- 1Department of Pharmacological and Physiological Science, School of Medicine, Saint Louis University, St. Louis, MO, United States
- 2Department of Pediatrics, School of Medicine, Saint Louis University, St. Louis, MO, United States
The mammalian diving response (DR) is a remarkable behavior that was first formally studied by Laurence Irving and Per Scholander in the late 1930s. The DR is called such because it is most prominent in marine mammals such as seals, whales, and dolphins, but nevertheless is found in all mammals studied. It consists generally of breathing cessation (apnea), a dramatic slowing of heart rate (bradycardia), and an increase in peripheral vasoconstriction. The DR is thought to conserve vital oxygen stores and thus maintain life by directing perfusion to the two organs most essential for life—the heart and the brain. The DR is important, not only for its dramatic power over autonomic function, but also because it alters normal homeostatic reflexes such as the baroreceptor reflex and respiratory chemoreceptor reflex. The neurons driving the reflex circuits for the DR are contained within the medulla and spinal cord since the response remains after the brainstem transection at the pontomedullary junction. Neuroanatomical and physiological data suggesting brainstem areas important for the apnea, bradycardia, and peripheral vasoconstriction induced by underwater submersion are reviewed. Defining the brainstem circuit for the DR may open broad avenues for understanding the mechanisms of suprabulbar control of autonomic function in general, as well as implicate its role in some clinical states. Knowledge of the proposed diving circuit should facilitate studies on elite human divers performing breath-holding dives as well as investigations on sudden infant death syndrome (SIDS), stroke, migraine headache, and arrhythmias. We have speculated that the DR is the most powerful autonomic reflex known.
Introduction
The complexity of an animal’s behavior is paralleled by the complexity of the neural systems driving that behavior. Indeed, numerous neurons within the mammalian brain modulate autonomic activity and these areas are interconnected in complex ways. Despite this complexity, an orderly functional organization exists because specific autonomic responses result from a specific stimulus, and these adjustments are appropriate to physiological needs. Moreover, behaviors that serve basic vegetative functions are usually less complex and more uniform across species. The substrate for “simple” reflexive behaviors is thought to be neural circuits located within the brainstem and spinal cord; it is probable that some of these same circuits are influenced by the more rostral parts of the brain and are utilized in more complex behaviors. It is therefore worthwhile to direct considerable effort toward studying those circuits that are the simplest, the most organized, and the most automatic. A behavior validating such a statement is the mammalian diving response (DR), a mechanism that operates in a variety of animals across a wide range of circumstances, thus suggesting it to be of fundamental physiological significance (Scholander, 1963). The threat of asphyxiation in numerous species quite distantly related was the common theme in the pioneering studies of Irving and Scholander, who showed an organism’s primary response was bradycardia, i.e., the dive reflex. A history of their work on various species’ responses to asphyxia has recently been documented (Hagen, 2018). The somatoautonomic DR is powerful and modulates intrinsic rhythms such as respiration and heart rate, as well as basic homeostatic reflexes such as the chemoreceptor and baroreceptor reflexes. The neural pathways mediating the DR are being explored; this treatise provides a summation of current understanding.
The mammalian DR is usually considered to consist of three independent reflex behaviors: an apnea, a parasympathetically mediated bradycardia and a sympathetically mediated peripheral vasoconstriction (Irving, 1938, 1939; Irving et al., 1942); splenic contraction is sometimes considered a fourth behavior by some (Cabanac et al., 1997, 1998; Cabanac, 2000). These autonomic adjustments are marked mostly in marine mammals such as seals, dolphins, or whales, which spend considerable time submerged underwater. Thus, when pinnipeds or cetaceans dive underwater, oxygen from air becomes non-existent, and the animal is forced to use oxygen bound either to hemoglobin in its blood or myoglobin in its muscles (Guyton et al., 1995; Noren and Williams, 2000), or to depend on anaerobic glycolysis (see Panneton, 2013 for discussion and references). Many of the physiological consequences of diving have been deciphered, and adaptations of cetaceans and pinnipeds widely reported (Kooyman et al., 1981; Butler and Jones, 1982, 1997; Blix and Folkow, 1983; Elsner and Gooden, 1983; de Burgh Daly, 1984; Kooyman and Ponganis, 1998; Panneton, 2013; Davis, 2014). The DR is also found in birds (prominently in ducks and penguins) (Butler and Jones, 1982, 1997; Kooyman and Ponganis, 1998; Ponganis and Kooyman, 2000), and even fish show a bradycardia in hypoxic environments (Scholander, 1963; Elsner and Gooden, 1983; Farrell, 2007).
This review focuses on the neural control of the DR only in terrestrial mammals, particularly rodents, and differs from most previous reviews which emphasize the adaptations and physiological consequences of aquatic mammals to underwater submersion with little or no discussion on central neural integration. This differs from a previous review (Panneton, 2013) by emphasizing a brainstem reflex circuit driving the DR and a more detailed exploration on its suprabulbar control as well as how this response may help humans clinically. Our premise is to decipher a conserved neural circuit driving the DR, which we suspect is uniform across species. We do this in rodents simply because these small mammals are abundant and bred for laboratory use, and much is known of their physiology and nervous systems (NSs), and rodents are significantly less challenging ethically than use of large marine mammals. Neural circuits located within the brainstem and the spinal cord are described, since they are the simplest, most organized, and most automatic. If our hypothesis is correct, the circuit outlined for rodents should be mimicked in the brains of higher marine mammals, as well as be applicable to humans. It may also provide a base for future studies on the mechanisms underlying the stunning physiologic changes induced in the mammalian DR. This review merely augments the wealth of knowledge obtained from the numerous studies obtained from marine mammals.
The DR is found in all mammals studied, including those terrestrial (Butler and Jones, 1982, 1997; Blix and Folkow, 1983; Elsner and Gooden, 1983; de Burgh Daly, 1984; Ferretti, 2001; Davis et al., 2004; Foster and Sheel, 2005). An animal model for many years was the feral muskrat, Ondatra zibethicus (Martin et al., 1977; Doyle et al., 1988; Panneton, 1990, 1991a,b; Panneton and Watson, 1991; Panneton and Yavari, 1995; Panneton et al., 1996, 2000; McCulloch and Panneton, 1997; McCulloch et al., 1999a) since this semi-aquatic rodent possesses a brisk and reliable DR even when anesthetized (Koppányi et al., 1929; Irving, 1939; Drummond and Jones, 1979; McCulloch and Jones, 1990). However, this feral species which must be trapped wild is somewhat difficult to obtain reliably, and no information about its brain was known; for these reasons, we sought another animal model. We found the common laboratory rat is better suited for deciphering the neural circuits driving the DR. Rats can be trained easily to dive underwater (McCulloch, 2012; Panneton et al., 2014; Supplementary Video S1), and the DR can be documented (Figure 1) with implanted telemetric transmitters (McCulloch et al., 2010; Panneton et al., 2010a, b, 2012a, 2014). The DR has also been documented recently in mice (Hult et al., 2019); the authors also provide a simple training protocol for these irascible creatures. These studies have shown that responses in diving rodents mimic those of marine mammals; the use of laboratory rodents for studying the DR has been reviewed recently (McCulloch, 2012). Thus, we and others (Lin, 1974; Lin and Baker, 1975; McCulloch et al., 1997, 2010; McCulloch, 2005; Fahlman et al., 2011) have shown that laboratory rats have an innate DR marked by a bradycardia reaching 80% in 100% of rats 100% of the time during submersion (Figure 1), which is typical of reflex behaviors. This reproducibility implies that reflex circuits are probably utilized by mammals to manifest the DR. However, the central neural pathways driving the DR have been relatively unexplored in any species. A purpose of our laboratory is to define the neural circuits driving the DR, especially those inducing the apnea, bradycardia, and peripheral vasoconstriction. We consider the DR the most powerful autonomic reflex known.
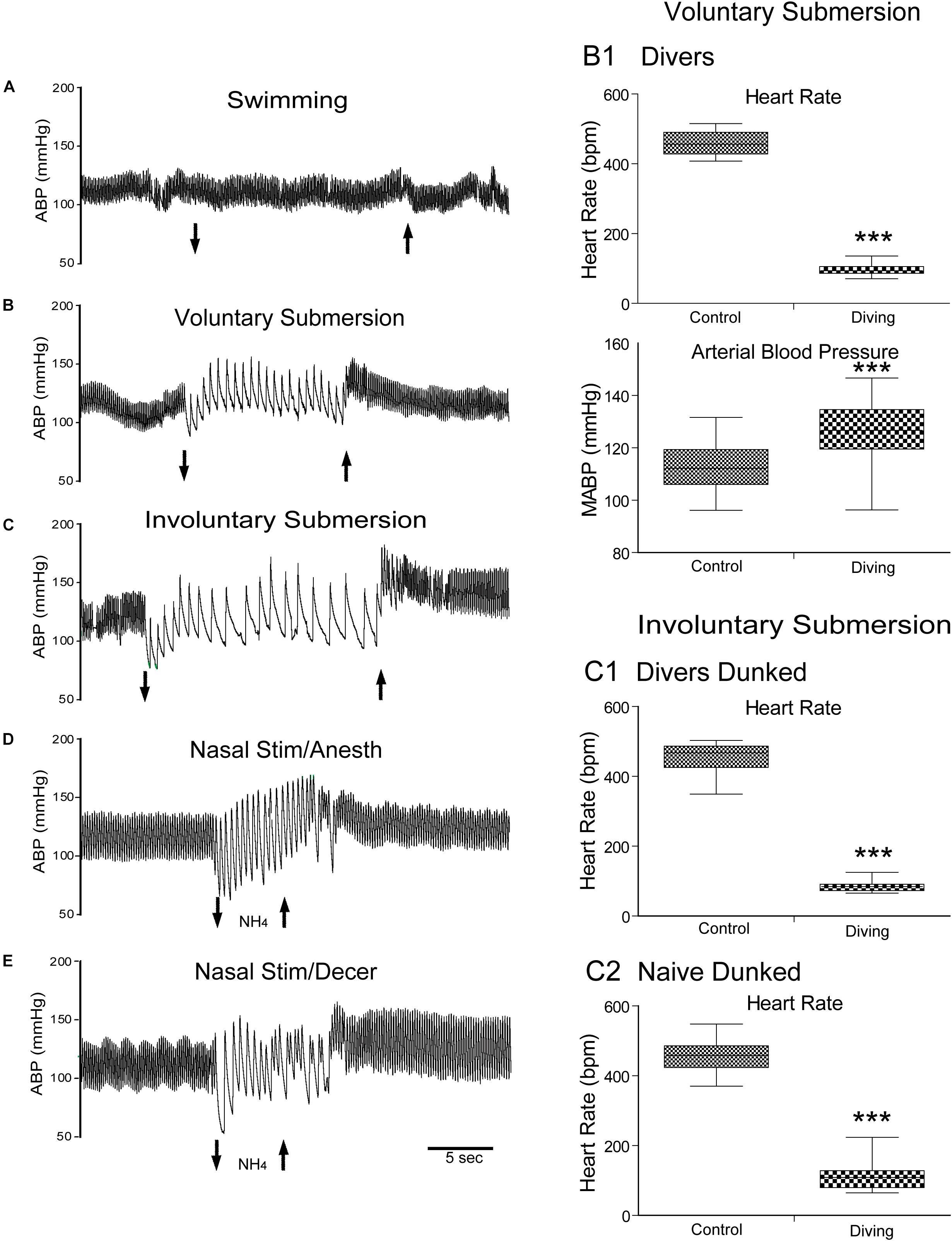
Figure 1. The mammalian diving response can be induced in different preparations of laboratory rodents. Cardiovascular responses either to swimming, underwater submersion, or nasal stimulation are shown. Traces of arterial blood pressure (ABP) of rats swimming (A), voluntarily diving underwater (B), involuntarily dunked underwater (C), stimulated nasally with ammonia vapors under anesthesia (D), and stimulated nasally with ammonia vapors after decerebration (E) are presented. Note the marked bradycardia and increase in ABP after submersion (B,C) or nasal stimulation (D,E) but no changes after swimming on the water’s surface (A). Composite of cardiovascular results of rats voluntarily diving underwater (B1; 30 rats, N = 104), involuntary dunking of rats trained to dive underwater (C1; 17 rats, n = 59), and involuntary dunking of untrained rats naïve to water (C2; 21 rats, n = 39). These charts show that changes in the heart rate (HR) and mean ABP (MABP) were highly significant after voluntary submersion (B1), and HR after involuntary submersion of trained rats (C1) or untrained naïve rats (C2). Compare the bradycardic responses to underwater submersion in these charts and note the wider spread in naïve rats than those familiar to water, suggesting more stress in this group as well as potential suprabulbar modulation. ***P < 0.001; paired samples T-test for C1–C3. A–E are reprinted from J. Appl. Physiol., 108, Panneton et al., The rat: a laboratory model for studies of the diving response, 811–820 (2010), with permission. See Panneton et al. (2010b) for discussion on these different preparations.
It is known that the apnea (breath-hold) is maintained during the DR despite gross alterations of blood gases, which reach levels that would normally drive respiration. Thus, it has been suggested that respiratory chemoreceptors, which normally induce vigorous ventilation when activated, are inhibited (Elsner et al., 1977; de Burgh Daly, 1984; McCulloch and West, 1992; McCulloch et al., 1997); this is indeed the case (Panneton et al., 2010a). Second, there is a dramatic bradycardia mediated by the vagus nerve of the parasympathetic NS, which reduces cardiac output. Third, there is vasoconstriction in the vascular beds to non-essential organs (i.e., muscle, abdominal organs, skin) while the two most essential organs, the heart and the brain, remain perfused. The vasoconstriction seen with diving is mediated via the sympathetic NS (Yavari et al., 1996; McCulloch et al., 1999b), maintaining internal oxygen stores for the brain and the heart (Ollenberger and West, 1998b).
Experimental Procedures
Discussions of techniques employed will not be emphasized in this review. However, interested readers are referred to manuscripts with numerous references detailing the location of the anterior ethmoidal nerve (AEN; Panneton, 1991a) and its recording/stimulation (McCulloch et al., 1999a) stimulating the nasal mucosa with vapors (Panneton, 1991b; Panneton and Yavari, 1995; Yavari et al., 1996; Panneton et al., 2010b), for transganglionic transport techniques from primary afferent fibers (Panneton, 1991a; Panneton et al., 2006; Panneton and Gan, 2014), for pharmacological blockade of central pathways (Panneton and Yavari, 1995; McCulloch et al., 1999b), for neuroanatomical tract-tracing of central pathways (Panneton et al., 2000, 2006), for the use of cFos as a neuroanatomical marker of function (Panneton et al., 2010a, 2012a), for the training of rats and mice to dive (McCulloch, 2014; Panneton et al., 2014; Hult et al., 2019), and for deployment of telemetric transmitters to measure cardiovascular changes in both trained rats (Panneton et al., 2010a, b, 2012a) and mice (Hult et al., 2019). The limitations of these techniques are discussed in these manuscripts. Also, nomenclature of the brainstem, particularly the reticular formation, and the terms used by us in this review, have been defined previously (Sun and Panneton, 2005; Panneton et al., 2006).
The Diving Response as a Reflex
A reflex is “an involuntary reaction in response to a stimulus applied to the periphery and transmitted to the nervous centers in the brain or spinal cord,” by definition. Most data suggest that the DR consists of three independent reflexes regulating respiration (an apneic reflex), heart rate (a bradycardic reflex), and arterial blood pressure (ABP; a vasoconstrictor reflex), respectively, but splenic contraction has also been documented in numerous species. Pharmacologic studies using peripheral application of antagonists/agonists show that heart rate and blood pressure responses can be blocked selectively while preserving the other two reflexes (Yavari et al., 1996; Elliott et al., 2002). However, peripheral autonomic fibers apparently mediate neither dive time nor surface intervals in seals after receptor blockade (Elliott et al., 2002). Moreover, similar blocking studies show that bradycardia to underwater submersion is cholinergically mediated, while sympathetic innervation is far less important (Elliott et al., 2002). The responses after stimulating the nasal mucosa with noxious vapors are similar to those of underwater submersion (Angell James and de Burgh Daly, 1969, 1972, 1973; White et al., 1974; Peterson et al., 1983; Panneton, 1990; Nakamura and Hayashida, 1992; Gieroba et al., 1994; Panneton and Yavari, 1995; Yavari et al., 1996; Kobayashi et al., 1999; Ho and Kou, 2000; Dutschmann and Paton, 2002), and both behaviors are considered reflexes by us. The circuitry for the DR is intrinsic to the medulla and the spinal cord (Panneton et al., 2012b), since the responses remain to nasal stimulation despite brainstem transection at the pontomedullary junction (Figure 2), sparing only the ventral third of the trigeminal sensory complex (Panneton et al., 2012b) and promoting its definition as a reflex. Thus, our conclusion differs from that of others (Dutschmann and Herbert, 1996, 1997, 1998a, 1999; Chamberlin and Saper, 1998; Dutschmann et al., 1998, 2004; Radulovacki et al., 2003, 2004; Chamberlin, 2004; Topchiy et al., 2009) who suggest that autonomic changes induced by trigeminal stimulation are dependent on neurons in the pons, including the parabrachial-Kölliker-Fuse nuclei and the intertrigeminal region. Maintaining a DR following pontomedullary transection implies that this life-saving response may be organized in redundant circuits, and failure or blockade of the pontine loops are compensated by medullary circuits. This is likely to happen with failure of critical NMDA receptors in the pons during progression of uncompensated hypoxia (e.g., during drowning). Since the pons receives significant ascending inputs from the trigeminal sensory relays (Panneton et al., 1994, 2006), it is reasonable to assume that the pons is an integral part of the neural circuit that mediates the DR under intact conditions. However, since all descending/ascending fibers from/to suprabulbar structures were cut yet the response was maintained, we find that these suprabulbar areas modulate a basic medullary reflex circuitry.
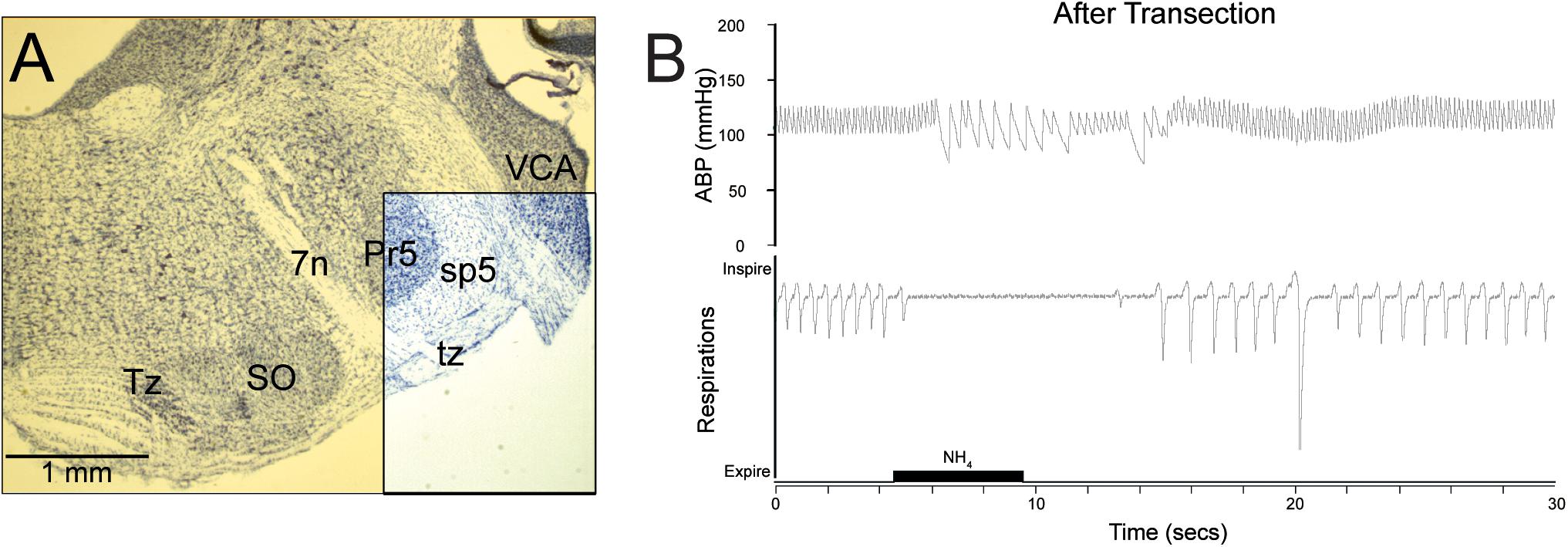
Figure 2. The reflex circuit driving the diving response is contained within the medulla and spinal cord. Transections through the pontomedullary junction (A, yellow transparency) were made, sparing only neuropil in the ventral part of the spinal trigeminal complex (A, boxed area), including primary afferent fibers of the AEN descending in the spinal trigeminal tract (sp5). Despite this trauma, cardiorespiratory depression similar to that seen in underwater submersion was maintained after nasal stimulation (B), promoting the idea that the neural circuits driving the DR are situated in the medulla, and thus are similar to other brainstem reflex circuits. Figures are reprinted from Respir. Physiol. Neurobiol., 180, Panneton et al., Persistence of the nasotrigeminal reflex after pontomedullary transection, 230–236 (2012), with permission. See Panneton et al. (2012b) for more details.
The Stimulus
The independent reflexes comprising the DR act harmoniously toward preserving vital oxygen stores and are initiated by activating peripheral receptors. Early studies (Koppányi et al., 1929; Irving, 1939; Irving et al., 1942; Tchobroutsky et al., 1969; Dykes, 1974; Lin, 1974; Whishaw and Schallert, 1977; Gandevia et al., 1978; Drummond and Jones, 1979; Schagatay and Van Kampen, 1995) noted that submersion or wetting of nasal areas was important to induce the DR, and this has been confirmed by others numerous times. Thus, underwater submergence is the usual stimulus to induce the DR in awake animals. This spurred many investigators to perform “forced” submersions, where the animals were tethered on boards or placed in cages and dunked underwater (Koppányi et al., 1929; Scholander, 1963; Dykes, 1974; Lin, 1974; Lin and Baker, 1975; Martner et al., 1977; Drummond and Jones, 1979; Jones et al., 1982; Schagatay and Van Kampen, 1995; Panneton et al., 2010a, b). However, forcing the animals underwater usually hinders the formidable interventions necessary to monitor respiration, arterial pressure, and heart rate, as well as access to structures in the brain.
Water flowing over the nasal mucosa has been used as a stimulus (Angell James and de Burgh Daly, 1972; Gandevia et al., 1978; Drummond and Jones, 1979; Doyle et al., 1988), but high flow rates often strip mucosa from nasal bones and create blood clots. Irritating vapors (smoke, ammonia, formaldehyde) wafted over the nasal mucosa prevents gross mucosal disruption and offers better control (time and intensity) of the stimulus to induce autonomic responses similar to diving (McRitchie and White, 1974; White et al., 1974, 1975; Drummond and Jones, 1979; Doyle et al., 1988; Lee et al., 1990; Panneton, 1990, 1991b; Nakamura and Hayashida, 1992; Gieroba et al., 1994; Panneton and Yavari, 1995; Yavari et al., 1996; McCulloch and Panneton, 1997; McCulloch et al., 1999b; Ho and Kou, 2000; Ho and Kou, 2002). Finally, the electrical stimulation of the AEN also induces an apnea, bradycardia, and sympathoactivation typical of the DR (Dutschmann and Herbert, 1996, 1997, 1998a,b; McCulloch et al., 1999a; Dutschmann and Paton, 2002), but with the caveat that electrical stimulation potentially activates a wide variety of fibers, including those nociceptive and others important for sneezing, and as such may muddle interpretations of those investigating the DR.
