- Instituto de Investigaciones Biológicas, Facultad de Ciencias Exactas y Naturales, Universidad Nacional de Mar del Plata-Consejo Nacional de Investigaciones Científicas y Técnicas, Mar del Plata, Argentina
Here, we review information on how plants face redox imbalance caused by climate change, and focus on the role of nitric oxide (NO) in this response. Life on Earth is possible thanks to greenhouse effect. Without it, temperature on Earth’s surface would be around -19∘C, instead of the current average of 14∘C. Greenhouse effect is produced by greenhouse gasses (GHG) like water vapor, carbon dioxide (CO2), methane (CH4), nitrous oxides (NxO) and ozone (O3). GHG have natural and anthropogenic origin. However, increasing GHG provokes extreme climate changes such as floods, droughts and heat, which induce reactive oxygen species (ROS) and oxidative stress in plants. The main sources of ROS in stress conditions are: augmented photorespiration, NADPH oxidase (NOX) activity, β-oxidation of fatty acids and disorders in the electron transport chains of mitochondria and chloroplasts. Plants have developed an antioxidant machinery that includes the activity of ROS detoxifying enzymes [e.g., superoxide dismutase (SOD), ascorbate peroxidase (APX), catalase (CAT), glutathione peroxidase (GPX), and peroxiredoxin (PRX)], as well as antioxidant molecules such as ascorbic acid (ASC) and glutathione (GSH) that are present in almost all subcellular compartments. CO2 and NO help to maintain the redox equilibrium. Higher CO2 concentrations increase the photosynthesis through the CO2-unsaturated Rubisco activity. But Rubisco photorespiration and NOX activities could also augment ROS production. NO regulate the ROS concentration preserving balance among ROS, GSH, GSNO, and ASC. When ROS are in huge concentration, NO induces transcription and activity of SOD, APX, and CAT. However, when ROS are necessary (e.g., for pathogen resistance), NO may inhibit APX, CAT, and NOX activity by the S-nitrosylation of cysteine residues, favoring cell death. NO also regulates GSH concentration in several ways. NO may react with GSH to form GSNO, the NO cell reservoir and main source of S-nitrosylation. GSNO could be decomposed by the GSNO reductase (GSNOR) to GSSG which, in turn, is reduced to GSH by glutathione reductase (GR). GSNOR may be also inhibited by S-nitrosylation and GR activated by NO. In conclusion, NO plays a central role in the tolerance of plants to climate change.
Introduction
Life on Earth, as it is, relies on the natural atmospheric greenhouse effect. This is the result of a process in which a planet’s atmosphere traps the sun radiation and warms the planet’s surface.
Greenhouse effect occurs in the troposphere (the lower atmosphere layer), where life and weather occur. In the absence of greenhouse effect, the average temperature on Earth’s surface is estimated around -19°C, instead of the current average of 14°C (Le Treut et al., 2007). Greenhouse effect is produced by greenhouse gasses (GHG). GHG are those gaseous constituents of the atmosphere that absorb and emit radiation in the thermal infrared range (IPCC, 2014). Traces of GHG, both natural and anthropogenic, are present in the troposphere. The most abundant GHG in increasing order of importance are: water vapor, carbon dioxide (CO2), methane (CH4), nitrous oxides (NxO) and ozone (O3) (Kiehl and Trenberth, 1997). GHG percentages vary daily, seasonally, and annually.
GHG Contribute Differentially to Greenhouse Effect
Water Vapor
Water is present in the troposphere both as vapor and clouds. Water vapor was reported by Tyndal in 1861 as the most important gaseous absorber of variations in infrared radiation (cited in Held and Souden, 2000). Further accurate calculation estimate that water vapor and clouds are responsible for 49 and 25%, respectively, of the long wave (thermal) absorption (Schmidt et al., 2010). However, atmospheric lifetime of water vapor is short (days) compared to other GHG as CO2 (years) (IPCC, 2014).
Water vapor concentrations are not directly influenced by anthropogenic activity and vary regionally. However, human activity increases global temperatures and water vapor formation indirectly, amplifying the warming in a process known as water vapor feedback (Soden et al., 2005).
Carbon Dioxide (CO2)
Carbon dioxide is responsible for 20% of the thermal absorption (Schmidt et al., 2010).
Natural sources of CO2 include organic decomposition, ocean release and respiration. Anthropogenic CO2 sources are derived from activities such as cement manufacturing, deforestation, fossil fuels combustion such as coal, oil and natural gas, etc. Surprisingly, 24% of direct CO2 emission comes from agriculture, forestry and other land use, and 21% comes from industry (IPCC, 2014).
Atmospheric CO2 concentrations climbed up dramatically in the past two centuries, rising from around 270 μmol.mol-1 in 1750 to present concentrations higher than 385 μmol.mol-1 (Mittler and Blumwald, 2010; IPCC, 2014). Around 50% of cumulative anthropogenic CO2 emissions between 1750 and 2010 have taken place since the 1970s (IPCC, 2014). It is calculated that the temperature rise produced by high CO2 concentrations, plus the water positive feedback, would increase by 3–5°C the global mean surface temperature in 2100 (IPCC, 2014).
Methane (CH4)
Methane (CH4) is the main atmospheric organic trace gas. CH4 is the primary component of natural gas, a worldwide fuel source. Significant emissions of CH4 result from cattle farming and agriculture, but mainly as a consequence of fossil fuel use. Concentrations of CH4 were multiplied by two since the pre-industrial era. The present worldwide-averaged concentration is of 1.8 μmol.mol-1 (IPCC, 2014).
Although its concentration represents only 0.5% that of CO2, concerns arise regarding a jump in CH4 atmospheric release. Indeed, it is 30 times more powerful than CO2 as GHG (IPCC, 2014). CH4 generates O3 (see below), and along with carbon monoxide (CO), contributes to control the amount of OH in the troposphere (Wuebbles and Hayhoe, 2002).
Nitrous Oxides (NxO)
Nitrous oxide (N2O) and nitric oxide (NO) are GHG. During the last century, their global emissions have rised, due mainly to human intervention (IPCC, 2014). The soil emits both N2O and NO. N2O is a strong GHG, whereas NO contributes indirectly to O3 synthesis. As GHG, N2O is potentially 300 times stronger than CO2. Once in the stratosphere, the former catalyzes the elimination of O3 (IPCC, 2014). In the atmosphere, N2O concentrations are climbing up due mainly to microbial activity in nitrogen (N)-rich soils related with agricultural and fertilization practices (Hall et al., 2008).
Anthropogenic emissions (from combustion of fossil fuels) and biogenic emissions from soils are the main sources of NO in the atmosphere (Medinets et al., 2015). In the troposphere, NO quickly oxidizes to nitrogen dioxide (NO2). NO and NO2 (termed as NOx) may react with volatile organic compounds (VOCs) and hydroxyl, resulting in organic nitrates and nitric acid, respectively. They access ecosystems through atmospheric deposition that has an impact on the N cycle as a result of acidification or N enrichment (Pilegaard, 2013).
NO Sources and Chemical Reactions in Plants
Two major pathways for NO production have been described in plants: the reductive and the oxidative pathways. The reductive pathway involves the reduction of nitrite to NO by NR under conditions such as acidic pH, anoxia, or an increase in nitrite levels (Rockel et al., 2002; Meyer et al., 2005). NR-dependent NO formation has been involved in processes such as stomatal closure, root development, germination and immune responses. In plants, nitrite may also be reduced enzymatically by other molybdenum enzymes such as, xanthine oxidase, aldehyde oxidase, and sulfite oxidase, in animals (Chamizo-Ampudia et al., 2016) or via the electron transport system in mitochondria (Gupta and Igamberdiev, 2016).
The oxidative pathway produces NO through the oxidation of organic compounds such as polyamines, hydroxylamine and arginine. In animals, NOS catalyzes arginine oxidation to citrulline and NO. Many efforts were made to find the arginine-dependent NO formation in plants, as well as of plant NOS (Frohlich and Durner, 2011). The identification of NOS in the green alga Ostreococcus tauri (Foresi et al., 2010) led to high-throughput bioinformatic analysis in plant genomes. This study shows that NOS homologs were not present in over 1,000 genomes of higher plants analyzed, but only in few photosynthetic microorganisms, such as algae and diatoms (Di Dato et al., 2015; Kumar et al., 2015; Jeandroz et al., 2016). In summary, although an arginine-dependent NO production is found in higher plants, the specific enzyme/s involved in the oxidative pathways remain elusive.
Ozone (O3)
Ozone (O3) is mainly found in the stratosphere, but a little amount is generated in the troposphere. Stratospheric ozone (namely the ozone layer) is formed naturally by chemical reactions involving solar ultraviolet (UV) radiation and O2. Solar UV radiation breaks one O2 molecule, producing two oxygen atoms (2 O). Then, each of these highly reactive atoms combines with O2 to produce an (O3) molecule. Almost 99% of the Sun’s medium-frequency UV light (from about 200 to 315 nm wavelength) is absorbed by the (O3) layer. Otherwise, they could damage exposed life forms near the Earth surface1.
The majority of tropospheric O3 appears when NOx, CO and VOCs, react in the presence of sunlight. However, it was reported that NOx may scavenge O3 in urban areas (Gregg et al., 2003). This dual interaction between NOx and O3 is influenced by light, season, temperature and VOC concentration (Jhun et al., 2015).
Besides, the oxidation of CH4 by OH in the troposphere gives way to formaldehyde (CH2O), CO, and O3, in the presence of high amounts of NOx1.
Tropospheric O3 is harmful to both plants and animals (including humans). O3 affects plants in several ways. Stomata are the cells, mostly on the underside of the plant leaves, that allow CO2 and water to diffuse into the tissue. High concentrations of O3 cause plants to close their stomata (McAdam et al., 2017), slowing down photosynthesis and plant growth. O3 may also provoke strong oxidative stress, damaging plant cells (Vainonen and Kangasjärvi, 2015).
