment using a sound wave activated nano-sieve (SWANS). Lab. Chip 20, 3633–3643. doi:10.1039/D0LC00623H
Hefner, B. T., and Marston, P. L. (1999). An acoustical helicoidal wave transducer with applications for the alignment of ultrasonic and underwater systems. J. Acoust. Soc. Am. 106, 3313–3316. doi:10.1121/1.428184
Horesh, A., Connacher, W., and Friend, J. (2023). Acoustothermal phase change and acoustically driven atomization for cold liquid microthrusters. Appl. Phys. Lett. 122, 1–7. doi:10.1063/5.0131467
Huang, A., Liu, H., Liu, P., and Friend, J. (2022). Overcoming the intrinsic limitations of fast charging lithium ion batteries using integrated acoustic streaming. Adv. Energy Sustain. Res. 4, 1–10. doi:10.1002/aesr.202200112
Huang, A., Liu, H., Manor, O., Liu, P., and Friend, J. (2020). Enabling rapid charging lithium metal batteries via surface acoustic wave-driven electrolyte flow. Adv. Mater. 32, 1907516. doi:10.1002/adma.201907516
Hultström, J., Manneberg, O., Dopf, K., Hertz, H., Brismar, H., and Wiklund, M. (2007). Proliferation and viability of adherent cells manipulated by standing-wave ultrasound in a microfluidic chip. Ultrasound Med. Biol. 33, 145–151. doi:10.1016/j.ultrasmedbio.2006.07.024
Imashiro, C., Azuma, T., Itai, S., Kuribara, T., Totani, K., Onoe, H., et al. (2021). Travelling ultrasound promotes vasculogenesis of three-dimensional-monocultured human umbilical vein endothelial cells. Biotechnol. Bioeng. 118, 3760–3769. doi:10.1002/bit.27852
James, A., Vukasinovic, B., Smith, M., and Glezer, A. (2003). Vibration-induced drop atomization and bursting. J. Fluid Mech. 476, 1–28. doi:10.1017/s0022112002002835
Jiang, X., Li, Y., Liang, B., Cheng, J. C., and Zhang, L. (2016). Convert acoustic resonances to orbital angular momentum. Phys. Rev. Lett. 117, 034301. doi:10.1103/PhysRevLett.117.034301
Jooss, V. M., Bolten, J. S., Huwyler, J., and Ahmed, D. (2022). In vivo acoustic manipulation of microparticles in zebrafish embryos. Sci. Adv. 8, eabm2785. doi:10.1126/sciadv.abm2785
Kang, B., Shin, J., Park, H. J., Rhyou, C., Kang, D., Lee, S. J., et al. (2018). High-resolution acoustophoretic 3d cell patterning to construct functional collateral cylindroids for ischemia therapy. Nat. Commun. 9, 5402. doi:10.1038/s41467-018-07823-5
Kim, M., Barnkob, R., and Meacham, J. M. (2021b). Rapid measurement of the local pressure amplitude in microchannel acoustophoresis using motile cells. J. Acoust. Soc. Am. 150, 1565–1576. doi:10.1121/10.0005910
Kim, M., Bayly, P. V., and Meacham, J. M. (2021a). Motile cells as probes for characterizing acoustofluidic devices. Lab a Chip 21, 521–533. doi:10.1039/d0lc01025a
King, L. (1934). On the acoustic radiation pressure on spheres. Proc. R. Soc. Lond. Ser. A, Math. Phys. Sci. 147, 212–240.