Nasal and Paranasal Receptive Fields
Since the mammalian DR can be induced with only snout immersion, this suggests that primary afferent fibers innervating nasal and paranasal areas may be important. Indeed, covering paranasal areas with petroleum jelly or numbing these areas with anesthetic eliminates the autonomic responses induced by submersion (Dykes, 1974; Drummond and Jones, 1979). Paranasal areas are innervated by branches of the maxillary branch of the trigeminal including its large infraorbital nerve, which innervates the ala of the nose and the upper lip, as well as the AEN of the ophthalmic division of the trigeminal, which innervates mucosa of the lateral and septal nasal walls in humans, the skin of the ala, and the vestibule and apex of the nose (Williams and Warwick, 1980; Wallois et al., 1991). The nasal mucosa has both respiratory and olfactory segments (Ross et al., 1995), but the olfactory epithelium is not considered important for the DR, since the DR remains after olfactory bulb ablation (Angell James and de Burgh Daly, 1972; McRitchie and White, 1974; Drummond and Jones, 1979; Panneton, 1990; Gieroba et al., 1994; Kratschmer, 2001). Indeed, cetaceans with their blowholes have neither olfactory bulbs nor an olfactory system, while baleen whales retain only small rudimentary olfactory organs, despite their diving prowess.
Innervation of the nasal mucosa is via free nerve endings from small diameter fibers (Cauna et al., 1969), most of which are C-fibers and contain peptides, notably calcitonin gene-related peptide (CGRP) and substance P (Petersson et al., 1989; Silverman and Kruger, 1989; Stjärne et al., 1989; Finger et al., 1990; Silver et al., 1991; Spit et al., 1993; Matsuda et al., 1994, 1998), derived from trigeminal ganglion neurons (Silverman and Kruger, 1989; Ichikawa et al., 1993; Matsuda et al., 1994; Schaefer et al., 2002). Most of these fibers are sensory in function, and many respond as chemoreceptors, creating the “common chemical sense,” or chemethesis (Cain and Murphy, 1980; Green and Lawless, 1991; Viana, 2011; Green, 2012) since sensations, including pain, can be elicited from stimulating the human nasal mucosa (Handwerker and Kobal, 1993; Thürauf et al., 1993; Cometto-Muñiz and Cain, 1997; Cometto-Muniz et al., 1998, 2001; Hummel et al., 2003). While inhaled irritants may stimulate these small free nerve endings directly, “solitary chemoreceptive cells” (SCCs) (Finger et al., 2003; Tizzano and Finger, 2013) within the mucosa of the upper respiratory tract of amniotes, including the nasal mucosa of humans (Barham et al., 2013), may also serve as intermediaries in a nociceptive or chemosensor pathway. SCCs in the nasal mucosa are found mostly anteriorly and innervated by small polymodal nociceptors of the trigeminal nerve (Finger et al., 2003; Tizzano et al., 2010). When these SCCs are activated they induce respiratory reflexes including apnea (Tizzano et al., 2010; Tizzano and Finger, 2013); their peripheral distribution greatly overlaps that of AEN innervation.
The Sensory Nerve
We consider the AEN as the “gatekeeper” nerve since it is the first to sense noxious gases or water entering the nasal passages. Stimulating the peripheral receptors of the AEN would prevent such toxins from entering the upper respiratory passages by inducing an apnea. While there are several reports in the literature documenting nerves innervating the blowholes of cetaceans, only motor fibers from the facial nerve are described; the sensory nerves from the trigeminal were rarely, if ever, considered. We suspect, however, that a nerve analogous to the AEN exists in marine mammals, and this nerve also functions as a gatekeeper of their respiratory system. More research may prove this to be the case.
The AEN of terrestrial animals contains both mechanoreceptors and chemoreceptors (Silver et al., 1986; Lucier and Egizii, 1989; Wallois et al., 1991; Sekizawa and Tsubone, 1994, 1996; Sekizawa et al., 1996, 1998; McKeegan et al., 2002) responsive to a variety of stimuli. Most of its fibers are small diameter in the Aγ or C range (Beidenbach et al., 1975; McCulloch et al., 1999a) and reach between the mucosal epithelial cells toward tight junctions (Cauna et al., 1969; Finger et al., 1990; Spit et al., 1993). The central fibers of the AEN descend in the ventral third of the spinal trigeminal tract (Panneton, 1991a; Panneton et al., 2006) and send fibers into the trigeminal sensory complex and lateral reticular formation. Moreover, CGRP in the lateral reticular formation, a peptide contained in numerous fibers of the AEN, is lost after unilateral trigeminal rhizotomy (Panneton and Gan, 2014), suggesting a direct route for primary afferent fibers to modulate cardiac and vascular activity during underwater submersion. The infraorbital nerve is very large in rodents and has numerous fibers responsive to multiple stimuli. It also sends central fibers in the spinal trigeminal tract and all trigeminal sensory nuclei (Panneton et al., 2010c, 2017), but its trigeminal distribution is much wider than that of the AEN.
Nevertheless, acute transection of the AEN attenuates the apnea and ABP changes, and greatly attenuates the bradycardia to nasal stimulation (Rybka and McCulloch, 2006), but such transection does not impair the induction of the DR in voluntary diving rats or nasally stimulated rats when allowed to survive for several days after transection (Chotiyanonta et al., 2013; McCulloch et al., 2016; McCulloch and DiNovo, 2018). Interestingly, the transection of the AEN never attenuated the rise in arterial pressure induced by the nasopharyngeal stimulation, possibly since posterior parts of the nasal mucosa are innervated by other nerves.
The AEN innervates only the nares partially, as well as anterior parts of the nasal mucosa, thus it is the first sensor to assess incoming air and earns its moniker as gatekeeper. However, the posterior aspects of the nasal mucosa receive several nerves branching from the maxillary division of the trigeminal, and these still were intact in these preparations. These small nerves to the posterior mucosa indeed effect cardiorespiratory reflexes induced by stimulation of the nasal mucosa (Kanamaru et al., 1999, 2001; Mutoh et al., 2000, 2001). The central termination of fibers of some posterior nasal nerves has been demonstrated (McCulloch et al., 2018), and they maintain the somatotopy dictated for the medullary dorsal horn (MDH; Panneton et al., 2017). Other paranasal nerves/areas also align to somatotopy in the MDH (McCulloch et al., 2018); all converge on central terminal fields related to the nose. It is of interest that tracers transported transganglionically after large injections into the infraorbital nerve (Panneton et al., 2010c) labeled the misplaced substantia gelatinosa just dorsal to that labeled from the AEN (Panneton, 2013; Panneton et al., 2017), again conforming to appropriate somatotopy.
These discussions on the innervation of the nasal mucosa must be considered moot, however, since water does not flow through the nose of voluntarily diving rats or mice, and certainly not in marine species without nasal cavities, but may be more important to consider in rats nasally stimulated with obnoxious vapors or water. Chotiyanonta et al. (2013); McCulloch et al. (2016), and McCulloch and DiNovo (2018) provide lengthy discussions on the retention of the DRs after the AEN section, speculating that sprouting of retained central fibers reinnervate denervated areas of the MDH. Another possibility is that growth of nearby non-lesioned peripheral fibers from nerves innervating areas of the nares may compensate for the loss of AEN fibers. Nevertheless, McCulloch et al. (2018) show overlap of central projections from areas surrounding the nares. If a diving rat does not flow water over its nasal mucosa during underwater submersion to induce the physiological manifestations we call the mammalian DR, perhaps the innervation of the initial portal to the upper respiratory tract, the nares, is most important. This is supported by those (Dykes, 1974; Drummond and Jones, 1979) who initially injected local anesthetic in paranasal areas and inhibited the DR.
The First Synapse
The sensory stimulus is linked to motor output via a reflex arc, “a route followed by nerve impulses in the production of a reflex act, from the peripheral receptor organ through the afferent nerve to the central NS synapse and then through the efferent nerve to the effector organ.” Peripheral physiologists know the stimulus (underwater submersion) as well as the output (e.g., an apnea via central inhibition of respiration, bradycardia via the vagus nerve, peripheral vasoconstriction via the sympathetic NS), but most elect not to explore central integration.
The trigeminal sensory complex is the principal relay for somatosensory afferent fibers innervating structures in the head (Beckstead and Norgren, 1979; Marfurt, 1981; Panneton and Burton, 1981; Matesz, 1983; Shigenaga et al., 1986; Marfurt and Rajchert, 1991; Panneton et al., 2017). The central projections of the AEN have been studied with transganglionic transport techniques in the cat (Lucier and Egizii, 1986), muskrat (Panneton, 1991a), guinea pig (Segade, 2003), and rat (Panneton et al., 2006; Hollandsworth et al., 2009), or after mucosal injections in the rat (Anton and Peppel, 1991; McCulloch et al., 2018). Most of these studies (Lucier and Egizii, 1986; Anton and Peppel, 1991; Panneton, 1991a; Panneton et al., 2006; McCulloch et al., 2018) show dense reaction product in superficial laminae of the subnucleus caudalis of the spinal trigeminal nucleus (currently called the MDH) (Figures 3A,B), while projections to more rostral parts of the trigeminal sensory complex also were shown in some reports (Panneton, 1991a; Panneton et al., 2006). Panneton et al. (2000) further showed transganglionic transport of herpes simplex virus (HSV-1, strain 29) from the AEN to similar areas of the trigeminal sensory complex (Figures 3A1,B1) as well as transneuronal projections to brainstem autonomic nuclei in the muskrat (Figures 5A4,B4,C4). The central projections of the infraorbital nerve partially overlap those of the AEN in the rostral MDH (Panneton et al., 2010c) and must be important since the DR in awake behaving rats is maintained despite cutting the AEN bilaterally (Chotiyanonta et al., 2013; McCulloch et al., 2016; McCulloch and DiNovo, 2018).
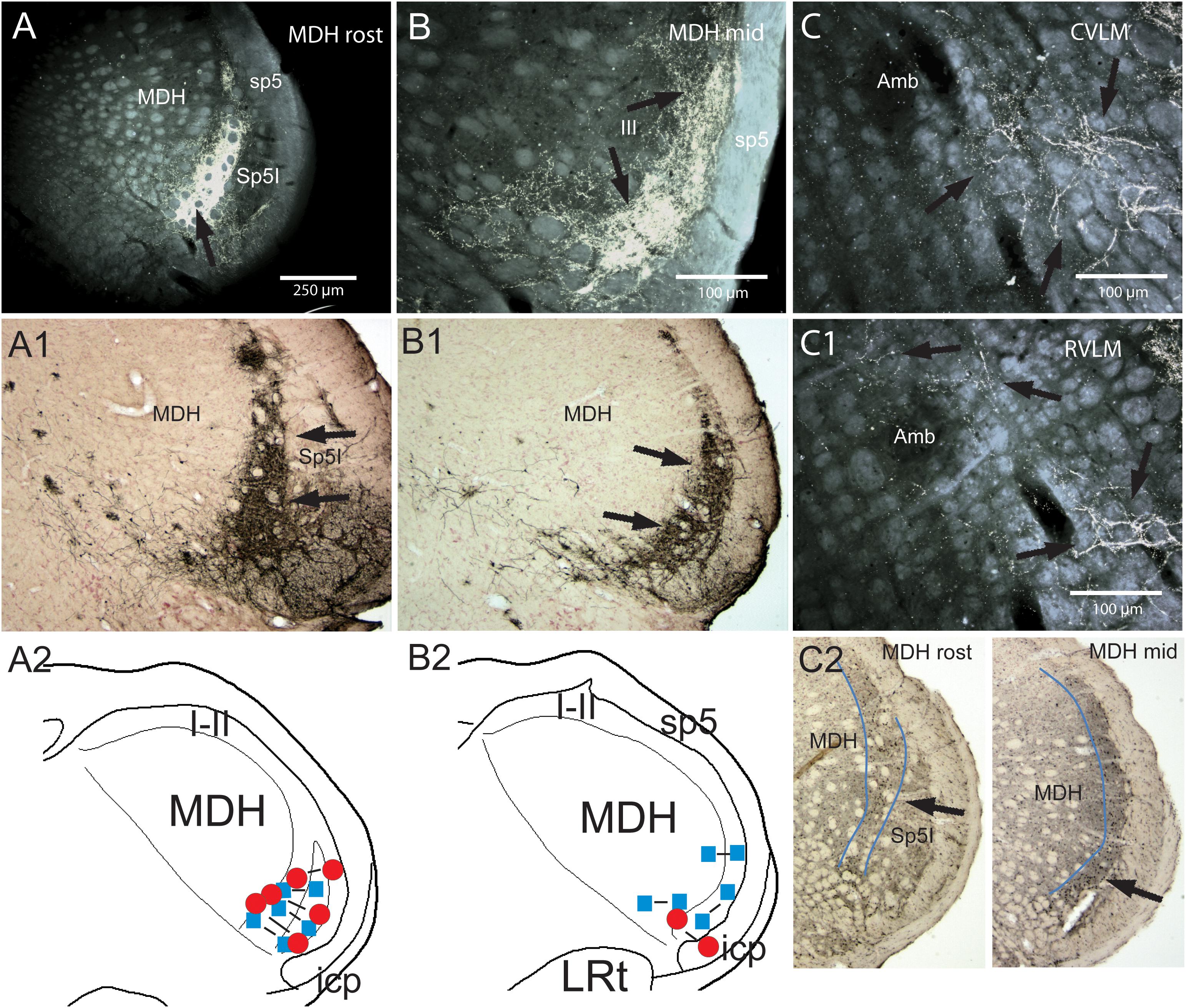
Figure 3. Support for the role of the anterior ethmoidal nerve (AEN) and the medullary dorsal horn of the trigeminal sensory complex as relays for the diving circuit. Figures showing some medullary projections of the AEN, locales where injections into the MDH disrupted the cardiorespiratory responses after nasal stimulation, and distribution of cFos-labeled neurons in the MDH after involuntary submersion. Dense projections are shown in darkfield photomicrographs at two levels of the rostral MDH in its substantia gelatinosa (laminae I and II) after transganglionic transport of an HRP cocktail after its application to the AEN in a rat (A,B; arrows; labeled axons/terminals appear bright white), or HSV-1 virus in the muskrat (A1,B1; arrows; immunoprecipitate appears dark). Note the similarity of data in the two species using different techniques. The cardiorespiratory responses to stimulating the nasal mucosa (see Figure 1) were blocked by small bilateral injections of either lidocaine (blue squares) or kyurenate (red circles) made into similar areas of the muskrat (A2,B2). The role of the MDH as a relay for the diving response was also supported by cFos in similar areas of the rat after underwater submersion (C2, arrows). Extratrigeminal projections of the AEN also were noted in the lateral reticular formation at levels of the CVLM (C, arrows) and RVLM (C1, arrows). Both areas are important for modulating cardiovascular activity and these projections suggest direct somato-autonomic connectivity. Location of the bilateral injections of blocking solutions is coupled in (A,B). Abbreviations: Amb, ambiguus nucleus; CVLM, depressor area of caudal medulla; LRt, lateral reticular nucleus; MDH, medullary dorsal horn; Sp5I, nucleus of the spinal tract of the trigeminal nerve, interpolar part; RVLM, pressor area of rostral medulla; icp, inferior cerebellar peduncle; sp5, spinal tract of the trigeminal nerve. Figure is compiled from others in Neuroscience, 141, Panneton et al., Brainstem projections from recipient zones of the anterior ethmoidal nerve in the medullary dorsal horn, 889–906 (2006); Physiology 28, Panneton, The mammalian diving response: an enigmatic reflex to preserve life?, 284–297 (2013), and Br. Res. 874, Panneton et al., Trigemino-autonomic connections in the muskrat: the neural substrate for the diving response, 48–65 (2000), with permission. See text for details.
The MDH is an important relay in autonomic reflexes such as the DR (Panneton, 1991b; Panneton and Yavari, 1995; Yavari et al., 1996), trigeminal depressor response (Kumada et al., 1975, 1977; Terui et al., 1981; Yu and Blessing, 1998), oculocardiac reflex (Gandevia et al., 1978), and adrenal cortical function (Bereiter and Gann, 1988a, b, 1989; Bereiter et al., 1990; Bereiter and Benetti, 1991; Lu and Bereiter, 1991; Bereiter, 1993). Indeed, underwater submersion activates numerous neurons immunolabeled with cFos in the MDH (McCulloch, 2005; Panneton et al., 2010a, 2012a; McCulloch et al., 2016) in locations similar to the termination of primary afferent fibers contained within the AEN (Figure 3C2). Moreover, Panneton (1991b) and Panneton and Yavari (1995) showed that small injections into similar areas (Figures 3A2,B2) of either lidocaine or kynurenate, both of which block synaptic transmission, selectively inhibited the cardiorespiratory sequelae to nasal stimulation. It should be noted, however, that the AEN also has extratrigeminal reticular projections (Figures 3C,C1) which are probably important for the cardiovascular responses in diving.
Respiration
All mammals submerged underwater, either voluntarily or involuntarily, became apneic and remain apneic, despite submersions exceeding their aerobic dive limit (Panneton et al., 2010a). However, such prolonged apneas (or breath-holds) are not maintained with long nasal stimulations in anesthetized muskrats. Nevertheless, most marine mammals work within their aerobic dive limit (Kooyman, 1985; Burns and Castellini, 1996; Ponganis et al., 1997), a metabolic threshold where diving duration goes beyond intrinsic oxygen stores and is marked by blood lactate concentration increasing above resting levels (Kooyman et al., 1980). An important question is how the prolonged apneas are maintained despite gross alterations in blood chemistry (Figures 4B–G) that normally increase ventilation. The neuronal circuitry driving respiration is complexly organized and its efficiency in fulfilling physiological needs is not fully understood (Feldman et al., 2013). Nevertheless, a reflex apnea is induced with either underwater submersion or nasal stimulation despite truncating the brain at the pontomedullary junction (Figure 2B). While it seems reasonable to believe influences over reflex behavior are manifested by many suprabulbar neurons, including those important in apneic reflexes and breath-holds (Dutschmann and Herbert, 1996, 1997, 1998b, 1999; Chamberlin and Saper, 1998; Radulovacki et al., 2003, 2004; Topchiy et al., 2009), it is likely that they are modulatory rather an intrinsic part of the diving reflex circuit. This is especially important to consider when studying the DR in species high in neural hierarchies, e.g., marine mammals and humans, who have considerable volitional control over respiration.
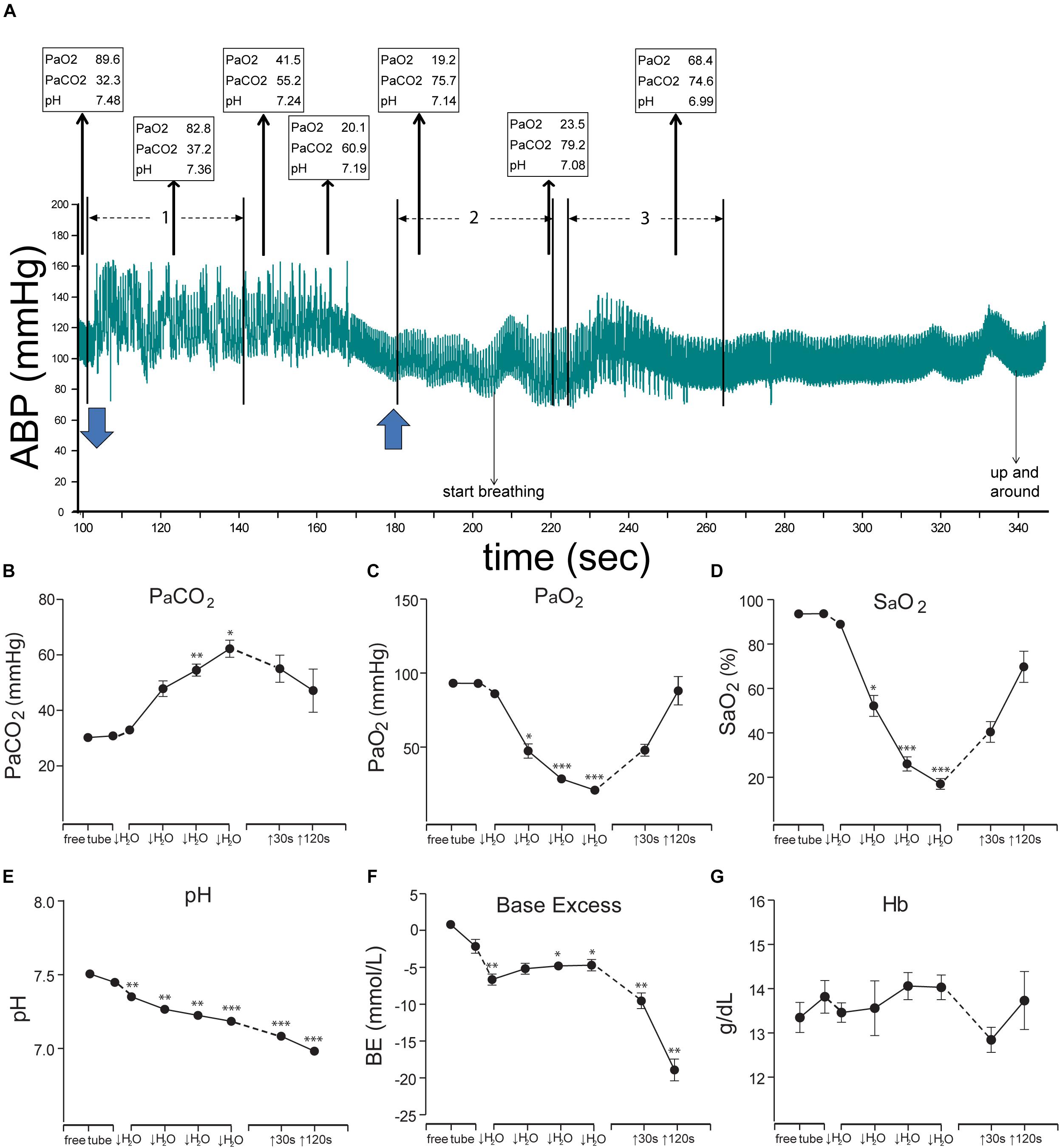
Figure 4. Figures illustrating the cardiovascular responses and the resultant blood chemistry to forced submersions of rats. A bradycardia and increase in ABP was seen approximately for the first minute of submersion (A); numerous ectopic beats are evident with increased pulse pressure (see expanded view in Figure 11). Blood chemistry changed radically during the period of submersion (B–G), but the rats remained apneic despite such changes. Thin arrows oriented upward in A show (in boxes) the PaO2, PaCO2, and pH of blood withdrawn over time from the submerged rat, while blue arrows at the bottom indicate time underwater. Note the extreme hypercapnia, hypoxia, and acidosis during the apnea. All the chemical indicators in the blood however suggested the rats should breathe vigorously, but the rats did not, nor did they drown. We speculate the apnea is refractory to gross changes in blood gases and is prolonged during diving, perhaps due to the activation of putative chemoreceptors on the ventral medullary surface (see Figures 6, 7C). These studies prove the homeostatic respiratory chemoreceptor reflex is inhibited during underwater submersion. ∗P < 0.05, ∗∗P < 0.01, ∗∗∗P < 0.001. Figures are reprinted from J. Appl. Physiol., 109, Panneton et al., Cardiorespiratory and neural consequences of rats brought past their aerobic dive limit, 1256–1269 (2010), with permission. See Panneton et al. (2010a), for more details.
Indeed, rhythmic depolarizations similar to respiration persists in many slice or brainstem-spinal preparations of only the medulla (Bianchi et al., 1995; Rekling and Feldman, 1998; Feldman et al., 2013), and much information has been garnered from such preparations. The ventral respiratory column (Feldman, 1986; Benarroch, 2007) holds many respiratory neurons and one part of it, the pre-Bötzinger complex, is where many neurons generating respiratory rhythm lie (Bianchi et al., 1995; Rekling and Feldman, 1998; Feldman et al., 2013). Dutschmann and Paton (2002) showed that inspiratory neurons ceased firing and were hyperpolarized while post-inspiratory neurons depolarized and discharged persistently after electrical stimulation of the AEN in a working heart-brainstem preparation. This novel preparation may be advantageous for future investigations of the apnea related to diving, since the “preparation” is unanesthetized but has intact and functioning cardiac and respiratory systems. Reflecting on the caveats associated with unnatural electrical stimulation of nerves, however, perhaps it would be worthwhile to determine if these preparations could also induce a DR with an immersion of the snout in water.