Global Climate Change: an Integrative Balance of the Impact on Plants
Anthropogenic activity alters global climate by interfering with the flows of energy through changes in atmospheric gasses composition, more than the actual generation of heat due to energy usage (Karl and Trenberth, 2003). Short-term consequences of GHG increase in plants are mainly associated with the rise in atmospheric CO2. Plants respond directly to elevated CO2 increasing net photosynthesis, and decreasing stomatal opening (Long et al., 2004). To a lesser extent, O3 uptake by plants may reduce photosynthesis and induce oxidative stress. In the middle and long term, prognostic consensus about climate change signal a rise in CO2 concentration and temperature on the Earth’s surface, unexpected variations in rainfall, and more recurrent and intense weather conditions, e.g., heat waves, drought and flooding events (Mittler and Blumwald, 2010; IPCC, 2014). These brief episodes bring plants beyond their capacity of adaptation; decreasing crop and tree yield (Ciais et al., 2005; Zinta et al., 2014).
Here we will not discuss plants capacity of adaptation to novel environmental conditions when considering large scales and long-term periods. Ecosystems are being affected by climate change at all levels (terrestrial, freshwater, and marine), and it was already reported that species are under evolutionary adaptation to human-caused climate change (for a review see Scheffers et al., 2016). Migration and plasticity are two biological mechanisms to cope with these changes. Data indicate that each population of a species has limited tolerance to sharp climate variations, and they could migrate to find more favorable environments. Habitat fragmentation limits plant movement, being other big threat for adaptation (Stockwell et al., 2003; Leimu et al., 2010). Despite the fact that individual plants are immobile, plant populations move when seeds are dispersed, resulting in differences in the general distribution of the species (Corlett and Westcott, 2013). In this sense, anthropogenic activities also contribute to seed dispersal.
Plasticity is a characteristic related to phenology and phenotype. Phenology is the timing of phases occurrence in the life cycle, and phenotypic plasticity is the range of phenotypes that a single genotype may express depending on its environment (Nicotra et al., 2010). Plasticity is adaptive when the phenotype changes occur in a direction favored by selection in the new environment.
Climate Change and ROS
Reactive Oxygen Species (ROS) are continuously generated by plants under normal conditions. However, they are increased in response to different abiotic stresses. One of the most important effects of climate change-related stresses at the molecular level is the increase of ROS inside the cells (Farnese et al., 2016). Among ROS, the most studied are superoxide anion (), H2O2 and the hydroxyl radical (⋅OH-).
Reactive Oxygen Species cause damage to proteins, lipids and DNA, affecting cell integrity, morphology, physiology, and, consequently, the growth of plants (Frohnmeyer and Staiger, 2003). The main sources of ROS in stress conditions are: augmented photorespiration, NADPH oxidase (NOX) activity, β-oxidation of fatty acids and disorders in the electron transport chains of mitochondrias and chloroplasts (Apel and Hirt, 2004; AbdElgawad et al., 2015). Hence, higher plants have evolved in the presence of ROS and have acquired pathways to protect themselves from its toxicity. Plant antioxidant system (AS) includes the activity of ROS detoxifying enzymes [e.g., superoxide dismutase (SOD), ascorbate peroxidase (APX), catalase (CAT), glutathione peroxidase (GPX), and peroxiredoxin (PRX)], as well as antioxidant molecules such as ascorbic acid (ASC) and glutathione (GSH) that are present in almost all subcellular compartments (reviewed by Choudhury et al., 2017).
In this context, plants have also developed a tight interaction between ROS and NO as a mechanism to reduce the deleterious consequences of these ROS-induced oxidative injuries. NO orchestrates a wide range of mechanisms leading to the preservation of redox homeostasis in plants. Consequently, NO at low concentration is considered a broad-spectrum anti-stress molecule (Lamattina et al., 2003; Tossi et al., 2009; Correa-Aragunde et al., 2015). Figure 1 shows the relationship among the different GHG and their impact on plants.
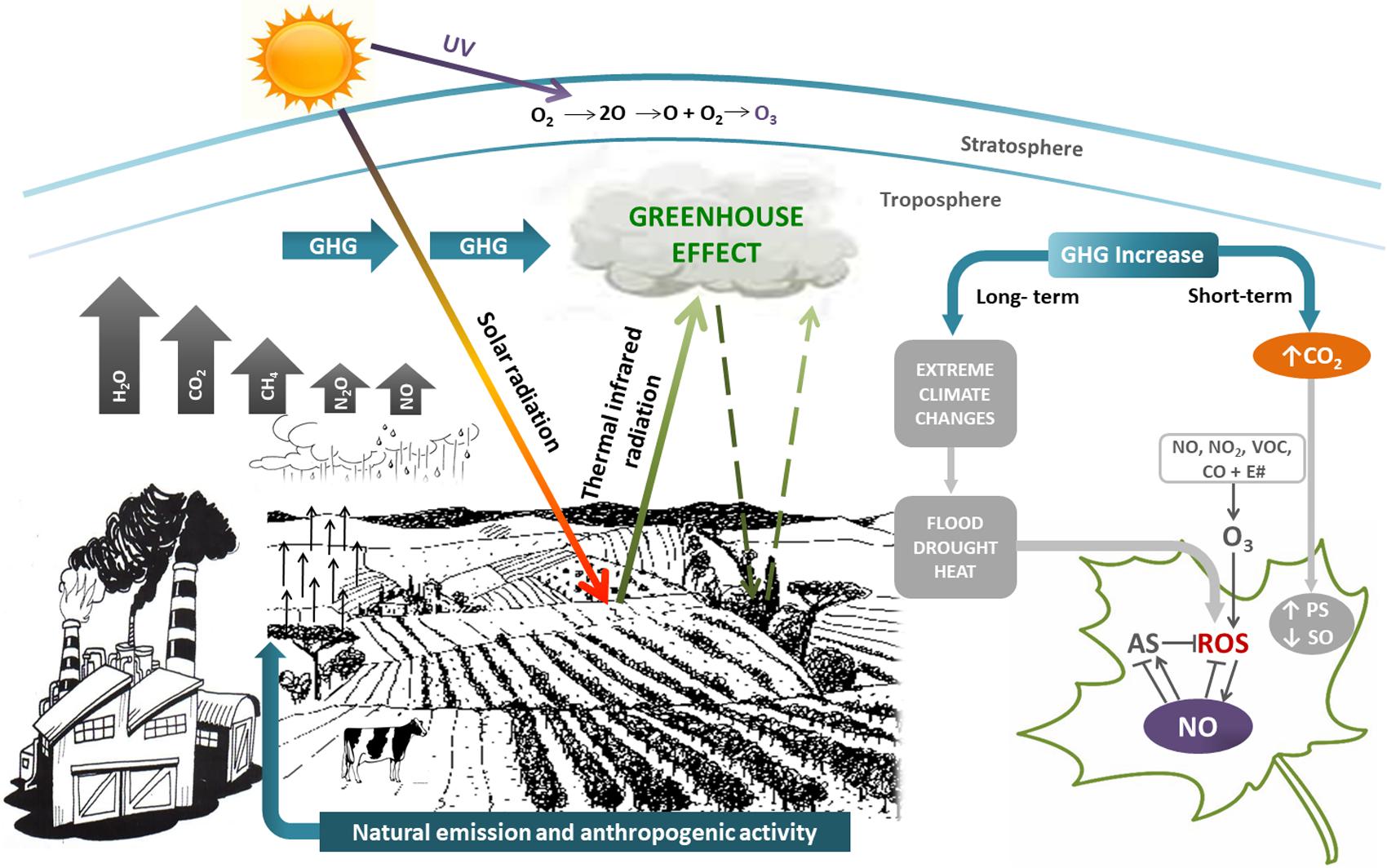
FIGURE 1. Simplified scheme showing greenhouse gasses (GHG) and their effects on plants. GHG (H2O vapor, clouds, CO2, CH4, N2O, and NO) have both natural and anthropogenic origin, contributing to greenhouse effect. Short-term effects of GHG increase is mainly CO2 rise, that activates photosynthesis (PS) and inhibits stomatal opening (SO). Long-term effects of GHG increase are extreme climate changes such as floods, droughts, heat. All of them induce the generation of reactive oxygen species (ROS) and oxidative stress in plants. Nitric oxide (NO) could alleviate oxidative stress by scavenging ROS and/or regulating the antioxidant system (AS). GHG and volatile organic compounds (VOC) react in presence of sunlight (E#) to give tropospheric O3. Although tropospheric O3 is prejudicial for life, stratospheric O3 is beneficial, because filters harmful UV-B radiation. The size of arrows are representative of the GHG concentration.
CO2 and NO Contribute to Regulate Redox Homeostasis in Plants
CO2 Increasing: Advantages and Disadvantages
Increased CO2 was suggested to have a “fertilization” effect, because crops would increase their photosynthesis and stomatal conductance in response to elevated CO2. This belief was supported by studies performed in greenhouses, laboratory controlled-environment chambers, and transparent field chambers, where emitted CO2 may be held back and readily controlled (Drake et al., 1997; Markelz et al., 2014). However, more realistic results, obtained by Free-Air Concentration Enrichment (FACE) technology, suggest that the fertilization response due to CO2 increase is probably dependent on genetic and environmental factors, and the duration of the study (Smith and Dukes, 2013). An extensive review of the literature in this field made by Xu et al. (2015) concluded that augmented CO2 normally increases photosynthesis in C3 species such as rice, soybean and wheat. On the other hand, they pointed out that a negative feedback of photosynthesis could take place in augmented CO2, as a result of overload of chemical and reactive generated substrates, leading to an imbalance in the sink:source carbon ratio. Moreover, the energetic cost of carbohydrate exportation increases in elevated CO2 level.
The most important photosynthetic enzyme is the ribulose-1,5-bisphosphate carboxylase-oxygenase (RuBisCO). Rubisco is located in mesophyll cells of C3 plants, in direct contact with the intercellular air space linked to the atmosphere by epidermal stomatal pores. Photosynthesis increases at high CO2, because Rubisco is not CO2 saturated and CO2 inhibits the oxygenation reactions and photorespiration (Long et al., 2006). However, long-term high concentration of CO2 may down regulate Rubisco activity because ribulose-1,5-bisphosphate is not regenerated. Hexokinase (HXK), a sensor of extreme photosynthate, may participate in the down regulation of Rubisco concentration (Xu et al., 2015). Moreover, severe abiotic stresses, such as temperature and drought, may restrain Rubisco carboxylation and foster oxygenation (Xu et al., 2015).