Kundt, A. (1866). Ueber eine neue art akustischer staubfiguren und über die anwendung derselben zur bestimmung der schallgeschwindigkeit in festen körpern und gasen. Ann. Phys. 203, 497–523. doi:10.1002/andp.18662030402
Kurashina, Y., Imashiro, C., Hirano, M., Kuribara, T., Totani, K., Ohnuma, K., et al. (2019). Enzyme-free release of adhered cells from standard culture dishes using intermittent ultrasonic traveling waves. Commun. Biol. 2, 393. doi:10.1038/s42003-019-0638-5
Kurashina, Y., Takemura, K., and Friend, J. (2017). Cell agglomeration in the wells of a 24-well plate using acoustic streaming. Lab. Chip 17, 876–886. doi:10.1039/c6lc01310d
Kurosawa, M., Watanabe, T., Futami, A., and Higuchi, T. (1995). Surface acoustic wave atomizer. Sensors Actuators A Phys. 50, 69–74. doi:10.1016/0924-4247(96)80086-0
Langelier, S., Yeo, L., and Friend, J. R. (2012). UV epoxy bonding for enhanced saw transmission and microscale acoustofluidic integration. Lab a Chip 12, 2970–2976. doi:10.1039/c2lc40085e
Laser, D., and Santiago, J. (2004). A review of micropumps. J. Micromechanics Microengineering 14, 35–64. doi:10.1088/0960-1317/14/6/r01
Lee, C. P., and Wang, T. G. (1993). Acoustic radiation force on a bubble. J. Acoust. Soc. Am. 93, 1637–1640. doi:10.1121/1.406823
Li, H., Dasvarma, A., Yeo, L., Friend, J., and Traianedes, K. (2009). Effect of surface acoustic waves on the viability, proliferation and differentiation of primary osteoblast-like cells. Biomicrofluidics 3, 034102. doi:10.1063/1.3194282
Li, H., Friend, J., and Yeo, L. (2008). Microfluidic colloidal island formation and erasure induced by surface acoustic wave radiation. Phys. Rev. Lett. 101, 084502. doi:10.1103/physrevlett.101.084502
Lighthill, J. (1978). Acoustic streaming. J. Sound Vib. 61, 391–418. doi:10.1016/0022-460x(78)90388-7
Lirette, R., Mobley, J., and Zhang, L. (2019). Ultrasonic extraction and manipulation of droplets from a liquid-liquid interface with near-field acoustic tweezers. Phys. Rev. Appl. 12, 061001. doi:10.1103/physrevapplied.12.061001
Manor, O., Dentry, M., Friend, J. R., and Yeo, L. Y. (2011). Substrate dependent drop deformation and wetting under high frequency vibration. Soft Matter 7, 7976–7979. doi:10.1039/c1sm06054f
Maresca, D., Lakshmanan, A., Abedi, M., Bar-Zion, A., Farhadi, A., Lu, G. J., et al. (2018). Biomolecular ultrasound and sonogenetics. Annu. Rev. Chem. Biomol. Eng. 9, 229–252. PMID: 29579400. doi:10.1146/annurev-chembioeng-060817-084034
Marston, P. L. (2013). Viscous contributions to low-frequency scattering, power absorption, radiation force, and radiation torque for spheres in acoustic beams. Proc. Meet. Acoust. 19, 045005. doi:10.1121/1.4799400
Marston, P. L., and Zhang, L. (2016). Unphysical consequences of negative absorbed power in linear passive scattering: Implications for radiation force and torque. J. Acoust. Soc. Am. 139, 3139–3144. doi:10.1121/1.4954044
McDonnell, A. G., Gopesh, T. C., Lo, J., O’Bryan, M., Yeo, L. Y., Friend, J. R., et al. (2015). Motility induced changes in viscosity of suspensions of swimming microbes in extensional flows. Soft Matter 11, 4658–4668. doi:10.1039/C4SM02742F
Mei, J., Vasan, A., Magaram, U., Takemura, K., Chalasani, S. H., and Friend, J. (2022). Well-free agglomeration and on-demand three-dimensional cell cluster formation using guided surface acoustic waves through a couplant layer. Biomed. Microdevices 24, 18–13. doi:10.1007/s10544-022-00617-z
Melde, K., Kremer, H., Shi, M., Seneca, S., Frey, C., Platzman, I., et al. (2023). Compact holographic sound fields enable rapid one-step assembly of matter in 3d. Sci. Adv. 9, eadf6182. doi:10.1126/sciadv.adf6182
Melde, K., Mark, A. G., Qiu, T., and Fischer, P. (2016). Holograms for acoustics. Nature 537, 518–522. doi:10.1038/nature19755
Moiseyenko, R. P., and Bruus, H. (2019). Whole-system ultrasound resonances as the basis for acoustophoresis in all-polymer microfluidic devices. Phys. Rev. Appl. 11, 014014. doi:10.1103/physrevapplied.11.014014
Nadal, F., and Lauga, E. (2014). Asymmetric steady streaming as a mechanism for acoustic propulsion of rigid bodies. Phys. Fluids 26. doi:10.1063/1.4891446
Ng, J. W., and Neild, A. (2021). Multiple outcome particle manipulation using cascaded surface acoustic waves (CSAW). Microfluid. Nanofluidics 25, 16–10. doi:10.1007/s10404-020-02417-4
Nyborg, W. L. (1965). Properties of polymers and nonlinear acoustics. Cambridge, UK: Academic Press. chap. 11: Acoustic Streaming.