Neuroanatomical projections from the MDH (Panneton et al., 2000, 2006) are relatively dense to caudal parts of the ventral respiratory column where expiratory neurons dominate (Figure 5A1). Projections from the MDH also are seen near the pre-Bötzinger complex (Figures 5B1–B4). However, there are very few neurons labeled with cFos in the ventral respiratory column (Figures 5B5,C5, 6), considered the area just ventral to the nucleus ambiguus, after underwater submersion (Panneton et al., 2010a, 2012a). This is perhaps due to the apnea induced with underwater submergence—inhibited neurons rarely, if ever, show Fos label because their inhibition precludes activation.
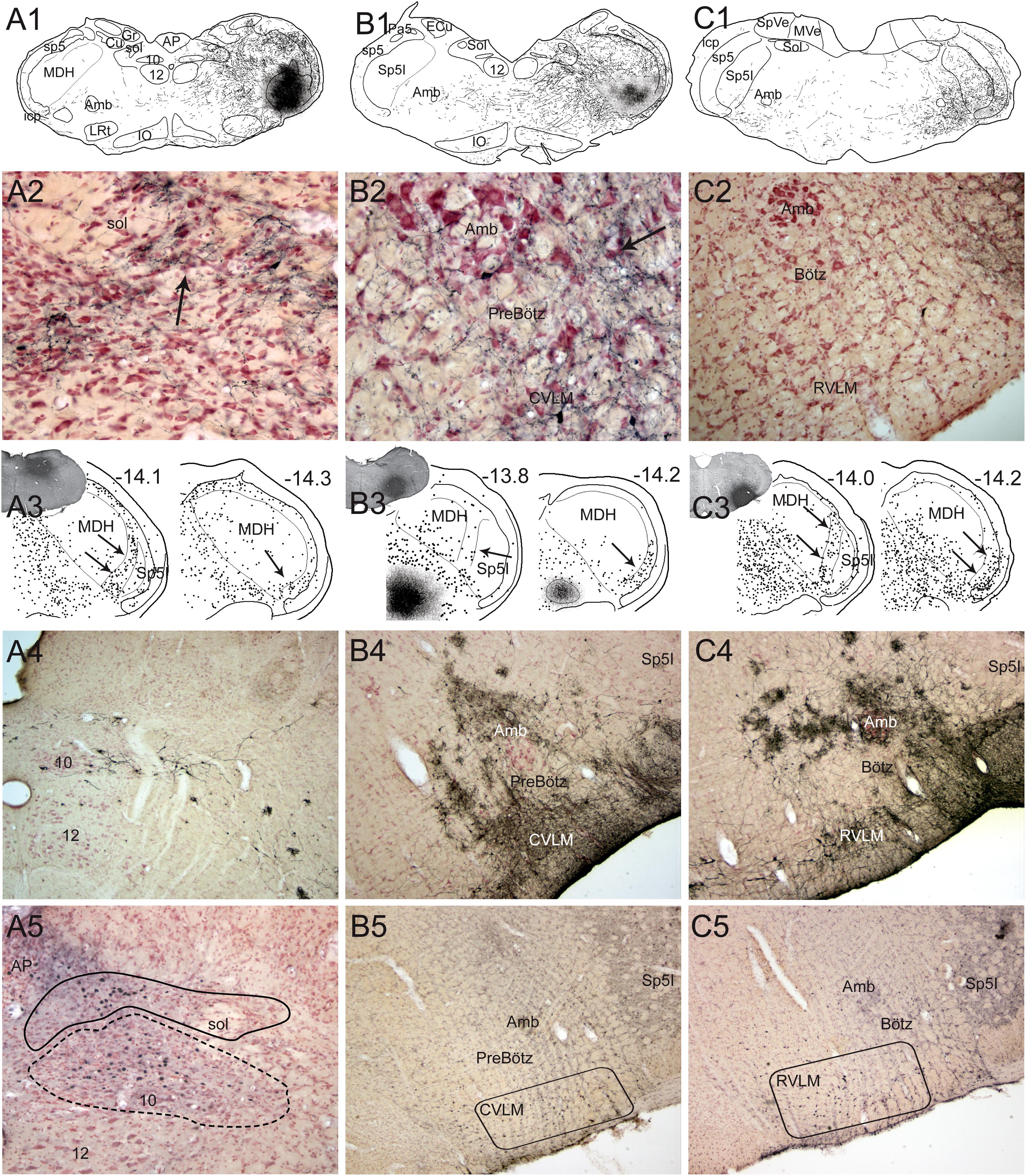
Figure 5. Neuroanatomical data implicating brainstem loci important for the diving response. Figures from rats (1–3 in A–C) and muskrats (4 in A–C) illustrating potential brainstem circuits driving the diving response. Injections of BDA were placed in the MDH (A1) where primary afferent fibers in the AEN project, where transganglionic transport of HSV-1 virus was found, where the DR could be reversibly inhibited, and where cFos labeled neurons were found (see Figure 3). Labeled fibers packed into the ventrolateral subnucleus of the nucleus tractus solitarius (Sol; A2, arrow) and extended toward the lateral part of the dorsal motor nucleus of the vagus. This projection was confirmed after injection of fluorogold into the Sol (A3, insert); note numerous retrogradely labeled neurons (dots) in the substantia gelatinosa of the MDH rostrally (-14.1; arrows) and more caudally (-14.3; arrow) mimicking the location of data seen in Figure 3. Terminal-like label was also seen in sections through the preBötzinger (PreBötz) area and CVLM (B1,B2) after MDH injections of BDA (A1) and confirmed by retrograde cases (B3). Sections through the more rostral Bötzinger (Bötz) complex and RVLM had very few large fibers labeled with BDA but numerous small fibers (C2). The origin of the projections was confirmed with retrograde analysis (C3, arrows) showing numerous neurons in the part of the MDH known to be important for diving behavior. cFos studies, considered functional neuroanatomy, suggest the reticular areas also are activated during underwater submergence (A5–C5; darkened nuclei represent activated neurons). Other neuroanatomical data from transneuronal transport of HSV-1 virus (A4–C4) injected into the AEN, suggest these areas are linked to the AEN as well as underwater submersion (see Panneton et al., 2000 for details). However, mismatches of label between functional and tract-tracing approaches in the subnuclei of Sol suggest the fibers seen with tract-tracing techniques have functions other than diving behavior. Also, there were few neurons labeled with Fos in the ventral respiratory column, perhaps since respiration is inhibited (the apnea) and Fos only labels activated cells (see text for discussion). Abbreviations: AP, area postrema; Cu, cuneate nucleus; Gr, gracile nucleus; IO, inferior olive nucleus; MVe, medial vestibular nucleus; Sol, nucleus tractus solitarii; SpVe, spinal vestibular nucleus; py, pyramidal tract; sol, solitary tract; 10, dorsal nucleus of the vagus nerve; 12, hypoglossal nucleus; 12n, hypoglossal nerve. See Figure 3 for other abbreviations. Figure is compiled from others in Neuroscience, 141, Panneton et al., Brainstem projections from recipient zones of the anterior ethmoidal nerve in the medullary dorsal horn, 889–906 (2006); and Br. Res. 874, Panneton et al., Trigemino-autonomic connections in the muskrat: the neural substrate for the diving response, 48–65 (2000), with permission.
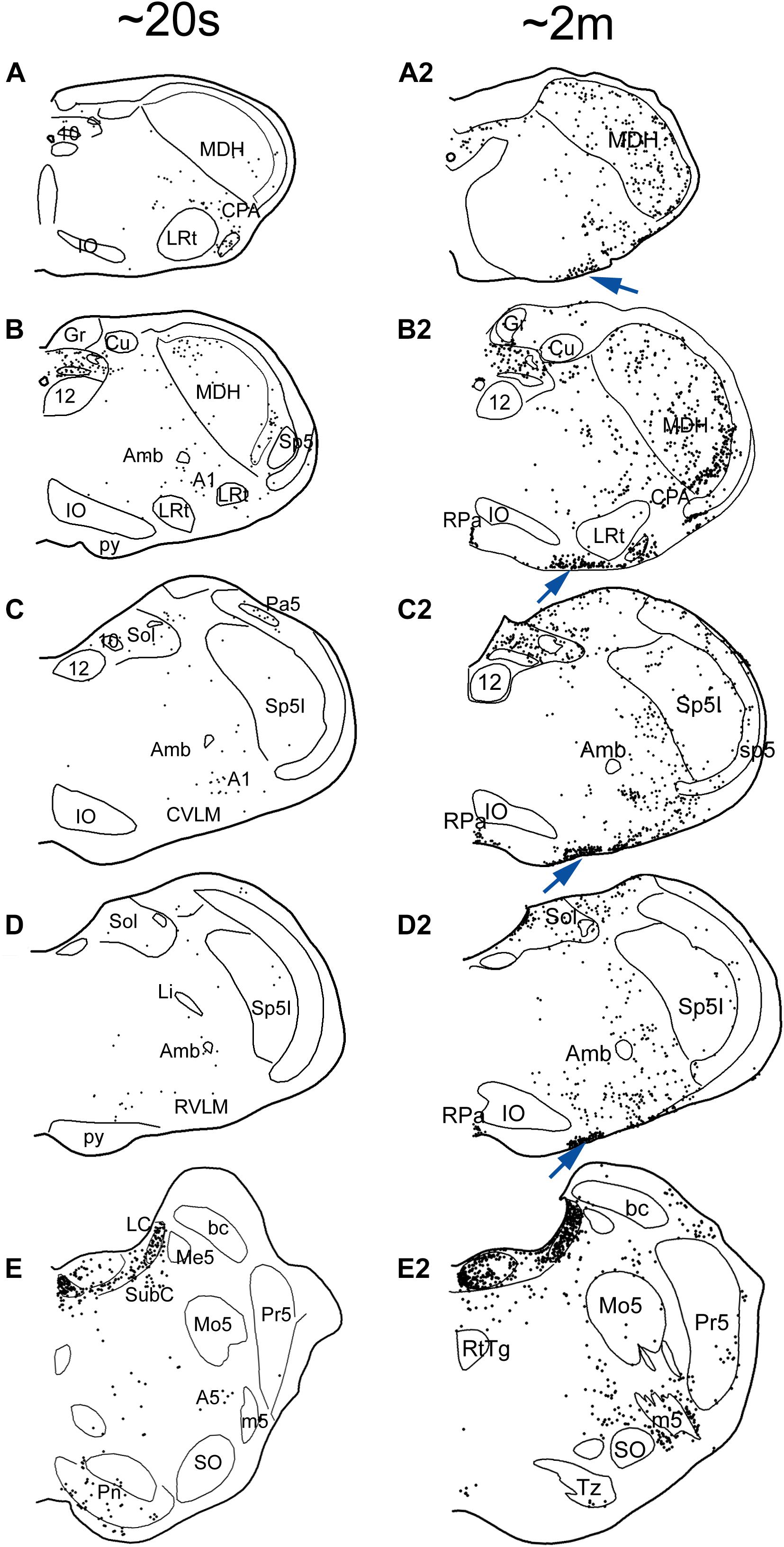
Figure 6. Line drawings comparing the distribution of cFos immunolabeling in the brainstem of rats after a single trial of voluntary submersion (A–E; see Panneton et al., 2012a) to that of a single prolonged submergence of a rat brought beyond its aerobic dive limit (A2–E2; see Panneton et al., 2010a). The density of immunolabeled cells in the MDH, CVLM, RVLM, and NTS after a single brief immersion (left column) is greatly increased after the more prolonged submersion (right column). Note, however, the emergence of myriad immunolabeled cells along the ventral surface of the medulla (arrows), most in the epi-pia, only after the prolonged submergence. These putative chemoreceptors could potentiate and prolong the apnea of diving via interaction with somatostatin neurons (Figure 7). See previous figures for abbreviations. Figures are reprinted from J. Appl. Physiol., 109, Panneton et al., Cardiorespiratory and neural consequences of rats brought past their aerobic dive limit, 1256–1269 (2010), with permission.
The pre-Bötzinger complex contains several types of neurons, including those marked by somatostatin (Stornetta et al., 2003; Tan et al., 2008; Cui et al., 2016), a peptide, which acts as an inhibitory respiratory modulator (Ramirez-Jarquin et al., 2012). Acute silencing of such somatostatin neurons results in persistent apnea (Tan et al., 2008) in awake mice. Moreover, somatostatin infusion in humans greatly reduced the acute hypoxia ventilatory response, as well as the acute hypercapnic ventilatory response (Pandit et al., 2014), thus blunting the respiratory chemoreceptor response. Indeed, somatostatin’s inhibitory effect on respiration was potentiated in vitro when the pH of brainstem’s bath was lowered from 7.4 to 7.3 (Llona et al., 2004). The pH of blood dropped from ∼7.5 to ∼7.2 during involuntary submergence of rats (see Figure 4E) and continued to drop after they emerged from the water. Thus, somatostatin neurons in the pre-Bötzinger complex may be important for the apnea induced in the DR.
Somatostatin neurons in the pre-Bötzinger complex (Figure 7A) have numerous processes which extend ventrally into the epi-pia on the ventral surface of the medulla. Our data provide two potential routes where such neurons in the preBötzinger complex may be modulated during the DR. The first is via direct projections from neurons in the ventral MDH that receives nasal afferent fibers (Figure 3) to the area of medium-sized neurons where somatostatin neurons lie (Figures 5B2,B4, 7B). [It is of interest that a cluster of neuron in a similar place were activated and immunolabeled for cFos after a prolonged submersion (Figure 6C2), but we unfortunately did not double label these neurons for somatostatin.] A second potential route is via projections from similar injections to the ventral surface of the caudal medulla (Figure 7D), where numerous Fos labeled cells are documented (Figure 7C) after involuntary submersion. These putative respiratory chemoreceptors are linked by gap junctions (Solomon et al., 2001; Dean et al., 2002) and may provide a fast link to somatostatin neurons of the brainstem respiratory network and modulate the apnea induced by underwater submergence. However, the function of neither of these projections is known, highlighting the technical limitations of neuroanatomical techniques. Fos immunohistochemistry fails to label inhibited neurons, while tract-tracing studies offer no insight into functional status. More precise experiments are needed, perhaps with genetically altered mice or working heart-brainstem preparations, to determine the genesis of the apnea in the mammalian DR.
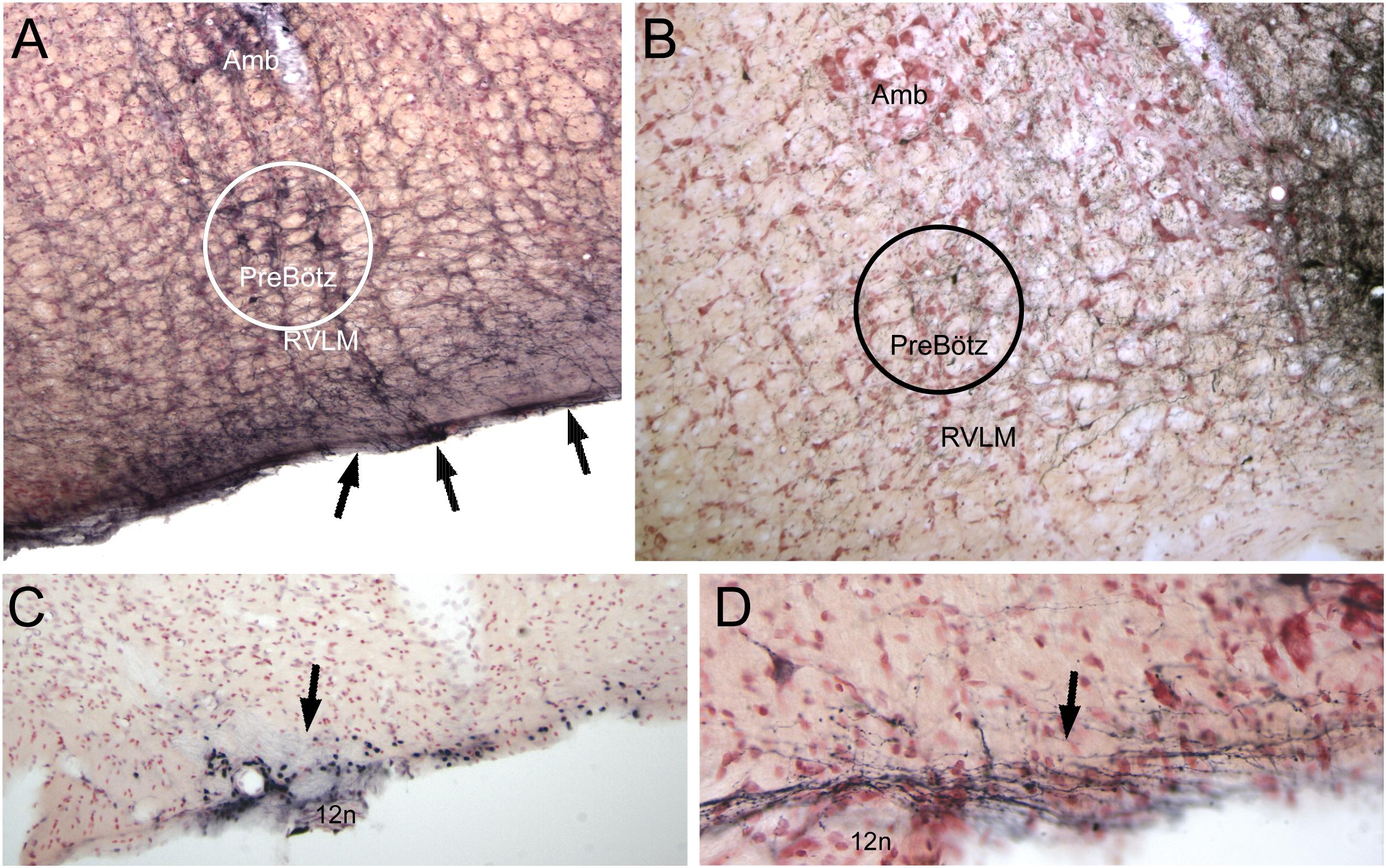
Figure 7. Neuroanatomical data implicating somatostatin neurons in the preBötzinger complex as well as neurons/cells near the ventral medullary surface as important for the diving circuit. Somatostatin neurons in the preBötzinger area have been shown important for apnea (see text for details). In A, several somatostatin immunostained neurons of medium size are encircled. Note that putative dendrites from these somatostatin neurons stream to the ventral medullary surface and appear to intertwine among the epi-glial cells found here (arrows). Anterograde transport of BDA after an injection in the ventral MDH is seen over similar medium-sized neurons in B (see also Figure 4B2). Neurons/cells near the ventral medullary surface always were immunolabeled with cFos after long submersions (see Figure 6, right column). Those found caudally near the pyramidal decussation (C, arrow), often surrounded the exit of the hypoglossal nerve (12n). The far majority of injections of BDA into the ventral MDH also showed small labeled fibers, with boutons, over similar areas (D, arrow). The epi-pia on the ventral medulla are linked by gap junctions; we hypothesize a depolarization of similar caudal epi-pia during diving would then rapidly flow rostrally, impinging on the distal dendrites of the apnea-inducing somatostatin neurons in the preBötzinger complex. See text for discussion.
Heart Rate
The dramatic bradycardia seen with underwater submersion, or after stimulation of the AEN or nasal mucosa, is mediated via the vagus nerve (see prodigious review by Ponganis et al., 2017 for their hypothesis). Most preganglionic parasympathetic cardiac motoneurons are found in the external formation of the nucleus ambiguus (Panneton et al., 1996, 2014; Taylor et al., 2001), an area of reticular formation separating sensory and somatic motor nuclei where many preganglionic autonomic neurons occur (Figures 8A1,A2; arrows). More cardiac motoneurons were found more rostrally in the CVLM (Figure 8A3) but most double labeled neurons were found in the RVLM. Double-labeling cardiac motoneurons (Panneton et al., 2014) with cFos after voluntary diving and cholera toxin after retrograde transport from cardiac injections (Figure 8B, arrow) showed that double-labeled neurons were mostly in the rostral medulla (Figure 8A4; red arrows), especially surrounding the compact formation of nucleus ambiguus. Neurons, possibly preganglionic parasympathetic cardiac motoneurons, in similar areas (see Figures 3, 5) are labeled transneuronally after HSV-1 virus injections into the AEN (Panneton et al., 2000), after injections of BDA into the MDH (Panneton et al., 2000, 2006), as well as after transganglionic transport of label in primary afferent fibers of the AEN (Panneton, 1991a; Panneton et al., 2006) (Figure 8C).
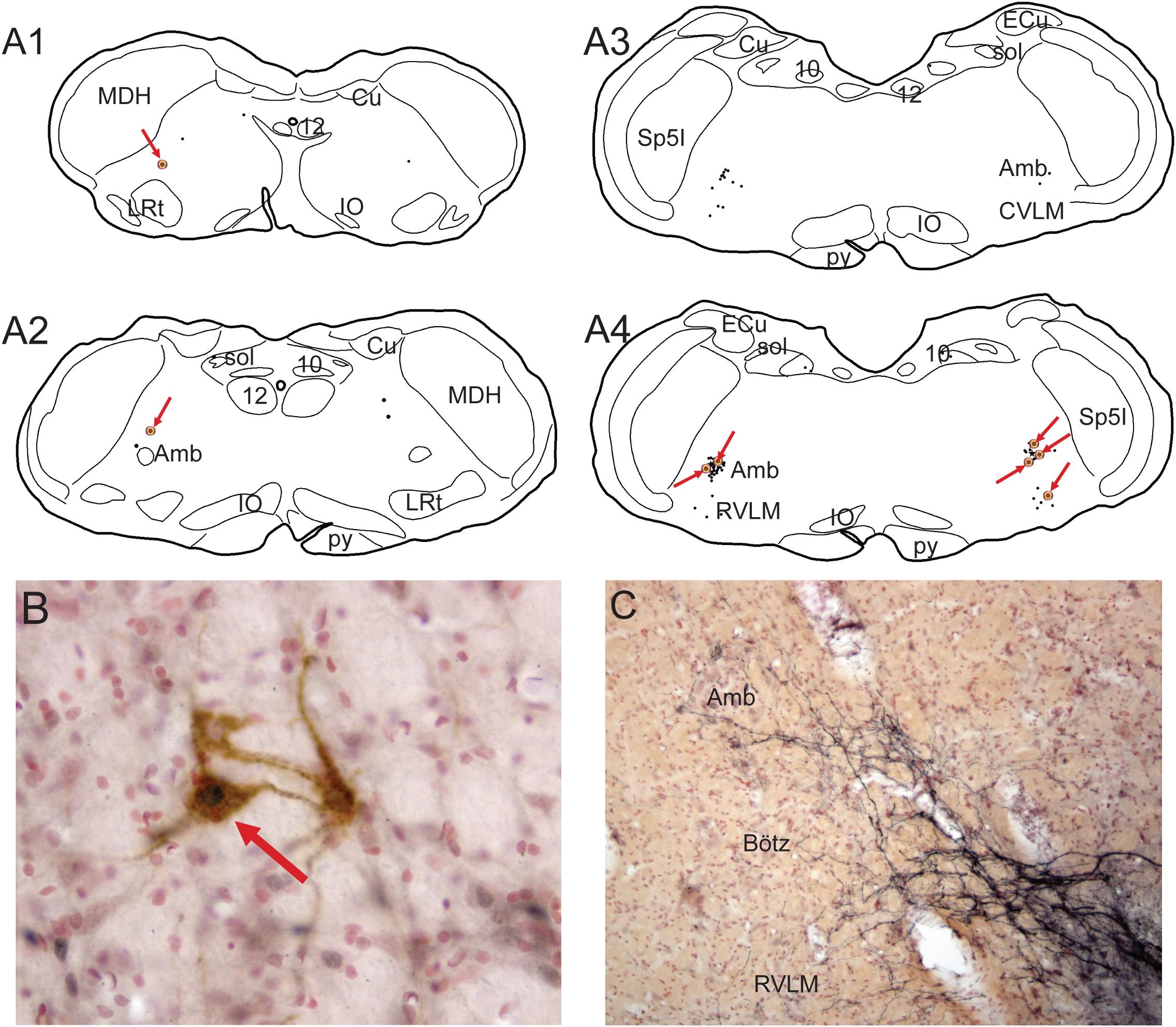
Figure 8. Illustrations showing functional neuroanatomical data from rats that voluntarily dove underwater after pericardial injections of cholera toxin into their pericardial cavities (Panneton et al., 2014) and its retrograde transport to the medulla. All rats showed marked bradycardia and an increase in arterial blood pressure typical of underwater submersion (see Figure 1B). Preganglionic cardiac motoneurons were found caudally mostly in the medullary reticular formation sandwiched between sensory and motor areas (A1,A2; arrows), but a few also were noted in the dorsal motor nucleus of the vagus nerve. Single black dots represent such preganglionic cardiac motoneurons labeled solely by cholera toxin. Double-labeled neurons represent preganglionic cardiac motoneurons activated by diving. Double-labeled neurons, marked by black dots labeled by Fos immunohistochemistry encircled by brown cytoplasmic labeling of cholera toxin (B, red arrow), were found throughout the ventrolateral medulla, but mostly rostrally (A1–4, encircled dots highlighted by red arrows). It is of interest that primary afferent fibers contained within the AEN project directly into similar reticular areas (C; compare to A4). See legends in Figures 3, 4 for abbreviations and Panneton et al. (2014) for details. Figures are reprinted from Front. Physiol., 5, Panneton et al., Parasympathetic preganglionic cardiac motoneurons labeled after voluntary diving, 8, (2014), with permission.