In C4 crops, such as maize and sorghum, the elevated concentration of CO2 inside the bundle sheath cells could prevent a large increase of Rubisco activity at higher atmospheric CO2 and, thereby, photosynthetic activity is not augmented. However, at high CO2 levels, the water status of C4 plants under drought conditions is improved, increasing photosynthesis and biomass accumulation (Long et al., 2006; Mittler and Blumwald, 2010). That envisages potential advantages for the C4 species in future climatic change scenarios, particularly in arid and semiarid areas.
In addition, high CO2 has the benefit of reducing stomatal conductance, decreasing 10% evapotranspiration in both C3 and C4 plants. Simultaneously, the cooling decreased resulting from reduced transpiration causes elevated canopy temperatures of around 0.7°C for most crops. Biomass and yield rise due to high CO2 in all C3 plants, but not in C4 plants exception made when water is a restraint. Yields of C3 grain crops jump around 19% on average at high CO2 (Kimball, 2016).
Some reports analyze the contribution of CO2 in the responses of plants to the combination of multiple stresses. For Arabidopsis thaliana, the combination of heat and drought induces photosynthesis inhibition of 62% under ambient CO2, but the drop in photosynthesis is just 40% at high CO2. Moreover, the protein oxidation increases significantly during a heat wave and drought, and this effect is repressed by increased CO2. Photorespiration is also reduced by high CO2 (Zinta et al., 2014).
Studying grasses (Lolium perenne, Poa pratensis) and legumes (Medicago lupulina, Lotus corniculatus) exposed to drought, high temperature and augmented CO2, AbdElgawad et al. (2015) demonstrated that drought suppresses plant growth, photosynthesis and stomatal conductance, and promotes in all species the synthesis of osmolytes and antioxidants. Instead, oxidative damage is more markedly observed in legumes than in grasses. In general, warming amplifies drought consequences. In contrast, augmented CO2 diminishes stress impact. Reduction in photosynthesis and chlorophyll, as a result of drought and elevated temperature, were avoided by high CO2 in the grasses. Noxious effects of oxidative stress, i.e., lipid peroxidation, are phased down in all species by augmented CO2. Normally, a reduced impact of oxidative stress is due to decreased photorespiration and diminished NOX activity. In legumes, a rise in levels of antioxidant molecules (flavonoids and tocopherols) contribute as well to the stress mitigation caused by augmented CO2. The authors draw the conclusion that these different responses point at an unequal future impact of climate change on the production of agricultural-scale legumes and grass crops.
Kumari et al. (2015) assessed the impact of various levels of CO2, ambient (382 ppm) and augmented (570 ppm), and O3, ambient (50 ppb) and augmented (70 ppb) on the potato physiological and biochemical responses (Solanum tuberosum). They observed that augmented CO2 cut down O3 uptake, enhanced carbon assimilation, and curbed oxidative stress. Elevated CO2 also mitigated the noxious effect of high O3 on photosynthesis.
Although some molecular mechanisms underpinning CO2 actions are unknown, the results presented highlight the importance of CO2 as a regulator that mitigates the potential climate change-induced deleterious consequences in plants. Recent reports suggest that some CO2-associated responses may be mediated by NO.
Du et al. (2016) determined that 800 μmol.mol-1 of CO2 increased the NO concentration in Arabidopsis leaves, through a mechanism related to nitrate availability. Moreover, NO increase, as a consequence of high CO2 levels, was reported as a general procedure to improve iron (Fe) nutrition in response to Fe deficiency in tomato roots (Jin et al., 2009).
The gas exchange between the atmosphere and plants is mainly regulated by stomata. But structure and physiology of stomata are also influenced by gasses (García-Mata and Lamattina, 2013). Elevated CO2 regulate stomatal density and conductance. Moreover, there is increasing evidence that this response is modified by interaction of CO2 with other environmental factors (Xu et al., 2016; Yan et al., 2017). Wang et al. (2015) reported that 800 μmol.mol-1 of CO2 increases the NO concentration in A. thaliana guard cells, inducing stomatal closure. Both NR and NO synthase (NOS)-like activities are necessary for CO2-induced NO accumulation. Comprehensive pharmacological and genetic results obtained in Arabidopsis by Chater et al. (2015), show that when CO2 concentration is around 700–1000 ppm, stomatal density and closure are reduced. They also illustrate that those elements necessary for this process are: activation of both ABA biosynthesis genes and the PYR/RCAR ABA receptor, and ROS increase. However, Shi et al. (2015) provide genetic and pharmacological evidence that high CO2 concentration induces stomatal closure by an ABA-independent mechanism in tomato. They show that 800 μmol.mol-1 of CO2 increase the expression of the protein kinase OPEN STOMATA 1 (OST1), NOX, and nitrate reductase (NR) genes. They also show that the sequential production of NOX-dependent H2O2 and NR-produced NO are mainly dependent of OST1, and are involved in the CO2-induced stomatal closure.
In ABA-dependent mechanisms, ABA is increased by CO2. The binding of ABA to its receptor (PYR/RCAR) inactivates PP2C, activating OST1. In ABA-independent mechanism, OST1 will be transcriptionally induced by CO2. Once activated, OST1 along with Ca2+, activates NOX, increasing ROS (Kim et al., 2010). The rise of guard cells ROS enhances NO, cytosolic free Ca2+, and pH (Song et al., 2014; Xie et al., 2014). ROS and NO release Ca2+ from internal reservoirs, or influx external Ca2+ through plasma membrane Ca2+in channels. Cytosolic free Ca2+ inactivate inward K+ channels (K+in) to prevent K+ uptake and activate outward K+ channels (K+out) and Cl- (anion) channels (Cl-) at the plasma membrane (Blatt, 2000; García-Mata et al., 2003). Ca2+ also activates slow anion channel homolog 3 (SLAH3), slow anion channel-associated 1 (SLAC1) and aluminum activated malate transporters (ALMT) (Roelfsema et al., 2012). The consequence of the regulation of cation/anion channels is the net efflux of K+/Cl-/malate and influx of Ca2+, making guard cells lose turgor by water outlet, causing stomatal closure.
All together, the results discussed here suggest that CO2-induced NO increase is a common plant physiological response to oxidative stresses. Figure 2 shows the importance of CO2 and NO in these processes.
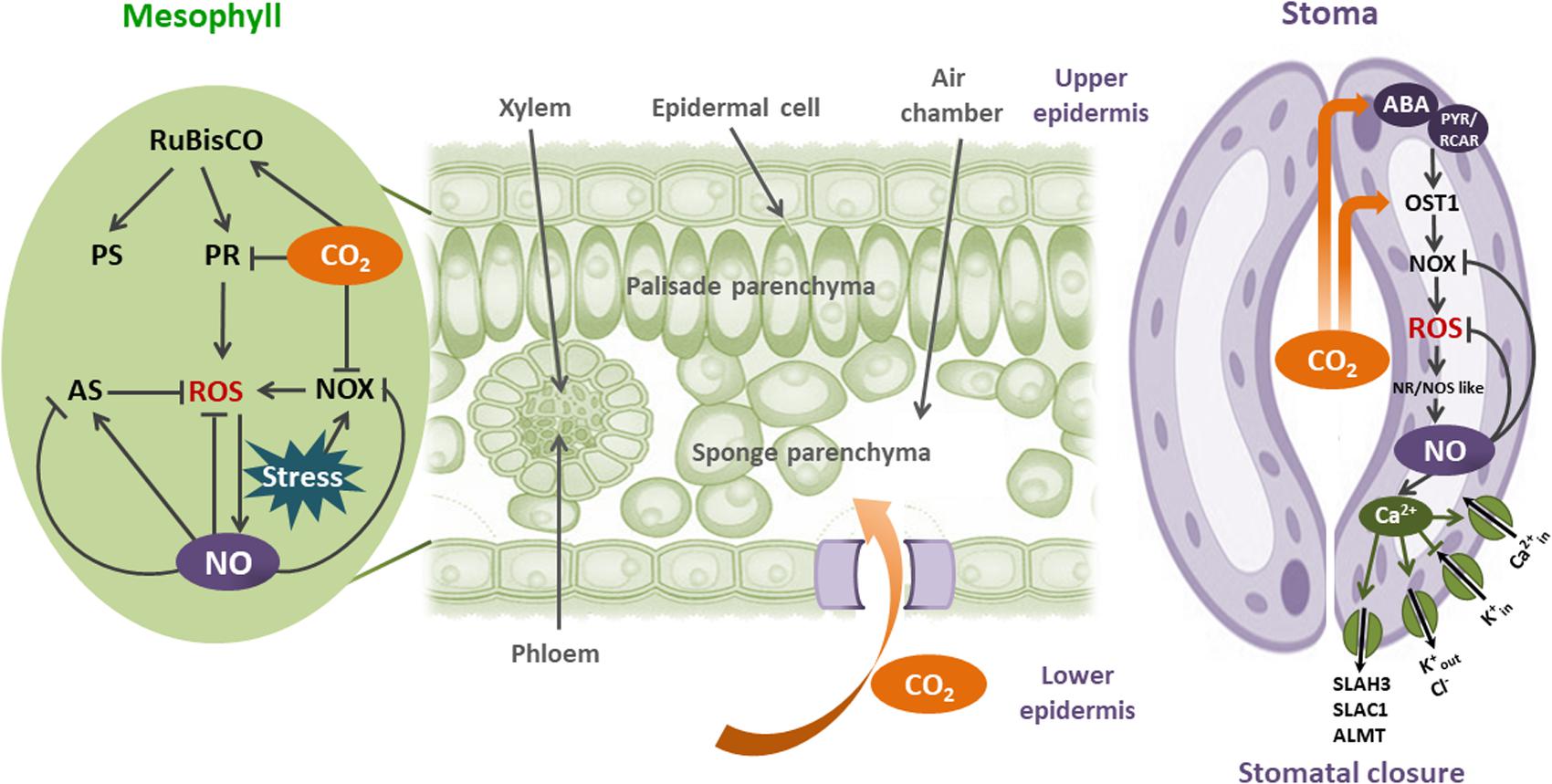
FIGURE 2. Interplay between CO2 and NO in plant redox physiology: CO2 enters to the leaves by stomata. Once in mesophyll cells, CO2 increase photosynthesis (PS) through the CO2-unsaturated Rubisco activity. When plants are in stress environments, ROS could be augmented by Rubisco-induced photorespiration and NADPH oxidase (NOX) activities. NOX- induced , in the apoplast is immediately transformed to H2O2 by the superoxide dismutase (SOD). Plasma membrane is permeable to H2O2. CO2 moderates oxidative stress in mesophyll cells by inhibiting both Rubisco photorespiration (PR) and NOX activities. Besides, NO is induced by CO2 and ROS, alleviating the consequences of oxidative stress by scavenging ROS and activating or inhibiting the antioxidant system (AS). In guard cells, CO2 increases the expression and activity of OPEN STOMATA 1 (OST1), in both ABA-dependent and independent mechanisms. OST1 activates NOX, producing ROS and consequently NO increase by nitrate reductase (NR), and NOS-like activities. NO prevents ROS increase by direct scavenging, and inhibiting NOX. NO-dependent Ca2+ regulated ion channels induces stomatal closure, modulating O3 and CO2 uptake, decreasing evapotranspiration, and rising leaf temperature.