Ozcelik, A., Rufo, J., Guo, F., Gu, Y., Li, P., Lata, J., et al. (2018). Acoustic tweezers for the life sciences. Nat. methods 15, 1021–1028. doi:10.1038/s41592-018-0222-9
Olofsson, K., Carannante, V., Ohlin, M., Frisk, T., Kushiro, K., Takai, M., et al. (2018). Acoustic formation of multicellular tumor spheroids enabling on-chip functional and structural imaging. Lab a Chip 18, 2466–2476. doi:10.1039/c8lc00537k
Orita, Y., Shimanuki, S., Okada, S., Nakamura, K., Nakamura, H., Kitamoto, Y., et al. (2023). Acoustic-responsive carbon dioxide-loaded liposomes for efficient drug release. Ultrason. Sonochemistry 94, 106326. doi:10.1016/j.ultsonch.2023.106326
Orosco, J., and Friend, J. (2023). Identification of weakly to strongly-turbulent three-wave processes in a micro-scale system. Chaos, Solit. Fractals 172, 1–7.
Orosco, J., and Friend, J. (2022). Modeling fast acoustic streaming: Steady-state and transient flow solutions. Phys. Rev. E 106, 045101–045117. doi:10.1103/physreve.106.045101
Qi, A., Yeo, L., and Friend, J. (2008). Interfacial destabilization and atomization driven by surface acoustic waves. Phys. Fluids 20, 074103. doi:10.1063/1.2953537
Rajapaksa, A. E., Ho, J. J., Qi, A., Bischof, R., Nguyen, T. H., Tate, M., et al. (2014). Effective pulmonary delivery of an aerosolized plasmid DNA vaccine via surface acoustic wave nebulization. Respir. Res. 15, 60–12. doi:10.1186/1465-9921-15-60
Rallabandi, B., Wang, C., and Hilgenfeldt, S. (2014). Two-dimensional streaming flows driven by sessile semicylindrical microbubbles. J. Fluid Mech. 739, 57–71. doi:10.1017/jfm.2013.616
Rufo, J., Cai, F., Friend, J., Wiklund, M., and Huang, T. (2022). Acoustofluidics for biomedical applications. Nat. Rev. Prim. 2, 30–21. doi:10.1038/s43586-022-00109-7
Saintillan, D. (2010). Extensional rheology of active suspensions. Phys. Rev. E 81, 056307. doi:10.1103/physreve.81.056307
Saito, M., Kitamura, N., and Terauchi, M. (2001). Micro-organism manipulation and microparticle arrangement by the use of ultrasonic standing waves. BioMEMS Smart Nanostructures (SPIE) 4590, 26–37.
Sandström, N., Carannante, V., Olofsson, K., Sandoz, P. A., Moussaud-Lamodière, E. L., Seashore-Ludlow, B., et al. (2022). Miniaturized and multiplexed high-content screening of drug and immune sensitivity in a multichambered microwell chip. Cell Rep. Methods 2, 100256. doi:10.1016/j.crmeth.2022.100256
Schlichting, H. (1932). Berechnung ebener periodischer grenzschichtstr omungen (calculation of plane periodic boundary layer streaming). Z. für Phys. 33, 327–335.