Work in in vitro brainstem slices show preganglionic parasympathetic cardiac motoneurons are modulated by both glutamatergic (Willis et al., 1996; Mendelowitz, 1998; Neff et al., 1998; Corbett et al., 2003) and GABAergic/glycinergic (Wang et al., 2001, 2003) inputs from the NTS. These cardiac neurons are also modulated by nicotinic cholinoceptors (Wang et al., 2001), which facilitate glutamatergic input to them (Huang et al., 2004), numerous peptides (Agarwal and Calaresu, 1991; Ruggeri et al., 2000; Irnaten et al., 2003; Blinder et al., 2005, 2007), and monoamines (Izzo et al., 1993; Wang and Ramage, 2001; Skinner et al., 2002; Gorini et al., 2009). Indeed, cardiac motoneurons activated by the stimulation of the trigeminal tract are modulated by serotonin (Gorini et al., 2009) and acetylcholine (Gorini et al., 2010) receptors. The bradycardia to nasal stimulation is enhanced when electrical stimulation of the AEN is paired with chemical stimulation of peripheral chemoreceptors (Rozloznik et al., 2009), and was even more potentiated by injections of a 5HT receptor agonist injected into the NTS, implying an integrative function of the NTS in the multimodal mediation of the DR. Such mechanisms may be important in the more prominent bradycardias seen in aquatic animals during deep dives.
Moreover, electrophysiological investigations on postganglionic cardiac motoneurons driven by diving have commenced (McAllen et al., 2011). Cardiac nerves from both the parasympathetic vagus nerve and the sympathetic system are activated during nasal stimulation with formaldehyde vapors in the rabbit (Nalivaiko et al., 2003), and the sympathetic contribution may maintain or enhance cardiac output during the bradycardia (Paton et al., 2005). We speculate that the bradycardia induced by underwater submersion activates cardiac motoneurons directly either by primary afferent fibers from the AEN projecting into the nearby reticular formation (Figures 3C,C1) and/or indirectly via projection neurons from the MDH.
Arterial Blood Pressure
Numerous studies have shown that neurons in the rostral ventrolateral medulla (RVLM) regulate ABP by maintaining sympathetic tone. Moreover, numerous studies have also implicated the RVLM as the brainstem relay to the spinal cord for the baroreceptor reflex (Guyenet, 1990; Schreihofer and Guyenet, 1997; McCulloch et al., 1999b) as well as somatosympathetic reflexes (Stornetta et al., 1989; Burke et al., 2011). The reflex circuitry driving the baroreceptor reflex has been described extensively and involves neurons in the nucleus tractus solitarii (NTS), the caudal ventrolateral medulla (CVLM), and the RVLM (Aicher et al., 2000). The increase in ABP from the induced vasoconstriction after underwater submersion activates the baroreflex, but the baroreceptive circuitry does not overlap that of the diving circuit until the RVLM (McCulloch et al., 1999b). Thus, the neuroanatomical projections from the MDH to the NTS (Figures 5A1–A3), as well as those from the transneuronal transfer of virus from the AEN (Figure 5A4), do not overlap with neurons labeled with cFos after underwater submersion (Figure 5A5). These neuroanatomical tract-tracing techniques label fibers/neurons indiscriminate of function; we believe MDH projections to the NTS are labeling fibers/neurons more associated with pain pathways versus those in the diving circuit. Moreover, bilateral injections of the excitatory amino acid receptor antagonist kynurenate made into the dorsolateral subnucleus of the NTS or the CVLM, where the baroreceptive neurons lie, greatly attenuated the baroreflex but failed to modify responses from nasal stimulation (McCulloch et al., 1999b) (Figure 9A). This view is contrary to that of others (Huang et al., 1991; Dutschmann and Herbert, 1998b) who concluded the NTS modulates diving behavior. However, injections into the RVLM greatly reduced effects of nasal stimulation on sympathetic nerve discharge but not that from baroreflex activation (Figures 9A,B). The lack of change in baroreceptor modulation of sympathetic activity after the RVLM injections of kainate is explained by the predominate GABAergic input from the CVLM to the RVLM, while blocking of RVLM activation after nasal stimulation suggests excitatory amino acids synaptically drive this projection.
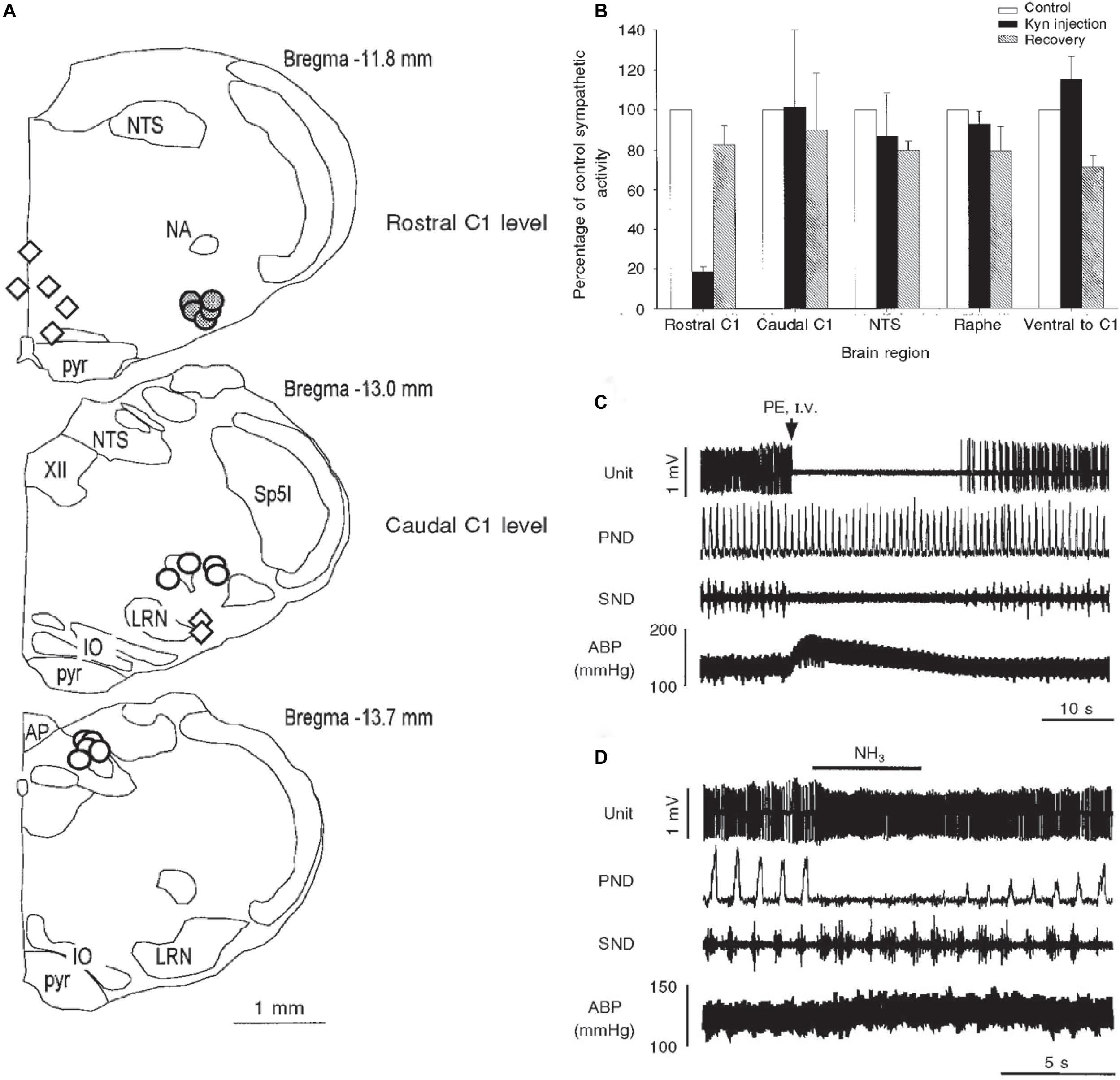
Figure 9. Illustrations mapping physiological data implicating several medullary areas important for the diving response. Bilateral injections of kyurenate made at levels of the rostral C1 area (RVLM), caudal C1 area (CVLM), nucleus tractus solitarii (NTS), and raphe obscuris are plotted in A. The effect of nasal stimulation on sympathetic nerve discharge (SND) was unchanged after injections into the CVLM, NTS (open circles) or raphe and ventral to CVLM (open diamonds), but was reduced by 80% after injections into the RVLM, suggesting the RVLM mediates the sympathetic response. Normalized sympathetic responses to nasal stimulation from multiple trials are shown (B); again, only injections into the rostral C1 induced a significant decrease in SND. The electrophysiological responses of a typical single baroreceptive neuron in the RVLM is seen in C; note that this unit is silenced by increases in blood pressure after phenylephrine administration and SND ceases, but phrenic nerve discharge (PND) is maintained. The same neuron is excited, however, after nasal stimulation (D), even with the increase in ABP; the PND is also silenced with nasal stimulation and the SND is increased. 24/39 of similar baroreceptive neurons, normally silent with increases of ABP, actually increased their firing rate by nearly 66% and increased SND by 102%, despite an increase of ABP of 28 ± 2 mmHg. These data suggest the homeostatic baroreceptor reflex is inhibited during diving. Figures are reprinted from J. Physiol., 516, McCulloch et al., The rostral ventrolateral medulla mediates the sympathoactivation produced by chemical stimulation of the nasal mucosa, 471–484 (1999), with permission. See McCulloch et al. (1999a) for more details.
The RVLM contains the rostral C1 adrenergic cell group (Ruggiero et al., 1985) that provides bulbospinal projections to the intermediolateral cell column in the spinal cord; many such neurons are activated by underwater submersion (Figures 5C5, 10D) (McCulloch and Panneton, 2003). However, both adrenergic and non-adrenergic spinally projecting neurons in the RVLM are responsive to nasal stimulation (McCulloch et al., 1999b). Moreover, 62% of the same baroreceptive RVLM neurons normally silenced by increases in ABP are excited by nasal stimulation despite increases in ABP (Figures 9C,D; McCulloch et al., 1999b), suggesting that the homeostatic baroreceptor reflex is overridden. Several lines of neuroanatomical evidence also suggest that these RVLM neurons are important in the DR, including their activation of cFos (Figure 10A) after diving, the overlap of CGRP fibers (from primary afferent fibers) (Figure 10B), and the overlap of projections from the MDH (Figures 10C,E). These bulbospinal neurons could get input from somatosensory neurons either directly from primary afferent fibers of the AEN into the ventrolateral reticular formation (Figures 3C,C1) or relay from non-baroreceptive neurons in the CVLM or from the MDH.
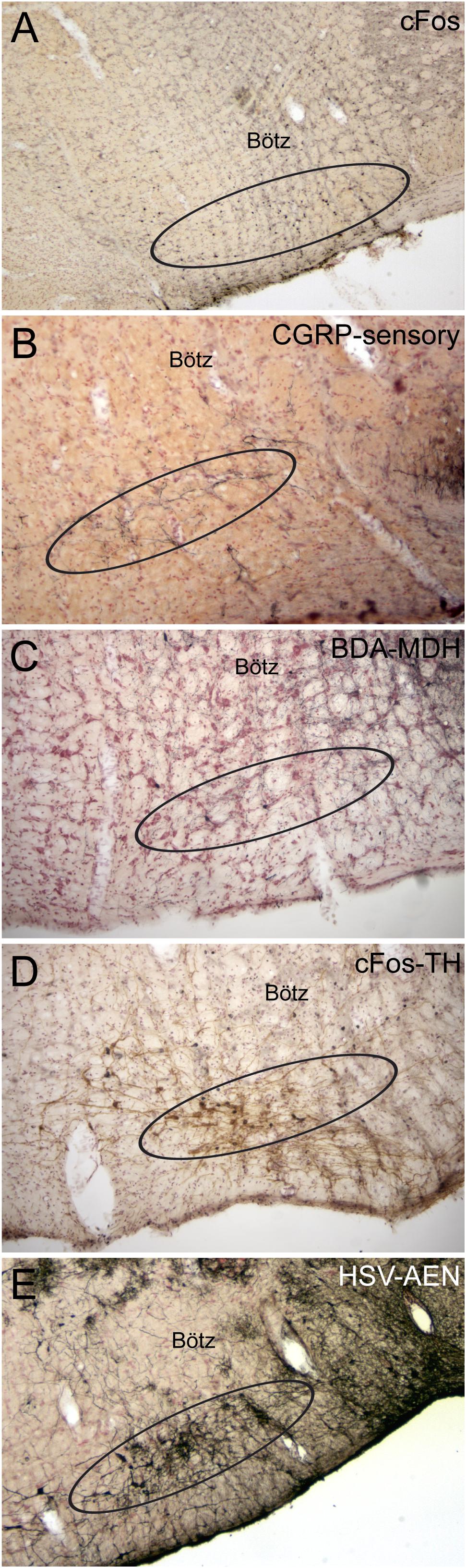
Figure 10. Photomicrographs of neuroanatomical data implicating the RVLM as an important link in the circuit driving the diving response. Ovals drawn ventral to the Bötzinger complex (Bötz) demarcate our definition of the RVLM in A–E. Numerous neurons always were immunolabeled with cFos in the RVLM after underwater submersion (A), and many of these neurons were also double labeled for tyrosine hydroxylase (D), suggesting that both noradrenergic as well as non-noradrenergic neurons are activated. We have shown (see Figure 8C) that the anterior ethmoidal nerve projects directly to the RVLM; B shows that many of these fibers are also immunoreactive to CGRP (see Panneton and Gan, 2014 for discussion). The RVLM also receives indirect projections from nasal areas of the MDH. Two tracing techniques, the anterograde transport of BDA after an injection in the ventral MDH of the rat (C; see Panneton et al., 2006 for details) and the transneuronal transport of HSV-1 virus in a muskrat (E; see Panneton et al., 2000 for details) suggest this is the case.
Suprabulbar Control of the Diving Response
We were initially impressed by reading many years ago that a seal showed an abrupt and dramatic bradycardia prior to underwater submergence and a tachycardia prior to emersion (Casson and Ronald, 1975). Also, differences in heart rate of marine mammals diving voluntarily show that the DR is more variable and less intense than during involuntary dives (Kooyman and Campbell, 1972; Hill et al., 1987; Jobsis et al., 2001). Similarly, the hemodynamic responses to “forced” submersions when mammals are involuntarily “dunked” underwater (Koppányi et al., 1929; Scholander, 1963; Tchobroutsky et al., 1969; Dykes, 1974; Lin, 1974; Lin and Baker, 1975; Martner et al., 1977; Drummond and Jones, 1979; Jones et al., 1982; Hill et al., 1987; McCulloch and Jones, 1990; Jobsis et al., 2001; Panneton et al., 2010b) are subtly dissimilar to the hemodynamics of voluntary diving (Drummond and Jones, 1979; Kooyman, 1989; McCulloch and Jones, 1990; Rybka and McCulloch, 2006; Panneton et al., 2010b). This suggests that marine mammals may have “control” over their “autonomic” NSs, which is considered taboo by many teachers of physiology but certainly has adherents (Blix et al., 2010). The compendium by Houser (2018), as well as that of Elmegaard et al. (2016) cite copious examples documenting volitional control of heart rate by marine mammals, supporting this case. However, there are numerous factors controlling the HR in diving mammals including temperature, apnea, and submergence duration and depth, as well as exercise intensity (Davis and Williams, 2012; Noren et al., 2012; McDonald and Ponganis, 2014; Williams et al., 2015; Kaczmarek et al., 2018; McDonald et al., 2018). For example, the HR increases with exercise (a sympathetic response) working against an increasing bradycardia (a parasympathetic response) with depth. Moreover, recent data on seals using non-invasive infrared spectroscopy show these animals routinely exhibit preparatory peripheral vasoconstriction accompanied by increased cerebral blood volume approximately 15 s before submersion (McKnight et al., 2019). These anticipatory adjustments confirm that blood redistribution in seals also is under some degree of cognitive control that precedes the mammalian dive response. Thus, while respiration is under volitional control in higher mammals, these data also suggest that higher marine mammals can also control their cardiovascular systems volitionally.
Thus, it is possible that preventing an organism from deciding its own fate by involuntary submersion may induce both fear and stress, and these emotions may alter normal reflex responses. Studies on terrestrial animals have shown both significant bradycardia and increases in ABP during extreme fear (Gabrielsen et al., 1977; Smith and Woodruff, 1980; Smith et al., 1981; Smith and Tobey, 1983; Carrive, 2000; Zhang et al., 2004), similar to that seen during diving. However, the bradycardia in all of our studies on rats has been locked tightly to the time submerged, and immediately returned to normal after exiting the water (Figures 1B,C). It is noteworthy, however, that changes in the HR and the ABP were more varied in dunked naïve rats (Figure 1C2) and there were more arrhythmias (Figures 4A1, 11; de Burgh Daly, 1984). We and others noted similar changes previously (Byku et al., 2004; McCulloch et al., 2010) and McCulloch et al. (2010) concluded that forced submergence is stressful to both naïve and trained rats but voluntary diving in trained rats is no more stressful than being handled by humans. While it is generally accepted that the bradycardia of voluntary diving is vagally mediated and dominant, forced underwater submersion stresses the animal and may also activate suprabulbar neurons influencing the sympathetic NS. Many have noted that coactivation of both parasympathetic and sympathetic cardiac nerves induces cardiac arrhythmias (Paton et al., 2005; Shattock and Tipton, 2012). The arrhythmias during forced diving possibly induced “fear” or stress, activating the sympathetic NS and countering the bradycardia of underwater submersion.
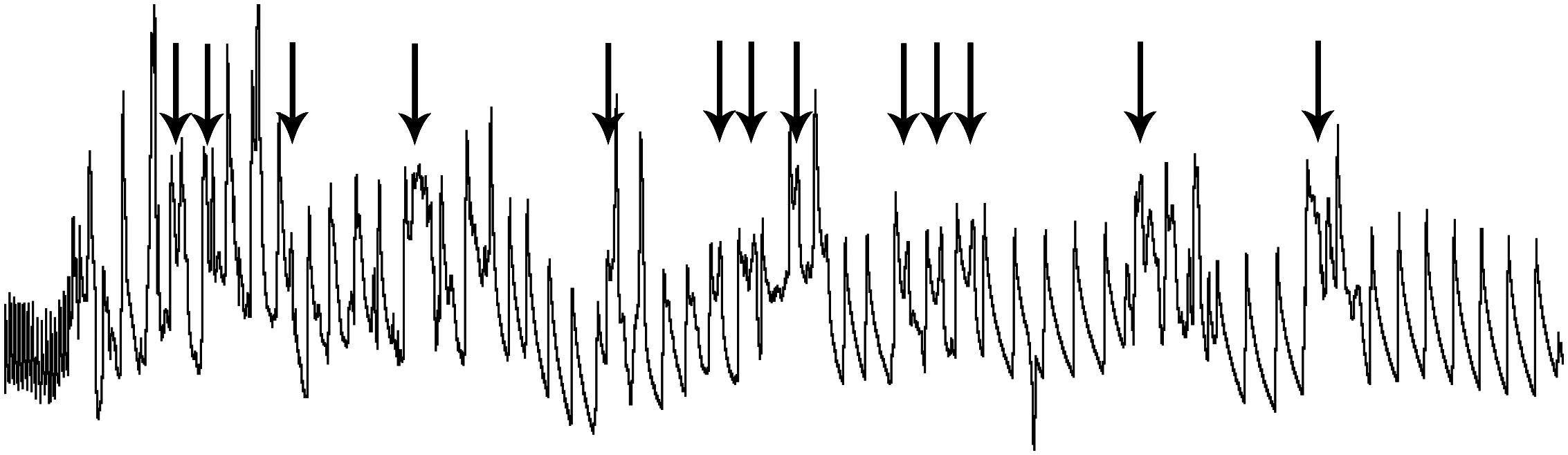
Figure 11. An expanded trace of ABP in a rat involuntarily submerged underwater. The involuntary submersion induces stress in the naïve animal, inducing sympathetic discharge. The marked slowing of the pulse on the left of the trace shows the remarkable bradycardia, the result of parasympathetic discharge, resulting as soon as the rat’s nose is submerged. Arrows above the trace illustrate the confound of ectopic beats, creating arrhythmias, when untrained rats are involuntarily submerged. Such arrhythmias are commonly seen in deep-diving marine mammals and elite human divers and are thought to result from the competitive sympathetic and parasympathetic influences over heart rate. Such “diving” rodents may prove valuable as a tool to study cardiac arrhythmias. Figure is reprinted from J. Appl. Physiol., 109, Panneton et al., Cardiorespiratory and neural consequences of rats brought past their aerobic dive limit, 1256–1269 (2010), with permission.
Seals often show either little bradycardia when diving voluntarily (Kooyman and Campbell, 1972), may reduce heart rate in anticipation of underwater submersion (Casson and Ronald, 1975), induce a bradycardia to non-somatic stimulation (Irving et al., 1942), or an anticipatory tachycardia prior to emerging (Casson and Ronald, 1975; Blix and Folkow, 1983). Sea lions conditioned to adjust their autonomic NSs to auditory or visual commands suggest they may “will” the bradycardia (Ridgeway et al., 1975; Ponganis et al., 1997) from suprabulbar sites. Such premature autonomic behavior in the diving laboratory rat has not been published, however, suggesting that cortical/suprabulbar influences on the DR in the rat is minimal. It is of interest that cetaceans and pinnipeds, considered intelligent species by most, have brains that approach humans’ brains in complexity with highly convoluted cortices (Marino, 1998; Marino et al., 2000, 2001, 2004; Hof et al., 2005; Eriksen and Pakkenberg, 2007; Hof and Van der Gucht, 2007); such complexity overwhelms that of lissencephalic rodent brains. We suggest that the DR has but minimal suprabulbar modulation in rodents, but suprabulbar neurons in higher species, perhaps those in the neocortex, may indeed direct autonomic behaviors seen in the DR. Perhaps the DR is analogous to the blink reflex, a reflex endemic in all mammals. The blink reflex has no suprabulbar control in lower species similar to that in neonatal humans. But as humans’ age and their neocortices mature, they can control their blink reflex volitionally and produce “winking” behavior. Intelligent marine mammals may have harnessed diving behavior similarly.