Abiotic Stress, ROS Generation, and Redox Balance: The Key Role of NO
Reactive oxygen species are generated in apoplast, plasma membrane, chloroplasts, mitochondria, and peroxisomes (Farnese et al., 2016). It was proposed that each stress produces its own “ROS signature” (Choudhury et al., 2017). For instance, drought may reduce the activity of Rubisco, decreasing CO2 fixation and NADP+ regeneration by the Calvin cycle. As a consequence, chloroplast electron transport is altered, generating ROS by electron leakage to O2 (Carvalho, 2008). In drought stress, ROS increase is produced by NOX activity (Farnese et al., 2016). In flooding, ROS generation is an ethylene-promoted process that involves calcium (Ca2+) flux, and NOX activity (Voesenek and Bailey-Serres, 2015).
In heat stress, a NOX-dependent transient ROS rise is an early event (Königshofer et al., 2008). Then, endogenous ROS are sensed through histidine kinases, and an Arabidopsis heat stress factor (HsfA4a) appears to sense exogenous ROS. As a result, the MAPK signal pathway is activated (Qu et al., 2013). Moreover, functional decrease in photosynthetic light reaction induces ROS concentration by high electron leakage from the thylakoid membrane (Hasanuzzaman et al., 2013). In this process, O2 is the acceptor, generating .
Thus, individual stresses or their different combinations may produce particular “ROS signatures.” Besides their deleterious effects, ROS are recognized as a signal in the plant reaction to biotic and abiotic stressors. ROS may induce programed cell death (PCD) to avoid pathogen spread (Mur et al., 2008), trigger a systemic defense response signal (Dubiella et al., 2013), or avoid the chloroplast antenna overloading by electrons divert (Choudhury et al., 2017).
Whatever the origin and function, ROS concentration must be adequately regulated to avoid excessive concentration and consequent cellular damages. Depending on NO and ROS concentrations, NO has the dual capacity to activate or inhibit the ROS production, and is a key molecule for keeping cellular redox homeostasis under control (Beligni and Lamattina, 1999a; Correa-Aragunde et al., 2015). NO has a direct ROS-scavenging activity because it holds an unpaired electron, reaching elevated reactivity with O2, , and redox active metals. NO can mitigate OH formation by scavenging either Fe or (Lamattina et al., 2003). However, NO reacting with ROS (mainly ) may generate reactive nitrogen species (RNS). An excess of RNS originates a nitrosative stress (Corpas et al., 2011). To avoid the toxicity of nitrosative stress, NO is stored as GSNO in the cell.
GSH as a Redox Buffer. GSNO as NO Reservoir. SNO and S-Nitrosylation
Glutathione (GSH) is a small peptide with the sequence γ-l-glutamyl-l-cysteinyl-glycine that has a cell redox homeostatic impact in most plant tissues. It is a soluble small thiol considered a non-enzymatic antioxidant. It exists in the reduced (GSH) or oxidized state (GSSG), in which two GSH molecules are joined by a disulfide bond (Rouhier et al., 2008). GSH alleviates oxidative damages in plants generated by abiotic stresses, including salinity, drought, higher, low temperature, and heavy metals. GSH is precursor of phytochelatins, polymers that chelate toxic metals and transport them to the vacuole (Grill et al., 1989). Studies shown that GSH contributes to tolerate nickel, cadmium, zinc, mercury, aluminum and arsenate heavy metals in plants (Asgher et al., 2017). Moreover, GSH has a role in the detoxification of ROS both directly, interacting with them, or indirectly, participating of enzymatic pathways. GSH is involved in glutathionylation, a posttranslational modification that causes a mixed disulfide bond between a Cys residue and GSH.
GSH can be oxidized to GSSG by H2O2 and can react with NO to form the nitrosoglutathione (GSNO) derivative. GSNO is an intracellular NO reservoir. It is also a vehicle of NO throughout the cell and organs, spreading NO biological function. GSNO is the largest low-molecular-mass S-nitrosothiol (SNO) in plant cells (Corpas et al., 2013). GSNO metabolism and its reaction with other molecules involve S-nitrosylation and S-transnitrosation which consist of the binding of a NO molecule to a cysteine residue in proteins. Thioredoxin produces protein denitrosylation (Correa-Aragunde et al., 2013). GSNO could be decomposed by the GSNO reductase (GSNOR) to GSSG which, in turn, is reduced to GSH by glutathione reductase (GR).
Glutathione also participates in the GSH/ASC cycle, a series of enzymatic reactions that degrade H2O2. APX degrades H2O2 using ASC, the other major antioxidant in plants, as cofactor. The oxidized ASC is reduced by monodehydroascorbate reductase (MDHAR) in an NAD(P)H-dependent manner and by dehydroascorbate reductase (DHAR) employing GSH as electron donor. The resulting GSSG is reduced in turn to GSH by GR (Foyer and Noctor, 2011).
Different Effects of NO in the Regulation of Antioxidant Enzymes
The application of NO donors alleviates oxidative stress in plants challenged to abiotic and/or biotic stresses (Laxalt et al., 1997; Beligni and Lamattina, 1999b, 2002; Shi et al., 2007; Xue et al., 2007; Leitner et al., 2009).
Besides the direct ROS-scavenging activity of NO, its beneficial effect is exerted by the regulation of the antioxidant enzymes activity that controls toxic levels of ROS and RNS (Uchida et al., 2002; Shi et al., 2005; Song et al., 2006; Romero-Puertas et al., 2007; Bai et al., 2011). NO can modulate cell redox balance in plants through the regulation of gene expression, posttranslational modification or by its binding to the heme prosthetic group of some antioxidant enzymes.
SOD catalyzes the dismutation of stress-generated in one of two less harmful species: either molecular oxygen (O2) or hydrogen peroxide (H2O2). APX and CAT are the most important enzymes degrading H2O2 in plants. They transform H2O2 to H2O and O2. APX isoforms are primarily found in the cytosol and chloroplasts, while the CAT isoforms are found in peroxisomes. APX has strong affinity for H2O2 and uses ASC as an electron donor. In contrast, CAT removes H2O2 generated in the peroxisomal respiratory pathway without the need to reduce power. Even though CAT affinity for H2O2 is low, its elevated rate of reaction offers an effective way to detoxify H2O2 inside the cell. PRX may reduce both hydroperoxide and peroxynitrite.
Many reports on different plant species demonstrate that NO induces the transcription and activity of antioxidative enzymes in response to oxidative stress. The tolerance to drought and salt-induced oxidative stress in tobacco is related to the ABA-triggered production of H2O2 and NO. In turn, they induce transcripts and activities of SOD, CAT, APX, and GR (Zhang et al., 2009). UV-B-produced oxidative stress in Glycine max was alleviated by NO donors, which induced transcription and activities of SOD, CAT, and APX (Santa-Cruz et al., 2014). Furthermore, in bean leaves, SOD, CAT, and APX activities are increased by NO donors, and protected from the oxidative stress generated by UV-B irradiation (Shi et al., 2005). Drought tolerance in bermudagrass is improved by ABA-dependent SOD and CAT activities. This effect is regulated by H2O2 and NO, NO acting downstream H2O2 (Lu et al., 2009).
Several antioxidant enzymes have been identified as target of S-nitrosylation, resulting in a change of their biological activity (Romero-Puertas et al., 2008; Bai et al., 2011; Fares et al., 2011). For instance, NO reinforces recalcitrant seed desiccation tolerance in Antiaris toxicaria by activating the ascorbate-glutathione cycle through S-nitrosylation to control H2O2 accumulation. Desiccation treatment reduced the level of S-nitrosylated APX, GR, and DHAR proteins. Instead, NO gas exposure activated them by S-nitrosylation (Bai et al., 2011). Furthermore, APX was S-nitrosylated at Cys32 during saline stress and biotic stress, enhancing its enzymatic activity (Begara-Morales et al., 2014; Yang et al., 2015). In addition, auxin-induced denitrosylation of cytosolic APX provoked inhibition of its activity, followed by an increase of H2O2 concentration and the consequent lateral root formation in Arabidopsis (Correa-Aragunde et al., 2013). Moreover, an inhibitory impact of S-nitrosylation on APX activity was also reported during programmed cell death in Arabidopsis (de Pinto et al., 2013). CAT was identified to be S-nitrosylated in a proteomic study of isolated peroxisomes (Ortega-Galisteo et al., 2012). A decrease of S-nitrosylated CAT under Cd treatment was reported. In addition, in vitro experiments demonstrated a reversible inhibitory effect of APX and CAT activities by NO binding to the Fe of the heme cofactor (Brown, 1995; Clark et al., 2000). In addition, NOXs have been involved in plant defense, development, hormone biosynthesis and signaling (Marino et al., 2012). Whereas S-nitrosylation did not affect SOD activities, nitration inhibited Mn-SOD1, Fe-SOD3, and CuZn-SOD3 activity to different degrees (Holzmeister et al., 2015). SOD isoforms could also regulate endogenous NO availability by competing for the common substrate, , and it was demonstrated that bovine SOD may release NO from GSNO (Singh et al., 1999). When GSNO is decomposed by GSNOR, it produces GSSG. GSNOR is also regulated by NO. Frungillo et al. (2014) demonstrated that NO-derived from nitrate assimilation in Arabidopsis inhibited GSNOR1 by S-nitrosylation, preventing GSNO degradation. They proposed that (S)NO controls its own generation and scavenging by modulating nitrate assimilation and GSNOR1 activity. It was also shown that chilling treatment in poplar increased S-nitrosylation of NR, along with a significant decrease of its activity (Cheng et al., 2015).