Settnes, M., and Bruus, H. (2012). Forces acting on a small particle in an acoustical field in a viscous fluid. Phys. Rev. E 85, 016327. doi:10.1103/physreve.85.016327
Shi, J., Ahmed, D., Mao, X., Lin, S., Lawit, A., and Huang, T. (2009). Acoustic tweezers: Patterning cells and microparticles using standing surface acoustic waves (SSAW). Lab a Chip 9, 2890–2895. doi:10.1039/b910595f
Shiokawa, S., Matsui, Y., and Ueda, T. (1989). Study on SAW streaming and its application to fluid devices. Jpn. J. Appl. Phys. 29, 137–139. doi:10.7567/jjaps.29s1.137
Stone, H., Stroock, A., and Ajdari, A. (2004). Engineering flows in small devices—Microfluidics toward a lab-on-a-chip. Annu. Rev. Fluid Mech. 36, 381–411. doi:10.1146/annurev.fluid.36.050802.122124
Strutt, J. W. (1883). On the circulation of air observed in Kundt’s tubes, and on some allied acoustical problems. Proc. R. Soc. Lond. 36, 10–11. doi:10.1098/rspl.1883.0075
Tan, M. K., Friend, J., and Yeo, L. Y. (2009a). Interfacial jetting phenomena induced by focused surface vibrations. Phys. Rev. Lett. 103, 024501. doi:10.1103/physrevlett.103.024501
Tan, M. K., Friend, J. R., Matar, O. K., and Yeo, L. Y. (2010). Capillary wave motion excited by high frequency surface acoustic waves. Phys. Fluids 22, 112112. doi:10.1063/1.3505044
Tan, M. K., Tjeung, R., Ervin, H., Yeo, L. Y., and Friend, J. (2009b). Double aperture focusing transducer for controlling microparticle motions in trapezoidal microchannels with surface acoustic waves. Appl. Phys. Lett. 95. doi:10.1063/1.3238313
Tan, W. M., Friend, J., and Yeo, L. (2007a). Direct visualization of surface acoustic waves along substrates using smoke particles. Appl. Phys. Lett. 91, 224101. doi:10.1063/1.2814054
Tan, W. M., Friend, J., and Yeo, L. (2007b). Microparticle collection and concentration via a miniature surface acoustic wave device. Lab a Chip 7, 618–625. doi:10.1039/b618044b
Tian, Z., Yang, S., Huang, P. H., Wang, Z., Zhang, P., Gu, Y., et al. (2019). Wave number-spiral acoustic tweezers for dynamic and reconfigurable manipulation of particles and cells. Sci. Adv. 5, eaau6062. doi:10.1126/sciadv.aau6062
Vasan, A., Allein, F., Duque, M., Magaram, U., Boechler, N., Chalasani, S. H., et al. (2022). Microscale concert hall acoustics to produce uniform ultrasound stimulation for targeted sonogenetics in hstrpa1-transfected cells. Adv. NanoBiomed Res. 2, 2100135–2100139. doi:10.1002/anbr.202100135
Vasan, A., Orosco, J., Magaram, U., Duque, M., Weiss, C., Tufail, Y., et al. (2021). Ultrasound mediated cellular deflection results in cellular depolarization. Adv. Sci. 9(2101950), 1–12. doi:10.1002/advs.202101950
White, R., and Voltmer, F. (1965). Direct piezoelectric coupling to surface elastic waves. Appl. Phys. Lett. 7, 314–316. doi:10.1063/1.1754276
Wu, D., Baresch, D., Cook, C., Ma, Z., Duan, M., Malounda, D., et al. (2023). Biomolecular actuators for genetically selective acoustic manipulation of cells. Sci. Adv. 9, eadd9186. doi:10.1126/sciadv.add9186
Xu, J., Cai, H., Wu, Z., Li, X., Tian, C., Ao, Z., et al. (2023). Acoustic metamaterials-driven transdermal drug delivery for rapid and on-demand management of acute disease. Nat. Commun. 14, 869. doi:10.1038/s41467-023-36581-2
Yosioka, K., and Kawasima, Y. (1955). Acoustic radiation pressure on a compressible sphere. Acustica 5, 167–173.