The Diving Response in the Human
Diving behavior is well documented in humans (Ferrigno et al., 1997; Ferretti, 2001; Foster and Sheel, 2005; Lindholm and Lundgren, 2009). However, metrics such as the HR are more variable in adults (Olsen et al., 1962; Hiebert and Burch, 2003; Caspers et al., 2011) than in infants (Goksör et al., 2002). The DR, with its elevated activation of vagal cardiac nerves, long has been acknowledged as a treatment for paroxysmal atrial tachycardia (Wildenthal et al., 1975; Gooden, 1982) by normalizing sinus rhythm. Moreover, cases of “cold water drowning” in humans, where children lie submerged underwater for prolonged periods, but recover basically unharmed, have been documented numerous times (Hayward et al., 1984; Golden et al., 1997; Xu et al., 1999; Giesbrecht, 2000). Thus, perhaps in these cases, a DR induces a persistent apnea, saving these victims from inhaling water and drowning.
The powerful DR has also been suggested to be deleterious to the human condition. For example, the DR has been implicated in the etiology of sudden infant death syndrome (SIDS) (Lobban, 1991, 1995; Matturri et al., 2005), where neonates apparently become apneic and die without pathology. Epidemiological data suggest that rebreathing asphyxial gases (mostly carbon dioxide), smoking, and reduced heat loss are important risk factors in SIDS (Kemp, 1996; Leiter and Böhm, 2007; Mitchell, 2009). It is of interest that others have noted the increased prevalence of infections of the upper respiratory tract in SIDS victims (Blackwell and Weir, 1999; Blackwell et al., 1999; Harrison et al., 1999; Molony et al., 1999; Morris, 1999; Rambaud et al., 1999; Goldwater, 2017). Such infections produce inflammatory mediators (Guntheroth, 1989; Lindgren and Grogaard, 1996), which sensitize C-fibers (Reeh et al., 1986; Handwerker et al., 1991; Lee and Widdicombe, 2001) and lower activation thresholds. Indeed, we have shown that small diameter fibers densely innervate the nasal mucosa via the AEN (McCulloch et al., 1999a), while others show inflammatory mediators in the upper respiratory tract promote apnea (Lindgren and Grogaard, 1996). It is of interest that the solitary chemosensory cells found in the anterior nasal mucosa and innervated by small diameter fibers of the trigeminal nerve (Tizzano et al., 2010), probably the AEN, are activated by acyl-homoserine lactones produced by Gram-negative bacteria. Activation of these chemosensory cells also promotes an apnea. Moreover, nasal applications of both smoke (White et al., 1974; Kobayashi et al., 1999; Ho and Kou, 2000) and carbon dioxide (Yavari et al., 1996), both risk factors for SIDS, induce the DR while involuntary submersion induces apneas beyond the aerobic dive threshold (Panneton et al., 2010a), suggesting perhaps the persisting apnea could induce death. Perhaps the DR is induced in infected SIDS victims who nasally rebreathe high levels of CO2 (Sakai et al., 2009) so that they hold their breath until they die. A plethora of citations providing background implicating the mammalian DR in sudden cardiac death, arrhythmias, and SIDS in the human clinical literature are found in the theoretical dissertations of Vincenzi (2019) and Vega (2018).
The bradycardia induced in diving humans often is combined with arrhythmias (Scholander et al., 1962; Lindholm and Lundgren, 2009; Shattock and Tipton, 2012), possibly mimicking the yin and yang of cardiac autonomic control seen in rodents (Figure 11). The dual activation of both systems is hypothesized to induce the numerous arrhythmias seen in deep dives in marine mammals. Forced submersion of rodents may provide a model to study these arrhythmias further. The theory of “autonomic conflict” that develops during underwater submersion in cold water, e.g., the activation of both parasympathetic and sympathetic cardiac nerves, may account for the genesis of cardiac arrhythmias and dysrhythmias seen during diving (Shattock and Tipton, 2012; Bierens et al., 2016). The fact that ectopic beats can be generated during diving experiments in rats (Figure 11) might be utilized to test therapies designed to quench these arrhythmias. While autonomic conflict often results in arrhythmias, fatal arrhythmias are much less common and usually coupled with predisposing factors including ischemic heart disease, long QT, channelopathies, and atherosclerosis.
The power of the DR might also be harnessed to combat other human maladies. Cerebral blood flow is significantly increased in humans by inducing a DR (Brown et al., 2003; Kjeld et al., 2009), similar to that of rodents (Irving, 1938; Ollenberger and West, 1998a,b; Ollenberger et al., 1998) and seals (Zapol et al., 1979; Blix and Folkow, 1983; Odden et al., 1999; Tift and Ponganis, 2019), probably in an effort to oxygenate this necessary organ. It is of interest that a cerebral hypotension precedes migraine headaches (Olesen, 1991; Thomsen et al., 1995); perhaps inducing a DR in patients experiencing a prefatory aura could alter such cerebrovascular dysregulation and prevent migraines. Using the DR to mitigate certain migraine headaches could be a natural, inexpensive remedy. The DR might also provide therapy in stroke and hemorrhagic shock by increasing cerebral blood flow (Golonov et al., 2016; Chiluwal et al., 2017). Perhaps this feature of the DR could be utilized to reduce the ischemic penumbra and infarct volume due to stroke (Pan et al., 2007; Golonov et al., 2016; Chiluwal et al., 2017). The penumbra describes compromised brain tissue with a decreased oxygen supply which may eventually become necrotic. Increasing blood flow to the penumbra after stroke during a DR may nourish the deprived cells enough to prevent further death. Moreover, the DR has been implicated in sudden unexpected death in epilepsy (Stewart, 2018; Vega, 2018), and experimental studies utilizing nasopharyngeal irrigation concluded that seizure-associated central apnea and the DR share a common neural basis and may reflect an attempt by brainstem networks to protect core physiology during seizure activity (Villiere et al., 2017; Stewart, 2018; Mooney et al., 2019).
Little is known of the neural circuitry driving the DR in humans but behaviors that serve basic vegetative functions are usually less complex and more uniform across species, so we suspect that much known from the reflex circuit of a rodent, or the unexplored circuits of marine mammals, would also apply to humans. Moreover, the fact that the more neurally developed marine mammals can “control” their HR’s at will could perhaps be exploited with techniques designed to show which higher levels of the brain are activated during this autonomic control. Seals and dolphins are increasingly being trained for the study of diving behaviors, including HR regulation. Perhaps they could be trained to perform such feats under a functional MRI or PET scan, an expensive experiment but probably would detail cortical areas directing this control over the autonomic NS. Similar efforts (e.g., fMRI) could be performed on humans, assuming some adult humans can be trained to induce a reliable DR, perhaps with biofeedback techniques, to gain volitional control over their autonomic NS. Indeed, reports on these lines are developing (Abukonna et al., 2013; Jones et al., 2015). Such mind–body interactions could be utilized to control affective symptoms of anxiety in humans (Jones et al., 2015), as well as a method to induce general relaxation for mitigating stress, a malady afflicting an overwhelming number of humans.
As current technology refines and new technologies are born, new discoveries are forthcoming concerning the enigmatic mammalian DR. Indeed, genetic studies are now underway illustrating how diving mammals, including humans, have adapted to their anoxic underwater environments (Fabrizius et al., 2016; Baranova et al., 2017; Hoff et al., 2017; Ilardo et al., 2018; Zhou et al., 2018; Xu et al., 2019). Introduction of the DR in the mouse (Hult et al., 2019) provides opportunity for an entirely novel set of techniques for genetic manipulation of neurons. Utilization of these data on the DR in rodents thus provides practical animal models for study of the mechanisms driving the response, data from which could be applied to humans.
Summary and Perspectives
The DR is indeed a dramatic perturbation of normal function, altering basic homeostatic mechanisms to fit physiological needs. This review emphasizes both the reflex nature of the DR and its neuronal circuitry maintained in the medulla and spinal cord, like numerous other reflexes. Many stimuli affecting paranasal areas initiate the DR, and nerves innervating these areas serve as its afferent limb. We suggest sensory fibers of the AEN projecting to the MDH (Figure 12; green lines) mediate much of the DR and noted relays from the MDH to neuronal ensembles driving respiration, heart rate, and vasoconstriction (Figure 12; purple lines). Although only sparse projections were noted to the ventral respiratory column from the MDH, we also suggest a projection to the ventral medullary surface transmitted along gap junctions to somatostatin neurons in the preBötzinger complex may reinforce the apnea (Figure 12; blue line) by disrupting normal rhythm generation. Our data suggest the bradycardia (Figure 12; red line) and peripheral vasoconstriction (Figure 12; orange line) are mediated by neurons in the rostral medulla, and their input by trigeminal neurons is either direct via primary afferent fibers or indirect via the MDH. Since there are inferences implicating that some marine species “will” the DR as well as numerous instances when humans breath-hold, suprabulbar control must intercede in the reflex circuitry, much like when humans induce a response similar to the blink reflex and “wink.”
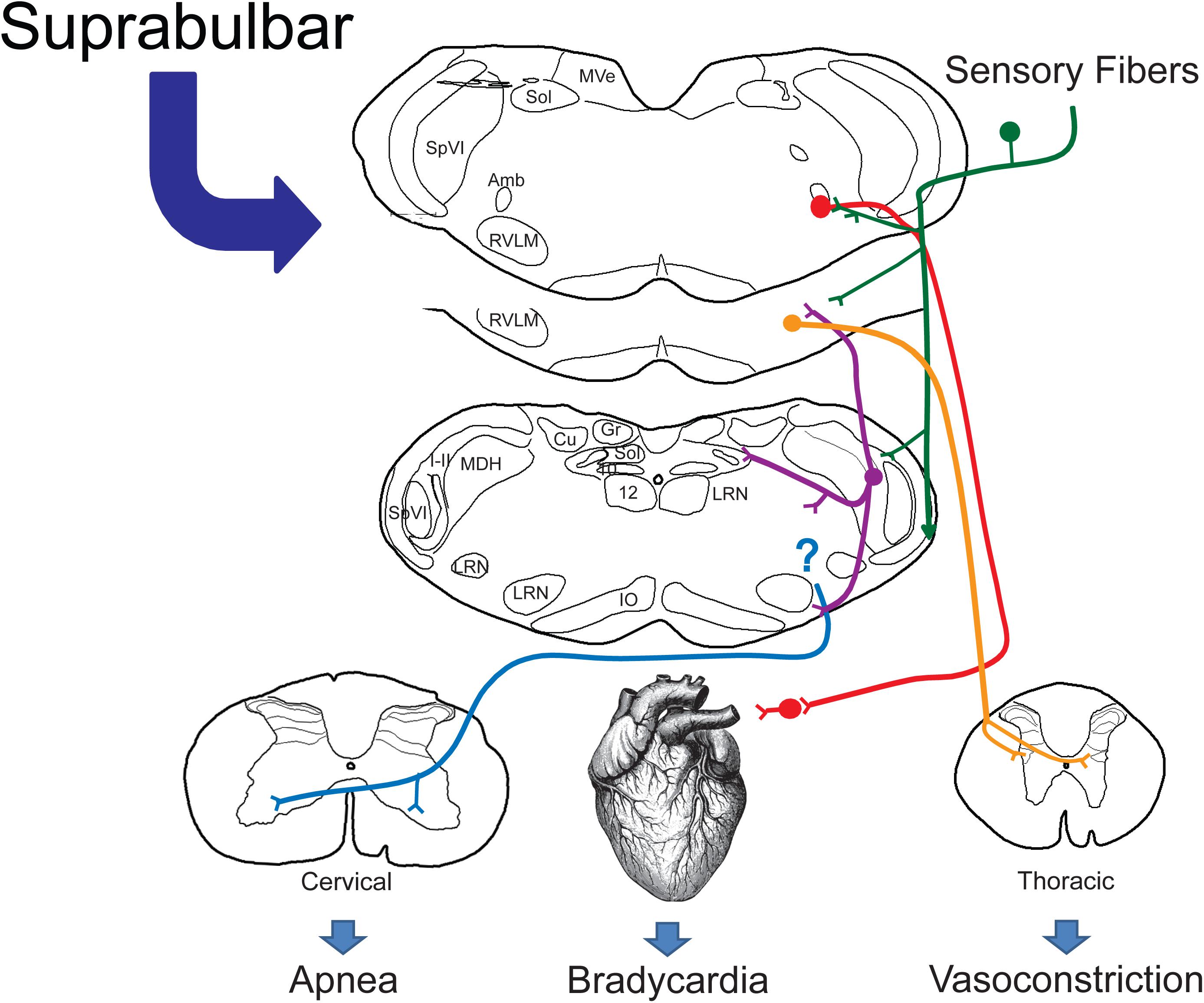
Figure 12. Proposed brainstem circuitry mediating the cardiorespiratory depression with underwater submersion, e.g., the mammalian diving response. We propose direct primary afferent projections from nasal and paranasal areas (green lines) both to the MDH for relay and to the lateral reticular formation, where cardiac motoneurons and sympathetic interneurons lie. We know that the DR can be blocked in the MDH (Figure 3) and neurons from this area project (purple lines) to the CVLM, the RVLM (including sympathetic interneurons and respiratory rhythm-generating neurons), and the ventral medullary surface, where respiratory chemosensitive neurons are found (see text for discussion). Although the location of respiratory neurons sending inhibitory signals to the phrenic motor nucleus in the cervical spinal cord (blue lines) is unknown, the respiratory inhibition may be the result of inhibition of the respiratory pattern generator. The presympathetic neurons in the RVLM project to the intermediolateral cell column of the thoracic spinal cord and are important for mediating the peripheral vasoconstriction (orange lines) during diving. While preganglionic cardiac motoneurons are found throughout the medulla, most neurons double-labeled after diving were near to the compact formation of the nucleus ambiguus juxtaposed to primary afferent fibers, many from the AEN. We propose that these neurons (red lines) project to postganglionic neurons near the heart, inducing the bradycardia seen in the diving response. We suggest this relatively simple but well-organized circuit orchestrates the automatic reflex responses (100% of our rodents 100% of the time show a diving response to underwater submersion). We also propose that such a circuit provides the substrate upon which suprabulbar neurons impinge, allowing higher mammals to willfully control their diving response.
The universal inclusion of the DR in a wide variety of vertebrates, both marine and terrestrial, is made throughout this review. Indeed, even early diverged mammals, like the platypus, exhibit a dive response (Johansen et al., 1966; Bethge et al., 2003). Although it is utilized best and most by marine mammals, the dive response is also pronounced in non-marine species like common laboratory rodents. While marine mammals have both harnessed the DR as well as adapted numerous systems to prolong underwater submersion, there is no explanation as to why terrestrial animals also have this profound response. It must be remembered, however, that pinnipeds and cetaceans evolved after a migration of terrestrial ungulates adapted to an aquatic environment (Thewissen et al., 2007). Thus, the physiological consequences of underwater submersion that we term the DR may have been directed by NSs before marine mammals even existed. This implies that perhaps the moniker “DR” is misleading and in fact a misnomer. Perhaps a purpose of this enigmatic reflex is to indeed to preserve life of the organism (Panneton, 2013).
The neural circuits driving the DR are probably intrinsic in all vertebrate species, implying these circuits are the simplest, the most organized and the most automatic. Those circuits driving the DR also probably were born early in our evolutionary history. The commonality speaks to ancient evolutionary adaptations shared by all vertebrates in their battles against asphyxiation (Hagen, 2018). There are some references in the diving literature about the evolutionary significance of the DR (Hochachka, 1997; Mangum and Hochachka, 1998; Mottishaw et al., 1999), but most of such references are implied only for marine mammals. More work must still be done in dissecting the components of the neural circuits important for the DR, but perhaps more discussions on the teleology of this phenomenal response also are in order to better understand it. The phrase “Master switch of life” (Scholander, 1963), or the striking redistribution of blood supply to organs most essential to life (the heart and brain), may provide new discussion on this phenomenal response.
This essay deals with the neural circuits driving the DR in the medulla of two species of rodents, which are the preferred animals for many laboratory experiments. However, the use of terrestrial rodents to study the mammalian DR does not discount the plethora of data accumulated over the past eighty years, and still being produced, on the DR of marine mammals. As mentioned previously, these large marine mammals are thought to possess considerable intelligence, and, in least in our opinion, make it ethically unfathomable to sacrifice these animals to study their brains. We thus offer these rodent models to those interested in studying the neural control of the mammalian DR. If the profound autonomic changes seen in the DR can be utilized in its clinical implications for SIDS, SUDEP, arrhythmias, stroke, headache, anxiety, and others in humans, perhaps the power of this response may be harnessed for the betterment of mankind. The DR is more than an invariant hardwired response and has many overlying factors effecting the DR are best seen in the variables regulating the HR in marine mammals. We suspect that its neural control in higher marine mammals makes adjustments for many conditions, even those for “anticipated” physiological needs, but eventually the output will traverse the basic circuit described.
Perhaps humans can be trained to harness the incredible power the DR has over the automatic systems which drive our organism, thus mimicking those seen in pinnipeds and cetaceans. The innumerable choices inflicted on contemporary man, from all strata of all societies, parallels that of the rise in anxiety and stress levels; some in the general public already promote inducing the DR as a relaxation technique. The DR’s power over cerebral blood flow may be a fast and efficient way to treat incipient migraine headaches as well as minimize the effects of transient ischemic attacks (TIA) and reduce the ischemic penumbra of stroke. Controlling the powerful DR may open doors into therapies for numerous human pathologies.
Author Contributions
WP designed the study and wrote the first draft of the manuscript. QG performed the statistical analysis and made comments on the manuscript.
Funding
This work was supported by NIH grants HL38471 and HL64772 as well as monies from Saint Louis University, all to WP. All grants were for brainstem research on neural circuits, including that of the diving response.
Conflict of Interest
The authors declare that the research was conducted in the absence of any commercial or financial relationships that could be construed as a potential conflict of interest.
Acknowledgments
We first thank our colleagues for their contributions toward understanding the circuitry driving diving behavior, especially Patrice Guyenet for his work on the sympathetic component of the diving response and Thomas Dahms for measuring blood gases in rats brought to their aerobic dive limit. We acknowledge the support of postdoctoral fellows Parviz Yavari and Paul McCulloch, graduate student Wei Sun, and the technical expertise of Rajko Juric and Whitney Panneton, whose work made these studies possible. We thank Stephen Swoap for his comments on earlier drafts of this work. We also thank the legion of scientists who find the diving response as fascinating as us, and their many published articles on marine mammals.
Supplementary Material
The Supplementary Material for this article can be found online at: https://www.frontiersin.org/articles/10.3389/fnins.2020.00524/full#supplementary-material
VIDEO S1 | A low resolution video showing a trained rat voluntarily diving through an underwater maze. We gave the rats no obvious reward for their endeavors; perhaps their ‘freedom’ (we allowed them to run uncaged on top of a table top) was an inducement. Rats were trained 5 days/week prior to the experiment. See Panneton et al. (2014) and McCulloch (2014), for more details.
References
Abukonna, A., Yu, X., Zhang, C., and Zhang, J. (2013). Volitional control of the heart rate. Int. J. Psychophysiol. 90, 143–148. doi: 10.1016/j.ijpsycho.2013.06.021
Agarwal, S. K., and Calaresu, F. R. (1991). Enkephalins, substance P and acetylcholine microinjected into the nucleus ambiguus elicit vagal bradycardia in rats. Brain Res. 563, 203–208. doi: 10.1016/0006-8993(91)91534-8
Aicher, S. A., Milner, T. A., Pickel, V. M., and Reis, D. J. (2000). Anatomical substrates for baroreflex sympathoinhibition in the rat. Brain Res. Bull. 51, 107–110. doi: 10.1016/s0361-9230(99)00233-6
Angell James, J. E., and de Burgh Daly, M. (1969). Nasal reflexes. Proc. R. Soc. Med. 62, 1287–1293.
Angell James, J. E., and de Burgh Daly, M. (1972). Reflex respiratory and cardiovascular effects of stimulation of receptors in the nose of the dog. J. Physiol. 220, 673–696. doi: 10.1113/jphysiol.1972.sp009729
Angell James, J. E., and de Burgh Daly, M. (1973). The interaction of reflexes elicited by stimulation of carotid body chemo-receptors and receptors in the nasal mucosa affecting respiration and pulse interval in the dog. J. Physiol. 229, 133–149. doi: 10.1113/jphysiol.1973.sp010131
Anton, F., and Peppel, P. (1991). Central projections of trigeminal primary afferents innervating the nasal mucosa: a horseradish peroxidase study in the rat. Neuroscience 41, 617–628. doi: 10.1016/0306-4522(91)90354-q
Baranova, T. I., Berlo, D. N., Glotov, O. S., Korf, E. A., Minigali, A. D., Mitrofanova, A. V., et al. (2017). Genetic determination of the vascular reactions in humans in response to the diving reflex. Am. J. Physiol. Heart Circ. Physiol. 312, H622–H631.
Barham, H. P., Cooper, S. E., Anderson, C. B., Tizzano, M., Kingdom, T. T., Finger, T. E., et al. (2013). Solitary chemosensory cells and bitter taste receptor signaling in human sinonasal mucosa. Intern. Forum Allergy Rhinol. 3, 450–457. doi: 10.1002/alr.21149
Beckstead, R. M., and Norgren, R. (1979). An autoradiographic examination of the central distribution of the trigeminal, facial, glossopharyngeal, and vagal nerves in the monkey. J. Comp. Neurol. 184, 455–472. doi: 10.1002/cne.901840303
Beidenbach, M. A., Beuerman, R. W., and Brown, A. C. (1975). Graphic-digitizer analysis of axon spectra in ethmoidal and lingual branches of the trigeminal nerve. Cell Tiss. Res. 157, 341–352.
Benarroch, E. E. (2007). Brainstem respiratory sensitivity: new insights and clinical implications. Neurology 68, 2140–2143. doi: 10.1212/01.wnl.0000266560.60371.98
Bereiter, D. A. (1993). Microinjections of glutamate within trigeminal subnucleus interpolaris alters adrenal and autonomic function in the cat. Brain Res. 622, 155–162. doi: 10.1016/0006-8993(93)90814-4
Bereiter, D. A., and Benetti, A. P. (1991). Microinjections of calcitonin gene-related peptide within the trigeminal subnucleus caudalis of the cat affects adrenal and autonomic function. Brain Res. 558, 53–62. doi: 10.1016/0006-8993(91)90713-6
Bereiter, D. A., Benetti, A. P., and Thrivikraman, K. V. (1990). Thermal nociception potentiates the release of ACTH and norepinephrine by blood loss. Am. J. Physiol. 259, R1236–R1242.
Bereiter, D. A., and Gann, D. S. (1988a). Adrenal secretion of catecholamines evoked by chemical stimulation of trigeminal nucleus caudalis in the cat. Neuroscience 25, 697–704. doi: 10.1016/0306-4522(88)90270-9
Bereiter, D. A., and Gann, D. S. (1988b). Glutamate activation of neurons within trigeminal nucleus caudalis increases adrenocorticotropin in the cat. Pain 33, 341–348. doi: 10.1016/0304-3959(88)90294-1
Bereiter, D. A., and Gann, D. S. (1989). Substance P and GABAergic effects on adrenal and autonomic function evoked by microinjections into trigeminal subnucleus caudalis in the cat. Brain Res. 490, 307–319. doi: 10.1016/0006-8993(89)90248-5
Bethge, P., Munks, S., Otley, H., and Nicol, S. (2003). Diving behaviour, dive cycles and aerobic dive limit in the platypus Ornithorhynchus anatinus. Comp. Biochem. Physiol. A Mol. Integr. Physiol. 136, 799–809. doi: 10.1016/s1095-6433(03)00198-3
Bianchi, A. L., Denavit-Saubié, M., and Champagnat, J. (1995). Central control of breathing in mammals: Neuronal circuitry, membrane properties, and neurotransmitters. Physiol. Rev. 75, 1–46.