The dual activity of Prx, suggests a role for this enzyme both in ROS and RNS regulation. S-nitrosylation of Arabidopsis PrxIIE inhibits its peroxynitrite activity, increasing peroxynitrite-mediated tyrosine nitration (Romero-Puertas et al., 2007). Pea mitochondrial PrxIIF was S-nitrosylated under salt stress, and its peroxidase activity was reduced by 5 mM GSNO (Camejo et al., 2013).
An interesting study demonstrated that NO controls hypersensitive response (HR) through S-nitrosylation of NOX, inhibiting ROS synthesis. This triggers a feedback loop limiting HR (Yun et al., 2011).
Other proteins related to abiotic stress response are regulated by S-nitrosylation (For a review see Fancy et al., 2017).
Figure 3 is a simplified diagram that illustrates the main oxidative and nitrosative effects that modulate the activities of key cell components, thus maintaining cell redox balance. Note the feedback and positive-negative regulatory processes occurring in the main pathways. They involve posttranslational modifications that activate and inhibit the components involved in cell antioxidant system.
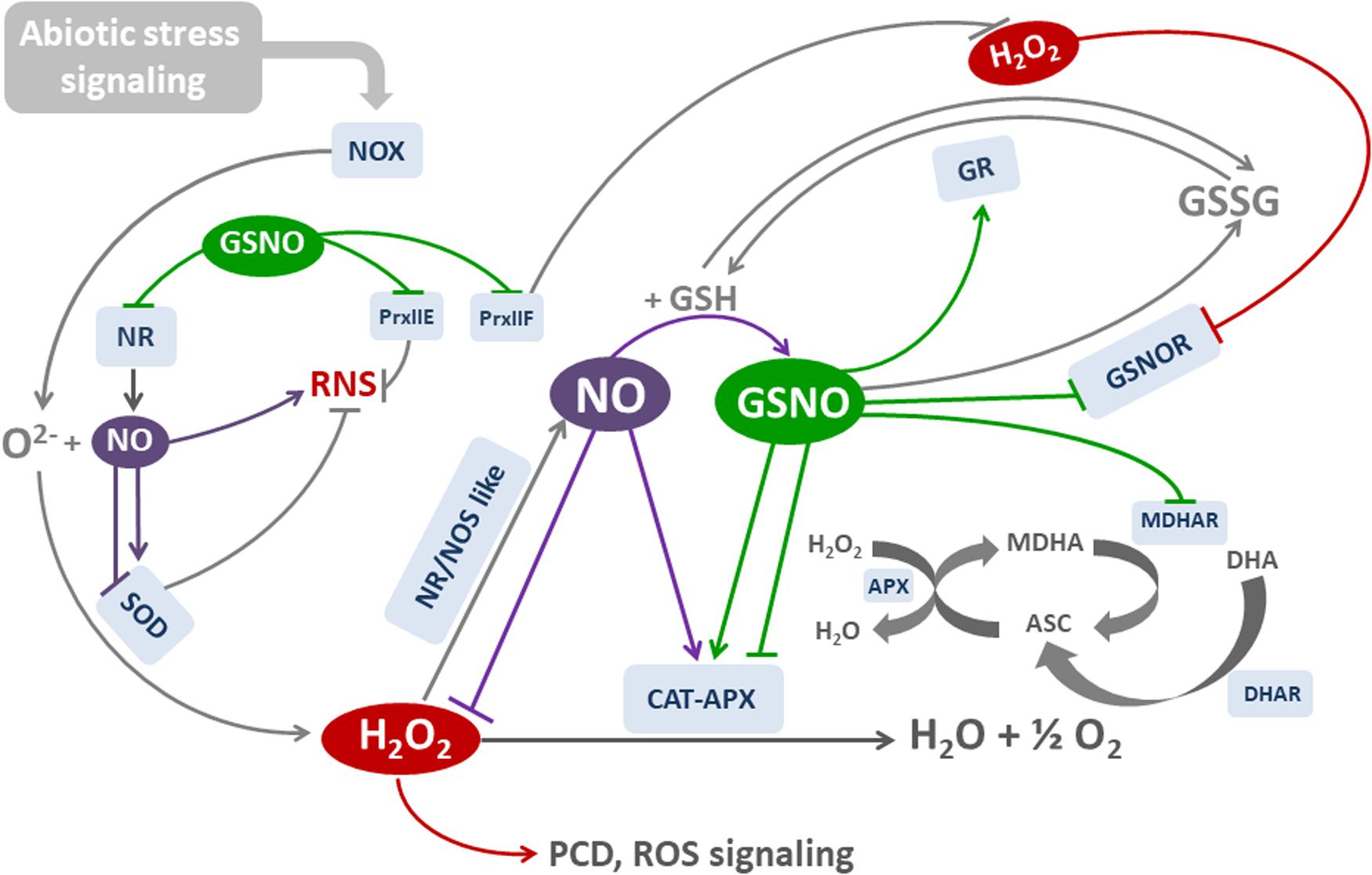
FIGURE 3. Molecules and mechanisms involved in NO-mediated redox balance. H2O2 is generated mainly by NOX and SOD as a response to (a)biotic stress. APX and CAT are the main H2O2-degrading enzymes. NO is increased by H2O2 through the induction of NR/NOS-like activities, and may scavenge ROS or induce both the transcription and activity of SOD, CAT, and APX. In parallel, NO is combined with GSH to form nitrosoglutathione GSNO. GSNO regulates many enzymatic activities by the posttranslational modification of cysteine residues through S-Nitrosylation. NOX and CAT activities are inhibited by S-nitrosylation, whereas APX is either activated or inhibited by S-nitrosylation. NO also inhibits APX by binding to heme group. GSNO is degraded by GSNOR, which could be inhibited by H2O2 and S-nitrosylation.NR could be inhibited by S-nitrosylation. GR reduces GSSG to GSH, and it is activated by S-nitrosylation. Ascorbate (ASC) is a cofactor of APX. Reduced ASC is generated by MDHAR and DHAR, using GSH as electron donor. Both enzymes are inhibited by S-nitrosylation. Reactive Nitrogen Species (RNS) may be originated by NO and reaction. SOD regulate RNS dismutating . Peroxiredoxins (Prx) reduce both ROS AND RNS. RNS are degraded by PrxIIe, and H2O2 by PrxIIF. Both enzymes are inhibited by S-nitrosylation. Red lines: H2O2-regulated reactions. Purple lines: NO-regulated reactions. Green lines: GSNO-regulated reactions.
Conclusions and Perspectives
The accelerating rate of climate change, together with habitat fragmentation caused by human activity, are part of the selective pressures building a new Earth’s landscape.
Climate change is a multidimensional and simultaneous variation in duration, frequency and intensity of parameters like temperature and precipitation, altering the seasons and life on the Earth. In this scenario, plant species with increased adaptive plasticity will be better equipped to tolerate changes in the frequency of extreme weather events. GHG are one of the forces driving climate change. However, CO2 and NO may contribute to maintaining the cell redox homeostasis, regulating the amount of ROS, GSH, GSNO, and SNO.
In this manuscript, we summarize the available evidence supporting the presence of broad spectrum anti-stress molecules, as NO in plants, for coping with unprecedented changes in environmental conditions. Future research should focus in better understanding the influence of GHG on plant physiology.
Author Contributions
RC conceived the project and wrote the manuscript. MN drew figures and collaborated in writing the manuscript. NC-A and LL supervised and complemented the drafting. All the persons entitled to authorship have been named and have approved the final version of the submitted manuscript.
Funding
This work was supported by grants from the Consejo Nacional de Investigaciones Cientificas y Tecnicas, the Agencia Nacional de Promoción Científica y Tecnológica, and the Universidad Nacional de Mar del Plata, Argentina. NC-A, LL, and RC are permanent members of the Scientific Research career of CONICET. MN is doctoral fellow of the ANPCYT.
Conflict of Interest Statement
The authors declare that the research was conducted in the absence of any commercial or financial relationships that could be construed as a potential conflict of interest.
The reviewer MCR-P and handling Editor declared their shared affiliation.
Acknowledgments
We thank ANPCYT for MN fellowship. We also thank Marta Terrazo for helping with the language revision of the manuscript.