Zhang, L., and Marston, P. L. (2011). Angular momentum flux of nonparaxial acoustic vortex beams and torques on axisymmetric objects. Phys. Rev. E 84, 065601. doi:10.1103/PhysRevE.84.065601
Zhang, N., Horesh, A., Floer, C., and Friend, J. (2021d). Unapodization: A method to produce laterally uniform surface acoustic waves for acoustofluidics. J. Micromechanics Microengineering 31, 104001–104012. doi:10.1088/1361-6439/ac1d2d
Zhang, N., Horesh, A., and Friend, J. (2021b). Manipulation and mixing of 200 femtoliter droplets in nanofluidic channels using MHz-order surface acoustic waves. Adv. Sci. 8, 2100408–2100412. doi:10.1002/advs.202100408
Zhang, N., Horesh, A., Manor, O., and Friend, J. (2021a). Powerful acoustogeometric streaming from dynamic geometric nonlinearity. Phys. Rev. Lett. 126, 164502–164505. doi:10.1103/physrevlett.126.164502
Zhang, N., Mei, J., Gopesh, T., and Friend, J. (2020a). Optimized, omnidirectional surface acoustic wave source: 152° Y-rotated cut of lithium niobate for acoustofluidics. IEEE Trans. Ultrasonics, Ferroelectr. Freq. Control 67, 2176–2186. doi:10.1109/TUFFC.2020.2993766
Zhang, N., Zuniga-Hertz, J. P., Zhang, E. Y., Gopesh, T., Fannon, M. J., Wang, J., et al. (2021c). Microliter ultrafast centrifuge platform for size-based particle and cell separation and extraction using novel omnidirectional spiral surface acoustic waves. Lab a Chip 21, 904–915. doi:10.1039/D0LC01012J
Zhang, S., Huang, A., Bar-Zion, A., Wang, J., Mena, O. V., Shapiro, M. G., et al. (2020b). The vibration behavior of sub-micrometer gas vesicles in response to acoustic excitation determined via laser Doppler vibrometry. Adv. Funct. Mater. 30, 2000239. doi:10.1002/adfm.202000239
Zhang, S., Orosco, J., and Friend, J. (2023). Onset of visible capillary waves from high-frequency acoustic excitation. Langmuir 39, 3699–3709. doi:10.1021/acs.langmuir.2c03403
Zhang, X., and Rallabandi, B. (2022). Three-dimensional streaming around an obstacle in a Hele-Shaw cell. J. Fluid Mech. 961, A35–A19. doi:10.1017/jfm.2023.276
Keywords: acoustofluidics, acoustic streaming, acoustophoresis, acoustic forces, acoustic pressure, non-linear acoustics, sonogenetics, acoustogeometric streaming
Citation: Friend J (2023) Acoustofluidics. Front. Acoust. 1:1261027. doi: 10.3389/facou.2023.1261027
Received: 18 July 2023; Accepted: 22 September 2023;
Published: 02 October 2023.
Edited by:
Likun Zhang, University of Mississippi, United StatesReviewed by:
Feiyan Cai, Chinese Academy of Sciences (CAS), ChinaCopyright © 2023 Friend. This is an open-access article distributed under the terms of the Creative Commons Attribution License (CC BY). The use, distribution or reproduction in other forums is permitted, provided the original author(s) and the copyright owner(s) are credited and that the original publication in this journal is cited, in accordance with accepted academic practice. No use, distribution or reproduction is permitted which does not comply with these terms.
*Correspondence: James Friend, amZyaWVuZEB1Y3NkLmVkdQ==
Disclaimer: All claims expressed in this article are solely those of the authors and do not necessarily represent those of their affiliated organizations, or those of the publisher, the editors and the reviewers. Any product that may be evaluated in this article or claim that may be made by its manufacturer is not guaranteed or endorsed by the publisher.
Research integrity at Frontiers
Learn more about the work of our research integrity team to safeguard the quality of each article we publish.