Bierens, J. J. L. M., Lunetta, P., Tipton, M., and Warner, D. S. (2016). Physiology of drowning: a review. Physiology 31, 147–166. doi: 10.1152/physiol.00002.2015
Blackwell, C. C., MacKenzie, D. A. C., James, V. S., Elton, R. A., Zorgani, A. A., Weir, D. M., et al. (1999). Toxigenic bacteria and sudden infant death syndrome (SIDS): nasopharyngeal flora during the first year of life. FEMS Immunol. Med. Microbiol. 25, 51–58. doi: 10.1111/j.1574-695x.1999.tb01326.x
Blackwell, C. C., and Weir, D. M. (1999). The role of infection in sudden infant death syndrome. FEMS Immunol. Med. Microbiol. 25, 1–6.
Blinder, K. J., Johnson, T. A., and Massari, V. J. (2005). Enkephalins and functionally specific vagal preganglionic neurons to the heart: ultrastructural studies in the cat. Auton. Neurosci. 120, 52–61. doi: 10.1016/j.autneu.2005.03.005
Blinder, K. J., Moore, C. T., Johnson, T. A., and John Massari, V. (2007). Central control of atrio-ventricular conduction and left ventricular contractility in the cat heart: synaptic interactions of vagal preganglionic neurons in the nucleus ambiguus with neuropeptide Y-immunoreactive nerve terminals. Auton. Neurosci. 131, 57–64. doi: 10.1016/j.autneu.2006.07.003
Blix, A. S., and Folkow, B. (1983). “Cardiovascular adjustments to diving in mammals and birds,” in Handbook of Physiology - The Cardiovascular System, eds J. T. Sheperd and F. M. Abboud, (Bethesda, MD: American Physiological Society), 917–945.
Blix, A. S., Walløe, L., Messelt, E. B., and Folkow, L. (2010). Selective brain cooling and its vascular basis in diving seals. J. Exp. Biol. 213, 2610–2613.
Brown, C. M., Sanya, E. O., and Hilz, M. J. (2003). Effect of cold face stimulation on cerebral blood flow in humans. Brain Res. Bull. 61, 81–86. doi: 10.1016/s0361-9230(03)00065-0
Burke, P. G. R., Neale, J. K., and McMullan, S. G. (2011). Patterning of somatosympathetic reflexes reveals nonuniform organization of presympathetic drive from C1 and non-C1 RVLM neurons. Am. J. Physiol. 301, R1112–R1122.
Burns, J. M., and Castellini, M. A. (1996). Physiological and behavioral determinants of the aerobic dive limit in Weddell seal (Leptonychotes weddellii) Pups. J. Comp. Physiol. 166, 473–483. doi: 10.1007/bf02338290
Butler, P. J., and Jones, D. R. (1982). The comparative physiology of diving in vertebrates. Adv. Comp. Physiol. Biochem. 8:179. doi: 10.1016/b978-0-12-011508-2.50012-5
Butler, P. J., and Jones, D. R. (1997). Physiology of diving of birds and mammals. Physiol. Rev. 77, 837–899. doi: 10.1152/physrev.1997.77.3.837
Byku, M., Gan, Q., and Panneton, W. M. (2004). A physiological and neuroanatomical comparison of rats after either voluntary or forced dives. FASEB J. 18:A1102.
Cabanac, A., Folkow, L. P., and Blix, A. S. (1997). Volume capacity and contraction control of the seal spleen. J. Appl. Physiol. 82, 1989–1994. doi: 10.1152/jappl.1997.82.6.1989
Cabanac, A., Folkow, L. P., and Blix, A. S. (1998). Effects of adrenergic and cholinergic drugs on splenic arteries and veins from hooded seals (Cystophora cristata). Comp. Biochem. Physiol. 120, 277–281. doi: 10.1016/s1095-6433(98)00029-4
Cabanac, A. J. (2000). Blood volume in hooded seals: implications for diving capacity. Can. J. Zool. 78, 1293–1299. doi: 10.1139/z00-077
Cain, W., and Murphy, C. L. (1980). Interaction between chemoreceptive modalities of odour and irritation. Nature 284, 255–257. doi: 10.1038/284255a0
Carrive, P. (2000). Conditioned fear to environmental context: cardiovascular and behavioral components in the rat. Brain Res. 858, 440–445. doi: 10.1016/s0006-8993(00)02029-1
Caspers, C., Cleveland, S., and Schipke, J. D. (2011). Diving reflex: can the time course of heart rate reduction be quantified? Scand. J. Med. Sci. Sports 21, 18–31. doi: 10.1111/j.1600-0838.2010.01246.x
Casson, D. M., and Ronald, K. (1975). The harp seal, Pagophilus groenlandicus. XIV. Cardiac arrhthmias. Comp. Biochem. Physiol. A 50, 307–314. doi: 10.1016/0300-9629(75)90018-3
Cauna, N., Hinderer, K. H., and Wentges, R. T. (1969). Sensory receptor organs of the human nasal respiratory mucosa. Am. J. Anat. 124, 187–210.
Chamberlin, N. L. (2004). Functional organization of the parabrachial complex and intertrigeminal region in the control of breathing. Respir. Physiol. Neurobiol. 143, 115–125. doi: 10.1016/j.resp.2004.03.015
Chamberlin, N. L., and Saper, C. B. (1998). A brainstem network mediating apneic reflexes in the rat. J. Neurosci. 18, 6048–6056. doi: 10.1523/jneurosci.18-15-06048.1998
Chiluwal, A., Narayan, R. K., Chaung, W., Mehan, N., Wang, P., Bouton, C. E., et al. (2017). Neuroprotective effects of trigeminal nerve stimulation in severe traumatic brain injury. Sci. Rep. 7:6792.
Chotiyanonta, J. S., Dinovo, K. M., and McCulloch, P. F. (2013). Bilateral sectioning of the anterior ethmoidal nerves does not eliminate the diving response in voluntarily diving rats. Physiol. Rep. 1:e00141. doi: 10.1002/phy2.141
Cometto-Muñiz, J. E., and Cain, W. S. (1997). Agonistic sensory effects of airborne chemicals in mixtures: odor, nasal pungency, and eye irritation. Percept. Psychophys. 59, 665–674. doi: 10.3758/bf03206014
Cometto-Muniz, J. E., Cain, W. S., and Abraham, M. H. (1998). Nasal pungency and odor of homologous aldehydes and carbosylic acids. Exp. Brain Res. 118, 180–188. doi: 10.1007/s002210050270
Cometto-Muniz, J. E., Cain, W. S., Abraham, M. H., and Gola, J. M. R. (2001). Ocular and nasal trigeminal detection of butyl acetate and toluene presented singly and in mixtures. Toxicol. Sci. 63, 233–244. doi: 10.1093/toxsci/63.2.233
Corbett, E. K. A., Saha, S., Deuchars, J., McWilliam, P. N., and Batten, T. F. C. (2003). Ionotropic glutamate receptor subunit immunoreactivity of vagal preganglionic neurones projecting to the rat heart. Auton. Neurosci. 105, 105–117. doi: 10.1016/s1566-0702(03)00047-x
Cui, Y., Kam, K., Sherman, D., Janczewski, W. A., Zheng, Y., and Feldman, J. L. (2016). Defining preBotzinger complex rhythm- and pattern-generating neural microcircuits in vivo. Neuron 91, 602–614. doi: 10.1016/j.neuron.2016.07.003
Davis, R. W. (2014). A review of the multi-level adaptations for maximizing aerobic dive duration in marine mammals: from biochemistry to behavior. J. Comp. Physiol. B 184, 23–53. doi: 10.1007/s00360-013-0782-z
Davis, R. W., Polasek, L., Watson, R., Fuson, A., Williams, T. M., and Kanatous, S. B. (2004). The diving paradox: new insights into the role of the dive response in air-breathing vertebrates. Comp. Biochem. Physiol. A Mol. Integr. Physiol. 138, 263–268. doi: 10.1016/j.cbpb.2004.05.003
Davis, R. W., and Williams, T. M. (2012). The marine mammal dive response is exercise modulated to maximize aerobic dive duration. J. Comp. Physiol. A 198, 583–591. doi: 10.1007/s00359-012-0731-4
de Burgh Daly, M. (1984). “. Breath-hold diving: mechanisms of cardiovascular adjustments in the mammal,” in Recent Advance in Physiology, ed. P. F. Baker, (Edinburgh: Churchill Livingstone), 201–245.
Dean, J. B., Ballantyne, D., Cardone, D. L., Erlichman, J. S., and Solomon, I. C. (2002). Role of gap junctions in CO2 chemoreception and respiratory control. Am. J. Physiol. 283, L665–L670.
Doyle, R. E., Panneton, W. M., Vogler, G. A., Romeo, J. P., Watson, B. J., and Higgins, B. (1988). The muskrat in biomedical research. J. Lab. Anim. Sci. 38, 667–674.
Drummond, P. C., and Jones, D. R. (1979). The initiation and maintenance of bradycardia in a diving mammal, the muskrat Ondatra zibethica. J. Physiol. 290, 253–271. doi: 10.1113/jphysiol.1979.sp012770
Dutschmann, M., Guthmann, A., and Herbert, H. (1998). NMDA receptor subunit NR1-immunoreactivity in the rat pons and brainstem and colocalization with Fos induced by nasal stimulation. Brain Res. 809, 221–230. doi: 10.1016/s0006-8993(98)00885-3
Dutschmann, M., and Herbert, H. (1996). The Kölliker-Fuse nucleus mediates the trigeminally induced apnoea in the rat. Neuroreport 7, 1432–1436. doi: 10.1097/00001756-199605310-00022
Dutschmann, M., and Herbert, H. (1997). Fos expression in the rat parabrachial and Kölliker-Fuse nuclei after electrical stimulation of the trigeminal ethmoidal nerve and water stimulation of the nasal mucosa. Exp. Brain Res. 117, 97–110. doi: 10.1007/s002210050203
Dutschmann, M., and Herbert, H. (1998a). NMDA and GABAA receptors in the rat Kolliker-Fuse area control cardiorespiratory responses evoked by trigeminal ethmoidal nerve stimulation. J. Physiol. 510, 793–804. doi: 10.1111/j.1469-7793.1998.793bj.x
Dutschmann, M., and Herbert, H. (1998b). The medial nucleus of the solitary tract mediates the trigeminally evoked pressor response. Neuroreport 9, 1053–1057.
Dutschmann, M., and Herbert, H. (1999). Pontine cholinergic mechanisms enhance trigeminally evoked respiratory suppression in the anesthetized rat. J. Appl. Physiol. 87, 1059–1065. doi: 10.1152/jappl.1999.87.3.1059
Dutschmann, M., Morschel, M., Kron, M., and Herbert, H. (2004). Development of adaptive behaviour of the respiratory network: implications for the pontine Kolliker-Fuse nucleus. Respir. Physiol. Neurobiol. 143, 155–165. doi: 10.1016/j.resp.2004.04.015
Dutschmann, M., and Paton, J. F. R. (2002). Influence of nasotrigeminal afferents on medullary respiratory neurones and upper airway patency in the rat. Pflugers Arch. 444, 227–235. doi: 10.1007/s00424-002-0797-x
Dykes, R. W. (1974). Factors related to the dive reflex in harbor seals: sensory contributions from the trigeminal region. Can. J. Physiol. Pharmacol. 52, 259–265. doi: 10.1139/y74-035
Elliott, N. M., Andrews, R. D., and Jones, D. R. (2002). Pharmacological blockade of the dive response: effects on heart rate and diving behaviour in the harbour seal (Phoca vitulina). J. Exp. Biol. 205, 3757–3765.
Elmegaard, S. L., Johnson, M., Madsen, P. T., and McDonald, B. (2016). Cognitive control of heart rate in diving harbor porpoises. Curr. Biol. 26, R1175–R1176.
Elsner, R., Angell-James, J. E., and de Burgh Daly, M. (1977). Carotid body chemoreceptor reflexes and their interactions in the seal. Am. J. Physiol. 232, H517–H525.
Elsner, R., and Gooden, B. (1983). Diving and Asphyxia: A Comparative Study Of Animals And Man. New York, NY: Cambridge University Press.
Eriksen, N., and Pakkenberg, B. (2007). Total neocortical cell number in the mysticete brain. Anat. Rec. 290, 83–95. doi: 10.1002/ar.20404
Fabrizius, A., Hoff, M. L., Engler, G., Folkow, L. P., and Burmester, T. (2016). When the brain goes diving: transcriptome analysis reveals a reduced aerobic energy metabolism and increased stress proteins in the seal brain. BMC Genom. 17:583.
Fahlman, A., Bostrom, B. L., Dillon, K. H., and Jones, D. R. (2011). The genetic component of the forced diving bradycardia response in mammals. Front. Physiol. 2:63. doi: 10.3389/fphys.2011.00063
Farrell, A. P. (2007). Tribute to P. L. Lutz: a message from the heart–why hypoxic bradycardia in fishes? J. Exp. Biol. 210, 1715–1725. doi: 10.1242/jeb.02781
Feldman, J. L. (1986). “Neurophysiology of breathing in mammals,” in Handbook of Physiology The Nervous System: Intrinsic Regulatory Systems Of The Brain, ed. F. E. Bloom, (Bethesda, MD: American Physiology Society), 463–524.
Feldman, J. L., Del Negro, C. A., and Gray, P. A. (2013). Understanding the rhytm of breathing: so near, yet so far. Annu. Rev. Physiol. 75:120.
Ferretti, G. (2001). Extreme human breath-hold diving. Eur. J. Appl. Physiol. 84, 254–271. doi: 10.1007/s004210000377
Ferrigno, M., Ferretti, G., Ellis, A., Warkander, D., Costa, M., Cerretelli, P., et al. (1997). Cardiovascular changes during deep breath-hold dives in a pressure chamber. J. Appl. Physiol. 83, 1282–1290. doi: 10.1152/jappl.1997.83.4.1282
Finger, T. E., Boettger, B., Hansen, A., Anderson, K. T., Alimohammadi, H., and Silver, W. L. (2003). Solitary chemoreceptor cells in the nasal cavity serve as sentinels of respiration. Proc. Natl. Acad. Sci. U.S.A. 100, 8981–8986. doi: 10.1073/pnas.1531172100
Finger, T. E., St. Jeor, V. L., Kinnamon, J. C., and Silver, W. L. (1990). Ultrastructure of substance P- and CGRP-Immunoreactive nerve fibers in the nasal epithelium of rodents. J. Comp. Neurol. 294, 293–305. doi: 10.1002/cne.902940212
Foster, G. E., and Sheel, A. W. (2005). The human diving response, its function, and its control. Scand. J. Med. Sci. Sports 15, 3–12. doi: 10.1111/j.1600-0838.2005.00440.x
Gabrielsen, G., Kanwisher, J., and Steen, J. B. (1977). “Emotional” bradycardia: a telemetry study on incubating willow grouse (Lagopus laopus). Acta Physiol. Scand. 100, 255–257. doi: 10.1111/j.1748-1716.1977.tb05944.x
Gandevia, S. C., McCloskey, D. I., and Potter, E. K. (1978). Reflex bradycardia occurring in response to diving, nasopharyngeal stimulation and ocular pressure, and its modification by respiration and swallowing. J. Physiol. 276, 383–394. doi: 10.1113/jphysiol.1978.sp012241
Gieroba, Z. J., Yu, Y.-H., and Blessing, W. W. (1994). Vasoconstriction induced by inhalation of irritant vapour is associated with appearance of Fos protein in C1 catecholamine neurons in rabbit medulla oblongata. Brain Res. 636, 157–161. doi: 10.1016/0006-8993(94)90192-9
Giesbrecht, G. G. (2000). Cold stress, near drowning and accidental hypothermia: a review. Aviat. Space Environ. Med. 71, 733–752.
Goksör, E., Rosengren, L., and Wennergren, G. (2002). Bradycardic response during submersion in infant swimming. Acta Paediatr. 91, 307–312. doi: 10.1111/j.1651-2227.2002.tb01720.x
Golden, F. S., Tipton, M. J., and Scott, R. C. (1997). Immersion, near-drowning and drowning. Br. J. Anaesth. 79, 214–225. doi: 10.1093/bja/79.2.214
Goldwater, P. N. (2017). Infection: the neglected paradigm in SIDS research. Arch. Dis. Child 102, 767–772. doi: 10.1136/archdischild-2016-312327
Golonov, E. V., Shiflett, J. M., and Britz, G. W. (2016). Diving response in rats: role of the subthalamic vasodilator Area. Front. Neurol. 10:00157. doi: 10.3389/fphys.2011.000157
Gooden, B. A. (1982). The diving response in clinical medicine. Aviat. Space Environ. Med. 53, 273–276.
Gorini, C., Jameson, H. S., and Mendelowitz, D. (2009). Serotonergic modulation of the trigeminocardiac reflex neurotransmission to cardiac vagal neurons in the nucleus ambiguus. J. Neurophysiol. 102, 1443–1450. doi: 10.1152/jn.00287.2009
Gorini, C., Philbin, K., Bateman, R., and Mendelowitz, D. (2010). Endogenous inhibition of the trigeminally evoked neurotransmission to cardiac vagal neurons by muscarinic acetylcholine receptors. J. Neurophysiol. 104, 1841–1848. doi: 10.1152/jn.00442.2010
Green, B. G. (2012). Chemesthesis and the chemical senses as components of a “chemofensor complex”. Chem. Sen. 37, 201–206. doi: 10.1093/chemse/bjr119
Green, B. G., and Lawless, H. T. (1991). “The psychophysics of somatosensory chemoreception in the nose and mouth,” in Smell and Taste in Health and Disease, eds T. V. Getchell, R. L. Dory, L. M. Bartoshuk, and J. B. Snow, (New York, NY: Raven Press).
Guntheroth, W. G. (1989). Interleukin-1 as intermediary causing prolonged sleep apnea and SIDS during respiratory infections. Med. Hypotheses 28, 121–123. doi: 10.1016/0306-9877(89)90025-x
Guyenet, P. G. (1990). “Role of the ventrolateteral medulla oblongata in blood pressure regulation,” in Central Regulation of Autonomic Function, eds A. D. Loewy and K. M. Spyer, (New York, NY: Oxford University Press), 145–167.
Guyton, G. P., Stanek, K. S., Schneider, R. C., Hochachka, P. W., Hurford, W. E., Zapol, D. G., et al. (1995). Myoglobin saturation in free-diving Weddell seals. J. Appl. Physiol. 79, 1148–1155. doi: 10.1152/jappl.1995.79.4.1148
Hagen, J. B. (2018). The diving reflex and asphyxia: working across species in physiological ecology. Hist. Philos. Life Sci. 40:18.
Handwerker, H. O., Kilo, S., and Reeh, P. W. (1991). Unresponsive afferent nerve fibres in the sural nerve of the rat. J. Physiol. 435, 229–242. doi: 10.1113/jphysiol.1991.sp018507
Handwerker, H. O., and Kobal, G. (1993). Psychophysiology of experimentally induced pain. Physiol. Rev. 73, 639–671. doi: 10.1152/physrev.1993.73.3.639
Harrison, L. M., Morris, J. A., Telford, D. R., Brown, S. M., and Jones, K. (1999). The nasopharyngeal bacterial flora in infancy: effects of age, gender, season, viral upper respiratory tract infection and sleeping position. FEMS Immunol. Med. Microbiol. 25, 19–28. doi: 10.1111/j.1574-695x.1999.tb01323.x
Hayward, J. S., Hay, C., Matthews, B. R., Overweel, C. H., and Radford, D. D. (1984). Temperature effect on the human dive response in relation to cold water near-drowning. J. Appl. Physiol. 56, 202–206. doi: 10.1152/jappl.1984.56.1.202
Hiebert, S. M., and Burch, E. (2003). Simulated human diving and heart rate: making the most of the diving response as a laboratory exercise. Adv. Physiol. Ed. 27, 130–145. doi: 10.1152/advan.00045.2002
Hill, R. D., Schneider, R. C., Liggins, G. C., Schuette, A. H., Elliott, R. L., Guppy, M., et al. (1987). Heart rate and body temperature during free diving of Weddell seals. Am. J. Physiol. 253, R344–R351.
Ho, C. Y., and Kou, Y. R. (2000). Protective and defensive airway reflexes evoked by nasal exposure to wood smoke in anesthetized rats. J. Appl. Physiol. 88, 863–870. doi: 10.1152/jappl.2000.88.3.863
Ho, C. Y., and Kou, Y. R. (2002). Mechanisms of wood smoke-induced increases in nasal airway resistance and reactivity in rats. Eur. J. Pharmacol. 436, 127–134. doi: 10.1016/s0014-2999(01)01608-9
Hochachka, P. W. (1997). Is evolutionary physiology useful to mechanistic physiology? The diving response in pinnipeds as a test case. Zool. Anal. Complex Syst. 100, 328–335.
Hof, P. R., Chanis, R., and Marino, L. (2005). Cortical complexity in cetacean brains. Anat. Rec. A Discov. Mol. Cell Evol. Biol. 287, 1142–1152. doi: 10.1002/ar.a.20258
Hof, P. R., and Van der Gucht, E. (2007). Structure of the cerebral cortex of the humpback whale, Megaptera novaeangliae (Cetacea, Mysticeti, Balaenopteridae). Anat. Rec. 290, 1–31. doi: 10.1002/ar.20407
Hoff, M. L., Fabrizius, A., Czech-Damal, N. U., Folkow, L. P., and Burmester, T. (2017). Transcriptome analysis identifies key metabolic changes in the hooded seal (Cystophora cristata) brain in response to hypoxia and reoxygenation. PLoS One 12:e0169366. doi: 10.1371/journal.pone.0169366
Hollandsworth, M. P., DiNovo, K. M., and McCulloch, P. F. (2009). Unmyelinated fibers of the anterior nerve of the rat co-localize with neurons in the medullary dorsal horn and ventrolateral medulla activated by nasal stimulation. Brain Res. 1298, 131–144. doi: 10.1016/j.brainres.2009.08.077
Houser, D. S. (2018). “Overview of diving responses,” in CRC Handbook of Marine Mammal Medicie, eds F. M. D. Gulland, J. Dierauf, A. Leslie, and L. Karyl, (Boca Raton: CRC Press), 79–89.
Huang, T. F., Peng, Y., and Huang, L. L. (1991). The effect of microinjection of amino acids into the nucleus tractus solitarius on the diving bradycardia in the rat. Chin. J. Physiol. 34, 167–177.
Huang, Z. G., Wang, X., Evans, C., Gold, A., Bouairi, E., and Mendelowitz, D. (2004). Prenatal nicotine exposure alters the types of nicotinic receptors that facilitate excitatory inputs to cardiac vagal neurons. J. Neurophysiol. 92, 2548–2554. doi: 10.1152/jn.00500.2004
Hult, E. M., Bingaman, M. J., and Swoap, S. J. (2019). A robust diving response in the laboratory mouse. J. Compar. Physiol. B Biochem. Syst. Environ. Physiol. 189, 685–692. doi: 10.1007/s00360-019-01237-5
Hummel, T., Mohammadian, P., Marchl, R., Kobal, G., and Loetsch, J. (2003). Pain in the trigeminal system: irritation of the nasal mucosa using short- and long-lasting stimuli. Int. J. Psychophysiol. 47, 147–158. doi: 10.1016/s0167-8760(02)00150-2
Ichikawa, H., Mitani, S., Hijiya, H., Nakago, T., Jacobowitz, D. M., and Sugimoto, T. (1993). Calretinin-immunoreactivity in trigeminal neurons innervating the nasal mucosa of the rat. Brain Res. 629, 231–238. doi: 10.1016/0006-8993(93)91325-m
Ilardo, M. A., Moltke, I., Korneliussen, T. S., Cheng, J., Stern, A. J., and Racimo, F. (2018). Physiological and genetic adaptations to diving in sea nomads. Cell 173, 569–580.