Footnotes
References
AbdElgawad, H., Farfan-Vignolo, E. R., de Vos, D., and Asard, H. (2015). Elevated CO2 mitigates drought and temperature-induced oxidative stress differently in grasses and legumes. Plant Sci. 231, 1–10. doi: 10.1016/j.plantsci.2014.11.001
Apel, K., and Hirt, H. (2004). Reactive oxygen species: metabolism, oxidative stress, and signal transduction. Annu. Rev. Plant Biol. 55, 373–399. doi: 10.1146/annurev.arplant.55.031903.141701
Asgher, M., Per, T. S., Anjum, S., Khan, M., Masood, A., Verna, S., et al. (2017). “Contribution of glutathione in heavy metal stress tolerance in plants,” in Reactive Oxygen Species and Antioxidant Systems in Plants: Role and Regulation under Abiotic Stress, eds M. Khan and N. Khan (Singapore: Springer), doi: 10.1007/978-981-10-5254-5_12
Bai, X., Yang, L., Tian, M., Chen, J., Shi, J., Yang, Y., et al. (2011). Nitric oxide enhances desiccation tolerance of recalcitrant Antiaris toxicaria seeds via protein S-nitrosylation and carbonylation. PLoS One 6:e20714. doi: 10.1371/journal.pone.0020714
Begara-Morales, J. C., Sánchez-Calvo, B., Chaki, M., Valderrama, R., Mata-Pérez, C., López-Jaramillo, J., et al. (2014). Dual regulation of cytosolic ascorbate peroxidase (APX) by tyrosine nitration and S -nitrosylation. J. Exp. Bot. 65, 527–538. doi: 10.1093/jxb/ert396
Beligni, M. V., and Lamattina, L. (1999a). Is nitric oxide toxic or protective? Trends Plant Sci. 4, 299–300. doi: 10.1016/S1360-1385(99)01451-X
Beligni, M. V., and Lamattina, L. (1999b). Nitric oxide protects against cellular damage produced by methylviologen herbicides in potato plants. Nitric Oxide 3, 199–208. doi: 10.1006/niox.1999.0222
Beligni, M. V., and Lamattina, L. (2002). Nitric oxide interferes with plant photo-oxidative stress by detoxifying reactive oxygen species. Plant Cell Environ. 25, 737–748. doi: 10.1046/j.1365-3040.2002.00857.x
Blatt, M. R. (2000). Cellular signaling and volume control in stomatal movements in plants. Annu. Rev. Cell Dev. Biol. 16, 221–241. doi: 10.1146/annurev.cellbio.16.1.221
Brown, G. C. (1995). Reversible binding and inhibition of catalase by nitric oxide. Eur. J. Biochem. 232, 188–191. doi: 10.1111/j.1432-1033.1995.tb20798.x
Camejo, D., Romero-Puertas, M. D. C., Rodríguez-Serrano, M., Sandalio, L. M., Lázaro, J. J., Jiménez, A., et al. (2013). Salinity-induced changes in S-nitrosylation of pea mitochondrial proteins. J. Proteomics 79, 87–99. doi: 10.1016/j.jprot.2012.12.003
Carvalho, M. H. C. (2008). Drought stress and reactive oxygen species. Plant Signal. Behav. 3, 156–165. doi: 10.4161/psb.3.3.5536
Chamizo-Ampudia, A., Sanz-Luque, E., Llamas, A., Ocana-Calahorro, F., Mariscal, V., Carreras, A., et al. (2016). A dual system formed by the ARC and NR molybdoenzymes mediates nitrite-dependent NO production in Chlamydomonas. Plant Cell Environ. 39, 2097–2107. doi: 10.1111/pce.12739
Cheng, T., Chen, J., Ef, A. A., Wang, P., Wang, G., Hu, X., et al. (2015). Quantitative proteomics analysis reveals that S-nitrosoglutathione reductase (GSNOR) and nitric oxide signaling enhance poplar defense against chilling stress. Planta 242, 1361–1390. doi: 10.1007/s00425-015-2374-5
Choudhury, F. K., Rivero, R. M., Blumwald, E., and Mittler, R. (2017). Reactive oxygen species, abiotic stress and stress combination. Plant J. 90, 856–867. doi: 10.1111/tpj.13299
Ciais, P., Reichstein, M., Viovy, N., Granier, A., Ogée, J., Allard, V., et al. (2005). Europe-wide reduction in primary productivity caused by the heat and drought in 2003. Nature 437, 529–533. doi: 10.1038/nature03972
Clark, D., Durner, J., Navarre, D. A., and Klessig, D. F. (2000). Nitric oxide inhibition of tobacco catalase and ascorbate peroxidase. Mol. Plant. Microbe. Interact. 13, 1380–1384. doi: 10.1006/niox.1999.0222
Corlett, R. T., and Westcott, D. A. (2013). Will plant movements keep up with climate change? Trends Ecol. Evol. 28, 482–488. doi: 10.1016/j.tree.2013.04.003
Corpas, F. J., Alché, J. D., and Barroso, J. B. (2013). Current overview of S-nitrosoglutathione (GSNO) in higher plants. Front. Plant Sci. 4:126. doi: 10.3389/fpls.2013.00126
Corpas, F. J., Leterrier, M., Valderrama, R., Airaki, M., Chaki, M., Palma, J. M., et al. (2011). Nitric oxide imbalance provokes a nitrosative response in plants under abiotic stress. Plant Sci. 181, 604–611. doi: 10.1016/j.plantsci.2011.04.005
Correa-Aragunde, N., Foresi, N., Delledonne, M., and Lamattina, L. (2013). Auxin induces redox regulation of ascorbate peroxidase 1 activity by S-nitrosylation/denitrosylation balance resulting in changes of root growth pattern in Arabidopsis. J. Exp. Bot. 64, 3339–3349. doi: 10.1093/jxb/ert172
Correa-Aragunde, N., Foresi, N., and Lamattina, L. (2015). Nitric oxide is a ubiquitous signal for maintaining redox balance in plant cells: regulation of ascorbate peroxidase as a case study. J. Exp. Bot. 66, 2913–2921. doi: 10.1093/jxb/erv073
Chater, C., Peng, K., Movahedi, M., Dunn, J. A., Walker, H. J., Liang, Y. K., et al. (2015). Elevated CO2-induced responses in stomata require ABA and ABA signaling. Curr. Biol. 25, 2709–2716. doi: 10.1016/j.cub.2015.09.013
de Pinto, M. C., Locato, V., Sgobba, A., Romero-Puertas, M. D. C., Gadaleta, C., Delledonne, M., et al. (2013). S-Nitrosylation of ascorbate peroxidase is part of programmed cell death signaling in tobacco bright yellow-2 cells. Plant Physiol. 163, 1766–1775. doi: 10.1093/jxb/ert172
Di Dato, V., Musacchia, F., Petrosino, G., Patil, S., Montresor, M., Sanges, R., et al. (2015). Transcriptome sequencing of three Pseudo-nitzschia species reveals comparable gene sets and the presence of nitric oxide synthase genes in diatoms. Sci. Rep. 5:12329. doi: 10.1038/srep12329
Drake, B. G., Gonzalez-Meler, M. A., and Long, S. P. (1997). More efficient plants: a consequence of rising atmospheric CO2? Annu. Rev. Plant Physiol. Plant Mol. Biol. 48, 609–639. doi: 10.1146/annurev.arplant.48.1.609
Du, S., Zhang, R., Zhang, P., Liu, H., Yan, M., Chen, N., et al. (2016). Elevated CO2-induced production of nitric oxide (NO) by NO synthase differentially affects nitrate reductase activity in Arabidopsis plants under different nitrate supplies. J. Exp. Bot. 67, 893–904. doi: 10.1093/jxb/erv506
Dubiella, U., Seybold, H., Durian, G., Komander, E., Lassig, R., Witte, C. P., et al. (2013). Calcium-dependent protein kinase/NADPH oxidase activation circuit is required for rapid defense signal propagation. Proc. Natl. Acad. Sci. U.S.A. 110, 8744–8749. doi: 10.1073/pnas.1221294110
Fancy, N. N., Bahlmann, A. K., and Loake, G. J. (2017). Nitric oxide function in plant abiotic stress. Plant Cell Environ. 40, 462–472. doi: 10.1111/pce.12707
Fares, A., Rossignol, M., and Peltier, J. (2011). Proteomics investigation of endogenous S-nitrosylation in Arabidopsis. Biochem. Biophys. Res. Commun. 416, 331–336. doi: 10.1038/srep12329
Farnese, F. S., Menezes-Silva, P. E., Gusman, G. S., and Oliveira, J. A. (2016). When bad guys become good ones: the key role of reactive oxygen species and Nitric Oxide in the plant responses to abiotic stress. Front. Plant Sci. 7:471. doi: 10.3389/fpls.2016.00471
Foresi, N., Correa-Aragunde, N., Parisi, G., Calo, G., Salerno, G., and Lamattina, L. (2010). Characterization of a nitric oxide synthase from the plant kingdom: NO generation from the green alga Ostreococcus tauri is light irradiance and growth phase dependent. Plant Cell 22, 3816–3830. doi: 10.1105/tpc.109.073510
Foyer, C. H., and Noctor, G. (2011). Ascorbate and glutathione: the heart of the redox hub. Plant Physiol. 155, 2–18. doi: 10.1038/srep12329
Frohlich, A., and Durner, J. (2011). The hunt for plant nitric oxide synthase (NOS): Is one really needed? Plant Sci. 181, 401–404. doi: 10.1016/j.plantsci.2011.07.014
Frohnmeyer, H., and Staiger, D. (2003). Ultraviolet-B-radiation-mediated responses in plants. Balancing damage and protection. Plant Physiol. 133, 1420–1428. doi: 10.1104/pp.103.030049
Frungillo, L., Skelly, M. J., Loake, G. J., Spoel, S. H., and Salgado, I. (2014). S-nitrosothiols regulate nitric oxide production and storage in plants through the nitrogen assimilation pathway. Nat. Commun. 5:5401. doi: 10.1038/ncomms6401
García-Mata, C., Gay, R., Sokolovski, S., Hills, A., Lamattina, L., and Blatt, M. R. (2003). Nitric oxide regulates K+ and Cl-channels in guard cells through a subset of abscisic acid-evoked signaling pathways. Proc. Natl. Acad. Sci. U.S.A. 100, 11116–11121. doi: 10.1073/pnas.1434381100
García-Mata, C., and Lamattina, L. (2013). Gasotransmitters are emerging as new guard cell signaling molecules and regulators of leaf gas exchange. Plant Sci. 201-202, 66–73. doi: 10.3389/fpls.2016.00277
Gregg, J., Jones, C., and Dawson, T. (2003). Urbanization effects on tree growth in the vicinity of New York City. Nature 424, 183–187. doi: 10.1038/nature01728
Grill, E., Löffler, S., Winnacker, E. L., and Zenk, M. H. (1989). Phytochelatins, the heavy-metal-binding peptides of plants, are synthesized from glutathione by a specific gamma-glutamylcysteine dipeptidyl transpeptidase (phytochelatin synthase). Proc. Natl. Acad. Sci. U.S.A. 86, 6838–6842. doi: 10.1038/srep12329
Gupta, K. J., and Igamberdiev, A. U. (2016). Reactive nitrogen species in mitochondria and their implications in plant energy status and hypoxic stress tolerance. Front. Plant Sci. 7:369. doi: 10.3389/fpls.2016.00369
Hall, S., Huber, D., and Grimm, N. (2008). Soil N2O and NO emissions from an arid, urban ecosystem. J. Geophys. Res. 113:G01016. doi: 10.1029/2007JG000523
Hasanuzzaman, M., Nahar, K., Alam, M. M., Roychowdhury, R., and Fujita, M. (2013). Physiological, biochemical, and molecular mechanisms of heat stress tolerance in plants. Int. J. Mol. Sci. 14, 9643–9684. doi: 10.3390/ijms14059643
Held, I., and Souden, B. (2000). Water vapor feedback and global warming. Annu. Rev. Energy Environ. 25, 441–475. doi: 10.1146/annurev.energy.25.1.441
Holzmeister, C., Gaupels, F., Geerlof, A., Sarioglu, H., Sattler, M., Durner, J., et al. (2015). Differential inhibition of Arabidopsis superoxide dismutases by peroxynitrite-mediated tyrosine nitration. J. Exp. Bot. 66, 989–999. doi: 10.1093/jxb/eru458
IPCC (2014). Climate Change 2014: Mitigation of Climate Change. Contribution of Working Group III to the Fifth Assessment Report of the Intergovernmental Panel on Climate Change, eds O. R. Edenhofer, Y. Pichs-Madruga, E. Sokona, S. Farahani, K. Kadner, A. Seyboth, et al. Cambridge: Cambridge University Press.
Jeandroz, S., Wipf, D., Stuehr, D. J., Lamattina, L., Melkonian, M., Tian, Z., et al. (2016). Occurrence, structure, and evolution of nitric oxide synthase-like proteins in the plant kingdom. Sci. Signal. 9:re2. doi: 10.1126/scisignal.aad4403
Jhun, I., Coull, B. A., Zanobetti, A., and Koutrakis, P. (2015). The impact of nitrogen oxides concentration decreases on ozone trends in the USA. Air Qual. Atmos. Health 8, 283–292. doi: 10.1088/1748-9326/10/8/084009
Jin, C. W., Du, S. T., Chen, W. W., Li, G. X., Zhang, Y. S., and Zheng, S. J. (2009). Elevated carbon dioxide improves plants iron nutrition through enhancing the iron-deficiency-induced responses under iron-limited conditions in tomato. Plant Physiol. 150, 272–280. doi: 10.1104/pp.109.136721
Karl, T. R., and Trenberth, K. E. (2003). Modern global climate change. Science 302, 1719–1723. doi: 10.1126/science.1090228
Kiehl, J., and Trenberth, K. (1997). Earth’s annual global mean energy budget. Bull. Amer. Meteor. Soc. 78, 197–208.
Kim, T., Böhmer, M., Hu, H., Nishimura, N., and Schroeder, J. I. (2010). Guard cell signal transduction network: advances in understanding abscisic acid, CO2, and Ca2+ signaling. Annu. Rev. Plant Biol. 61, 561–591. doi: 10.1146/annurev-arplant-042809-112226
Kimball, B. (2016). Crop responses to elevated CO2 and interactions with H2O, N, and temperature. Curr. Opin. Plant Biol. 31, 36–43. doi: 10.1016/j.pbi.2016.03.006
Königshofer, H., Tromballa, H. W., and Löppert, H. G. (2008). Early events in signaling high-temperature stress in tobacco BY2 cells involve alterations in membrane fluidity and enhanced hydrogen peroxide production. Plant Cell Environ. 31, 1771–1780. doi: 10.1111/j.1365-3040.2008.01880.x
Kumar, A., Castellano, I., Patti, F. P., Palumbo, A., and Buia, M. C. (2015). Nitric oxide in marine photosynthetic organisms. Nitric Oxide 47, 34–39. doi: 10.1038/srep12329
Kumari, S., Agrawal, M., and Singh, A. (2015). Effects of ambient and elevated CO2 and ozone on physiological characteristics, antioxidative defense system and metabolites of potato in relation to ozone flux. Environ. Exp. Bot. 109, 276–287. doi: 10.1016/j.envexpbot.2014.06.015
Lamattina, L., García-Mata, C., Graziano, M., and Pagnussat, G. (2003). Nitric oxide: the versatility of an extensive signal molecule. Annu. Rev. Plant Biol. 54, 109–136. doi: 10.1146/annurev.arplant.54.031902.134752
Laxalt, A. M., Beligni, M. V., and Lamattina, L. (1997). Nitric oxide preserves the level of chlorophyll in potato leaves infected by Phytophthora infestans. Eur. J. Plant Pathol. 103, 643–651. doi: 10.1023/A:1008604410875
Le Treut, H., Somerville, R., Cubasch, U., Ding, Y., Mauritzen, C., Mokssit, A., et al. (2007). “Historical overview of climate change science,” in Climate change 2007: The physical science basis. Contribution of Working Group I to the Fourth Assessment Report of the Intergovernmental Panel on Climate Change, eds S. Solomon, D. Qin, M. Manning, Z. Chen, M. Marquis, K. B. Averyt, et al. (Cambridge: Cambridge University Press).
Leimu, R., Vergeer, P., Angeloni, F., and Ouborg, N. (2010). Habitat fragmentation, climate change, and inbreeding in plants. Ann. N. Y. Acad. Sci. 1195, 84–98. doi: 10.1111/j.1749-6632.2010.05450.x
Leitner, M., Vandelle, E., Gaupels, F., Bellin, D., and Delledonne, M. (2009). NO signals in the haze: nitric oxide signalling in plant defence. Curr. Opin. Plant Biol. 12, 451–458. doi: 10.1016/j.pbi.2009.05.012
Long, S., Ainsworth, E., Leakey, A., Nösberger, J., and Ort, D. (2006). Food for thought: lower-than-expected crop yield stimulation with rising CO2 concentrations. Science 312, 1918–1921. doi: 10.1126/science.1114722
Long, S., Ainsworth, E., Rogers, A., and Ort, D. (2004). Rising atmospheric carbon dioxide: plants FACE the future. Annu. Rev. Plant Biol. 55, 591–628. doi: 10.1146/annurev.arplant.55.031903.141610
Lu, S., Su, W., Li, H., and Guo, Z. (2009). Abscisic acid improves drought tolerance of triploid bermudagrass and involves H2O2- and NO-induced antioxidant enzyme activities. Plant Physiol. Biochem. 7, 132–138. doi: 10.1016/j.plaphy.2008.10.006
Marino, D., Dunand, C., Puppo, A., and Pauly, N. (2012). A burst of plant NADPH oxidases. Trends Plant Sci. 17, 9–15. doi: 10.1016/j.tplants.2011.10.001
Markelz, R. J., Lai, L. X., Vosseler, L. N., and Leakey, A. D. (2014). Transcriptional reprogramming and stimulation of leaf respiration by elevated CO2 concentration is diminished, but not eliminated, under limiting nitrogen supply. Plant Cell Environ. 37, 886–988. doi: 10.1111/pce.12205
McAdam, E. L., Brodribb, T. J., and McAdam, S. A. (2017). Does ozone increase ABA levels by non-enzymatic synthesis causing stomata to close? Plant Cell Environ. 40, 741–747. doi: 10.1111/pce.12893
Medinets, S., Skiba, U., Rennenberg, H., and Butterbach-Bahl, K. (2015). A review of soil NO transformation: associated processes and possible physiological significance on organisms. Soil Biol. Biochem. 80, 92–117. doi: 10.1016/j.soilbio.2014.09.025
Meyer, C., Lea, U. S., Provan, F., Kaiser, W. M., and Lillo, C. (2005). Is nitrate reductase a major player in the plant NO (nitric oxide) game? Photosynth. Res. 83, 181–189. doi: 10.1016/j.tplants.2011.10.001
Mittler, R., and Blumwald, E. (2010). Genetic engineering for modern agriculture: challenges and perspectives. Annu. Rev. Plant Biol. 61, 443–462. doi: 10.1146/annurev-arplant-042809-112116
Mur, L. A., Kenton, P., Lloyd, A. J., Ougham, H., and Prats, E. (2008). The hypersensitive response; the centenary is upon us but how much do we know? J. Exp. Bot. 59, 501–520. doi: 10.1093/jxb/erm239
Nicotra, A., Atkin, O., Bonser, S., Davidson, A., Finnegan, E., Mathesius, U., et al. (2010). Plant phenotypic plasticity in a changing climate. Trends Plant Sci. 15, 684–692. doi: 10.1016/j.tplants.2010.09.008
Ortega-Galisteo, A. P., Rodríguez-Serrano, M., Pazmiño, D. M., Gupta, D. K., Sandalio, L. M., and Romero-Puertas, M. C. (2012). S-Nitrosylated proteins in pea (Pisum sativum L.) leaf peroxisomes: changes under abiotic stress. J. Exp. Bot. 63, 2089–2103. doi: 10.1016/j.tplants.2011.10.001
Pilegaard, K. (2013). Processes regulating nitric oxide emissions from soils. Philos. Trans. R. Soc. Lond. B Biol. Sci. 368:20130126. doi: 10.1098/rstb.2013.0126
Qu, A. L., Ding, Y. F., Jiang, Q., and Zhu, C. (2013). Molecular mechanisms of the plant heat stress response. Biochem. Biophys. Res. Commun. 432, 203–207. doi: 10.1016/j.bbrc.2013.01.104
Rockel, P., Strube, F., Rockel, A., Wildt, J., and Kaiser, W. M. (2002). Regulation of nitric oxide (NO) production by plant nitrate reductase in vivo and in vitro. J. Exp. Bot. 53, 103–110. doi: 10.1093/jexbot/53.366.103
Roelfsema, M. R., Hedrich, R., and Geiger, D. (2012). Anion channels: master switches of stress responses. Trends Plant Sci. 17, 221–229. doi: 10.1016/j.tplants.2012.01.009
Romero-Puertas, M. C., Campostrini, N., Mattè, A., Righetti, P. G., Perazzolli, M., Zolla, L., et al. (2008). Proteomic analysis of S-nitrosylated proteins in Arabidopsis thaliana undergoing hypersensitive response. Proteomics 8, 1459–1469. doi: 10.1002/pmic.200700536
Romero-Puertas, M. C., Laxa, M., Mattè, A., Zaninotto, F., Finkemeier, I., Jones, A. M. E., et al. (2007). S-nitrosylation of peroxiredoxin II E promotes peroxynitrite-mediated tyrosine nitration. Plant Cell 19, 4120–4130. doi: 10.1105/tpc.107.055061