Irnaten, M., Aicher, S. A., Wang, J., Venkatesan, P., Evans, C., Baxi, S., et al. (2003). Mu-opioid receptors are located postsynaptically and endomorphin-1 inhibits voltage-gated calcium currents in premotor cardiac parasympathetic neurons in the rat nucleus ambiguus. Neuroscience 116, 573–582. doi: 10.1016/s0306-4522(02)00657-7
Irving, L. (1938). Changes in the blood flow through the brain and muscles during the arrest of breathing. Am J Physiol 122, 207–214. doi: 10.1152/ajplegacy.1938.122.1.207
Irving, L. (1939). Respiration in diving mammals. Physiol. Rev. 19, 112–134. doi: 10.1152/physrev.1939.19.1.112
Irving, L., Scholander, P. F., and Grinnell, S. W. (1942). The regulation of arterial blood pressure in the seal during diving. Am. J. Physiol. 135, 557–566. doi: 10.1152/ajplegacy.1942.135.3.557
Izzo, P. N., Deuchars, J., and Spyer, K. M. (1993). Localization of cardiac vagal preganglionic motoneurons in the rat: immunocytochemical evidence of synaptic inputs containing 5-hydroxytryptamine. J. Comp. Neurol. 327, 572–583. doi: 10.1002/cne.903270408
Jobsis, P. D., Ponganis, P. J., and Kooyman, G. L. (2001). Effects of training on forced submersion responses in harbor seals. J. Exp. Biol. 204, 3877–3885.
Johansen, K., Lenfant, C., and Grigg, G. C. (1966). Respiratory properties of blood and responses to diving of the platypus, Ornithorhynchus anatinus (Shaw). Comp. Biochem. Physiol. 18, 597–608. doi: 10.1016/0010-406x(66)90243-x
Jones, C. L., Minati, L., Nagai, Y., Medford, N., Harrison, N. A., Gray, M., et al. (2015). Neuroanatomical substrates for the volitional regulation of heart rate. Front. Psychol. 6:300. doi: 10.3389/fphys.2011.00300
Jones, D. R., Furilla, R. A., Heieis, M. R., Cabbott, A., and Smith, F. M. (1982). The effect of the stress of forcible submergence on the diving response in muskrats (Ondatra zibethica). Can. J. Zool. 60, 187–193. doi: 10.1139/z82-026
Kaczmarek, J., Reichmuth, C., McDonald, B. I., Kristensen, J. H., Larson, J., Johansson, F., et al. (2018). Drivers of the dive response in pinnipeds; apnea, submergence or temperature? J. Exp. Biol. 221:jeb176545. doi: 10.1242/jeb.176545
Kanamaru, A., Mutoh, T., Kojima, K., Nishimura, R., Sasaki, N., Kuwahara, M., et al. (1999). The posterior nasal nerve plays an important role on cardiopulmonary reflexes to nasal application of capsaicin, distilled water and l-menthol in anesthetized dogs. J. Vet. Med. Sci. 61, 85–88. doi: 10.1292/jvms.61.85
Kanamaru, A., Mutoh, T., Nishimura, R., Sasaki, N., Kuwahara, M., and Tsubone, H. (2001). Respiratory and cardiovascular reflexes elicited by nasal instillation of capsaicin to anesthetized, spontaneously breathing dogs. J. Vet. Med. Sci. 63, 439–443. doi: 10.1292/jvms.63.439
Kemp, J. S. (1996). Rebreathing of exhaled gases: importance as a mechanism for the causal association between prone sleep and sudden infant death syndrome. Sleep 19, S263–S266.
Kjeld, T., Pott, F. C., and Secher, N. H. (2009). Facial immersion in cold water enhances cerebral blood velocity during breath-hold exercise in humans. J. Appl. Physiol. 106, 1243–1248. doi: 10.1152/japplphysiol.90370.2008
Kobayashi, M., Cheng, Z. B., and Nosaka, S. (1999). Inhibition of baroreflex vagal bradycardia by nasal stimulation in rats. Am. J. Physiol. 276, H176–H184.
Kooyman, G. L. (1985). Physiology without restraint in diving mammals. Mar. Mammal. Sci. 1, 166–178. doi: 10.1111/j.1748-7692.1985.tb00004.x
Kooyman, G. L., and Campbell, W. B. (1972). Heart rates in freely diving Weddell seals. Comp. Biochem. Physiol. 43A, 31–36. doi: 10.1016/0300-9629(72)90465-3
Kooyman, G. L., Castellini, M. A., and Davis, R. W. (1981). Physiology of diving in marine mammals. Annu. Rev. Physiol. 43, 343–356.
Kooyman, G. L., and Ponganis, P. J. (1998). The physiological basis of diving to depth: birds and mammals. Ann. Rev. Physiol. 60, 19–32. doi: 10.1146/annurev.physiol.60.1.19
Kooyman, G. L., Wahrenbrock, E. A., Castellini, M. A., Davis, R. W., and Sinnett, E. E. (1980). Aerobic and anaerobic metabolism during diving in Weddell seals: evidence of preferred pathways from blood chemistry and behavior. J. Comp. Physiol. 138, 335–346. doi: 10.1007/bf00691568
Koppányi, T., and Dooley, M. S. (1929). Submergence and postural apnea in the muskrat.. J. Comp. Physiol. 138, 335–346. doi: 10.1007/bf00691568
Kratschmer, F. (2001). On reflexes from the nasal mucous membrane on respiration and circulation. Am. J. Physiol. 88, 592–595. doi: 10.1016/s0034-5687(01)00234-1
Kumada, M., Dampney, R. A. L., and Reis, D. J. (1975). The trigeminal depressor response: a cardiovascular reflex originating from the trigeminal system. Brain Res. 92, 485–489. doi: 10.1016/0006-8993(75)90335-2
Kumada, M., Dampney, R. A. L., and Reis, D. J. (1977). The trigeminal depressor response: a novel vasodepressor response originating from the trigeminal system. Brain Res. 119, 305–326. doi: 10.1016/0006-8993(77)90313-4
Lee, L.-Y., Morton, R. F., and Kou, Y. R. (1990). Acute effects of cigarette smoke on breathing in rats: vagal and nonvagal mechanisms. J. Appl. Physiol. 68, 955–961. doi: 10.1152/jappl.1990.68.3.955
Lee, L. Y., and Widdicombe, J. G. (2001). Modulation of airway sensitivity to inhaled irritants: role of inflammatory mediators. Environ. Health Perspect. 109, 585–589. doi: 10.1289/ehp.01109s4585
Leiter, J. C., and Böhm, I. (2007). Mechanisms of pathogenesis in the sudden infant death syndrome. Respir. Physiol. Neurobiol. 159, 127–138. doi: 10.1016/j.resp.2007.05.014
Lin, Y. C. (1974). Autonomic nervous control of cardiovascular response during diving in the rat. Am. J. Physiol. 227, 601–605. doi: 10.1152/ajplegacy.1974.227.3.601
Lin, Y. C., and Baker, D. G. (1975). Cardiac output and its distribution during diving in the rat. Am. J. Physiol. 228, 733–737. doi: 10.1152/ajplegacy.1975.228.3.733
Lindgren, C., and Grogaard, J. B. (1996). Reflex apnoea response and inflammatory mediators in infants with respiratory tract infection. Science 85, 798–803. doi: 10.1111/j.1651-2227.1996.tb14154.x
Lindholm, P., and Lundgren, C. E. (2009). The physiology and pathophysiology of human breath-hold diving. J. Appl. Physiol. 106, 284–292. doi: 10.1152/japplphysiol.90991.2008
Llona, I., Ampuero, E., and Eugenin, J. L. (2004). Somatostatin inhibition of fictive respiration is modulated by pH. Brain Res. 1026, 136–142. doi: 10.1016/j.brainres.2004.08.028
Lobban, C. D. (1995). The oxygen-conserving dive reflex re-examined as the principal contributory factor in sudden infant death. Med. Hypoth. 44, 273–277. doi: 10.1016/0306-9877(95)90179-5
Lobban, C. D. R. (1991). The human dive reflex as a primary cause of SIDS: a review of the literature. Med. J. Aust. 155, 561–563. doi: 10.5694/j.1326-5377.1991.tb93898.x
Lu, J., and Bereiter, D. A. (1991). Microinjections of norepinephrine within the superficial laminae of trigeminal Subnucleus caudalis evoke increases in plasma adrenocorticotropin in the rat. Brain Res. 568, 152–158. doi: 10.1016/0006-8993(91)91391-d
Lucier, G. E., and Egizii, R. (1986). Central projections of the ethmoidal nerve of the cat as determined by the horseradish peroxidase tracer technique. J. Comp. Neurol. 247, 123–132. doi: 10.1002/cne.902470108
Lucier, G. E., and Egizii, R. (1989). Characterization of cat nasal afferents and brain stem neurones receiving ethmoidal input. Exp. Neurol. 103, 83–89. doi: 10.1016/0014-4886(89)90189-1
Mangum, C. P., and Hochachka, P. W. (1998). New directions in comparative physiology and biochemistry: mechanisms, adaptations, and evolution. Physiol. Zool. 71, 471–484. doi: 10.1086/515953
Marfurt, C. F. (1981). The central projections of trigeminal primary afferent neurons in the cat as determined by the transganglionic transport of horseradish peroxidase. J. Comp. Neurol. 203, 785–798. doi: 10.1002/cne.902030414
Marfurt, C. F., and Rajchert, D. M. (1991). Trigeminal primary afferent projections to “non-trigeminal” areas of the rat central nervous system. J. Comp. Neurol. 303, 489–511. doi: 10.1002/cne.903030313
Marino, L. (1998). A comparison of encephalization between odontocete cetaceans and anthropoid primates. Brain Behav. Evol. 51, 230–238. doi: 10.1159/000006540
Marino, L., Murphy, T. L., Deweerd, A. L., Morris, J. A., Fobbs, A. J., Humblot, N., et al. (2001). Anatomy and three-dimensional reconstructions of the brain of the white whale (Delphinapterus leucas) from magnetic resonance images. Anat. Rec. 262, 429–439. doi: 10.1002/ar.1051
Marino, L., Rilling, J. K., Lin, S. K., and Ridgway, S. H. (2000). Relative volume of the cerebellum in dolphins and comparison with anthropoid primates. Brain Behav. Evol. 56, 204–211. doi: 10.1159/000047205
Marino, L., Subheimer, K., Mclellan, W. A., and Johnson, J. I. (2004). Neuroanatomical structure of the spinner dolphin (stenella longirostris orientalis) brain from magnetic resonance images. Anat. Record Part A 279A, 601–610. doi: 10.1002/ar.a.20047
Martin, G. F., Beattie, M. S., Hughes, H. C., Linauts, M., and Panneton, M. (1977). The organization of reticulo-olivo-cerebellar circuits in the North American opossum. Brain Res. 137, 253–266. doi: 10.1016/0006-8993(77)90337-7
Martner, J., Wadenvik, H., and Lisander, B. (1977). Apnoea and bradycardia from submersion in “chronically” decerebrated cats. Acta Physiol. Scand. 101, 476–480. doi: 10.1111/j.1748-1716.1977.tb06031.x
Matesz, C. (1983). Termination areas of primary afferent fibers of the trigeminal nerve in the rat. Acta Biol. Hung. 34, 31–44.
Matsuda, H., Kusakabe, T., Hayashida, Y., Furukawa, M., Kawakami, T., Takenaka, T., et al. (1998). Substance P- and calcitonin gene-related peptide-containing nerve fibers in the nasal mucosa of chronically hypoxic rats. Brain Res. Bull. 45, 563–569. doi: 10.1016/s0361-9230(97)00450-4
Matsuda, H., Tsukuda, M., Kadota, T., Kusunoki, T., and Kishida, R. (1994). Coexistence of galanin and substance P in the mouse nasal mucosa, including the vomeronasal organ. Neurosci. Lett. 173, 55–58. doi: 10.1016/0304-3940(94)90148-1
Matturri, L., Ottaviani, G., and Lavezzi, A. M. (2005). Sudden infant death triggered by dive reflex. J. Clin. Pathol. 58, 77–80. doi: 10.1136/jcp.2004.020867
McAllen, R. M., Salo, L. M., Paton, J. F. R., and Picikering, A. E. (2011). Processing of central and reflex vagal drives by rat cardiac ganglion neurons: an intracellular analysis. J. Physiol. 589, 5801–5818. doi: 10.1113/jphysiol.2011.214320
McCulloch, P. F. (2005). Activation of the trigeminal medullary dorsal horn during voluntary diving in rats. Brain Res. 1051, 194–198. doi: 10.1016/j.brainres.2005.05.059
McCulloch, P. F. (2012). Animal models for investigating the central control of the mammalian diving response. Front. Physiol. 3:169. doi: 10.3389/fphys.2011.00169
McCulloch, P. F. (2014). Training rats to voluntarily dive underwater: investigations of the mammalian diving response. J. Visual. Exp. 12:e52093.
McCulloch, P. F., and DiNovo, K. M. (2018). Restoration of the nasopharyngeal response after bilateral sectioning of the anterior ethmoidal nerve in the rat. Physiol. Rep. 6:e13830. doi: 10.14814/phy2.13830
McCulloch, P. F., Dinovo, K. M., and Connolly, T. M. (2010). The cardiovascular and endocrine responses to voluntary and forced diving in trained and untrained rats. Am. J. Physiol. 298, R224–R234.
McCulloch, P. F., Faber, K. M., and Panneton, W. M. (1999a). Electrical stimulation of the anterior ethmoidal nerve produces the diving response. Brain Res. 830, 24–31. doi: 10.1016/s0006-8993(99)01374-8
McCulloch, P. F., and Jones, D. R. (1990). Cortical influences on diving bradycardia in muskrats (Ondatra zibethicus). Physiol. Zool. 63, 1098–1117. doi: 10.1086/physzool.63.6.30152635
McCulloch, P. F., Lahrman, K. A., DelPrete, B., and DiNovo, K. M. (2018). Innervation of the nose and nasal region of the rat: implications for initiating the mammalian diving response. Front. Neuroanat. 12:85. doi: 10.3389/fphys.2011.00085
McCulloch, P. F., Ollenberger, G. P., Bekar, L. K., and West, N. H. (1997). Trigeminal and chemoreceptor contributions to bradycardia during voluntary dives in rats. Am. J. Physiol. 273, R814–R822.
McCulloch, P. F., and Panneton, W. M. (1997). Fos immunohistochemical determination of brainstem neuronal activation in the muskrat after nasal stimulation. Neuroscience 78, 913–925. doi: 10.1016/s0306-4522(96)00633-1
McCulloch, P. F., and Panneton, W. M. (2003). Activation of brainstem catecholaminergic neurons during voluntary diving in rats. Brain Res. 984, 42–53. doi: 10.1016/s0006-8993(03)03051-8
McCulloch, P. F., Panneton, W. M., and Guyenet, P. G. (1999b). The rostral ventrolateral medulla mediates the sympathoactivation produced by chemical stimulation of the nasal mucosa. J. Physiol. 516, 471–484. doi: 10.1111/j.1469-7793.1999.0471v.x
McCulloch, P. F., Warren, E. A., and DiNovo, K. M. (2016). Repetitive diving in trained rats still increases Fos production in brainstem neurons after bilateral sectioning of the anterior ethmoidal nerve. Front. Physiol. 7:148. doi: 10.3389/fphys.2011.00148
McCulloch, P. F., and West, N. H. (1992). Cardiovascular responses to nasal water flow in rats are unaffected by chemoreceptor drive. Am. J. Physiol. 263, R1049–R1056.
McDonald, B. I., Johnson, M., and Madsen, P. T. (2018). Dive heart rate in harbour porpoises is influenced by exercise and expectations. J. Exp. Biol. 221:jeb168740. doi: 10.1242/jeb.168740
McDonald, B. I., and Ponganis, P. J. (2014). Deep-diving sea lions exhibit extreme bradycardia in long-duration dives. J. Exp. Biol. 217, 1525–1534. doi: 10.1242/jeb.098558
McKeegan, D. E. F., Demmers, T. G. M., Wathes, C. M., Jones, R. B., and Gentle, M. J. (2002). Response characteristics of nasal trigeminal nociceptors in Gallus domesticus. Neuroreport 13, 1033–1035. doi: 10.1097/00001756-200206120-00011
McKnight, J. C., Bennett, K. A., Bronkhorst, M., Russell, D. J. F., Balfour, S., Milne, R., et al. (2019). Shining new light on mammalian diving physiology using wearable near-infrared spectroscopy. PLoS Biol. 17:e3000306. doi: 10.1371/journal.pone.03000306
McRitchie, R. J., and White, S. W. (1974). Role of trigeminal olfactory, carotid sinus and aortic nerves in the respiratory and circulatory response to nasal inhalation of cigarette smoke and other irritants in the rabbit. Aust. J. Exp. Biol. Med. Sci. 52, 127–140. doi: 10.1038/icb.1974.10
Mendelowitz, D. (1998). Nicotine excites cardiac vagal neurons via three sites of action. Clin. Exp. Pharmacol. Physiol. 25, 453–456. doi: 10.1111/j.1440-1681.1998.tb02233.x
Mitchell, E. A. (2009). What is the mechanism of SIDS? Clues from epidemiology. Dev. Psychobiol. 51, 215–222. doi: 10.1002/dev.20369
Molony, N., Blackwell, C. C., and Busuttil, A. (1999). The effect of prone posture on nasal temperature in children in relation to induction of staphylococcal toxins implicated in sudden infant death syndrome. FEMS Immunol. Med. Microbiol. 25, 109–113. doi: 10.1111/j.1574-695x.1999.tb01333.x
Mooney, S., Chin, B., Villiere, S., Nakase, K., Kollmar, R., Kim, S., et al. (2019). Diving responses elicited by nasopharyngeal irrigation mimic seizure-associated central apneic episodes in a rat model. Neurobiol. Dis. 124, 408–415. doi: 10.1016/j.nbd.2018.12.019
Morris, J. A. (1999). The common bacterial toxins hypothesis of sudden infant death syndrome. FEMS Immunol. Med. Microbiol. 25, 11–17. doi: 10.1111/j.1574-695x.1999.tb01322.x
Mottishaw, P. D., Thornton, S. J., and Hochachka, P. W. (1999). The diving response mechanism and its surprising evolutionary path in seals and sea lions. Am. Zool. 39, 434–450. doi: 10.1093/icb/39.2.434
Mutoh, T., Kanamaru, A., Suzuki, H., Tsubone, H., Nishimura, R., and Sasaki, N. (2001). Respiratory reflexes in spontaneously breathing anesthetized dogs in response to nasal administration of sevoflurane, isoflurane, or halothane. Am. J. Vet. Res. 62, 311–319. doi: 10.2460/ajvr.2001.62.311
Mutoh, T., Kanamaru, A., Tsubone, H., Nishimura, R., and Sasaki, N. (2000). Respiratory reflexes in response to nasal administration of halothane to anesthetized, spontaneously breathing dogs. Am. J. Vet. Res. 61, 260–267. doi: 10.2460/ajvr.2000.61.260
Nakamura, T., and Hayashida, Y. (1992). Autonomic cardiovascular responses to smoke exposure in conscious rats. Am. J. Physiol. 262, R738–R745.
Nalivaiko, E., De Pasquale, C. G., and Blessing, W. W. (2003). Electrocardiographic changes associated with the nasopharyngeal reflex in conscious rabbits: vago-sympathetic co-activation. Auton. Neurosci. 105, 101–104. doi: 10.1016/s1566-0702(03)00048-1
Neff, R. A., Humphrey, J., Mihalevich, M., and Mendelowitz, D. (1998). Nicotine enhances presynaptic and postsynaptic glutamatergic neurotransmission to activate cardiac parasympathetic neurons. Circ. Res. 83, 1241–1247. doi: 10.1161/01.res.83.12.1241
Noren, S. R., Kendall, T., Cuccurullo, V., and Williams, T. M. (2012). The dive response redefined: underwater behavior influences cardiac variability in freely diving dolphins. J. Exp. Biol. 215, 2735–2741. doi: 10.1242/jeb.069583
Noren, S. R., and Williams, T. M. (2000). Body size and skeletal muscle myoglobin of cetaceans: adaptations for maximizing dive duration. Comp. Biochem. Physiol. A 126, 181–191. doi: 10.1016/s1095-6433(00)00182-3
Odden, A., Folkow, L. P., Caputa, M., Hotvedt, R., and Blix, A. S. (1999). Brain cooling in diving seals. Acta Physiol. Scand. 166, 77–78. doi: 10.1046/j.1365-201x.1999.00536.x
Olesen, J. (1991). Cerebral and extracranial circulatory disturbances in migraine: pathophysiological implications. Cerebrovasc. Brain Metab. Rev. 3, 1–28.
Ollenberger, G. P., Matte, G., Wilkinson, A. A., and West, N. H. (1998). Relative distribution of blood flow in rats during surface and submerged swimming. Comp. Biochem. Physiol. 119A, 271–277. doi: 10.1016/s1095-6433(97)00427-3
Ollenberger, G. P., and West, N. H. (1998a). Contribution of hypercapnia and trigeminal stimulation to cerebrovascular dilation during simulated diving. Am. J. Physiol. 274, R921–R930.
Ollenberger, G. P., and West, N. H. (1998b). Distribution of regional cerebral blood flow in voluntarily diving rats. J. Exp. Biol. 201, 549–558.
Olsen, C. R., Fanestil, D. D., and Scholander, P. F. (1962). Some effects of breath holding and apneic underwater diving on cardiac rhythm in man. J. Appl. Physiol. 17, 461–466. doi: 10.1152/jappl.1962.17.3.461
Pan, Y., Zhang, H., VanDeripe, D. R., Cruz-Flores, S., and Panneton, W. M. (2007). Heliox and oxygen reduce infarct volume in a rat model of focal ischemia. Exp. Neurol. 205, 587–590. doi: 10.1016/j.expneurol.2007.03.023
Pandit, J. J., Wordsworth, H., Little, E., Formenti, F., and Robbins, P. A. (2014). The peripheral actions of the central neuropeptide somatostatin on control of breathing: effect on metabolic rate and chemoreflex responses in humans. Prog. Brain Res. 209, 331–340. doi: 10.1016/b978-0-444-63274-6.00017-5
Panneton, M., and Watson, B. J. (1991). Stereotaxic atlas of the brainstem of the muskrat, Ondatra zibethicus. Brain Res. Bull. 26, 479–509. doi: 10.1016/0361-9230(91)90087-z
Panneton, W. M. (1990). Controlled bradycardia induced by nasal stimulation in the muskrat, Ondatra zibethicus. J. Auton. Nerv. Syst. 30, 253–264.