Rouhier, N., Lemaire, S. D., and Jacquot, J.-P. (2008). The role of glutathione in photosynthetic organisms: emerging functions for glutaredoxins and glutathionylation. Annu. Rev. Plant Biol. 59, 143–166. doi: 10.1146/annurev.arplant.59.032607.092811
Santa-Cruz, D., Pacienza, N., Zilli, C., Tomaro, M., Balestrasse, K., and Yannarelli, G. (2014). Nitric oxide induces specific isoforms of antioxidant enzymes in soybean leaves subjected to enhanced ultraviolet-B radiation. J. Photochem. Photobiol. B 141, 202–209. doi: 10.1016/j.jphotobiol.2014.09.019
Scheffers, B. R., De Meester, L., Bridge, T. C., Hoffmann, A. A., Pandolfi, J. M., Corlett, R. T., et al. (2016). The broad footprint of climate change from genes to biomes to people. Science 354:aaf7671. doi: 10.1126/science.aaf7671
Schmidt, G. A., Ruedy, R. A., Miller, R. L., and Lacis, A. A. (2010). Attribution of the present-day total greenhouse effect. J. Geophys. Res. 115:D20106. doi: 10.1029/2010JD014287
Shi, K., Li, X., Zhang, H., Zhang, G., Liu, Y., Zhou, Y., et al. (2015). Guard cell hydrogen peroxide and nitric oxide mediate elevated CO2-induced stomatali movement in tomato. New Phytol. 208, 342–353. doi: 10.1111/nph.13621
Shi, Q., Ding, F., Wang, X., and Wei, M. (2007). Exogenous nitric oxide protect cucumber roots against oxidative stress induced by salt stress. Plant Physiol. Biochem. 45, 542–550. doi: 10.1016/j.plaphy.2007.05.005
Shi, S., Wang, G., Wang, Y., Zhang, L., and Zhang, L. (2005). Protective effect of nitric oxide against oxidative stress under ultraviolet-B radiation. Nitric Oxide 13, 1–9. doi: 10.1016/j.niox.2005.04.006
Singh, R., Hogg, N., Goss, S., Antholine, W., and Kalyanaraman, B. (1999). Mechanism of superoxide dismutase/H2O2-mediated nitric oxide release from S-nitrosoglutathione–role of glutamate. Arch. Biochem. Biophys. 372, 8–15. doi: 10.1006/abbi.1999.1447
Smith, N. G., and Dukes, J. S. (2013). Plant respiration and photosynthesis in global-scale models: incorporating acclimation to temperature and CO2. Glob. Change Biol. 19, 45–63. doi: 10.1111/j.1365-2486.2012.02797.x
Soden, B. J., Jackson, D. L., Ramaswamy, V., Schwarzkopf, M. D., and Huang, X. (2005). The radiative signature of upper tropospheric moistening. Science 310, 841–844. doi: 10.1126/science.1115602
Song, L., Ding, W., Zhao, M., Sun, B., and Zhang, L. (2006). Nitric oxide protects against oxidative stress under heat stress in the calluses from two ecotypes of reed. Plant Sci. 171, 449–458. doi: 10.1016/j.plantsci.2006.05.002
Song, Y., Miao, Y., and Song, C. P. (2014). Behind the scenes: the roles of reactive oxygen species in guard cells. New Phytol. 201, 1121–1140. doi: 10.1111/nph.12565
Stockwell, C., Hendry, A., and Kinnison, M. (2003). Contemporary evolution meets conservation. Trends Ecol. Evol. 18, 94–101. doi: 10.1016/S0169-5347(02)00044-7
Tossi, V., Lamattina, L., and Cassia, R. (2009). An increase in the concentration of abscisic acid is critical for nitric oxide-mediated plant adaptive responses to UV-B irradiation. New Phytol. 181, 871–879. doi: 10.1111/j.1469-8137.2008.02722.x
Uchida, A., Jagendorf, A. T., Hibino, T., Takabe, T., and Takabe, T. (2002). Effects of hydrogen peroxide and nitric oxide on both salt and heat stress tolerance in rice. Plant Sci. 163, 515–523. doi: 10.1016/S0168-9452(02)00159-0
Vainonen, J. P., and Kangasjärvi, J. (2015). Plant signalling in acute ozone exposure. Plant Cell Environ. 38, 240–252. doi: 10.1111/pce.12273
Voesenek, L. A., and Bailey-Serres, J. (2015). Flood adaptive traits and processes: an overview. New Phytol. 206, 57–73. doi: 10.1111/nph.13209
Wang, H., Xiao, W., Niu, Y., Chai, R., Jin, C., and Zhang, Y. (2015). Elevated carbon dioxide induces stomatal closure of Arabidopsis thaliana (L.) heynh. through an increased production of nitric oxide. J. Plant Growth Regul. 34, 372–380. doi: 10.1007/s00344-014-9473-6
Wuebbles, D. J., and Hayhoe, K. (2002). Atmospheric methane and global change. Earth Sci. Rev. 57, 177–210. doi: 10.1016/S0012-8252(01)00062-9
Xie, Y., Mao, Y., Zhang, W., Lai, D., Wang, Q., and Shen, W. (2014). Reactive oxygen species-dependent nitric oxide production contributes to hydrogen-promoted stomatal closure in Arabidopsis. Plant Physiol. 165, 759–773. doi: 10.1104/pp.114.237925
Xu, Z., Jiang, Y., Jia, B., and Zhou, G. (2016). Elevated-CO2 response of stomata and its dependence on environmental factors. Front. Plant Sci. 7:657. doi: 10.3389/fpls.2016.00657
Xu, Z., Jiang, Y., and Zhou, G. (2015). Response and adaptation of photosynthesis, respiration, and antioxidant systems to elevated CO2 with environmental stress in plants. Front. Plant Sci. 6:701. doi: 10.3389/fpls.2015.00701
Xue, L., Li, S., Sheng, H., Feng, H., Xu, S., and An, L. (2007). Nitric oxide alleviates oxidative damage induced by enhanced ultraviolet-B radiation in cyanobacterium. Curr. Microbiol. 55, 294–301. doi: 10.1007/s00284-006-0621-5
Yan, W., Zhong, Y., and Shangguan, Z. (2017). Contrasting responses of leaf stomatal characteristics to climate change: a considerable challenge to predict carbon and water cycles. Glob. Chang. Biol. 23, 3781–3793. doi: 10.1111/gcb.13654
Yang, H., Mu, J., Chen, L., Feng, J., Hu, J., Li, L., et al. (2015). S -Nitrosylation positively regulates ascorbate peroxidase activity during plant stress responses. Plant Physiol. 167, 1604–1615. doi: 10.1104/pp.114.255216
Yun, B.-W., Feechan, A., Yin, M., Saidi, N. B., Le Bihan, T., Yu, M., et al. (2011). S-nitrosylation of NADPH oxidase regulates cell death in plant immunity. Nature 478, 264–268. doi: 10.1038/nature10427
Zhang, Y., Tan, J., Guo, Z., Lu, S., He, S., Shu, W., et al. (2009). Increased abscisic acid levels in transgenic tobacco over-expressing 9 cis-epoxycarotenoid dioxygenase influence H2O2 and NO production and antioxidant defenses. Plant Cell Environ. 32, 509–519. doi: 10.1111/j.1365-3040.2009.01945.x
Zinta, G., AbdElgawad, H., Domagalska, M. A., Vergauwen, L., Knapen, D., Nijs, I., et al. (2014). Physiological, biochemical, and genome-wide transcriptional analysis reveals that elevated CO2 mitigates the impact of combined heat wave and drought stress in Arabidopsis thaliana at multiple organizational levels. Glob. Chang. Biol. 20, 3670–3685. doi: 10.1111/gcb.12626
Keywords: climate change, greenhouse effect, oxidative stress, nitric oxide, plants
Citation: Cassia R, Nocioni M, Correa-Aragunde N and Lamattina L (2018) Climate Change and the Impact of Greenhouse Gasses: CO2 and NO, Friends and Foes of Plant Oxidative Stress. Front. Plant Sci. 9:273. doi: 10.3389/fpls.2018.00273
Received: 18 November 2017; Accepted: 16 February 2018;
Published: 01 March 2018.
Edited by:
Luisa M. Sandalio, Consejo Superior de Investigaciones Científicas (CSIC), SpainReviewed by:
María C. Romero-Puertas, Consejo Superior de Investigaciones Científicas (CSIC), SpainDiana Bellin, University of Verona, Italy
Copyright © 2018 Cassia, Nocioni, Correa-Aragunde and Lamattina. This is an open-access article distributed under the terms of the Creative Commons Attribution License (CC BY). The use, distribution or reproduction in other forums is permitted, provided the original author(s) and the copyright owner are credited and that the original publication in this journal is cited, in accordance with accepted academic practice. No use, distribution or reproduction is permitted which does not comply with these terms.
*Correspondence: Raúl Cassia, cm9jYXNzaWFAbWRwLmVkdS5hcg==