Panneton, W. M. (1991a). Primary afferent projections from the upper respiratory tract in the muskrat. J. Comp. Neurol. 308, 51–65. doi: 10.1002/cne.903080106
Panneton, W. M. (1991b). Trigeminal mediation of the diving response in the muskrat. Brain Res. 560, 321–325. doi: 10.1016/0006-8993(91)91251-u
Panneton, W. M. (2013). The mammalian diving response: an enigmatic reflex to preserve life? Physiology 28, 284–297. doi: 10.1152/physiol.00020.2013
Panneton, W. M., Anch, A. M., Panneton, W. M., and Gan, Q. (2014). Parasympathetic preganglionic cardiac motoneurons labeled after voluntary diving. Front. Physiol. 5:8. doi: 10.3389/fphys.2011.0008
Panneton, W. M., and Burton, H. (1981). Corneal and periocular representation within the trigeminal sensory complex in the cat studied with transganglionic transport of horseradish peroxidase. J. Comp. Neurol. 199, 327–344. doi: 10.1002/cne.901990303
Panneton, W. M., and Gan, Q. (2014). Direct reticular projections of trigeminal sensory fibers immunoreactive to CGRP: potential monosynaptic somatoautonomic projections. Front. Neurosci. 8:136. doi: 10.3389/fphys.2011.000136
Panneton, W. M., Gan, Q., and Dahms, T. E. (2010a). Cardiorespiratory and neural consequences of rats brought past their aerobic dive limit. J. Appl. Physiol. 109, 1256–1269. doi: 10.1152/japplphysiol.00110.2010
Panneton, W. M., Hsu, H., and Gan, Q. (2010c). Distinct central representations for sensory fibers innervating either the conjunctiva or cornea of the rat. Exp. Eye Res. 90, 388–396. doi: 10.1016/j.exer.2009.11.018
Panneton, W. M., Gan, Q., and Juric, R. (2006). Brainstem projections from recipient zones of the anterior ethmoidal nerve in the medullary dorsal horn. Neuroscience 141, 889–906. doi: 10.1016/j.neuroscience.2006.04.055
Panneton, W. M., Gan, Q., Le, J., Livergood, R. S., Clerc, P., and Juric, R. (2012a). Activation of brainstem neurons by underwater diving in the rat. Front. Physiol. 3:111. doi: 10.3389/fphys.2012.00111
Panneton, W. M., Gan, Q., and Sun, D. W. (2012b). Persistence of the nasotrigeminal reflex after pontomedullary transection. Respir. Physiol. Neurobiol. 180, 230–236. doi: 10.1016/j.resp.2011.11.012
Panneton, W. M., Gan, Q., and Juric, R. (2010b). The rat: a laboratory model for studies of the diving response. J. Appl. Physiol. 108, 811–820. doi: 10.1152/japplphysiol.00600.2009
Panneton, W. M., Johnson, S. N., and Christensen, N. D. (1994). Trigeminal projections to the peribrachial region in the muskrat. Neuroscience 58, 605–625. doi: 10.1016/0306-4522(94)90085-x
Panneton, W. M., McCulloch, P. F., and Sun, W. (2000). Trigemino-autonomic connections in the muskrat: the neural substrate for the diving response. Brain Res. 874, 48–65. doi: 10.1016/s0006-8993(00)02549-x
Panneton, W. M., McCulloch, P. F., Tan, Y., Tan, Y. X., and Yavari, P. (1996). Brainstem origin of preganglionic cardiac motoneurons in the muskrat. Brain Res. 738, 342–346. doi: 10.1016/s0006-8993(96)01048-7
Panneton, W. M., Pan, B., and Gan, Q. (2017). Somatotopy in the medullary dorsal horn as a basis for orofacial reflex behavior. Front. Neurol. 8:522. doi: 10.3389/fphys.2012.00522
Panneton, W. M., and Yavari, P. (1995). A medullary dorsal horn relay for the cardiorespiratory responses evoked by stimulation of the nasal mucosa in the muskrat, Ondatra zibethicus: evidence for excitatory amino acid transmission. Brain Res. 691, 37–45. doi: 10.1016/0006-8993(95)00597-j
Paton, J. F., Boscan, P., Pickering, A. E., and Nalivaiko, E. (2005). The yin and yang of cardiac autonomic control: vago-sympathetic interactions revisited. Brain Res. Rev. 49, 555–565. doi: 10.1016/j.brainresrev.2005.02.005
Peterson, D. F., Coote, J. H., Gilbey, M. P., and Futuro-Neto, H. A. (1983). Differential pattern of sympathetic outflow during upper airway stimulation with smoke. Am. J. Physiol. 245, R433–R437.
Petersson, G., Malm, L., Ekman, R., and Håkanson, R. (1989). Capsaicin evokes secretion of nasal fluid and depletes substance P and calcitonin gene-related peptide from the nasal mucosa in the rat. Br. J. Pharmacol. 98, 930–936. doi: 10.1111/j.1476-5381.1989.tb14623.x
Ponganis, P. J., and Kooyman, G. L. (2000). Diving physiology of birds: a history of studies on polar species. Comp. Biochem. Physiol. A 126, 143–151. doi: 10.1016/s1095-6433(00)00208-7
Ponganis, P. J., Kooyman, G. L., Winter, L. M., and Starke, L. N. (1997). Heart rate and plasma lactate responses during submerged swimming and trained diving in California sea lions, Zalophus californianus. J. Comp. Physiol. B 167, 9–16. doi: 10.1007/s003600050042
Ponganis, P. J., McDonald, B. I., Tift, M. S., and Williams, C. L. (2017). Heart rate regulation in diving sea lions: the vagus nerve rules. J. Exp. Biol. 220, 1372–1381. doi: 10.1242/jeb.146779
Radulovacki, M., Pavlovic, S., and Carley, D. W. (2004). Pontine intertrigeminal region attenuates sleep apneas in rats. Sleep 27, 383–387. doi: 10.1093/sleep/27.3.383
Radulovacki, M., Pavlovic, S., Saponjic, J., and Carley, D. W. (2003). Intertrigeminal region attenuates reflex apnea and stabilizes respiratory pattern in rats. Brain Res. 975, 66–72. doi: 10.1016/s0006-8993(03)02587-3
Rambaud, C., Guibert, M., Briand, E., Grangeot-Keros, L., Coulomb-L’Hermine, A., and Dehan, M. (1999). Microbiology in sudden infant death syndrome (SIDS) and other childhood deaths. FEMS Immunol. Med. Microbiol. 25, 59–66. doi: 10.1111/j.1574-695x.1999.tb01327.x
Ramirez-Jarquin, J. O., Lara-Hernandez, S., Lopez-Guerrero, J. J., Aguileta, M. A., Rivera-Angulo, A. J., Sampieri, A., et al. (2012). Somatostatin modulates generation of inspiratory rhythms and determines asphyxia survival. Peptides 34, 360–372. doi: 10.1016/j.peptides.2012.02.011
Reeh, P. W., Kocher, L., and Jung, S. (1986). Does neurogenic inflammation alter the sensitivity of unmyelinated nociceptors in the rat? Brain Res. 384, 42–50. doi: 10.1016/0006-8993(86)91217-5
Rekling, J. C., and Feldman, J. L. (1998). Prebötzinger complex and pacemaker neurons: hypothesized site and kernel for respiratory rhythm generation. Ann. Rev. Physiol. 60, 385–405. doi: 10.1146/annurev.physiol.60.1.385
Ridgeway, S. H., Carder, D. A., and Clark, W. (1975). Conditioned bradycardia in the sea lion Zalophus californianus. Nature 256, 37–38. doi: 10.1038/256037a0
Ross, M. H., Romrell, L. J., and Kaye, G. I. (1995). Histology: A Text And Atlas. Baltimore: Williams & Wilkens.
Rozloznik, M., Paton, J. F., and Dutschmann, M. (2009). Repetitive paired stimulation of nasotrigeminal and peripheral chemoreceptor afferents cause progressive potentiation of the diving bradycardia. Am. J. Physiol. Regul. Integr. Comp. Physiol. 296, R80–R87.
Ruggeri, P., Battaglia, A., Ermirio, R., Grossini, E., Molinari, C., Mary, D., et al. (2000). Role of nitric oxide in the control of the heart rate within the nucleus ambiguus of rats. Neuroreport 11, 481–485. doi: 10.1097/00001756-200002280-00011
Ruggiero, D. A., Ross, C. A., Anwar, M., Park, D. H., Joh, T. H., and Reis, D. J. (1985). Distribution of neurons containing phenylethanolamine N-methyltransferase in medulla and hypothalamus of rat. J. Comp. Neurol. 239, 127–154. doi: 10.1002/cne.902390202
Rybka, E. J., and McCulloch, P. F. (2006). The anterior ethmoidal nerve is necessary for the initiation of the nasopharyngeal response in the rat. Brain Res. 1075, 122–132. doi: 10.1016/j.brainres.2005.12.112
Sakai, J., Takahshi, S., and Funayama, M. (2009). Gas dispersal potential of infant bedding of sudden death cases (1): CO2 accumulation around the face of infant mannequin model. Leq. Med. 11, S404–S405.
Schaefer, M. L., Boettger, B., Silver, W. L., and Finger, T. E. (2002). Trigeminal collaterals in the nasal epithelium and olfactory bulb: a potential route for direct modulation of olfactory information by trigeminal stimuli. J. Comp. Neurol. 444, 221–226. doi: 10.1002/cne.10143
Schagatay, E., and Van Kampen, M. (1995). Apneic snout immersion in trained pigs elicits a “diving response”. Adv. Exp. Med. Biol. 393, 73–76. doi: 10.1007/978-1-4615-1933-1_14
Scholander, P. F. (1963). The master switch of life. Sci. Am. 209, 92–106. doi: 10.1038/scientificamerican1263-92
Scholander, P. F., Hammel, H. T., LeMessurier, H., Hemmingsen, E., and Garey, W. (1962). Circulatory adjustment in pearl divers. J. Appl. Physiol. 17, 184–190. doi: 10.1152/jappl.1962.17.2.184
Schreihofer, A. M., and Guyenet, P. G. (1997). Identification of C1 presympathetic neurons in rat rostral ventrolateral medulla by juxtacellular labeling in vivo. J. Comp. Neurol. 387, 524–536. doi: 10.1002/(sici)1096-9861(19971103)387:4<524::aid-cne4>3.0.co;2-4
Segade, L. A. (2003). Trigeminal primary afferent projections to the peribrachial area in the guinea pig. Neurosci. Lett. 351, 63–66. doi: 10.1016/s0304-3940(03)00437-3
Sekizawa, S., and Tsubone, H. (1994). Nasal receptors responding to noxious chemical irritants. Respir. Physiol. 96, 37–48. doi: 10.1016/0034-5687(94)90104-x
Sekizawa, S., Tsubone, H., Kuwahara, M., and Sugano, S. (1996). Nasal receptors responding to cold and l-menthol airflow in the guinea pig. Respir. Physiol. 103, 211–219. doi: 10.1016/0034-5687(95)00091-7
Sekizawa, S., Tsubone, H., Kuwahara, M., and Sugano, S. (1998). Does histamine stimulate trigeminal nasal afferents? Respir. Physiol. 112, 13–22. doi: 10.1016/s0034-5687(98)00009-7
Sekizawa, S. I., and Tsubone, H. (1996). Nasal mechanoreceptors in guinea pigs. Respir. Physiol. 106, 223–230. doi: 10.1016/s0034-5687(96)00085-0
Shattock, M. J., and Tipton, M. J. (2012). ‘Autonomic conflict’: a different way todie during cold water immersion? J. Physiol. 590, 3219–3230. doi: 10.1113/jphysiol.2012.229864
Shigenaga, Y., Chen, I. C., and Suemune, S. (1986). Oral and facial representation within the medullary and upper cervical dorsal horns in the cat. J. Comp. Neurol. 243, 388–408. doi: 10.1002/cne.902430309
Silver, W. L., Farley, L. G., and Finger, T. E. (1991). The effects of neonatal capsaicin administration on trigeminal nerve chemoreceptors in the rat nasal cavity. Brain Res. 561, 212–216. doi: 10.1016/0006-8993(91)91597-t
Silver, W. L., Mason, J. R., Adams, M. A., and Smeraski, C. A. (1986). Nasal trigeminal chemoreception: responses to n-aliphatic alcohols. Brain Res. 376, 221–229. doi: 10.1016/0006-8993(86)90183-6
Silverman, J. D., and Kruger, L. (1989). Calcitonin-gene-related-peptide-immunoreactive innervation of the rat head with emphasis on specialized sensory structures. J. Comp. Neurol. 280, 303–330. doi: 10.1002/cne.902800211
Skinner, M. R., Ramage, A. G., and Jordan, D. (2002). Modulation of reflexly evoked vagal bradycardias by central 5-HT1A receptors in anaesthetized rabbits. Br. J. Pharmacol. 137, 861–873. doi: 10.1038/sj.bjp.0704941
Smith, E. N., Johnson, C., and Martin, K. J. (1981). Fear bradycardia in captive eastern chipmunk, Tamias striatus. Comp. Biochem. Physiol. 70, 529–532. doi: 10.1016/0300-9629(81)92565-2
Smith, N. E., and Tobey, E. W. (1983). Heart rate response to forced and voluntary diving in swamp rabbits, Sylvilagus aquaticus. Physiol. Zool. 56, 632–638. doi: 10.1086/physzool.56.4.30155886
Smith, N. E., and Woodruff, R. A. (1980). Fear bradycardia in free ranging woodchucks, Marmota monax. J. Mammal 61, 750–753. doi: 10.2307/1380333
Solomon, I. C., Halat, T. J., El-Maghrabi, M. R., and O’Neal, M. H. I. (2001). Localization of connexin26 and connexin32 in putative CO2- chemosensitive brainstem regions in rat. Resp. Physiol. 129, 101–121. doi: 10.1016/s0034-5687(01)00299-7
Spit, B. J., Bretschneider, F., Hendriksen, E. G. J., and Kuper, C. F. (1993). Ultrastructure of free nerve endings in respiratory and squamous epithelium on the rat nasal septum. Cell Tissue Res. 274, 329–335. doi: 10.1007/bf00318751
Stewart, M. (2018). An explanation for sudden death in epilepsy (SUDEP). J. Physiol. Sci. 68, 307–320. doi: 10.1007/s12576-018-0602-z
Stjärne, P., Lundblad, L., Änggård, A., Hökfelt, T., and Lundberg, J. M. (1989). Tachykinins and calcitonin gene-related peptide: co-existence in sensory nerves of the nasal mucosa and effects on blood flow. Cell Tissue Res. 256, 439–446.
Stornetta, R. L., Morrison, S. F., Ruggiero, D. A., and Reis, D. J. (1989). Neurons of rostral ventrolateral medulla mediate somatic pressor reflex. Am. J. Physiol. 256, R448–R462.
Stornetta, R. L., Rosin, D. L., Wang, H., Sevigny, C. P., Weston, M. C., and Guyenet, P. G. (2003). A group of glutamatergic interneurons expressing high levels of both neurokinin-1 receptors and somatostatin identifies the region of the pre-Botzinger complex. J. Comp. Neurol. 455, 499–512. doi: 10.1002/cne.10504
Sun, W., and Panneton, W. M. (2005). Defining projections from the caudal pressor area of the caudal ventrolateral medulla. J. Comp. Neurol. 482, 273–293. doi: 10.1002/cne.20434
Tan, W., Janczewski, W. A., Yang, P., Shao, X. M., Callaway, E. M., and Feldman, J. L. (2008). Silencing preBotzinger complex somatostatin-expressing neurons induces persistent apnea in awake rat. Nat. Neurosci. 11, 538–540. doi: 10.1038/nn.2104
Taylor, T., Al-Ghamdi, M. S., Ihmied, I. H., Wang, T., and Abe, A. S. (2001). The neuranatomical basis of central control of cardiorespiratory interactions in vertebrates. Exp. Physiol. 86, 771–776. doi: 10.1111/j.1469-445x.2001.tb00043.x
Tchobroutsky, C., Merlet, C., and Rey, P. (1969). The diving reflex in rabbit, sheep and newborn lamb and its afferent pathways. Resp. Physiol. 8, 108–117. doi: 10.1016/0034-5687(69)90048-6
Terui, N., Numao, Y., Kumada, M., and Reis, D. J. (1981). Identification of the primary afferent fiber group and adequate stimulus initiating the trigeminal depressor response. J. Auton. Nerv. Syst. 4, 1–16. doi: 10.1016/0165-1838(81)90002-3
Thewissen, J. G., Cooper, L. N., Clementz, M. T., Bajpai, S., and Tiwari, B. N. (2007). Whales originated from aquatic artiodactyls in the Eocene epoch of India. Nature 450, 1190–1194. doi: 10.1038/nature06343
Thomsen, L. L., Iversen, H. K., and Olesen, J. (1995). Cerebral blood flow velocities are reduced during attacks of unilateral migraine without aura. Cephalalgia 15, 109–116. doi: 10.1046/j.1468-2982.1995.015002109.x
Thürauf, N., Hummel, T., Kettenmann, B., and Kobal, G. (1993). Nociceptive and reflexive responses recorded from the human nasal mucosa. Brain Res. 629, 293–299. doi: 10.1016/0006-8993(93)91333-n
Tift, M., and Ponganis, P. (2019). Time domains of hypoxia adaptation-elephant seals stand out among divers. Front. Physiol. 10:677. doi: 10.3389/fphys.2012.00677
Tizzano, M., and Finger, T. E. (2013). Chemosensors in the nose: guardians of the airways. Physiology 28, 51–60. doi: 10.1152/physiol.00035.2012
Tizzano, M., Gulbransen, B. D., Vandenbeuch, A., Clapp, T. R., Herman, J. P., Sibhatu, H. M., et al. (2010). Nasal chemosensory cells use bitter taste signaling to detect irritants and bacterial signals. PNAS 107, 3210–3215. doi: 10.1073/pnas.0911934107
Topchiy, I., Radulovacki, M., Waxman, J., and Carley, D. W. (2009). Cardiorespiratory effects of intertrigeminal area stimulation in vagotomized rats. Brain Res. 1250, 120–129. doi: 10.1016/j.brainres.2008.10.071
Vega, J. L. (2018). Ictal mammalian dive response: a likely cause of sudden unexpected death in epilepsy. Front. Neurol. 9:677. doi: 10.3389/fneur.2018.00677
Viana, F. (2011). Chemosensory properties of the trigeminal system. ACS Chem. Neurosci. 2, 38–50. doi: 10.1021/cn100102c
Villiere, S. M., Nakase, K., Kollmar, R., Silverman, J., Sundaram, K., and Stewart, M. (2017). Seizure-associated central apnea in a rat model: evidence for resetting the respiratory rhythm and activation of the diving reflex. Neurobiol. Dis. 101, 8–15. doi: 10.1016/j.nbd.2017.01.008
Vincenzi, F. F. (2019). Sudden unexpected death and the mammalian dive response: catastrophic failure of a complex tightly coupled system. Front. Physiol. 10:97. doi: 10.3389/fphys.2019.00097
Wallois, F., Macron, J. M., Jounieaux, V., and Duron, B. (1991). Trigeminal nasal receptors related to respiration and to various stimuli in cats. Resp. Physiol. 85, 111–125. doi: 10.1016/0034-5687(91)90010-g
Wang, J., Irnaten, M., and Mendolowitz, D. (2001). Characteristics of spontaneous and evoked GABAergic synaptic currents in cardiac vagal neurons in rats. Brain Res. 889, 78–83. doi: 10.1016/s0006-8993(00)03112-7
Wang, J., Wang, X., Irnaten, M., Venkatesan, P., Evans, C., Baxi, S., et al. (2003). Endogenous acetylcholine and nicotine activation enhances GABAergic and glycinergic inputs to cardiac vagal neurons. J. Neurophysiol. 89, 2473–2481. doi: 10.1152/jn.00934.2002
Wang, Y., and Ramage, A. G. (2001). The role of central 5-HT1A receptors in the control of B-fibre cardiac and bronchoconstrictor vagal preganglionic neurones in anaesthetized cats. J. Physiol. 536, 753–767. doi: 10.1111/j.1469-7793.2001.00753.x
Whishaw, I. Q., and Schallert, T. (1977). Hippocampal RSA (theta), apnea, bradycardia, and effects of atropine during underwater swimming in the rat. Electroenceph. Clin. Neurophysiol. 42, 389–396. doi: 10.1016/0013-4694(77)90175-4
White, S., McRitchie, R. J., and Korner, P. I. (1975). Central nervous system control of cardiorespiratory nasopharyngeal reflexes in the rabbit. Am. J. Physiol. 228, 404–409. doi: 10.1152/ajplegacy.1975.228.2.404
White, S. W., McRitchie, R. J., and Franklin, D. L. (1974). Autonomic cardiovascular effects of nasal inhalation of cigarette smoke in the rabbit. Aust. J. Exp. Biol. Med. Sci. 52, 111–126. doi: 10.1038/icb.1974.9
Wildenthal, K., Atkins, J. M., Leshin, S. J., and Skelton, C. L. (1975). The diving reflex used to treat paroxysmal atrial tachycardia. Lancet 1, 12–14. doi: 10.1016/s0140-6736(75)92374-0
Williams, T. M., Fuiman, L. A., Kendall, T., Berry, P., Richter, B., Noren, S. R., et al. (2015). Exercise at depth alters bradycardia and incidence of cardiac anomalies in deep-diving marine mammals. Nat. Commun. 6:6055.
Willis, A., Mihalevich, M., Neff, R. A., and Mendelowitz, D. (1996). Three types of postsynaptic glutamatergic receptors are activated in DMNX neurons upon stimulation of NTS. Cell 271, R1614–R1619.
Xu, S., Tian, R., Lin, Y., Yu, Z., Zhang, Z., Niu, X., et al. (2019). Widespread positive selection on cetacean TLR extracellular domain. Mol. Immunol. 106, 135–142. doi: 10.1016/j.molimm.2018.12.022
Xu, X. J., Tikuisis, P., and Giesbrecht, G. (1999). A mathematical model for human brain cooling during cold-water near-drowning. J. Appl. Physiol. 86, 265–272. doi: 10.1152/jappl.1999.86.1.265
Yavari, P., McCulloch, P. F., and Panneton, W. M. (1996). Trigeminally-mediated alteration of cardiorespiratory rhythms during nasal application of carbon dioxide in the rat. J. Auton. Nerv. Syst. 61, 195–200. doi: 10.1016/s0165-1838(96)00072-0
Yu, Y. H., and Blessing, W. W. (1998). Constriction of the ear pinna vascular bed accompanies the trigeminal depressor response in rabbits. Neurosci. Lett. 255, 172–174. doi: 10.1016/s0304-3940(98)00717-4
Zapol, W. M., Liggins, G. C., Schneider, R. C., Qvist, J., Snider, M. T., Creasy, R. K., et al. (1979). Regional blood flow during simulated diving in the conscious Weddell seal. J. Appl. Physiol. 47, 968–973. doi: 10.1152/jappl.1979.47.5.968
Zhang, W.-N., Murphy, C. A., and Feldon, J. (2004). Behavioural and cardiovascular responses during latent inhibition of conditioned fear: measurement by telemetry and conditioned freezing. Behav. Brain Res. 154, 199–209. doi: 10.1016/j.bbr.2004.02.016
Keywords: marine mammals, respiratory chemoreceptors, bradycardia, medulla, SIDS, headache, stroke, arrhythmias
Citation: Panneton WM and Gan Q (2020) The Mammalian Diving Response: Inroads to Its Neural Control. Front. Neurosci. 14:524. doi: 10.3389/fnins.2020.00524
Received: 07 March 2020; Accepted: 27 April 2020;
Published: 05 June 2020.
Edited by:
Eugene Nalivaiko, The University of Newcastle, AustraliaReviewed by:
Mathias Dutschmann, The University of Melbourne, AustraliaThiago S. Moreira, University of São Paulo, Brazil
Julian Paton, University of Bristol, United Kingdom
Copyright © 2020 Panneton and Gan. This is an open-access article distributed under the terms of the Creative Commons Attribution License (CC BY). The use, distribution or reproduction in other forums is permitted, provided the original author(s) and the copyright owner(s) are credited and that the original publication in this journal is cited, in accordance with accepted academic practice. No use, distribution or reproduction is permitted which does not comply with these terms.
*Correspondence: W. Michael Panneton, cGFubmV0d21Ac2x1LmVkdQ==